- 1Research Unit Sustainability and Climate Policy, Leipzig, Germany
- 2Faculty of Agricultural and Environmental Sciences, University of Rostock, Rostock, Germany
- 3Faculty of Law and Interdisciplinary Faculty, University of Rostock, Rostock, Germany
The Farm to Fork Strategy of the EU aims at sustainable food systems. One objective of the Strategy is to reduce nutrient losses by at least 50% resulting in at least 20% less fertilizer use by 2030. To this end, Member States are expected to extend digital precision fertilization and sustainable agricultural practices through the Common Agricultural Policy. In this context, this article applies a qualitative governance analysis which aims to assess the extent to which the measures proposed by the Farm to Fork Strategy, i.e., digital precision fertilization and sustainable agricultural practices, contribute to the nutrient objective of the Farm to Fork Strategy. The article analyses how these measures are implemented through the Common Agricultural Policy in Germany and Saxony. Results show that the nutrient objective of the Farm to Fork Strategy itself offers shortcomings. Germany offers some, yet overall limited, support for sustainable agricultural practices and digital precision fertilization. Hence, the Common Agricultural Policy will to a limited extend only contribute to the objective of the Strategy. The results furthermore highlight some general shortcomings of digitalization as sustainability strategy in the agricultural sector including typical governance issues (rebound and enforcement problems), and point to the advantages of quantity-based policy instruments.
1. Introduction
Climate change and biodiversity loss put pressure on the agricultural sector. This pressure has recently increased due to rising resource and food prices and the war in the Ukraine (Ekardt and Rath, 2022; European Commission, 2022a). In fact, food security has moved to the top of the political agenda as grain exports from the Ukraine have basically come to a halt and fertilizer prices skyrocketed (The World Bank, 2022). To combat these issues, policy instruments are urgently needed which transition the agricultural sector away not only from fossil fuel dependency and intensive livestock farming but also from unsustainable nutrient management. Thereby, unsustainable nutrient management is connected with both, fossil fuel dependency and intensive livestock farming. Nitrogen (N) fertilizer production requires substantial amounts of fossil fuels (International Energy Agency, 2021). To a smaller extend, this also applies for Phosphorus (P) fertilizer production from phosphate rock as well as for recycled P fertilizers (Garske, 2019; Jupp et al., 2021). Furthermore, environmentally-damaging P and N hotspots frequently occur in regions with intensive livestock farming (Rothwell et al., 2020; Withers et al., 2020). To overcome these challenges, for decades, calls have been made to fundamentally transform agricultural practices to become sustainable (e.g., Carson, 1962). In the recent past, these calls have been supplemented by great hopes in digital innovations (Ekardt, forthcoming; Lowry et al., 2019; Zhang et al., 2021; Ekardt and Rath, 2022; Maffezzoli et al., 2022). Digital innovations are expected to contribute to environmental protection by increasing resource and energy efficiency for example through digital precision fertilization. In doing so, digital innovations are expected to enable resource-extensive growth that combines economic and environmental aspirations (Balafoutis et al., 2017, p. 7–9; Rose and Chilvers, 2018; Lowry et al., 2019; Ekardt, 2020, chap. 1.4; Garske and Ekardt, 2021; Garske et al., 2021).
In this context, the EU Commission adopted the Farm to Fork Strategy. The Farm to Fork Strategy is one element of the European Green Deal (European Commission, 2020b). Both initiatives establish the framework for a sustainable transition across all sectors including the agricultural sector in the EU. They are legally non-binding Communications and expected to contribute to the goals of the Paris Agreement and the Convention on Biological Diversity. The Farm to Fork Strategy aims at food security, sustainable food value chains and sustainable nutrient management (European Commission, 2020a). It addresses environmental pollution caused by unsustainable nutrient management—in particular by N and P: The Strategy aims to reduce nutrient losses by at least 50%, while ensuring soil fertility, and reducing fertilizer use by at least 20% by 2030. To achieve this objective, the EU Commission aims at digital approaches, i.e., extending the application of digital precision fertilization, and sustainable agricultural practices. They are to be implemented through the Common Agricultural Policy (CAP) (European Commission, 2020a, p. 7–8).
Earlier research on the Farm to Fork Strategy provided comprehensive assessments (Schebesta and Candel, 2020), economic implications (Wesseler, 2022), (potential) impacts on the organic farming sector (Moschitz, 2021; Purnhagen et al., 2021), sustainable food consumption (König and Araújo-Soares, 2021) and animal welfare (Molitorisová and Burke, 2022). Others modeled the impact of the Strategy on input use including fertilizer input reduction in the EU (Beckman et al., 2022) and Italy (Cortignani et al., 2022). Input reduction is also discussed in light of its effects on food (in)security (Baquedano et al., 2022). Digital innovations are also analyzed (Reinhardt, 2022). Regarding the CAP, one study broadly investigates the Strategy's implementation through the CAP (Schebesta and Candel, 2020; Wesseler, 2022) while another discusses the implementation of the Strategy through the lens of policy integration in the second pillar of the CAP in Germany (Bazzan et al., 2022). Against this backdrop, this article aims to assess the extent to which the measures of the Farm to Fork Strategy, namely digital precision fertilization and sustainable agricultural practices, contribute to its nutrient objective, i.e., fertilizer input reduction and nutrient loss reduction. To this end, the analysis focuses on how these measures are implemented through the CAP in Germany. Saxony will serve as example for the second pillar of the CAP which is regulated at state level. In doing so, the present article extends the current research and offers new perspective by adopting an interdisciplinary governance analysis on sustainable nutrient management.
The following section describes the qualitative governance analysis. The section thereafter introduces digital precision fertilization and its potential to reduce fertilizer input and nutrient losses into the environment. We will not discuss sustainable agricultural practices and their potential to reduce fertilizer input and nutrient losses into the environment as we have extensively covered this topic in earlier publications (Garske, 2019; Stubenrauch, 2019). An overview of CAP measures which support the application of digital precision fertilization and measures which support sustainable agricultural practices in Germany follows. The discussion critically reviews the CAP measures in relation to the nutrient objective of the Farm to Fork Strategy and analyses this objective in the context of the goals of the Paris Agreement and the Convention on Biological Diversity. Conclusions follow.
2. Materials and methods
This article applies a qualitative governance analysis which aims to identify effective policy instruments to achieve a policy goal. The present qualitative governance analysis assesses policy instruments against the legally binding goals of the Paris Agreement and the Convention on Biological Diversity. The Paris Agreement aims to keep global warming “well below 2 °C above pre-industrial levels” and “pursuing efforts to limit the temperature increase to 1.5 °C above pre-industrial levels” [Art. 2(1) Paris Agreement]. The Kunming-Montreal Global biodiversity framework aims at the maintenance and restoration of global ecosystems, and halting human induced extinction by 2050 (Goal A). The Convention on Biological Diversity aims to stop global biodiversity loss (Aichi Target B and C). Achieving these goals requires comprehensive transitions in the agricultural sector including in nutrient management which is, as indicated before, associated with various environmental issues such as eutrophication of water bodies and N2O emissions from agricultural soils (Tubiello et al., 2013; European Commission, 2021; Nessel et al., 2021; Refsnider et al., 2021). In turn, aligning the agricultural sector and nutrient management with the goals of the Paris Agreement and the Convention on Biological Diversity will limit manure for fertilization due to reduced livestock numbers, demand stopping fossil-based N fertilizer production and carefully using rock phosphate—being a scarce resource and primarily sourced for fertilizers (Garske, 2019; Stubenrauch, 2019; European Commission, 2020c, p. 80).
In this context, the qualitative governance analysis builds on findings from behavioral studies which shed light on typical governance problems such as shifting and rebound effects, enforcement and depicting problems, and lacking target stringency (Garske, 2019, p. 69–75; Ekardt, 2020, chap. 4.4; depicting problems in Smith et al., 2020; shifting effects in Jansson et al., 2020). Besides that, the qualitative governance analysis builds on findings from natural sciences. For the purpose of this article, we use our findings of earlier work on sustainable P management (Garske, 2019; Stubenrauch, 2019). In addition, we reviewed literature on digital precision fertilization. The aim of the literature review was to (1) provide an overview of digital precision fertilization and (2) understand their fertilizer savings potentials. As regards the second aim, we were looking for studies which assess the fertilizer reduction potential of digital precision fertilization in Germany. However, a first literature screening revealed that there were little to no studies. Therefore, we extended our search scope to include studies outside Germany. Google scholar served as starting point for the literature search. We used the search terms “precision agriculture, Germany”; “Präzisionslandwirtschaft, Düngung”; “precision agriculture, fertilization”; “precision agriculture, phosphorus” and “digitalization, agriculture, Germany”. We screened the first 100 results of each search. Articles were included into our databank that either investigate the effects of precision agriculture with or without a focus on fertilization and soils in Germany or elsewhere as well as studies which provide an overview over precision farming techniques. We focused on peer-reviewed articles and book chapters since 2000. Going through the articles and book chapters, we frequently sourced their bibliography for further literature (“snowballing”). The results of the literature review are provided in Section 3.
The qualitative governance furthermore includes an analysis of legal acts and jurisprudence and their impact as well as opportunities for optimization. For this qualitative governance analysis, we build on earlier work on the reformed CAP (Heyl et al., 2021). In short, the CAP is the major agricultural policy of the EU and includes a comprehensive subsidy scheme which has been reformed multiple times during its six-decade long existence. In 2018, the most recent reform started and is currently in its final steps. The CAP will continue to consist of two pillars. The first pillar covers market management and income support. The hectare-based income support for farmers is conditional on compliance with the requirements of conditionality. Approximately one quarter of the first pillar budget is allocated to voluntary eco-schemes. The second pillar covers multi-year rural development programs. In February 2022, Germany submitted its Strategic Plan to the Commission which establishes the national implementation of the EU provisions (Federal Ministry of Food Agriculture, 2022). The EU Commission assessed the Strategic Plan and in May 2022, published its observation letter. In November 2022, the Commission approved the Strategic Plan (European Commission, 2022b).
