- 1NSW Department of Primary Industries, Orange, NSW, Australia
- 2Gulbali Institute (Agriculture, Water and Environment), Charles Sturt University, Wagga Wagga, NSW, Australia
- 3South Australian Research and Development Institute (SARDI), Adelaide, SA, Australia
- 4College of Science and Engineering, Flinders University, Bedford Park, SA, Australia
- 5School of Agriculture, Food and Wine, University of Adelaide, Urrbrae, SA, Australia
- 6Faculty of Engineering, University of New South Wales, Kensington, NSW, Australia
Extreme bushfire is having considerable negative effects on the sustainability of agricultural landscapes in various parts of the world. Fire-induced damages to tree crops have led to significant effects on perennial horticultural production systems with associated lower returns and decline in economic sustainability. Australia is one of the most fire-prone countries in the world and contributes to global horticultural production with production forecast level estimated at $18.2 billion in 2023–24, according to the Australian Department of Agriculture, Fisheries and Forestry. Bushfire-related damages to horticultural production may however threaten this promising potential. This review provides a commentary on the history, scale and impacts of extreme bushfires in Australia. The effects of bushfire on horticulture, including soil nutrient availability, fruit tree physiology and carbohydrate sink-source dynamics are discussed. Given the increasing frequency and severity of bushfires as a result of climate change, the negative effects of heat and fire damage on fruit tree production are expected to increase. Based on the Australian experience with bushfires in horticultural landscapes, this review outlines proactive responses for minimising bushfire impacts on horticultural production in temperate regions, with particular reference to the Rosaceae family. Adaptation strategies must be planned and set up before orchard establishment and should include defensible space or safety zones around the orchard, as well as internal and external fuel reduction strategies for the orchard lifespan.
1. Introduction
Current extreme bushfire incidences around the world including Australia and Southeast Asia, parts of the Mediterranean, boreal North America, the western side of temperate North America particularly California, and the Amazon rainforest, have resulted in higher scale of destruction affecting lives, properties, and physical infrastructures (Huijnen et al., 2016; Brewer, 2018; Turco et al., 2018; Kirchmeier-Young et al., 2019; Nolan et al., 2021; Singh, 2021; van Oldenborg et al., 2021; Ma et al., 2022). Unplanned and uncontrollable fires could also result in direct and indirect environmental and economic consequences through the destruction of biotic and abiotic components of ecosystems. Quantifying the global losses due to extreme bushfires in monetary terms will run into billions of dollars because of the enormity of damage associated with it (Campanharo et al., 2019).
In Australia and other countries particularly in North America, fire is an integral part of the landscape and bushfires have been used in traditional indigenous land management for thousands of years (McKemey et al., 2020; Morgan et al., 2020). Due to the dry climate, Australia is one of the most fire-prone countries in the world. Extreme uncontrolled bushfires have been recorded in Australia since white settlement in the late 1800s/early 1900s and will likely continue (Morgan et al., 2020). While bushfires are widespread in Australia, they are more common in the subtropical/temperate woodlands of densely populated south-eastern Australia, particularly in the states and territories of New South Wales (NSW), Victoria, South Australia (SA) Tasmania and Australian Capital Territory (ACT) (Lucas et al., 2007; Morgan et al., 2020). These regions are fire-prone due to the mostly cool, wet winters and hot and dry summers, resulting in fuel accumulation and associated increased fire risk (Lucas et al., 2007). Fire risk and severity is further increased by drought conditions, coupled with strong and frequent winds that regularly occur in summer. Other factors contributing to the severity of bushfires in south-eastern Australia are topography, weather patterns, sclerophyllous vegetation, broad natural and human ignition sources and high human population at the urban-forest interface (Morgan et al., 2020).
While bushfires are a natural part of the Australian environment, there has been an increase in the frequency and intensity of bushfires and their geographical scale in recent decades (Table 1). The total land area burnt in eastern Australia during the 2019–2020 fire season was estimated to be around 12.6 million hectares [almost the area of England (13 million ha)] (Wintle et al., 2020) and was over half of the 20 million hectares that were burnt across Australia (Deb et al., 2020). It is generally believed that these persistent and catastrophic fires are principally due to anthropogenic climate change and that the increasing frequency and severity of bushfires will continue (Chhetri et al., 2012; Adams, 2013). Prolonged drought conditions, continuously sustained high temperatures and decreased annual rainfall are argued to be the predisposing factors for bushfires (Nolan et al., 2020).
Climate change is directly affecting Australia’s climate. In 2019, before the catastrophic bushfires, the national annual rainfall was 40% lower than the long-term annual average, and maximum temperatures were 2.1°C above long-run average maxima (Wintle et al., 2020). The resultant effects of higher temperatures and lower rainfall are very dry soil organic and plant materials that are ready fuels for naturally or anthropogenically ignited fires (Adams, 2013). Highly flammable trees, such as eucalyptus and pines, dominate the landscape fringes of Australia and serve as ready fuels contributing to the intensity and destructive ability of bushfires. Some authors attribute the increased frequency and severity of bushfires to poor forest management that allows fuel to build up as a result of policies of fire suppression and insufficient forest fuel reduction (Adams, 2013).
Bushfires not only destroy the environment but also tragically devastate the lives and livelihoods of local communities. Between 1901 and 2011, a total of 825 bushfire-caused fatalities were recorded in Australia (Blanchi et al., 2014). The 2019–2020 bushfires claimed 28 human lives and 1.25 billion animals (Deb et al., 2020). In addition, bushfires caused tremendous economic damage, destroying countless houses and other physical structures, leading to a widespread need for aid and support (Gibbons et al., 2018). The total direct and indirect national economic losses caused by the 2019–2020 Australian bushfires are estimated at AUD110 billion (Deb et al., 2020). Recovering from a bushfire in Australia is becoming increasingly difficult due to the lengthened fire season and increasing frequency of bushfires. Consequently, bushfires are affecting community and people’s wellbeing and livelihoods more severely than previously.
Bushfires can have significant negative effects on perennial horticulture production systems. Heat injuries from bushfires usually exert negative effects on tree physiology, particularly through cambium/phloem and xylem damage (Bär et al., 2019). As a result, carbohydrates, nutrients and water transport within the trees become compromised. The impaired functionality and associated factors, such as pest and pathogen invasion, soil nutrient loss and increased soil erosion, could ultimately result in tree mortality (Wallbrink et al., 2004; Verma and Jayakumar, 2012; Bär et al., 2019; Bowman et al., 2020) and reduced orchard productivity. According to the Department of Regional NSW Australia, total losses to horticultural production from the 2019–2020 bushfires were up to AUD94 million (USD69 million) if critical assets and infrastructure damage are considered. This cost could be significantly higher if potential productivity losses from indirect fire effects, such as smoke taint in fruits and extra costs for restoring affected orchards to uniform and optimum productivity are included (Idowu et al., 2021).
On a more general note, the 2019–2020 bushfires destroyed more than 2.5 million hectares of Australian agricultural land being one-quarter of the total land area of 10 million hectares destroyed by bushfires. In terms of economic losses, the bushfires caused about AUD4-5 billion losses to the Australian food system, an equivalent of 6–8% of the value of national agricultural production in the same period (WWF, 2022). These losses covered the damage to farm properties, infrastructure and land, food production losses, and associated health impacts on farmers (WWF, 2022). Consideration of the inevitable bushfire damage to natural capital stocks and flows associated with food production system will raise the economic impact to even higher values. The adverse impacts on natural capital stocks and flows may affect food production for many years to come implying potential ongoing economic impacts (WWF, 2022). Beyond Australia, bushfires in other warm and dry regions of the world such as California, Spain and Portugal, are having comparable consequential negative effects on agricultural production. Bushfires have been reported to destroy agricultural lands with significant effects on rural economies and are postulated to cause greater destructions in the future (FAO, 2021).
2. Drivers and impact of catastrophic bushfires within the Australian context
There is a noticeable decline in intervals between major bushfires in Australia, with less than 5 years between them, in the last two decades (Table 1) (Morgan et al., 2020). In addition, bushfires in Australia (particularly southern Australia) have increased in intensity and become more catastrophic. This has given rise to various fire descriptions such as “extreme bushfires,” “catastrophic bushfires,” “fire storms,” and “mega-fires,” among others. Extreme bushfires are very dangerous with high energy levels, chaotic, non-linear movements and deep or widespread flaming (Sharples et al., 2016). This is conducive to the development of violent pyro-convection, which manifests as towering pyrocumulus or pyrocumulonimbus storms (Sharples et al., 2016). While surface temperature, relative humidity, wind speed and drought are the normal drivers of regular bushfires, other factors that contribute to the expression of “extreme” bushfires include extended drought, low fuel moisture content, high atmospheric instability, dry lightning and eruptive fire behaviours (Sharples et al., 2016).
The current Australian climate warming realities and future projections indicate a strong potential for increased incidences of extreme bushfires. At present, a vegetation-rich Australian landscape that results from favourable temperature/solar radiation, and high rainfalls in spring and winter coupled with pronounced dry spells and drought, provide adequate fuel and favourable conditions for extreme bushfires (Melia et al., 2022). The fire weather during a fire event can also contribute to the severity of fire as hot, dry and windy weather conditions enable the spread of fire at rates faster than expected (Abram et al., 2021). Ultimately, the combined interaction of fuel load, fuel dryness, ignition source and fire weather will contribute to the strength and severity of bushfire. More than 23% of temperate forests in south-eastern Australia were burnt in the 2019–2020 fire season, a scale described as unprecedented from an Australian and global point of view (Abram et al., 2021). Anticipated worsening and more frequent incidences of bushfire in the future will be highly dependent on future global atmospheric greenhouse gas concentrations and their contribution to global warming (Melia et al., 2022).
