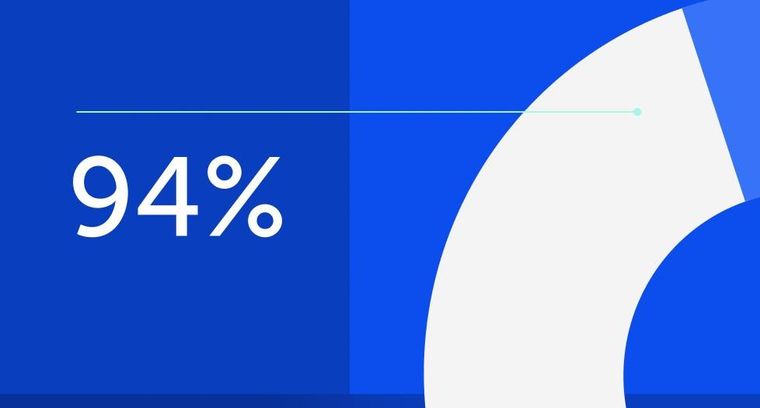
94% of researchers rate our articles as excellent or good
Learn more about the work of our research integrity team to safeguard the quality of each article we publish.
Find out more
ORIGINAL RESEARCH article
Front. Sustain. Food Syst., 02 May 2023
Sec. Crop Biology and Sustainability
Volume 7 - 2023 | https://doi.org/10.3389/fsufs.2023.1170374
This article is part of the Research TopicSoil Microbiome Metabolomics: A Way Forward to Sustainable IntensificationView all 8 articles
Plant Growth Promoting Rhizobacteria (PGPR), a microbial biostimulant has been widely studied to stimulate plant growth through induction of natural biological processes. However, lack of successful validation under open field remains a barrier to mark their standard in agriculture. Therefore, the aim of the research was to highlight the potential of PGPR biostimulants via in vitro studies and subsequently to demonstrate the in planta evaluation in oilseed crop, Arachis hypogaea L. (Groundnut) under pot and field trials. The two rhizobacterial strain was identified as Pseudomonas fluorescens and Bacillus subtilis by 16s rRNA gene sequence analysis. Both the strains were functionally screened for plant growth promoting traits. Pot and field trials were conducted where seeds were inoculated with PGPR biostimulants and harvested at 30 and 90 days. Biostimulant treatments were applied as single and co-inoculation in groundnut and the growth factors, biochemical parameters, yield, and seed quality were analyzed. The study inferred that the consortium of PGPR biostimulants significantly (p < 0.05) showed highest growth, photosynthetic pigments, nodulation status, leghaemoglobin content, yield attributes, and also biofortification of seed nutrients in oilseed crop under both pot and field conditions than un-inoculated control. This study supports the idea of the application of PGPR as microbial biostimulants through successful open field trial to facilitate its implementation as a feasible and potential agricultural product to synthetic fertilizers thereby influencing sustainable and stable crop production.
Agricultural crop productivity and food security are currently the challenges in the center of global attention for resilience and sustainable food system towards eradication of all versions of malnutrition and world hunger. It was predicted that in 2020 between 720 and 811 million people in the world faced hunger. Furthermore, food insecurity and malnutrition have been now exacerbated beneath the shadow of the COVID-19 pandemic that downturns all the progress made in agriculture (FAO, IFAD, UNICEF, WFP and WHO, 2021). To tackle this critical demand, FAO focuses on the implementation of four betters (Better Production, Better Nutrition, Better Environment, and Better Life) to ensure food security, persistent livelihoods and double the food production to attain the Zero Hunger target by 2030 (FAO, 2021).
Groundnut or peanut (Arachis hypogaea L.) is an important and fourth major economic oil yielding crop worldwide with the production of 42.4 million tons. It is an invaluable source of protein, essential fatty acids and other biologically active compounds (Deshmukh et al., 2020). The concerted efforts must be undertaken to face the ever increasing demand for high groundnut production due to its incredible therapeutic values to treat malnutrition (Sayyed et al., 2019). Conventional approaches such as chemical fertilizers and pesticides have been adopted to ensure maximum food production. However, the indispensable input of these chemical products creates a global concern due to their harmful impacts on environments (Rachidi et al., 2021). Thus, the incorporation of plant biostimulant as a novel and environment-friendly strategy in agriculture hold a promise to secure crop performance, yield stability and soil quality (Rouphael et al., 2020; Basu et al., 2021; Hamid et al., 2021).
According to European Regulation (EU, 2019/1009), Plant biostimulant is the “EU fertilizing product the function of which is to stimulate plant nutrition processes independently of the product’s nutrient content with the sole aim of improving one or more of the following plant characteristics: (i) high nutrient utilization efficiency, (ii) abiotic stress tolerance, (iii) quality traits, or (iv) accessibility of soil or rhizospheric nutrients” (EU, 2019). Biostimulants are broadly classified into microbial and non-microbial biostimulant. Beneficial bacteria and fungi comprise the microbial biostimulants whereas the non-microbial biostimulants consist of protein hydrolysates, humic substances, algal extracts and silicon (Rouphael and Colla, 2020; Hamid et al., 2021).
Among the microbial biostimulant, plant growth promoting rhizobacteria (PGPR) as the focus of the study are the most promising category and have attracted the worldwide attention due to their ability to colonize plant roots and produce various secondary metabolites thereby optimizing crop productivity (Ricci et al., 2019; Stoll et al., 2021). The strains of PGPR belong to the genera Azospirillum, Azotobacter, Bacillus, Pseudomonas, and Rhizobium (Castiglione et al., 2021). Over the years, several research studies revealed the positive stimulation impact of PGPR on the plant growth by numerous modes of action such as (i) hormone regulation (Chandra et al., 2018); (ii) phosphate solubilization (Suleman et al., 2018); (iii) siderophore production (Liu et al., 2017; Nithyapriya et al., 2021; Srivastava et al., 2022); (iv) Nutrient acquisition (Nguyen et al., 2019; Jabborova et al., 2020, 2021, 2022); and (v) antioxidant activity (Chandrasekaran et al., 2019; Gowtham et al., 2022). Taking this background into consideration, the present study aimed to hypothesize that the PGPR based microbial biostimulant can stimulate the growth and yield of groundnut through proper validation. To confirm the hypothesis, isolation, characterization and product formulation of PGPR were done followed by pot and field experiments to investigate the growth promotion of groundnut by PGPR biostimulants.
The rhizospheric soil samples were collected from the groundnut field of Poosaripatty (11°86′N, 78°05′E), Salem district, Tamil Nadu, India on January 2020. At the day sampling, average temperature was 26.4°C and relative humidity was 56%. The soil pH was alkaline (7.7), electrical conductivity was 1.2 dSm−1, available nitrogen; 57.6 mg kg−1, available phosphorus; 19.7 mg kg−1, available potassium; 210.0 mg kg−1 and organic carbon was 4.7%. All the obtained rhizospheric soil samples were pooled and subsequently used for chemical and microbiological analysis or stored at −80°C for further studies.
The serially diluted soil samples were processed for isolation of single bacterial colonies on Tryptic Soy Agar (TSA) plates (Hi-Media, Mumbai, India). Upon inoculation, petriplates were incubated at 30°C for 24 h. Distinct colonies of isolates were selected and purified by sub-culturing on nutrient agar under maximum aseptic conditions. Pure cultures were stored and maintained at −80°C as glycerol stocks.
The rhizobacteria were analyzed for PGP attributes.
Detection of IAA production was determined by Ahmad et al. (2008). The isolates were inoculated in Nutrient broth medium supplemented with L-tryptophan (100 μg ml−1) on a shaker at 28 ± 1°C for 48 h in dark. The grown cultures were centrifuged at 10,000 rpm for 10 min at 4°C. Then 2 ml of supernatant was mixed with 4 ml of Salkowski reagent. The positive reaction was visualized by the appearance of pink color. The quantitative estimation of IAA was measured spectrophometrically at 530 nm using the standard curve of IAA ranging from 10 to 100 μg ml−1.