This qualitative governance includes the following legal documents of the CAP (Table 1).
At the EU level, the CAP Strategic Plan Regulation (EU) 2021/2115 (CAPSpR)1 establishes the framework for both pillars of the CAP. In Germany, the first pillar of the CAP is regulated at the federal level. The national CAP laws (“Gesetze”) of the first pillar were adopted by the German Parliament in summer 2021. The GAPDZG establishes the overall framework for the direct payments (income support) and the eco-schemes. The GAPKondG contains the rules on the standards for good agricultural and environmental condition of land which form one element of conditionality. The national CAP regulations (“Verordnungen”) were adopted by the Federal Council in winter 2021. These regulations contain details for the implementation of the direct payments (GAPDZV) and conditionality (GAPKondV). The legal framework for second pillar implementation is established in the GAKG. The GAKG establishes that the second pillar is regulated at the state level. Saxony will serve as example for the present analysis. However, as the legislative process has not been completed, the analysis builds on draft fact sheets for investment and environmental support measures of the Saxon State Ministry for Energy, Climate Protection, Environment and Agriculture (https://www.smekul.sachsen.de/foerderung/entwurfsdokumente-zum-gap-strategieplan-11,798.html).
3. Overview and potentials of digital precision fertilization
Precision farming is based on processing detailed data to monitor and optimize agricultural production (Schrijver, 2016; Nair et al., 2021). Data can be provided by remote sensing applications. Remote sensing describes non-contact measurement of different parameters. Various sensor platforms can be used. For example, platforms include satellites and drones as well as tractors and hand-held instruments whereby the latter is sometimes called proximate sensing (Mulla, 2013; Segarra et al., 2020). These optical methods are based on measuring radiation after interacting, e.g., reflectance and absorption, with soils and plants. For precision farming in general, remote sensing combined with artificial intelligence, e.g., image recognition, can be used to identify farmland parcels (Dong et al., 2018). For fertilizer management in particular, data on soil and plant properties is needed (e.g., Laskar and Mukherjee, 2016; Ahmadi et al., 2021). There, remote sensing in combination with modeling or artificial intelligence is used to e.g., predict plant available P in soils thus enabling site specific fertilizer management (Dong et al., 2018; Erler et al., 2020, p. 10). However, some common approaches are not effective in sensing (plant available) P in soils such as near-infrared sensing (Kuang et al., 2012, p. 168–169; Soriano-Disla et al., 2014, p. 163; Laskar and Mukherjee, 2016; Leenen et al., 2022). Still, plant available P can indirectly be measured by investigating other soil properties, such as organic carbon, which correlate with plant available P. However, more research is needed to better understand these relationships (Stenberg et al., 2010, chap. 2.4; Kuang et al., 2012, p. 160–162, 197–198; Pätzold et al., 2020, p. 738–739). Yet overall, these approaches avoid labor and time-intensive conventional soil testing and consecutive analysis in laboratories (Bogrekci and Lee, 2005; Laskar and Mukherjee, 2016).
An application of optical and electrochemical sensing is the identification of crop nutrient status and fertilizer demand. For example, optical proximate sensors are used for leaf analysis to capture foliar P concentrations (Dao, 2017). Another approach is the calculation of vegetation indices (Xue and Su, 2017; Segarra et al., 2020, p. 5–7; Sishodia et al., 2020, p. 8–10; Oberti and Schmilovitch, 2021, p. 120–121). One index to estimate plant nutrient status is the normalized difference vegetation index. The index highlights differences in reflectance of crops on a parcel: Healthy vegetation reflects more radiation in the infrared spectrum compared to unhealthy vegetation. Algorithms convert the vegetation index into fertilizer application rates. In doing so, nutrient use efficiency can be increased and nutrient losses into the environment reduced (Maes and Steppe, 2019, p. 157–158; Sishodia et al., 2020, p. 8–10, 14–15; Rovira-Más and Saiz-Rubio, 2021). However, depending on the nutrient, the predictability of different indices varies (Mahajan et al., 2014, 2017). Another approach is the application of electrochemical sensing to measure nutrients in plants and soils. These sensors capture an electrical signal that results from a chemical reaction with the substance under investigation. The electrical signal offers insights about this substance (Kim et al., 2009; Kuang et al., 2012, p. 186–189; Nadporozhskaya et al., 2022). Overall, these methods provide valuable data to optimize nutrient management.
Overall, digital precision fertilization can reduce pressure on the environment by e.g., reducing nutrient losses into water bodies. A review discusses the effects of sensor-based applications for site-adapted N management on yield, N use efficiency, profitability and environmental protection. Reviewed studies show that N input can be reduced between 2 and 82%. However, in these studies, the effects of sensor-based applications are compared to different parameters, e.g., farmer application, models, regional fertilizer recommendation, which distorts the results including the relative benefit of sensor-based applications (Colaço and Bramley, 2018). Besides that, models for precision P management have been developed. For example, one model aims to optimize P application based on P soil reserves. The model integrates the links between soil P reserves and fertilization, and soil P reserves and P losses into waterbodies as well as economic and ecological factors. Results show that P input into water bodies could be reduced by 20–31% in different locations (Iho and Laukkanen, 2012). Lastly, research on machine learning develops algorithms which enable site-adapted management of land, including fertilization, by considering many environmental and managerial factors. An experiment finds that one algorithm was able to reduce N input by about one quarter after five learning years (Saikai et al., 2020). Experiments, models and algorithms highlight that nutrient losses into the environment can be reduced through digital precision fertilization. However, the scope of these benefits may be distorted.
Site-adapted (or variable-rate) fertilization aims to optimize plant nutrient supply. To this end, fully automated, partly automated or non-automated technologies are used (Essl et al., 2021, p. 48). Partly automated or non-automated technologies enable precision fertilization based on prescription maps (sometimes called application maps). Partly automated technologies process these maps directly in bord computers of tractors (ibid.; Balafoutis et al., 2017, p. 7–8). Prescription maps are created based on data from remote sensing, soil maps or soil samples. These maps contain information about how much fertilizer has to be applied in a specific location (Yang et al., 2016, p. 182–185). When prescription maps are based on soil samples, nutrient demand is approximated by interpolation or artificial intelligence as soil sampling cannot be done in every location of a parcel. For example, machine learning methods process large amounts of e.g., spatial data from remote sensing to estimate nutrient demand of crops in a specific location (European Commission, 2018, p. 10; Singh et al., 2020; Radočaj et al., 2022). Then, forward speed of a tractor or the valve position is adjusted according to the fertilizer amount specified by the prescription map. This approach works for mineral fertilizers as well as farm manure and slurry (Grisso et al., 2011; Calcante et al., 2015). Fully automated technologies do not require prescription maps but use real-time sensors to collect and process data in situ (Yang et al., 2016, p. 180–186; Balafoutis et al., 2017, p. 7). Tractors as well as robots can be used for fertilizer application (Fountas et al., 2020, p. 10–11). A study on fully automated technologies, i.e., sensor-based variable-rate fertilization, found an 8% reduction in the total amount of N that was applied on the fields compared to a constant fertilizer rate chosen by farmers, and approximately 25% less N losses into the environment (Scharf et al., 2011). Application of site-adapted fertilization is diverse and as such can principally offer suitable solutions for many farmers.
Overall, studies show that digital precision fertilization can increase resource efficiency through reduced fertilizer input and less nutrient losses into the environment. In doing so, digital precision fertilization can generally contribute to the nutrient objective of the Farm to Fork Strategy. Building on these insights, the following section discusses the national implementation of the CAP in Germany and the extent to which measures support digital precision fertilization and sustainable agricultural practices.
4. Digital precision fertilization in Germany's CAP implementation
The Farm to Fork Strategy lists multiple digital applications to achieve the nutrient objective. Relevant CAP instruments include the Farm Sustainability Tool for Nutrients, investments, advisory services and EU space technologies (European Commission, 2020a, p. 7–8). Access to fast broadband internet is a prerequisite for (extending) the application of precision farming including digital precision fertilization which is expected to reduce fertilizer use (ibid., p. 16). In Germany, these measures are implemented through the second pillar of the CAP. This section firstly discusses Saxony's support for investments and secondly support for knowledge transfer and farm advisory services, both of which can directly and indirectly support digital precision fertilization.
4.1. Precision fertilization: Investment support
EU Member States have to provide investment support under the second pillar of the CAP [Art. 69 lit. (d) CAPSpR]. Saxony offers investment support for tangible and intangible assets that contribute to climate and environmental protection. For example, support is provided for investments into assets which reduce nitrate pollution in water bodies. This includes support for livestock farming and crop production such as environmentally-friendly manure storage, machinery for resource-saving manure application, and site-adapted fertilization. Besides that, support is provided for digitalizing business processes such as creating digital prescription maps which are a basis for site-adapted fertilization (Section 3) (Saxon State Ministry for Energy, 2022b, p. 7–8). These instruments can increase nutrient use efficiency by e.g., reducing fertilizer input and targeted fertilizer application. A reduction in nutrient hotspots which is caused by intensive livestock farming could furthermore be achieved by requiring recipients to have a livestock density of less than two livestock units per hectare (ibid.). This also benefits nutrient management, i.e., to better closed nutrient cycles.
Saxony furthermore provides support for investments which increase farm competitiveness in particular through modernization. This includes support for purchasing digitally supported machinery to e.g., mechanically manage weeds, for purchasing drones to apply insects for pest control, and for digitalizing business processes (ibid., p. 9–10). While these measures do not directly target precision fertilization, they aim at pushing precision farming more broadly. At last, to enhance biodiversity, Saxony provides support for machinery and equipment for conservation management and maintenance (ibid., p. 17) which can indirectly contribute to sustainable nutrient management by e.g., enhancing soil biodiversity.
