Corrigendum: Could microalgae offer promising options for climate action via their agri-food applications?
- 1School of International Development, University of East Anglia, Norwich, United Kingdom
- 2Norwich Institute for Sustainable Development, Norwich, United Kingdom
In 2021 the Intergovernmental Panel on Climate Change (IPCC) issued the first volume of its latest authoritative report on climate change. Underlining the seriousness of the situation, the United Nations Secretary-General branded its findings a “code red for humanity.” The need for climate action is now evident, but finding viable pathways forward can be elusive. Microalgae have been attracting attention as a category of “future food,” with species like Arthrospira platensis (spirulina) and Chlorella vulgaris (chlorella) seeing growing uptake by consumers while research interest continues to expand. One timely but neglected question is whether microalgae might offer options for promising climate actions via their agri-food applications. Specifically, might they offer scope to help secure food supplies, while also providing climate resilient livelihood pathways for vulnerable farmers already grappling with food insecurity and environmental degradation? This paper reports on a review of the academic literature on microalgae as an agri-food technology, notably their uses as a food, feed, biofertilizer, biostimulant, and biochar. This family of applications was found to offer promising climate actions vis-à-vis both mitigating and adapting to climate change. Aspects pertinent to adaptation include growing rapidly under controlled conditions, reusing water, providing potent nutrition for humans and animals, and supporting resilient crop production. Agri-food applications of microalgae also provide opportunities to mitigate climate change that could be explored. The paper concludes by flagging possible risks and obstacles as well as research and policy priorities to elaborate and harness this potential.
Introduction
What does the “code red for humanity” mean for food and agriculture?
United Nations Secretary-General António Guterres branded the latest authoritative report by the Intergovernmental Panel on Climate Change (IPCC) a “code red for humanity.” Responding to its findings, he said, “The alarm bells are deafening, and the evidence is irrefutable… putting billions of people at immediate risk. If we combine forces now, we can avert climate catastrophe. But… there is no time for delay and no room for excuses” (UNRIC, 2021).
The emerging and predicted impacts on agriculture and food supplies are stark (Table 1).
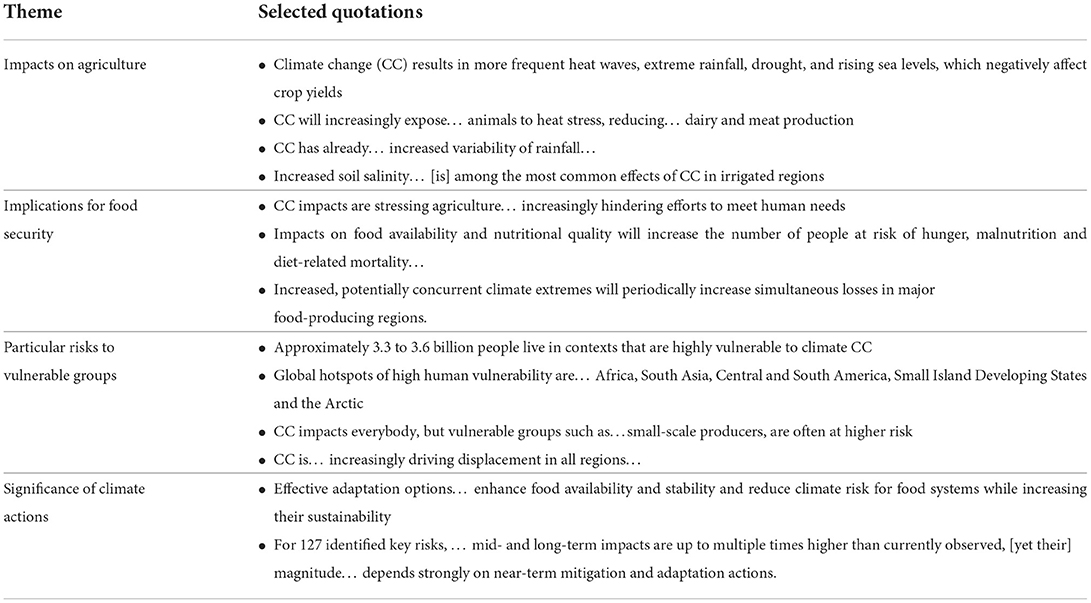
Table 1. Selected quotations from relevant sections of the latest IPCC report (IPCC, 2022a,b).
Sky-high rates of climate anxiety among young people underline the gravity of climate change (CC). A recent survey (Thompson, 2021) asked 10,000 16–25 year-olds from 10 countries how they felt about CC. It found nearly 60% felt “very worried” or “extremely worried” about it, while 45% said these feelings affected their daily lives, due in part to a sense that governments aren't doing enough to avoid catastrophic outcomes. Similarly, a climate scientist reported that the top five questions she has been asked on TikTok regarding CC are: Is it too late? Should I go to college or will the world end by then? Am I a bad person for having a child? Will we run out of food and water soon? How can I help as an individual with no power? (Wood, 2022).
Agriculture as a source of CC response options
While agriculture is clearly impacted by CC, it is also a key cause of CC and offers various potential response options. When talking about agriculture as a locus of CC mitigation options, the IPCC groups it together with forestry under the heading “agriculture, forestry, and other land uses” (AFOLU). The AFOLU sector is currently responsible for 13–21% of the anthropogenic greenhouse gas (GHG) emissions causing CC, and key contributing activities include deforestation, enteric fermentation from ruminants, and soil fertilization effects. At the same time, the AFOLU sector offers significant near-term CC mitigation potential at relatively low cost, and these CC mitigation measures can potentially also deliver multiple co-benefits including food security (IPCC, 2022c).
In short, some agricultural practices and technologies could be termed “climate smart.” Climate-smart agriculture has been defined as a family of technological options that can simultaneously address three objectives, namely increasing farm productivity and incomes, adapting and building resilience to CC, and mitigating CC. As such, climate-smart agriculture offers scope for “win-win” outcomes (FAO, 2013). Emerging critiques suggest however that this optimistic narrative can mask difficult questions like how any trade-offs are managed and who decides this (Ellis and Tschakert, 2019).
Various proven climate-smart agriculture practices have already been identified, but given the magnitude of the CC threat all promising technologies should be explored. Notably, innovative options are needed to permit a step change vis-à-vis food and agriculture. The United Nations Food Systems Summit (United Nations, 2021a) in September 2021 was premised on such a need for fundamental change. Its vision was to support the United Nations Sustainable Development Goals by fostering tangible changes to food systems, building on extensive consultations with stakeholders around the world.
Agri-food uses of microalgae as possible climate response actions
Algae are already attracting attention as a possible basis of climate action, but the focus to date has primarily been macroalgae, or seaweeds, rather than microalgae, notably on their potential contributions to CC mitigation (Seaweed Climate, 2022). For instance, seaweeds were discussed at the high-level CC conference in November 2021 (COP 26, 2021) in the context of the Global Methane Pledge (2021), which seeks to rapidly reduce methane emissions by advancing technical and policy work. This follows evidence that using seaweeds as feed supplements can sharply lower enteric methane emissions from cattle (Kinley et al., 2016; Makkar, 2018; Mihaila et al., 2022).
Microalgae are a diverse group of microscopic aquatic organisms that can be either single-celled or multicellular. Like plants, they typically generate energy from sunlight via photosynthesis, but they differ from plants in basic ways. One example is growing in fresh, brackish or sea water instead of on land, while another is absorbing nutrients from their substrate instead of via roots (Petersen et al., 2021). Some microalgae are seen as harmful, such as those that cause algal blooms which kill aquatic organisms (Shaw et al., 2003), while others can provide or serve as feedstocks for useful products like dyes, biofuels, or bioplastics.
Some microalgae uses involve food and agriculture, and may be termed agri-food technologies. This includes using microalgae as human dietary supplements, livestock feeds, or means to foster “climate smart” cropping via products like biofertilizers, biostimulants, and biochar.
One critical difference between macroalgae and microalgae stems from how they are produced. Microalgae are grown in either ponds or enclosed tanks, and hence as an inland crop. By contrast, macroalgae are typically either harvested from or farmed in the sea. While macroalgae can also be grown in ponds or tanks (Mata et al., 2015), such facilities must be situated on or near the coast. The fact that microalgae can grow inland means they can be produced diverse locations, and might thus serve as the basis of climate actions in a wide range of contexts. Microalgae production systems typically rely on photosynthesis, but recent technological developments also allow cultivation in the dark via heterotrophy (Linder, 2019). Still another possibility is producing microalgae via mixotrophy, which is a combination of these two strategies (Tibbetts, 2018).
This review considered whether agri-food uses of microalgae might offer options to help society face the looming CC threat, and hence represent a fruitful focus area for intensified research and development efforts. This study question stems from the urgent need faced coupled with encouraging early evidence on these technologies.
One demographic that is emphasized by this paper is small-scale farmers in the Global South, given their elevated vulnerability to CC, notably threats to their farming operations and food security. Critically, farming is the primary livelihood for many of the world's poor, while agricultural development is central to addressing development goals like ending poverty and achieving food security. It is estimated that 590 M of the world's 656 M farms are family farms, of which 551 M are small-scale (<2 ha; Erenstein et al., 2021). Such small-scale farmers are a key focus of international development efforts. The numbers of undernourished people are higher still due to also including many working in other sectors. Namely, 768 M people worldwide are undernourished of which 700 M live in Africa or Asia, while 2.37 B are unable to access adequate food year-round (FAO, 2021).
Charting climate resilient livelihood pathways for these populations is important not just for them but also others, given predictions of sharp rises of climate refugees from such areas under current trends (IPCC, 2022d), which could prove difficult for both migrants and recipient countries (Krieger et al., 2020). This paper thus specifically considers whether the technologies it examines may offer pertinent resilience building options for such communities.
Methodology
The study question was investigated via a systematic review of the academic literature conducted using the Web of Science and Google Scholar.
The literature search began by using the term climate change in combination with the terms microalgae, spirulina, chlorella, resilience, food, feed, and livestock, which delivered 191 papers. Scanning these for relevance to the study question gave 63 pertinent papers, while excluded papers addressed themes like microalgae as a biofuel or impacts of CC on natural microalgae populations. This search was supplemented by the 70 papers delivered at the AlgaEurope conference in December 2021 (AlgaEurope, 2021).
Due to insufficient evidence obtained on three key agri-food applications of microalgae, supplementary searches on biofertilizers, biostimulants, and biochar were also conducted. These searches were broadened by excluding the term climate change. Relevant studies cited by the various papers identified were also incorporated into this review.
Analysis of these papers involved grouping them then distilling their findings under target themes. The potential of each agri-food use of microalgae to help meet both of the key climate action objectives—CC mitigation1 and CC adaptation2—was then explored, since viable options are needed in both these domains. The scope for microalgae to help build climate resilience—a form of CC adaptation—was emphasized, given the importance of resilience considerations for both farmer livelihoods and food supplies. A conceptual framework was also proposed on the potential future role of such technologies as CC response options. Finally, risks and obstacles were highlighted then research and policy priorities were flagged.
