- Laboratory of Analytical Chemistry, Department of Chemistry, National and Kapodistrian University of Athens, Athens, Greece
The packaging has proven to be a source of some serious food contaminants, with several chemicals migrating from the food contact material into the food. Therefore, efficient means of control of the migration extend and identification of the migrating substances must be established. The necessity of migration tests has been underlined by the European Union (EU) Regulation No. 10/2011, requiring the evaluation of the presence of intentionally and non-intentionally added substances. To facilitate this purpose, highly sensitive, multi-analyte methods are required. Targeting a wide range of volatile migrating compounds, we developed and validated a GC-EI-QqQ-MS and a GC-APCI-QTOF-MS method for the simultaneous determination of 131 and 126 food packaging substances, respectively. Even though the GC-EI-QqQ-MS method presented increased sensitivity for several of the investigated compounds, covering the existing EU requirements and specific migration limits (SMLs) for all targeted analytes; the complementary high-resolution method inherently enables the possibility of further utilization of the obtained raw data among others for retrospective analysis. The applicability of both methods was tested using 95% v/v aqueous ethanol food simulant, representative for the worst foreseeable conditions of intended use for many food contact material applications. Real food packaging samples belonging to different types of plastic materials were tested toward chemical migration, utilizing different migration protocols, depending on the sample characteristics. The favorable analytical features of both methods enable their use for the direct analysis of the investigated food simulant, overcoming the need for sample preparation. Thus, labor intensive and/or time-consuming pre-concentration procedures, which would furthermore restrict the applicability of the methods to certain groups of analytes and add to the uncertainty of the overall results, could be eliminated.
1. Introduction
The packaging has become an indispensable element in the food manufacturing process, as it protects the food, e.g., from light, foreign aroma compounds and microbial contaminations; improving the self-life and stability of products during storage. However, it has been found to represent a serious source of contamination itself through the migration of substances from the packaging into food. The identification and control of these substances is an issue that deserves great attention to assure food quality and consumer safety.
Many of the substances leaching into the food are intentionally added (IAS) to the packaging materials, in order to enhance their properties (Lahimer et al., 2017; Hahladakis et al., 2018). Nevertheless, non-intentionally added substances (NIAS) can also be present at the food packaging materials, with impurities, side or break-down products and process contaminants being the main sources of such compounds. When these substances find their way to the food, unexpected changes in its composition may occur (Muncke, 2009). Therefore, European regulations have been established, dictating the control of FCMs against chemical migration. In particular, European Regulation (EC) 1935/2004 (European Commission, 2004) underlines that any material or article intended to come into contact either directly or indirectly with food must be sufficiently inert to preclude substances from being transferred to food in quantities large enough to endanger human health; or to bring about unacceptable changes in the composition of the food or a deterioration in its organoleptic properties. Additionally, the European Commission (EC) has established with the European Union (EU) Regulation No. 10/2011 (European Commission, 2011) a positive list of more than 1,000 substances or mixtures that are allowed to be used as additives in plastic food contact materials (FCM), providing specific migration limits (SMLs) or other restrictions for their application. However, about half of these substances are not commercially available, and when it comes to their identification, only a limited number of analytical standard methods exist, focusing mainly on a limited number of substances (Silva et al., 2006). The restricted availability of validated multi-analyte methods can be attributed, among others, to the many existing chemical classes of IAS and NIAS, the lack of analytical standards and the fact that many of these substances are not included in chemical or spectral databases, with further issues being extensively discussed in a recent publication (Tsochatzis et al., 2020).
Concerning the analysis of migrant substances from plastic FCMs, several works have been published in the recent analytical literature, employing different hyphenated analytical techniques (Wrona and Nerín, 2020). Some of the most significant contributions in the field of FCMs are the determination of 41 food contact related contaminants in fatty food by HPLC-MS/MS (Vavrouš et al., 2019); the determination of 48 contaminant residues in by microwave-assisted extraction and UPLC-Q Orbitrap HRMS (Zhang et al., 2018) and the determination of 63 photoinitiators and amine synergists by QuEChERS and UPLC-MS/MS (Jung and Simat, 2014). Respectively, on the side of volatile substances, 60 migrant substances were simultaneously analyzed by vortex-assisted liquid–liquid extraction (VA-LLE) followed by GC-Q-Orbitrap HRMS (Miralles et al., 2021); while 84 migrants were determined by LLE-GC–MS (Tsochatzis et al., 2020) and 75 migrants by salt-assisted liquid–liquid extraction (SALLE)-GC–MS/MS (Tsochatzis et al., 2021).
With the wide range of substances potentially migrating from the FCM, the selection of a universal sample preparation procedure for the extraction and pre-concentration of all migrant species present at a FCM sample seems to be an almost unlikely task. Therefore, highly sensitive analytical techniques, in combination with wide-scope sample preparation protocols, need to be applied in the controls of food packaging materials. When the targeted analytes involve exclusively IAS, or some few NIAS compounds, such protocols can be established. However, there are always matrix effects and selectivity restrictions that reduce the applicability of such protocols to different groups of migrating substances. Particularly, when further NIAS compounds need to be introduced into such screening methods, problems related to the sample preparation need to be eliminated. Since these limitations cannot be easily overcome, studies on NIAS identification have so far been restricted to a small number of substances (Pack et al., 2021; Yusà et al., 2021) or have been based on non-target screening approaches (Sapozhnikova et al., 2021; Canellas et al., 2022; Tisler and Christensen, 2022). Therefore, the direct analysis of the food simulant appears to be the best practice, whenever applicable, for the development of wide-scope methodologies.
