- 1Department of Agronomy and Plant Genetics, University of Minnesota, St. Paul, MN, United States
- 2Department of Soil, Water, and Climate, University of Minnesota, St. Paul, MN, United States
Nitrate (-N) leaching into groundwater as a result of high nitrogen (N) fertilizer rates to annual crops presents human health risks and high costs associated with water treatment. Leaching is a particularly serious concern on sandy soils overlying porous bedrock. Intermediate wheatgrass (IWG) [Thinopyrum intermedium (Host.) Barkw. & D.R. Dewey], is a perennial grass that is being bred to produce agronomically and economically viable grain, which is commercially available as Kernza®. Intermediate wheatgrass is a low-input crop has the potential to produce profitable grain and biomass yields while reducing -N leaching on sandy soils compared with common annual row crop rotations in the Upper Midwest. We compared grain yields, biomass yields, soil solution -N concentration, soil extractable -N, soil water content, and root biomass under IWG and a conventionally managed corn (Zea mays L.) and soybean [Glycine max (L.) Merr.] rotation for 3 years on a Verndale sandy loam in Central Minnesota. Mean soil solution -N was 77–96% lower under IWG than the annual crop rotation. Soil water content was greater under annuals compared to IWG early in the growing season, suggesting greater water use by IWG during this time. Interactions between crop treatments and depth were observed for soil water content in Year 3. Root biomass from 0 to 60 cm below the soil surface was five times greater beneath IWG compared to soybean, which may explain differences in soil extractable and solution -N among crops. With irrigation on coarse structured soils, IWG grain yields were 854, 434, and 222 kg ha−1 for Years 1–3 and vegetative biomass averaged 4.65 Mg ha−1 yr−1; comparable to other reports on heavier soils in the region. Annual crop grain yields were consistent with local averages. These results confirm that IWG effectively reduces soil solution -N concentrations even on sandy soils, supporting its potential for broader adoption on land vulnerable to -N leaching.
Introduction
Water quality in the Upper Midwest is threatened by the intensive management practices used in annual cropping systems, including tillage and fertilizer application that lead to nutrient losses and water contamination through leaching and runoff (Randall and Mulla, 2001; Dinnes et al., 2002; Feyereisen et al., 2006; Erisman et al., 2013). While annual commodity crops like corn provide the potential for high economic return, nutrient losses cause eutrophication and hypoxia in surface waters and contamination of groundwater, posing significant risks to human health (Ward et al., 2010, 2018; Brender et al., 2013). Impacts are often high where shallow aquifers and sandy soils make drinking water sources vulnerable to contamination. This leads to additional water treatment costs of over $5 million for some counties (Keeler et al., 2016). In Southeast Minnesota, for example, conversion of grassland to agriculture is expected to cause a 45% increase in private wells exceeding 10 ppm -N, resulting in between $700,000 and $12,000,000 in associated costs over a 20-year period (Keeler and Polasky, 2014). New alternative cropping systems that provide economic returns comparable to those of annual systems and which effectively reduce nutrient losses will be essential for protecting drinking water sources in the future.
Replacing annual crops with perennials has the potential to help reduce leaching to groundwater and provide other ecosystem services (Asbjornsen et al., 2014; Ferchaud and Mary, 2016). Cropping systems that include perennial grasses for conservation, forage, and biofuel production have lower leaching losses than corn-soybean systems, largely because perennial grasses have greater root biomass that extends deeper into the soil, increasing N recovery and reducing leaching (Culman et al., 2013b; Pugesgaard et al., 2015; Ferchaud and Mary, 2016). Deep roots may be particularly important in reducing leaching since they can expand the total volume of soil from which -N is taken up, and because is highly mobile and more prone to leaching from deep soil horizons (Maeght et al., 2013). losses in the subsurface drainage water for a corn-soybean system were about 37 times higher than from a Conservation Reserve Program (CRP) planting dominated by perennial grasses (Randall et al., 1997). This reduction was attributed to the greater season-long evapotranspiration (ET) that resulted in less drainage and greater uptake and/or immobilization of N. In that study, average concentrations in the water during the flow period were 24 mg/L for the corn-soybean rotation and 2 mg/L for the perennial grass CRP (Randall et al., 1997). Although plantings that include perennial grasses are effective at reducing leaching, a lack of economic return has prevented their large-scale adoption in Midwestern agricultural landscapes.
Intermediate wheatgrass (IWG), [Thinopyrum intermedium (Host.) Barkw. & D.R. Dewey] is a perennial cool-season grass being domesticated to produce a grain marketed as Kernza® (DeHaan et al., 2018) with the first commercial variety, “MN-Clearwater,” released in 2020 (Bajgain et al., 2020). The crop has potential to provide economic return for producers (Hunter et al., 2020a,b; Law et al., 2022) while reducing leaching compared to corn (Jungers et al., 2019). Intermediate wheatgrass initiates growth earlier in the season than warm-season forage and bioenergy grasses and is thus better able to reduce -N losses early in the season (Jungers et al., 2019) when losses are typically the highest in the Upper Midwest (Randall and Mulla, 2001; Crews and Peoples, 2005). Vegetative regrowth following IWG grain harvest helps reduce post-harvest nitrate losses and erosion late into the fall.
One potential mechanism by which IWG can reduce leaching compared to annual crops is related to water demand. Although total growing season ET and drainage were similar between IWG and corn, soil water content was lower under IWG compared to corn and switchgrass at 50 and 100 cm depths (Jungers et al., 2019), suggesting that soil moisture may be stored in other regions of the soil profile. Compared to annual wheat (Triticum aestivum L.), IWG had lower soil moisture up to a depth of 70–100 cm, which was associated with -N leaching reductions of up to 86% (Culman et al., 2013b). The distribution of IWG root biomass and its effects on soil water content throughout the soil profile are largely unknown.
Reductions in leaching beneath IWG compared to annual crops can also be related to differences in nitrogen fertilization regimes and associated losses of N in the form of soluble -N in the soil water. Soil solution increased from 0.1 to 0.3 mg L−1 when IWG was fertilized with 120 kg N ha−1 compared to an unfertilized control, yet this was still lower than the 24.0 mg L−1 measured beneath corn fertilized at 160 kg ha−1 (Jungers et al., 2019). Integrating legumes such as soybean into annual crop rotations can limit N fertilizing needs, yet the effects of legume crops in rotation on -N leaching compared to IWG are unknown.