Results
The following subsections share results for the five agri-food applications examined, with biofertilizers and biostimulants being combined due to overlaps in the literature on these technologies. One overarching finding was that many of the papers reviewed reported evidence on microalgae and their agri-food applications that is directly relevant to the objectives of climate action, namely CC mitigation and/or CC adaptation (Figure 1). For the most part, however, these papers did not highlight these linkages or frame these technologies as potential bases for climate action. Given this apparent disconnect, two further searches were conducted to elucidate these dynamics. The mitigation and adaptation volumes of the 2022 landmark CC report were also searched (IPCC, 2022f). Microalgae was mentioned four times, of which two involved possible agri-food uses as a climate action, specifically microalgae as an alternative protein source for livestock or aquaculture. Finally, the 191 Nationally Determined Contributions (NDCs), or national climate action plans, submitted by governments as the basis of their Paris Accord commitments were searched, and no mentions of microalgae were found (United Nations, 2021b). In short, it seems that researchers and practitioners alike are largely failing to make these connections at present. Possible explanations include the body of relevant evidence being thin, most studies being recent, and mapping such linkages requiring input from different disciplines and an interdisciplinary perspective.
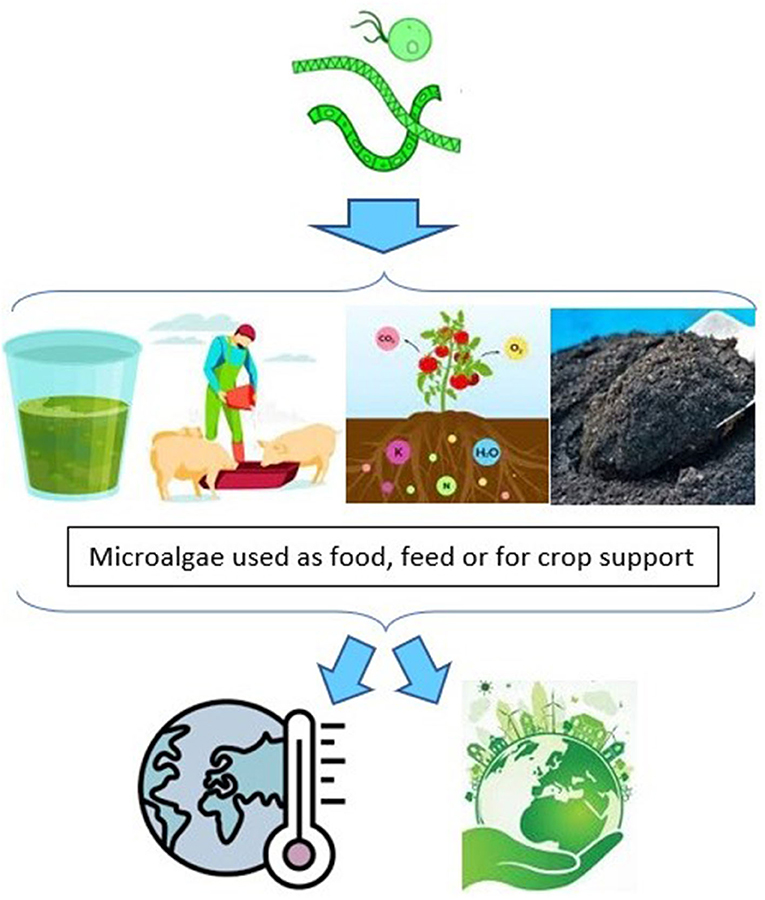
Figure 1. Agri-food applications of microalgae can potentially contribute to CC mitigation and/or CC adaptation objectives.
Microalgae as food supplements
Microalgae have been used as traditional foods in various countries where suitable species occur naturally. For instance, spirulina was harvested from lakes for use as a food by the Aztec civilization of Mexico (Ciferri, 1983), while communities living nearby Lake Chad in Africa still harvest spirulina from its waters (Abdulqader et al., 2000). The ancient Maya are believed to have both cultivated spirulina as a food and incorporated it into crop irrigation waters (Piccolo and Short, 2014).
Contemporary production of microalgae is dominated by two species—spirulina and chlorella—that account for 80% of estimated global production at 15,000 and 5,000 tons/year, respectively (Ciani et al., 2021). Besides these two, only a few other species are authorized for use as food in key jurisdictions like the European Union and United States (Ciani et al., 2021).
In addition to being the most widely produced microalgae, spirulina (Arthrospira platensis) is a key focus of published research on agri-food applications of microalgae (Habib et al., 2008), so the reported findings focus largely on this species.
Microalgae food supplements are principally eaten by health-conscious consumers, but can also be used to help address malnutrition and improve health in places where diet is poor (Piccolo, 2011). They can be sold as a dry powder or capsules, or incorporated into food products to boost their nutritional profile (Gantar and Svircev, 2008). Examples of studies on such products include pasta (Fradinho et al., 2020), baked goods (Uribe-Wandurraga et al., 2019), and sweetened snacks (Batista et al., 2017).
Microalgae can be potent sources of various nutrients, including high-quality proteins, lipids, and vitamins.
Some microalgae species are protein-rich, such as spirulina and chlorella. At 45–65% of dry weight biomass, spirulina has a protein content that is two times higher than beef, pork, or poultry, four times higher than eggs, and 1.5 times higher than soy (Ciani et al., 2021). A key determinant of protein quality is its amino acid profile. The essential amino acids are especially important, since they are not produced by the human body and hence must be obtained from food (Damodaran et al., 2007). Some authors suggest that microalgae can contain all the essential amino acids (Wells et al., 2017), while others report examples of microalgae supplying most but not all of the essential amino acids, such as Chlorella vulgaris being deficient in isoleucine (Amorim et al., 2021).
Digestibility is another important consideration, though findings differ between studies. Machado et al. (2022) suggest that microalgae cell walls could affect their digestibility and bioaccessibility without prior processing, but specify this does not apply to spirulina. Acquah et al. (2020) report that pre-treating microalgal biomass is important prior to using it as a food due to poor digestibility (Acquah et al., 2020). Niccolai et al. (2019) examined the digestibility of crude protein from several microalgae species and the values obtained for spirulina and chlorella compared favorably with the values for established human foods like beans, oats, and wheat.
Lipids are essential to all living organisms for membrane formation, energy storage, and cell signaling (Eyster, 2007). Although humans and other mammals synthesize lipids, some essential lipids must be obtained from diet, notably the long-chain polyunsaturated fatty acids (PUFAs; Holdt and Kraan, 2011). Microalgae like spirulina and chlorella are good sources of some PUFAs, such as linoleic acid (an omega 6 fatty acid) and α-linolenic acid (an omega 3 fatty acid), but less effective as sources of some other PUFAs (Wells et al., 2017). Microalgae may offer a sustainable means to obtain essential fatty acids amidst fears fish stocks are being depleted (Merz and Main, 2014; Morao et al., 2021).
Vitamins are micronutrients needed for essential metabolic functions that must be obtained from diet since organisms cannot synthesize them in sufficient quantities (Wells et al., 2017). Vitamin deficiencies, meanwhile, can lead to diseases like beriberi, pellagra, and scurvy (Stabler and Allen, 2004; Martin et al., 2011). Microalgae are rich in vitamins. For instance, spirulina has been found to be rich in vitamins A, B1, B2, B12, and E (Becker, 2013), while Chlorella is a good source of vitamin B12 (Watanabe et al., 2002).
In addition to their nutritional value, there is growing evidence on the scope for microalgae to serve as functional foods or nutraceuticals. These are defined as foods that contain bioactive compounds or phytochemicals that may benefit health beyond basic nutrition (Wells et al., 2017; Kholssi et al., 2021). Such studies might examine potential anti-inflammatory, antibacterial, antioxidative, antiviral, hypolipidemic, and/or anti-carcinogenic activity associated with microalgae consumption (Hosseini et al., 2013; Qinghua et al., 2016). This work, however, falls outside the scope of the present review.
Microalgae as a family of crops
Most microalgae produce biomass via photosynthesis using carbon dioxide (CO2), nutrients and light. Some species including chlorella can also grow heterotrophically (i.e., in the dark) via chemical processes. This can deliver much higher yields than production via photosynthesis, namely 150–200 vs. 5–10 g/L of dry biomass (Ciani et al., 2021). Recent technological developments make it possible for microalgae to be commercially produced via heterotrophy, but for the moment most production still relies on photosynthesis (Linder, 2019; Ciani et al., 2021).
In nature, spirulina can be found growing in marshes, lakes, seawater and thermal springs. It grows best under bright sunshine in alkaline (pH 8.5–11) and saline water (ideally 20–70 g/L but up to 270 g/L) and at elevated temperatures (35–37°C; Habib et al., 2008). These properties help limit the risk of biotic contaminants, since other microorganisms may struggle to survive under such conditions (Kebede and Ahlgren, 1996). For instance, it thrives in highly alkaline, saline lakes in Africa's Rift Valley, where it strongly dominates other microalgae populations (Habib et al., 2008).
Spirulina production currently falls into two broad categories: Commercial operations supplying the health food market and initiatives to bolster food security in developing countries. It can be produced using expensive equipment like photobioreactors with precise parameter control systems. Alternatively, simple technologies can be used like unlined ditches, rudimentary stirring devices, harvesting with cloth and drying in the sun (Habib et al., 2008). Production operations could also use intermediate technologies like PVC-lined culture ponds stirred by electrically driven paddle wheels (Piccolo and Short, 2014). The quality control of the algal biomass produced via simple systems will be lower, potentially raising questions about its suitability as a food. Alternatively, such algal biomass could perhaps be used for other agri-food applications for which quality standards are less stringent.
At present microalgae is generally produced in open ponds under natural light, but it can also be produced under artificial light. As things stand this imposes additional costs but solar PV costs continue to plummet (IRENA, 2022), which could make this option economically competitive in time for microalgae uses like food. The benefits of using artificial light include greater independence from weather conditions and continuous operation throughout the day and year. By facilitating use of closed production systems, artificial light also creates scope for providing higher and more constant biomass quality (Ciani et al., 2021).
Microalgae production can be much more efficient than growing crops or raising livestock, since it occurs under controlled conditions that foster efficient nutrient utilization and stable, rapid growth, while also having low land and water demands and not requiring pesticides or antibiotics (Tredici, 2010). This is reflected in comparisons of securing dietary protein via different foods, notably estimates of inputs required and environmental impacts imposed (Ciani et al., 2021). The protein production per unit land (kg/ha/year) of spirulina was 500x more than beef, 60x more than pork, 15x more than poultry, and 5x more than soybeans. Water requirements per kg protein from spirulina were comparatively low, namely 0.7–3% of beef, 1.5–5% of pork, 3–10% of poultry, 2–9% of eggs, and 13–50% of soy. GHG emissions per kg protein of spirulina were likewise comparatively low, namely well below 1% of beef, 5–12% of pork, 8–12% of poultry and 8–11% of eggs (Values for soy were not provided.).