Phosphate solubilization of the bacterial strains was detected by Pikovskaya’s agar plates. The plates were spot inoculated and incubated at 28°C for 7 days. The halo zone around the bacterial colonies was observed (Pikovskaya, 1948) and the phosphate solubilization index was expressed as:
The production of siderophore was assayed according to universal CAS agar plate as described by Schwyn and Neilands (1987). The bacterial culture was spot inoculated in the center of CAS agar plate. After incubation at 28°C for 72 h, the plate was evaluated for the color change.
The reaction mixture containing 1 ml of CAS reagent and 1 ml of cell-free supernatant was used for the quantitative assessment of siderophore production at 630 nm. The siderophore unit (SU) was calculated by:
As = absorbance at 630 nm (test sample).
Ar = absorbance at 630 nm (control).
Production of ammonia was assessed by growing bacterial isolates in peptone water broth at 30°C for 5–6 days. Following incubation 0.5 ml of Nessler’s reagent was added and observed for the development of brown to yellow color (Cappuccino and Sherman, 2014).
Estimation of HCN was carried out by sub culturing the isolates on king’s B medium and nutrient agar amended with 0.4% glycine. The development of brown color on the overlaid Whatman filter paper presaturated with 0.5% picric acid and 2% sodium carbonate solution indicates HCN production (Lorck, 1948).
The morphology and gram characteristics of the isolated rhizobacteria were ascertained using a light microscope. Biochemical characterization such as indole, methyl red (MR), voges-proskaur (VP), starch hydrolysis, citrate, catalase, and oxidase tests were done using standard protocols (Cappuccino and Sherman, 2014).
Molecular identification of the selected isolates were done by the amplification of 16S rRNA gene sequence. The fresh bacterial culture was used for the extraction of total genomic DNA using the protocol of Sharma and Singh (2005). The universal primer pair 27F (5′-AGAGTTTGATCMTGGCTCAG-3′) and 1492R (5′-TACGGYTACCTTGTTACGACTT-3′) was used for the amplification of 16S rRNA gene by Polymerase chain reaction. The PCR generated amplicon was confirmed and purified using GeneJET PCR purification kit (Thermo Scientific, EU-Lithuania). The sequencing of gel purified PCR products was done using Big Dye Terminator 3.1 sequencing kit (Applied Biosystems, USA). The sequences were aligned and edited using Mega Software version 11 and the homology was analyzed by BLASTn search program (Tamura et al., 2021). Neighbor-joining method (Saitou and Nei, 1987) was used to construct phylogenetic tree by MEGA 6 with the boostrap of 100 replicates. The evolutionary distance was computed using Maximum Composite Likelihood method. The gene sequence of 16S rRNA of the selected isolates were subsequently deposited in GenBank database (NCBI).
The groundnut cultivar used for both the pot and field assay was TMV 7 (Arachis hypogaea L.). Groundnut seeds were procured from the Tamil Nadu Agricultural University (TNAU), Coimbatore, Tamil Nadu, India.
A talc based formulation of biostimulants was prepared for both pot and field experiments. The Pseudomonas strain was inoculated in 400 ml of king’s B medium and Bacillus was grown in 400 ml of nutrient broth for 48 h and incubated at 30°C in rotary shaker (180 × g). The fermented liquor containing 3.2 × 108 cfuml−1 was mixed with sterilized talc (1 kg) amended with CaCO3 (15 g L−1) and carboxymethyl cellulose (20 g L−1) and glucose (4 g) under aseptic conditions and allowed to shade dry to attain 35% humidity and then packed in sterile polythene bags and stored at 4°C (Vidhyasekaran and Muthamilan, 1995).
Screened groundnut seeds were surface sterilized with 0.1% of mercury chloride for 5 min and washed thrice with distilled water. Then they are treated with microbial biostimulants at the rate of 10 g kg−1 of seeds and shade dried before sowing.
PGPR biostimulant as a soil drench was supplied directly into the soil near the roots at the rate of 5 g pot−1 and 3 kg acre−1 two times, 20 and 40 days after seed sowing.
The experimental treatments comprised of: control (no inoculation), PSF1 (Pseudomonas fluorescens) inoculation, BAS2 (Bacillus subtilis) inoculation, dual inoculation of PSF1 + BAS2.
The pot assay studies were performed with three replication of each treatment under green house. Ten sterilized and bioinoculated seeds were sown in each pot (12–15 cm diameter), filled with sterilized garden soil, sand and manure (2:1:1). The garden soil used in the study had the following physical and chemical characteristics, pH; 7.2, electrical conductivity; 0.23 dSm−1, organic matter; 3.16%, available nitrogen; 35.50 mg kg−1, available phosphorus; 24.62 mg kg−1 and available potassium was 98.43 mg kg−1. All the treated pots were arranged over a slap under green-house conditions (24/20°C and 70% relative humidity, with alternate cycle of day and night photoperiod of 18/6 h) throughout the experiment. After germination, three germinated seedlings were maintained in each pot. Nitrogen – free sterile tap water was used to irrigate the plants on alternate days. During the bioinoculation, watering should be delayed for a day. No agrochemicals were used during the whole growth period. Plants were uprooted after 90 days of growth and subjected to yield assessment.
The open field trial was carried out in a farmer’s farmland at Thanni Thotti (11°72′N, 78°07′E), Salem, Tamil Nadu, India during June to August 2022. The average temperature of the site was 28.3°C and the relative humidity was 61%.The farmland soil analysis were performed and characterized by alkaline pH (7.2), electrical conductivity EC (0.58 dSm−1), available nitrogen (35.2 mg kg−1), available phosphorus (57 mg kg−1), available potassium (151 mg kg−1) and iron (6.5 ppm). The seeds were sown in the field as per the fore mentioned treatments in a completely randomized block design of 5 × 3 m2 (15m2) and seven lines (225 holes three seed per hole) with 3 replicate blocks. Plant to plant and row to row spacing were arranged at 30 and 20 cm, respectively. Weeding was performed manually. Sufficient irrigation was given as and when required at different growth stages. No agrochemicals were applied to the farmland during the entire growth period other than biostimulants.
All groundnut plants were harvested at 90 days after inoculation (DAI). The morpho - physiological parameters like fresh weight, dry weight, shoot length and root length were measured after harvest from different treatments. Shoots and roots were oven dried at 70°C for 48 h until constant weight to record their dry weight.
The number of nodules per treated plants were counted and subsequently weighed to determine their fresh weight. These nodules were then transferred to petri plates, dried at 70°C for 72 h and weighted to obtain dry mass of nodules.
Nitrogenase activity was measured according to Abd-Alla et al. (2014) in a detached root system by gas chromatography (Thermo Scientific TRACE GC). Briefly, the dissected roots with nodules were inserted into the 500 ml bottles with a rubber septum. 50 ml of acetylene gas was injected into the bottle with the release of same amount of air. Following incubation at 37°C, the root samples were introduced into gas chromatograph and analyzed for nitrogenase activity.
The estimation of leghaemoglobin content was performed as described by Larue and Child (1979). One gram nodules was washed with distilled water and instantly grounded with 5 ml distilled water in an ice-chilled mortar. Then the homogenates were filtered using cheesecloth and centrifuged for 2 min at 500 × g. The filtered supernatant was again centrifuged for 15 min at 12,000 × g to remove the bacteroid content. The resultant supernatant (nodule cytosol) was used to quantify leghaemoglobin level at 540 nm using UV spectrophotometer.
Total chlorophyll contents from the fresh leaves of inoculated plants were quantified and calculated by the method of Arnon (1949) and the carotenoid content was calculated according to Krick and Allen (1965).