Our objective was to assess the potential of IWG grain production to reduce -N leaching compared to an annual soybean-corn-soybean rotation on irrigated sandy soil by measuring soil solution -N concentration and soil water content. We hypothesized that soil water -N concentrations and soil water content would be lower under IWG, and that this would be related to increased root biomass and rooting depth of IWG compared to corn and soybean. Crop yields and vegetative biomass were measured to assess potential profitability.
Methods
Site description
Field research was conducted from 2018 to 2020 at the Central Lakes Community College in Staples, MN, USA (lat. 46.38, long. −94.80). The soil type was a Verndale sandy loam (Typic Argiudoll). The soil contains 1–1.7% organic matter, is excessively well-drained, and is considered low fertility potential (USDA-NRCS, 2021). Local climate data are reported in Table 1. Plots had previously been planted to a corn-soybean rotation followed by barley fertilized with 40 kg N ha−1 applied in spring prior to IWG planting in 2017. Baseline soil samples from 0 to 30 cm were collected by block in the fall of 2017. Soil extractable nitrogen was 10.0 mg kg soil−1 for -N and 3.9 mg kg soil−1 for ammonium (-N). Soil phosphorus (P) and potassium (K) concentrations were 9.13 and 72.21 ppm, respectively.
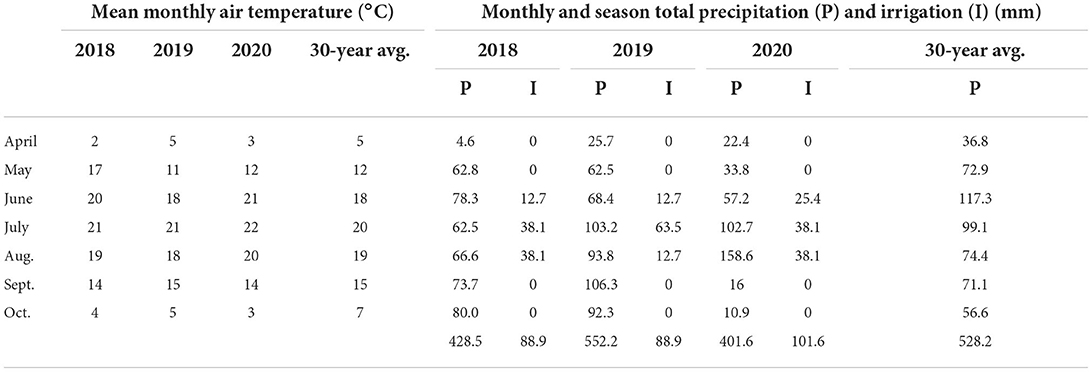
Table 1. Average air temperature, precipitation, irrigation, and 30-year averages for each month of the growing season in Staples, MN.
Experimental design
Treatments were applied in a randomized block design with two cropping systems replicated once in each of six blocks for a total of twelve plots. Plots were 4.11 by 9.14 m (13.5 by 30 ft.). The annual cropping system was a soybean-corn-soybean rotation. The perennial system was IWG. Soybeans were planted as the first phase of the soybean-corn rotation in May 2018, followed by corn in May 2019 and soybean again as the third phase in June 2020. Corn and soybeans were seeded in 75 cm rows at rates of 346,000 and 84,000 seeds ha−1, respectively, with four rows per plot. The corn variety was Organic Viking O.84-95UP Seed Corn and the soybean was Organic MN0810CN.
An improved population of IWG bred for increased grain yield was used in this study. The population came from the fourth cycle of selection by Land Institute (Salina, KS) and was seeded at a rate of 15 kg ha−1. The IWG was seeded in 15-cm rows with 20 rows per plot on 20 August 2017. Intermediate wheatgrass was fertilized with urea at rates of 80, 100, and 100 kg N ha−1 in May 2018, 2019, and 2020, respectively. Urea was split-applied to corn at 140 and 80 kg Nha−1 in May and June 2019. Soybean was not fertilized. Weed pressure was low and when present, weeds were manually removed in all plots. The experiment was irrigated with a linear irrigation system with events based on ET estimates and water demand for the annual crop. The fields received 89 mm of irrigation water over five events in 2018, 89 mm over seven events in 2019, and 102 mm over eight events in 2020. Dates of irrigation events are in Figure 1. Each individual irrigation event resulted in an application of 13 mm of water with the exception of 7/16/2018 and 8/22/2018, which received 25 mm.
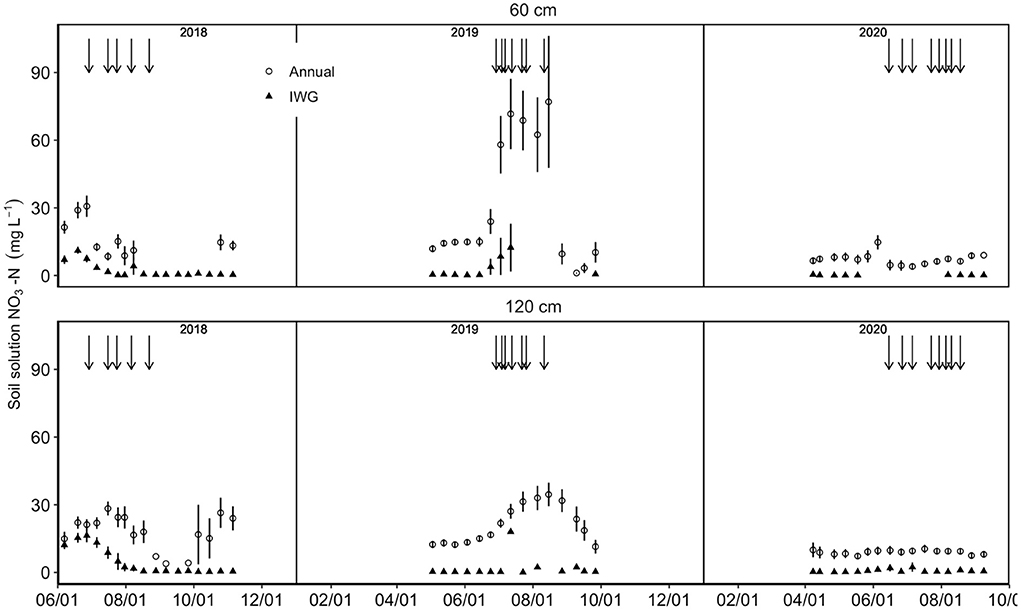
Figure 1. Mean soil solution -N from lysimeters 60 and 120 cm below annual crops (soybean in 2018 and 2020, corn in 2019) and intermediate wheatgrass (IWG) sampled during the growing season in 2018, 2019, and 2020. Points are means from two lysimeters per treatment replicate and six replicates (n = 12), while error bars indicate standard error of the mean. Arrows indicate dates of irrigation events.