An FAO report (Habib et al., 2008) on spirulina as a food or feed set the stage for the current review. It observes, “spirulina appears to have considerable potential for development… as a small-scale crop for nutritional enhancement, livelihood development and environmental mitigation.” It laments that spirulina “has not yet received the serious consideration it deserves as a potentially key crop in… areas where traditional agriculture struggles” due to factors like “salination and water shortages,” especially given the “impacts of global CC.” It suggests that governments and international organizations should reassess the potential utility of spirulina as an alternative crop, notably in “communities where the staple diet is poor or inadequate.” This report did not however explore the ways that spirulina might help deliver on CC objectives. Another FAO report (Piccolo, 2011) suggests producing spirulina to serve markets like health conscious consumers and institutions supporting food insecure populations offers an attractive business opportunity, since this can be done with a modest investment while the needed technical expertise is simple to obtain.
Is microalgae cultivation climate resilient?
Various authors list distinguishing characteristics of microalgae cultivation as part of their analysis. These are sometimes framed as key advantages of such cultivation relative to conventional crop or livestock production. Table 2 shares characteristics cited by several papers analyzed for this review.
Taken together, these characteristics could be seen as different ways in which microalgae production is resilient to stresses and shocks. Critically, the technological profile they evoke seems well-suited to boosting the climate resilience of production, or enhancing the scope to deliver dynamic production despite climatic shocks. Still another characteristic of microalgae that supports the contention that it can help build climate resilience is their capacity to evolve quickly to adjust their biology to stresses like elevated temperatures and ultraviolet radiation (Wong et al., 2015; Padfield et al., 2016).
According to the IPCC, climate resilience is defined as “The capacity of social, economic and environmental systems to cope with a hazardous event or trend or disturbance, responding, or reorganizing in ways that maintain their essential function, identity and structure” (IPCC, 2014). For perspective on climate resilience priorities for agriculture and food supplies, see Table 1 on the different ways CC threatens agriculture.
Table 3 distills the main characteristics of microalgae repeatedly evoked in this literature, then highlights their linkages to fostering climate resilient farming and food supplies.
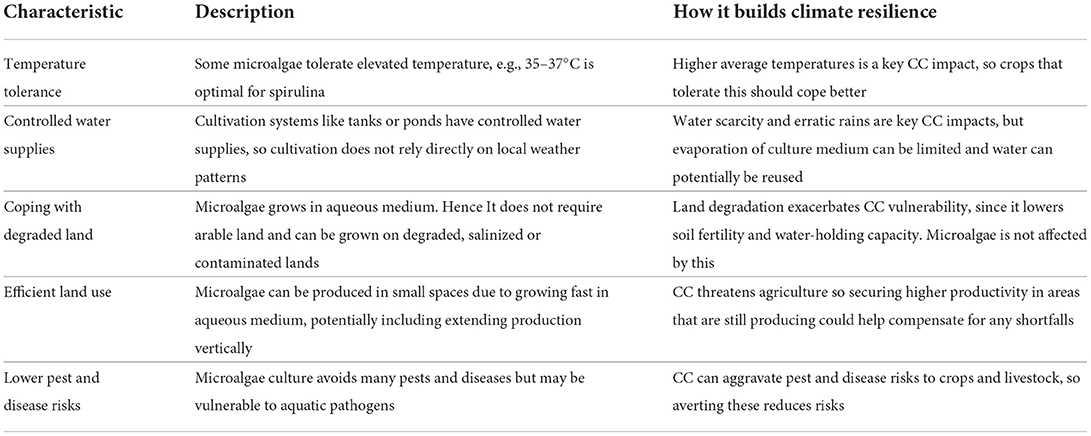
Table 3. Key characteristics of microalgae flagged in the papers reviewed and their linkages to climate resilience.
Microalgae as livestock feeds
Livestock production is key to the human food system, with animal-sourced foods like meat and dairy key parts of diet in cultures across the world, notably as a source of protein. Moreover, livestock can transform substances inedible to humans like grasses or food waste into nutritious, palatable foods (Halmemies-Beauchet-Filleau et al., 2018). Livestock is also a key source of rural employment and savings and can be central to culture, particularly in the developing world (Dumont et al., 2018; Chia et al., 2020).
A key challenge facing this sector is sustainably securing ample and nutritious livestock feed. Feed is a particular problem in parts of the tropics, where forages often have poor nutritive value including low protein content, which limits the efficiency of animal production (Halmemies-Beauchet-Filleau et al., 2018). Yet feed demand is expected to continue rising strongly, particularly in developing countries (Chia et al., 2020). Securing feed is estimated to account for 60–70% of livestock production costs (Chia et al., 2020), with meeting the protein needs of animals a particular concern (Amorim et al., 2021). Proteins are needed for key physiological and biochemical processes such as those by which dairy cows convert feed into milk (Hof et al., 1994).
Feed supply concerns dovetail with CC concerns in two distinct ways.
Feed insecurity is an emerging concern among livestock keepers that is linked to CC (Cordeiro M. R. C. et al., 2022). CC can adversely affect feed supplies by threatening the quantity and quality of pastures and feed crops, thus hampering livestock production or even causing animal deaths. Maluleke and Mokwena (2017) report that livestock in South Africa are highly vulnerable to CC due primarily to adverse effects on feed supplies, while Catley et al. (2014) found that elevated livestock mortality rates in Ethiopia were due primarily to starvation or dehydration linked to climatic effects.
Meanwhile, steps taken to secure feed can increase GHG emissions and thus aggravate CC, notably where this involves converting forests to pastures or cropland for feed crops. Clearance of forest to produce feed crops like soya is the main cause of global deforestation, and two-thirds of forest losses in Brazil involve conversion to pastures (Guéneau, 2018). A related source of GHG emissions is transporting feed to its target users. For instance, ruminant milk and meat production in Europe relies largely on imported soya from distant South America (Lindberg et al., 2016). Livestock are estimated to account for 14.5% of total current GHG emissions (Gerber et al., 2013), making it a key cause of CC, though a more recent analysis suggests this may be an underestimate (Xu et al., 2021).
Novel feeds like microalgae (Lamminen et al., 2017), seaweed (Makkar et al., 2016), bacteria (Halmemies-Beauchet-Filleau et al., 2018), and insects (Van Huis et al., 2015; Shah et al., 2022) offer options to improve the sustainability of livestock production. They can provide environmentally friendly protein-rich feed supplements that complement staple feeds like grasses and feed crops. Moreover, they can do so without compromising the quality of animal products, and may actually improve the quality of products like meat (Meale et al., 2014) and milk (Boeckaert et al., 2008).
Microalgae have been flagged as especially promising future livestock feeds, despite their minimal use at present (Halmemies-Beauchet-Filleau et al., 2018).
Microalgae have been tested in feed formulations for cattle, goat, sheep, pigs, poultry and fish, and results have typically included higher productivity and/or better nutritional quality of products (Amorim et al., 2021). Such feeds also provide avenues to face the looming climate threat, including both adapting to and mitigating CC.
Using microalgae as feed supplements offers scope to enhance the climate resilience of livestock production in two distinct ways. First, it could ensure access to protein supplements thanks to the fact that production of microalgae biomass seems likely to be climate resilient, as discussed in Section Is microalgae cultivation climate resilient?. Second, this feed could have positive effects on animals that may enhance their capacity to cope with CC impacts. Notably, it can improve the digestive function of ruminants—including rumen-based protein synthesis—thus enhancing animal performance given available feed resources (Halmemies-Beauchet-Filleau et al., 2018). For instance, chlorella was found to improve the digestive function and milk yield of goats (Kholif et al., 2017). Amino acid profile is a key determinant of feed quality, and microalgae like chlorella and spirulina have profiles that resemble soybean, the leading source of feed protein (Amorim et al., 2021). Microalgae can also provide key minerals, thus avoiding imbalances that can hamper feed conversion and animal growth (Tibbetts et al., 2015).
Using microalgae as a feed supplement also provides CC mitigation opportunities in two distinct ways. Most simply, it offers scope to partially replace leading supplements like soy (Walsh et al., 2015; Lamminen et al., 2019) that have a large carbon footprint due to causing deforestation and transport emissions. By contrast, microalgae requires comparatively little land and could be locally sourced (see Section Is microalgae cultivation climate resilient?). Microalgae-based supplements may also help reduce enteric methane emissions from ruminants, since these emissions are associated with poor digestive function and lost feed energy. The potential for protein supplements to improve ruminant digestive function and mitigate enteric emissions is thought to be highest in the tropics (Knapp et al., 2014; Halmemies-Beauchet-Filleau et al., 2018).
Walsh et al. (2015) modeled possible impacts of wider use of microalgae as a livestock feed. They suggest this could boost the efficiency of livestock production and enable better use of land and water, thus supporting food security while creating scope to reduce deforestation and GHG emissions. They estimate microalgae feed could free up 40% of existing pastures and feedcrop land by 2100 while securing large GHG emissions reductions, and suggest this merits serious consideration as a CC mitigation option. They note however that realizing such outcomes would require addressing challenges like designing systems that are robust against contaminants and can recycle water across successive harvests. Palatability issues may also require attention, notably at higher concentrations of microalgae in feed (Van Emon et al., 2015).
Microalgae-based biofertilizers and biostimulants for crop support
World agriculture has depended heavily on chemical fertilizers to boost crop productivity and meet food needs over the past several decades, yet mounting evidence suggests these chemicals can undermine agricultural sustainability. Critically, excessive use of such products is not climate smart. It can lead to soil degradation (Guo et al., 2010) including depletion of soil organic matter (Odhiambo and Magandini, 2020), and hence aggravate the vulnerability of cropping to CC by reducing the water-holding capacity of soils. Chemical fertilizers also seem ill-suited to coping with CC, since under hot and/or dry conditions crop response can be marginal (Odhiambo and Magandini, 2020) or even deleterious (Bushong et al., 2016). They also contribute to GHG emissions via their status as fossil-fuel based products.
Biofertilizers and biostimulants are natural alternatives that can boost crop production while making it more sustainable and resilient to stresses (Garcia-Gonzalez and Sommerfeld, 2016; Van Oosten et al., 2017). They also offer scope to make crop production climate smart, though most authors do not explicitly make this link. While definitions of these terms vary between papers, both are substances of biological origin that can either take the form of extracted chemical compounds or inoculants of living microorganisms.
Biofertilizers contain key plant nutrients in forms that support plant growth. They can increase soil nutrient availability, thus fostering plant growth while also reducing reliance on chemical fertilizers (Ronga et al., 2019; Guo et al., 2020; BHU, 2022; Cordeiro M. R. C. et al., 2022).
Biostimulants are substances that promote plant growth when applied to plants or soil without being nutrient sources, soil improvers, or pesticides. They function by stimulating biological and chemical processes in plants and/or associated microbes like mycorrhizal fungi. This can facilitate nutrient uptake, raise yields, improve crop quality, and/or enhance crop tolerance to stresses like heat and salinity (Du Jardin, 2015; Ronga et al., 2019; Navarro-López et al., 2020).