When the plants reached the maturity after 90 DAI, number of pods per plant, 100 seed weight and yield per plant were measured from the treated plants. The pods were dried at 80°C until the weight was constant.
Groundnut seeds were retrieved from harvested plants. Seed quality parameters like total protein (Lowry et al., 1951), carbohydrates (Yemm, 1954), seed iron content (Konieczyński and Wesołowski, 2007), and oil content (Sadasivam and Manickam, 2008) were analyzed.
The rhizospheric microbial population were recorded at harvest. The rhizospheric soil samples were taken and transferred to laboratory and stored at 4°C. Total microbial counts were determined by serial dilution method described by Alexander and Zuberer (1991). The samples were inoculated in agar plates and incubated at 28 ± 2°C for 48 h. The microbial populations from each treatment were counted using the colony counter.
All the experimental measurements were performed in triplicate and standard deviation (SD) were calculated for all mean values. The data were statistically evaluated by one-way ANOVA (SPSS 20.0 version Japan Inc., Tokyo, Japan) and differences between treatments were considered to be significant at p ≤ 0.05 using Tukey’s post-hoc test for multiple comparison.
In the present research, two rhizobacteria were isolated from the groundnut rhizospheric soil. Both the isolate were rod shaped and PSF1 was Gram negative whereas BAS2 was Gram positive. They were then functionally validated for their plant growth promoting (PGP) potential, eventually biochemical and molecular characterization were analyzed.
The IAA production in the culture broth of the isolates was determined by the progression of pink color. The pink color was due to the reaction of indole group of IAA with salkowski reagent. PSF1 and BAS2 showed positive reaction and were found to synthesis IAA of about 103.82 ± 1.02 μg ml−1 and 98.51 ± 0.16 μg ml−1, respectively. Both the isolates were positive for phosphate solubilization by spot inoculation in Pikovskaya’s agar medium. The halo zone showed the solubilization of insoluble phosphate as a result of organic acids production by PGPR. PSF1 exhibited higher phosphate solubilization efficiency of about 9.2 mm and BAS2 with 8.7 mm solubilization efficiency. PSF1 and BAS2 were confirmed for the siderophore production by showing orange halo zone around the colonies with more than 80% siderophore unit. The removal of iron from the dye complex of CAS medium by the siderophore of PGPR resulted in orange zone formation. The isolate PSF1 and BAS2 were positive for both ammonia and HCN production (Table 1).
The isolate PSF1 was Gram negative and tested positive for indole, MR, citrate, starch hydrolysis, catalase, oxidase, and negative for VP test. But BAS1 was Gram positive and reacted to indole, VP, citrate, starch hydrolysis, catalase, oxidase tests positively and reacted negatively to MR test.
The 16S rRNA bacterial gene was amplified by PCR, employing universal primers and the product was analyzed through gel electrophoresis and displayed amplicon of 1 kb size. The 16S rRNA gene sequences were subjected to BLASTn for the identification of the isolates and showed similarity with available sequences in Genbank (Table 2). The phylogenetic tree revealed that PSF1 was Pseudomonas fluorescens (Figure 1), and BAS2 was Bacillus subtilis (Figure 2). The 16S rRNA gene sequence of P. fluorescens and B. subtilis were deposited in GenBank database (NCBI) under the accession number MK478897, and ON495960, respectively.
Figure 1. Phylogenetic tree of Pseudomonas fluorescens based on a partial 16S rRNA nucleotide sequences constructed with neighbor joining method.
Figure 2. Phylogenetic tree of Bacillus subtilis based on a partial 16S rRNA nucleotide sequences constructed with neighbor joining method.
The rhizobacterial isolates as PGPR biostimulants were chosen to determine their beneficial effects on groundnut under pot and field trials. Significant results were obtained in terms of growth parameters, physiological and yield parameters, and seed nutrients. Among all treatments, the consortium (PSF1 + BAS2) of PGPR biostimulants had a higher statistically significant impact followed by sole inoculation of PSF1 and BAS2.
In pot trial, the shoot length was enhanced 28.50 and 13.56% by PSF1 and BAS2, respectively, followed by significant (p < 0.05) increase of 36.44% by the co-inoculation of both strains over the control (Figure 3A). Similarly, the root length showed significant (p < 0.05) increase in co-inoculation by 63.16% over other treatments. In field trial, the shoot length of co-inoculated plants was statistically increased 55.71% over control (p < 0.05). The similar pattern was observed in root length of treated plants (Figure 3B). The fresh and dry weight of the plants was considerably higher in co-inoculation as 83.92 and 66.83%, respectively, over control in pot trial. Similarly under field trial, the highest fresh weight and dry weight of 92.12 and 69.36% were obtained by dual inoculation compared to other treatments and control (p < 0.05; Figures 3C,D).
Figure 3. Effect of PGPR biostimulants Pseudomonas fluorescens (PSF1) and Bacillus subtilis (BAS2) on plant growth parameters in pot and field trial. Measured parameters (A) Shoot length; (B) root length; (C) plant fresh; and (D) dry weight. Error bar represents the standard deviation (n = 3). Small alphabetical letters above the error bar indicate significant differences between treatments according to Tukey’s post-hoc test (p ≤ 0.05).
The results on the effect of inoculation of bacteria in number of root nodules were counted at 30 DAI. In pot trial, the number of root nodules was enhanced 39.07 and 25.21% by PSF1 and BAS2, respectively, and 65.52% enhancement was recorded on the consortium application. In field trial, the effect of consortium was greater than the pot trial. It had maximum of 78.35% nodule number than other treatments as shown in Figure 4A. The nodule fresh and dry weight exhibited a similar trend to numbers of nodules in both pot and field experiments (Figures 4B,C).
Figure 4. Effects of PGPR biostimulants Pseudomonas fluorescens (PSF1) and Bacillus subtilis (BAS2) on nodulation, nitrogenase activity and leghaemoglobin content under pot and field conditions. (A) Number of nodules; (B) nodule fresh weight; (C) nodule dry weight; (D) nitrogenase activity; and (E) leghaemoglobin content. Error bar represents the standard deviation (n = 3). Small alphabetical letters above the error bar indicate significant difference between treatments according to Tukey’s post-hoc test (p ≤ 0.05).
Statistical difference was noted in nitrogenase activity of root nodules in pot and field trials (p < 0.05). However, plants with dual inoculation of PSF1 and BAS2 showed higher nitrogenase activity in both trials (Figure 4D). The leghaemoglobin content of nodules was reduced in single inoculation and control (Figure 4E). An increase of 56.92 and 66% was caused by dual inoculation in pot and field trial, respectively.
Photosynthetic pigments (Total chlorophyll and carotenoid) were recorded with significant increase (p < 0.05) upon application of PGPR biostimulant consortium, as compared to control. Plants treated with both PSF1 and BAS2 led to increase in total chlorophyll by 68.09 and 89.23% under pot and field trial, respectively (Figure 5A). The results were analogue to carotenoid content with maximum of 46.58% (pot) and 79.01% (field) in consortium (Figure 5B).
Figure 5. Effect of PGPR biostimulants Pseudomonas fluorescens (PSF1) and Bacillus subtilis (BAS2) on photosynthetic pigments (A) chlorophyll; and (B) carotenoid under pot and field conditions. Error bar represents the standard deviation (n = 3). Small alphabetical letters above the error bar indicate significant difference between treatments according to Tukey’s post-hoc test (p ≤ 0.05).
The pod number per plant was significantly improved (p < 0.05) by the application of PGPR consortium in both pot and field studies (Figure 6A). Higher values of 87.12% in pot and 89.21% in field trial were observed. Inoculation of PGPR consortium had a significant (p < 0.05) effect on 100 seed weight. In pot trial, maximum seed weight of 14.22% by PSF1 + BAS2 was observed. It also exhibited higher seed weight of 16.08% in field trial (Figure 6B). Co-inoculated plants achieved statistically superior yield over other treatments (p < 0.05). PGPR consortium caused maximum increase in the per hectare yield of groundnut, improved it by 39.59% compared to control (Figure 6C). In pot trial, it increased by 38.28% over control. Under both trials, the next substantial yield improvements were produced by single inoculation of PFS1, followed by BAS2.