Soil fertility and extractable N
Soil was sampled at four depth intervals (0–15, 15–30, 30–45, and 45–60 cm) in June 2019 and October 2020 and analyzed for organic matter, K, P, pH, and extractable -N and -N. Samples were taken from eight cores in each plot and aggregated by depth, stored in a cooler when transported, and kept refrigerated until analyzed or processed for shipping. All soil analyses except extractable N were conducted by Agvise Laboratories (Benson, MN; www.agvise.com). Agvise samples were oven-dried prior to shipping. Soil extractable N was determined by extraction with a 2 M KCl solution, where 40 ml solution was added to 10 g fresh soil followed by 1 h shaking (Culman et al., 2013a). Extractions were performed within 48 h of field collection. -N and analyses of the extractions were performed at the UMN Research Analytical Lab. Method details can be found at http://ral.cfans.umn.edu/tests-analysis/soil-analysis.
Crop yields
Crop yields were estimated each year from 2018 to 2020. Samples were taken in August of each year when the IWG had reached physiological maturity from two 76 by 76 cm quadrats with a total area of 0.58 m2. Seed heads were removed from all IWG plants within the quadrat by cutting approximately 2 cm below the basal spikelet. After seed heads were removed, all remaining IWG biomass was harvested to an 8 cm stubble height. The remaining biomass was mechanically harvested and removed from the plots following quadrat sampling.
Biomass and seed heads were dried at 35°C for 72 h or until constant mass before being weighed. Grain was removed from spikes using a Wintersteiger LD 350 laboratory thresher (Wintersteiger; www.wintersteiger.com/us/Plant-Breeding-and-Research). Grain was separated from the chaff and other debris by hand-sieving and with a fractionating aspirator (Carter-Day International, Inc.; http://www.carterday.com).
Corn and soybean yields were determined by harvesting a subsection of the middle two rows of each plot. For corn, two 2-m sections of rows were cut from each corn plot. The number of corn stalks cut was recorded for each plot. All ears from the cut stalks were collected, dried (35°C for 72 h), shelled, and both cobs and kernels were weighed. Three stalks from each row section were randomly selected, dried, and weighed to estimate stover mass. Soybean yields were determined by harvesting whole plants from two 1-m sections of rows from each plot, followed by drying, threshing, and weighing. Following harvest for yield measurement, the remaining corn and soybean plants were mechanically harvested and removed from the plots.
Root biomass
Root biomass samples were taken in September 2020 with two 5-cm diameter manual push cores per plot at depths of 0–15, 15–30, 30–45, and 45–60 cm. Roots were separated from soil and debris using a hydropneumatic elutriation system (Smucker et al., 1982), then removed manually from sieves using tweezers. Due to the difficulty of distinguishing live from dead roots, no effort was made to separate them. Roots were dried at 35°C for 72 h. Samples were checked after drying for any remaining sand and debris, which was removed before weighing.
Soil solution -N concentration
Soil solution -N concentrations were determined by collecting soil solution samples with suction lysimeters. Lysimeters consisted of a porous ceramic end cap, a PVC tube, and an airtight rubber stopper (Jungers et al., 2019). Two pairs of 60 and 120 cm lysimeters were installed in each plot. Samples were collected every 7–10 days from April to October each year and analyzed by depth for soil solution -N concentration using a colorimetric assay with a HACH DR 6000 spectrophotometer (Hach, https://www.hach.com).
Soil water content
Soil water content was measured on four dates in 2019 (June 17, July 19, August 21, October 31) and six dates in 2020 (May 12, June 23, July 15, August 5, September 1, and September 25) at 10, 20, 30, 40, 60, and 100 cm using a Delta-T Devices PR2/6 Probe (Delta-T Devices, 2021).
Statistical analysis
Analysis of variance (ANOVA) was conducted using mixed effects models to explain variation in soil water -N concentration, soil water content, soil extractable -N, root biomass, and crop yields. Predictor variables for the ANOVA were cropping system, depth (for soil variables), and their interaction. Years were analyzed separately because the annual crop varied. Cropping system was treated as a categorical variable; depth was treated as a categorical variable for root biomass and soil extractable -N. Soil solution -N concentrations from the 60 and 120 cm depths were not statistically different, based on preliminary statistics, and thus were averaged for the analysis. The treatment applied to the nearest neighboring plot was included in the model as a covariate to account for possible lateral movement of N applied to the neighboring plot. Data were analyzed with block as a random effect. For the soil solution -N, which included two pairs of lysimeters per plot, plot was nested within block in the random effects structure. An autoregressive 1 correlation structure was fit to the model to account for temporal correlation in sample results within each plot. Analysis of variance was used to explain variation in soil water content for each sampling date, with a model including treatment, depth, and their interaction. Total water content from 0 to 100 cm was calculated for each plot and date using trapezoidal integration (Hupet et al., 2004) and compared among treatments using ANOVA. Mean comparisons using Tukey's adjusted P-value were used to generate estimated means for effects. Statistical analysis was carried out using statistical software program R (Version 3.5.2 GUI 1.70) including emmeans and nlme packages (R Core Team, 2018; Length, 2019; Pinheiro et al., 2019).
Results
Soil solution -N concentration
Annual average soil solution -N concentration differed by cropping system treatment in 2018 (P < 0.001), 2019 (P = 0.004), and 2020 (P = 0.003; Table 2; Figure 1), but did not vary by sampling depth or show an interaction effect in any year (P > 0.05). The average soil solution -N concentration was 77%, 96%, and 96% lower in the perennial system than the annual system in Years 1–3, respectively (Table 2).
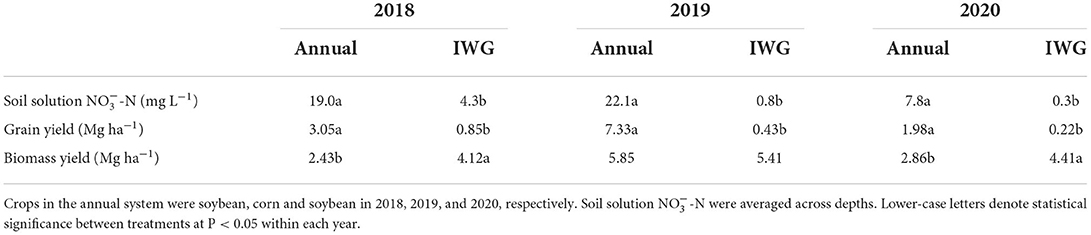
Table 2. Average soil solution -N, grain, and biomass yields in the annual and IWG systems in 2018, 2019, and 2020.