Biofertilizers and biostimulants derived from microalgae are attracting growing attention from both researchers and farmers. This is reflected in spiking numbers of both academic papers and patents in recent years driven by factors like sustainability concerns and demand for organic food (Murata et al., 2021). At present, however, these products remain largely unexploited (Ronga et al., 2019).
Various studies have clearly demonstrated the potential efficacy of microalgae-based biofertilizers and biostimulants (Tables 4, 5), although factors like timing and dosage can be important (Vernieri et al., 2005).
Crop production can be threatened by abiotic stresses linked to CC like elevated temperatures, water scarcity and soil salinity (Biancardi et al., 2010; Ronga et al., 2018). An emerging literature on pertinent aspects of microalgae-based biofertilizers and biostimulants offers grounds for hope that these technologies might in time provide ways to build crop resilience to these stresses (Table 6).
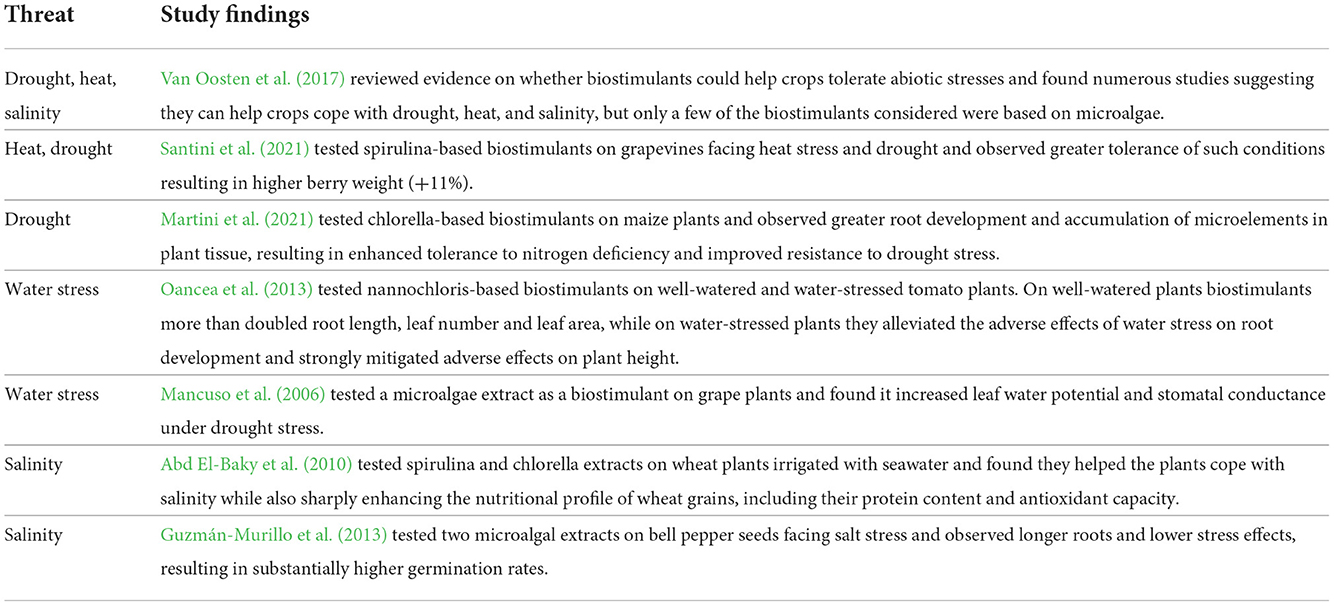
Table 6. Examples of studies that explored aspects of these technologies pertinent to climate resilience.
Some microalgae can also function as soil amendments when applied to fields and may offer ways to control erosion and limit any yield losses from CC impacts like erratic rainfall. Kheirfam et al. (2017) examined the effect of using a microalgal inoculant on rainfall-induced soil erosion in Iran and found that soil losses decreased by 73–98% following treatment thanks to creating biofilms that formed larger more stable soil particles. Malam Issa et al. (2007) investigated the impact of microalgal inoculation on degraded soils in South Africa and found treated soils showed improved aggregate stability that increased gradually over time due to binding and gluing effects by microbes. Trejo et al. (2012) tested the potential for alginate beads to restore eroded, infertile soil in an arid part of Mexico and found they sharply increased fertility measures like soil carbon and growth of sorghum crops.
Microalgae as biochar feedstocks
Another way microalgae could potentially support crop production is via using algal biomass to make biochar, or charred biomass. Biochar is formed by heating organic matter like wheat stover, sawdust or microalgae over time in the absence of oxygen (i.e., pyrolysis). The resulting product can serve as a soil amendment, as advocated by entities like the International Biochar Initiative (2022). This can improve the availability of soil nutrients, enhance soil water-holding capacity, and support beneficial soil microbes, with impacts greatest on degraded soils (Roberts et al., 2010; Whitman et al., 2010; Lehmann and Joseph, 2015). Such changes can restore degraded soils and hence support crop production in areas where land degradation is prevalent. They can also build the climate resilience of production by helping crops cope with CC impacts like erratic rainfall and extreme weather events, though none of the papers reviewed made this link.
A key feature of biochar is its stability. Notably, when used as a soil amendment its carbon components are highly recalcitrant, with estimated residence times in soil of hundreds to thousands of years (Spokas, 2010; Lehmann and Joseph, 2015). Biochar use was a traditional soil management practice in some cultures, and some treated fields have remained distinct over time (Lehmann and Joseph, 2015). For instance, terra preta soils in Brazil treated by the Amerindians many centuries ago were found to contain up to 9% carbon compared with 0.5% on neighboring lands, while their productivity was estimated to be twice as high (Marris, 2006). This stability enables biochar to make lasting changes to soils, including fixing atmospheric carbon in soils and thus potentially offering options for CC mitigation, though net GHG flux effects will also depend on factors like the feedstock and heat source used to produce biochar (Lehmann and Joseph, 2015).
Some early studies have investigated biochar made from microalgae and its potential as a soil amendment. Wang et al. (2013) studied the organic elements and minerals in biochar made from chlorella and suggested it had good prospects as a soil amendment. Gong et al. (2014) examined biochar made from two types of microalgae and found they had a higher content of nitrogen and various minerals compared to those made from lignocellulosic feedstocks like sawdust or rice husk. Chang et al. (2015) conducted chemical analysis of biochar derived from chlorella and suggested it could be used as a mineral-rich soil amendment. One caveat however is that if this chlorella contains heavy metals then these could be incorporated into biochar and released into soil. Minimizing or removing any such contaminants is thus a priority where microalgae are to be used as biochar feedstock.
Other studies have investigated the potential of microalgae-based biochar as both a soil amendment and CC mitigation option. Yu et al. (2018) found that biochar derived from chlorella was suitable for use on agricultural soils while also contributing to CC mitigation thanks to its chemical composition. Zhao et al. (2013) compared different biochar feedstocks including chlorella and found that biochar properties key to crop support and CC mitigation potential (e.g., total carbon, carbon sequestration capacity) were largely a function of feedstock type, and that chlorella performed well by such measures. Chen et al. (2021) examined biochar derived from chlorella compared to those derived from soybean straw and sawdust and found that it had higher nitrogen content but lower carbon content, though all three showed potential to both improve soils and mitigate CC.
Discussion
CC poses an existential threat to agriculture and food supplies via impacts like erratic rainfall and more frequent or severe extreme weather events. Parts of the Global South may be at particular risk from climatic shocks, notably areas where agriculture and herding are already marginal due to factors like poor soil conditions and limited access to inputs like irrigation, improved seeds, or vaccines.
Conventional agricultural production is highly vulnerable to CC especially when coupled with environmental degradation. For instance, maize or sorghum may struggle due to poor or erratic rains, while cattle or goats may struggle where pastures or water sources become depleted. Inputs like irrigation, fertilizers, and improved seeds can overcome such stresses to a point but may see diminishing returns over time due to factors like salinization and depletion of soil organic matter or aquifers (Wise, 2020). There is thus an urgent need for innovative options to ensure the climate resilience of agriculture and the security of food supplies.
Future foods, agri-food uses of microalgae, and building climate resilience
Future foods (FFs) like microalgae, insects and mycoprotein (i.e., fungus) are an emerging class of foods that show promise as nutritious and sustainable dietary options. These foods can also complement or substitute for conventional plant-sourced foods (PSFs) and animal-sourced foods (ASFs) in various ways (Tzachor et al., 2021).
The defining characteristic of FFs is that they are typically produced within controlled systems that are often also enclosed. For instance, microalgae is cultivated in man-made ponds or enclosed bioreactors while insects are grown in stackable, multicompartment nurseries. Such farming systems enable greater control over the physical, chemical, and biological conditions of production, which can boost performance and potentially deliver elevated production per unit time and area. These farms can also sharply reduce exposure to biotic and abiotic stresses. Critically, such systems may enable farmers to sidestep threats like water scarcity and pest attacks that might otherwise jeopardize farm production (Tzachor et al., 2021).
Given their profile, FFs like microalgae hold promise as options to deliver vigorous and sustainable production despite stresses including CC, in contrast to farms producing PSFs and/or ASFs. Figure 2 considers how well PSF, ASF, and FF farming systems cope with different stresses using maize, cattle, and spirulina as case studies. While this typology shows tendencies for these different farm types, farm performance given stresses clearly also depends on local factors like whether or not climate smart practices are employed.

Figure 2. How well PSF, ASF, and FF farming systems cope with major stresses (adapted from Tzachor et al., 2021).
The present review builds on this foundation by exploring the linkages between one group of future foods and one abiotic stress, namely microalgae and CC. This includes examining how different agri-food uses of microalgae might offer options to build the climate resilience of both farmers and food supplies.
Via their agri-food applications, microalgae offer several distinct avenues for building climate resilience. Figure 3 shows the ways they can boost the climate resilience of wider food supplies, namely fostering robust, sustained production of this FF despite CC while also enhancing the resilience of PSF and ASF production. Figure 4 shows how they can provide climate resilient livelihood pathways for farming communities that are vulnerable to CC shocks, such as small-scale farmers in Africa.
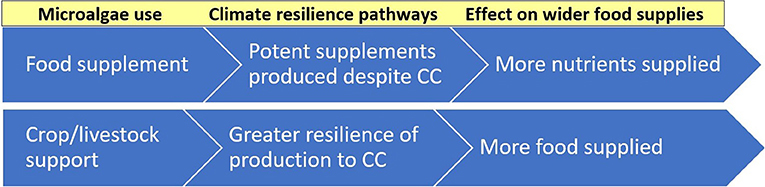
Figure 3. Agri-food uses of microalgae and pathways to enhanced climate resilience of food supplies.