Figure 6. Effect of PGPR biostimulants Pseudomonas fluorescens (PSF1) and Bacillus subtilis (BAS2) on yield attributes (A) number of pods; (B) 100 seed weight; and (C) pod yield under pot and field conditions. Error bar represents standard deviation (n = 3). Small alphabetical letters above the error bar indicate significant difference between treatments according to Tukey’s post-hoc test (p ≤ 0.05).
The different treatments applied had an important effect on seed nutrients. Dual inoculation showed significantly maximum nutrients (p < 0.05) over control. Protein content was observed with 20.58 and 27.74% increment (Figure 7A) and carbohydrate with 16.66 and 18.78% increment (Figure 7B) when applied with PGPR consortium under both pot and field trials. The maximum biofortification of seed iron increase of 147.26 and 153.41% by PSF1 + BAS2 were noted under pot and field trial, respectively (Figure 7C). Similar result was observed in seed oil content with highest increase of 48.26% (pot) and 52.94% (field) by co-inoculation of PGPR (Figure 7D).
Figure 7. Effect of PGPR biostimulants Pseudomonas fluorescens (PSF1) and Bacillus subtilis (BAS2) on seed nutrients (A) protein; (B) carbohydrate; (C) iron; and (D) oil content. Error bar represents standard deviation (n = 3). Small alphabetical letters above the error bar indicate significant difference between treatments according to Tukey’s post-hoc test (p ≤ 0.05).
The viability of the bacteria after PGPR application in the rhizosphere of groundnut was shown in the Table 3. In pot trial, the application of the PGPR consortium significantly enhanced the microbial population as compared to the un-inoculated treatment. The maximum increase in the microbial count was observed to be 26 × 107 CFUg−1 dry soil under the application of the PGPR consortium (PSF1 + BAS2). Similar findings were obtained from field trial as the maximum of 30 × 107 CFUg−1 dry soil was obtained by the dual inoculation followed by sole inoculation and control.
Microbial plant biostimulant have been successfully used to positively impact the agriculture productivity in a more sustainable manner (Fadiji et al., 2022; Rana et al., 2022; Rai et al., 2023). PGPR as biostimulants enhance the crop performance through various mechanisms under stress condition (Khan et al., 2021; Bhat et al., 2022; Kapadia et al., 2022; Khairnar et al., 2022; Khumairah et al., 2022; Sagar et al., 2022). Therefore, the work sought to isolate, characterize and explore PGPR as microbial biostimulant for their plant growth promotion and yield enhancement in groundnut and successful validation through pot and field experiments. The biochemical and phylogenetic analysis of 16S rRNA gene sequence confirmed the isolated PGPR strains as Pseudomonas fluorescens and Bacillus subtilis. The isolation of various species of Pseudomonas and Bacillus has been extensively studied (Pandey and Gupta, 2020; Yasmin et al., 2020; Kapadia et al., 2022; Srivastava et al., 2022).
For the effective PGPR selection, the PGP traits were identified. Both the strains produce IAA, phosphate solubilization, siderophore, ammonia and HCN. Biosynthesis of IAA by PGPR stimulates the plant growth. Previous studies reported that various IAA producers (Pseudomonas and Bacillus) can promote lateral root growth with the IAA production of about 213.25 ± 1.32 μg ml−1 (Ansari and Ahmad, 2019; Baliyan et al., 2021). In the present study, the maximum IAA was found to be 103.82 ± 1.02 μg ml−1 by PSF1. PGPR often associated with the synthesis of organic acids to facilitate solubilization of inorganic phosphate in soil (Mei et al., 2021). PSF1 and BAS2 were found to be promising phosphate solubilizers. A report suggested that the PGPR (Burkholderia and Rhodopseudomonas) increased the gene regulation for phosphate solubilization and uptake (Wang et al., 2021).
Siderophore production is one of the strategies of PGPR to hinder the growth of pathogens by depriving iron in the micro environment thereby shows indirect biostimulation effect on plants (Khare et al., 2018; Sayyed et al., 2019). Previous studies have reported that more than 70% of the isolated PGPR showed the siderophore production (Dutta and Thakur, 2017; Patel et al., 2018). PSF1 and BAS2 produced siderophore with PSF1 being the best siderophore producer (88.87% siderophore units). Ammonia and HCN are other essential indirect mechanisms of PGPR biostimulant for plant growth promotion and were produced by both strains. Biosynthesis of ammonia linked with the nitrogen supplement to plants eventually result in root and shoot elongation (Bhattacharyya et al., 2020; Kalam et al., 2020; Khumairah et al., 2022; Mir et al., 2022; Rana et al., 2022). Numerous bacterial species of Pseudomonas and Bacillus produce HCN which reacts with the substrate indirectly, leading to an increase in the nutrient availability for rhizobacteria and suppression of plant pathogen of their host plant (Abd El-Rahman et al., 2019).
Numerous studies documented the plant growth promotion activity of PGPR based biostimulants and emphasis it’s potential to stimulate crop health and productivity (Nguyen et al., 2019; Khan et al., 2020; Vetrano et al., 2020; Baba et al., 2021). However, its application faced a huge constrains when scaling up from lab to field. Therefore, the proposed research was focused to assess the efficiency of PGPR based biostimulants on both pot and field studies. The use of PGPR based biostimulants resulted in positive impacts on growth parameters, biomass, photosynthetic pigments, nodulation status, leghaemoglobin content, yield and seed nutrients under both pot and field experiments, with the highest significant increment in dual inoculation.
It is well documented that inoculation of PGPR increases plant growth attributes of groundnut and other oilseed crops (Chen et al., 2018; Ansari and Ahmad, 2019; Gupta et al., 2020; Jabborova et al., 2021, 2022; Manasa et al., 2021; Ilyas et al., 2022). The dual inoculation of the isolates significantly enhanced the plant (root and shoot) length, plant fresh and dry weight in both pot and field trial. The increased vegetative parameters of the plant was due to the production of plant growth regulators like IAA by PSF1 and BAS2 in the rhizosphere as they play a crucial role in stimulating the plant growth by regulating the host genes related to auxin, hormones and cell wall synthesis (Backer et al., 2018).
The nodulation and nitrogenase activity of groundnut were ameliorated by dual inoculation of PSF1 and BAS2 with the highest values in field than in pot trials. Similarly, Abd-El-Khair et al. (2019) proved that P. fluorescens (Pf1) achieved the highest nodulation number (78%) in cowpea followed by B. pumilus (Bp1) with 44%. Dual inoculation with Pseudomonas aeruginosa (LSE-2) and Bradyrhizobium (LSBR-3) significantly improved number of nodules, dry weight of nodules and also leghaemoglobin content in soybean at flowering stage (Kumawat et al., 2019). The proposed mechanism of enhancement of nitrogenase and nodulation activity is due to the response of host to the iron availability by siderophore production of PGPR biostimulants. Iron is an important element for the activity of nitrogen fixation, nitrogenase enzyme and leghaemoglobin (Wu et al., 2023).
The current study illustrated that total chlorophyll and carotenoid contents were increased in co-inoculated than other treatments. Similar results were observed by Ansari and Ahmad (2019) with improved chlorophyll and photosynthetic activity in the co-inoculation of P. fluorescens and Bacillus licheniformis in wheat. Also, significant increase in chlorophyll was observed in wheat plant which received a combined dose of Azospirillum lipoferum, Azospirillum brazilense, and Bacillus sp. compared to control (Akhtar et al., 2021). The formation of chlorophyll synthesis precursor 5-amino ketones pentanoic acid (ALA) requires iron. The synthesis of iron chelating siderophore by the isolates resulted in increased trend in active iron and therefore photosynthetic pigments (Khare et al., 2018).
