- 1School of Food Science and Engineering, South China University of Technology, Guangzhou, China
- 2Overseas Expertise Introduction Center for Discipline Innovation of Food Nutrition and Human Health (111 Center), Guangzhou, China
- 3National Institute of Food Science and Technology, University of Agriculture, Faisalabad, Pakistan
- 4Institute of Food Science and Nutrition, University of Sargodha, Sargodha, Pakistan
- 5Department of Clinical Laboratory Sciences, College of Applied Medical Sciences, Jouf University, Sakaka, Saudi Arabia
- 6Department of Medical Microbiology and Parasitology, Faculty of Medicine, King AbdulAziz University, Jeddah, Saudi Arabia
- 7Yousef Abdullatif Jameel Scientific Chair of Prophetic Medicine Application, Faculty of Medicine, King Abdulaziz University, Jeddah, Saudi Arabia
- 8Department of Communication and Computing Engineering, Higher Institute of Engineering, El-Shorouk Academy, El-Shorouk City, Egypt
A raw or processed meat product can be a breeding ground for spoilage bacteria (Enterobacteriaceae, Lactobacillus spp., Pseudomonas spp., etc.). Failure of decontamination results in food quality loss and foodborne illnesses caused by pathogens such as Salmonella, Escherichia coli, Staphylococcus aureus, and Listeria monocytogenes. Often, meat processors decontaminate the carcass using cheap chemicals or artificial antimicrobial agents not listed on the ingredient list, which is discouraged by health-conscious consumers. Foods with clean labels became more popular during the COVID-19 pandemic, which led consumers to choose healthier ingredients. Novel methods of controlling or improving meat safety are constantly being discovered. This review focuses on novel means of electrochemically activate water that is being investigated as a sanitizing agent for carcasses and processing area decontamination during production or at the end. Water can be activated by using non-thermal techniques such as ozonation, electrolysis, and cold plasma technologies. Recent studies showed that these activated liquids are powerful tools for reducing microbial activity in raw and processed meat. For instance, plasma-activated water can be used to enhance microbiological safety and avoid the negative effects of direct gaseous plasma on the organoleptic aspects of food products. In addition, electrolyzed water technology offers hurdle enhancement by combining with non-thermal strategies that have great potential. Ozonation is another way of activating water which provides a very convenient way to control microbiological safety and finds several recent applications as aqueous ozone for meat decontamination. These solutions are highly reactive and convenient for non-conventional applications in the meat industry related to food safety because of their antimicrobial or antiviral impact. The present review highlights the efficacy of activated-water decontamination of raw and processed meat via non-thermal solutions.
1. Introduction
Food safety and demand for cleaner labels continue to be an ongoing concern with consumers. Raw and minimally processed meat contains common foodborne pathogens such as Listeria monocytogenes (Zhang et al., 2020), Salmonella Enteritidis (Garrido-Maestu et al., 2019), Escherichia coli O157: H7 (de Assis et al., 2021), Campylobacter (Thomas et al., 2020) and Staphylococcus aureus (Ribeiro et al., 2018), which contributes to foodborne illnesses. The risk of microbial spoilage in raw, minimally processed, and ready-to-eat meat products is associated with temperature variations and extended distances of distribution. The solution is to apply an appropriate technology to tackle these issues or add some synthetic chemicals or artificial antimicrobial agents such as butylated and hydroxyanisole, butylated hydroxytoluene, tert-butyl hydroquinone, propyl gallate, phosphate, nitrate, and nitrite which are always discouraged by health-conscious consumers (Roobab et al., 2021). However, it is technically impossible to produce a bacteria-free, fresh-meat supply.
The meat industry continues tirelessly researching new strategies that reduce bacteria to as close to zero as possible. Traditional meat processing line usually involves cleaning and disinfection steps to reduce pathogens (Smith et al., 2015). Some of the most common conventional mild processing technologies used for the decontamination of commercial meat products include physical treatments, i.e., washing Sá Júnior et al. (2021), steaming (Dixon et al., 2019), chemical treatments, i.e., organic acids (Heir et al., 2022), chlorinated chemicals (Kocharunchitt et al., 2020), hydrogen peroxide (Walsh et al., 2018), phosphate-based compounds (Sallam L. et al., 2020). Sodium hypochlorite is one of the most used, efficient, available, and low-cost disinfectants. Moreover, chlorine dioxide and lactic acid have broad antibacterial effects (Burfoot et al., 2015). In the meat industry, chlorinated water (0.5–1 ppm) is used to reduce microorganisms, prevent cross-contamination, and ensure carcass safety. Several research works revealed that chlorine efficacy is dependent on its concentration; however, its by-products such as trihalomethanes, haloacetic acids, and chloramines have carcinogenic potential (Gil et al., 2016). Similar is the case with chemical sanitizers which produce toxic by-products that are harmful to human health and the environment. Moreover, secondary rinsing with potable water may be needed to remove these acids when higher concentrations are used. This could be a challenge where potable water is scarce (Van Schalkwyk and Hoffman 2016). Besides, several pathogens such as Salmonella are becoming resistant to these traditional disinfectants (Youn et al., 2017; Cadena et al., 2019). Furthermore, the efficiency of chemical sanitizers depends on free chlorine availability, organic material load, microbial load, washing water quality, etc. (Murray et al., 2017). Nowadays, acetic, peroxyacetic acid, and lactic acid is more common for treating meat as a tool to improve meat safety (Nkosi et al., 2021).
Expanding applications for the existing decontamination technologies continue to be constantly studied and investigated by industry, technology suppliers, and academia. Currently, non-thermal interventions are being considered by the meat and poultry industries as alternative technologies. For instance, functionalized water as an environmentally clean label technology is a less expensive and safer technique as compared to traditional chemical sanitizers. The functionally activated water produced by using electricity (Du et al., 2016; Iram et al., 2021; Moghassem Hamidi et al., 2021) ozone, (Fathul Karamah and Wajdi, 2018; Kanaan, 2018; Kalchayanand et al., 2019), and cold plasma technology (Liao et al., 2018; Yong et al., 2018; Inguglia et al., 2020; Zhao et al., 2020; Wang et al., 2021) have become a popular research topic. The antimicrobial effectiveness of electrochemically activated water is not only depending on its constituents but also on its oxidative power which accepts the electrons because of the highly deficient electron clusters. As explained by Aider et al. (2012) anode/solution interface produced anolytes (oxidizing agents), which contain a mixture of free radicals (Cl*, O*, OH*, ) having strong antimicrobial activities. However, the electrochemical activation of the anode reduced the surface tension of the electro-activated medium, increased electric conductivity, and modified the water structure. Water has been utilized for large-scale washing applications, which can be electrolyzed or chemically functionalized to be used for meat safety purposes. Considering the popularity of ozonated water, electrolyzed water, and cold plasma water, their recent applications for meat safety are reviewed. The research on novel non-thermal applications is ongoing, and the potential of electrochemically activated water for ensuring meat safety is being greatly explored. This review covers different non-thermal sources employed for water activation and the advancement of activated electrochemical solutions as the sanitizing agent in various meat products.
2. Activated water as enhancing microbiological safety
2.1. Ozonated water
Ozone is a Generally Recognized as Safe (GRAS) food processing aid, which has a powerful disinfectant and a strong sanitizing ability as compared to other chemical sanitizers. Current usage of ozone has proven effective antimicrobial agent against microbial populations that leaves no toxic residues on food or equipment, making it a greener environment-friendly technology (Pandiselvam et al., 2020; Roobab et al., 2022). Generally, low temperature (4°C) and pH of aqueous medium increased the solubility of ozone and enhanced its effectiveness. Hence, cool, damp, and refrigerated storage conditions for meat products facilitate the use of ozone in meat processing industries. However, excessive usage can produce oxidative spoilage, undesirable odors, and surface discoloration (Bridges et al., 2018). Moreover, its antimicrobial efficacy depends on the solubility in water and the reaction stability with organic and inorganic compounds. Other critical parameters affecting the antimicrobial efficiency of ozone include pH, temperature, and organic residues in food (Bridges et al., 2018). Ozonation is another way of activating water, accomplished by passing gas through ultraviolet irradiations (188 nm wavelength) and corona discharge that excites the oxygen electrons (Figure 1).