4.2. Precision fertilization: Knowledge exchange and advisory services
Support for advisory services and knowledge transfer can directly and indirectly push digital precision fertilization (and farming). Member States of the EU are required to offer farm advisory services which include up-to-date technological information. Assistance must be offered for innovative practices and agricultural techniques for resilience to climate change [Art. 15(2) CAPSpR]. Farm advisory services have to cover digital technologies in agriculture and sustainable management of nutrients, including the use of a digital Farm Sustainability Tool for Nutrients from 2024 onwards [Art. 15(4) lit. (f), (g) CAPSpR]. The tool has to provide a balance of the main nutrients at field scale, legal requirements on nutrients, soil data, and data from the integrated administration and control system relevant for nutrient management [Art. 15(4) lit. (g) CAPSpR]. This tool could serve as a basis for digital precision fertilization (Section 3). However, the Farm Sustainability Tool for Nutrients is currently neither covered explicitly in Germany's CAP Strategic Plan nor in the second pillar measures of Saxony.
Member States furthermore have to provide support for knowledge exchange and dissemination of information [Art. 69 lit. (h) CAPSpR]. Saxony provides support for conferences, events, trainings, workshops and working groups, expert excursions and demonstration projects which cover land management and measures to minimize substance discharge in priority areas of the Water Framework Directive 2000/60/EC, to minimize fertilizer input and to reduce soil erosion and promote soil protection in general (Saxon State Ministry for Energy, 2022b, p. 30). These events could contribute to expand the application of digital precision fertilization in Saxony.
Overall, Saxony's second pillar measures support digital precision fertilization. However, while some measures directly address site-adapted fertilization, most programs broadly aim at farm modernization and innovation. Besides, the extent to which support for advisory services and knowledge transfer will address digital precision fertilization will ultimately depend on the actual events that will receive funding.
5. Sustainable agricultural practices in Germany's CAP implementation
Alongside digital precision fertilization, the Farm to Fork Strategy considers sustainable agricultural practices for achieving the nutrient objective. Both pillars of the CAP support sustainable agricultural practices. First pillar measures include the requirements of conditionality, which is made up of statutory management requirements (SMRs) and standards of good agricultural and environmental conditions of land (GAEC standards). Besides, some eco-schemes support sustainable agricultural practices which are beneficial for the nutrient objective of the Farm to Fork Strategy. Second pillar measures include in particular agri-environment-climate commitments.
The requirements of conditionality impact nutrient management directly and indirectly. Table 2 provides an overview of nutrient relevant requirements of conditionality and their effects on nutrient management. Some requirements such as SMR 1, SMR 2 and GAEC 4 directly address P and N management while other requirements cover sustainable soil management which have an indirect impact on nutrient management, e.g., minimum soil cover (GAEC 6) and crop rotation (GAEC 7). These requirements can principally reduce fertilizer demand, limit nutrient losses into the environment, and improve monitoring of nutrient management.
Eco-schemes are a new CAP instrument, some of which impact nutrient management. In general, support is provided for annual voluntary agricultural practices that are beneficial for climate, environment and animal welfare (Art. 18 GAPDZG). These practices have to go beyond the requirements of conditionality and other legal requirements on e.g., fertilization [Art. 31(5) lit. (a), (b) CAPSpR]. Germany allocates 23% of the first pillar budget for eco-schemes [Art. 15(1) GAPDZV], and offers seven eco-schemes (Art. 20 GAPDZG) whose details are established in the GAP-Direktzahlungen-Verordnung (GAPDZV). One nutrient-relevant eco-scheme covers support for the extensification of permanent grassland. Support is provided for holdings which have a livestock density of 0.3–1.4 livestock units per hectare. Fertilizer application is limited to the extend which corresponds to the manure of 1.4 livestock units per hectare [Art. 20(1) No. 4 GAPDZG i.c.w. Annex 5 No. 4 GAPDZV]. Hence, nutrient input into the environment can be reduced. Another nutrient-relevant eco-scheme covers the cultivation of diverse crops including 10% legumes. A minimum of five different crops must be grown on at least 10% and at most 30% of the arable land. Grains must not be cultivated on more than 66% of the arable land (Art. 20(1) No. 2 GAPDZG i.c.w. Annex 5 No. 2 GAPDZV). Legumes can fix nitrogen and supply it to plants (Hillel, 2008, p. 155; Blume et al., 2016, p. 102; Stagnari et al., 2017). Thus, N fertilizer demand can be reduced. Besides, when legumes are used as catch crops, they benefit the prevention of erosion and improve soil structure, thus avoid P losses and potentially enhance P availability for plants (Eichler-Löbermann et al., 2016; Vitow et al., 2021).
Agri-environment-climate commitments of the second pillar can also contribute to the nutrient objective of the Farm to Fork Strategy. These measures differ from eco-schemes by their multi-annual length. Still, eco-schemes and agri-environment-climate commitments can be combined. For some agri-environment-climate commitments, such as result-based payments for grassland management, participation in an eco-scheme is a mandatory prerequisite (Saxon State Ministry for Energy, 2022a, p. 25). In Saxony, agri-environment-climate commitments are divided into support for arable land, support for grassland, support for organic farming and compensatory allowances. Figure 1 provides an overview of one measure of each category—except for compensatory allowances—which impact nutrient management.
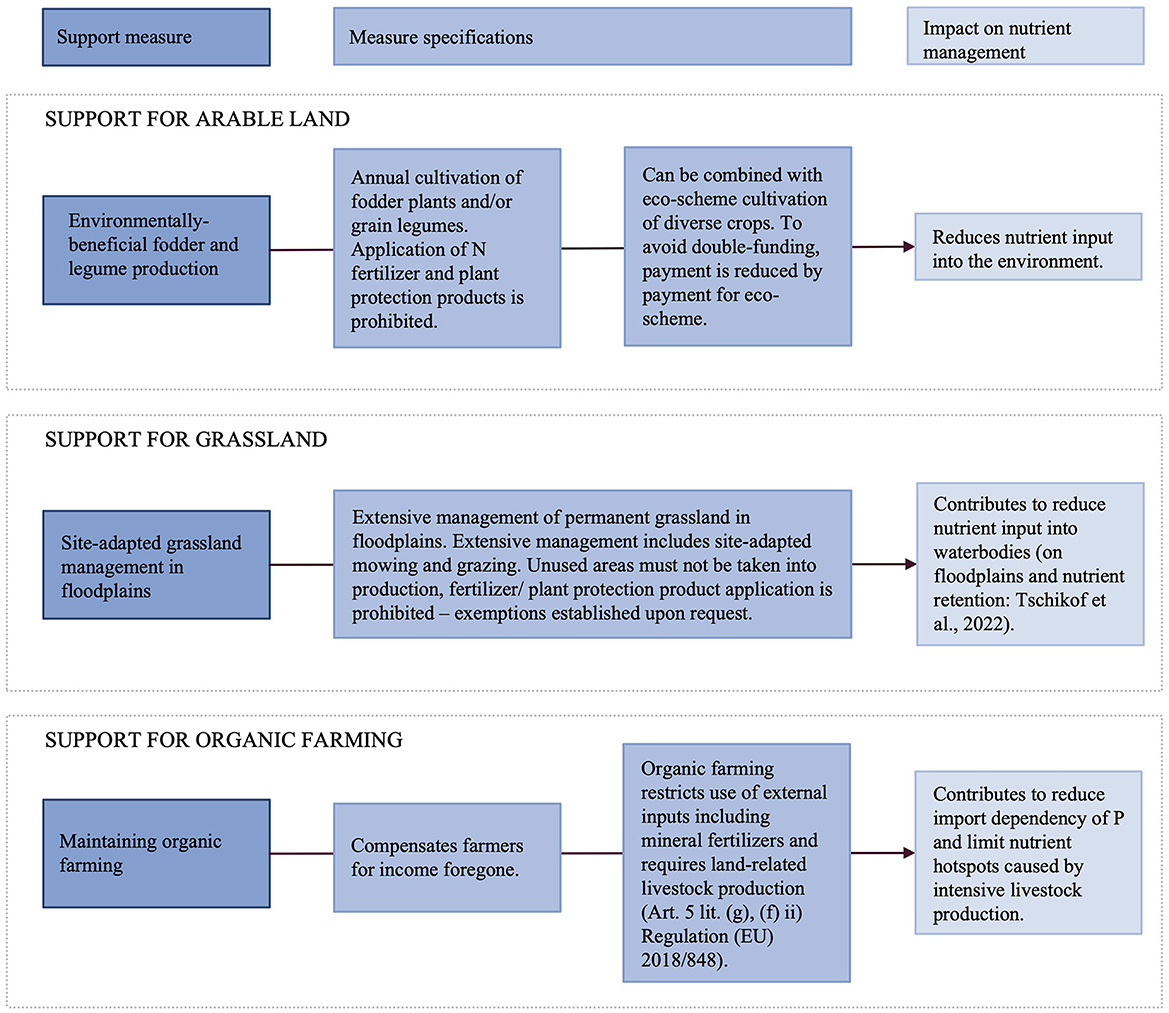
Figure 1. Agri-environment-climate commitments in Saxony (based on Saxon State Ministry for Energy, 2022a, pp. 7, 26, 42).
Overall, both pillars of the CAP support sustainable agricultural practices which can directly and indirectly contribute to the nutrient objective of the Farm to Fork Strategy. Two measures are voluntary (eco-schemes and agri-environment-climate commitments), one measure (conditionality) is mandatory. They address nutrient management differently, for example by reducing fertilizer demand, limiting nutrient losses into the environment and enhancing nutrient monitoring. Whether or not they provide effective means to achieve the nutrient objective of the Farm to Fork Strategy is discussed in the second part of the following section.
6. Discussion
6.1. Critical assessment of the measures and objective of the Farm to Fork Strategy
This section assesses the two measures, i.e., support for digital precision fertilization and sustainable agricultural practices, against the nutrient objective of the Farm to Fork Strategy. Besides, these measures and the Strategy itself are assessed against global environmental goals (Section 2).