The impact of bushfires exceeds the direct destruction of physical structures such as buildings, natural and plantation forests, orchards and farms. Mega-fires can have devastating impacts on biodiversity, environment, climate, economic livelihood and human health (Table 2).
2.1. Impacts of bushfire on horticultural production
2.1.1. Assumptions and limitations
The observations presented in this review were majorly derived from the experience of the Australian 2019–2020 bushfires. These lessons could be useful in reducing bushfire devastation in other fruit producing regions of the world. In preparing this review paper, we consulted recently published (mostly 2010 till date) bushfire-related articles in the literature. The lack of information on the effects of bushfire on horticultural production systems led to greater consultation of publications on fire-effects on forest trees, being the most abundant in the literature. Literatures on the effect of girdling on carbohydrate translocation in tree crops were similarly consulted as smouldering fires could cause similar effects as tree girdles. Furthermore, this review provides detailed commentary on how fire affects fruit tree carbohydrate translocation and water/nutrient transportation as these two critical physiological processes can determine fruit tree survival or mortality after bushfires. Fire-effect on soil physical, chemical and biological processes are also discussed.
2.1.2. Bushfire visible and non-visible effects on fruit trees
Most orchards are located within and around bushlands and forests. This contiguity to forests and bushlands often makes orchards prone to direct fire incursions or entrance of fire embers during bushfires leading to their damage or destruction (Figure 1). The nature and condition of the prevalent vegetation within the orchard floor may also contribute to the occurrence and spread of fire (Figure 1). High biomass production in annual and perennial grasses could increase orchard fire hazards when grasses dominate the orchard interrow and when dry and dead biomass accumulate on orchard floor due to prolonged hot and dry weather conditions (Winkler et al., 2023). In spite of the potential danger of fire, most orchards in Australia and other parts of the world are not designed to withstand fire. This was the case with the 2019–2020 Australian bushfires when fires burn through orchards damaging trees and destroying other orchard installations (Figure 1).
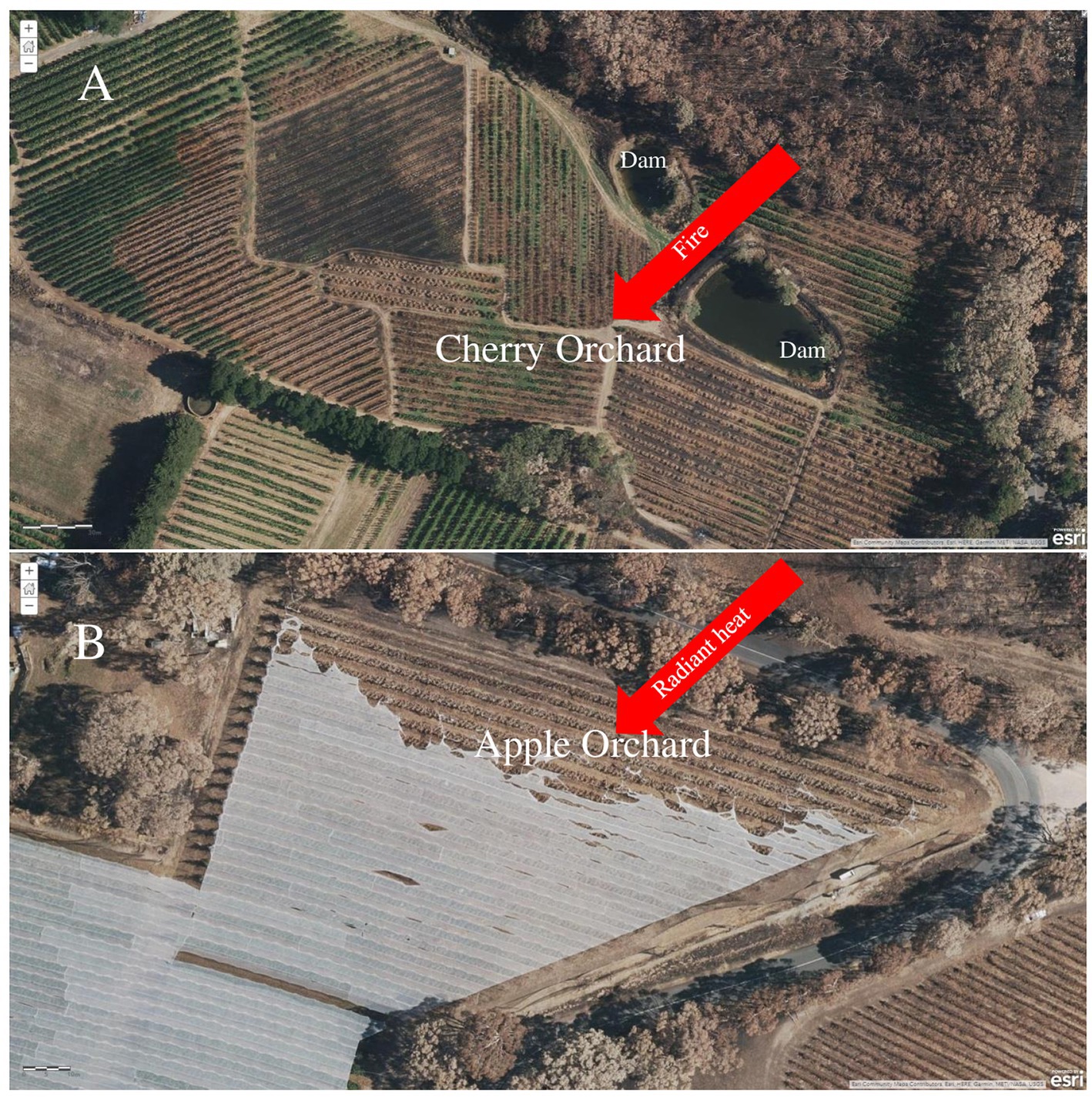
Figure 1. Aerial imageries of a forest-bounded (A) cherry and (B) apple orchards in Adelaide Hill, Australia. The orchards were impacted by bushfire during the 2019–2020 Australian bushfires due to fire invasion from adjoining forests. The fire came from the north for image A and moved through the orchard burning the cover crop in the inter row—the season had been dry, and this burnt easily. For image (A), the fire was within the orchard and damaged the trees directly. Fire also came from the north for image (B). This orchard was exposed to radiant heat damage that was especially intense from burning bush that was located to the north of the block. Note the tattered remains along the northern edge of the net in the image.
Generally, the interplay of various physical and biological processes will determine the effects of bushfire on tree functionality and mortality (Michaletz and Johnson, 2007; Bär et al., 2019). Factors contributing to these processes include site and tree characteristics such as topography, soil and tree physical and thermal properties, and weather conditions including wind profiles, humidity and temperature (Butler and Dickinson, 2010). Fire behaviour, such as smouldering or flaming characteristics, is an additional factor that contributes to bushfire effects on trees (Butler and Dickinson, 2010). Direct effects of fire include noticeable leaf scorching, seared tree bark, burnt branches, flowers and fruits. However, fire damage might not be visible, such as the effects to the tree’s vascular system and other physiological mechanisms. The direct and indirect effects of fire on tree physiology have been well enunciated elsewhere (Bär et al., 2019).
In fruit trees, the frequency, intensity and duration of fire as well as the parts of tree affected will likely determine the extent of damage and subsequent productivity. This review will focus on fruit crops in the Rosaceae family such as apple, pear, cherry, peach and nectarine because they are important horticultural crops, widely grown in climatic zones with high fire risks such as Mediterranean and Temperate climates. Like forest trees, fire effects on fruit trees can be categorised into first-order, which are instant and observable heat injuries on plant tissues, and second-order effects or physiological defects (Campanharo et al., 2019). Depending on the intensity of fire, direct fire damage could result in cell, cambium and phloem necrosis, tissue mortality or instant death of fine roots, tree trunk and crown bud/foliage (Bär et al., 2019).
For tree trunks, the susceptibility to fire is largely dependent on the thermal insulation potential of the trunk, which is a factor of bark thickness, density and moisture content (Michaletz and Johnson, 2007). Vascular tissues of trees with high bark thermal insulation could thus be protected from the lethal effects of fire. Conversely, permanent interruption of the phloem pathway and xylem hydraulic impairments might result in poorly insulated trees, particularly if the flame temperature, residence time and convection rates are substantially high. Horticultural nut trees such as chestnuts and hazelnuts might be less susceptible to bushfire damages because of their propensity to have thicker barks compared to fruit trees. In terms of crown damage, first-order effects on crowns could be influenced by several factors including crown width, surface area, shape, orientation, mass, water content and specific heat capacity (Bär et al., 2019). Direct effects, particularly cambium and phloem necrosis, could trigger a series of physiological or second-order consequences resulting in detrimental effects on tree carbohydrate dynamics, water relations, susceptibility to pathogenic invasions and ultimately mortality (Bär et al., 2019).