The electrochemical process in water generates high energy (around 6–7 eV) that breaks O–O bond and form ozone. The unstable molecule of ozone can decompose into superperoxide, hydroxyl, and hydroperoxyl radicals which have a strong oxidizing ability (2.07 mV oxidizing potential) (Gonçalves 2009). These active radicals can drive a series of chemical reactions when they encounter the bacterial cell. Ozone (2.07 V) causes peroxidation of phospholipids (present in the bacterial cell wall), leading to the cell wall decomposition and leakage of intracellular components (Pandiselvam et al., 2017; Rudolphi-Skórska et al., 2017). The continuous degradative reactions alter the cellular integrity which could be a reason for cell death. However, the ozone efficacy responds differently to Gram-positive and Gram-negative bacteria due to the difference in the cell wall structure such as wavy or smooth cell wall, thick or thin peptidoglycan layer, presence, and absence of outer membrane and periplasmic space (Dizengremel et al., 2009; Sheng L. et al., 2018). Besides, ozone modified the primary structure of proteins and enzymes, thus inactivating several cytosolic enzymes (Dizengremel et al., 2009). Furthermore, secondary reactive species modified purines and pyrimidines (the building blocks of DNA) and damaged the genetic material of the host cell (Ito et al., 2005). However, it also altered the structure of prokaryotic plasmid DNA (Asfahl and Savin, 2012).
2.1.1. Decontamination of meat
Kalchayanand et al. (2019) evaluated a liquid ozone spray chill technique (at 4.6–5.6°C) to inactivate E. coli O157:H7 on surfaces of fresh beef as an alternative to traditional water sprays chill. Results showed 1.46 logs of E. coli O157:H7 reduction and 0.99 logs of aerobic bacteria reduction. Ozonated spray treatment caused sublethal injury to the target bacterial cells, which retarded their growth under highly stressed conditions such as low-temperature storage. Similarly, the treatments of aqueous ozone (0.5 ppm for 45 min) on S. aureus in fresh and frozen chicken meat exhibited 2–4 logs CFU/mL reduction (Kanaan, 2018). The underlying mechanism has been explained by Aponte et al. (2018) who observed the modifications in cell membrane permeability as ozone may attack glycoproteins, glycolipids, and other amino acids (structural components of cells) which inhibit the enzymatic reactions and interrupt the growth and functionality of bacterial cells (Sheng X. et al., 2018). Table 1 lists the parameters used in ozonation to effectively decontaminate raw and processed meat.
The impact of ozonated water is not only limited to fresh carcasses but also effective for processed meat products. Botta et al. (2018) investigated the complexity and dynamics of aqueous ozone treated (6 mg/L for 90 s at 4°C) beefsteaks microbiota using RNA-based amplicon sequencing. Pseudomonas fragi were prominent in treated and control samples. While other microorganisms such as Lactobacillus sakei, Leuconostoc gasicomitatum, and Lactococcus piscium became active during chilled vacuum-packaged storage due to the unavailability of oxygen. Initially, aqueous ozone treatments were unable to modify the microbiota composition, dynamics, and the related volatilome; however, the efficacy to reduce microbial count increased during refrigerated storage. Because the injured bacterial cells cannot proliferate under stressed conditions of low-temperature storage and usually subsequently die under those conditions (Kalchayanand et al., 2019).
2.2. Electrolyzed water
Electrolyzed water (EW) was first developed in Japan as a medical product in the mid-1980s. EW has strong antimicrobial properties because of its pH, oxidation-reduction potential (ORP), and available chlorine content (ACC: 50 mg/L). EW is produced by passing a dilute salt solution through an electrolytic chamber containing anode and cathode electrodes with a bipolar membrane separation (Figure 2).
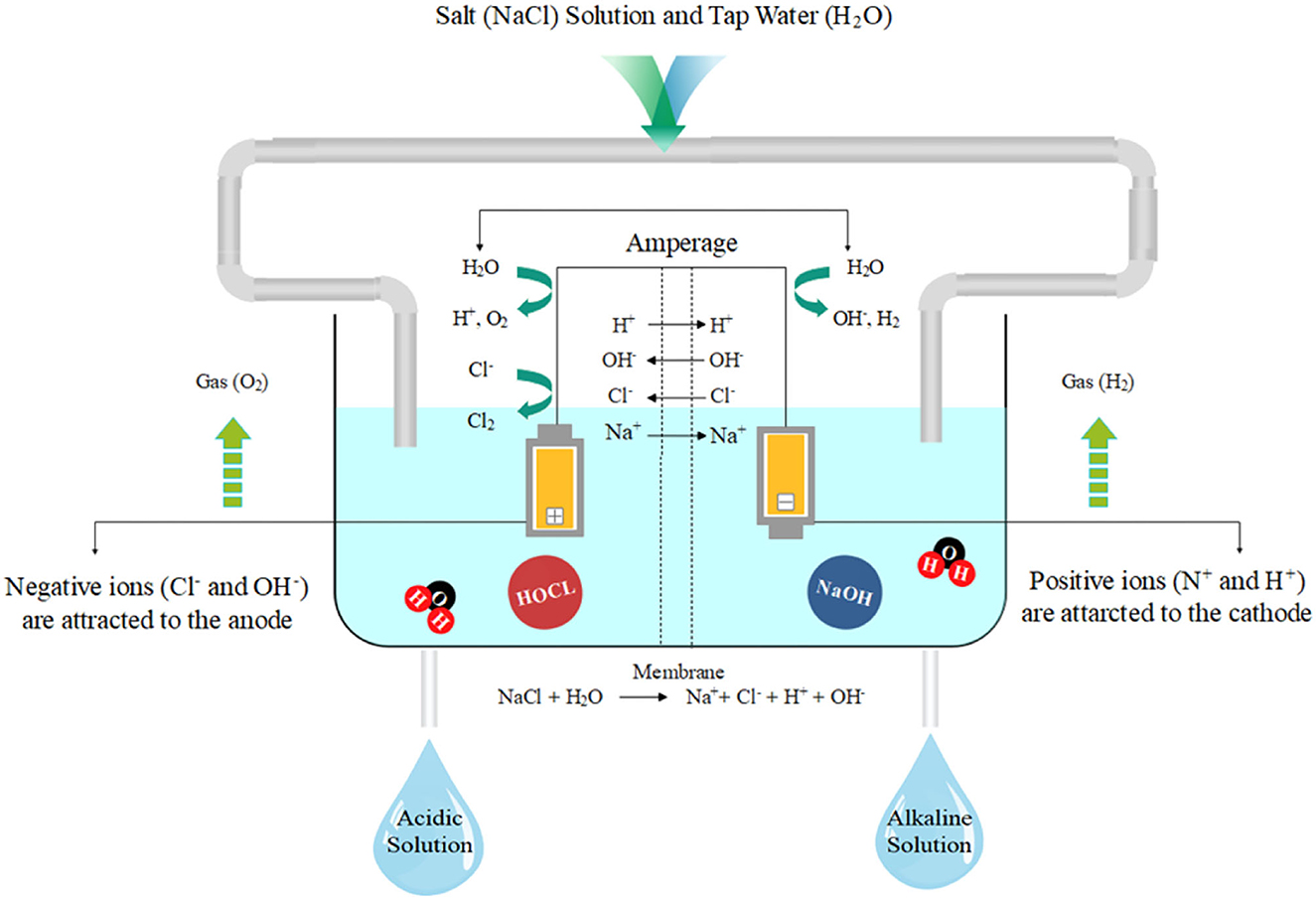
Figure 2. Schematics of electrolyzed water production representing acidic electrolyzed water and alkaline electrolyzed water.
The salt electrolysis dissociates sodium and chloride into positive (Na+) and negative (Cl−) ions (Degala et al., 2020). The resulting solution can be collected separately from the anode or cathode regions and categorized into acidic (AEW) and alkaline electrolyzed water (AlEW), respectively (Hernández-Pimentel et al., 2020). The resultant solution contains active oxygen and other oxidants, including hydrogen peroxide, ozone, free chlorine, and chlorine dioxide. AEW (pH < 2.7, ORP > 1,000 mV) contains the hypochlorous acid (HOCl), which induced destructive oxidation in membrane-bound complexes and disturbed cellular electrical charge. HOCl as a sanitizer has about 80 times stronger antimicrobial capacity against E. coli compared to an equivalent concentration of the hypochlorite ion (ClO−). EW modified the meat protein secondary structure and effectively inactivates pathogens more than conventional chlorine compounds (sodium hypochlorite), hence its applications in meat products would be the second topic of interest in the future (Iram et al., 2021). However, AEW has great potential for use as an antimicrobial agent in the meat industry. For instance, AEW-treated beef, chevon, and pork samples exhibited the highest inactivation of E. coli K12 by 1.16 logs in 4 min, 1.22 logs in 12 min, and 1.30 logs in 10 min, respectively; however, AlEW treatments (pH 10–11.5, ORP: 800–900 mV) reduced 1.61, 0.96, and 1.52 logs in 12 min, respectively (Arya et al., 2018). Several other types of EW are slightly acidic electrolyzed oxidizing water (SAEW), and neutral electrolyzed water (NEW). SAEW (pH 5.0–6.5, ORP 600 mV) is produced by the electrolysis of hydrochloric acid in a chamber without a membrane (Arya et al., 2018). However, NEW is generated by mixing the AEW and AlEW or using an electrolysis chamber without a membrane. NEW has solved the problems related to the storage and corrosion effect of AEW. Recently, the research focus has been shifted toward SAEW applications alone or with ultrasound and UV radiation (Iram et al., 2021).