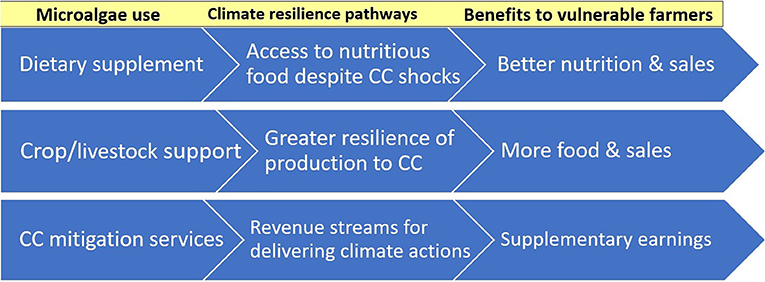
Figure 4. Agri-food uses of microalgae and pathways to enhanced climate resilience of vulnerable farmers.
Figure 4 includes an additional resilience factor besides the effects of microalgae on food production, namely creating scope to harness climate finance flows. Climate finance is defined as “all financial flows whose expected effect is to reduce net GHG emissions and/or to enhance resilience to the impacts of climate variability and the projected climate change” (IPCC, 2014). The prerequisite for any such flows would be for microalgae investments to be recognized as viable means to deliver on CC objectives, which is not presently the case. If this were addressed, it would set the stage for climate finance to be channeled to microalgae initiatives via NDC plans or offset projects (see Section Agri-food applications of microalgae and CC mitigation below).
Climate resilient development pathways are defined as trajectories that successfully integrate sustainability, CC adaptation and CC mitigation considerations to deliver human wellbeing and planetary health. Climate resilient development has emerged as a guiding principle for climate policy and action (IPCC, 2022e), as reflected in a growing literature on pathways (e.g., Quandt et al., 2017; Ellis and Tschakert, 2019).
Whether or not vulnerable farmers can access climate resilient livelihood pathways could have profound consequences over time both locally and more widely. Figure 5 illustrates how the effects of CC shocks and related stresses can differ depending on whether or not farmers can access viable technological options to build climate resilience. Without such options, farmers might resort to desperate measures like depleting key resource stocks (Adger et al., 2014; IEP, 2020; Siedenburg, 2021) that could see them fall into a “vicious circle” of rising climate vulnerability and deepening poverty. Conversely, if they could access viable technologies, this might enable them to instead reach a “virtuous circle” of climate resilience and food security (Pimbert, 2012; Hendrix and Brinkman, 2013).
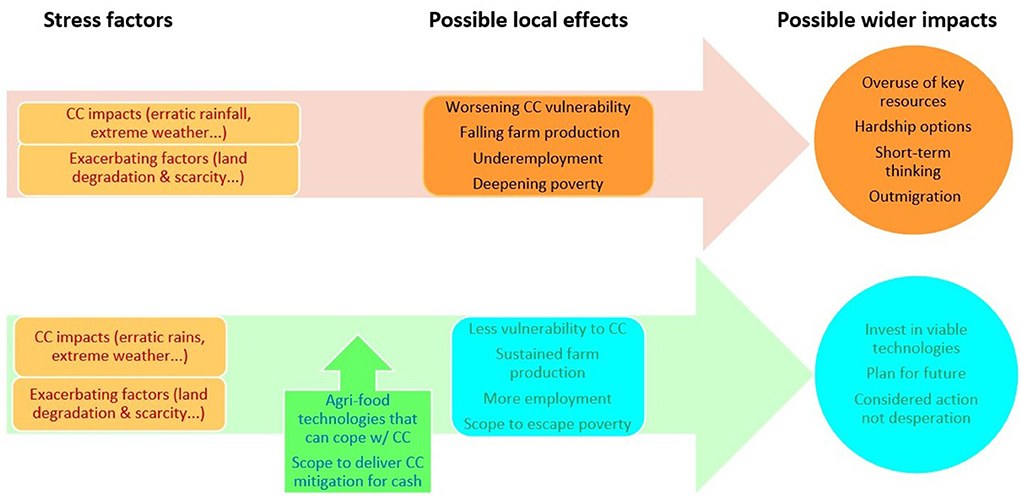
Figure 5. Differing effects of CC on vulnerable small-scale farmers depending on whether they can access viable technologies.
Agri-food applications of microalgae and CC mitigation
AFOLU measures like agroforestry or livestock management are seen as offering scope for substantial CC mitigation at relatively low cost. The IPCC estimates that AFOLU could provide 20–30% of needed CC mitigation to remain within a 1.5–2°C emissions trajectory to 2050, and that 30–50% of this could be achieved at a carbon price of $20/ton CO2 equivalent (tCO2e) or less. AFOLU measures are also well-positioned to deliver co-benefits that foster climate resilience like boosting soil productivity, water availability, and food security (IPCC, 2022c).
Despite such promise, implementation of AFOLU measures remains limited due to barriers such as insufficient financial and policy support. The IPCC has suggested that national investment plans under NDCs are urgently needed to accelerate AFOLU deployment, coupled with policy levers like payments for ecosystem services and fostering engagement from the private sector and charities (IPCC, 2022c).
The linkages of microalgae to GHG emissions and the goal of CC mitigation are complex. Microalgae absorb CO2 and transform it into microalgal biomass and hence could potentially help mitigate CC, especially since some studies suggest they can fix atmospheric carbon significantly more efficiently than terrestrial plants (Pires et al., 2014). Alternatively, however, microalgae could have ambiguous or even negative impacts on GHG dynamics.
One key question is the sourcing of microalgae production inputs like light, heat, water, nutrients, and CO2. Notably, utilizing fossil-based energy inputs undermines CC mitigation potential, while relying on renewable energy or waste streams enhances it (Fonteinis et al., 2018; Khan et al., 2019).
Another key question is how microalgal biomass is used. Several agri-food uses of microalgae offer possible pathways to deliver CC mitigation (Table 7). This includes using microalgae-based feeds to reduce deforestation pressures, replacing agro-chemical inputs with biofertilizers and biopesticides, and producing biochar to sequester CO2 in a stable form.
Carbon offsets are a major source of climate finance that can foster delivery of CC mitigation actions by entities such as businesses and charities (Ecosystem Marketplace, 2021). They involve buyers paying those who deliver verifiable CC mitigation outcomes to carbon markets via approved activities (Lee, 2017). If any agri-food applications of microalgae were formally recognized as offset options, this could provide a mechanism for those delivering these applications to earn income from these markets. While the theory that offsetting is mutually beneficial has sometimes proven problematic in practice (Watt, 2021), efforts to foster sound offsetting practice continue (Allen et al., 2020).
Notwithstanding such competing perspectives, one critical reality is that the agri-food uses of microalgae flagged as possible CC mitigation options could, it seems, also foster climate resilience based on the available evidence (Table 7). It follows that any offset payments to fund such options may foster both outcomes simultaneously. This would fit with the IPCC's suggestion that integrated measures which contribute to both mitigation and adaptation have a greater likelihood of being successful (IPCC, 2022c).
One factor that fundamentally affects any discussions about CC mitigation options is the carbon price. A higher carbon price could augment the profitability of activities or products that qualify as CC mitigation options, thus strengthening the incentives for economic actors to deliver them. Until 2018 the price of the leading carbon market was typically under €10/ton, but since early 2022 it has been approximately €80/ton, so prices have risen sharply (Trading Economics, 2022).
One risk is that efforts to explore such CC mitigation prospects may face accusations of “greenwashing” that could have a chilling effect. For instance, a leading environmentalist recently tweeted “Every sector needs to be decarbonised” and “offsetting… solves nothing” (Monbiot, 2021), while a leading climate scientist tweeted, “Normalize ridiculing carbon offsets and net zero” (Kalnus, 2021). Such critics support their arguments by citing troubling examples of immoral practice, like Shell telling its customers that thanks to its global portfolio of offset projects, “you don't even have to change the way you work” (Monbiot, 2021).
Regrettably, such blanket condemnations neglect economic realities, notably the fact that financial considerations can be key determinants of action. Poorer populations like small-scale farmers in the Global South may be especially unlikely to take innovative actions without clear incentives, given their often tenuous circumstances (Siedenburg et al., 2015). Such communities often address shocks or stresses via short-term coping responses rather than transformative adaptations, but strategic financial investments can help overcome barriers to more proactive responses (IPCC, 2022d). Given the scale of the climate challenge, it is to be hoped that such critics move toward being more constructive about offsetting, ideally by deploying their influence to improve practice and minimize abuses.
While agri-food applications of microalgae may offer potential CC mitigation opportunities, realizing this potential will depend on essential building blocks being in place. This includes further developing the relevant technologies and institutional factors like elaborating carbon market methodologies to enable selected microalgae technologies to qualify for mitigation finance.
Summarizing findings on microalgae and climate action
Table 7 summarizes this paper's analysis, highlighting how the five agri-food uses of microalgae examined might serve as the basis for climate action, including both adaptation to and mitigation of CC. In cases where microalgae are used for agricultural support, it should be noted this can be compatible with organic farming and the price premiums it offers (Colla and Rouphael, 2020). This summary assumes energy needs are met by renewable power given its plummeting costs, thus minimizing GHG emissions from energy. One factor not included in the table is how climate finance could potentially be provided to support initiatives on any of these technologies, and how such cashflows could represent an additional aspect of climate resilience for farming communities.
Potential risks and obstacles
Of the many microalgae species relatively few are well-known, yet several (e.g., Arthrospira, Chlorella, Dunaliella) have been designated as generally safe for human consumption by the regulatory authorities of key jurisdictions like the European Union (Niccolai et al., 2019) and United States (Chacón-Lee and González-Mariño, 2010).
Consumption of any food is not without risk, however, so potential harm to consumers must be considered. One possible concern is allergic reactions to eating microalgae. Another is contamination of microalgae with biotic pathogens like microcystins or abiotic toxins like heavy metals (Inthorn et al., 2002; Becker, 2013; Wells et al., 2017). Some early studies have sought to examine these linkages, but further research is needed. Al-Dhabi (2013) examined 25 commercial spirulina supplements and found that heavy metal levels were well below the allowable daily intake in all cases. Roy-Lachapelle et al. (2017) examined 18 microalgae supplements and found that four contained microcystins at levels exceeding tolerable daily intake.
Risk mitigation measures are clearly needed for microalgae destined for use as food or feed (Amorim et al., 2021). Microalgae cultivated in open ponds are more vulnerable to contamination (Heussner et al., 2012), while enclosed cultivation systems can reduce contamination risk but can also be capital-intensive (Linder, 2019). Hybrid options like open ponds within greenhouses offer scope to balance these considerations by reducing contamination risk cost-effectively (Lu et al., 2011). Another option that has shown promise is varying the temperature or salinity of the culture medium in ways that microalgae can tolerate but other species may not (Wang et al., 2016).
Despite growing use of microalgae as foods, consumer acceptance remains an issue, as does the affordability of microalgal biomass. These issues are likely to be key determinants of future demand for edible microalgae (Linder, 2019) and hence merit greater attention. One possible way to enhance consumer acceptance is raising awareness of the health and environmental benefits of microalgae (Linder, 2019), but early efforts to foster microalgae consumption suggest that information provision on its own may be ineffective due to strong cultural associations with food (AIM, 2016).