Throughout the seasons, both intra- and inter-annual variation was observed (Figure 1). Soil solution -N concentrations under IWG initially had mean values between 10 and 20 mg L−1 in Year 1 but declined to nearly zero by the end of July 2018 and remained at those levels for all 3 years except for occasional deviations. In 2018, soil solution -N concentrations under soybean were initially high at levels above 20 mg L−1, declining to near zero in mid-September, but increasing to early season levels after harvest. In 2019, however, soil solution -N concentrations under corn were between 10 and 20 mg L−1 but spiked to levels over twice that between late June and late August. Concentrations slowly declined over the remainder of the year. In 2020, mean soil solution -N concentrations under soybean were consistently around 10 mg L−1.
Soil extractable -N
There was an effect of cropping system treatment, depth, and a depth by treatment interaction (p-values < 0.001) on soil extractable -N measured at the end of the study in 2020 (Table 3). Soil -N was greater in the annual cropping system compared to IWG at 0–15, 15–30, and 30–45 cm depths at the end of the study (P < 0.001), but extractable -N levels were similar among treatments at the 45–60 cm depth. Soil extractable -N was greatest at the 0–15 cm depth below the annual crops and decreased with each depth interval until 45–60 cm, which was similar to the 30–45 cm depth interval. There was no difference in soil extractable -N across depths beneath the IWG.
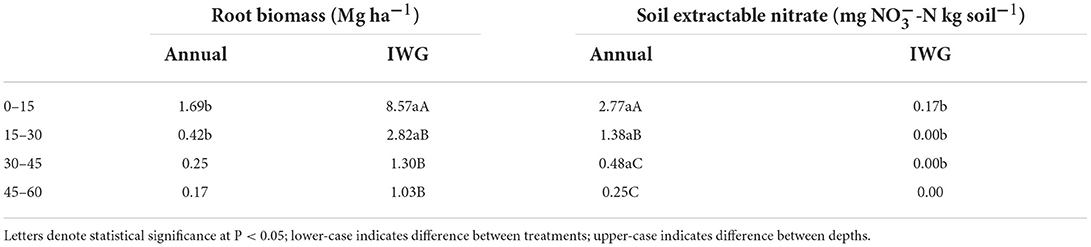
Table 3. Mean root biomass and soil extractable nitrate (mg -N kg soil−1) at four depth intervals from 0 to 60 cm at the end of the study in 2020.
Root biomass
Root biomass collected at the end of the study in 2020 was affected by treatment (P = 0.006), depth (P < 0.001), and a treatment by depth interaction (P < 0.001). Root biomass was greater under IWG compared to the annual cropping system at all depths (Table 3). Soybean root biomass was 80%, 85%, 81%, and 83% lower than IWG root biomass at 0–15, 15–30, 30–45, and 45–60 cm, respectively. Summed over all the depths, total IWG root biomass was 13.73 Mg ha−1 while soybean root biomass was 2.54 Mg ha−1, 82% lower (P < 0.001).
Crop yield
Grain yield was higher for the annual crops than for IWG in all years (P < 0.001, Table 2). Intermediate wheatgrass vegetative biomass yields (Table 2) were higher than soybean in 2018 (P = 0.001) and 2020 (P = 0.009) but similar to corn in 2019 (P = 0.322).
Soil water content
Of the four dates when soil water content was measured in 2019, there were very few effects of treatment, depth, or an interaction. Dates had a significant treatment by depth interaction. There was a main effect of cropping system treatment on soil water content on July 19 and October 31 (P < 0.001), in which soil water content was greater beneath the annual cropping system (0.09 m m−3) compared to the perennial (0.03 m m−3) on July 19 but lower in the annual (0.04 m m−3) compared to the perennial (0.05 m m−3) on October 31, 2019. In 2020, soil water content varied by treatment on June 23 (P < 0.001), in which soil water content was greater beneath the annual compared to the perennial. There was a significant interaction between treatment and depth on three other dates in 2020. Soil water content by treatment and depth is shown in Figure 2 to illustrate the interaction.
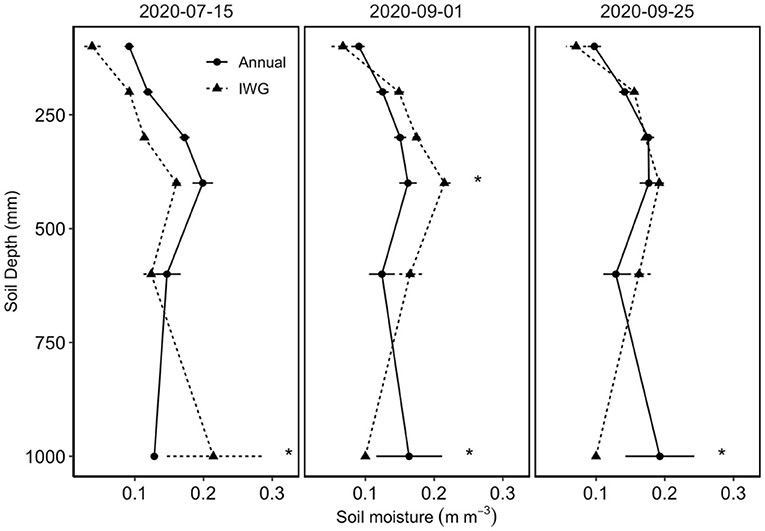
Figure 2. Soil water content beneath soybean (annual) and intermediate wheatgrass (IWG) on days when there was a significant interaction between cropping system treatment and depth. Asterisks indicate depths for which soil water content differed significantly between cropping systems.
Discussion
Soil solution -N concentration
Consistent with previous findings, we observed drastically lower concentrations of soil solution -N beneath IWG compared to the annual cropping system (Figure 1). Concentrations under IWG were initially between 10 and 20 mg L−1 during June and July of 2018, the first spring after seeding, but approached zero by August and remained very low for the duration of the experiment. A previous study in Minnesota found that soil solution -N beneath IWG averaged 0.09–0.3 mg L−1 when fertilized with 80 kg N ha−1 (Jungers et al., 2019). Despite only receiving 20 kg N ha−1 more fertilizer annually in this study, annual average soil solution -N concentrations ranged from 4.3 mg L−1 in the first-year to 0.3 mg L−1 at the end of the study. Higher -N concentrations found in this study compared to previous finding in Minnesota could be related to the potentially higher drainage rate associated with coarse structured soil at our study. These relatively higher soil solution -N levels observed in the first-year of our study were also likely attributable to lower root biomass during stand establishment and thus reduced ability to capture and assimilate soil solution -N. In line with this thinking, our results were similar to a study on sandy soil in Michigan during stand establishment (Culman et al., 2013b). Despite the slightly higher soil solution -N concentrations observed in Year 1 here and on other sandy soils, values were comparable to mixtures of perennial grasses and forbs found in CRP plantings (Randall et al., 1997) and consistently below the EPA safe drinking standard of 10 mg L−1.