In order to enhance further the group of target IAS and NIAS migrants that can be analyzed simultaneously, we developed and validated a GC-EI-QqQ-MS (Gas Chromatography-Electron Ionization-Triple Quadrupole Mass Spectrometry) and a GC-APCI-QTOF-MS (Gas Chromatography-Atmospheric Pressure Chemical Ionization-Quadrupole-Time-of-Flight Mass Spectrometry) method for the direct analysis of 95% v/v aqueous ethanol extracts. This high organic content food simulant was selected in order to represent the worst-case scenario of migration for many FCM applications. Direct analysis of this simulant utilizing techniques of high sensitivity liberates us from the need for laborious and/or time-consuming sample preparation methods that would pose an additional source of uncertainty to the overall results.
2. Materials and equipment
2.1. Chemicals
For the applied food simulant (95% v/v aqueous ethanol) and solutions, ethanol of Chromasolv grade purity was purchased from Merck (Darmstadt, Germany), while Ultrapure water (18.2 MΩ) was obtained by a Milli-Q purification system (Millipore Direct-Q UV, Bedford, MA, United States). All the analytical standards were obtained either from Sigma-Aldrich (Steinheim, Germany) or from TCI Chemicals (Tokyo, Japan). All relevant information regarding the target substances are presented in Table 1, including FCM numbers extracted from the positive list of the EU Regulation No. 10/2011 (European Commission, 2011), CAS numbers, molecular formulas, purity (as stated by the supplier) and Cramer Class (provided by the toxtree software). To the group of IAS investigated, several NIAS were added, due to their previously established presence in migration experiments (e.g., as degradation products of certain substances intentionally added to FCMs) (Zhiqing et al., 2016; García Ibarra et al., 2019; Tsochatzis et al., 2020) and the associated interest in their assessment (Silano et al., 2019).
2.2. Preparation of standard solutions
For each analyte and internal standard (IS), stock solutions containing 10 mg mL−1 were prepared, using ethanol as solvent. Appropriate working solution mixtures were prepared by diluting the stock standard solutions with ethanol. All stock solutions were stored at −18°C, while working solutions were stored at 4°C and prepared on a weekly basis. In order to prevent any light-induced degradation or isomeric conversion of the substances, all standard solutions were prepared and stored in amber vials.
2.3. Analysis of real FCM samples
The applicability of the developed methods to real samples was tested using 6 FCM products provided by EU plastic producers. Before the analysis, all samples were stored in wrapped aluminum foil at room temperature (20 ± 5°C). The samples tested were general purpose FCM materials and the migration experiments were performed according to the type of each material, using the 95% ethanol simulant for the prediction of migration under a worst-case scenario. For the flexible film samples, pouches were prepared, using a 2 dm2 material surface in contact with 100 mL food simulant. For the bottles and trays, total immersion experiments were performed, using parts of the samples that were previously cut into rectangular pieces (approximately 0.2 dm2; 4 × 5 cm). The time and temperature conditions applied were in compliance with the Regulation (EU) No. 10/2011 (European Commission, 2011). All relevant information concerning the material types, the type of migration experiment, the amount of food simulant and the contact time and temperature, are presented in Table 2.
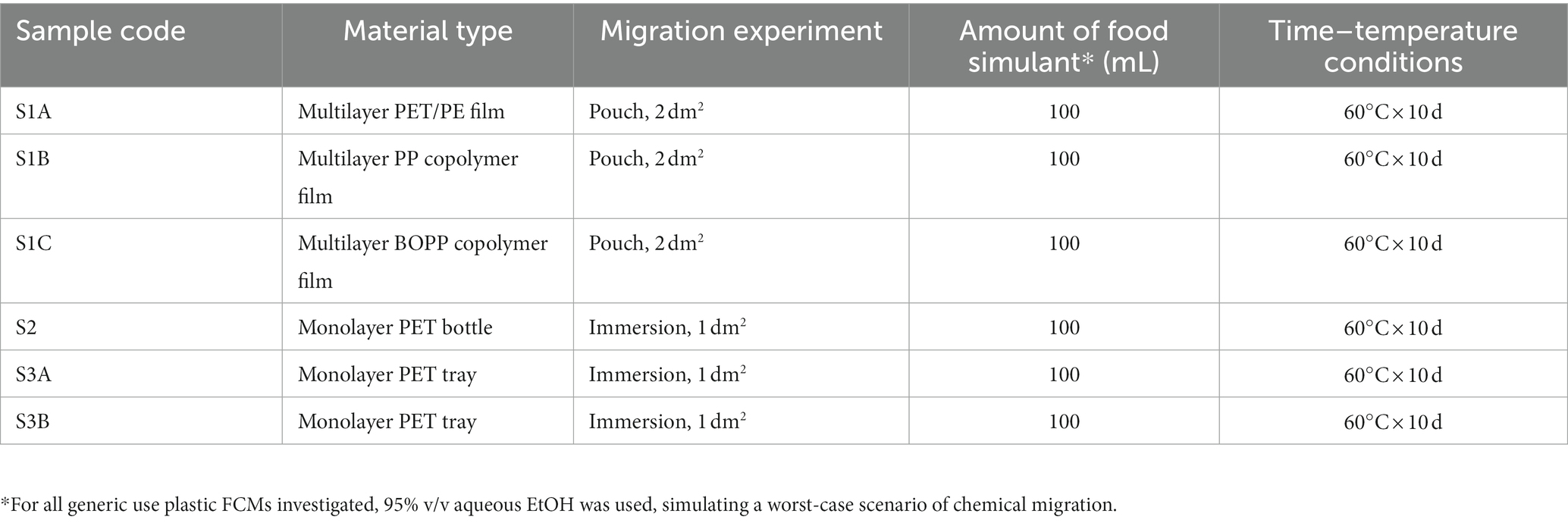
Table 2. Description of the analyzed FCM samples, type of migration experiment, the applied volume of food simulant (95% v/v aqueous EtOH), and the contact time and temperature conditions.