The effect of EW on microorganisms is complex; however, some researchers linked it with active species produced during the process such as hydroxyl ions and hypochlorite (Wang et al., 2019). The electrolysis phenomenon (produced by immersed electrodes) is based on electrochemical reactions, which involve electron excitation and generates a variety of oxidants. The action of electrons (donors/acceptors) could induce irreversible modifications in the bacterial transmembrane potential. The resultant powerful electro-osmotic reactions disturb the active transport of substances into the cell and diffuse water against oxidation-reduction gradients. It induces an electric charge in the bacterial membrane, following membrane rupture and an outflow of intracellular components (Athayde et al., 2017). Besides, excess anions (present in the anolyte solution) interrupt the functionality of the electrically charged bacterial cell membrane with modifications in solution transport or availability. However, solute transport is depending on the electrostatic interactions and small charged molecules. Thus, any significant change in the ORP of the immediate medium of the bacterial cells can negatively affect the electrochemical gradient (Aider et al., 2012).
2.2.1. Decontamination of meat
EW decontamination involves the disruption of bacterial membrane integrity and induces cell necrosis and apoptosis (Liao et al., 2017). Previously published studies on the effect of EW on raw and processed meat are listed in Table 2.
EW spraying is more convenient in mobile chicken slaughter lines as compared to dip and immersion methods. Additionally, spraying treatments reduce water waste and the formation of disinfectants by-products. Duan et al. (2017) compared the impact of traditional disinfectant sprays [sodium hypochlorite (50 and 100 mg/L), chlorine dioxide (50–100 mg/L), lactic acid (1–2%)] with AEW and SAEW on chicken carcasses. According to the results, 2% lactic acid, AEW, and SAEW reduced 0.47–0.83 log (CFU/cm2) total viable counts and 0.49–0.96 log (MPN/cm2) total coliforms. Moreover, AEW and SAEW exhibited a 2-day extension of microbial shelf life compared to other treatments. Although sodium hypochlorite and chlorine dioxide treatments were ineffective to reduce microorganisms, sodium hypochlorite promotes trihalomethanes formation when reacted with the organic matter. Besides, lactic acid exceeded the 2 mg/kg limit on the 8th day of storage, which led to high thiobarbituric acid reactive substances values and odors and flavors deterioration.
Similarly, Wang et al. (2018) reduced 1.0 logs total viable counts and total coliform in AEW-or SAEW-treated chicken carcasses by spraying with a nozzle at 0.3 MPa pressure for 15 s. Despite all advantages, AEW is becoming less popular because of corrosion and instability. Besides, SAEW (a pH of 5.0–6.5, an ORP of 750–850 mV, and an ACC of 10–30 mg/L) showed significant bactericidal effects with more stability and less corrosion as compared to AEW (Duan et al., 2017). Although 30 mg/L of SAEW has the same antimicrobial effect as 60 mg/L of AEW (Wang et al., 2018). SAEW is allowed to control the pH of the water used in the US meat industries (USDA, 2022). The total viable counts in the SAEW-treated beef meat decreased to 2.28 logs from 3.06 logs (Sheng L. et al., 2018). HOCl (present in SAEW) reduced microbial growth via modifying bacterial electron transfer mechanism (redox potential), which promoted cellular protein oxidation, and DNA damage. Furthermore, SEAW achieved high antimicrobial efficiency with a low available chlorine concentration (Xuan et al., 2016). According to Liao et al. (2020), SAEW thawing caused a 0.83–1.76 log reduction of total bacteria, fungi, and yeast without negatively affecting the texture, pH, and color of the beef carcass. Moreover, SAEW thawing prevented oxidation and degradation of lipid/protein as compared to traditional thawing treatments (air thawing, water thawing, and microwave thawing). Because it did not induce detrimental effects due to protein oxidation or changes in muscle microstructure (Liao et al., 2020).
NEW is another form of EW, approved in the US for poultry processing at doses ≤ 50 mg/L (USDA, 2022). NEW (ORP 750–900 mV) exhibited 10 times higher antimicrobial efficacy against Salmonella in pure culture than sodium hypochlorite treatments via bacterial cell enlargement and membrane structural modifications. In this study, the resistant Salmonella pure cultures mixture (adjusted to 6 log CFU/mL) was inoculated, allowing it to drain through the walls of the gastrointestinal cavity of the previously eviscerated carcasses and then air dried for 10 min in a laminar flow cabinet. Furthermore, broiler carcasses were immersed in NEW or NaClO at 50 mg/L of total available chlorine, and the control using distilled water at 3°C for 1.5 h. According to Hernández-Pimentel et al. (2020), NEW at 14 mg/L (total available chlorine) completely inactivated (>6 logs CFU/mL) the Salmonella pure cultures mixture after 1 min contact time. Moreover, NEW effectively reduced total viable counts and coliforms on broiler chicken carcasses, without color and pH modification. Though Hawkins et al. (2016) achieved 0.56 log CFU/g reduction of Salmonella by Near-NEW spraying (60 s) in raw chicken thigh meat. The active agents of NEW include HOCl and other components (~5%) such as hypochlorite ions and chlorine.
When compared to AEW, NEW has limited human health and safety concerns from chlorine dioxide off-gassing, reduces corrosion of surfaces, and limits phototoxic side effects while maximizing the application of hypochlorous acid species (Moghassem Hamidi et al., 2021). Besides, NEW produces less corrosion compared to other disinfectants such as AEW because of its neutral pH. NEW has comparable antimicrobial efficacy to the other types of sanitizers. For instance, NEW spray (free chlorine value of 58 ppm) and sodium hypochlorite (35 ppm) reduced 0.64 logs of Listeria monocytogenes and 0.3 log of Salmonella Typhi in highly contaminated pork chops (106 CFU/mL) compared to the traditional saline solution (Torres-Rosales et al., 2020). NEW resulted in slight color modifications as compared to sodium hypochlorite or saline solution; however, it decreased lactic acid production and total volatile basic nitrogen during storage at 4°C for 19 days. Similarly, significant log reductions were observed in E. coli O157:H7 and Salmonella cultures when pork chops were treated with 6% NEW, while Yersinia enterocolitica required about 15% NEW for 2.5 logs reduction (Han et al., 2018). On the other hand, the color changes caused by NEW treatments were minor compared to sterilize deionized water on treated pork chops. Furthermore, high organic matter on the pork chops renders antimicrobial functioning of NEW and generates chloramines (Han et al., 2018). However, the application of NEW was more effective when supplemented with other physical and chemical treatment methods (Rahman et al., 2016; Oh et al., 2019) such as peroxyacetic acid (Moghassem Hamidi et al., 2021), nisin (Arevalos-Sánchez et al., 2012), UV-C radiation (Jemni et al., 2014), UV-A radiation (Jee and Ha, 2021), AEW (Jiménez-Pichardo et al., 2016), AlEW (Jadeja and Hung, 2014), ultrasound (Afari et al., 2016), modified atmosphere packaging (Posada-Izquierdo et al., 2014) and so on.
2.3. Plasma activated water
Several studies have reported the direct impact of gaseous plasma on food stuff for enhancing microbiological safety; however, it produced some negative effects on organoleptic characteristics such as color, firmness, etc. (Asghar et al., 2022; Roobab et al., 2022). To solve this problem, plasma-activated water (PAW) could be an alternative way to efficiently inactivate microorganisms and enhance food safety (Liao et al., 2020; Wang et al., 2021). PAW-a product of cold atmospheric plasma reacting with water- is a potential antimicrobial agent which has a rich diversity of highly reactive oxygen and nitrogen species. Chemical reactivity and energy transferred from gaseous plasmas to water without using any chemicals make it a clean-label alternative to conventional chemical disinfectants (Ostrikov et al., 2020). The working principle of PAW is based on the direct or indirect application of electrical discharge, which ionizes neutral gases in water (helium, argon, neon, nitrogen, oxygen, air) and forms excited/ionized atoms, ultraviolet rays, electric fields, and abundant reactive oxygen and nitrogen species (Figure 3). Based on the working principle, several plasma devices have been used such as plasma jets and dielectric barrier discharge.