2.1.3. Effects of bushfire on carbohydrate dynamics of Rosaceae fruit trees
The main translocated carbohydrates in apples and other fruits in the Rosaceae family are sorbitol and to a lesser degree sucrose (Tijero et al., 2021). Expressed sap from shoots subtending fruit in apple trees, has been shown to have the highest concentration of sorbitol followed by glucose, fructose and sucrose and much lower concentrations of myo-inositol, galactose, raffinose and stachyose (Archbold et al., 2011). Sorbitol is synthesised through the catalysis of glucose-6-phosphate (G6P) to sorbitol-6-phosphate (S6P) by sorbitol-6-phosphate dehydrogenase (S6PDH) and the subsequent dephosphorylation of S6P to sorbitol (Figure 2). This occurs in the leaf mesophyll before translocation to the sink via the complex formed between the companion cell and the sieve element, where it is predominantly converted to fructose by sorbitol dehydrogenase (SDH) (Tijero et al., 2021). Sorbitol is the source of energy and carbon backbones for growth and development in the Rosaceae family as well as a signalling molecule for the regulation of plant growth, development, and environmental stress response (Fang et al., 2020; Tijero et al., 2021). Increased accumulation of sorbitol for enhanced tolerance has been reported in plants facing environmental stresses such as water-logging and salinity (Wang et al., 1996; Fang et al., 2020).
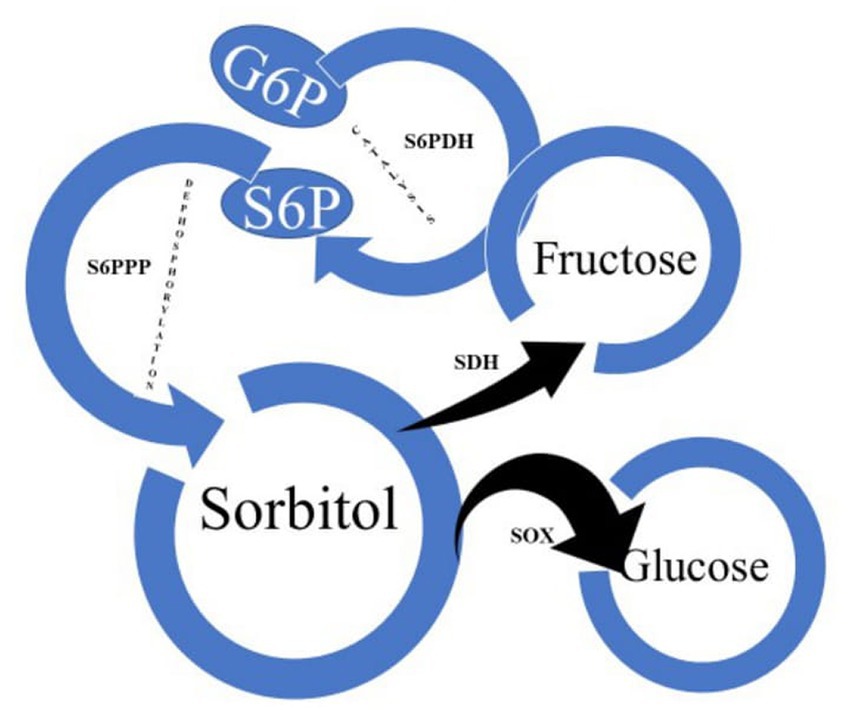
Figure 2. Pathway of sorbitol biosynthesis and metabolism in Rosaceae plants. G6P, glucose-6-phosphate; S6PDH, sorbitol-6-phosphate dehydrogenase; S6P, sorbitol-6-phosphate; S6PPP, sorbitol-6-phosphate phosphatase; SDH, sorbitol dehydrogenase; SOX, sorbitol oxidase.
Crown fires with scorching heat effects on leaves and branches can disrupt the process of photosynthesis by destroying the photosynthetic ability of trees after a bushfire (Michaletz and Johnson, 2007). This disruption will depend on the level of leaf and vegetative bud damage as tree photosynthetic functions will commence with new leaf sprouts (Michaletz and Johnson, 2007; Bär et al., 2019). Smouldering fires could result in longer carbohydrate disruptions within the tree through the formation of heat-induced girdle around affected tree trunks. Like traditional tree girdling, the rise in vascular tissue temperature beyond critical levels could lead to the interruption of the phloem pathway and blockage of the downward translocation of photosynthates (Michaletz and Johnson, 2007; Bär et al., 2019). This interruption may become permanent when heat-induced trunk damage affects the cambium, as phloem regeneration will depend on the cambium condition (Bär et al., 2019). Bär et al. (2019) showed that the effects of fire on tree vascular tissues are similar to trunk-girdled trees. The girdling-like effects of fire on non-structural carbohydrates (NSCs) will vary depending on the part of the tree where it occurs, as cambium and phloem necrosis can occur in different parts of the tree including the trunk, branches and roots. It is proposed that the carbohydrate dynamics in girdled and ungirdled trees could provide clearer perspectives about fire effects on NSCs.
To fully understand the potential of fire-girdling effects on NSC partitioning, particularly in deciduous Rosaceae fruit trees, the seasonal patterns of NSCs in fruit trees need to be elucidated. In general, NSC mobilisation and storage vary from organ to organ. In woody tissue, NSC contents usually increase from bud break until dormancy. However, the rate of NSC accumulation, particularly in summer, is usually higher in the current year shoots compared to “more than 1-year-old shoots,” which often accumulate wood biomass more rapidly compared to NSCs (Jordan and Habib, 1996). Conversely in the roots, NSC concentrations normally decrease in spring to early summer and then re-accumulate strongly through late summer, to reach the highest levels in late autumn, in readiness for the provision of required energy needs of trees during the dormant season and early spring (Naschitz et al., 2010; Mei et al., 2015). A comparison of NSC concentrations in wood and roots at dormancy will often indicate lower concentrations of NSCs in the wood. Tree roots are therefore regarded as the key storage organ in deciduous trees because of the highly important role played by root NSCs during early season development and natural disturbances, such as bushfires (Mei et al., 2015). On the other hand, an inverse trend is expected in NSC concentrations of leaves compared to roots during summer until autumn. NSC leaf concentrations could decline as a result of their movement to sink organs, such as roots (Jordan and Habib, 1996).
The concentration dynamics of starch and soluble sugars such as sucrose and sorbitol, are not the same in tree organs (Figure 3). For example, starch concentrations often increase with wood age and soluble sugar concentrations might remain small throughout the season (Figure 3). While the concentration trends of soluble sugars and starch across the seasons are similar in roots, starch concentration levels are usually higher (Figure 3). Soluble sugar concentration was about one-third of starch concentration in the roots of control peach plants in a girdling experiment (Jordan and Habib, 1996). Lenz (2009) illustrated the seasonal changes in NSCs of Rosaceae trees in 3-year old “Golden Delicious” trees from bud break to leaf abscission (Figure 4).
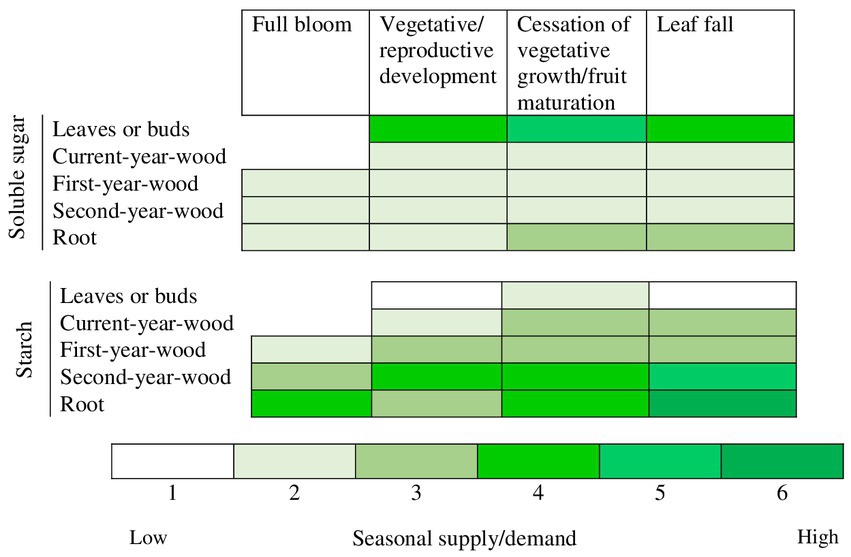
Figure 3. Seasonal partitioning of non-structural carbohydrates in sink and source organs of Rosaceae trees. Adapted from Jordan and Habib (1996).
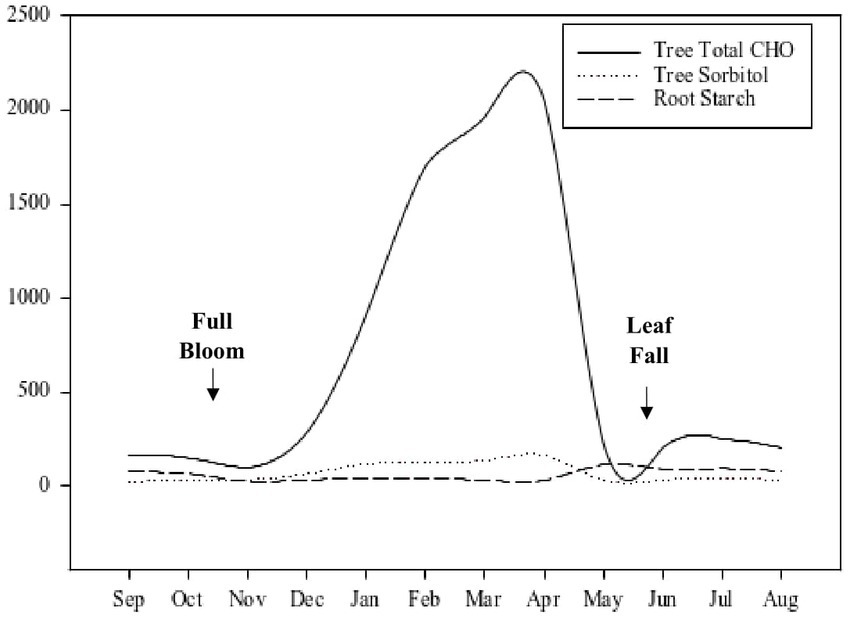
Figure 4. Seasonal changes in the non-structural carbohydrate content (g) of 3-year old “Golden Delicious” apple trees. Adapted from Lenz (2009).