Environmental challenges such as disrupted/open nutrient cycles, climate change and biodiversity loss require addressing the major drivers of these challenges, i.e., fossil fuels and intensive livestock farming (Weishaupt et al., 2020; Garske and Ekardt, 2021, p. 245; Stubenrauch et al., 2021, p. 716; van Asselt, 2021; e.g., Eisen and Brown, 2022). In this context, the Farm to Fork Strategy aims to transform the food system of the EU which points in the right direction. In aiming to close P cycles, the Strategy not only addresses open nutrient cycles but also biodiversity loss (Section 1). For example, biodiversity is improved if P fertilization was adjusted to soil properties and plant demand and hence eutrophication of water bodies limited (Section 2). Likewise, in aiming to close N cycles, the Strategy not only addresses open nutrient cycles but also greenhouse gas emissions. Greenhouse gases are reduced if fossil-based N fertilizer was eliminated, livestock farming substantially downscaled and thus manure limited and eutrophication limited. However, the Farm to Fork Strategy fails to target the drivers of environmental pollution when it calls for extending digital precision fertilization and sustainable agricultural practices notably in regions with intensive livestock farming (European Commission, 2020a, p. 8). In doing so, the Farm to Fork Strategy addresses the symptoms of environmental pollution rather than targeting the driver of excess nutrients, i.e., high livestock intensity. As such, this approach will have—if at all—a very limited steering effect.
Likewise, the Farm to Fork Strategy touches upon managing the entire nutrient cycle but effective actions appear unlikely. Sustainable nutrient management is not only an agro-ecological challenge but requires integrated solutions along the entire value chain (Withers et al., 2020; Bieroza et al., 2021). For example, at the beginning of the value chain, P fertilizer production creates radioactive by-products (United States Environmental Protection Agency, 2021). At the end of the value chain, diets have different P intensities (Forber et al., 2020; Papangelou et al., 2021). The Farm to Fork Strategy points to an integrated nutrient management action plan ‘to address nutrient pollution at source' (European Commission, 2020a, p. 8), but many aspects of nutrient management remain unconsidered. Moreover, as Communication, the integrated nutrient management action plan will not be legally-binding and hence also have a limited steering effect.
At last, the general objective of reducing nutrient losses by at least 50% resulting in at least 20% less fertilizer use by 2030 offers shortcomings (see also Bieroza et al., 2021, p. 3–5). Focusing only on P fertilizers containing rock phosphate (and N fertilizers produced by the Haber-Bosch process) bears the danger of shifting effects: Manure could replace nutrient supply from fertilizers containing rock phosphate (proposed by delegations at the Council of the European Union, 2022). While this would limit the import dependency of rock phosphate, nutrient hotspots will remain. These nutrient hotspots would have to be addressed by a supplementary livestock-to-land ratio. However, only adopting a livestock-to-land-ratio will to a limited extend only address greenhouse gas emissions and biodiversity issues of livestock farming. Instead, if the drivers of environmental pollution were addressed at EU level by (1) extending the Emissions Trading System with an emission cap zero by 2030 (Ekardt et al., 2018, 2022; Ekardt, 2020, chap. 4) and (2) implementing emission trading for livestock products (Weishaupt et al., 2020), livestock numbers were reduced and nutrient losses automatically downscaled (but) without a specific reduction rate. Reduced livestock numbers will limit manure supply, but a livestock-to-land ratio is still needed for nutrient hotspots. The advantage of targeting the drivers of climate change and biodiversity loss instead of focusing only on nutrient management is that this approach tackles the intertwined global challenges simultaneously and hence contributes to achieving the goals of the Paris Agreement and the Convention on Biological Diversity. If these policies instruments were adopted, nutrient supply would depend on the extent to which mineral fertilizers could be produced with renewable energies, and the extent to which policy instruments push P recycling (Garske and Ekardt, 2021). These conditions will virtually lead to nutrient loss reductions by at least 50% (probably more) and hence to achieving the objective of the Farm to Fork Strategy. However, it remains an open question whether further support for digital precision fertilization and sustainable agricultural practices would be required in addition to measures which address the major drivers of environmental pollution—given that a ETS for the major drivers as such makes precision farming more attractive. In any case, failing to address fossil fuels and intensive livestock farming impedes achieving the nutrient objective of the Strategy—and the goals of the Paris Agreement and the Convention on Biological Diversity. Measures, whether digital (precision fertilization) or not (sustainable agricultural practices), will then miss the mark.
6.2. Critical assessment of Germany's CAP Strategic Plan
The Farm to Fork Strategy aims to establish a sustainable food system in the EU—alongside the EU's major agricultural policy, the CAP. However, the CAP has been criticized for its catastrophic impact on the environment for decades and the recent reform does not mark a turnaround (Scown and Nicholas, 2020; European Court of Auditors, 2021; Heyl et al., 2021; Scheffler et al., 2022). It is thus unsurprising that Germany's Strategic Plan of the reformed CAP reflects the longstanding inadequacies of the agricultural subsidies. Therefore, developments like ongoing soil degradation in the EU (Panagos et al., 2021) are likely to progress and will not only negatively affect nutrient cycles but also contribute to climate change and biodiversity loss. The reformed CAP thus clearly stands against the goals of the Paris Agreement and the Convention on Biological Diversity. In this context, the Commission's assessment of Germany's Strategic Plan required amendments (Art. 118 CAPSpR). In fact, the Commission requested Germany to establish a clearer alignment of the Strategic Plan with the Farm to Fork Strategy (European Commission, 2020a, p. 5). However, substantial improvements of the Strategic Plan are unlikely as the national legislative process has been completed.
The following section discusses the limited support for sustainable agricultural practices in Germany's CAP Strategic Plan. The section thereafter assesses the support for digital precision fertilization to then draw some general conclusions on the limitations of digitalization as a purely technical strategy for a sustainable transition in the agricultural sector.
6.2.1. Limited support for sustainable agricultural practices
CAP support for sustainable agricultural practices is limited and unable to counteract the damaging impact of e.g., hectare-based income support with its weak environmental requirements. This is unfortunate as sustainable agricultural practices can support soil protection and soil biodiversity and thereby contribute to close nutrient cycles, and benefit climate and biodiversity (Jian et al., 2020; Köninger et al., 2021; Redlich et al., 2021; Xiao et al., 2021b). In doing so, these practices could support achieving the Farm to Fork objective and global environmental goals. Still, the voluntary measures of the CAP contain some promising instruments to support sustainable agricultural practices including on nutrient management. For example, the eco-schemes of the first pillar will likely reduce nutrient losses into the environment (Section 5). However, the effectiveness of eco-schemes is limited by their annual length as some impacts require multiple years to occur (Russi et al., 2016; Lønborg and Markager, 2021). Limited length is not an issue of the agri-environment-climate commitments of the second pillar which take multiple years. Instead, these programs are limited by substantial funding cuts (Nègre, 2022). Above all, both measures suffer from unclear uptake due to their voluntariness. Besides, a general issue of CAP subsidies is that they fail to incentivize landscape-scale adoption for example because of missing collaboration between different stakeholders (Leventon et al., 2017; Manolache et al., 2020; Berthet et al., 2021; Falco et al., 2021). Landscape-scale (or catchment-scale) adoption has been called for to reduce nutrient losses into the Baltic Sea (Bitschofsky and Nausch, 2019; Bieroza et al., 2021). These approaches could not only enhance effectiveness but also limit potential shifting effects. To counteract these shortcomings, a complete restructuring of CAP subsidies (toward the provision of public goods) is needed.
The steering effect of conditionality on nutrient management is limited as well. The effectiveness of these requirements is limited by multiple exemptions (GAEC 4,7,8), voluntariness (SMR 2) and very limited controls of a minimum of 1% of subsidy recipients [Art. 16(1) GAPKondG]. Moreover, concerns about food security due to the war in the Ukraine have recently led to a dilution of GAEC 7 (crop rotation) and GAEC 8 (non-productive areas) (Commission Implementing Regulation (EU) 2022/1317). Apart from that, Germany does not use its flexibility to implement ambitious standards under conditionality. A comparatively high environmental protection standard could have been achieved if Germany had oriented national standards toward the upper end of EU policy leeway. However, Germany frequently only adopts the minimum requirements. The establishment of buffer strips along water courses offers one example. According to EU provisions, farmers have to establish buffer strips with a minimum width of three meters (Annex III CAPSpR). Germany adopts this minimum requirement (Section 5). However, studies show that a limited buffer strip width limits P retention capacity (Sidemo-Holm et al., 2018, p. 212–213; see also Dal Ferro et al., 2019). Hence, a buffer strip width of three meters will have a limited effect on reducing P losses and on the nutrient objective of the Farm to Fork Strategy. It is thus good to see that the Commission picked up this issue in its observation letter and demands an explanation on the limited width. Another example of the limited environmental protection ambitions of Germany concerns the eco-scheme's budget. EU provisions require a minimum budget for the eco-schemes (Art. 31 CAPSpR i.c.w. EU-document 10217/21). Again, Germany orients national implementation at the minimum requirements. However, in its observation letter, the Commission does not even see these minimum requirements fulfilled. The Commission demands a budget increase (European Commission, 2022a, p. 3). Overall, while the CAP Strategic Plan of Germany contains support for sustainable agricultural practices, this support is limited. Sustainable agricultural practices under the reformed CAP will thus to a limited extend only contribute to the nutrient objective of the Farm to Fork Strategy.
6.2.2. Policy issues of support for digital precision farming
While quantity-based policy instruments virtually redirect nutrient management toward sustainability (Section 6.1), CAP subsidies on precision fertilization are unlikely to induce such a development (Heyl et al., 2022). Saxony supports investments which contribute to climate and environmental protection such as investments into resource-saving manure application and site-adapted fertilization (Section 4). However, how to ensure that these investments will clearly contribute to climate and environmental protection – rather than environmental degradation – remains an open question. Besides, typical governance problems of these subsidies such as rebound and shifting effects are likely (Section 2). For example, the application of digital technologies in the agricultural sector is frequently associated with production extension and intensification due to efficiency gains (Schieffer and Dillon, 2015; Garske et al., 2021). Moreover, instruments that address single products, actions or sites like CAP subsidies suffer from enforcement issues and substantial administrative burden. While Member States may use remote sensing or an area monitoring system to check compliance with conditionality (Art. 83(6) lit. (c) Regulation (EU) 2021/2116), thus use digital technologies to limit these governance issues, their actual application could be further extended (José Martínez, 2019; Pe'er et al., 2019). Hence, these subsidies will to a limited extend only contribute to the nutrient objective of the Farm to Fork Strategy.