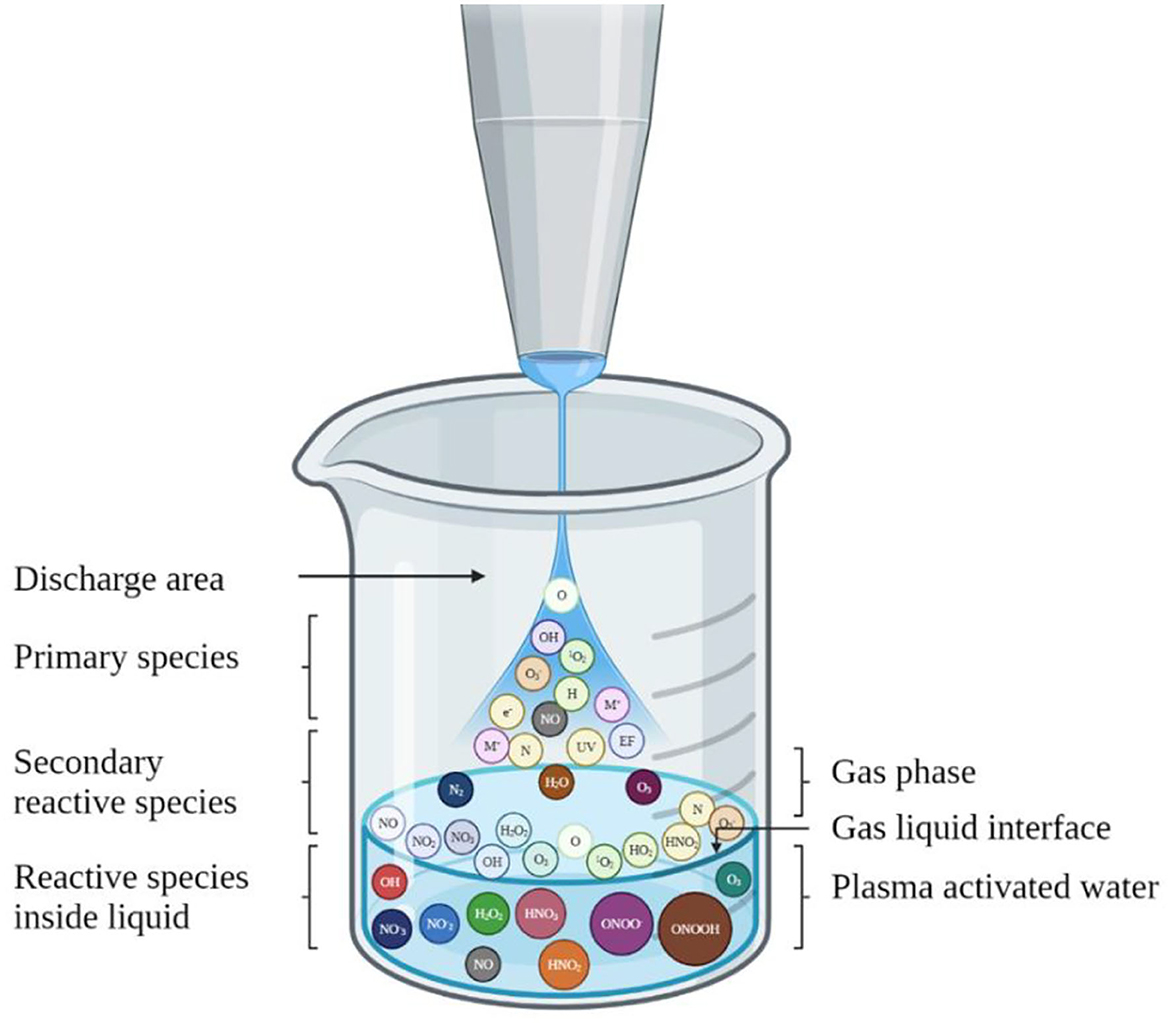
Figure 3. A schematic diagram of the formation of plasma active water via a conventional plasma jet.
Due to the advantages of environmental friendliness and green aspects PAW has gained attention for replacing conventional chemical disinfectant treatments, such as chlorine (Thirumdas et al., 2018). PAW has several reactive species, including hydrogen peroxide, peroxynitrite, nitric oxide, nitrates, and nitrite ions, which have strong antimicrobial efficiency against a wide range of microorganisms. These active species can destroy bacterial cell membranes and disrupt genetic material (DNA and protein) inducing damage to cell macromolecules (Liao et al., 2020). According to Royintarat et al. (2019), reactive species such as •OH promote peroxidation of the bacterial cell membrane, induce oxidative stress, alter molecular structures, disrupt pH hemostasis, and cause cell death (Figure 3).
2.3.1. Decontamination of meat
PAW from underwater plasma jet treatments has been used on tap water to produce reactive species for the decontamination of chicken. According to Royintarat et al. (2018), PAW tap water treatments reduced Salmonella Typhimurium by 0.54 and 0.13 log CFU/mL in muscle and skin samples, respectively. The bactericidal efficiency of PAW was reduced in chicken muscle and skin due to high polyunsaturated fatty acids (omega 6 and omega 3), which inhibited reactive species (Ambrozova et al., 2010). However, the efficiency could be improved by increasing plasma intensity to generate more reactive species. Besides, PAW treatments reduced 0.46 log CFU/mL of E. coli K12 and 0.33 logs CFU/mL of Staph. aureus; however, the combined treatments with ultrasound inactivated E. coli K12 by 1.33 logs CFU/mL and Staph. aureus by 0.83 logs CFU/mL in chicken meat. The synergistic interaction of combined PAW–ultrasound enhanced the porosity of muscle structure with a dramatic difference in quality characteristics. According to Royintarat et al. (2019), ultrasonication was the reason behind the increased penetration of PAW into the sample. Ultrasonication induced lipid oxidation of bacterial cell membranes and speeded the reaction between cell membrane unsaturated lipids and free radicals in PAW. Moreover, chicken muscle bacteria were more physically damaged as compared to chicken skin bacteria, indicating more organic material on the skin inhibits the formation of reactive species. The antimicrobial potential of PAW depends on the process parameters such as exposure time, targeted surface, microbial strain, reactive species, and so on (Perinban et al., 2019). Additional studies with different microorganisms' inactivation in several meat products with plasma-activated liquids have been mentioned in Table 3.
According to Zhao et al. (2020), 24 h PAW treatment achieved 3.1 logs reduction of surface bacteria and extended the shelf life of fresh beef for 4–6 days. A 30 min plasma activation contains 116 mg/L of hydrogen peroxide concentration in deionized water. However, the bactericidal impact of PAW decreased with the increase of the treatment interval time as microbial count increased during storage. Moreover, the treatment interval of more than 48 h was ineffective for controlling microbial growth in beef. Besides, excessive treatments could negatively affect the beef's physiochemical quality such as damage to the structural integrity. PAW thawing could be an effective approach to ensure microbial safety in meat.
Plasma-activated brine (plasma beam system at 20 kHz using air or nitrogen gas) was used to protect the jerky beef from L. innocua. Beef slices were cured in brine solutions [sodium nitrite (0–150 ppm) + sodium chloride (150 ppm) + sugar (100 ppm)] for about 18 h at 4°C then subjected to plasma treatment. Results showed a significant reduction in L. innocua population of about 0.5 log CFU/mL in brine and 0.8 CFU/mL when treated with plasma-activated brine (Inguglia et al., 2020). According to the authors, plasma technology could be used as an alternative nitrite source for meat curing with minimal impact on product quality characteristics. However, further optimization of plasma technology is required to ensure the microbiological safety of meat products.
2.4. Synergistic applications of meat decontamination
Ozonated water treatments can be combined with other clean-label ingredients such as lactic acid (Megahed et al., 2020) and sodium citrate (Zhang et al., 2020). Megahed et al. (2020) investigated the microbial killing capacity of ozonated water and ozone–lactic acid blend on Salmonella-contaminated chicken drumsticks using a multi-sequential application. The results showed that six consecutive soaking and spraying cycles with 8 ppm aqueous ozone reduced 7 logs Salmonella. However, the combination with 0.3% lactic acid increased the decontamination power of aqueous ozone with average differences of 0.3- and 0.2-log10/cm2 on the skin surface using soaking and spraying approaches, respectively. Lactic acid reduced the pH of ozonated water, which intensified the antimicrobial effect. According to the authors, spraying or soaking in low pH ozonated water (pH range from 2 to 3) caused cytoplasmic acidification. The resultant aqueous medium has stable ozone, which disrupted the energy and regulatory parameters of the host bacterial cell. Moreover, the accumulated free acid anions inactivate microorganisms through malfunctioning of microbial growth and survival. However, the antimicrobial impact of ozone also depends on the initial bacterial load on the chicken carcass.