Average annual soil solution -N concentrations beneath the annual crops were similar to or slightly lower than those reported by other studies in Minnesota. During the corn phase of the annual rotation, our annual soil solution -N of 22.1 mg L−1 was similar to findings by Ochsner et al. (2017), who reported an average soil solution -N of 21.2 mg L−1 beneath a corn-soybean rotation with corn phases fertilized at 146 kg N ha−1 as urea annually. In another study also conducted on coarse-structured soils in Minnesota, Struffert et al. (2016) reported an average annual soil solution -N concentration of 18.8 mg L−1 beneath soybean, and determined that soil solution -N during the soybean phase was not affected by N fertilizer rates applied to corn the previous year.
This is also among the first studies to compare soil solution -N levels of fertilized IWG to an unfertilized legume crop. Despite applying 100 kg N ha−1 of urea annually to the IWG, lower soil solution -N concentration were observed in the IWG compared to the unfertilized soybean. Biologically fixed N may have been mineralized after exudation or sloughing of soybean roots, which may have contributed to higher soil solution -N levels compared to IWG. The elevated soil solution -N in the soybean could also have originated from N fertilizer applied during the previous crops. However, as previously mentioned, N fertilizer rates applied to a previous corn crop did not affect soil solution -N beneath subsequent soybean (Struffert et al., 2016). Significant N demand by IWG may have also contributed to the large difference in soil solution -N.
Soil extractable -N
In addition to lower soil solution -N concentration, we also found less extractable -N in the soil after 3 years of IWG production compared to the annual rotation system. This suggests that the IWG assimilated more thoroughly from the soil than the annual rotation system, especially because the Year 3 crop was unfertilized soybean. Extractable -N remaining in the soil is a major factor determining the concentration of dissolved -N in soil solution, which in turn determines total leaching loads (Randall and Mulla, 2001; Culman et al., 2013a; Jungers et al., 2019).
The low levels of extractable -N under IWG also suggest that the plants may have been N-limited, despite being fertilized at the high end of optimal rates (Jungers et al., 2017). Nitrogen removal during IWG grain and biomass harvest can exceed 150 kg N ha−1 in the first-year of production (Crews et al., 2022; Tautges et al., 2018). Intermediate wheatgrass tissue N concentrations at the time of grain harvest in Minnesota peaked above 10 g N kg−1 biomass and declined with stand age (Jungers et al., 2017). If tissue N concentrations were similar to previous studies in MN, removal rates could have been between 46 and 58 kg N ha−1 year−1, thus less than the N applied as fertilizer (100 kg N ha−1). However, total N demand may have been greater to support root biomass production. If root tissue N was similar to previously reported estimates between 9 and 11 g N kg−1 (Dobbratz, 2019), then there would be another pool of nearly 130 kg N ha−1 in belowground root tissues. It is not known what fraction of root N is recycled during root death and mineralization of root biomass from year to year in an IWG system, but our results suggest that the N fertilizer applied was needed to support above and belowground IWG biomass and that little N was likely lost via leaching or left in the soil.
Root biomass
Root biomass is considered an important trait of perennial crops for providing ecosystem services such as reduced nitrate leaching to groundwater. Intermediate wheatgrass root biomass averaged 13.7 Mg ha−1 after the third-year of production, while soybean root biomass was 2.5 Mg ha−1 when sampled from 0 to 60 cm. These values are similar to other reported values for these crops. For example, Intermediate wheatgrass fertilized at 80 kg N ha−1 had root biomass of 4.10, 7.32, and 9.51 Mg ha−1 (0–60 cm depth) in Years 1–3 of a 3-year study, while a soybean-corn-soybean rotation had root biomass of 2.22, 2.93, and 2.30 Mg ha−1 in Years 1–3 (Bergquist, 2019). Root biomass accumulation over time allows IWG to more effectively capture -N before it reaches depths below the rooting zone where it is subject to leaching to groundwater. Nearly 63% of the IWG root biomass was found in the top 0–15 cm depth. Previous work has reported IWG belowground biomass to be 3.28 Mg ha−1 in the first 10 cm, on average, in Minnesota and Wisconsin (Sakiroglu et al., 2020). In an intra-annual study of root biomass beneath IWG, total root biomass from 0 to 20 cm peaked between 3.5 and 4 Mg ha−1 in June and July before declining to 1 Mg ha−1 at the end of the growing season (Pugliese et al., 2019). This concentration of root biomass at shallow depths also likely increases -N capture and consequently reduce soil solution -N below the rooting zone.
Soil water content
We found inconsistent differences in soil water content between annual crops and IWG. In the second-year of the study, soil water content was greater beneath the corn compared to the IWG in July, perhaps because IWG biomass would have been approaching peak biomass and thus been demanding more water than corn. A similar early-season pattern was found in Year 3 when soil water content was greater beneath the soybean compared to the IWG when measured in June. By the end of Year 2 (October), soil water content was greater in IWG compared to corn. Only in Year 3 did we observe any differences in soil water content by depth across treatments (Figure 2). In July, soil water content was greater beneath IWG compared to soybean at the deepest measured depth of 1,000 mm. This treatment effect was opposite at the 1,000 mm depth in September, where soil moisture content was greater for the soybean compared to IWG. Our results do match those from previous studies. In one comparison of perennial and annual systems, soil water content beneath Miscanthus and switchgrass was lower than a corn-soybean rotation earlier in the season, but the treatment effect flipped later in the season when switchgrass had higher soil water content (McIsaac et al., 2010). It has also been observed that soil water content tended to be higher under annuals than semi-perennials, and that there was less drainage from semi-perennials and perennials than annuals (Ferchaud and Mary, 2016). In studies with IWG, researchers have reported less in soil water content under IWG compared to annual wheat (Culman et al., 2013b) and corn (Jungers et al., 2019).