Studies have been previously reported that the synergistic interaction of PGPR strains in groundnut increased the pod yield under pot and field experiments (Shifa et al., 2016; Liu et al., 2017). These results corroborated the current results exhibiting drastic improvement in the yield and seed weight of the groundnut to the maximum by the co-application of PGPR biostimulant. It has been reported that inoculation of P. fluorescens (Pf1) in cowpea resulted in increased yield percentage (Abd-El-Khair et al., 2019). It has also been shown that co-inoculation with three or four PGPR (Bacillus amyloliquefaciens, Bacillus subtilis, Bacillus pumilus, and Pseudomonas putida) produced significantly higher fruit yield of 46.28 and 39.11% in tomato compared to control (He et al., 2019).
Groundnut plants when treated with PGPR biostimulant enhanced the seed protein, carbohydrate, iron and oil content. This increase in nutrients corresponds with higher seed yield. A similar study by El-Sawah et al. (2021) found that consortium effect of PGPR (Bradyrhizobium and B. subtilis) and AM fungi increased the guar seed protein, carbohydrate and starch content. The result is also in consistent with the previous work which showed clear increase in the oil content of Brassica napus, Brassica arvensis, and Glycine max when inoculated with P. fluorescens (LBUM677) which was closely related with the increase in seed number per plant (Jiménez et al., 2020). Combined action of biochar and rhizobacteria (PGPR) significantly enhanced the seed oil and protein content in A. hypogaea (Ijaz et al., 2020). Particularly, the iron content of seed was attributed to the solubilization and reduction of Fe3+ to Fe2+ by PGPR biostimulant (Sarwar et al., 2022).
When the groundnut plants were treated with PGPR biostimulant, the bacteria well multiplied and colonized in the rhizospheric region. The improved bacterial colonization is responsible for the enhanced activity of PGPR for crop growth and productivity (Hussain et al., 2022). Safdar et al. (2022) reported the enhanced microbial population by the application of biofertilizers favoring the presence of inoculated bacteria for a longer time.
In this study, the ability of the two selected bacterial strains such as PSF1 and BAS2 to promote growth of groundnut as microbial biostimulants in pot trial and its efficacy in the field trials were demonstrated. The microbial strain PSF1 showed the maximum ability to produce IAA, siderophore, ammonia, HCN, and phosphate solubilization than BAS2. The consortium of PGPR biostimulants exhibited maximum positive influence on overall plant growth, nodulation, and yield and seed nutrient in groundnut compared to single inoculation and control in both pot and field experiments.
Application of PGPR biostimulants provided better consequence in terms of both quantity and quality parameters of groundnut. This is an important finding, due to the fact that most of the research studies focused on the application of PGPR under controlled conditions. Our findings clearly demonstrated the positive effectiveness of PGPR biostimulants on groundnut cultivation with the real data obtained from field experiment. In addition, our data showed that co-inoculation of PGPR were more desirable than single inoculation. Therefore, PGPR based microbial biostimulants may help to maintain the ecological balance of agro-ecosystem and limit the usage of pesticides in agriculture. Future research focusing on unlocking the action mechanism of microbial biostimulants in plants will be investigated and reported.
The datasets presented in this study can be found in online repositories. The names of the repository/repositories and accession number(s) can be found at: https://www.ncbi.nlm.nih.gov/-, MK478897 and ON495960.
LS: conceptualization, supervision, and project administration. SE: methodology, investigation, data acquisition, and draft preparations. CT, SA, and TA, and SK: formal analysis, data curation, writing – review and editing, and fund acquisition. All authors contributed to the article and approved the submitted version.
The authors thanks the Researchers Supporting Project number (RSP2023R230) King Saud University, Riyadh, Saudi Arabia, Basic Science Research Program through the National Research Foundation of Korea (NRF) funded by the Ministry of Education (NRF-2016R1A6A1A05011910) and Periyar University for providing University Research Fellowship (PU/AD-3/URF/21F31701/2021).
The authors declare that the research was conducted in the absence of any commercial or financial relationships that could be construed as a potential conflict of interest.
All claims expressed in this article are solely those of the authors and do not necessarily represent those of their affiliated organizations, or those of the publisher, the editors and the reviewers. Any product that may be evaluated in this article, or claim that may be made by its manufacturer, is not guaranteed or endorsed by the publisher.
Abd El-Rahman, A. F., Shaheen, H. A., Abd El-Aziz, R. M., and Ibrahim, D. S. S. (2019). Influence of hydrogen cyanide-producing rhizobacteria in controlling the crown gall and root-knot nematode, meloidogyne incognita. Egypt. J. Biol. Pest Control. 29, 1–11. doi: 10.1186/s41938-019-0143-7
Abd-Alla, M. H., El-Enany, A. W. E., Nafady, N. A., Khalaf, D. M., and Morsy, F. M. (2014). Synergistic interaction of Rhizobium leguminosarumbv. Viciaeand arbuscular mycorrhizal fungi as a plant growth promoting biofertilizers for faba bean (Viciafaba L.) in alkaline soil. Microbiol. Res. 169, 49–58. doi: 10.1016/j.micres.2013.07.007
Abd-El-Khair, H., El-Nagdi, W., Youssef, M., Abd-Elgawad, M. M., and Dawood, M. G. (2019). Protective effect of Bacillus subtilis, Bacillus pumilus, and Pseudomonas fluorescens isolates against root knot nematode Meloidogyne incognita on cowpea. Bull. Natl. Res. Cent. 43, 1–7. doi: 10.1186/s42269-019-0108-8
Ahmad, F., Ahmad, I., and Khan, M. S. (2008). Screening of free-living rhizospheric bacteria for their multiple plant growth promoting activities. Microbiol. Res. 163, 173–181. doi: 10.1016/j.micres.2006.04.001
Akhtar, N., Ilyas, N., Mashwani, Z. R., Hayat, R., Yasmin, H., Noureldeen, A., et al. (2021). Synergistic effects of plant growth promoting rhizobacteria and silicon dioxide nano-particles for amelioration of drought stress in wheat. Plant Physiol. Biochem. 166, 160–176. doi: 10.1016/j.plaphy.2021.05.039
Alexander, D. B., and Zuberer, D. A. (1991). Use of chrome Azurol S reagents to evaluate siderophore production by rhizosphere bacteria. Biol. Fertil. Soils 12, 39–45. doi: 10.1007/BF00369386
Ansari, F. A., and Ahmad, I. (2019). Fluorescent Pseudomonas-FAP2 and Bacillus licheniformis interact positively in biofilm mode enhancing plant growth and photosynthetic attributes. Sci. Rep. 9, 1–12. doi: 10.1038/s41598-019-40864-4
Arnon, O. I. (1949). Copper enzymes in isolated chloroplasts. Polyphenol oxidase in beta vulgaris. Plant Physiol. 24, 1–15. doi: 10.1104/pp.24.1.1
Baba, Z. A., Hamid, B., Sheikh, T. A., Alotaibi, S., Enshasy, H. E., Ansari, M. J., et al. (2021). Psychrotolerant Mesorhizobiumsp. Isolated from temperate and cold desert regions solubilize potassium and produces multiple plant growth promoting metabolites. Molecules 26:5758. doi: 10.3390/molecules26195758
Backer, R., Rokem, J. S., Ilangumaran, G., Lamont, J., Praslickova, D., Ricci, E., et al. (2018). Plant growth-promoting rhizobacteria: context, mechanisms of action, and roadmap to commercialization of biostimulants for sustainable agriculture. Front. Plant Sci. 9:1473. doi: 10.3389/fpls.2018.01473
Baliyan, N., Dhiman, S., Dheeman, S., Kumar, S., and Maheshwari, D. K. (2021). Optimization of indole-3-acetic acid using response surface methodology and its effect on vegetative growth of chick pea. Rhizosphere 17:100321. doi: 10.1016/j.rhisph.2021.100321
Basu, A., Prasad, P., Das, S. N., Sadaf Kalam, R. Z., Sayyed, M. S., Reddy,, et al. (2021). Plant growth promoting rhizobacteria (PGPR) as green bioinoculants: recent developments, constraints, and prospects. Sustainability 13:1140. doi: 10.3390/su13031140
Bhat, B. A., Tariq, L., Nissar, S., Islam, S. T., Islam, S. U., Mangral, Z., et al. (2022). Plant-associated rhizobacteria in plant growth and metabolism as a tool for sustainable agriculture. J. Appl. Microbiol. 1–25. doi: 10.1111/jam.157962022
Bhattacharyya, C., Banerjee, S., Acharya, U., Mitra, A., Mallick, I., Haldar, A., et al. (2020). Evaluation of plant growth promotion properties and induction of antioxidative defense mechanism by tea rhizobacteria of Darjeeling, India. Sci. Rep. 10, 1–19. doi: 10.1038/s41598-020-72439-z
Cappuccino, J. C., and Sherman, N. (2014). Microbiology: a laboratory manual, 10th edition, Pearson, New York.