Zhang et al. (2020) have found that sodium citrate and aqueous ozone combination with vacuum-packaged beef inhibited microbial growth more effectively as compared to high temperature, alkali, or salt stress. Moreover, the treated beef meat had the longest shelf life (21 days) compared to individual treatments. However, aqueous ozonation negatively impacted the water-holding capacity (purge loss) and tenderness. The oxidative modifications of tenderizing enzymes strengthen muscle fibers; however, the reduced proteolytic activity caused cross-linking of muscle proteins. Furthermore, myoglobin oxidation (met myoglobin) produced darker and redder beef steaks compared to the control. However, the addition of sodium citrate (antioxidant) exhibited a higher L* value (lightness), lower a* (redness) value, and lower metmyoglobin of ozonated beef steaks. High dosages and prolonged contact time used for the antimicrobial purpose could negatively affect the quality parameters of meat. Adequate precautions should be taken while applying high ozone doses to inactivate microorganisms.
Several other non-thermal combinations have been introduced for meat decontamination such as Moghassem Hamidi et al. (2021) combined NEW (100 mg/mL) with peroxyacetic acid (200 mg/mL) to reduce aerobic plate counts, psychrophilic plate count, Enterobacteriaceae, LAB, and Pseudomonas counts of chicken breast meat. Peroxyacetic acid with high-oxidizing potential affects bacterial cell integrity and modifies protein synthesis. Furthermore, it acidifies the meat surface and enhances the diffusion of the undissociated acids into the bacterial cell, which enhanced the antimicrobial efficacy of NEW. Veasey and Muriana (2016) suggested using EW for surface sanitizing of meat processing equipment (i.e., slicing blades) rather than a raw carcass. According to the author, the surface of raw meat (beef carcass) and ready-to-eat meat (frankfurters) contain high levels of organic material, which make HOCl ineffective as an antimicrobial. Although higher intensities of EW could inactivate microorganisms, they may produce undesirable sensory characteristics. Alternatively, a combined approach could be used to lower individual treatment intensities (Liao et al., 2020).
Similarly, the combination of ozonated water AEW, and AlEW showed promising antimicrobial potential against E. coli K12 in goat meat. According to Degala et al. (2020) the synergistic approach reduced 1.03 log CFU/mL in 8 min as compared to individual treatments of AEW, AlEW, and ozonated water, which caused 0.86; 0.74 and 0.53 log CFU/mL reduction in 10–12 min, respectively. In the same vein, Athayde et al. (2017) sprayed AEW, SAEW, and AlEW for 40 s with a pressure of 30 and 45 psi from 10 cm distance on pork loin with rotation at a 360° angle during application. The individual application of AEW and SAEW (compared to AlEW) increased carbonyl groups, shortly after application due to the molecular species (HCl, Cl2, HOCl), which produced carbonylated proteins. However, the addition of AlEW exerted an antioxidant effect and protected the meat protein from the adverse effects of AEW and SAEW. Moreover, the combination AlEW + AEW exhibited a strong antimicrobial effect against mesophilic and psychotropic bacteria without producing carbonyl compounds and oxidating thiol groups. However, the production of acidic compounds by lactic acid bacteria stabilized the pH values throughout the refrigerated storage (Athayde et al., 2017).
Other non-thermal combinations have been used against different microorganisms on various types of meat. The ultrasound + SAEW treatments significantly reduced enterobacteria, mesophilic bacteria, lactic acid bacteria, and psychotropic bacteria without affecting lipid and protein oxidation, shear force, anaerobic glycolysis, and muscle structure of chicken breast (Cichoski et al., 2019). However, the thick peptidoglycan layer of Staphylococcus spp. cells renders the antimicrobial potential of SAEW by preventing entry into the microbial cell. According to the author, large cavitation bubbles produced by ultrasonication generated much energy to rupture bacterial cytoplasmic membranes. Hence, ultrasonic cavitation helped SAEW to enter the cell. Furthermore, SAEW before and after immersion did not show mesophilic and psychotropic bacteria growth, hence, could be reused in the cooling process (Joyce et al., 2011). Furthermore, Liao et al. (2020) used PAW as a thawing media, which effectively inactivated the total bacteria, yeast, and molds in beef by 0.83–1.76 logs. Moreover, both PAW and SEAW maintained the quality characteristics such as texture, pH, and color as well as prevented lipid/protein oxidation and protein degradation in beef as compared to traditional thawing methods (air thawing, water thawing, microwave thawing) (Liao et al., 2020).
3. Conclusion
Non-thermal electrochemical activation of water is a relatively new and fast-emerging technology. Non-thermal technologies used in water affect the chemistry of reactive species and their interactions with microorganisms. Non-thermal activation of water allows the use of aqueous solutions at lower dose rates compared to conventional chemical disinfectants, preventing intoxication and adverse environmental impacts. In the meat industry, electrochemically activated solutions with increased antimicrobial capacity could be applied as an alternative to costly decontamination methods. However, a more detailed study is needed to understand the thermodynamics behind the electro-activation phenomena of water and the interaction of aqueous solutions with food and targeted microorganisms. Further, ozone, electrolyzed water, and cold plasma technologies are concentration-dependent as they can harm health if used beyond their recommended limits, while their electrochemically treated water can be applied at very high concentrations. For instance, ozone is allowed to be used at levels of 5 ppm; however, its activated water could be used at levels as high as 95 ppm. Therefore, non-thermal functional water at low levels has enough active components to ensure meat quality and safety. Furthermore, the synergistic combination of these non-thermal technologies could enhance the functionality of rinsing solutions. However, these treatments could further enhanced by taking into account the stability of activated water during storage conditions.
Author contributions
UR and RA: conceptualization. UR, GM, and AK: reviewed and refined tables and figures. UR and MR: writing—original draft preparation. UR and MS: writing—review and editing. MA, SS, X-AZ, and RA: supervision. All authors have read and agreed to the published version of the manuscript.
Funding
This work was funded by the Deanship of Scientific Research at Jouf University under grant No. DSR-2021-01-0347.
Acknowledgments
The first author is thankful to the China Scholarship Council for awarding research scholarship. Figures have been created with some icons from BioRender.com.
Conflict of interest
The authors declare that the research was conducted in the absence of any commercial or financial relationships that could be construed as a potential conflict of interest.
Publisher's note
All claims expressed in this article are solely those of the authors and do not necessarily represent those of their affiliated organizations, or those of the publisher, the editors and the reviewers. Any product that may be evaluated in this article, or claim that may be made by its manufacturer, is not guaranteed or endorsed by the publisher.