2.4. GC-EI-QqQ-MS
The GC-EI-QqQ-MS system used consisted of a Bruker 456 GC, a CTC PAL3 RSI Autosampler and a Triple Quadruple mass spectrometer (EVOQ-GC TQ, Bruker Daltonics) with an EI source. Single taper liners and a Restek Rxi-5Sil MS column of 30 m (0.25 mm i.d. × 0.25 μm film thickness) were used, with helium as carrier gas, at a constant flow rate of 1.5 mL min−1. The injection volume was 1 μL and pulsed pressure splitless injection was applied, in order to improve the sensitivity and reproducibility of the analysis of ethanolic extracts. The pulse pressure was set at 2.76 bar, with a pulse duration of 0.8 min, while the spitless purge valve was activated 1 min after injection. The GC oven was programmed as follow: 55°C initial hold for 3 min, increased at a rate of 15°C min−1 to 180°C, then increase with a step of 6.5°C min−1 to 280°C and hold for 5 min, followed by an increase of 10°C min−1 to 300°C and hold for 3 min. The injector port, transfer line and MS source temperature was maintained at 280, 290, and 250°C, respectively.
In order to be able to proceed with the method development for the target screening, all analytes investigated were incorporated into a database, containing all the required information, such as retention time, precursor ions, etc. The method development started analyzing small groups of the compounds in Full Scan mode and identifying their retention times and most abundant, as well as characteristic, fragment ions. Then, Product Scans were performed at different collision energies. After careful control of the provided data, the most abundant product ions for every compound and for every parent ion and collision energy were selected, creating the Multiple Reaction Monitoring (MRM) method. However, for some analytes with relatively small molecular weight, the additional fragmentation step was not applicable. Consequently, the Single Ion Monitoring (SIM) scan mode was also employed, where no MRMs could be established. Thus, the final method was a combination of SIM and MRM segments (Table 3). This method was able to simultaneously monitor 131 IAS and NIAS (Figure 1) at the low ppb level. Since, for 111 out of the 131 compounds investigated, characteristic MRMs were applied, for the majority of the analytes there was no requirement for peak resolution, reducing significantly the analysis time.
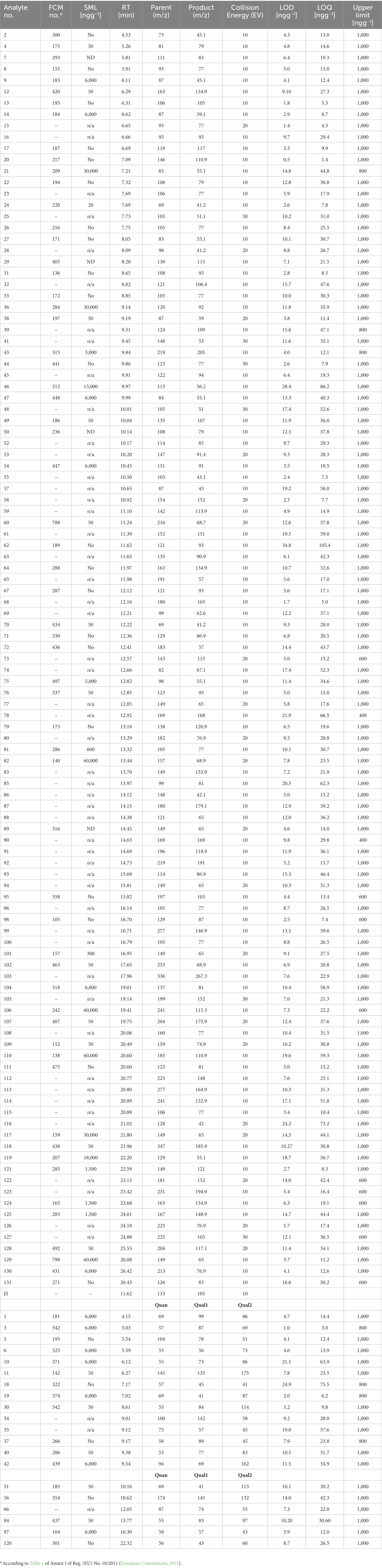
Table 3. Characteristic MS detection parameters, retention times and analytical features of the validated GC-EI-QqQ-MS method.
2.5. GC-APCI-QTOF-MS
The GC-APCI-QTOF system consisted of a Bruker 456 GC, a CP-8400 Autosampler and a hybrid quadrupole time of flight mass spectrometer (QTOF-MS) (Maxis Impact, Bruker Daltonics) with an APCI ion source. An equivalent analytical column and single taper liners were used, with pulsed pressure injection being again applied, following the same pressure, flow and temperature conditions as for the aforementioned GC-EI-QqQ-MS method.