Soil water content can be used to make inferences on transpiration and drainage, the latter being an important component of nitrate leaching. The timing and frequency of our soil water content measurements precluded us from determining if both treatments had similar ET and drainage rates. Irrigation at our experiment could also have minimized our ability to detect differences in soil water content from plant ET. It is also established that greater root biomass increases water and nutrient uptake, which could reduce soil water content (Ehdaie et al., 2010; Matsunami et al., 2012; Carvalho et al., 2014). In our study, the similar soil moisture contents observed in the perennial and annual treatments may have been a function of the low water holding capacity of the sandy soil, which may have promoted drainage regardless of root biomass.
Grain and biomass yields
Intermediate wheatgrass grain yields at our sandy site were comparable to previous reports from sites with higher soil fertility levels. Under similar fertilizer treatments, reported first-year values range from 763 kg ha−1 (Zimbric et al., 2020) to 1,089 kg ha−1 at sites in Wisconsin (Favre et al., 2019) and from 893 kg ha−1 (Jungers et al., 2017) to 1,150 kg ha−1 (Fernandez et al., 2020) in Minnesota. Second- and third-year yields tend to be much lower, typically ranging from 150 kg ha−1 (Fernandez et al., 2020) to 630 kg ha−1 (Sakiroglu et al., 2020) in Year 2 and from 153 kg ha−1 (Jungers et al., 2017) to 371 kg ha−1 (Zimbric et al., 2020) in Year 3. Our yields suggest that this soil type and climate is appropriate for IWG grain and biomass production with irrigation.
Forage production is important for profitable IWG systems, since a major challenge of IWG grain production is the substantial yield declines in later years of production (Jungers et al., 2017; Pugliese et al., 2019; Hunter et al., 2020a). Intermediate wheatgrass biomass yields in this study included the stems and leaves that were remaining after grain harvest soon after peak productivity. Biomass harvested at this time, after physiological maturity, is relatively low in terms of forage quality compared to IWG biomass harvested at vegetative stages, but high compared to annual small grain biomass after grain harvest (Hunter et al., 2020b). Intermediate wheatgrass biomass yields were similar to those of other reports in Minnesota, though they were at the lower end of the range. Reported summer aboveground biomass values include 5,130 kg ha−1 in the second-year and 5,850 kg ha−1 in the third-year for IWG fertilized at 90 and 134 kg ha−1 in Wisconsin and 10,600 kg ha−1 for third-year stands in Minnesota (Sakiroglu et al., 2020). Similarly, summer yields of approximately 6,200 kg ha−1 were reported for first-year monocultures fertilized at 100 kg N ha−1 as urea (Favre et al., 2019). Biomass yields averaged 13,400 to 14,320 kg ha−1 for control treatments in a management study fertilized at 56 kg ha−1 the previous year (Pinto et al., 2021). Our results support that understanding that post-grain harvest biomass yields can be high enough for growers to consider harvesting for used as feed or straw on the farm or marketed for an additional revenue stream.
Conclusion
We found that soil solution -N concentrations were 77–96% lower under IWG than the annual corn-soybean rotation, even in the unfertilized soybean phase of rotation, but soil water content was similar. This suggests that the IWG captured and utilized a greater proportion of soil solution -N, which is also demonstrated by very low residual soil extractable -N levels at the end of the experiment relative to the annual crops. The lower -N concentrations in soil solution would be expected to translate to reductions in total leaching load of a similar magnitude. The increased uptake of N by IWG was likely facilitated by its greater root biomass, which was 5.4 times higher than that under the annual system. Despite the challenges associated with production of IWG on low-fertility sandy soils, grain yields were comparable to other locations and the system would likely be profitable in the first-year for grain alone. Biomass yields would support additional revenue streams in subsequent years to improve economic viability, and together our study provides evidence that IWG could be a good option for coarse textured soils that are prone to nitrate pollution.
Data availability statement
The raw data supporting the conclusions of this article will be made available by the authors, without undue reservation.
Author contributions
ER: collected data, analyzed data, wrote the first draft of the manuscript, and contributed to the final draft of the manuscript. JG: oversaw soil sample processing and contributed to the final draft of the manuscript. CS: designed the field experiment and contributed to the final draft of the manuscript. JJ: acquired funding, designed the field experiment, oversaw field sampling and data collection, data visualization, and contributed to the final draft of the manuscript. All authors contributed to data interpretation, manuscript writing, and revision.
Acknowledgments
The authors would like to thank Lindsay Wilson, Katherine Bohn, and the rest of the Sustainable Cropping Systems Lab staff for their dedication and assistance with essential research activities. We would also like to thank Ryan Perish and Hannah Barrett for their invaluable contributions to data collection and site maintenance. We thank Matthew Leung and Manbir Rakkar for assisting with soil nitrate extractions. We also thank Margaret Wagner for her contribution to project administration. Funding for this project was provided by the Minnesota Environment and Natural Resources Trust Fund as recommended by the Legislative-Citizen Commission on Minnesota Resources (LCCMR).
Conflict of interest
The authors declare that the research was conducted in the absence of any commercial or financial relationships that could be construed as a potential conflict of interest.
Publisher's note
All claims expressed in this article are solely those of the authors and do not necessarily represent those of their affiliated organizations, or those of the publisher, the editors and the reviewers. Any product that may be evaluated in this article, or claim that may be made by its manufacturer, is not guaranteed or endorsed by the publisher.
References
Asbjornsen, H., Hernandez-Santana, V., Liebman, M., Bayala, J., Chen, J., Helmers, M., et al. (2014). Targeting perennial vegetation in agricultural landscapes for enhancing ecosystem services. Renew. Agric. Food Syst. 29, 101–125. doi: 10.1017/S1742170512000385
Bajgain, P., Zhang, X., Jungers, J. M., DeHaan, L. R., Heim, B., Sheaffer, C. C., et al. (2020). ‘MN-Clearwater’, the first food-grade intermediate wheatgrass (Kernza perennial grain) cultivar. J. Plant Regist. 14, 288–297. doi: 10.1002/plr2.20042
Bergquist, G. (2019). Biomass Yield and Soil Microbial Response to Management of Perennial Intermediate Wheatgrass (Thinopyrum intermedium) as Grain Crop and Carbon Sink. Master's Theses, University of Minnesota.