The potential effects of bushfire on NSC storage and mobilisation in Rosaceae fruit trees could be explained through observations made in a trunk girdling study in peach trees (Jordan and Habib, 1996). In this study, girdling treatments were implemented at a similar time to when bushfires are likely in orchards. Summer girdling, 2 weeks before fruit harvest, resulted in starch depletion in roots and rootstock-trunk bark, and significant accumulation in leaves and shoots. On the other hand, soluble sugars in organs above and below the girdled circumference were not significantly affected by the treatment (Jordan and Habib, 1996), as the concentrations were maintained at levels similar to ungirdled trees. Reduced concentration of starch below the girdle could have resulted from starch hydrolysis to supply the sugar needs of the basal part of trees (Glenn and Campostrini, 2011), implying that starch reserves can be mobilised at any time by Rosaceae trees when under stress such as bushfire, and not only during the dormant period of winter. Trunk girdling could consequently reduce root metabolism and growth, and ultimately result in root death (Glenn and Campostrini, 2011). Soluble sugar contents resulting from starch metabolism were maintained at normal concentrations below the girdle while contents above the girdle were similar in concentrations, possibly because of feedback regulation of photosynthesis, due to inability of the photosynthates to be transported beyond the disconnected portion of the tree (Naschitz et al., 2010).
In another girdling study in apple trees, the effects of girdling on carbohydrate partitioning in apple fruits during the period of active starch synthesis are presented in Table 3 (Beruter and Feusi, 1997). The branches bearing fruits were girdled at 82 days after full bloom and the effects of girdling on carbohydrate dynamics in fruits were assessed. This experiment provides insights into scenarios where fire damage resulted in branch girdling within the canopy. Fruit sorbitol, sucrose, glucose and starch concentrations were all affected by branch girdling (Table 3).
The seasonal changes in non-structural carbohydrate reserves of poplar (Populus deltoides × nigra cv. Dorskamp) following trunk girdling were reported by Regier et al. (2010). Poplar is a pioneer tree species with a high probability of recurrent disturbances including fire, and this study provided similar explanations of possible starch and soluble sugar changes in trees. The seasonal variations in starch, sucrose, glucose and fructose concentrations for autumn and spring girdled poplar trees are presented in Table 4. Like Rosacae family, significant starch accumulation above the girdle, and similar soluble sugar concentrations below and above the girdle were observed. It should be noted, however, that the complexities of the period of the season when the disturbance occurs, the level of root reserves and the intensity of fire could determine the rate of source-sink imbalance and ultimate plant response in a bushfire scenario (Regier et al., 2010).
While literature, on the effects of bushfire on carbohydrate dynamics in fruit trees, is scarce and requires further investigation, the results from traditional girdling studies can lead us to conclude that fire damage of the phloem prevents the transportation of assimilates, and particularly starch, towards the roots (Jordan and Habib, 1996; Regier et al., 2010). This interruption may lead to starch accumulation in the trunk tissue and the tree canopy, above the girdle (Regier et al., 2010). Excessive accumulation of non-structural carbohydrates in leaves could cause feedback suppression of CO2 assimilation thereby lowering the photosynthetic rate (Jordan and Habib, 1996; Naschitz et al., 2010). For the lower stem and root region of the fruit tree, fire girdling effects on tree trunk could trigger exhaustive depletion of starch below the girdling zone, leading to carbon starvation of coarse and fine roots. Ultimately, cessation of fine root production, and disruption of water and nutrient uptake/movement to the tree trunk and canopy, may occur (Regier et al., 2010; Bär and Mayr, 2020).
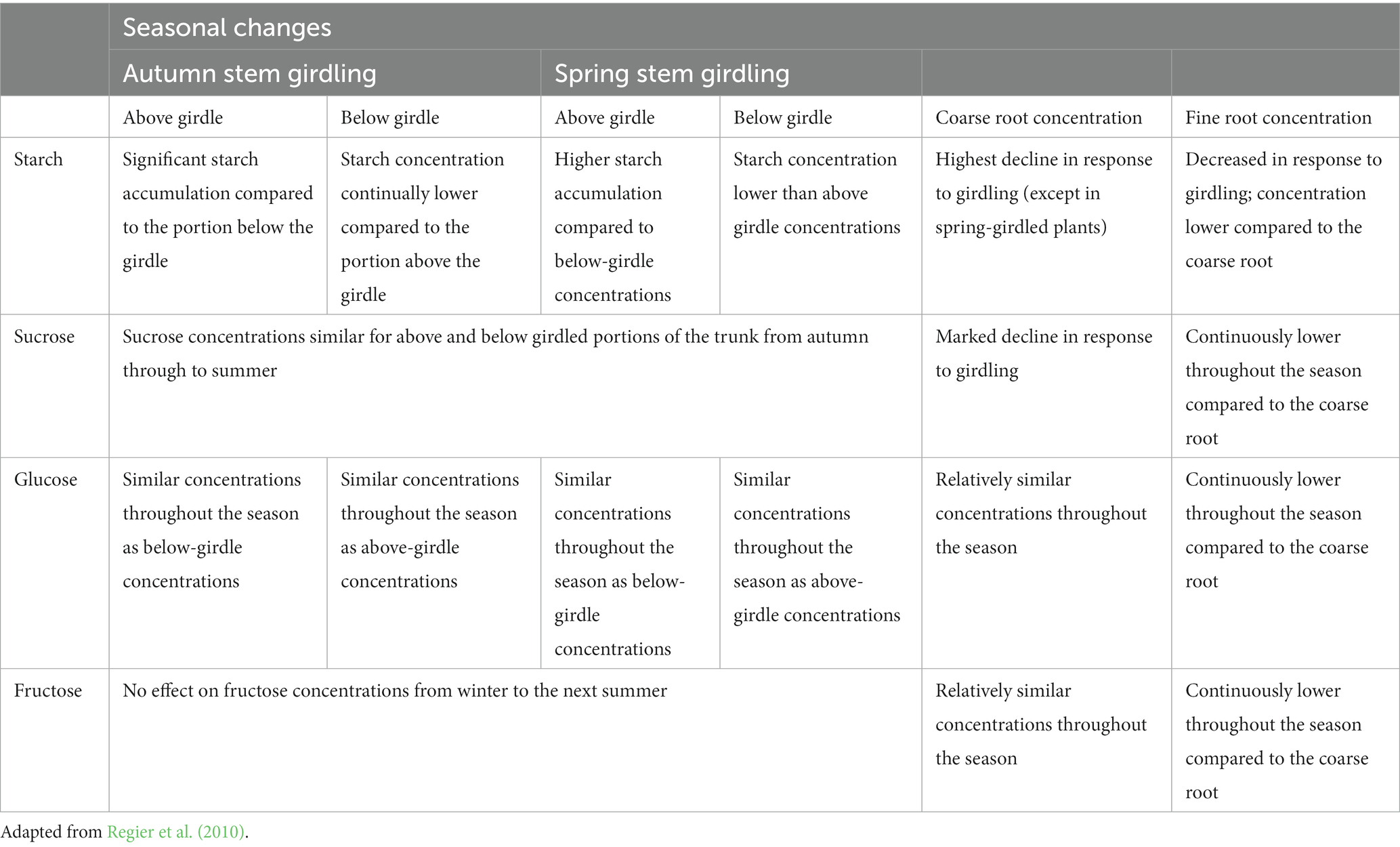
Table 4. Girdling effects on seasonal non-structural carbohydrate concentrations in the stem and root of Populous deltoides.
In situations when tree canopy, leaves and other photosynthetic parts are destroyed, the carbohydrate manufacturing ability of trees could be hindered resulting in a switch to stored carbohydrates, in root and other perennial storage organs, for the various metabolic processes (Glenn and Campostrini, 2011). Physiologically weak trees, as a result of fire damage, may have reduced ability to mobilise and transfer carbohydrate reserves from storage tissues. In the same vein, assimilate production and carbohydrate export in fire-scorched leaves may be reduced and/or inhibited (Glenn and Campostrini, 2011). Furthermore, since plant organs are interdependent for growth and development, disruption of carbohydrate synthesis and metabolism could affect other plant processes such as hormonal balance, floral induction and fruit initiation and development, among others. For example, in addition to being a source of carbohydrates, leaves are known to produce FLOWERING LOCUS T (FT) protein, which is transported to shoot apical meristem to activate floral induction in fruit trees (Belhassine et al., 2019). Fire damage to leaves could therefore alter the process, thereby impeding future floral induction and fruit production.