A promising proposal of the reformed CAP was the inclusion of a digital Farm Sustainability Tool for Nutrients in conditionality (Art. 12(3) CO (2018)392 final). As standard of good agricultural and environmental conditions of land, the application of this tool would have been compulsory to receive income support under the first pillar of the CAP (Section 2). However, Germany successfully pushed for the removal of the tool from conditionality (German Bundestag, 2020). Now, the tool is adopted as voluntary farm advisory service (Section 4) which drastically limits its steering effect and thus its contribution to the nutrient objective of the Farm to Fork Strategy.
6.3. Limitations of digitalization as technical sustainability strategy
The findings above lead to some general implications on digitalization as sustainability strategy in the agricultural sector. Digital precision fertilization practices like sensor-based measurement of soil properties (Section 3) enable or simplify efficient and site-adapted fertilization and thus contribute to reduce nutrient input into the soil and nutrient losses into the environment. However, “precise” does not always mean “less”. Besides, digital precision fertilization is useless in regions with intensive livestock farming and corresponding nutrient hotspots due to manure excretion (Section 6.1). As such, the application area of digital precision fertilization is limited, and hence their geographical contribution to the nutrient objective of the Farm to Fork Strategy. Moreover, the potentials of remote sensing to optimize fertilization management is frequently overestimated (Colaço and Bramley, 2018), and applying artificial intelligence offers further challenges. For example, algorithms can be guided by different objectives such as reducing fertilizer input, minimizing environmental impacts or improving fertilization profitability. The latter objective demands maximum yields in relation to financial input which is likely to induce negative environmental impacts (see e.g., Saikai et al., 2020; Tzachor et al., 2022). Therefore, to contribute to the nutrient objective of the Farm to Fork Strategy, digital precision fertilization must clearly aim at fertilizer reduction and nutrient loss minimization.
Overall, great hopes are placed into the digitalization of the agricultural sector. These hopes reflect a widely shared expectation that digital applications can—as purely technical and efficiency-oriented strategy—achieve global environmental goals (Ekardt, 2020, chap. 1.3). However, the complexity of climate change, biodiversity loss (and nutrient cycling) counteracts these expectations. Still, digitalization offers many potentials to transition the sector toward sustainability but these potentials frequently come with substantial governance problems (see above). In general, the digitalization in the agricultural sector must not lead to an expansion of land for agricultural production but lead to an expansion of land for biodiversity. To this end, livestock farming has to be reduced due to its land and resource intensity (Weishaupt et al., 2020). Overall, the complexity of the environmental challenges and the efforts it requires to achieve the goals of the Paris Agreement and Convention on Biological Diversity will most likely require voluntary or politically-induced sufficiency. This applies to digitalization and all other areas of society. The proposed quantity-based instruments will induce technological efficiency and consistency as well as sufficiency and thus achieve the goals of global environmental agreements.
7. Conclusions
The Farm to Fork Strategy aims at sustainable food systems. To this end, the Farm to Fork Strategy objective is to reduce nutrient losses by at least 50% resulting in at least 20% less fertilizer use by 2030. Digital precision fertilization and sustainable agricultural practices are expected to contribute to this nutrient objective. Support for these measures is provided through the CAP. In this context, this article aimed to assess the extent to which the national implementation of the CAP in Germany provides support for digital precision fertilization and sustainable agricultural practices. While results highlight some fundamental issues of the nutrient objective of the Farm to Fork Strategy, the Strategy generally points in the right direction. Besides, the CAP as implementing instrument of the nutrient objective principally provides some support for sustainable agricultural practices under both pillars. The adoption of the voluntary eco-schemes in particular is a welcome addition. Like agri-environment-climate commitments, these measures can be beneficial for sustainable nutrient management and hence contribute to the nutrient objective of the Farm to Fork Strategy. At the same time, the effectiveness of these measures is limited by limited length (eco-schemes), reduced budget (agri-environment-climate commitments) and voluntariness (both measures). Besides, Germany frequently adopts only minimum requirements for the receipt of income support (conditionality) which further limits the contribution of CAP support to the nutrient objective of the Farm to Fork Strategy. Support for digital precision fertilization is provided under the second pillar of the CAP. However, these subsidies suffer from typical governance issues. Besides, digital precision fertilization will only contribute to the nutrient objective of the Farm to Fork Strategy when (1) clearly aligned with fertilizer reduction and nutrient loss minimization and (2) combined with quantity-based policy instruments which target the major drivers of global environmental challenges, i.e., fossil fuels and livestock farming. Currently, CAP subsidies fail to address these drivers. In doing so, these subsidies will to a limited extent only contribute to the nutrient objective of the Farm to Fork Strategy and the goals of the Paris Agreement and the Convention on Biological Diversity. Therefore, these subsidies have to be restructured toward the provision of public goods only.
Data availability statement
The original contributions presented in the study are included in the article/supplementary material, further inquiries can be directed to the corresponding author.
Author contributions
KH: conceptualization, methodology, formal analysis, writing—original draft preparation, and writing—review and editing. FE: methodology, writing—review and editing, and supervision. PR: background and formal analysis. BG: formal analysis and writing—review and editing. All authors contributed to the article and approved the submitted version.
Funding
This research was partly funded by the German Federal Ministry of Education and Research (BMBF) within the BonaRes project InnoSoilPhos (No. 031B1061A) and by the Leibniz Association within the Leibniz ScienceCampus Phosphorus Research Rostock.
Conflict of interest
The authors declare that the research was conducted in the absence of any commercial or financial relationships that could be construed as a potential conflict of interest.
Publisher's note
All claims expressed in this article are solely those of the authors and do not necessarily represent those of their affiliated organizations, or those of the publisher, the editors and the reviewers. Any product that may be evaluated in this article, or claim that may be made by its manufacturer, is not guaranteed or endorsed by the publisher.
Footnotes
References
Ahmadi, A., Emami, M., Daccache, A., and He, L. (2021). Soil properties prediction for precision agriculture using visible and near-infrared spectroscopy: a systematic review and meta-analysis. Agronomy. 11, 433. doi: 10.3390/agronomy11030433
Alewell, C., Ringeval, B., Ballabio, C., Robinson, D. A., Panagos, P., and Borrelli, P. (2020). Global phosphorus shortage will be aggravated by soil erosion. Nat. Commun. 11, 4546. doi: 10.1038/s41467-020-18326-7
Balafoutis, A., Beck, A., Fountas, S., Vangeyte, J., Wal, T., Soto, I., et al. (2017). Precision agriculture technologies positively contributing to GHG emissions mitigation, farm productivity and economics. Sustainability. 9, 1339. doi: 10.3390/su9081339
Baquedano, F., Jelliffe, J., Beckman, J., Ivanic, J., Zereyesus, Y., and Johnson, M. (2022). Food security implications for low- and middle-income countries under agricultural input reduction: the case of the European Union's farm to fork and biodiversity strategies. Appl. Econ. Perspect. Policy. 44, 1942–1954. doi: 10.1002/aepp.13236
Bazzan, G., Daugbjerg, C., and Tosun, J. (2022). Attaining policy integration through the integration of new policy instruments: the case of the Farm to Fork Strategy. Appl. Econ. Perspect. Policy.13235. doi: 10.1002/aepp.13235
Beckman, J., Ivanic, M., and Jelliffe, J. (2022). Market impacts of Farm to Fork: Reducing agricultural input usage. Appl. Econ. Perspect. Policy. 44, 1995–2013. doi: 10.1002/aepp.13176
Berthet, A., Vincent, A., and Fleury. (2021). Water quality issues and agriculture: An international review of innovative policy schemes. Land Use Policy. 109, 105654. doi: 10.1016/j.landusepol.2021.105654
Bieroza, M. Z., Bol, R., and Glendell, M. (2021). What is the deal with the green deal: will the new strategy help to improve european freshwater quality beyond the Water Framework Directive?. Sci. Total Environ. 791, 148080. doi: 10.1016/j.scitotenv.2021.148080
Bitschofsky, F., and Nausch, M. (2019). Spatial and seasonal variations in phosphorus speciation along a river in a lowland catchment (Warnow, Germany). Sci. Total Environ. 657, 671–685. doi: 10.1016/j.scitotenv.2018.12.009
Blume, H., Brummer, G. W., Fleige, H., Horn, R., Kandeler, E., Knabner, I., et al. (2016). Scheffer/Schachtschabel Soil Science. 1st edn. Berlin, Heidelberg: Springer. doi: 10.1007/978-3-642-30942-7
Bogrekci, I., and Lee, W. S. (2005). Spectral phosphorus mapping using diffuse reflectance of soils and grass. Biosyst. Eng. 91, 305–312. doi: 10.1016/j.biosystemseng.2005.04.015
Borrelli, P., Pangos, P., Ballablo, C., Lugato, E., Weynants, M., and Montanarlla, L. (2016). Towards a pan-European assessment of land susceptibility to wind erosion. Land Degrad. Dev. 27, 1093–1105. doi: 10.1002/ldr.2318
Calcante, A., Brambilla, M., Oberti, R., and Bisaglia, C. (2015). A retrofit variable-rate control system for pressurized slurry tankers. Appl. Eng. Agric. 31, 569–579. doi: 10.13031/aea.31.10885
Colaço, A. F., and Bramley, R. G. V. (2018). Do crop sensors promote improved nitrogen management in grain crops?. Field Crops Res. 218, 126–140. doi: 10.1016/j.fcr.2018.01.007
Cortignani, R., Buttinelli, R., and Dono, G. (2022). Farm to Fork strategy and restrictions on the use of chemical inputs: Impacts on the various types of farming and territories of Italy. Sci. Total Environ. 810, 152259. doi: 10.1016/j.scitotenv.2021.152259
Council of the European Union (2022). Promoting RENURE Fertilizers as Part of a Circular Economy - Information from the Belgian Delegation, Supported by Hungary, the Netherlands, Portugal and Spain [13473/22]. Brussels: Council of the EU.