Brender, J. D., Weyer, P. J., Romitti, P. A., Mohanty, B. P., Shinde, M. U., Vuong, A. M., et al. (2013). Prenatal nitrate intake from drinking water and selected birth defects in offspring of participants in the national birth defects prevention study. Environ. Health Perspect. 121, 1083–1089. doi: 10.1289/ehp.1206249
Carvalho, P., Azam-Ali, S., and Foulkes, M. J. (2014). Quantifying relationships between rooting traits and water uptake under drought in Mediterranean barley and durum wheat: root traits and water uptake. J. Integr. Plant Biol. 56, 455–469. doi: 10.1111/jipb.12109
Crews, T. E., Kemp, L., Bowden, J. H., and Murrell, E. G. (2022). How the nitrogen economy of a perennial cereal-legume intercrop affects productivity: can synchrony be achieved? Front. Sustain. Food Syst. 6:755548. doi: 10.3389/fsufs.2022.755548
Crews, T. E., and Peoples, M. B. (2005). Can the synchrony of nitrogen supply and crop demand be improved in legume and fertilizer-based agroecosystems? A review. Nutr. Cycl. Agroecosystems 72, 101–120. doi: 10.1007/s10705-004-6480-1
Culman, S. W., Snapp, S. S., Green, J. M., and Gentry, L. E. (2013a). Short- and long-term labile soil carbon and nitrogen dynamics reflect management and predict corn agronomic performance. Agron. J. 105, 493–502. doi: 10.2134/agronj2012.0382
Culman, S. W., Snapp, S. S., Ollenburger, M., Basso, B., and DeHaan, L. R. (2013b). Soil and water quality rapidly responds to the perennial grain Kernza wheatgrass. Agron. J. 105, 735–744. doi: 10.2134/agronj2012.0273
DeHaan, L., Christians, M., Crain, J., and Poland, J. (2018). Development and evolution of an intermediate wheatgrass domestication program. Sustainability 10:1499. doi: 10.3390/su10051499
Delta-T Devices (2021). PR2 Profile Probe - Analogue Version. Cambridge, UK. Available online at: www.delta-t.co.uk/product/pr2/
Dinnes, D. L., Karlen, D. L., Jaynes, D. B., Kaspar, T. C., Hatfield, J. L., Colvin, T. S., et al. (2002). Review and interpretation: nitrogen management strategies to reduce nitrate leaching in tile-drained Midwestern soils. Agron. J. 94, 153–171. doi: 10.2134/agronj2002.1530
Dobbratz, M. (2019). Perennial fuel, feed, and cereal: High diversity perennials for biofuel and intermediate wheatgrass for grain and forage. PhD Dissertation retrieved from University of Minnesota Digital Conservancy. Available online at: https://hdl.handle.net/11299/211748
Ehdaie, B., Merhaut, D. J., Ahmadian, S., Hoops, A. C., Khuong, T., Layne, A. P., et al. (2010). Root system size influences water-nutrient uptake and nitrate leaching potential in wheat: root system and nutrient uptake in wheat. J. Agron. Crop Sci. 196, 455–466. doi: 10.1111/j.1439-037X.2010.00433.x
Erisman, J. W., Galloway, J. N., Seitzinger, S., Bleeker, A., Dise, N. B., Petrescu, A. M. R., et al. (2013). Consequences of human modification of the global nitrogen cycle. Philos. Trans. R. Soc. B Biol. Sci. 368, 20130116. doi: 10.1098/rstb.2013.0116
Favre, J. R., Castiblanco, T. M., Combs, D. K., Wattiaux, M. A., and Picasso, V. D. (2019). Forage nutritive value and predicted fiber digestibility of Kernza intermediate wheatgrass in monoculture and in mixture with red clover during the first production year. Anim. Feed Sci. Technol. 258, 114298. doi: 10.1016/j.anifeedsci.2019.114298
Ferchaud, F., and Mary, B. (2016). Drainage and nitrate leaching assessed during 7 years under perennial and annual bioenergy crops. Bioenergy Res. 9, 656–670. doi: 10.1007/s12155-015-9710-2
Fernandez, C. W., Ehlke, N., Sheaffer, C. C., and Jungers, J. M. (2020). Effects of nitrogen fertilization and planting density on intermediate wheatgrass yield. Agron. J. 112, 4159–4170. doi: 10.1002/agj2.20351
Feyereisen, G. W., Wilson, B. N., Sands, G. R., Strock, J. S., and Porter, P. M. (2006). Potential for a rye cover crop to reduce nitrate loss in Southwestern Minnesota. Agron. J. 98, 1416–1426. doi: 10.2134/agronj2005.0134
Hunter, M. C., Sheaffer, C. C., Culman, S. W., and Jungers, J. M. (2020a). Effects of defoliation and row spacing on intermediate wheatgrass I: Grain production. Agron. J. 112, 1748–1763. doi: 10.1002/agj2.20128
Hunter, M. C., Sheaffer, C. C., Culman, S. W., Lazarus, W. F., and Jungers, J. M. (2020b). Effects of defoliation and row spacing on intermediate wheatgrass II: forage yield and economics. Agron. J. 112, 1862–1880. doi: 10.1002/agj2.20124
Hupet, F., Bogaert, P., and Vanclooster, M. (2004). Quantifying the local-scale uncertainty of estimated actual evapotranspiration. Hydrol. Process. 18, 3415–3434. doi: 10.1002/hyp.1504
Jungers, J. M., DeHaan, L. H., Mulla, D. J., Sheaffer, C. C., and Wyse, D. L. (2019). Reduced nitrate leaching in a perennial grain crop compared to maize in the Upper Midwest, USA. Agric. Ecosyst. Environ. 272, 63–73. doi: 10.1016/j.agee.2018.11.007
Jungers, J. M., DeHaan, L. R., Betts, K. J., Sheaffer, C. C., and Wyse, D. L. (2017). Intermediate wheatgrass grain and forage yield responses to nitrogen fertilization. Agron. J. 109, 462–472. doi: 10.2134/agronj2016.07.0438
Keeler, B. L., Gourevitch, J. D., Polasky, S., Isbell, F., Tessum, C. W., Hill, J. D., et al. (2016). The social costs of nitrogen. Sci. Adv. 2, e1600219. doi: 10.1126/sciadv.1600219
Keeler, B. L., and Polasky, S. (2014). Land-use change and costs to rural households: a case study in groundwater nitrate contamination. Environ. Res. Lett. 9, 074002. doi: 10.1088/1748-9326/9/7/074002
Law, E. P., Wayman, S., Pelzer, C. J., Culman, S. W., Gómez, M. I., DiTommaso, A., et al. (2022). Multi-criteria assessment of the economic and environmental sustainability characteristics of intermediate wheatgrass grown as a dual-purpose grain and forage crop. Sustainability. 14, 3548. doi: 10.3390/su14063548