The QTOF mass spectrometer was calibrated with Perfluorotributylamine (PFTBA or FC43) prior to each injection. The system was operating in broadband collision-induced dissociation (bbCiD) acquisition mode and recorded spectra over the range m/z 50–1,000 with a scan rate of 2 Hz. The Bruker bbCiD mode provides MS and MS/MS spectra at the same time, working at two different collision energies. At the low collision energy of 4 eV, MS spectra are acquired, with all of the ions from the pre-selected mass range passing through the flight tube without isolation at the quadrupole and without any collision-induced dissociation at the collision cell. At the high collision energy of 25 eV, isolation is taking place at the quadrupole, while the ions from the preselected mass range being fragmented at the collision cell. This is a Data Independent Acquisition (DIA) mode, resulting in better sensitivity, since more time is dedicated to every spectrum recorded, and is generally used for structure elucidation and retrospective analysis. However, during the database creation, the Data Dependent Acquisition (DDA) mode was also implemented for analysis of the standard mixture, recording MS/MS data that correspond only to the five most abundant masses. The application of DDA results in clearer spectra, due to sample matrix elimination, with the obtained spectra being used for compound identification and verification purposes, ensuring that specific fragments belong unambiguously to a particular analyte. Both MS and MS/MS spectra, in both DIA and DDA acquisition modes were recorded and utilized for the development of the equivalent positive HRMS database for the analytes of interest, while sample analysis was performed under DIA.
In contrast to the electron impact ionization, which is considered to be a “hard” ionization technique, APCI is a soft source that generally imparts less energy to an analyte molecule than the EI ionization, resulting in less fragmentation and usually a simpler spectrum, while it allows the determination of the molecular mass. The developed GC-APCI-QTOF-MS method was able to simultaneously monitor 126 IAS and NIAS (Table 4 and Figure 2), with only five from the targeted analytes not being successfully ionized under APCI conditions. However, the advantage of high-resolution enables again the determination of all analytes without the requirement for peak resolution, maintaining reduced analysis time.
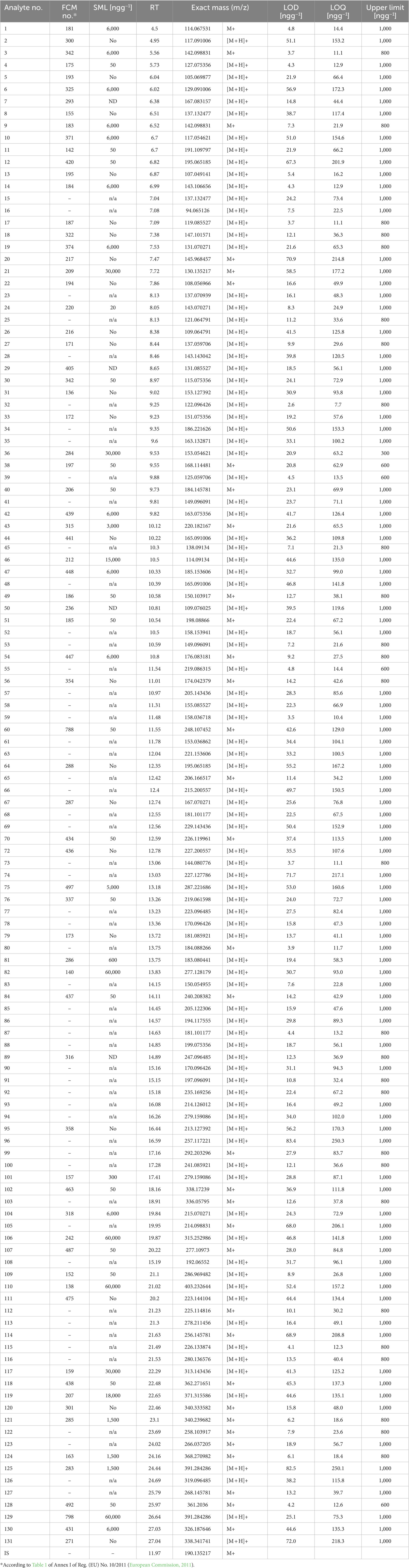
Table 4. Characteristic MS detection parameters, retention times and analytical features of the validated GC-APCI-QTOF-MS method.
3. Methods
Both methods developed were validated in terms of linearity, precision, trueness, limit of detection (LOD) and quantification (LOQ), taking into consideration the international guidelines for performance criteria and validation procedures (Thompson et al., 2002; Bratinova et al., 2009; European Commission, Directorate General for Health and Food Safety, 2017). The linearity for every analyte was assessed by analyzing standard solution mixtures at six concentration levels for each of the target analytes and calculating the linear regression coefficients (R2) and residuals plots. The linear regression coefficients were above 0.99 for all studied substances with the GC-EI-QqQ-MS/MS method, indicating a good linearity of the calibration curves. The same requirement was applied for the GC-APCI-QTOF-MS method, where 100 compounds had regression coefficients above 0.99 and only for 26 substances those values were above 0.98, with the residuals plots indicating no particular trend for the concentration ranges investigated. The upper linear limit was calculated by consecutive injections of standard mixtures, where signal suppression was observed due to high concentrations and overlapping of peaks. Even though the upper linear limit for many compounds can be considered high, no effects on linearity were identified.