References
Afari, G. K., Hung, Y. C., King, C. H., and Hu, A. (2016). Reduction of E. coli O157: H7 and Salmonella Typhimurium DT 104 on fresh produce using an automated washer with near neutral electrolyzed (NEO) water and ultrasound. Food Control 63, 246–254. doi: 10.1016/j.foodcont.2015.11.038
Aider, M., Gnatko, E., Benali, M., Plutakhin, G., and Kastyuchik, A. (2012). Electro-activated aqueous solutions: theory and application in the food industry and biotechnology. Innovat. Food Sci. Emerg. Technol. 15, 38–49. doi: 10.1016/j.ifset.2012.02.002
Ali, M. M., Al-Mousawi, M. R., and Abidalmutalib Aljabawi, R. A. (2022). Studying the penetration ability of various pathogenic bacteria into raw beef meat surface and the antibacterial effect of ozonated water. J. Pure Appl. Microbiol. 16, 1252–1262. doi: 10.22207/JPAM.16.2.54
Ambrozova, G., Pekarova, M., and Lojek, A. (2010). Effect of polyunsaturated fatty acids on the reactive oxygen and nitrogen species production by raw 264.7 macrophages. Eur. J. Nutr. 49, 133–139. doi: 10.1007/s00394-009-0057-3
Aponte, M., Anastasio, A., Marrone, R., Mercogliano, R., Peruzy, M. F., and Murru, N. (2018). Impact of gaseous ozone coupled to passive refrigeration system to maximize shelf-life and quality of four different fresh fish products. Lwt 93, 412–419. doi: 10.1016/j.lwt.2018.03.073
Arevalos-Sánchez, M., Regalado, C., Martin, S. E., Domínguez-Domínguez, J., and García-Almendárez, B. E. (2012). Effect of neutral electrolyzed water and nisin on Listeria monocytogenes biofilms, and on listeriolysin O activity. Food Control. 24, 116–122. doi: 10.1016/j.foodcont.2011.09.012
Arya, R., Bryant, M., Degala, H. L., Mahapatra, A. K., and Kannan, G. (2018). Effectiveness of a low-cost household electrolyzed water generator in reducing the populations of E. coli K12 on inoculated beef, chevon, and pork surfaces. J. Food Process. Preserv. 42, 13636. doi: 10.1111/jfpp.13636
Asfahl, K., and Savin, M. (2012). Destruction of E. coli and broad-host-range plasmid dna in treated wastewater by dissolved ozone disinfection under laboratory and field conditions. Adv. Microbiol. 02, 1–7. doi: 10.4236/aim.2012.21001
Asghar, A., Rashid, M. H., Ahmed, W., Roobab, U., Inam-ur-Raheem, M., Shahid, A., et al. (2022). An in-depth review of novel cold plasma technology for fresh-cut produce. J. Food Process Preserv. 46, e16560. doi: 10.1111/jfpp.16560
Astorga, J. B., Hadinoto, K., Cullen, P., Prescott, S., and Trujillo, F. J. (2022). Effect of plasma activated water on the nutritional composition, storage quality and microbial safety of beef. LWT. 154, 112794. doi: 10.1016/j.lwt.2021.112794
Athayde, D. R., Flores, D. R. M., da Silva, J. S., Genro, A. L. G., Silva, M. S., Klein, B., et al. (2017). Application of electrolyzed water for improving pork meat quality. Food Res. Int. 100, 757–763. doi: 10.1016/j.foodres.2017.08.009
Bing, S., Zang, Y., Li, Y., Zhang, B., Mo, Q., Zhao, X., et al. (2022). A combined approach using slightly acidic electrolyzed water and tea polyphenols to inhibit lipid oxidation and ensure microbiological safety during beef preservation. Meat Sci. 183, 108643. doi: 10.1016/j.meatsci.2021.108643
Botta, C., Ferrocino, I., Cavallero, M. C., Riva, S., Giordano, M., and Cocolin, L. (2018). Potentially active spoilage bacteria community during the storage of vacuum packaged beefsteaks treated with aqueous ozone and electrolyzed water. Int. J. Food Microbiol. 266, 337–345. doi: 10.1016/j.ijfoodmicro.2017.10.012
Bridges, D. F., Rane, B., and Wu, V. C. H. (2018). The effectiveness of closed-circulation gaseous chlorine dioxide or ozone treatment against bacterial pathogens on produce. Food Control 91, 261–267. doi: 10.1016/j.foodcont.2018.04.004
Burfoot, D., Allen, V., Mulvey, E., Jewell, K., Harrison, D., and Morris, V. (2015). Reducing Campylobacter numbers on chicken carcasses using lactic acid in processing plants. Int. J. Food Sci. Technol. 50, 2451–2457. doi: 10.1111/ijfs.12912
Cadena, M., Kelman, T., Marco, M. L., and Pitesky, M. (2019). Understanding antimicrobial resistance (amr) profiles of salmonella biofilm and planktonic bacteria challenged with disinfectants commonly used during poultry processing. Foods 8, 275. doi: 10.3390/foods8070275
Cichoski, A. J., Flores, D. R. M., De Menezes, C. R., Jacob-Lopes, E., Zepka, L. Q., Wagner, R., et al. (2019). Ultrasound and slightly acid electrolyzed water application: an efficient combination to reduce the bacterial counts of chicken breast during pre-chilling. Int. J. Food Microbiol. 301, 27–33. doi: 10.1016/j.ijfoodmicro.2019.05.004
de Assis, D. C. S., da Silva, T. M. L., Brito, R. F., da Silva, L. C. G., Lima, W. G., and Brito, J. C. M. (2021). Shiga toxin-producing Escherichia coli (STEC) in bovine meat and meat products over the last 15 years in Brazil: A systematic review and meta-analysis. Meat Sci. 173, 108394.
Degala, H. L., Scott, J. R., Rico Espinoza, F. I., Mahapatra, A. K., and Kannan, G. (2020). Synergistic effect of ozonated and electrolyzed water on the inactivation kinetics of E. coli on goat meat. J. Food Saf. 40, 12740. doi: 10.1111/jfs.12740
Dixon, E., Rabanser, I., Dzieciol, M., Zwirzitz, B., Wagner, M., Mann, E., et al. (2019). Reduction potential of steam vacuum and high-pressure water treatment on microbes during beef meat processing. Food Cont. 106, 106728.
Dizengremel, P., Le Thiec, D., Hasenfratz-Sauder, M. P., Vaultier, M. N., Bagard, M., and Jolivet, Y. (2009). Metabolic-dependent changes in plant cell redox power after ozone exposure. Plant Biol. 11, 35–42. doi: 10.1111/j.1438-8677.2009.00261.x
Du, S., Zhang, Z., Xiao, L., Lou, Y., Pan, Y., and Zhao, Y. (2016). Acidic electrolyzed water as a novel transmitting medium for high hydrostatic pressure reduction of bacterial loads on shelled fresh shrimp. Front. Microbiol. 7, 1–12. doi: 10.3389/fmicb.2016.00305
Duan, D., Wang, H., Xue, S., Li, M., and Xu, X. (2017). Application of disinfectant sprays after chilling to reduce the initial microbial load and extend the shelf-life of chilled chicken carcasses. Food Control 75, 70–77. doi: 10.1016/j.foodcont.2016.12.017
Fathul Karamah, E., and Wajdi, N. (2018). Application of ozonated water to maintain the quality of chicken meat: effect of exposure time, temperature, and ozone concentration. E3S Web Confer. 67, 44. doi: 10.1051/e3sconf/20186704044
Garrido-Maestu, A., Fuciños, P., Azinheiro, S., Carvalho, C., Carvalho, J., and Prado, M. (2019). Specific detection of viable Salmonella Enteritidis by phage amplification combined with qPCR (PAA-qPCR) in spiked chicken meat samples. Food Cont. 99, 79–83.
Gil, M. I., Marín, A., Andujar, S., and Allende, A. (2016). Should chlorate residues be of concern in fresh-cut salads? Food Control 60, 416–421. doi: 10.1016/j.foodcont.2015.08.023
Hadi, M., Kanaan, G., Saad Abdullah, S., and Baghdad, I. (2021). Evaluation of aqueous Ozone as a method to combat multidrug-resistant S. aureus tainting cattle meat sold in Wasit marketplaces. Mansoura Vet. Med. J. 22, 117–122. doi: 10.21608/mvmj.2021.196988
Han, D., Hung, Y. C., and Wang, L. (2018). Evaluation of the antimicrobial efficacy of neutral electrolyzed water on pork products and the formation of viable but non-culturable (VBNC) pathogens. Food Microbiol. 73, 227–236. doi: 10.1016/j.fm.2018.01.023
Hawkins, J. L., Vimini, B., Schwarz, J. G., Nichols, P., and Parveen, S. (2016). Application of antimicrobial agents via commercial spray cabinet to inactivate Salmonella on skinless chicken meat. J. Food Protect. 79, 569–573. doi: 10.4315/0362-028X.JFP-15-248
Heir, E., Solberg, L. E., Jensen, M. R., Skaret, J., Grøvlen, M. S., and Holck, A. L. (2022). Improved microbial and sensory quality of chicken meat by treatment with lactic acid, organic acid salts and modified atmosphere packaging. Int J Food Microbiol. 362, 109498.