2.1.4. Effects of bushfire on xylem hydraulics of fruit trees
Smouldering bushfires often affect the outer portion of tree vascular tissues thereby damaging or destroying the phloem and disrupting normal carbohydrate translocation. In addition, increased fire intensity and duration of smouldering fires have been shown to cause destruction of the xylem hydraulic functions in forest trees. Fire may directly destroy the structure of the xylem resulting in the compromise of its structural integrity and functionality (Michaletz et al., 2012; Bär et al., 2019). There could also be enhanced risk of embolism formation within the xylem walls due to air seeding. Xylem cavitation through embolism will cause a reduction in the conductive xylem area leading to a compromised hydraulic efficiency within the xylem (Michaletz et al., 2012; Bär et al., 2019). Short-term hydraulic failure and decreasing resistance to embolism in burned plants were observed in Pinus ponderosa saplings subjected to lethal and non-lethal fire intensities, respectively (Partelli-Feltrin et al., 2020). These short and long-term fire effects both resulted in Pinus ponderosa sapling mortality at different time scales with the decrease in embolism resistance contributing to sapling mortality years following the fire (Partelli-Feltrin et al., 2020).
For scorching crown fires resulting in the burning of foliage, the heat plume could cause acute loss of water during and after the fires (Hoffmann et al., 2021). This could trigger embolism and hydraulic failure in trees, just like smouldering fires. In a simulated foliage scorching experiment on the fire-sensitive evergreen Magnolia grandiflora species, burning caused a 22-fold mean increase in water uptake, with greatest rates of water loss observed at burn intensities that caused complete consumption of leaves (Hoffmann et al., 2021). Several other temperate species such as Oxydendrum aroboreum, Liquidambar styraciflua, Acer rubrum, IIex opaca and Liriodendron tulipfera exhibited increased water uptake during and, ongoing uptake, after burning (Hoffmann et al., 2021). Rapid uptake of water and associated low xylem water potentials could result in possible negative consequences such as xylem cavitation and embolism which could lead to tree mortality (Hoffmann et al., 2021).
For fruit trees such as the Rosaceae family, investigations on the effects of bushfire on xylem hydraulics are still at a nascent stage. We, however, hypothesize that intense crown and smouldering fires will cause similar effects in fruit trees as forest trees. Bushfire induced xylem cavitation and deformation may ultimately impede water and nutrient transport to various tree parts with grievous consequences that can lead to immediate or delayed mortality.
2.2. Effects of bushfire on soil and fruit tree nutrition and health
Apart from the direct effects of fire on the hydraulic functions of the tree, fire can also have negative effects on various soil physical, chemical and biological properties such as soil organic matter content, micro/macro-nutrient composition and structure (Figure 5). High-intensity bushfires (300–315°C) can result in the loss of soil organic matter, volatilisation of nitrogen and partial reduction in phosphorus and sulphur concentrations (Verma and Jayakumar, 2012). In addition, extreme bushfires may lead to the formation of hydrophobic organic compounds and high water repellent soils (Wallbrink et al., 2004; Verma and Jayakumar, 2012). Soil water repellency could reduce soil wetting by a factor of >700 and enhance run-off processes by up to 50% (Doerr et al., 2003). Intense or extreme bushfire ultimately destroys the physical and chemical properties of the topsoil, leading to a structurally and nutritionally deficient medium that limits healthy tree growth (Figure 5).
Soil biology can also be affected directly by the high temperatures of fire (200–400°C), potentially killing beneficial soil microorganisms and invertebrates (Wallbrink et al., 2004; Verma and Jayakumar, 2012). Additionally, the girdling effect of fire on trees could reduce or eliminate tree carbohydrate leachates into the soil with consequential effects on microorganism functioning and reproduction. Reduced microorganism biomass, particularly around the root zone, will lessen the amount of nutrients processed into available forms by microorganisms for the uptake by trees (Zhang et al., 2022). Consequently, vital plant metabolic processes, such as organic compound synthesis, could be impaired as a result of the unavailability of nutrients following a fire. Deficiencies of nitrogen, magnesium, iron, manganese and phosphorus, for instance, can have strong negative effects on the photosynthetic capacity and assimilate production of trees (Wallbrink et al., 2004).
Many studies in the literature showed that forest trees were more susceptible to pests and diseases after bushfires (Mchugh et al., 2003; Parker et al., 2006; Hood and Bentz, 2007; Breece et al., 2008; Catry et al., 2013; Wiley et al., 2016; Hood et al., 2018). Injuries such as wounds often serve as entry points for insects and pathogens, thereby increasing tree vulnerability (Bär et al., 2019). With the release of high amounts of ethanol and volatile terpenes by fire-affected trees, many insects, particularly bark and ambrosia beetles, became attracted to forest trees (Kelsey and Westlind, 2017). Invading insects can be phloeophagous or xylophagous, colonising the phloem or xylem and indirectly introducing pathogenic elements (particularly fungi) into the tree (Hood and Bentz, 2007; Catry et al., 2013). The defence system of trees is also weakened due to fire-induced changes in carbohydrate dynamics (Wiley et al., 2016).
Similar changes would be expected in fire-weakened trees within perennial horticulture production systems. In addition, fire could eliminate the ecological balance of integrated pest and disease management (IPDM) in the orchard, with an opportunistic re-establishment of pests and diseases, which could lead to further post-fire disease and pest pressures.
3. Curtailing the effects of bushfire on horticultural production
3.1. Existing management strategies following fires (based on grower interactions)
Maintaining global horticultural production at current levels require the development of cutting-edge science-based bushfire prevention and management strategies rather than the current unproven approaches. Such management strategies must entail precautionary and proactive measures for minimising the potential effects of bushfire on horticultural production.
Using the 2019–2020 catastrophic fire in Australia as a template, there were no established response strategies for fruit tree orchard recovery after the fires (Dodds, 2020; Idowu et al., 2021). Initial responses after the catastrophic bushfires, in no particular order, were to ensure orchard safety, repair damaged irrigation systems to restore water supply to orchards, and take decisions on trees to be removed or kept based on bark cuts that revealed level of vascular tissue damage. Trees with relatively moist and milky cambium were evaluated as “alive,” while those with a greater proportion of dry and brownish cambium were regarded as “dead.” Decisions on tree mortality were also made based on the assessment of damage to other structures. For example, trees in areas of the orchards that had melted irrigation drip lines were assumed to be affected beyond recovery and removed. Besides, trees were pruned to remove dead parts and protected from pest and pathogenic infestation of resultant wounds through wound dressing procedures such as the application of Bordeaux mixture. In some cases, all the tree branches were removed (poling) to optimise resource use. To stimulate the growth of fine roots and protect from further desiccation, soil was covered with mulch. Lastly, the “watch and act” approach, to observe the effects of fire on trees before taking any major decision, was adopted by some growers (Dodds, 2020; Idowu et al., 2021).
3.2. Recommended horticultural bushfire management strategies
Reducing the effect of bushfires on orchards should start with minimising the potential of fires moving from existing neighbouring bushland. Reducing fuel loads with prescribed burning of neighbouring bushland is a proven method for achieving this (McKemey et al., 2020).
The initial responses after the 2019–2020 fires as described above were reasonable and suitable, contributing to a reduction in the overall mortality of fruit trees within orchards. However, the management of an orchard after a fire must be well-coordinated to reduce possible tree stresses including nutrient, water and carbohydrate requirements.
Supply of resources including the restoration of irrigation must be undertaken slowly and cautiously to prevent any adverse effect on tree recovery. Techniques to improve soil water infiltration and retention must be considered. Three-dimensional cross-linked hydrophilic hydrogel polymer is one option generally believed to have great potential in sustainable agriculture (Singh et al., 2021). If added to soil, hydrogel’s excellent attributes such as water absorption, biodegradability, water retention and slow-release capacity (Singh et al., 2021) could be extremely useful in stabilising water/nutrient supply and reducing erosion risk after orchard fire. In addition, foliar spray of essential nutrients might be used to augment immediate tree needs, if soil nutrient losses are anticipated while growth hormones, mycorrhiza and other beneficial microbes could be added to soil for the improvement of plant root growth, particularly after smouldering fires (Bär et al., 2019).
Another important goal of post-bushfire orchard management is the avoidance or minimization of mortality of fire-damaged trees and the enhancement of their recovery in an economically sustainable manner. Our current research is investigating possible science-based bushfire recovery solutions in order to contribute to reducing the current insufficiency of information in the literature. Orchard management strategies under investigation include bridge grafting of trees with necrotic phloem. Bridge grafting could be a useful technique for re-establishing bushfire associated cambium/phloem dislocations and preventing associated delayed mortality.
Future orchard protection from extreme bushfire should target the establishment of a near-fire-proof systems. This should be envisioned before orchard establishment by paying considerable attention to important issues such as orchard location, components and response in case of fire incursion. Based on noticeable factors that contributed to orchard destruction during the 2019–2020 bushfires in Australia, important considerations should be given to the following in order to develop a relatively more resilient orchard entity:
• Selecting orchard sites on relatively levelled landscape rather than a steep slope terrain.
• Using fire-resistant roofing and wall materials for sheds and trellis posts within the orchard.
• Establishing strategic fire-protection sprinkler systems along susceptible orchard fire entry points.
• Creating within-orchard fuel load reduction plans, particularly in summer, to reduce the possibility of smouldering fires.
• Creating a defensible space or safety zone for fuel breaks (e.g., green strips) around orchards.
• Ensuring fuel reduction in vegetations around fire-prone orchards through effective prescribed burning.
The creation of defensible space and regular reduction of fuel load through prescribed burning will play very critical role in the protection of orchards from approaching fire. These activities will require the corporation of other stakeholders including the responsible government departments and agencies.