Dao, T. H. (2017). Sensing soil and foliar phosphorus fluorescence in Zea mays in response to large phosphorus additions. Precis. Agric. 18, 685–700. doi: 10.1007/s11119-016-9480-7
Dong, W., Wu, T., Sun, Y., and Luo, J. (2018). “Digital mapping of soil available phosphorus supported by AI Technology for precision agriculture,” in 2018 7th International Conference on Agro-geoinformatics, p. 1–5. doi: 10.1109/Agro-Geoinformatics.2018.8476007
Eichler-Löbermann, B., Bachmann, S., Busch, S., Schiemenz, K., Krey, T., Pfahler, V., et al. (2016). “Management Options for an Efficient Utilization of Phosphorus in Agroecosystems,” in Phosphorus in Agriculture: 100 % Zero. Dordrecht: Springer Netherlands, Schnug, E., and De Kok, L. J. (eds). p. 179–193. doi: 10.1007/978-94-017-7612-7_9
Eisen, M. B., and Brown, P. O. (2022). Rapid global phaseout of animal agriculture has the potential to stabilize greenhouse gas levels for 30 years and offset 68 percent of CO2 emissions this century. PLOS Climate. 1, e0000010. doi: 10.1371/journal.pclm.0000010
Ekardt, F. (2020). Sustainability: Transformation, Governance, Ethics, Law. Cham, Switzerland: Springer (Environmental humanities: transformation, governance, ethics, law). doi: 10.1007/978-3-030-19277-8
Ekardt, F. (2022) 'Für eine integrierte nachhaltige und digitale, sozial(rechtlich) flankierte Transformation. 10 Thesen für Politik und Forschung' Zeitschrift für neues Energierecht. 6, 433–436.
Ekardt, F., Bärenwaldt, M., and Heyl, K. (2022). The Paris Target, Human Rights, and IPCC Weaknesses: legal arguments in favour of smaller carbon budgets. Environments. 9, 112. doi: 10.3390/environments9090112
Ekardt, F., and Rath, T. (2022). Digitalisierung in der Wärmewende als Rechts- und Governance-Problem: Chancen und Grenzen. Zeitschrift für Neues Energierecht. 3, 211–218.
Ekardt, F., Wieding, J., and Zorn, A. (2018). Paris agreement, precautionary principle and human rights: zero emissions in two decades?. Sustainability. 10, 2812–2827. doi: 10.3390/su10082812
Erler, A., Riebe, D., Beitz, T., Löhmannsröben, H-G., and Gebbers, R. (2020). Soil nutrient detection for precision agriculture using handheld laser-induced breakdown spectroscopy (LIBS) and multivariate regression methods (PLSR, Lasso and GPR). Sensors. 20, 418. doi: 10.3390/s20020418
Essl, L., Atzberger, C., Sandén, T., Spiegel, H., Blasch, J., and Vuolo, F. (2021). Multidisziplinäre Untersuchungen zur nachhaltigen Stickstoffdüngung unter Berücksichtigung der Möglichkeiten der satellitengestützten Präzisionslandwirtschaft. Multidisciplinary studies on sustainable nitrogen fertilisation considering the potential of satellite-based precision agriculture. Die Bodenkultur. 72, 45–56. doi: 10.2478/boku-2021-0005
European Commission (2020c). Study on the EU's list of Critical Raw Materials (2020). Brussels: European Union.
European Commission (2022a). Anlage. Bemerkungen zum GAP-Strategieplan von Deutschland. Brussels: European Commission.
European Commission (2022b). The Commission approves the CAP Strategic Plans of Germany, Greece and Lithuania [News Article]. Available online at: https://agriculture.ec.europa.eu/news/commission-approves-cap-strategic-plans-germany-greece-and-lithuania-2022-11-21_en (accessed 9 January, 2023).
European Commission. (2020a). Communication from the Commission to the European Parliament, the Council, the European Economic and Social Committee and the Committee of the Regions. A Farm to Fork Strategy for a Fair, Healthy and Environmentally-Friendly Food System [CO) 2020) 381 final]. Brussels: European Commission.
European Commission. (2020b). Communication from the Commission to the European Parliament, the European Council, the Council, the European Economic and Social Committee and the Committee of the Regions. The European Green Deal [CO) 2019) 640 final]. Brussels: European Commission.
European Commission. (2021). Report from the Commission to the Council and the European Parliament on the Implementation of Council Directive 91/676/EEC concerning the protection of waters against Pollution Caused by Nitrates from Agricultural Sources Based on Member State Reports for the Period 2016-2019. CO) 2021) 1000 Final. Brussels: European Commission.
European Court of Auditors (2021). Common Agricultural Policy and climate. Half of EU climate spending but farm emissions are not decreasing [Special Report]. Luxemburg: European Union.
Falco, F. L., Feitelson, E., and Dayan, T. (2021). Spatial scale mismatches in the EU agri-biodiversity conservation policy. the case for a shift to landscape-scale design. Land. 10, 846. doi: 10.3390/land10080846
Federal Ministry of Food and Agriculture. (2022). DE - GAP-Strategieplan für die Bundesrepublik Deutschland. Berlin: Federal Ministry of Food and Agriculture.
Ferro, N. D., Borin, M., Cardinali, A., Cavalli, R., Grigolato, S., and Zanin, G. (2019). Buffer strips on the low-lying plain of veneto region (italy): environmental benefits and efficient use of wood as an energy resource. J. Environ. Qual. 48, 280–288. doi: 10.2134/jeq2018.07.0261
Forber, K. J., Rothwell, S. A., Metson, G. S., Jarvie, H., and Withers, P. J. A. (2020). Plant-based diets add to the wastewater phosphorus burden. Environ. Res. Lett. 15, 094018. doi: 10.1088/1748-9326/ab9271
Fountas, S., Mylonas, N., Malounas, I., Rodias, E., Santos, C. H., and Pekkeriet, E. (2020). Agricultural robotics for field operations. Sensors. 20, 2672. doi: 10.3390/s20092672
Garske, B. (2019). “Phosphor-Governance,” in Rechtliche Steuerungsinstrumente der landwirtschaftlichen Phosphornutzung und ihre Bezüge zu den ökologischen Problemfeldern Böden, Gewässer, Biodiversität und Klima. Ekardt, F., et al. (eds). Marburg: Metropolis-Verlag (Beiträge zur sozialwissenschaftlichen Nachhaltigkeitsforschung).
Garske, B., Bau, A., and Ekardt, F. (2021). Digitalization and AI in European agriculture: a strategy for achieving climate and biodiversity targets?. Sustainability. 13, 4652. doi: 10.3390/su13094652
Garske, B., and Ekardt, F. (2021). Economic policy instruments for sustainable phosphorus management: taking into account climate and biodiversity targets. Environ. Sci. Eur. 33, 245–256. doi: 10.1186/s12302-021-00499-7
German Bundestag (2020). Antwort der Bundesregierung auf die Kleine Anfrage der Abgeordneten Stephan Protschka, Peter Felser, Franziska Gminder, weiterer Abgeordneter und der Fraktion der AfD.Drucksache 19/16646. Berlin: German Bundestag.
Grisso, R., Alley, M. M., Thomason, W. E., Holshouser, D. L., and Roberson, G. T. (2011). Precision farming tools: Variable-rate application. Blacksburg: Virginia Cooperative Extension. p. 442–505.
Heyl, K. (2022). Potentials and limitations of subsidies in sustainability governance: the example of agriculture. Sustainability. 14, 15859. doi: 10.3390/su142315859
Heyl, K., Doring, T., Garske, B., Stubenrauch, J., and Ekardt, F. (2021). The common agricultural policy beyond 2020: a critical review in light of global environmental goals. Rev. Eur. Comp. Int. 30, 95–106. doi: 10.1111/reel.12351
Hillel, D. (2008). Soil in the Environment. Crucible of Terrestrial Life. Burlington, San Diego and London: Academic Press.
Iho, A., and Laukkanen, M. (2012). Precision phosphorus management and agricultural phosphorus loading. Ecol. Econ. 77, 91–102. doi: 10.1016/j.ecolecon.2012.02.010
International Energy Agency (2021). Ammonia Technology. Roadmap Towards more sustainable nitrogen fertiliser production. Paris: International Energy Agency.
Jansson, T., Mordin, I., Wilhelmensson, F., Witzke, P., Tasevske, G., Weiss, F., et al. (2020). Coupled agricultural subsidies in the EU undermine climate efforts. Appl. Econ. Perspect. Policy. 13092, 1503–1519.
Jian, J., Ros, G. H., Young, M. D., and de Vries, W. (2020). A meta-analysis of global cropland soil carbon changes due to cover cropping. Soil Biol. Biochem. 143, 107735. doi: 10.1016/j.soilbio.2020.107735
José Martínez (2019). “Möglichkeiten, Ansatzpunkte und Grenzen einer Verwaltungsvereinfachung der Gemeinsamen Agrarpolitik der EU,” in Berichte über Landwirtschaft, (Sonderheft 226).