Hernández-Pimentel, V. M., Regalado-González, C., Nava-Morales, G. M., Meas-Vong, Y., Castañeda-Serrano, M. P., and García-Almendárez, B. E. (2020). Effect of neutral electrolyzed water as antimicrobial intervention treatment of chicken meat and on trihalomethanes formation. J. Appl. Poult. Res. 29, 622–635. doi: 10.1016/j.japr.2020.04.001
Inguglia, E. S., Oliveira, M., Burgess, C. M., Kerry, J. P., and Tiwari, B. K. (2020). Plasma-activated water as an alternative nitrite source for the curing of beef jerky: influence on quality and inactivation of Listeria innocua. Innovat. Food Sci. Emerg. Technol. 59, 102276. doi: 10.1016/j.ifset.2019.102276
Iram, A., Wang, X., and Demirci, A. (2021). Electrolyzed oxidizing water and its applications as sanitation and cleaning agent. Food Eng. Rev. 13, 411–427. doi: 10.1007/s12393-021-09278-9
Ito, K., Inoue, S., Hiraku, Y., and Kawanishi, S. (2005). Mechanism of site-specific DNA damage induced by ozone. Mutat. Res. Genet. Toxicol. Environ. Mutagen. 585, 60–70. doi: 10.1016/j.mrgentox.2005.04.004
Jadeja, R., and Hung, Y. C. (2014). Efficacy of near neutral and alkaline pH electrolyzed oxidizing waters to control E. coli O157: H7 and Salmonella Typhimurium DT 104 from beef hides. Food Control 41, 17–20. doi: 10.1016/j.foodcont.2013.12.030
Jee, D. Y., and Ha, J. W. (2021). Synergistic interaction of tap water-based neutral electrolyzed water combined with UVA irradiation to enhance microbial inactivation on stainless steel. Food Res. Int. 150, 110773. doi: 10.1016/j.foodres.2021.110773
Jemni, M., Gómez, P. A., Souza, M., Chaira, N., Ferchichi, A., Otón, M., et al. (2014). Combined effect of UV-C, ozone and electrolyzed water for keeping overall quality of date palm. Lwt 59, 649–655. doi: 10.1016/j.lwt.2014.07.016
Jiménez-Pichardo, R., Regalado, C., Castaño-Tostado, E., Meas-Vong, Y., Santos-Cruz, J., and García-Almendárez, B. E. (2016). Evaluation of electrolyzed water as cleaning and disinfection agent on stainless steel as a model surface in the dairy industry. Food Control 60, 320–328. doi: 10.1016/j.foodcont.2015.08.011
Joyce, E., Al-Hashimi, A., and Mason, T. J. (2011). Assessing the effect of different ultrasonic frequencies on bacterial viability using flow cytometry. J. Appl. Microbiol. 110, 862–870. doi: 10.1111/j.1365-2672.2011.04923.x
Kalchayanand, N., Worlie, D., and Wheeler, T. (2019). A novel aqueous ozone treatment as a spray chill intervention against E. coli o157:h7 on surfaces of fresh beef. J. Food Protect. 82, 1878. doi: 10.4315/0362-028X.JFP-19-093
Kanaan, M. H. G. (2018). Antibacterial effect of ozonated water against methicillin-resistant S. aureus contaminating chicken meat in Wasit Province, Iraq. Vet. World 11, 1445–1453. doi: 10.14202/vetworld.2018.1445-1453
Kang, C., Xiang, Q., Zhao, D., Wang, W., Niu, L., and Bai, Y. (2019). Inactivation of Pseudomonas deceptionensis CM2 on chicken breasts using plasma-activated water. J. Food Sci. Technol. 56, 4938–4945. doi: 10.1007/s13197-019-03964-7
Kocharunchitt, C., Mellefont, L., Bowman, J. P., and Ross, T. (2020). Application of chlorine dioxide and peroxyacetic acid during spray chilling as a potential antimicrobial intervention for beef carcasses. Food Microbiol. 87, 103355.
Liao, X., Su, Y., Liu, D., Chen, S., Hu, Y., Ye, X., et al. (2018). Application of atmospheric cold plasma-activated water (PAW) ice for preservation of shrimps (Metapenaeus ensis). Food Control 94, 307–314. doi: 10.1016/j.foodcont.2018.07.026
Liao, X., Xiang, Q., Cullen, P. J., Su, Y., Chen, S., Ye, X., et al. (2020). Plasma-activated water (PAW) and slightly acidic electrolyzed water (SAEW) as beef thawing media for enhancing microbiological safety. Lwt 117, 108649. doi: 10.1016/j.lwt.2019.108649
Liao, X., Xuan, X., Li, J., Suo, Y., Liu, D., Ye, X., et al. (2017). Bactericidal action of slightly acidic electrolyzed water against E. coli and S. aureus via multiple cell targets. Food Control 79, 380–385. doi: 10.1016/j.foodcont.2017.03.050
Megahed, A., Aldridge, B., and Lowe, J. (2020). Antimicrobial efficacy of aqueous ozone and ozone–lactic acid blend on salmonella-contaminated chicken drumsticks using multiple sequential soaking and spraying approaches. Front. Microbiol. 11, 1–11. doi: 10.3389/fmicb.2020.593911
Moghassem Hamidi, R., Shekarforoush, S. S., Hosseinzadeh, S., and Basiri, S. (2021). Evaluation of the effect of neutral electrolyzed water and peroxyacetic acid alone and in combination on microbiological, chemical, and sensory characteristics of poultry meat during refrigeration storage. Food Sci. Technol. Int. 27, 499–507. doi: 10.1177/1082013220968713
Murray, K., Wu, F., Shi, J., Jun Xue, S., and Warriner, K. (2017). Challenges in the microbiological food safety of fresh produce: limitations of post-harvest washing and the need for alternative interventions. Food Qual. Saf. 1, 289–301. doi: 10.1093/fqsafe/fyx027
Nkosi, D. V., Bekker, J. L., and Hoffman, L. C. (2021). The use of organic acids (Lactic and acetic) as a microbial decontaminant during the slaughter of meat animal species: A review. Foods. 10(10), 2293.
Oh, D. H., Khan, I., and Tango, C. N. (2019). Hurdle enhancement of electrolyzed water with other techniques. Electrolyzed Water Food Fund. Appl. 38, 231–260. doi: 10.1007/978-981-13-3807-6_10
Ostrikov, K., Zhou, R., Zhou, R., Wang, P., Xian, Y., Mai-Prochnow, A., et al. (2020). Plasma-activated water: generation, origin of reactive species and biological applications. J. Phys. D Appl. Phys. 53, 303001. doi: 10.1088/1361-6463/ab81cf
Pandiselvam, R., Kaavya, R., Jayanath, Y., Veenuttranon, K., Lueprasitsakul, P., Divya, V., et al. (2020). Ozone as a novel emerging technology for the dissipation of pesticide residues in foods: a review. Trends Food Sci. Technol. 97, 38–54. doi: 10.1016/j.tifs.2019.12.017
Pandiselvam, R., Sunoj, S., Manikantan, M. R., Kothakota, A., and Hebbar, K. B. (2017). Application and kinetics of ozone in food preservation. Ozone Sci. Eng. 39, 115–126. doi: 10.1080/01919512.2016.1268947
Perinban, S., Orsat, V., and Raghavan, V. (2019). Non-thermal plasma–liquid interactions in food processing: a review. Compreh. Rev. Food Sci. Food Saf. 18, 1985–2008. doi: 10.1111/1541-4337.12503
Posada-Izquierdo, G. D., Pérez-Rodríguez, F., López-Gálvez, F., Allende, A., Gil, M. I., and Zurera, G. (2014). Modeling growth of E. coli O157: H7 in fresh-cut lettuce treated with neutral electrolyzed water and under modified atmosphere packaging. Int. J. Food Microbiol. 177, 1–8. doi: 10.1016/j.ijfoodmicro.2013.12.025
Qian, J., Zhuang, H., Nasiru, M. M., Muhammad, U., Zhang, J., and Yan, W. (2019). Action of plasma-activated lactic acid on the inactivation of inoculated Salmonella Enteritidis and quality of beef. Innovat. Food Sci. Emerg. Technol. 57, 102196. doi: 10.1016/j.ifset.2019.102196
Rahman, S., Khan, I., and Oh, D. H. (2016). Electrolyzed water as a novel sanitizer in the food industry: current trends and future perspectives. Compreh. Rev. Food Sci. Food Saf. 15, 471–490. doi: 10.1111/1541-4337.12200
Ribeiro, C. M., Stefani, L. M., Lucheis, S. B., Okano, W., Cruz, J. C. M., Souza, G. V., et al. (2018). Methicillin-resistant Staphylococcus aureus in poultry and poultry meat: A meta-analysis. J. Food Protect. 81, 1055–1062.
Roobab, U., Chacha, J. S., Abida, A., Rashid, S., Muhammad Madni, G., Lorenzo, J. M., et al. (2022). Emerging trends for nonthermal decontamination of raw and processed meat: ozonation, high-hydrostatic pressure and cold plasma. Foods. 11, 2173.
Roobab, U., Khan, A. W., Lorenzo, J. M., Arshad, R. N., Chen, B. R., Zeng, X. A., et al. (2021). A systematic review of clean-label alternatives to synthetic additives in raw and processed meat with a special emphasis on high-pressure processing (2018–2021). Food Res. Int. 150, 110792.
Royintarat, T., Choi, E. H., Boonyawan, D., Seesuriyachan, P., and Wattanutchariya, W. (2020). Chemical-free and synergistic interaction of ultrasound combined with plasma-activated water (PAW) to enhance microbial inactivation in chicken meat and skin. Sci. Rep. 10, 1–14. doi: 10.1038/s41598-020-58199-w
Royintarat, T., Choi, E. H., Seesuriyachan, P., and Wattanutchariya, W. (2019). “Ultrasound-assisted plasma-activated water for bacterial inactivation in poultry industry,” in Proceedings of the IEEE International Conference on Industrial Technology (Cham: Springer), 1028–1032. doi: 10.1109/ICIT.2019.8755067
Royintarat, T., Wattanutchariya, W., Boonyawan, D., Seesuriyachan, P., and Meerak, J. (2018). “Inactivation of Salmonella Typhimurium in chicken meat by plasma-activated water,” in Proceedings of Ph.D. Symposium on Industrial Engineering, 1–3.