Castiglione, A. M., Mannino, G., Contartese, V., Bertea, C. M., and Ertani, A. (2021). Microbial biostimulants as response to modern agriculture needs: composition, role and application of these innovative products. Plan. Theory 10:1533. doi: 10.3390/plants10081533
Chandra, D., Srivastava, R., Glick, B. R., and Sharma, A. K. (2018). Drought-tolerant Pseudomonas spp. improve the growth performance of finger millet (Eleusinecoracana L.) Gaertn under non-stressed and drought-stressed conditions. Pedosphere 28, 227–240. doi: 10.1016/S1002-0160(18)60013-X
Chandrasekaran, M., Chun, S. C., Oh, J. W., Paramasivan, M., Saini, R. K., and Sahayarayan, J. J. (2019). Bacillus subtilis CBR05 for tomato (Solanum lycopersicum) fruits in South Korea as a novel plant probiotic bacterium (PPB): implications from total phenolics, flavonoids, and carotenoids content for fruit quality. Agronomy 9:838. doi: 10.3390/agronomy9120838
Chen, Y., Sun, R., Sun, T., Liang, Y., Jiang, Y., and Sun, B. (2018). Organic amendments shift the phosphorus-correlated microbial co-occurrence pattern in the peanut rhizosphere network during long-term fertilization regimes. Appl. Soil Ecol. 124, 229–39. doi: 10.1016/j.apsoil.2017.11.023
Deshmukh, D. B., Marathi, B., Sudini, H. K., Variath, M. T., Chaudhari, S., Manohar, S. S., et al. (2020). Combining high oleic acid trait and resistance to late leaf spot and rust diseases in groundnut (Arachishypogaea L.). Front. Genet. 11:514. doi: 10.3389/fgene.2020.00514
Dutta, J., and Thakur, D. (2017). Evaluation of multifarious plant growth promoting traits, antagonistic potential and phylogenetic affiliation of rhizobacteria associated with commercial tea plants grown in Darjeeling, India. PLoS One 12:e0182302. doi: 10.1371/journal.pone.0182302
El-Sawah, A. M., El-Keblawy, A., Ali, D. F. I., Ibrahim, H. M., El-Sheikh, M. A., Sharma, A., et al. (2021). Arbuscular mycorrhizal fungi and plant growth-promoting rhizobacteria enhance soil key enzymes, plant growth, seed yield, and qualitative attributes of guar. Agriculture 11:194. doi: 10.3390/agriculture11030194
EU (2019). Regulation of the European Parliament and of the council laying down rules on the making available on the market of EU Fertilising products and amending regulations. Italy.
Fadiji, A. E., Babalola, O. O., Santoyo, G., and Perazzolli, M. (2022). The potential role of microbial biostimulants in the amelioration of climate change-associated abiotic stresses on crops. Front. Microbiol. 12:829099. doi: 10.3389/fmicb.2021.829099
FAO (2021). FAO’s strategic framework 2022–31. Rome: Food and Agriculture Organization of the United Nations.
FAO, IFAD, UNICEF, WFP and WHO (2021). The State of Food Security and Nutrition in the World 2021.Transforming food systems for food security, improved nutrition and affordable healthy diets for all, Rome.
Gowtham, H. G., Singh, S. B., Shilpa, N., Aiyaz, M., Nataraj, K., Udayashankar, A. C., et al. (2022). Insight into recent progress and perspectives in improvement of antioxidant machinery upon PGPR augmentation in plants under drought stress: a review. Antioxidants 11:1763. doi: 10.3390/antiox11091763
Gupta, V., Kumar, G. N., and Buch, A. (2020). Colonization by multi-potential Pseudomonas aeruginosa P 4 stimulates peanut (Arachis hypogaea L.) growth, defence physiology and root system functioning to benefit the root-rhizobacterial interface. J. Plant Physiol. 248:153144. doi: 10.1016/j.jplph.2020.153144
Hamid, B., Zaman, M., Farooq, S., Fatima, S., Sayyed, R. Z., Baba, Z. A., et al. (2021). Bacterial plant biostimulants: a sustainable way towards improving growth, productivity, and health of crops. Sustainability 21:2856. doi: 10.3390/su13052856
He, Y., Pantigoso, H. A., Wu, Z., and Vivanco, J. M. (2019). Co-inoculation of Bacillus sp. and Pseudomonas putida at different development stages acts as a biostimulant to promote growth, yield and nutrient uptake of tomato. J. Appl. Microbiol. 127, 196–207. doi: 10.1111/jam.14273
Hussain, A., Iqbal, Z., Aurangzaib, M., Naeem, M., Mustafa, S., Shoaib, M., et al. (2022). Comparative effectiveness of plant growth promoting rhizobacteria and various organic carriers on wheat growth, physiology, antioxidative activities, and rhizosphere properties. Pak. J. Bot. 54, 317–324. doi: 10.30848/PJB2022-1(43)
Ijaz, M., Perveen, S., Nawaz, A., Ul-Allah, S., Sattar, A., Sher, A., et al. (2020). Eco-friendly alternatives to synthetic fertilizers for maximizing peanut (Arachis hypogea L.) production under arid regions in Punjab, Pakistan. J. Plant Nutr. 43, 762–772. doi: 10.1080/01904167.2019.1702203
Ilyas, N., Akhtar, N., Naseem, A., Qureshi, R., Majeed, A., and Sayyed, R. Z. (2022). The potential of Bacillus subtilis and phosphorus in improving the growth of wheat under chromium stress. J. Appl. Microbiol. 133, 3307–3321. doi: 10.1111/jam.15676
Jabborova, D., Annapurna, K., Azimov, A., Tyagi, S., Pengani, K. R., Sharma, S., et al. (2022). Co-inoculation of biochar and arbuscular mycorrhizae for growth promotion and nutrient fortification in soybean under drought conditions. Front. Plant Sci. 13:947547. doi: 10.3389/fpls.2022.947547
Jabborova, D., Kannepalli, A., Davranov, K., Narimanov, A., Enakiev, Y., Syed, A., et al. (2021). Co-inoculation of rhizobacteria promotes growth, yield, and nutrient contents in soybean and improves soil enzymes and nutrients under drought conditions. Sci. Report. 11:22081. doi: 10.1038/s41598-021-01337-9
Jabborova, D., Wirth, S., Kannepalli, A., Narimanov, A., Desouky, S., Davranov, K., et al. (2020). Co-inoculation of rhizobacteria and biochar application improves growth and nutrient in soybean and enriches soil nutrients and enzymes. Agronomy 10:1142. doi: 10.3390/agronomy10081142