The calibration curves were constructed with the ratio of the analyte peak area to the IS peak area. The performance of two different internal standards was studied, namely the 3-(4-Isopropylphenyl) isobutylaldehyde (IS1), which has also been used as IS in previous studies (Tsochatzis et al., 2020), and the deuterated diethyl phthalate-d4 (IS2). Starting with the GC-EI-QqQ-MS method, for each internal standard we constructed the corresponding calibration curve for every investigated analyte and noticed that the appropriate standard, improving the analyte’s correlation coefficient for 129 out of the 131 compounds investigated was the IS1. Diethyl phthalate-d4 proved to perform better only for 2,2′-methylene bis(4-ethyl-6-tert-butylphenol) (FCM 163) and 2,2′-methylene bis(4-methyl-6-tert-butylphenol) (FCM 185), with differences in the correlation coefficients that were statistically insignificant. Thus, only the IS1 was further used, and will be from now on referred to as IS. In addition, since no sample preparation steps are involved in the overall analytical procedure, the use of IS compensates for variations related to sample injection. Thus, the selection of additional standards, applied for particular groups of compounds, was not considered necessary.
The estimation of target analytes’ LODs and LOQs was based on the data from regression analysis, using the equations LOD = 3 × Sb/a, where Sb is the standard deviation of the intercept and a is the slope of the curves; and LOQ = 3 × LOD. The method’s LOQ had the additional requirement of covering the existing EU requirements and limits (SMLs). When the LOQ values were compared with the SMLs as listed in the Regulation, all LOQs were below the proposed SMLs for the GC-EI-QqQ-MS method. For the GC-APCI-QTOF-MS method, the LOQs of 13 substances were higher than the provided SMLs (Table 4). Thus, for quantification of these particular substances, namely FCM 142, 185, 197, 206, 220, 337, 342, 420, 438, 434, 463, 487, 788; with SMLs equal to 50 ng g−1, the GC-EI-QqQ-MS method should be applied. In addition, the use of the APCI source at the HRMS system prevented the fragmentation for 5 of the targeted analytes (FCM 104, 105, 189, 266, 330), reducing the applicability range of the method from 131 to 126 compounds.
Precision and trueness were assessed by analyzing fortified simulant (95% EtOH) with all the selected analytes at several mass fraction levels. For the GC-EI-QqQ-MS method the levels used were 100, 250, and 500 μg kg−1, while for the complementary GC-APCI-QTOF-MS method the 250, 400, and 800 μg kg−1 were selected. For intra-day precision or repeatability, six replicates of the 250 μg kg−1 sample were analyzed during 1 day, while for intermediate precision 3 replicates of all investigated levels were analyzed over two consecutive days. The trueness of the analytical method was based on the calculation of the recovery (%), expressed as the amount found in the fortified food simulant over the known amount added × 100. For the GC-EI-QqQ-MS method, reported results show good precision of the method, with RSDs for the repeatability and intermediate precision being lower that 20% for all studied substances. Recoveries for all studied substances were also between 70 and 120%. For the GC-APCI-QTOF-MS method, the recoveries of the analytes investigated were again between 70 and 120%. However, 86% of the analytes had RSDs below 20% for the repeatability measurements, with the remaining 14% ranging from 20.4 to 29.6%. Concerning the intermediate precision, 77% of the analytes had RSDs below 20%, while for 29 analytes the RSD values were between 20.1 and 37.3%.
4. Results
The applicability of the method was evaluated by studying six commercial FCM polymeric samples comprised of different materials (Table 2). It is important to mention that the FCM samples to be examined toward chemical migration should be tested before coming into contact with the respective food product. This ensures that any compounds detected (at the applied food simulant) originate from the packaging material itself and are not constituents of the packaged food. Removal of the food product and subsequent analysis of the packaging material cannot provide reliable results, since, food contaminations could remain at the food contact material and be falsely detected as migrating substances, while other actual migrating compounds may have already be transferred to the packaged food and consequently removed without being detected. Thus, testing commercially available packaged food products could significantly compromise the integrity of the results in terms of compound identification as well as migration rate and corresponding material inertness. Therefore, such evaluations should only be performed at original materials and not at final, commercially available products. However, original FCM samples are not easily accessible and obtaining them requires the collaboration with companies that either produce the materials themselves or use them for the packaging of their products. Such a co-operation is hard to establish, since the non-legislative nature of the EU regulation allows the companies to perform the bare minimum of migration tests necessary for compliance, avoiding the application of highly sensitive and quantitative methods for the identification of migrating substances. In addition, even when a FCM is provided for analysis, the recipe of the material is rarely disclosed, since either the manufacturer is not willing to share these information for reasons of confidentiality or competitiveness, or the retailer or collaborating food company may also not have access to them.
Even though finding original, intact samples of food contact materials is a difficult task, having performed an open call for co-operation through the national association of manufacturers of packaging materials, we managed to collect six representative samples of polymeric FCMs. The migration experiments were designed according to the type of each product, using the 95% EtOH food simulant, while keeping the samples for 10 days at 60°C. This time and temperature combination was selected, since it testing under these conditions shall cover long term storage above 6 months at room temperature and below including heating up to 70°C for up to 2 h, or heating up to 100°C for up to 15 min, according to the EU Regulation 10/2011. In particular, pouches were formed for the flexible films examined, while the tray and bottle samples undergo total immersion experiments. The results of the experiments in terms of detected substances and corresponding concentrations, are presented in Table 5. Two out of the six samples investigated did not release any of the method’s target analytes under the defined test conditions. These were the multilayer PP copolymer film and the monolayer PET bottle, with the other PET samples showing higher migration rates. In particular, low levels of Butyl acetate (FCM 300) processing aid were detected for sample S3A, while the antioxidant Butylated hydroxytoluene (FCM 315) and the UV inhibitor 4-Methoxyphenol were also detected at both PET trays. The presence of 4-Methoxyphenol, even though not included in the positive list of substances at the EU Regulation 10/2011, has already been reported in previous work (Lago and Ackerman, 2016) and was, thus, added to our list of target analytes. From the remaining FCMs, only sample S1A released caprolactam (FCM 177) monomer at a concentration level of 2.3 mg kg−1, as calculated with both applied methods. This concentration was more than six times lower than the SML provided for the particular substance and was calculated taking into consideration the area which was in contact with the food simulant and the standard surface-to-food mass ratio of 6 dm2 kg−1 food. Only traces of tri-n-butyl acetyl citrate (FCM 138) plasticizer were also detected in sample S1C, proving that all investigated copolymers were highly inert, according to the migration tests performed.