Jupp, A. R., Beijer, S., Narain, G., Schipper, W., and Slootweg, J. (2021). Phosphorus recovery and recycling – closing the loop. Chemical Society Rev. 50, 87–101. doi: 10.1039/D0CS01150A
Kim, H.-J., Sudduth, K. A., and Hummel, J. W. (2009). Soil macronutrient sensing for precision agriculture. J. Environ. Monitor. 11, 1810. doi: 10.1039/b906634a
König, L. M., and Araújo-Soares, V. (2021). Will the Farm to Fork strategy be effective in changing food consumption behavior? A health psychology perspective. Appl. Econ. Perspect. Policy. 13220. doi: 10.1002/aepp.13220
Köninger, J., Lugato, E., Panagos, P., Kochupilla, M., Orgizzai, A., and Briones, M. (2021). Manure management and soil biodiversity: towards more sustainable food systems in the EU. Agricultural Syst. 194, 103251. doi: 10.1016/j.agsy.2021.103251
Kuang, B., Mahmood, H., Quraishi, M., Hoogmed, W., Mouazan, A., and Henten, E. (2012). “Sensing Soil Properties in the Laboratory, In Situ, and On-Line,” in Advances in Agronomy. Amsterdam: Elsevier p. 155–223. doi: 10.1016/B978-0-12-394275-3.00003-1
Laskar, S., and Mukherjee, S. (2016). Optical Sensing Methods for Assessment of Soil Macronutrients and other Properties for Application in Precision Agriculture: A review. ADBU Journal of Engineering Technology 4, 206–210.
Leenen, M., Patzold, S., Toth, G., and Welp, G. (2022). A LUCAS-based mid-infrared soil spectral library: its usefulness for soil survey and precision agriculture. J. Plant Nutr. Soil Sci. 185, 370–383. doi: 10.1002/jpln.202100031
Leventon, J., Schaal, T., Velten, S., Danhardt, J., Fischer, J., Abson, D., et al. (2017). Collaboration or fragmentation? Biodiversity management through the common agricultural policy. Land Use Policy 64, 1–12. doi: 10.1016/j.landusepol.2017.02.009
Lønborg, C., and Markager, S. (2021). Nitrogen in the Baltic Sea: Long-term trends, a budget and decadal time lags in responses to declining inputs. Estuarine, Coastal and Shelf Science. 261, 107529. doi: 10.1016/j.ecss.2021.107529
Lowry, G. V., Avellan, A., and Gilbertson, L. M. (2019). Opportunities and challenges for nanotechnology in the agri-tech revolution. Nature Nanotechnol. 14, 517–522. doi: 10.1038/s41565-019-0461-7
Maes, W. H., and Steppe, K. (2019). Perspectives for remote sensing with unmanned aerial vehicles in precision agriculture. Trends in Plant Sci. 24, 152–164. doi: 10.1016/j.tplants.2018.11.007
Maffezzoli, F., Ardolino, M., Bacchetti, A., Perona, M., and Renga, F. (2022). Agriculture 4.0: A systematic literature review on the paradigm, technologies and benefits. Futures. 142, 102998. doi: 10.1016/j.futures.2022.102998
Mahajan, G. R., Pandey, R., Sahoo, R., Gupta, V., Datta, S., and Dinesh, K. (2017). Monitoring nitrogen, phosphorus and sulphur in hybrid rice (Oryza sativa L.) using hyperspectral remote sensing. Precis. Agric. 18, 736–761. doi: 10.1007/s11119-016-9485-2
Mahajan, G. R., Sahoo, R. N., Pandey, R. N., Gupta, V. K., and Dinesh, K. (2014). Using hyperspectral remote sensing techniques to monitor nitrogen, phosphorus, sulphur and potassium in wheat (Triticum aestivum L.). Precis. Agric. 15, 499–522. doi: 10.1007/s11119-014-9348-7
Manolache, S., Nita, A., Hartel, T., Miu, I. V., Ciocanea, C. M., and Rozylowicz, L. (2020). Governance networks around grasslands with contrasting management history. Journal of Environmental Management 273, 111152. doi: 10.1016/j.jenvman.2020.111152
Molitorisová, A., and Burke, C. (2022). Farm to fork strategy: Animal welfare, EU trade policy, and public participation. Appl. Econ. Perspect. Policy. 13326. doi: 10.1002/aepp.13326
Moschitz, H., Muller, A., Kretzschmar, U., Haller, L., de Porras, M., Pfeifer, C., et al. (2021). How can the EU Farm to Fork strategy deliver on its organic promises? Some critical reflections. EuroChoices 20, 30–36. doi: 10.1111/1746-692X.12294
Mulla, D. J. (2013). Twenty five years of remote sensing in precision agriculture: Key advances and remaining knowledge gaps. Biosyst. Eng. 114, 358–371. doi: 10.1016/j.biosystemseng.2012.08.009
Nadporozhskaya, M., Kovsh, N., Paolesse, R., and Lvovva, L. (2022). Recent advances in chemical sensors for soil analysis: a review. Chemosensors. 10, 35. doi: 10.3390/chemosensors10010035
Nair, A. S., Nof, S. Y., and Bechar, A. (2021). “Emerging Directions of Precision Agriculture and Agricultural Robotics,” in Innovation in Agricultural Robotics for Precision Agriculture. Cham: Springer International Publishing (Progress in Precision Agriculture), Bechar, A. (ed.). p. 177–210. doi: 10.1007/978-3-030-77036-5_8
Nègre, F. (2022). The Common Agricultural Policy in Figures [Fact Sheet]. Brussels: European Parliament.
Nessel, M. P., Konnovitch, T., Romero, G. Q., and González, A. L. (2021). Nitrogen and phosphorus enrichment cause declines in invertebrate populations: a global meta-analysis. Biological Rev. 96, 2617–2637. doi: 10.1111/brv.12771
Oberti, R., and Schmilovitch, Z. (2021). “Robotic Spraying for Precision Crop Protection,” in Innovation in Agricultural Robotics for Precision Agriculture. Cham: Springer International Publishing (Progress in Precision Agriculture), Bechar, A. (ed.) p. 117–150. doi: 10.1007/978-3-030-77036-5_6
Panagos, P., Ballabio, C., Himics, M., Scarpe, S., Matthews, F., Bogonoos, M., et al. (2021). Projections of soil loss by water erosion in Europe by 2050. Environ. Sci. Policy. 124, 380–392. doi: 10.1016/j.envsci.2021.07.012
Papangelou, A., Towa, E., Achten, W. M. J., and Mathijs, E. (2021). A resource-based phosphorus footprint for urban diets. Environm. Res. Lett. 16, 075002. doi: 10.1088/1748-9326/ac07d6
Pätzold, S., Leenen, M., Frizen, P., Heggemann, T., Wagner, P., and Rodionov, A. (2020). Predicting plant available phosphorus using infrared spectroscopy with consideration for future mobile sensing applications in precision farming. Precis. Agric. 21, 737–761. doi: 10.1007/s11119-019-09693-3
Pe'er, G., Zinngrebe, Y., Moreira, F., Sirami, C., Schindler, S., Müller, R., et al. (2019). A greener path for the EU common agricultural policy. Science. 365, 449–451. doi: 10.1126/science.aax3146
Purnhagen, K., Clemens, S., Eriksson, D., Fresco, L., Tosun, J., Qaim, M., et al. (2021). Europe's Farm To Fork Strategy and its commitment to biotechnology and organic farming: conflicting or complementary goals?. Trends Plant Sci. 26, 600–606. doi: 10.1016/j.tplants.2021.03.012
Radočaj, D., Juriši,ć, M., and Gašparovi,ć, M. (2022). The role of remote sensing data and methods in a modern approach to fertilization in precision agriculture. Remote Sens.14, 778. doi: 10.3390/rs14030778
Redlich, S., Martin, E. A., and Steffan-Dewenter, I. (2021). Sustainable landscape, soil and crop management practices enhance biodiversity and yield in conventional cereal systems. J. Appl. Ecol. 58, 507–517. doi: 10.1111/1365-2664.13821
Refsnider, J. M., Garcia, J. A., Holliker, B., Hulbert, A. C., Nunez, A., and Streby, H. M. (2021). Effects of harmful algal blooms on stress levels and immune functioning in wetland-associated songbirds and reptiles. Sci. Total Environ. 788, 147790. doi: 10.1016/j.scitotenv.2021.147790
Reinhardt, T. (2022). The Farm To Fork Strategy and the digital transformation of the agrifood sector—An assessment from the perspective of innovation systems. Appl. Econ. Perspect. Policy., aepp.13246. doi: 10.1002/aepp.13246
Rose, D. C., and Chilvers, D. (2018). Agriculture 4.0: broadening responsible innovation in an era of smart farming. Front. Sust. Food Syst. 2, 87. doi: 10.3389/fsufs.2018.00087
Rothwell, S. A., Doody, D. G., Johnston, C., Forber, K. J., Cencic, O, Rechberger, H., et al. (2020). Phosphorus stocks and flows in an intensive livestock dominated food system. Resources, Conservat. Recycl. 163, 105065. doi: 10.1016/j.resconrec.2020.105065
Rovira-Más, F., and Saiz-Rubio, V. (2021). “Robotics for Precision Viticulture,” in Innovation in Agricultural Robotics for Precision Agriculture. Cham: Springer International Publishing (Progress in Precision Agriculture) Bechar, A. (ed.) p. 91–115. doi: 10.1007/978-3-030-77036-5_5
Russi, D., Margue, H., Oppermann, R., and Keenleyside, C. (2016). Result-based agri-environment measures: Market-based instruments, incentives or rewards? The case of Baden-Württemberg. Land Use Policy 54, 69–77. doi: 10.1016/j.landusepol.2016.01.012
Saikai, Y., Patel, V., and Mitchell, P. D. (2020). Machine learning for optimizing complex site-specific management. Comp. Electronics Agri. 174, 105381. doi: 10.1016/j.compag.2020.105381
Saxon State Ministry for Energy Climate Protection Environment Agriculture. (2022a). Entwürfe der Umwelt-, Klima- und anderen Bewirtschaftungsverpflichtungen. Förderperiode 2023 – 2027. Dresden. Available online at: https://www.smekul.sachsen.de/foerderung/entwurfsdokumente-zum-gap-strategieplan-11798.html (accessed 17 February, 2022).