3.2.1. Fire break establishment for bushfire management in orchards
Orchard establishment in this era of increased bushfires must integrate fuel breaks as an important component of orchard planning and establishment. Fuel breaks are any treatment involving the removal or modification of vegetation to disrupt fuel continuity or reduce fuel load. Green strips are subsets of fuel breaks developed to strategically install perennial plants with beneficial fire-suppressing attributes in locations where they might suppress or slow the advancement of bushfires. This is done by changing the dynamics of the fire behaviour triangle (fuel, weather and topography) (Tilley, 2019).
The required attributes of green strip tree species include ecological traits such as high moisture retention throughout the bushfire season, site adaptability and ability to persist through periodic extended drought conditions. Tolerance to pests and diseases, low fuel load and flammability, high environmental adaptability, persistence and fire tolerance are other required elements needing consideration (Cuia et al., 2019; Tilley, 2019). Important silvicultural qualities include fast growth rate, ease of establishment and management, smooth bark, branch shedding and rapid decomposition of litter (Cuia et al., 2019; Dieleman et al., 2020). The economic value of the species is also a very important consideration (Cuia et al., 2019).
Most of the effects to orchards during the 2019–2020 fire season in Australia resulted from uncontrollable fires in adjoining monoculture coniferous plantations, which are known for their high flammability. For greatest effectiveness, coniferous plantations within the vicinity of orchards should be replaced by green strips consisting of mixed multilayered species that can serve as a natural barrier for reducing fire intensity and spread, thereby minimising ember attacks. Sustained horticultural protection must include prompt and effective management of previously burnt forests around horticultural enterprises, as unmanaged burnt forest scenarios may result in the growth of fire-prone vegetations and greater future fire risks. The effectiveness of green strips was demonstrated by field-based empirical testing in Guangdong, China in 1985 where a 20-year old Shima superba (Theaceae) green strip suppressed an extreme fire (>10,000 kWm−1), capable of intense rapid spread and continuous crown flames (Cuia et al., 2019).
In addition to green strips, fire-proof orchards will depend to a large extent, on the proactive protection of adjoining forests from severe bushfires through prescribed burning compared to the current fire suppression approach.
3.2.2. Prescribed burning as an efficient bushfire control tool
Prescribed burning has played significant role in the control of bushfires in different parts of the world, including Australia. The evolution of prescribed burning (also referred to as “planned burning,” “fuel reduction burning,” and “hazard reduction burning”) as a management tool in Australia has been well described elsewhere (Morgan et al., 2020). The phases of evolution according to Morgan et al. (2020) were pre-human fire (pre-60,000 years ago), aboriginal period (60,000 years ago to 1788), European settlement period (1788–1901), national development period (1901–1960), development of science-based fire management (1961–1985) and political pressures and land management era (1985 – present).
Indigenous people all over the world gained considerable knowledge in fire management and utilized fire as a potent land management tool on their ancestral estates (McKemey et al., 2020; Morgan et al., 2020). Australian aborigines were the first to utilize fire as a management tool (Morgan et al., 2020) for preventing extensive bushfires and associated economic and environmental damages. However, this practice was disrupted during the era of colonisation when traditional practices were prohibited (Wintle et al., 2020). This resulted in intensive and damaging fires, particularly in southern Australia, with significant threat to economic development (Morgan et al., 2020).
After an initial embrace of fire suppression for dealing with bushfires, there was a gradual use of prescribed burning in forest management starting from 1961 (Morgan et al., 2020). Recent advances are reinforcing the role indigenous fire management could play in the prevention of extreme fire events. Programs, such as Contemporary Indigenous Savanna Burning in northern Australia, are remarkable proactive efforts that had been put in place. Complex traditional ecological and indigenous knowledge, with western science and technology, are being deployed to prevent build-up of fuel and resultant destructive bushfires in the dry season (McKemey et al., 2020). In south-eastern Australia, all relevant agencies involved in prescribed burning of landscapes now involve Aborigines to some extent (McKemey et al., 2020). Apart from the fuel reduction role of prescribed burning which has been in use from time immemorial, it has also been used as a management tool in forestry and agricultural activities to remove pest and diseases, regenerate land, improve soil fertility and quality, among others (Damianidis et al., 2021).
Increases in soil pH, nitrogen, total carbon, potassium and phosphorus contents have been reported immediately after prescribed burning (Ubeda et al., 2005) with pH and total carbon values returning to their pre-fire levels and, nitrogen and phosphorus levels remaining higher after 1 year (Ubeda et al., 2005).
Plant density and floral diversity are important factors that could enhance floral visitation by pollinators (Van Nuland et al., 2013). Fire regimes are important tools for maintaining or increasing plant density and diversity (Van Nuland et al., 2013). Therefore, prescribed burning around an orchard vicinity can indirectly enhance pollinator diversity and abundance by altering neighbouring plant communities. In the long-term, prescribed burning, will not only reduce bushfire impacts on orchards, it could serve as a useful management tool of enhancing orchard productivity.
4. Conclusion: recommendations, knowledge gaps, and prospects
Limiting the effects of fire on fruit tree production requires a thorough comprehension of the effects of fire on tree physiology and soil physical, chemical, and biological properties including IPDM. Knowledge of environmental factors that enhance fire destructiveness is critical. This review described extreme bushfire effects on orchard tree ecosystems, including the visible effects and the resultant physiological disruptions in fruit trees, such as altered carbohydrate dynamics and water/nutrient transportation. Strategic responses for developing fire-proof orchards and preventing future fire incursions were also recommended. Reducing fire effects on orchards will depend to a large extent, on strategic and proactive orchard protection plans that will span its natural life. Management practices within the orchard must ensure reduced fuel load, particularly around the tree trunks to ensure minimal impact, in case of fire incursion. Green strips comprising mixed species of evergreen trees, shrubs and herbaceous plants should form the outer parts of orchard establishments. These vegetation barriers will serve as natural fire retardants that will minimise or halt fire invasion of orchards. More research is needed to identify combinations of trees, shrubs and herbaceous plants that will form excellent fire-inhibiting features, microclimate and hosts for beneficial insects in IPDM programs. To achieve the best results to minimise potential bushfire incursions, green strips should always be used in combination with other protection measures, such as abiotic fire breaks, controlled burning of adjoining vegetation and strategic fire protection sprinklers. The possible combinations of these fire protection tools also require empirical investigations. Research to determine suitable tree pruning operations after fire incursions and the fire-resistant capabilities of the various fruit and nut crops, including rootstocks, will contribute to orchards that can withstand more severe bushfires, which will inevitably occur.
Author contributions
OI: conceptualization, literature review, discussion, and writing of original draft. TP, KD, JG, JF, and PP: discussion, review, and editing of article. BH: conceptualization, discussion, review of article, and supervision. All authors contributed to the article and approved the submitted version.
Funding
Developing management strategies to enhance the recovery of horticulture from bushfires (AS19002) is funded by the Hort Frontiers Advanced Production Systems Fund, part of the Hort Frontiers strategic partnership initiative developed by Hort Innovation, with co-investment from NSW Department of Primary Industries and South Australian Research and Development Institute (SARDI) and contributions from the Australian Government.
Conflict of interest
The authors declare that the research was conducted in the absence of any commercial or financial relationships that could be construed as a potential conflict of interest.
Publisher’s note
All claims expressed in this article are solely those of the authors and do not necessarily represent those of their affiliated organizations, or those of the publisher, the editors and the reviewers. Any product that may be evaluated in this article, or claim that may be made by its manufacturer, is not guaranteed or endorsed by the publisher.
References
Abram, N. J., Henley, B. J., Gupta, A. S., Lippmann, T. J. R., Clarke, H., Dowdy, A. J., et al. (2021). Connections of climate change and variability to large and extreme forest fires in Southeast Australia. Commu. Earth Environ. 2:2. doi: 10.1038/s43247-020-00065-8
Adams, M. A. (2013). Mega-fires, tipping points and ecosystem services: managing forests and woodlands in an uncertain future. For. Ecol. Manag. 294, 250–261. doi: 10.1016/j.foreco.2012.11.039
Archbold, D. D., Nosarzewski, M., Wu, B., and Vuppalapati, P. (2011). “Does availability of soluble carbohydrate reserves determine apple fruit set?” in Acta Horticulturae (Leuven, Belgium: International Society for Horticultural Science), 795–801.