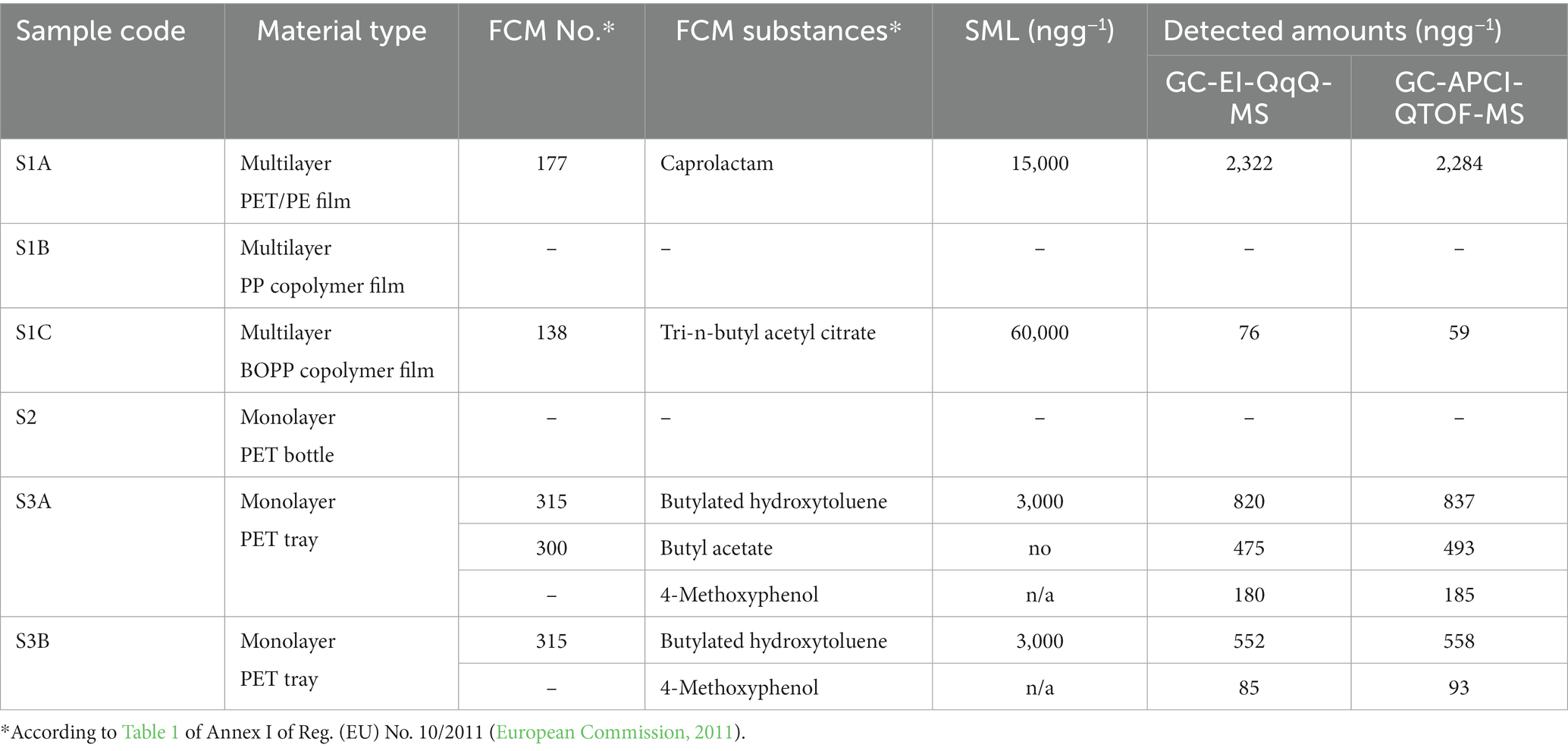
Table 5. Identification and quantification data for the substances migrating from the tested FCM plastic samples into food simulant 95% EtOH.
Overall, the analysis of real FCM samples showed that the migrated amounts of the regulated substances of all tested materials were compliant with the requirements in the corresponding Regulation, with only one NIAS compound, belonging to Cramer Class I being additionally detected at the low mg kg−1 range.
5. Discussion
In the frame of this study, two methods were developed and validated for the target screening of a large group of IAS and NIAS migrating from plastic food contact materials, covering all different types of virgin or recycled plastics. Both methods were used for the direct analysis of sample migrants, where the 95% EtOH food simulant was applied. The GC-EI-QqQ-MS method was used for the determination of more volatile analytes, with 131 compounds being simultaneously analyzed, over the 126 compounds being successfully identified and quantified by the complementary GC-APCI-QTOF-MS method. Additionally, the low-resolution method maintained the sensitivity advantage, while presenting wider linearity ranges for several of the targeted species. These features are mandatory for the analysis of substances with significantly different SMLs. In particular, the GC-EI-QqQ-MS method was able to reach the appropriate LOQs and cover the legislative limits for all investigated substances, without the requirement of any pre-concentration step. For the respective GC-APCI-QTOF-MS method, only for 13 out of the 126 investigated compounds the obtained LOQs were higher than the proposed SMLs. At the same time, both methods are still able to identify at the low ppb level targeted NIAS, for which such limits have not been yet established, but considering that some of them belong to Cramer Class III, low SMLs have to be expected. Furthermore, the proposed GC-APCI-QTOF-MS method provides the significant advantage for retrospective analysis. Building up target screening databases, this is an important property, allowing further compounds to be added to the controls as the list of analytes of interest is expanding with more and more NIAS compounds being detected.