Research and policy priorities
While the literature reviewed suggests clear potential for the agri-food technologies examined to serve as the basis for climate actions, the evidence base remains thin. Research priorities include:
• Conducting further investigations of these agri-food technologies to explore and verify their suitability for building climate resilience and mitigating CC. This should include studies under real world conditions, since such studies are critical for assessing the viability of technologies.
• Identifying ways to lower the costs of microalgae production while maintaining quality standards, including developing improved “appropriate technology” production systems.
• Identifying effective, accessible strategies to minimize contamination risks to microalgae production.
• Trialing agri-food applications of microalgae with small-scale farmers in the Global South to assess their efficacy in a context that is a focus of global concerns about food insecurity and vulnerability to CC.
• Quantifying tons of CO2 equivalent that could be delivered by microalgae technologies identified as promising CC mitigation measures, coupled with developing improved and affordable measurement, reporting and verification measures.
• Assessing the scope for business engagement with deploying agri-food applications of microalgae as climate actions, given claims like “businesses are now in hyperdrive when it comes to climate (Financial Times, 2022).”
• Exploring why there is not greater interest in microalgae and their agri-food uses from governments and international agencies, given their technological promise.
• Examining why farmers and consumers do not show greater interest in microalgae as a crop and food given their promise, while also identifying and investigating any barriers to demand.
• Assessing whether other microalgae species besides those emphasized by research and commercial applications to date show promise as agri-food technologies given CC.
Policy measures would also be needed to elaborate and harness the technological potential suggested by this review. Policy priorities include (i) funding studies to address research priorities; (ii) incorporating these technological options into policy documents such as NDC plans and climate finance schemes; and (iii) developing carbon market methodologies for the most promising CC mitigation options.
Conclusions
Across the globe, CC poses a serious and growing risk to agricultural production and food security, especially for vulnerable populations like small-scale farmers in the Global South. Effective response actions are needed, notably ones that offer scope to secure continued agricultural production and ample, nutritious food despite CC. The academic evidence reviewed suggests that using microalgae as a food, feed or crop support technology shows promise in this regard for both vulnerable farmers and wider society, while also potentially offering CC mitigation options. Characteristics of microalgae that make them well-suited to facing the looming CC challenge include (i) growing fast and not needing arable land or regular rainfall, (ii) being highly nutritious as a food or feed, and (iii) capacity to support “climate smart” crop and livestock production. While the promise of these agri-food technologies is clear, further research is needed to explore and substantiate their potential, and to examine issues like risks and barriers to uptake. In the meantime, such technologies merit greater attention from practitioners like farmers and governments as promising and timely technologies.
Author contributions
JS conducted the literature search, analysis, and wrote the paper.
Acknowledgments
I would like to thank the various researchers on whose work this review builds for generating valuable evidence and analysis on these promising and potentially important technologies.
Conflict of interest
The author declares that the research was conducted in the absence of any commercial or financial relationships that could be construed as a potential conflict of interest.
Publisher's note
All claims expressed in this article are solely those of the authors and do not necessarily represent those of their affiliated organizations, or those of the publisher, the editors and the reviewers. Any product that may be evaluated in this article, or claim that may be made by its manufacturer, is not guaranteed or endorsed by the publisher.
Footnotes
1. ^“A human intervention to reduce the sources or enhance the sinks of GHGs...” (IPCC, 2014).
2. ^“The process of adjustment to actual or expected climate and its effects. In human systems, adaptation seeks to moderate or avoid harm or exploit beneficial opportunities…” (IPCC, 2014).
References
Abd El-Baky, H. H., El-Baz, F. K., and El Baroty, G. S. (2010). Enhancing antioxidant availability in wheat grains from plants grown under seawater stress in response to microalgae extract treatments. J. Sci. Food Agric. 90, 299–303. doi: 10.1002/jsfa.3815
Abdulqader, G., Barsanti, L., and Tredici, M. (2000). Harvest of Arthrospira platensis from Lake Kossorom (Chad) and its household usage among the Kanembu. J. Appl. Phycol. 12, 493–498. doi: 10.1023/A:1008177925799
Acquah, C., Tibbetts, S. M., Pan, S., and Udenigwe, C. (2020). “Nutritional quality and bioactive properties of proteins and peptides from microalgae,” in Handbook of Microalgae-Based Processes and Products: Fundamentals and Advances in Energy, Food, Feed, Fertilizer, and Bioactive Compounds, eds E. Jacob-Lopes, M. M. Maroneze, M. I. Queiroz, and L. Q. Zepka (Elsevier Inc.; Academic Press), 493–531. doi: 10.1016/B978-0-12-818536-0.00019-1
Adger, W. N., Pulhin, J. M., Barnett, J., Dabelko, G. D., Hovelsrud, G. K., Levy, M., et al. (2014). “Human security,” in Climate Change 2014: Impacts, Adaptation, and Vulnerability. Part A: Global and Sectoral Aspects, eds C. B. Field, V. R. Barros, D. J. Dokken, K. J. Mach, M. D. Mastrandrea, T. E. Bilir, M. Chatterjee, et al., Contribution of Working Group II to the Fifth Assessment Report of the Intergovernmental Panel on Climate Change (Cambridge: Cambridge University Press), 755–791. Available online at: https://www.ipcc.ch/site/assets/uploads/2018/02/WGIIAR5-Chap12_FINAL.pdf.
AIM (2016). India's Spirulina Conundrum. Algae Industry Magazine Available online at: India's spirulina conundrum - algaeindustrymagazine.com (accessed June 9, 2022).
Al-Dhabi, N. A. (2013). Heavy metal analysis in commercial Spirulina products for human consumption. Saudi J. Biol. Sci. 20, 383–388. doi: 10.1016/j.sjbs.2013.04.006
AlgaEurope (2021). AlgaEurope Conference. Available online at: https://algaeurope.org (accessed June 10, 2022).
Allen, M., Axelsson, K., Caldecott, B., Hale, T., Hepburn, C., Hickey, C., et al (2020). The Oxford Principles for Net Zero Aligned Carbon Offsetting. Smith School of Enterprise and the Environment; University of Oxford. Available online at: https://www.smithschool.ox.ac.uk/research/oxford-offsetting-principles
Amorim, M.L, Soares, J., Coimbra, J. S. R., Leite, M. O., Albino, L.F. T. , et al. (2021). Microalgae proteins: production, separation, isolation, quantification and application in food and feed. Crit. Rev. Food Sci. Nutr. 61, 1976–2002. doi: 10.1080/10408398.2020.1768046
Batista, A. P., Niccolai, A., Fradinho, P., Fragoso, S., Bursic, I., Rodolfi, L., et al. (2017). Microalgae biomass as an alternative ingredient in cookies: sensory, physical and chemical properties, antioxidant activity and in vitro digestibility. Algal Res. 26, 161–171. doi: 10.1016/j.algal.2017.07.017
Becker, E. W. (2013). “Microalgae for human and animal nutrition,” in Handbook of Microalgal Culture, ed A. Richmond and Q. Hu (Oxford, UK: John Wiley and Sons, Ltd.), 461–503. doi: 10.1002/9781118567166.ch25
BHU (2022). Understanding Biofertilisers and Biostimulants via on-Farm Trials. Biological Husbandry Unit Organics Trust Future Farming Centre. Available online at: bhu.org.nz (accessed June 10, 2022).
Biancardi, E., McGrath, J. M., Panella, L. W., Lewellen, R. T., and Stevanato, P. (2010). “Sugar beet,” in Handbook of Plant Breeding, Tuber and Root Crops, Vol. 4, ed J. Bradshaw (New York, NY: Springer), 173–219. doi: 10.1007/978-0-387-92765-7_6
Boeckaert, C., Vlaeminck, B., Dijkstra, J., Issa-Zacharia, A., Van Nespen, T., Van Straalen, W., et al. (2008). Effect of dietary starch or micro algae supplementation on rumen fermentation and milk fatty acid composition of dairy cows. J. Dairy Sci. 91, 4714–4727. doi: 10.3168/jds.2008-1178
Bushong, J. T., Miller, E. C., Mullock, J. L., Arnall, D. B., and Raun, W. R. (2016). Irrigated and rain-fed maize response to different nitrogen fertilizer application methods. J. Plant Nutr. 39, 1874–1890. doi: 10.1080/01904167.2016.1187747
Catley, A., Admassu, B., Bekele, G., and Abebe, D. (2014). Livestock mortality in pastoralist herds in Ethiopia and implications for drought response. Disasters 38, 500–516. doi: 10.1111/disa.12060
Chacón-Lee, T. L., and González-Mariño, G. E. (2010). Microalgae for 'healthy' foods - possibilities and challenges. Comprehens. Rev. Food Sci. Food Saf. 9, 655–675. doi: 10.1111/j.1541-4337.2010.00132.x
Chang, Y. M., Tsai, W. T., and Li, M. H. (2015). Chemical characterisation of char derived from slow pyrolysis of microalgal residue. J. Anal. Appl. Pyrolysis 111, 88–93. doi: 10.1016/j.jaap.2014.12.004
Chen, J., Wang, P., Ding, L., Yu, T., Leng, S., Chen, J., et al. (2021). The comparison study of multiple biochar stability assessment methods. J. Analyt. Appl. Pyrolys. 156:105070. doi: 10.1016/j.jaap.2021.105070
Chia, S. Y., Macharia, J., Diiro, G. M., Kassie, M., Ekesi, S., Van Loon, J. J. A., et al. (2020). Smallholder farmers' knowledge and willingness to pay for insect-based feeds in Kenya. PLoS ONE 15:e0230552. doi: 10.1371/journal.pone.0230552
Ciani, M., Lippolis, A., Fava, F., Rodolfi, L., Niccolai, A., and Tredici, M. R. (2021). Microbes: food for the future. Foods 10:971. doi: 10.3390/foods10050971
Ciferri, O. (1983). Spirulina, the edible microorganism. Microbiol. Rev. 47, 551–578. doi: 10.1128/mr.47.4.551-578.1983
Colla, G., and Rouphael, Y. (2020). Microalgae: new source of plant biostimulants. Agronomy 10:1240. doi: 10.3390/agronomy10091240
COP 26 (2021). 26th United Nations Climate Change Conference of the Parties. Available online at: https://www.un.org/en/climatechange/cop26 (accessed June 20, 2022).
Cordeiro, E. C. N., Mógor, Á. F., de Oliveira Amatussi, J., Mógor, G., de Lara, G. B., and Marques, H. M. C. (2022). Microalga biofertilizer triggers metabolic changes improving onion growth and yield. Horticulturae 8:223. doi: 10.3390/horticulturae8030223
Cordeiro, M. R. C., Mengistu, G. F., Pogue, S. J., Legesse, G., Gunte, K. E., Taylor, A. M., et al. (2022). Assessing feed security for beef production within livestock-intensive regions. Agric. Syst. 196:103348. doi: 10.1016/j.agsy.2021.103348
Damodaran, S., Parkin, K. L., and Fennema, O. R. (2007). Fenneman's Food Chemistry, 4th Edn. Boca Raton, FL: CRC Press.