Bär, A., and Mayr, S. (2020). Bark insulation: ten central alpine tree species compared. For. Ecol. Manag. 474:118361. doi: 10.1016/j.foreco.2020.118361
Bär, A., Michaletz, S. T., and Mayr, S. (2019). Fire effects on tree physiology. New Phytol. 223, 1728–1741. doi: 10.1111/nph.15871
Belhassine, F., Martinez, S., Bluy, S., Fumey, D., Kelner, J. J., Costes, E., et al. (2019). Impact of within-tree organ distances on floral induction and fruit growth in apple tree: implication of carbohydrate and gibberellin organ contents. Front. Plant Sci. 10:1233. doi: 10.3389/fpls.2019.01233
Beruter, J., and Feusi, M. E. S. (1997). The effect of girdling on carbohydrate partitioning in the growing apple fruit. J. Plant Physiol. 151, 277–285. doi: 10.1016/S0176-1617(97)80253-2
Blanchi, R., Leonard, J., Haynes, K., Opie, K., James, M., and Oliveira, F. D. D. (2014). Environmental circumstances surrounding bushfire fatalities in Australia 1901-2011. Environ Sci Policy 37, 192–203. doi: 10.1016/j.envsci.2013.09.013
Blanchi, R., Lucas, C., Leonard, J., and Finkele, K. (2010). Meteorological conditions and wildfire-related house loss in Australia. Int. J. Wildl. Fire 19, 914–926. doi: 10.1071/WF08175
Booth, T. H., and Muir, P. R. (2020). Climate change impacts on Australia's eucalypt and coral species: comparing and sharing knowledge across disciplines. WIREs Clim. Chang. 11:e657. doi: 10.1002/wcc.657
Bowman, D. M. J. S., Williamson, G. J., Price, O. F., Ndalila, M. N., and Bradstock, R. A. (2020). Australian forests, megafires and the risk of dwindling carbon stocks. Plant Cell Environ. 44, 347–355. doi: 10.1111/pce.13916
Breece, C. R., Kolb, T. E., Dickson, B. G., McMillin, J. D., and Clancy, K. M. (2008). Prescribed fire effects on bark beetle activity and tree mortality in southwestern ponderosa pine forests. For. Ecol. Manag. 255, 119–128. doi: 10.1016/j.foreco.2007.08.026
Brewer, M. J. A. C. C. B. (2018). The camp fire: meteorological analysis using in situ observations and numerical simulations. Atmos. 11:11. doi: 10.3390/atmos11010047
Butler, B. W., and Dickinson, M. B. (2010). Tree injury and mortality in fires: developing process-based models. Fire Ecol. 6, 55–79. doi: 10.4996/fireecology.0601055
Campanharo, W. A., Lopes, A. P., Anderson, L. O., Da Silva, T. F. M. R., and Aragão, L. E. O. C. (2019). Translating fire impacts in southwestern Amazonia into economic costs. Remote Sens. 11:11. doi: 10.3390/rs11070764
Catry, F. X., Pausas, J. G., Moreira, F., Fernandes, P. M., and Rego, F. (2013). Post-fire response variability in Mediterranean Basin tree species in Portugal. Int. J. Wildland Fire 22, 919–932. doi: 10.1071/WF12215
Chhetri, P., Hashemi, A., Basic, F., Manzoni, A., and Jayatilleke, G. (2012). Bushfire, Heat Wave and Flooding - Case Studies from Australia. Melbourne: Report from the International Panel of the WEATHER project funded by the European Commission’s 7th framework programme.
Cuia, X., Alama, M., Perry, G. L. W., Patersona, A. M., Wysec, S. V., and Currana, T. J. (2019). Green firebreaks as a management tool for wildfires: lessons from China. J. Environ. Manag. 233, 329–336. doi: 10.1016/j.jenvman.2018.12.043
Damianidis, C., Santiago-Freijanes, J. J., den Herder, M., Burgess, P., Mosquera-Losada, M. R., Graves, A., et al. (2021). Agroforestry as a sustainable land use option to reduce wildfires risk in European Mediterranean areas. Agrofor. Syst. 95, 919–929. doi: 10.1007/s10457-020-00482-w
Deb, P., Moradkhani, H., Abbaszadeh, P., Kiem, A. S., Engström, J., Keellings, D., et al. (2020). Causes of the widespread 2019–2020 Australian bushfire season. Earths Fut. 8:8. doi: 10.1029/2020EF001671
Dieleman, C. M., Rogers, B. M., Potter, M., Veraverbeke, S., Johnstone, J. F., Laflamme, J., et al. (2020). Wildfire combustion and carbon stocks in the southern Canadian boreal forest: implications for a warming world. Glob. Chang. Biol. 26, 6062–6079. doi: 10.1111/gcb.15158
Dodds, K. (2020). Bushfires in apple orchards: Observations from the 2019–20 season. NSW, Australia: NSW Department of Primary Industries.
Doerr, S. H., Ferreira, A. J. D., Walsh, R. P. D., Shakesby, R. A., Leighton-Boyce, G., and Coelho, C. O. A. (2003). Soil water repellency as a potential parameter in rainfall-runoff modelling: experimental evidence at point to catchment scales from Portugal. Hydrol. Process. 17, 363–377. doi: 10.1002/hyp.1129
Fang, T., Cai, Y., Yang, Q., Ogutu, O. C., Liao, L., and Han, Y. (2020). Analysis of sorbitol content variation in wild and cultivated apples. J. Sci. Food Agric. 100, 139–144. doi: 10.1002/jsfa.10005
FAO, The impact of disaster and crises on agriculture and food security. (2021), Food and Agriculture Organization of the United Nations, Rome.
Gibbons, P., Gill, A. M., Shore, N., Moritz, M. A., Dovers, S., and Cary, G. J. (2018). Options for reducing house-losses during wildfires without clearing trees and shrubs. Landsc. Urban Plan. 174, 10–17. doi: 10.1016/j.landurbplan.2018.02.010
Glenn, D. M., and Campostrini, E. (2011). Girdling and summer pruning in apple increase soil respiration. Sci. Hortic. 129, 889–893. doi: 10.1016/j.scienta.2011.04.023
Guo, L., Ma, Y., Tigabu, M., Guo, X., Zheng, W., and Guo, F. (2020). Emission of atmospheric pollutants during forest fire in boreal region of China. Environ. Pollut. 264:114709. doi: 10.1016/j.envpol.2020.114709
Hoffmann, W. A., Rodrigues, A. C., Uncles, N., and Rossi, L. (2021). Hydraulic segmentation does not protect stems from acute water loss during fire. Tree Physiol. 41:1785. doi: 10.1093/treephys/tpab057
Hood, S., and Bentz, B. (2007). Predicting postfire Douglas-fir beetle attacks and tree mortality in the northern Rocky Mountains. Can. J. For. Res. 37, 1058–1069. doi: 10.1139/X06-313
Hood, S. M., Varner, J. M., van Mantgem, P., and Cansler, C. A. (2018). Fire and tree death: understanding and improving modeling of fire-induced tree mortality. Environ. Res. Lett. 13:113004. doi: 10.1088/1748-9326/aae934
Hu, Y., Bellaloui, N., Tigabu, M., Wang, J., Diao, J., Wang, K., et al. (2015). Gaseous NO2 effects on stomatal behavior, photosynthesis and respiration of hybrid poplar leaves. Acta Physiol. Plant. 37:39. doi: 10.1007/s11738-014-1749-8
Huijnen, V., Wooster, M. J., Kaiser, J. W., Gaveau, D. L. A., Flemming, J., Parrington, M., et al. (2016). Fire carbon emissions over maritime Southeast Asia in 2015 largest since 1997. Sci. Rep. 6:6. doi: 10.1038/srep26886
Idowu, O., Pitt, T., Dodds, K., Golding, J., Fearnley, J., Petrie, P., et al. (2021). Orchard fire-proofing and post-fire management strategies: a case study of the 2019–2020 bushfires. Aust. Fruitgrower 15, 63–65.
Idowu, O. D., Semple, K. T., Ramadass, K., O'Connord, W., Hansbro, P., and Thavamani, P. (2019). Beyond the obvious: environmental health implications of polar polycyclic aromatic hydrocarbons. Environ. Int. 123, 543–557. doi: 10.1016/j.envint.2018.12.051
Jordan, M. O., and Habib, R. (1996). Mobilizable carbon reserves in young peach trees as evidenced by trunk girdling experiments. J. Exp. Bot. 47, 79–87. doi: 10.1093/jxb/47.1.79
Kelsey, R. G., and Westlind, D. J. (2017). Physiological stress and ethanol accumulation in tree stems and woody tissues at sublethal temperatures from fire. Bioscience 67, 443–451. doi: 10.1093/biosci/bix037
Kirchmeier-Young, M. C., Gillett, N. P., Zwiers, F. W., Cannon, A. J., and Anslow, F. S. (2019). Attribution of the influence of human-induced climate change on an extreme fire season. Earth’s Future 7, 2–10. doi: 10.1029/2018EF001050
Lawes, M. J., Murphy, B. P., Fisher, A., Woinarski, J. C. Z., Edwards, A. C., and Russell-Smith, J. (2015). Small mammals decline with increasing fire extent in northern Australia: evidence from long-term monitoring in Kakadu National Park. Int. J. Wildl. Fire 24, 712–722. doi: 10.1071/WF14163
Lenz, F. (2009). “Fruit effects on the dry matter- and carbohydrate distribution in apple trees” in Acta Horticulture (Belgium: International Society for Horticultural Science), 21–38.
Liu, J. C., Pereira, G., Uhl, S. A., Bravo, M. A., and Bell, M. L. (2015). A systematic review of the physical health impacts from non-occupational exposure to wildfire smoke. Environ. Res. 136, 120–132. doi: 10.1016/j.envres.2014.10.015
Lucas, C., Hennessy, K., Mills, G., and Bathols, J. (2007). Bushfire weather in Southeast Australia: Recent trends and projected climate change impacts Consultancy Report Climate Institute of Australia by Bushfire CRC and CSIRO.