When both methods were applied for the analysis of real FCM samples, the results obtained proved the efficiency of both methods. They also showed that all investigated samples, in their examined form, are highly inert toward the investigated group of substances. Additionally, the calculated concentrations of the detected migrants illustrated the complementarity of both methods, which could in most of the cases also be used interchangeably, since they provided comparable concentration rates for all detected analytes.
In conclusion, this is the first work addressing the simultaneous determination of over 130 volatile migrants of plastic FCMs, utilizing both low- and high-resolution MS systems. The direct, highly sensitive analysis of such a large group of EU regulated substances and NIAS compounds is of particular importance for both the competent authorities and control laboratories, since it would enable them to perform efficient screening on different instrumentations available and enhance the validity of safety statements for the food contact materials tested.
Data availability statement
The raw data supporting the conclusions of this article will be made available by the authors, without undue reservation.
Author contributions
CK and NT contributed to the conception and design of the study. NT provided the resources. CK performed the experiments and development of both analytical methods and wrote the first draft of the manuscript. CK and TT performed the data evaluation and statistical analysis. All authors contributed to manuscript revision, read, and approved the submitted version.
Funding
This study was partially funded by a Scholarship (scholarship number 2019–050–0503-18008), provided by the State Scholarships Foundation (IKY), within the framework of the project “Reinforcement of Postdoctoral Researchers—2nd Cycle” (MIS-5033021) of the operational program “Human Resource Development, Education and Lifelong Learning,” co-funded by Greece and the European Union (European Social Fund).
Acknowledgments
We would like to thank Emmanouil D. Tsochatzis for all the discussions and useful suggestions during the course of this study.
Conflict of interest
The authors declare that the research was conducted in the absence of any commercial or financial relationships that could be construed as a potential conflict of interest.
Publisher’s note
All claims expressed in this article are solely those of the authors and do not necessarily represent those of their affiliated organizations, or those of the publisher, the editors and the reviewers. Any product that may be evaluated in this article, or claim that may be made by its manufacturer, is not guaranteed or endorsed by the publisher.
References
Bratinova, S., Raffael, B., and Simoneau, C. (2009). Guidelines for performance criteria and validation procedures of analytical methods used in controls of food contact materials. EUR 24105 EN. Luxembourg: Publications Office of the European Union, JRC53034.
Canellas, E., Vera, P., Nerin, C., Dreolin, N., and Goshawk, J. (2022). The detection and elucidation of oligomers migrating from biodegradable multilayer teacups using liquid chromatography coupled to ion mobility time-of-flight mass spectrometry and gas chromatography-mass spectrometry. Food Chem. 374:131777. doi: 10.1016/j.foodchem.2021.131777
European Commission, Council of the European Union. (2004). Regulation (EC) no 1935/2004 of the European Parliament and of the council of 27 October 2004 on materials and articles intended to come into contact with food and repealing Directives 80/590/EEC and 89/109/EEC. OJ L 338, 4–17.
European Commission. (2011). Commission regulation (EU) no 10/2011 of 14 January 2011 on plastic materials and articles intended to come into contact with food. OJ L 12, 1–89.
European Commission, Directorate General for Health and Food Safety (DG SANTE). (2017). SANTE/11813/2017, Guidance document on analytical quality control and method validation procedures for pesticide residues analysis in food and feed.