Saxon State Ministry for Energy Climate Protection, Environment and Agriculture. (2022b). Steckbriefe ELER-investiv Förderperiode 2023-2027. Available online at: https://www.smekul.sachsen.de/foerderung/entwurfsdokumente-zum-gap-strategieplan-11798.html (accessed 17 February, 2022).
Scharf, P. C., Shannon, D., Palm, H., Sudduth, K., Drummond, S., Kitchen, N., et al. (2011). Sensor-based nitrogen applications out-performed producer-chosen rates for corn in on-farm demonstrations. Agronomy J. 103, 1683–1691. doi: 10.2134/agronj2011.0164
Schebesta, H., and Candel, J. J. L. (2020). Game-changing potential of the EU's Farm to Fork Strategy. Nature Food 1, 586–588. doi: 10.1038/s43016-020-00166-9
Scheffler, M., Wiegmann, K., Lakner, S., Sommer, P., and Meyer-Jürshof, M. (2022). How much climate action is offered in the first pillar of the CAP? Dessau-Roßlau: Umweltbundesamt.
Schieffer, J., and Dillon, C. (2015). The economic and environmental impacts of precision agriculture and interactions with agro-environmental policy. Precis. Agric. 16, 46–61. doi: 10.1007/s11119-014-9382-5
Schrijver, R. (2016). Precision agriculture and the future of farming in Europe Scientific Foresight Study. Luxemburg: European Union.
Scown, M. W., and Nicholas, K. A. (2020). European agricultural policy requires a stronger performance framework to achieve the Sustainable Development Goals. Global Sustainability 3, 1–11. doi: 10.1017/sus.2020.5
Segarra, J., Buchaillot, M. L., Araus, J. L., and Kefauver, S. C. (2020). Remote sensing for precision agriculture: sentinel-2 improved features and applications. Agronomy 10, 641. doi: 10.3390/agronomy10050641
Sidemo-Holm, W., Smith, H. G., and Brady, M. V. (2018). Improving agricultural pollution abatement through result-based payment schemes. Land Use Policy. 77, 209–219. doi: 10.1016/j.landusepol.2018.05.017
Singh, P., Garg, C., and Namdeo, A. (2020). Applying machine learning techniques to extract dosages of fertilizers for precision agriculture. IOP Conference Series: Earth and Environm. Sci. 614, 012136. doi: 10.1088/1755-1315/614/1/012136
Sishodia, R. P., Ray, R. L., and Singh, S. K. (2020). Applications of Remote Sensing in Precision Agriculture: A Review. Remote Sensing 12, 3136. doi: 10.3390/rs12193136
Smith, P., Soussana, J-F., Angers, D., Schipper, L., Chenu, C., Rasse, D. P., et al. (2020). How to measure, report and verify soil carbon change to realize the potential of soil carbon sequestration for atmospheric greenhouse gas removal. Global Chang. Biol. 26, 219–241. doi: 10.1111/gcb.14815
Soriano-Disla, J. M. (2014). The performance of visible, near-, and mid-infrared reflectance spectroscopy for prediction of soil physical, chemical, and biological properties. Appl. Spectros. Rev. 49, 139–186. doi: 10.1080/05704928.2013.811081
Stagnari, F., Maggio, A., Galieni, A., and Pisante, M. (2017). Multiple benefits of legumes for agriculture sustainability: an overview. Chem. Biol. Technol. Agric. 4, 2. doi: 10.1186/s40538-016-0085-1
Stenberg, B., Viscarra Rossel, R., Mouazen, A. M., and Wetterlind, J. (2010). Visible and Near Infrared Spectroscopy in Soil Science. in Advances in Agronomy. Amsterdam: Elsevier. p. 163–215. doi: 10.1016/S0065-2113(10)07005-7
Stubenrauch, J. (2019). Phosphor-Governance in ländervergleichender Perspektive – Deutschland, Costa Rica, Nicaragua. Ekardt, F., et al. (eds). Marburg: Metropolis-Verlag (Beiträge zur sozialwissenschaftlichen Nachhaltigkeitsforschung).
Stubenrauch, J., Ekardt, F., Heyl, K., Garske, B., Schott, V. L., and Ober, S. (2021). How to legally overcome the distinction between organic and conventional farming - governance approaches for sustainable farming on 100% of the land. Sustain. Prod. Consum. 28, 716–725. doi: 10.1016/j.spc.2021.06.006
Stutter, M., Kronvang, B., hUallacháin, D. Ó., and Rozemeijer, J. (2019). Current Insights into the Effectiveness of Riparian Management, Attainment of Multiple Benefits, and Potential Technical Enhancements. J. Environ. Qual. 48, 236–247. doi: 10.2134/jeq2019.01.0020
The World Bank (2022). Commodity Markets Outlook, April 2022 : The Impact of the War in Ukraine on Commodity Markets. Commodity Market Outlook. Washington, D.C.: The World Bank.
Tschikof, M., Gericke, A., Venohr, M., Weigelhofer, G., Bondar-Kunze, E., Kaden, U. S., et al. (2022). The potential of large floodplains to remove nitrate in river basins – the Danube case. Sci. Total Environ. 843, 156879. doi: 10.1016/j.scitotenv.2022.156879
Tubiello, R. P., Wint, G. R. W., Conchedda, G., Van Boeckel, T. P., Ercoli, V., Palamara, E., et al. (2013). The FAOSTAT database of greenhouse gas emissions from agriculture. Environ. Res. Lett. 8, 015009. doi: 10.1088/1748-9326/8/1/015009
Tzachor, A., Devare, M., King, B., Avin, S., and ÓhÉigeartaigh, S. (2022). Responsible artificial intelligence in agriculture requires systemic understanding of risks and externalities. Nat. Machine Int. 4, 104–109. doi: 10.1038/s42256-022-00440-4
United States Environmental Protection Agency (2021). TENORM: Fertilizer and Fertilizer Production Wastes. Available online at: https://www.epa.gov/radiation/tenorm-fertilizer-and-fertilizer-production-wastes (accessed 10 January, 2023).
Uzoh, I. M., Igwe, C. A., Okebalama, C. B., and Babalola, O. O. (2019). Legume-maize rotation effect on maize productivity and soil fertility parameters under selected agronomic practices in a sandy loam soil. Scientific Rep. 9, 8539. doi: 10.1038/s41598-019-43679-5
van Asselt, H. (2021). Governing fossil fuel production in the age of climate disruption: Towards an international law of “leaving it in the ground”. Earth System Govern. 9, 100118. doi: 10.1016/j.esg.2021.100118
Venter, Z. S., Jacobs, K., and Hawkins, H.-J. (2016). The impact of crop rotation on soil microbial diversity: a meta-analysis. Pedobiologia. 59, 215–223. doi: 10.1016/j.pedobi.2016.04.001
Vitow, N., Zicker, T., Chiba, A., Zacher, A., Eichler-Löbermann, B., Schulz, S., et al. (2021). Impact of the legume catch crop serradella on subsequent growth and p mobilization under barley in different fertilization treatments. Agronomy. 11, 2437. doi: 10.3390/agronomy11122437
Weishaupt, A. (2020). land use, livestock, quantity governance, and economic instruments—sustainability beyond big livestock herds and fossil fuels. Sustainability. 12, 2053–2080. doi: 10.3390/su12052053
Wesseler, J. (2022). The EU's Farm-to-Fork Strategy: An assessment from the perspective of agricultural economics. Appl. Econ. Perspect. Policy. 44, 1826–1843. doi: 10.1002/aepp.13239
Withers, P. J. A., Forber, K. G., Lyon, C., Rothwell, S., Doody, D. G., Jarvie, H. P., et al. (2020). Towards resolving the phosphorus chaos created by food systems. Ambio. 49, 1076–1089. doi: 10.1007/s13280-019-01255-1
Xiao, L, Kuhn, N. J, Zhao, R, and Cao, L. (2021b). Net effects of conservation agriculture principles on sustainable land use: a synthesis. Global Change Biol. 27, 6321–6330. doi: 10.1111/gcb.15906
Xiao, L., Li, G., Zhao, R., and Zhang, L. (2021a). Effects of soil conservation measures on wind erosion control in China: a synthesis. Sci. Total Environ. 778, 146308. doi: 10.1016/j.scitotenv.2021.146308
Xue, J., and Su, B. (2017). Significant remote sensing vegetation indices: a review of developments and applications. Journal of Sensors. 2017, 1–17. doi: 10.1155/2017/1353691
Yang, C., Sui, R., and Lee, W. S. (2016). “Precision Agriculture in Large-Scale Mechanized Farming,” in Precision agriculture technology for crop farming, Zhang, Q. (ed.). Boca Raton: CRC Press. p. 177–211. doi: 10.1201/b19336-6
Keywords: Farm to Fork Strategy, Common Agricultural Policy, precision fertilization, precision farming, digitalization, sustainable agriculture, agricultural subsidies, phosphorus
Citation: Heyl K, Ekardt F, Roos P and Garske B (2023) Achieving the nutrient reduction objective of the Farm to Fork Strategy. An assessment of CAP subsidies for precision fertilization and sustainable agricultural practices in Germany. Front. Sustain. Food Syst. 7:1088640. doi: 10.3389/fsufs.2023.1088640
Received: 03 November 2022; Accepted: 11 January 2023;
Published: 26 January 2023.
Edited by:
Katrien Steenmans, University of Copenhagen, DenmarkReviewed by:
Luciano Gebler, Brazilian Agricultural Research Corporation (EMBRAPA), BrazilAndreea Nita, University of Bucharest, Romania
Copyright © 2023 Heyl, Ekardt, Roos and Garske. This is an open-access article distributed under the terms of the Creative Commons Attribution License (CC BY). The use, distribution or reproduction in other forums is permitted, provided the original author(s) and the copyright owner(s) are credited and that the original publication in this journal is cited, in accordance with accepted academic practice. No use, distribution or reproduction is permitted which does not comply with these terms.
*Correspondence: Katharine Heyl, a2F0aGFyaW5lLmhleWwmI3gwMDA0MDt1bmktcm9zdG9jay5kZQ==