Ma, C. P. R., Downs, J., and Jin, H. (2022). Characterizing spatial patterns of Amazon rainforest wildfires and driving factors by using remote sensing and GIS geospatial technologies. Geosciences 12:12. doi: 10.3390/geosciences12060237
Mchugh, C. W., and Kolb, T. E. And Wilson, J. L., Bark beetle attacks on ponderosa pine following fire in northern Arizona. Environ. Entomol., (2003). 332: p. 510–522, doi: 10.1603/0046-225X-32.3.510
McKemey, M., Ens, E., Rangers, Y. M., Costello, O., and Reid, N. (2020). Indigenous knowledge and seasonal calendar inform adaptive savanna burning in northern Australia. Sustainability (Switzerland) 12, 1–18. doi: 10.3390/su12030995
Mei, L., Xiong, Y., Gu, J., Wang, Z., and Guo, D. (2015). Whole-tree dynamics of non-structural carbohydrate and nitrogen pools across different seasons and in response to girdling in two temperate trees. Oecologia 177, 333–344. doi: 10.1007/s00442-014-3186-1
Melia, N., Dean, S., Pearce, H. G., Harrington, L., Frame, D. J., and Strand, T. (2022). Aotearoa New Zealand's 21st-century wildfire climate. Earth's. Future 10:10. doi: 10.1029/2022EF002853
Michaletz, S. T., and Johnson, E. A. (2007). How forest fires kill trees: a review of the fundamental biophysical processes. Scand. J. For. Res. 22, 500–515. doi: 10.1080/02827580701803544
Michaletz, S. T., Johnson, E. A., and Tyree, M. T. (2012). Moving beyond the cambium necrosis hypothesis of post-fire tree mortality: cavitation and deformation of xylem in forest fires. New Phytol. 194, 254–263. doi: 10.1111/j.1469-8137.2011.04021.x
Morgan, G. W., Tolhurst, K. G., Poynter, M. W., Cooper, N., McGuffog, T., Ryan, R., et al. (2020). Prescribed burning in South-Eastern Australia: history and future directions. Aust. For. 83, 4–28. doi: 10.1080/00049158.2020.1739883
Naschitz, S., Naor, A., Genish, S., Wolf, S., and Goldschmidt, E. E. (2010). Internal management of non-structural carbohydrate resources in apple leaves and branch wood under a broad range of sink and source manipulations. Tree Physiol. 30, 715–727. doi: 10.1093/treephys/tpq028
Nolan, R. H., Boer, M. M., Collins, L., Resco de Dios, V., Clarke, H., Jenkins, M., et al. (2020). Causes and consequences of eastern Australia's 2019–20 season of mega-fires. Glob. Chang. Biol. 26, 1039–1041. doi: 10.1111/gcb.14987
Nolan, R. H., Bowman, D. M. J. S., Clarke, H., Haynes, K., Ooi, M. K. J., Price, O. F., et al. (2021). What do the Australian Black summer fires signify for the global fire crisis? Fire 4:97. doi: 10.3390/fire4040097
Parker, T. J., Clancy, K. M., and Mathiasen, R. L. (2006). Interactions among fire, insects and pathogens in coniferous forests of the interior western United States and Canada. Agric. For. Entomol. 8, 167–189. doi: 10.1111/j.1461-9563.2006.00305.x
Partelli-Feltrin, R., Smith, A. M. S., Adams, H. D., Kolden, C. A., and Johnson, D. M. (2020). Short- and long-term effects of fire on stem hydraulics in Pinus ponderosa saplings. Plant Cell Environ. 4, 696–705. doi: 10.1111/pce.13881
Regier, N., Streb, S., Zeeman, S. C., and Frey, B. (2010). Seasonal changes in starch and sugar content of poplar (Populus deltoides x nigra cv. Dorskamp) and the impact of stem girdling on carbohydrate allocation to roots. Tree Physiol. 30, 979–987. doi: 10.1093/treephys/tpq047
Sharples, J. J., Cary, G. J., Fox-Hughes, P., Mooney, S., Evans, J. P., Fletcher, M., et al. (2016). Natural hazards in Australia: extreme bushfire. Clim. Chang. 139, 85–99. doi: 10.1007/s10584-016-1811-1
Singh, M. A. Z. X. (2021). Analysis of how the spatial and temporal patterns of fire and their bioclimatic and anthropogenic drivers vary across the Amazon rainforest in El Niño and non-El Niño years. PeerJ 9:e12029. doi: 10.7717/peerj.12029
Singh, N., Agarwal, S., Jain, A., and Khan, S. (2021). 3-dimensional cross linked hydrophilic polymeric network “hydrogels”: an agriculture boom. Agric. Water Manag. 253:106939. doi: 10.1016/j.agwat.2021.106939
Tijero, V., Girardi, F., and Botton, A. (2021). Fruit development and primary metabolism in apple. Agronomy 11:1160. doi: 10.3390/agronomy11061160
Tilley, D. A. W. M. (2019). Curlycup gumweed (Grindelia squarrosa [Pursh] Dunal [Asteraceae]): a native forb candidate for inclusion in Great Basin greenstrips. USDA Final Study Report 21, 138–149. doi: 10.3368/npj.21.2.138
Tse, K., Chen, L., Tse, M., Zuraw, B., and Christiansen, S. (2015). Effect of catastrophic wildfires on asthmatic outcomes in obese children: breathing fire. Ann. Allergy Asthma Immunol. 114, 308–311.e4. doi: 10.1016/j.anai.2015.01.018
Turco, M., Rosa-Cánovas, J. J., Bedia, J., Jerez, S., Montávez, J. P., Llasat, M. C., et al. (2018). Exacerbated fires in Mediterranean Europe due to anthropogenic warming projected with nonstationary climate-fire models. Nat. Commun. 9:9. doi: 10.1038/s41467-018-06358-z
Ubeda, X., Lorca, M., Outeiro, L. R., Bernia, S., and Castellnou, M. (2005). Effects of prescribed fire on soil quality in Mediterranean grassland (Prades Mountains, north-East Spain). Int. J. Wildland Fire 14, 379–384. doi: 10.1071/WF05040
Van Nuland, M. E., Haag, E. N., Bryant, J. A. M., Read, Q. D., Klein, R. N., Douglas, M. J., et al. (2013). Fire promotes pollinator visitation: implications for ameliorating declines of pollination services. PLoS One 8:e79853. doi: 10.1371/journal.pone.0079853
van Oldenborg, G. J. V., Krikken, F., Lewis, S., Leach, N. J., Lehner, F., Saunders, K., et al. (2021). Attribution of the Australian bushfire risk to anthropogenic climate change. Nat. Hazards Earth Syst. Sci. 21, 941–960. doi: 10.5194/nhess-21-941-2021
Verma, S., and Jayakumar, S. (2012). Impact of forest fire on physical, chemical and biological properties of soil: a review. Proc. Int. Acad. Ecol. Environ. Sci. 2, 168–176. doi: 10.0000/issn-2220-8860-piaees-2012-v2-0018
Wallbrink, P., Blake, W., Doerr, S., Shakesby, R., Humphreys, G., and English, P. (2005). Using tracer based sediment budgets to assess redistribution of soil and organic material after severe bush fires IAHS-AISH Publication.
Wallbrink, P., English, P., Chafer, C., Humphreys, G., Shakesby, R., Blake, W., et al. (2004). Impacts of water quality by sediments and nutrients released during extreme bushfires: Report 1: a review of the literature pertaining to the effect of fire on erosion and erosion rates, with emphasis on the Nattai catchment, NSW, following the 2001 bushfires. NSW, Australia: CSIRO Land and Water Client Report.
Wang, Z., Quebedeaux, B., and Stutte, G. W. (1996). Partitioning of [14C]glucose into sorbitol and other carbohydrates in apple under water stress. Aust. J. Plant Physiol. 23, 245–251.
Wiley, E., Rogers, B. J., Hodgkinson, R., and Landhausser, S. M. (2016). Nonstructural carbohydrate dynamics of lodgepole pine dying from mountain pine beetle attack. New Phytol. 209, 550–562. doi: 10.1111/nph.13603
Winkler, J., Ježová, M., Punˇcocháˇr, R., Hurajová, E., Martínez Barroso, P., Kopta, T., et al. (2023). Fire Hazard: undesirable ecosystem function of orchard vegetation. Fire 6:25. doi: 10.3390/fire6010025
Wintle, B. A., Legge, S., and Woinarski, J. C. Z. (2020). After the megafires: what next for Australian wildlife? Trends Ecol. Evol. 35, 753–757. doi: 10.1016/j.tree.2020.06.009
WWF (2022). Fire on the farm: Assessing the impacts of the 2019–2020 bushfires on food and agriculture in Australia. NSW, Australia: World Wide Fund for Nature.
Xia, C., and Westwood, T. (2020). Horticulture. Agricult. Commod. 10:55. doi: 10.3316/informit.058544814319716
Keywords: adaptation strategies, carbohydrate dynamics, extreme bushfire, horticultural production, tree nutrition, tree physiology
Citation: Idowu O, Pitt T, Dodds K, Golding J, Fearnley J, Petrie P and Holzapfel B (2023) Building bushfire resilience in horticultural production systems: important insights from Australia. Front. Sustain. Food Syst. 7:1173331. doi: 10.3389/fsufs.2023.1173331
Edited by:
Matteo Balderacchi, Independent Researcher, Piacenza, ItalyReviewed by:
Edward James Raynor, Colorado State University, United StatesHüseyin BarışTecimen, Istanbul University-Cerrahpasa, Türkiye
Tomasz B. Falkowski, New Mexico Highlands University, United States
Copyright © 2023 Idowu, Pitt, Dodds, Golding, Fearnley, Petrie and Holzapfel. This is an open-access article distributed under the terms of the Creative Commons Attribution License (CC BY). The use, distribution or reproduction in other forums is permitted, provided the original author(s) and the copyright owner(s) are credited and that the original publication in this journal is cited, in accordance with accepted academic practice. No use, distribution or reproduction is permitted which does not comply with these terms.
*Correspondence: Oluyoye Idowu, b2x1eW95ZS5pZG93dUBkcGllLm5zdy5nb3YuYXU=