Jiménez, J. A., Novinscak, A., and Filion, M. (2020). Pseudomonas fluorescens LBUM677 differentially increases plant biomass, total oil content and lipid composition in three oilseed crops. J. Appl. Microbiol. 128, 1119–1127. doi: 10.1111/jam.14536
Kalam, S., Basu, A., Ahmad, I., Sayyed, R. Z., Enshasy, H. E., Dailin, D. J., et al. (2020). Recent understanding of soil acidobacteria and their ecological significance: a critical review. Front. Microbiol. 11:580024. doi: 10.3389/fmicb.2020.580024
Kapadia, C., Kachhdia, R., Singh, S., Gandhi, K., Poczai, P., Alfarraj, S., et al. (2022). Pseudomonas aeruginosa inhibits quorum sensing mechanisms of soft rot pathogen Lelliottia amnigena RCEto regulate its virulence factors and biofilm formation. Front. Microbiol. 13:977669. doi: 10.3389/fmicb.2022.977669
Khairnar, M., Hagir, A., Parmar, K., Sayyed, R., James, E., and Rahi, P. (2022). Phylogenetic diversity and plant growth-promoting activities of rhizobia nodulating fenugreek (Trigonella foenumgraecum Linn.) cultivated in different agroclimatic regions of India. FEMS Microbiol. Ecol. 98:fiac 014. doi: 10.1093/femsec/fiac014
Khan, N., Ali, A., Shahi, M. A., Mustafa, A., Sayyed, R. Z., and Curaá, J. A. (2021). Insights into the interactions among roots, rhizosphere and rhizobacteria for improving plant growth and tolerance to abiotic stresses: a review. Cells 10:1551. doi: 10.3390/cells10061551
Khan, I., Awan, S. A., Ikram, R., Rizwan, M., Akhtar, N., Yasmin, H., et al. (2020). Effects of 24-epibrassinolide on plant growth, antioxidants defense system, and endogenous hormones in two wheat varieties under drought stress. Physiol. Plant. 172, 1–11. doi: 10.1111/ppl.13237
Khare, E., Mishra, J., and Arora, N. K. (2018). Multifaceted interactions between endophytes and plant developments and prospects. Front. Microbiol. 9:2732. doi: 10.3389/fmicb.2018.02732
Khumairah, F. H., Setiawati, M. R., Fitriatin, B. N., Simarmata, T., Alfaraj, S., Ansari, M. J., et al. (2022). Halotolerant plant growth promoting rhizobacteria isolated from saline soil improve nitrogen fixation and alleviate salt stress. Front. Microbiol. 13:905210. doi: 10.3389/fmicb.2022.905210
Konieczyński, P., and Wesołowski, M. (2007). Determination of zinc, iron, nitrogen and phosphorus in several botanical species of medicinal plants. Pol. J. Environ. Stud. 16, 785–790.
Krick, J. T. O., and Allen, R. L. (1965). Dependence of chloroplast pigments synthesis on protein synthetic selenium dioxide were powdered separately and mixed together effects on actilione. Biochem. Biophys. Res. Commun. 21, 523–530. doi: 10.1016/0006-291x(65)90516-4
Kumawat, K. C., Sharma, P., Sirari, A., Singh, I., Gill, B. S., Singh, U., et al. (2019). Synergism of Pseudomonas aeruginosa (LSE-2) nodule endophyte with Bradyrhizobium sp. (LSBR-3) for improving plant growth, nutrient acquisition and soil health in soybean. World J. Microbiol. Biotechnol. 35:47. doi: 10.1007/s11274-019-2622-0
Larue, T. A., and Child, J. J. (1979). Sensitive fluorometric assay for leghemoglobin. Anal. Biochem. 92, 11–15. doi: 10.1016/0003-2697(79)90618-3
Liu, D., Yang, Q., Ge, K., Hu, X., Qi, G., Du, B., et al. (2017). Promotion of iron nutrition and growth on peanut by Paenibacillus illinoisensis and Bacillus sp. strains in calcareous soil. Braz. J. Microbiol. 48, 656–670. doi: 10.1016/j.bjm.2017.02.006
Lorck, H. (1948). Production of hydrocyanic acid by bacteria. Physiol. Plant. 1, 142–146. doi: 10.1111/j.1399-3054.1948.tb07118.x
Lowry, O. H., Rosebrough, N. R., Farr, A. L., and Randall, R. J. (1951). Protein measurement with the Folin phenol reagent. J. Biol. Chem. 193, 265–275. doi: 10.1016/S0021-9258(19)52451-6
Manasa, M., Ravinder, P., Gopalakrishnan, S., Srinivas, V., Sayyed, R. Z., Enshasy, H. E., et al. (2021). Co-inoculation of Bacillus spp. for growth promotion and iron fortification in sorghum. Sustainability 13:12091. doi: 10.3390/su132112091
Mei, C., Chretien, R. L., Amaradasa, B. S., He, Y., Turner, A., and Lowman, S. (2021). Characterization of phosphate solubilizing bacterial endophytes and plant growth promotion in vitro and in greenhouse. Microorganisms 9:1935. doi: 10.3390/microorganisms9091935
Mir, M. I., Bee, H., Quadriya, H., Kumar, B. K., Ilyas, I., Kasem, H. S., et al. (2022). Multifarious indigenous diazotrophic rhizobacteria of rice (Oryzasativa L.) rhizosphere and their effect on plant growth promotion, Frontiers. Nutrition 8:781764. doi: 10.3389/fnut.2021.781764
Nguyen, M. L., Spaepen, S., du Jardin, P., and Delaplace, P. (2019). Biostimulant effects of rhizobacteria on wheat growth and nutrient uptake depend on nitrogen application and plant development. Arch. Agron. Soil Sci. 65, 58–73. doi: 10.1080/03650340.2018.1485074
Nithyapriya, S., Lalitha, S., Sayyed, R. Z., Reddy, M. S., Dailin, D. J., Enshasy, H. E., et al. (2021). Production, purification, and characterization of bacillibactinsiderophore of Bacillus subtilis and its application for improvement in plant growth and oil content in sesame. Sustainability 13:5394. doi: 10.3390/su13105394
Pandey, S., and Gupta, S. (2020). Evaluation of Pseudomonas sp. for its multifarious plant growth promoting potential and its ability to alleviate biotic and abiotic stress in tomato (Solanum lycopersicum) plants. Sci. Rep. 10, 1–15. doi: 10.1038/s41598-020-77850-0
Patel, P. R., Shaikh, S. S., and Sayyed, R. Z. (2018). Modified chrome azurol S method for detection and estimation of siderophores having affinity for metal ions other than iron. Environ. Sustain. 1, 81–87. doi: 10.1007/s42398-018-0005-3
Pikovskaya, R. I. (1948). Mobilization of phosphorus in soil in connection with the vital activity of some microbial species. Mikrobiologiya 17, 362–370.