Du Jardin, P. (2015). Plant biostimulants: definition, concept, main categories and regulation. Sci. Horitic. 196, 3–14. doi: 10.1016/j.scienta.2015.09.021
Dumont, B., Groot, J. C. J., and Tichit, M. (2018). Review: make ruminants green again - How can sustainable intensification and agroecology converge for a better future? Animal 12, s210–s219. doi: 10.1017/S1751731118001350
Ecosystem Marketplace (2021). Markets in Motion: State of the Voluntary Carbon Markets 2021. 'Markets in Motion', State of the Voluntary Carbon Markets 2021, Installment 1. Ecosystem Marketplace. Available online at: ecosystemmarketplace.com.
Ekinci, K., Erdal, I., Uysal, O., Uysal, F. O., Tunce, H., and Dogan, A. (2019). Anaerobic digestion of three microalgae biomasses and assessment of digestates as biofertilizer for plant growth. Environ. Prog. Sustain. Energy 38:e13024. doi: 10.1002/ep.13024
Ellis, N. R., and Tschakert, P. (2019). Triple-wins as pathways to transformation? A critical review. Geoforum 103, 167–169. doi: 10.1016/j.geoforum.2018.12.006
Erenstein, O., Chamberlin, J., and Sonder, K. (2021). Farms worldwide: 2020 and 2030 outlook. Outlook Agric. 50, 221–229. doi: 10.1177/00307270211025539
Eyster, K. M. (2007). The membrane and lipids as integral participants in signal transduction: lipid signal transduction for the non-lipid biochemist. Adv. Physiol. Educ. 31, 5–16. doi: 10.1152/advan.00088.2006
FAO (2013). Climate-Smart Agriculture Sourcebook. Rome: Food and Agriculture Organization of the United Nations.
FAO (2021). State of Food Security and Nutrition in the World 2021. Rome: United Nations Food and Agriculture Organization.
Financial Times (2022). The Corporate World's Tipping Point on Climate. Partner content from Lloyds' Bank. Available online at: https://www.ft.com/partnercontent/lloyds-bank/the-corporate-worlds-tipping-point-on-climate.html (accessed May 9, 2022).
Fonteinis, S., Antoniadis-Gavriil, A., and Tsoutsos, T. (2018). Life cycle assessment of algae-to-biodiesel shallow pond production systems in the Mediterranean: influence of species, pond type, by(co)-product, valorisation and electricity mix. Biofuels Bioprod. Biorefining 12, 542–558. doi: 10.1002/bbb.1871
Fradinho, P., Niccolai, A., Soares, R., Rodolfi, L., Biondi, N., Tredici, M. R., et al. (2020). Effect of Arthrospira platensis (spirulina) incorporation on the rheological and bioactive properties of gluten-free fresh pasta. Algal Res. 45:101743. doi: 10.1016/j.algal.2019.101743
Gantar, M., and Svircev, Z. (2008). Microalgae and cyanobacteria: food for thought. J. Phycol. 44, 260–268. doi: 10.1111/j.1529-8817.2008.00469.x
Garcia-Gonzalez, J., and Sommerfeld, M. (2016). Biofertilizer and biostimulant properties of the microalga Acutodesmus dimorphus. J. Appl. Phycol. 28, 1051–1061. doi: 10.1007/s10811-015-0625-2
Gerber, P. J., Steinfeld, H., Henderson, B., Mottet, A., Opio, C., Dijkman, J., et al (2013). Tackling Climate Change Through Livestock - a Global Assessment of Emissions and Mitigation Opportunities. Rome: United Nations Food and Agriculture Organization. Available online at: http://www.fao.org/3/a-i3437e.pdf
Global Methane Pledge (2021). Fast Action on Methane to Keep a 1.5 Future Within Reach. Global Methane Pledge (accessed April 28, 2022).
Gong, X., Zhang, B., Zhang, Y., Huang, Y., and Xu, M. (2014). Investigation on pyrolysis of low lipid microalgae Chlorella vulgaris and Dunaliella salina. Energy Fuels 28, 95–103. doi: 10.1021/ef401500z
Guéneau, S. (2018). Neoliberalism and the emergence of private sustainability initiatives: the case of the Brazilian cattle value chain. Bus. Strat. Environ. 27, 240–251. doi: 10.1002/bse.2013
Guo, J. H., Liu, X., J; Zhang, Y., Shen, J. L., Han, W. X., Zhang, W. F., et al. (2010). Significant acidification in major Chinese croplands. Science. 327, 1008–1010. doi: 10.1126/science.1182570
Guo, S., Wang, P., Wang, X., Zou, M., Liu, C., and Hao, J. (2020). “Microalgae as biofertilizer in modern agriculture,” in Microalgae Biotechnology for Food, Health and High Value Products, eds M. Alam, J. L. Xu and Z. Wang (Singapore: Springer). 397–411. doi: 10.1007/978-981-15-0169-2_12
Guzmán-Murillo, M. A., Ascencio, F., and Larrinaga-Mayoral, J. A. (2013). Germination and ROS detoxification in bell pepper (Capsicum annuum L.) under NaCl stress and treatment with microalgae extracts. Protoplasma 250, 33–42. doi: 10.1007/s00709-011-0369-z
Habib, M. A. B., Parvin, M., Huntington, T. C., and Hasan, M. R. (2008). A Review on the Culture, Production and Use of Spirulina as a Food for Humans and Feeds for Domestic Animals and Fish. FAO Fisheries and Aquaculture Circular No. 1034. United Nations Food and Agriculture Organization. Available online at: https://www.fao.org/3/i0424e/i0424e.pdf
Halmemies-Beauchet-Filleau, A., Rinne, M., Lamminen, M., Mapato, C., Ampapon, T., Wanapat, M., et al. (2018). Alternative and novel feeds for ruminants: nutritive value, product quality and environmental aspects. Animal 12, s295–s309. doi: 10.1017/S1751731118002252
Hendrix, C., and Brinkman, H. J. (2013). Food insecurity and conflict dynamics: Causal linkages and complex feedbacks. Stability. Int. J. Secur. Develop. 2, 26. doi: 10.5334/sta.bm
Heussner, A. H., Mazija, L., Fastner, J., and Dietrich, D. R. (2012). Toxin content and cytotoxicity of algal dietary supplements. Toxicol. Appl. Pharmacol. 265, 263–271. doi: 10.1016/j.taap.2012.10.005
Hof, G., Tamminga, S., and Lenaers, P. J. (1994). Efficiency of protein utilization in dairy cows. Livestock Prod. Sci. 38, 169–178. doi: 10.1016/0301-6226(94)90168-6
Holdt, S. L., and Kraan, S. (2011). Bioactive compounds in seaweed: functional food applications and legislation. J. Appl. Phycol. 23, 543–597. doi: 10.1007/s10811-010-9632-5
Hosseini, S. M., Khosravi-Darani, K., and Mozafari, M. R. (2013). Nutritional and medical applications of spirulina microalgae. Mini Rev. Med. Chem. 13, 1231–1237. doi: 10.2174/1389557511313080009
IEP (2020). Ecological Threat Register 2020: Understanding Ecological Threats, Resilience and Peace. Report to the Institute for Economics and Peace. Available online at: https://www.visionofhumanity.org/wp-content/uploads/2020/10/ETR_2020_web-1.pdf
International Biochar Initiative (2022). Available online at: https://biochar-international.org (accessed May 12, 2022).
Inthorn, D., Nalin, S., Suthep, S., and Incharoensakdi, A. (2002). Sorption of mercury, cadmium and lead by microalgae. ScienceAsia 28:253. doi: 10.2306/scienceasia1513-1874.2002.28.253
IPCC (2014). “Source: IPCC, 2014: Annex II: Glossary,” in Climate Change 2014: Synthesis Report. Contribution of Working Groups I, II and III to the Fifth Assessment Report of the Intergovernmental Panel on Climate Change, eds Core Writing Team, R. K. Pachauri, and L. A. Meyer (Geneva: IPCC), 117–130.
IPCC (2022a). “Summary for policymakers,” in Climate Change 2022: Impacts, Adaptation, and Vulnerability. Contribution of Working Group II to the Sixth Assessment Report of the Intergovernmental Panel on Climate Change, eds H.-O. Pörtner, D. C. Roberts, E. S. Poloczanska, K. Mintenbeck, M. Tignor, A. Alegría, M. Craig, S. Langsdorf, S. Löschke, V. Möller, A. Okem (Cambridge University Press).
IPCC (2022b). Chapter 5: Food, Fibre and Other Ecosystem Products. From IPCC WGII Sixth Assessment Report: Impacts, Adaptation, and Vulnerability. IPCC. Available online at: https://www.ipcc.ch/report/ar6/wg2/
IPCC (2022c). Chapter 7: Agriculture, Forestry and Other Land Uses (AFOLU). From IPCC WGIII Sixth Assessment Report: Mitigation of Climate Change. IPCC. Available online at: https://www.ipcc.ch/report/ar6/wg3/
IPCC (2022d). Chapter 9: Africa. From IPCC WGII Sixth Assessment Report: Impacts, Adaptation, and Vulnerability. IPCC. Available online at: https://www.ipcc.ch/report/ar6/wg2/
IPCC (2022e). Chapter 18: Climate Resilient Development Pathways. From IPCC WGII Sixth Assessment Report: Impacts, Adaptation, and Vulnerability. IPCC. Available online at: https://www.ipcc.ch/report/ar6/wg2/
IPCC (2022f). Full reports of WGII and WGIII. IPCC. Available online at: https://www.ipcc.ch/report/ar6
IRENA (2022). Renewable Power Generation Costs in 2021. International Renewable Energy Agency. Abu Dhabi: IRENA.
Jha, M. N., and Prasad, A. N. (2006). Efficacy of new inexpensive cyanobacterial biofertilizer including its shelf-life. World J. Microbiol. Biotechnol. 22, 73–79. doi: 10.1007/s11274-005-7024-9
Kalnus, P. (2021). Tweet from Peter Kalnus @ClimateHuman. Available online at: https://twitter.com/ClimateHuman/status/1388499799322763269 (accessed May 1, 2021).