Rudolphi-Skórska, E., Filek, M., and Zembala, M. (2017). The Effects of the structure and composition of the hydrophobic parts of phosphatidylcholine-containing systems on phosphatidylcholine oxidation by ozone. J. Memb. Biol. 250, 493–505. doi: 10.1007/s00232-017-9976-8
Sá Júnior, P. L. S., Silva, L. J., Andrade, H. A., Maciel, M. I. S., Shinohara, N. K. S., Gloria, M. B. A., et al. (2021). Optimization of mechanically separated meat washing cycles and of corn starch addition in saramunete (Pseudupeneus maculatus) sausages. J. Food Process. Preserv. 45, e16093.
Sallam, K. I., Abd-Elghany, S. M., Hussein, M. A., Imre, K., Morar, A., Morshdy, A. E., et al. (2020). Microbial decontamination of beef carcass surfaces by lactic acid, acetic acid, and trisodium phosphate sprays. BioMed Res. Int. 2020, 2314358. doi: 10.1155/2020/2324358
Sheng, L., Hanrahan, I., Sun, X., Taylor, M. H., Mendoza, M., and Zhu, M. J. (2018). Survival of Listeria innocua on Fuji apples under commercial cold storage with or without low dose continuous ozone gaseous. Food Microbiol. 76, 21–28. doi: 10.1016/j.fm.2018.04.006
Sheng, X., Shu, D., Tang, X., and Zang, Y. (2018). Effects of slightly acidic electrolyzed water on the microbial quality and shelf life extension of beef during refrigeration. Food Sci. Nutr. 6, 1975–1981. doi: 10.1002/fsn3.779
Sierra, B. G., Deicy, V. R., Sandra, C., Mitra, D., and Adriana, S. (2022). Neutral electrolyzed water in chillers: a viable option in the microbiological disinfection of giblets chicken. Ener. Nexus. 7, 100096. doi: 10.1016/j.nexus.2022.100096
Smith, J., Corkran, S., McKee, S. R., Bilgili, S. F., and Singh, M. (2015). Evaluation of post-chill applications of antimicrobials against Campylobacter jejuni on poultry carcasses. J. Appl. Poult. Res. 24, 451–456. doi: 10.3382/japr/pfv046
Thirumdas, R., Kothakota, A., Annapure, U., Siliveru, K., Blundell, R., Gatt, R., et al. (2018). Plasma activated water (PAW): chemistry, physico-chemical properties, applications in food and agriculture. Trends Food Sci. Technol. 77, 21–31. doi: 10.1016/j.tifs.2018.05.007
Thomas, K. M., de Glanville, W. A., Barker, G. C., Benschop, J., Buza, J. J., Cleaveland, S., et al. (2020). Prevalence of Campylobacter and Salmonella in African food animals and meat: A systematic review and meta-analysis. Int J Food Microbiol. 315, 108382.
Torres-Rosales, E., Rivera-Garcia, A., Rosario-Perez, P. J., Ramirez-Orejel, J. C., Paez-Esquiliano, D., Martinez-Vidal, S., et al. (2020). Application of neutral electrolyzed water on pork chops and its impact on meat quality. Sci. Rep. 10, 1–10. doi: 10.1038/s41598-020-76931-4
Ünal, D. (2017). Effects of Ozone Treatment Ve Oxygen Scavenger on the Quality of ModiFiEd Atmosphere Packaged ChiCken Meat. Ankara: Ankara University.
USDA. (2022). Safe and Suitable Ingredients Used in the Production of Meat, Poultry, and Egg Products. New York, NY: USDA. p. 1–75. Available online at: http://www.fsis.usda.gov/wps/wcm/connect/bab10e09-aefa-483b-8be8-809a1f051d4c/7120.1.pdf (accessed October 24, 2022).
Vargas, D. A., Casas, D. E., Chávez-Velado, D. R., Jiménez, R. L., Betancourt-Barszcz, G. K., Randazzo, E., et al. (2021). In-plant intervention validation of a novel ozone generation technology (bio-safe) compared to lactic acid in variety meats. Foods 10, 2106. doi: 10.3390/foods10092106
Veasey, S., and Muriana, P. M. (2016). Evaluation of electrolytically-generated hypochlorous acid (“electrolyzed water”) for sanitation of meat and meat-contact surfaces. Foods 5, 1–15. doi: 10.3390/foods5020042
Walsh, R. J., White, B., Hunker, L., Leishman, O., Hilgren, J., and Klein, D. (2018). Peracetic acid and hydrogen peroxide post-dip decay kinetics on red meat and poultry. Food Protect. Trends. 38, 96–103.
Wang, H., Duan, D., Wu, Z., Xue, S., Xu, X., and Zhou, G. (2019). Primary concerns regarding the application of electrolyzed water in the meat industry. Food Control 95, 50–56. doi: 10.1016/j.foodcont.2018.07.049
Wang, H., Qi, J., Duan, D., Dong, Y., Xu, X., and Zhou, G. (2018). Combination of a novel designed spray cabinet and electrolyzed water to reduce microorganisms on chicken carcasses. Food Control 86, 200–206. doi: 10.1016/j.foodcont.2017.11.027
Wang, J., Han, R., Liao, X., and Ding, T. (2021). Application of plasma-activated water (PAW) for mitigating methicillin-resistant S. aureus (MRSA) on cooked chicken surface. Lwt 137, 110465. doi: 10.1016/j.lwt.2020.110465
Xuan, X. T., Wang, M. M., Ahn, J., Ma, Y. N., Chen, S. G., Ye, X. Q., et al. (2016). Storage stability of slightly acidic electrolyzed water and circulating electrolyzed water and their property changes after application. J. Food Sci. 81, E610–E617. doi: 10.1111/1750-3841.13230
Yong, H. I., Park, J., Kim, H. J., Jung, S., Park, S., Lee, H. J., et al. (2018). An innovative curing process with plasma-treated water for production of loin ham and for its quality and safety. Plasma Process. Polym. 15, 1–9. doi: 10.1002/ppap.201700050
Youn, S. Y., Jeong, O. M., Choi, B. K., Jung, S. C., and Kang, M. S. (2017). Comparison of the antimicrobial and sanitizer resistance of Salmonella isolates from chicken slaughter processes in Korea. J. Food Sci. 82, 711–717. doi: 10.1111/1750-3841.13630
Zhang, L., Wang, Z., Song, Y., Li, M., and Yu, Q. (2020). Quality of vacuum packaged beef as affected by aqueous ozone and sodium citrate treatment. Int. J. Food Propert. 23, 1475–1489. doi: 10.1080/10942912.2020.1814322
Keywords: ozone, electrolyzed water, cold plasma, meat quality and safety, Salmonella, Escherichia coli, Staphylococcus aureus, Listeria
Citation: Roobab U, Madni GM, Ranjha MMAN, Khan AW, Selim S, Almuhayawi MS, Samy M, Zeng X-A and Aadil RM (2023) Applications of water activated by ozone, electrolysis, or gas plasma for microbial decontamination of raw and processed meat. Front. Sustain. Food Syst. 7:1007967. doi: 10.3389/fsufs.2023.1007967
Received: 31 July 2022; Accepted: 06 January 2023;
Published: 10 February 2023.
Edited by:
Mahendran Radhakrishnan, Indian Institute of Food Processing Technology, IndiaReviewed by:
Keith Warriner, University of Guelph, CanadaMohan Naik, Karunya Institute of Technology and Sciences, India
Copyright © 2023 Roobab, Madni, Ranjha, Khan, Selim, Almuhayawi, Samy, Zeng and Aadil. This is an open-access article distributed under the terms of the Creative Commons Attribution License (CC BY). The use, distribution or reproduction in other forums is permitted, provided the original author(s) and the copyright owner(s) are credited and that the original publication in this journal is cited, in accordance with accepted academic practice. No use, distribution or reproduction is permitted which does not comply with these terms.
*Correspondence: Samy Selim, c2FiZHVsc2FsYW1AanUuZWR1LnNh; Xin-An Zeng,
eGF6ZW5nQHNjdXQuZWR1LmNu; Rana Muhammad Aadil,
bXVoYW1tYWQuYWFkaWxAdWFmLmVkdS5waw==