Rachidi, F., Benhima, R., Kasmi, Y., Sbabou, L., and Arroussi, H. E. (2021). Evaluation of microalgae polysaccharides as biostimulants of tomato plant defence using metabolomics and biochemical approaches. Sci. Rep. 11, 1–16. doi: 10.1038/s41598-020-78820-2
Rai, S., Omar, A. F., Rehan, M., Al-Turki, A., Sagar, A., Ilyas, N., et al. (2023). Crop microbiome: their role and advances in molecular and omic techniques for the sustenance of agriculture. Planta 257:27. doi: 10.1007/s00425-022-04052-5
Rana, K. L., Kour, D., Kaur, T., Devi, R., Rai, P. K., Singh, S., et al. (2022). Endophytic nitrogen-fixing bacteria: untapped treasurer for agricultural sustainability. J. Appl. Biol. Biotechnol. 11, 75–93. doi: 10.7324/JABB.2023.110207
Ricci, M., Tilbury, L., Daridon, B., and Sukalac, K. (2019). General principles to justify plant biostimulant claims. Front. Plant Sci. 10:494. doi: 10.3389/fpls.2019.00494
Rouphael, Y., and Colla, G. (2020). Toward a sustainable agriculture through plant biostimulants: from experimental data to practical applications. Agronomy 10, 1–10. doi: 10.3390/agronomy10101461
Rouphael, Y., Lucini, L., Miras-Moreno, B., Colla, G., Bonini, P., and Cardarelli, M. (2020). Metabolomic responses of maize shoots and roots elicited by combinatorial seed treatments with microbial and non-microbial biostimulants. Front. Microbiol. 11:664. doi: 10.3389/fmicb.2020.00664
Sadasivam, S., and Manickam, A. (2008). Biochemical methods, 3rd edition, New Age International (P) Limited, New Delhi, India.
Safdar, H., Jamil, M., Hussain, A., Albalawi, B. F. A., Ditta, A., Dar, A., et al. (2022). The effect of different carrier materials on the growth and yield of spinach under pot and field experimental conditions. Sustainability 14:12255. doi: 10.3390/su141912255
Sagar, A., Sayyed, R. Z., Ramteke, P. W., Ramakrishna, W., Poczai, P., Obaid, S. A., et al. (2022). Synergistic effect of Azotobacter nigricansand NPK fertilizer on agronomic and yield traits of maize (Zea mays L.). Front. Plant Sci. 13:952212. doi: 10.3389/fpls.2022.952212
Saitou, N., and Nei, M. (1987). The neighbor-joining method: a new method for reconstructing phylogenetic trees. Mol. Biol. Evol. 4, 406–425. doi: 10.1093/oxfordjournals.molbev.a040454
Sarwar, S., Khaliq, A., Yousra, M., and Sultan, T. (2022). Iron biofortification potential of siderophore producing rhizobacterial strains for improving growth, yield and iron contents of groundnut. J. Plant Nutr. 45, 2332–2347. doi: 10.1016/j.rhisph.2021.100321
Sayyed, R. Z., Sonia Seifi, P. R., Patel, S. S. S., Jadhav, H. P., and El Enshasy, H. (2019). Siderophore production in groundnut rhizosphere isolate, Achromobacter sp. RZS2 influenced by physicochemical factors and metal ions. Environ. Sustain. 2, 117–124. doi: 10.1007/s42398-019-00070-4
Schwyn, B., and Neilands, J. B. (1987). Universal chemical assay for the detection and determination of siderophores. Anal. Biochem. 160, 47–56. doi: 10.1016/0003-2697(87)90612-9
Sharma, A. D., and Singh, J. (2005). A nonenzymatic method to isolate genomic DNA from bacteria and actinomycete. Anal. Biochem. 337:354356, 354–356. doi: 10.1016/j.ab.2004.11.029
Shifa, H., Tasneem, S., Gopalakrishnan, C., and Velazhahan, R. (2016). Biological control of pre-harvest aflatoxin contamination in groundnut (Arachishypogaea L.) with Bacillus subtilis G1. Arch. Phytopathol. Plant Protect. 49, 137–148. doi: 10.1080/03235408.2016.1160642
Srivastava, P., Sahgal, M., Sharma, K., Enshasy, H. E., Gafur, A., Alfarraj, S., et al. (2022). Optimization and identification of siderophores produced by Pseudomonas monteilli strain MN759447 and its antagonism towards fungus associated with mortality in Dalbergiasissoo plantation forests. Front. Plant Sci. 13:984522. doi: 10.3389/fpls.2022.984522
Stoll, A., Salvatierra-Martínez, R., González, M., Cisternas, J., Rodriguez, Á., Vega-Gálvez, A., et al. (2021). Importance of crop phenological stages for the efficient use of PGPR inoculants. Sci. Rep. 11, 19548–19549. doi: 10.1038/s41598-021-98914-9
Suleman, M., Yasmin, S., Rasul, M., Yahya, M., Atta, B. M., and Mirza, M. S. (2018). Phosphate solubilizing bacteria with glucose dehydrogenase gene for phosphorus uptake and beneficial effects on wheat. PLoS One 13:e0204408. doi: 10.1371/journal.pone.0204408
Tamura, K., Stecher, G., and Kumar, S. (2021). MEGA11: molecular evolutionary genetic analysis version 11. Mol. Biol. Evol. 38, 3022–3027. doi: 10.1093/molbev/msab120
Vetrano, F., Miceli, C., Angileri, V., Frangipane, B., Moncada, A., and Miceli, A. (2020). Effect of bacterial inoculum and fertigation management on nursery and field production of lettuce plants. Agronomy 10, 1–19. doi: 10.3390/agronomy10101477
Vidhyasekaran, P., and Muthamilan, M. (1995). Development of formulations of Pseudomonas fluorescens for control of chickpea wilt. Plant Dis. 79, 782–786. doi: 10.1094/PD-79-0782
Wang, Y., Peng, S., Hua, Q., Qiu, C., Wu, P., Liu, X., et al. (2021). The long-term effects of using phosphate-solubilizing bacteria and photosynthetic bacteria as biofertilizers on peanut yield and soil bacteria community. Front. Microbiol. 12:693535. doi: 10.3389/fmicb.2021.693535
Wu, X., Wang, Y., Ni, Q., Li, H., Wu, X., Yuan, Z., et al. (2023). GmYSL7 controls iron uptake, allocation and cellular response of nodule in soybean. J. Integr. Plant Biol. 65, 167–187. doi: 10.1111/jipb.13364
Yasmin, H., Naseem, S., Bakhtawar, M., Jabeen, Z., Nosheen, A., Naz, R., et al. (2020). Halotolerant rhizobacteria Pseudomonas pseudoalcaligenes and Bacillus subtilis mediate systemic tolerance in hydroponically grown soybean (Glycine max L.) against salinity stress. PLoS One 15:e0231348. doi: 10.1371/journal.pone.0231348
Keywords: Arachis hypogaea, Bacillus subtilis, biostimulants, groundnut, Pseudomonas fluorescens
Citation: Eswaran SUD, Sundaram L, Siang TC, Alharbi SA, Alahmadi TA and Kadam SK (2023) Multifarious microbial biostimulants promote growth in Arachis hypogaea L. Front. Sustain. Food Syst. 7:1170374. doi: 10.3389/fsufs.2023.1170374
Received: 20 February 2023; Accepted: 05 April 2023;
Published: 02 May 2023.
Edited by:
Dilfuza Egamberdieva, Leibniz Center for Agricultural Landscape Research (ZALF), GermanyReviewed by:
Dewa Ngurah Suprapta, Udayana University, IndonesiaCopyright © 2023 Eswaran, Sundaram, Siang, Alharbi, Alahmadi and Kadam. This is an open-access article distributed under the terms of the Creative Commons Attribution License (CC BY). The use, distribution or reproduction in other forums is permitted, provided the original author(s) and the copyright owner(s) are credited and that the original publication in this journal is cited, in accordance with accepted academic practice. No use, distribution or reproduction is permitted which does not comply with these terms.
*Correspondence: Lalitha Sundaram, bGFyYTlrQGdtYWlsLmNvbQ==; Tan Ching Siang, dGNzaWFuZ0BrcGp1Yy5lZHUubXk=
Disclaimer: All claims expressed in this article are solely those of the authors and do not necessarily represent those of their affiliated organizations, or those of the publisher, the editors and the reviewers. Any product that may be evaluated in this article or claim that may be made by its manufacturer is not guaranteed or endorsed by the publisher.
Research integrity at Frontiers
Learn more about the work of our research integrity team to safeguard the quality of each article we publish.