Length, R. (2019). emmeans: Estimated Marginal Means, aka Least-Squares Means. R package version 1.3.3. Available online at: https://CRAN.R-project.org/package=emmeans
Maeght, J.-L., Rewald, B., and Pierret, A. (2013). How to study deep roots—and why it matters. Front. Plant Sci. 4:299. doi: 10.3389/fpls.2013.00299
Matsunami, M., Matsunami, T., Ogawa, A., Toyofuku, K., Kodama, I., and Kokubun, M. (2012). Genotypic variation in biomass production at the early vegetative stage among rice cultivars subjected to deficient soil moisture regimes and its association with water uptake capacity. Plant Prod. Sci. 15, 82–91. doi: 10.1626/pps.15.82
McIsaac, G. F., David, M. B., and Mitchell, C. A. (2010). Miscanthus and switchgrass production in Central Illinois: impacts on hydrology and inorganic nitrogen leaching. J. Environ. Qual. 39, 1790–1799. doi: 10.2134/jeq2009.0497
Ochsner, T. E., Schumacher, T. W., Venterea, R. T., Feyereisen, G. W., and Baker, J. M. (2017). Soil water dynamics and nitrate leaching under corn–soybean rotation, continuous corn, and kura clover. Vadose Zone J. 17, 1–11. doi: 10.2136/vzj2017.01.0028
Pinheiro, J., Bates, D., DebRoy, S., Sarkar, D., and R. Core Team (2019). nlme: Linear and Nonlinear Mixed Effects Models. R package version 3.1-139,. Available online at: https://CRAN.R-project.org/package=nlme
Pinto, P., De Haan, L., and Picasso, V. (2021). Post-harvest management practices impact on light penetration and Kernza intermediate wheatgrass yield components. Agronomy 11, 442. doi: 10.3390/agronomy11030442
Pugesgaard, S., Schelde, K., Larsen, S. U., Laerke, P. E., and Jørgensen, U. (2015). Comparing annual and perennial crops for bioenergy production - influence on nitrate leaching and energy balance. GCB Bioenergy 7, 1136–1149. doi: 10.1111/gcbb.12215
Pugliese, J. Y., Culman, S. W., and Sprunger, C. D. (2019). Harvesting forage of the perennial grain crop Kernza (Thinopyrum intermedium) increases root biomass and soil nitrogen cycling. Plant Soil 437, 241–254. doi: 10.1007/s11104-019-03974-6
R Core Team (2018). R: A Language and Environment for Statistical Computing. Vienna: R Foundation for Statistical Computing Available online at: https://www.R-project.org/
Randall, G. W., Huggins, D. R., Russelle, M. P., Fuchs, D. J., Nelson, W. W., and Anderson, J. L. (1997). Nitrate losses through subsurface tile drainage in Conservation Reserve Program, Alfalfa, and row crop systems. J. Environ. Qual. 26, 1240–1247. doi: 10.2134/jeq1997.00472425002600050007x
Randall, G. W., and Mulla, D. J. (2001). Nitrate nitrogen in surface waters as influenced by climatic conditions and agricultural practices. J. Environ. Qual. 30, 337–344. doi: 10.2134/jeq2001.302337x
Sakiroglu, M., Dong, C., Hall, M. B., Jungers, J., and Picasso, V. (2020). How does nitrogen and forage harvest affect belowground biomass and nonstructural carbohydrates in dual-use Kernza intermediate wheatgrass? Crop Sci. 60, 2562–2573. doi: 10.1002/csc2.20239
Smucker, A. J. M., McBurney, S. L., and Srivastava, A. K. (1982). Quantitative separation of roots from compacted soil profiles by the hydropneumatic elutriation system. Agron. J. 74, 500–503. doi: 10.2134/agronj1982.00021962007400030023x
Struffert, A. M., Rubin, J. C., Fernández, F. G., and Lamb, J. A. (2016). Nitrogen management for corn and groundwater quality in Upper Midwest irrigated sands. J. Environ. Qual. 45. 1557–1564. doi: 10.2134/jeq2016.03.0105
Tautges, N. E., Jungers, J. M., DeHaan, L. R., Wyse, D. L., and Sheaffer, C. C. (2018). Maintaining grain yields of the perennial cereal intermediate wheatgrass in monoculture v. bi-culture with alfalfa in the Upper Midwestern USA. J. Agric. Sci. 156, 758–773. doi: 10.1017/S0021859618000680
USDA–NRCS (2021). Web Soil Survey. USDA – Natural Resources Conservation Service. Available online at: https://websoilsurvey.sc.egov.usda.gov/App/HomePage.htm
Ward, M., Jones, R., Brender, J., de Kok, T., Weyer, P., Nolan, B., et al. (2018). Drinking water nitrate and human health: an updated review. Int. J. Environ. Res. Public Health 15, 1557. doi: 10.3390/ijerph15071557
Ward, M. H., Kilfoy, B. A., Weyer, P. J., Anderson, K. E., Folsom, A. R., and Cerhan, J. R. (2010). Nitrate intake and the risk of thyroid cancer and thyroid disease. Epidemiology 21, 389–395. doi: 10.1097/EDE.0b013e3181d6201d
Keywords: intermediate wheatgrass, nitrate, leaching, Kernza, groundwater, perennial grains
Citation: Reilly EC, Gutknecht JL, Sheaffer CC and Jungers JM (2022) Reductions in soil water nitrate beneath a perennial grain crop compared to an annual crop rotation on sandy soil. Front. Sustain. Food Syst. 6:996586. doi: 10.3389/fsufs.2022.996586
Received: 17 July 2022; Accepted: 05 September 2022;
Published: 26 September 2022.
Edited by:
Johann G. Zaller, University of Natural Resources and Life Sciences Vienna, AustriaReviewed by:
Alexandra Huddell, Columbia University, United StatesArdeshir Adeli, United States Department of Agriculture (USDA), United States
Stephen K. Hamilton, Michigan State University, United States
Copyright © 2022 Reilly, Gutknecht, Sheaffer and Jungers. This is an open-access article distributed under the terms of the Creative Commons Attribution License (CC BY). The use, distribution or reproduction in other forums is permitted, provided the original author(s) and the copyright owner(s) are credited and that the original publication in this journal is cited, in accordance with accepted academic practice. No use, distribution or reproduction is permitted which does not comply with these terms.
*Correspondence: Jacob M. Jungers, anVuZ2UwMzdAdW1uLmVkdQ==