García Ibarra, V., Bernaldo, R., de Quirós, A., Paseiro Losada, P., and Sendón, R. (2019). Non-target analysis of intentionally and non-intentionally added substances from plastic packaging materials and their migration into food simulants. Food Packag. Shelf Life 21:100325. doi: 10.1016/j.fpsl.2019.100325
Hahladakis, J. N., Velis, C. A., Weber, R., Iacovidou, E., and Purnell, P. (2018). An overview of chemical additives present in plastics: migration, release, fate and environmental impact during their use, disposal and recycling. J. Hazard. Mater. 344, 179–199. doi: 10.1016/j.jhazmat.2017.10.014
Jung, T., and Simat, T. J. (2014). Multi-analyte methods for the detection of photoinitiators and amine synergists in food contact materials and foodstuffs – part II: UHPLC-MS/ MS analysis of materials and dry foods. Food Addit. Contam. A 31, 743–766. doi: 10.1080/19440049.2014.882519
Lago, M. A., and Ackerman, L. K. (2016). Identification of print-related contaminants in food packaging. Food Addit. Contam. A 33, 518–529. doi: 10.1080/19440049.2015.1136435
Lahimer, M. C., Ayed, N., Horriche, J., and Belgaied, S. (2017). Characterization of plastic packaging additives: food contact, stability and toxicity. Arab. J. Chem. 10, S1938–S1954. doi: 10.1016/j.arabjc.2013.07.022
Miralles, P., Yusà, V., Sanchís, Y., and Coscollà, C. (2021). Determination of 60 migrant substances in plastic food contact materials by vortex-assisted liquid-liquid extraction and GC-Q-Orbitrap HRMS. Molecules 26:7640. doi: 10.3390/molecules26247640
Muncke, J. (2009). Exposure to endocrine disrupting compounds via the food chain: is packaging a relevant source? Sci. Total Environ. 407, 4549–4559. doi: 10.1016/j.scitotenv.2009.05.006
Pack, E. C., Lee, K. Y., Jung, J. S., Jang, D. Y., Kim, H. S., Koo, Y. J., et al. (2021). Determination of the migration of plastic additives and non-intentionally added substances into food simulants and the assessment of health risks from convenience food packaging. Food Packag. Shelf Life 30:100736. doi: 10.1016/j.fpsl.2021.100736
Sapozhnikova, Y., Nuñez, A., and Johnston, J. (2021). Screening of chemicals migrating from plastic food contact materials for oven and microwave applications by liquid and gas chromatography—orbitrap mass spectrometry. J. Chromatogr. 1651:462261. doi: 10.1016/j.chroma.2021.462261
Silano, V., Barat Baviera, J. M., Bolognesi, C., Chesson, A., Cocconcelli, P. S., Crebelli, R., et al. (2019). Update of the risk assessment of di-butylphthalate (DBP), butyl-benzyl-phthalate (BBP), bis(2- ethylhexyl)phthalate (DEHP), di-isononylphthalate (DINP) and diisodecylphthalate (DIDP) for use in food contactmaterials. EFSA J. 17:5838. doi: 10.2903/j.efsa.2019.5838
Silva, A. S., Sendón García, R., Cooper, I., Franz, R., and Paseiro Losada, P. (2006). Compilation of analytical methods and guidelines for the determination of selected model migrants from plastic packaging. Trends Food Sci. Technol. 17, 535–546. doi: 10.1016/j.tifs.2006.04.009
Thompson, M., Ellison, S. L. R., and Wood, R. (2002). Harmonized guidelines for single laboratory validation of methods of analysis. Pure Appl. Chem. 74, 835–855. doi: 10.1351/pac200274050835
Tisler, S., and Christensen, J. H. (2022). Non-target screening for the identification of migrating compounds from reusable plastic bottles into drinking water. J. Hazard. Mater. 429:128331. doi: 10.1016/j.jhazmat.2022.128331
Tsochatzis, E. D., Alberto Lopes, J., Hoekstra, E., and Emons, H. (2020). Development and validation of a multi-analyte GC-MS method for the determination of 84 substances from plastic food contact materials. Anal. Bioanal. Chem. 412, 5419–5434. doi: 10.1007/s00216-020-02758-7
Tsochatzis, E. D., Lopes, J. A., Gika, H., Dalsgaard, T. K., and Theodoridis, G. (2021). A fast SALLE GC-MS/MS multi-analyte method for the determination of 75 food packaging substances in food simulants. Food Chem. 361:129998. doi: 10.1016/j.foodchem.2021.129998
Vavrouš, A., Ševčík, V., Dvořáková, M., Čabala, R., Moulisová, A., and Vrbík, K. (2019). Easy and inexpensive method for multiclass analysis of 41 food contact related contaminants in fatty food by liquid chromatography-tandem mass spectrometry. J. Agric. Food Chem. 67, 10968–10976. doi: 10.1021/acs.jafc.9b02544
Wrona, M., and Nerín, C. (2020). Analytical approaches for analysis of safety of modern food packaging: a review. Molecules 25:752. doi: 10.3390/molecules25030752
Yusà, V., López, A., Dualde, P., Pardo, O., Fochi, I., Miralles, P., et al. (2021). Identification of 24 unknown substances (NIAS/IAS) from food contact polycarbonate by LC-orbitrap tribrid HRMS-DDMS3: safety assessment. Int. J. Anal. Chem. 2021, 1–13. doi: 10.1155/2021/6654611
Zhang, X., Zhang, P., Shi, C., Hua, H., Rong, Y., Lu, J., et al. (2018). Rapid determination of 48 contaminant residues in food contact plastic products by ultra performance liquid chromatography-quadrupole/electrostatic field orbitrap high resolution mass spectrometry coupled with microwave-assisted extraction. Chin. J. Chromatogr. 36, 634–642. doi: 10.3724/SP.J.1123.2018.01047
Keywords: food contact materials, IAS, NIAS, GC-EI-QqQ-MS, GC-APCI-QTOF-MS
Citation: Kanakaki C, Traka T and Thomaidis NS (2023) Development and validation of multi-analyte methods for the determination of migrating substances from plastic food contact materials by GC-EI-QqQ-MS and GC-APCI-QTOF-MS. Front. Sustain. Food Syst. 7:1159002. doi: 10.3389/fsufs.2023.1159002
Edited by:
Milena Corredig, Aarhus University, DenmarkReviewed by:
Fátima Poças, Escola Superior de Biotecnologia - Universidade Católica Portuguesa, PortugalOlivier Vitrac, INRA Centre Versailles-Grignon, France
Copyright © 2023 Kanakaki, Traka and Thomaidis. This is an open-access article distributed under the terms of the Creative Commons Attribution License (CC BY). The use, distribution or reproduction in other forums is permitted, provided the original author(s) and the copyright owner(s) are credited and that the original publication in this journal is cited, in accordance with accepted academic practice. No use, distribution or reproduction is permitted which does not comply with these terms.
*Correspondence: Chrysoula Kanakaki, Y2thbmFrYWtpQGNoZW0udW9hLmdy; Nikolaos S. Thomaidis, bnRob0BjaGVtLnVvYS5ncg==