Kebede, E., and Ahlgren, G. (1996). Optimum growth conditions and light utilization efficiency of Spirulina platensis (= Arthrospira fusiformis) (Cyanophyta) from Lake Chitu, Ethiopia. Hydrobiologica 332, 99–109. doi: 10.1007/BF00016689
Khan, S. A., Sharma, G. K., Malla, F. A., Kumar, A., and Gupta, N. (2019). Microalgae based biofertilizers: a biorefinery approach to phycoremediate wastewater and harvest biodiesel and manure. J. Clean. Prod. 211, 1412–1419. doi: 10.1016/j.jclepro.2018.11.281
Kheirfam, H., Sadeghi, S. H., Zarei Darki, B., and Homaee, M. (2017). Controlling rainfall-induced soil loss from small experimental plots through inoculation of bacteria and cyanobacteria. Catena 152, 40–46. doi: 10.1016/j.catena.2017.01.006
Kholif, A. E., Morsy, T. A, Matloup, O. H., Anele, U. Y., Mohamed, A. G., and ElSayed, A. B. (2017). Dietary Chlorella vulgaris microalgae improves feed utilization, milk production and concentrations of conjugated linoleic acids in the milk of damascus oats. J. Agric. Sci. 155, 508–518. doi: 10.1017/S0021859616000824
Kholssi, R., Ramos, P., V; Marks, E. A., N; Montero, O., and Rad, C. (2021). Biotechnological uses of microalgae: a review on the state of the art and challenges for the circular economy. Biocatal. Agric. Biotechnol. 36:102114. doi: 10.1016/j.bcab.2021.102114
Kinley, R. D., Nys, R., Vucko, M. J., Machado, L., and Tomkins, N. W. (2016). The red macroalgae Asparagopsis taxiformis is a potent natural antimethanogenic that reduces methane production during in vitro fermentation with rumen fluid. Anim. Prod. Sci. 56, 282–289. doi: 10.1071/AN15576
Knapp, J. R., Laur, G. L., Vadas, P. A., Weiss, W. P., and Tricarico, J. M. (2014). Enteric methane in dairy cattle production: quantifying the opportunities and impact of reducing emissions. J. Dairy Sci. 97, 3231–3261. doi: 10.3168/jds.2013-7234
Krieger, T., Panke, D., and Pregernig, M. (2020). Environmental Conflicts, Migration and Governance. Bristol: Bristol University Press. doi: 10.1332/policypress/9781529202168.001.0001
Kumar, M., Prasanna, R., Bidyarani, N., Babu, S., Mishra, B. K., Kumar, A., et al. (2013). Evaluating the plant growth promoting ability of thermotolerant bacteria and cyanobacteria and their interactions with seed spice crops. Sci. Hortic. 164, 94–101. doi: 10.1016/j.scienta.2013.09.014
Lamminen, M., Halmemies-Beauchet-Filleau, A., Kokkonen, T., Jaakkola, S., and Vanhatalo, A. (2019). Different microalgae species as a substitutive protein feed for soya bean meal in grass silage based dairy cow diets. Anim. Feed Sci. Technol. 247, 112–126. doi: 10.1016/j.anifeedsci.2018.11.005
Lamminen, M., Halmemies-Beauchet-Filleau, A., Kokkonen, T., Simpura, I., Jaakkola, S., and Vanhatalo, A. (2017). Comparison of microalgae and rapeseed meal as supplementary protein in the grass silage based nutrition of dairy cows. Anim. Feed Sci. Technol. 234, 295–311. doi: 10.1016/j.anifeedsci.2017.10.002
Lee, J. (2017). Farmer participation in a climate-smart future: evidence from Kenya Agriculgtural Carbon Project. Land Use Policy 68, 72–79. doi: 10.1016/j.landusepol.2017.07.020
Lehmann, J., and Joseph, S. (2015). Biochar for Environmental Management: Science, Technology and Implementation, 2nd Edn. Oxford: Routledge. doi: 10.4324/9780203762264
Lindberg, J. E., Lindberg, G., Teräs, J., Poulsen, G., Solberg, S. Ø., Tybirk, K., et al. (2016). Nordic Alternative Protein Potentials: Mapping of Regional Bioeconomy Opportunities. Nordic Council of Ministers. Available online at: http://www.nordic-ilibrary.org/environment/nordic-alternative-protein-potentials_tn2016-527
Linder, T. (2019). Making the case for edible microorganisms as an integral part of a more sustainable and resilient food production system. Food Sec. 11, 265–278. doi: 10.1007/s12571-019-00912-3
Lu, Y. M., Xiang, W., and Wen, Y. H. (2011). Spirulina (Arthrospira) industry in Inner Mongolia of China: current status and prospects. J. Appl. Phycol. 23, 265–269. doi: 10.1007/s10811-010-9552-4
Machado, L., Carvalho, G., and Pereira, R. N. (2022). Effects of innovative processing methods on microalgae cell wall: prospects towards digestibility of protein-rich biomass. Biomass 2, 80–102. doi: 10.3390/biomass2020006
Makkar, H. P. S. (2018). Feed demand landscape and implications of food-not feed strategy for food security and climate change. Animal 12, 1744–1754. doi: 10.1017/S175173111700324X
Makkar, H. P. S., Tran, G., Heuzé, V., Giger-Reverdin, S., Lessire, M., Lebas, F., et al. (2016). Seaweeds for livestock diets: a review. Anim. Feed Sci. Technol. 212, 1–17. doi: 10.1016/j.anifeedsci.2015.09.018
Malam Issa, O., Défarge, C., Le Bissonnais, Y., Marin, B., Duval, O., Bruand, A., et al. (2007). Effects of the inoculation of cyanobacteria on the microstructure and the structural stability of a tropical soil. Plant Soil 290, 209–219. doi: 10.1007/s11104-006-9153-9
Maluleke, W., and Mokwena, R. J. (2017). The effect of climate change on rural livestock farming: case study of Giyani Policing Area, Republic of South Africa. South Afr. J. Agric. Extens. 45, 26–40. doi: 10.17159/2413-3221/2017/v45n1a404
Mancuso, S., Azzarello, E., Mugnai, S., and Briand, X. (2006). Marine bioactive substances (IPA extract) improve foliar ion uptake and water stress tolerance in potted Vitis vinifera plants. Adv. Hortic. Sci. 20, 156–161. Available online at: http://www.jstor.org/stable/42882475
Martin, C., Butelli, E., Petroni, K., and Tonelli, C. (2011). How can research on plants contribute to promoting human health? Plant Cell 23, 1685–1699. doi: 10.1105/tpc.111.083279
Martini, F., Beghini, G., Zanin, L., Varanini, Z., Zamboni, A., and Ballottari, M. (2021). The potential use of Chlamydomonas reinhardtii and Chlorella sorokiniana as biostimulants on maize plants. Algal Res. 60:102515. doi: 10.1016/j.algal.2021.102515
Mata, L., Magnusson, M., Paul, N. A., and de Nys, R. (2015). The intensive land-based production of the green seaweeds Derbesia tenuissima and Ulva ohnoi: biomass and bioproducts. J. Appl. Phycol. 28, 365–375. doi: 10.1007/s10811-015-0561-1
Meale, S. J., Chaves, A. V., He, M. L., and McAllister, T. A. (2014). Dose-response of supplementing marine algae (Schizochytrium spp.) on production performance, fatty acid profiles, and wool parameters of growing lambs. J. Anim. Sci. 92, 2202–2213. doi: 10.2527/jas.2013-7024
Merz, C., and Main, K. (2014). “Microalgae (Diatom) production - the aquaculture and biofuel nexus,” in 2014 Oceans - St. John's (St. John's, NL). doi: 10.1109/OCEANS.2014.7003242
Mihaila, A. A., Glasson, C. R. K., Lawton, R., Muetzel, S., Molano, G., and Magnusson, M. (2022). New temperate seaweed targets for mitigation of ruminant methane emissions: an in vitro assessment. Appl. Phycol. 1–11. doi: 10.1080/26388081.2022.2059700
Monbiot, G. (2021). Tweet from George Monbio @GeorgeMonbiot. Available online at: https://twitter.com/GeorgeMonbiot/status/1360994456036605957 (accessed February 14, 2021).
Morao, A., North-Davis, N., Morao, A., Kauffman, J. J., and Shen, L. (2021). “Algae omega-3s as a sustainable alternative to fish oil,” in Paper Delivered at the AlgaEurope 2021 Virtual Conference (AlgaEurope 2021 Organisers).
Murata, M. M., Morioka, L. R. I., Da Silva Marques, J. B., Bosso, A., and Sugimoto, H. H. (2021). What do patents tell us about microalgae in agriculture? AMB Express 11:154. doi: 10.1186/s13568-021-01315-4
Navarro-López, E., Ruíz-Nieto, A., Ferreira, A., Gabriel Acién, F., and Gouveia, L. (2020). Biostimulant potential of Scenedesmus obliquus grown in brewery wastewater. Molecules 25, 1–16. doi: 10.3390/molecules25030664
Niccolai, A., Zittelli, G. C., Rodolfi, L., Biondi, N., and Tredici, M. R. (2019). Microalgae of interest as a food source: biochemical composition and digestibility. Algal Res. 42:101617. doi: 10.1016/j.algal.2019.101617
Oancea, F., Velea, S., Fãtu, V., Mincea, C., and Ilie, L. (2013). Micro-algae based plant biostimulant and its effect on water stressed tomato plants. Roman. J. Plant Protect. 6, 104–117. Available online at: https://www.cabdirect.org/cabdirect/abstract/20143380895
Odhiambo, J. J. O., and Magandini, V. N. (2020). An assessment of the use of mineral and organic fertilizers by small holder farmers in Vhembe district, Limpopo province, South Africa. Int. J. Manures Fertil. 8, 1–6. Available online at: https://www.internationalscholarsjournals.com/articles/an-assessment-of-the-use-of-mineral-and-organicfertilizers-by-smallholder-farmers-in-vhembedistrict-limpopo-province-sou.pdf
Padfield, D., Yvon-Durocher, G., Buckling, A., Jennings, S., and Yvon-Durocher, G. (2016). Rapid evolution of metabolic traits explains thermal adaptation in phytoplankton. Ecol. Lett. 19, 133–142. doi: 10.1111/ele.12545
Petersen, J., Rredhi, A., Szyttenholm, J., Oldemeyer, S., Kittke, T., and Mittag, M. (2021). The world of algae reveals a broad variety of cryptochrome properties and functions. Front. Plant Sci. 12:766509. doi: 10.3389/fpls.2021.766509
Piccolo, A. (2011). Spirulina: A Livelihood and a Business Venture. United Nations Food and Agriculture Organization and Indian Ocean Commission. Available online at: www.fao.org/3/az386e/az386e.pdf
Piccolo, A., and Short, C. (2014). Spirulina Production in the ESA-IO Region: The Way Forward. United Nations Food and Agriculture Organization and Indian Ocean Commission. Available online at: https://www.fao.org/3/br807e/br807e.pdf
Pimbert, M. (2012). Fair and Sustainable Food Systems: From Vicious Cycles to Virtuous Circles. International Institute for Environment and Development Briefing Paper.
Pires, J. C. M., Goncalves, A. L., Martins, F. G., Alvim-Ferraz, M. C. M., and Simoes, M. (2014). Effect of light supply on CO2 capture from atmosphere by Chlorella vulgaris and Peudokirchneriella subcapitata. Mitigat. Adaptat. Strat. Glob. Change 19, 1109–1117. doi: 10.1007/s11027-013-9463-1
Qinghua, W., Lian, L., Miron, A., Klimova, B., Wan, D., and Kuca, K. (2016). The antioxidant, immunomodulatory, and anti-inflammatory activities of spirulina: an overview. Arch. Toxicol. 90, 1817–1849. doi: 10.1007/s00204-016-1744-5
Quandt, A., Neufeldt, H., and McCabe, J. T. (2017). The role of agroforestry in building livelihood resilience to floods and drought in semiarid Kenya. Ecol. Soc. 22:10. doi: 10.5751/ES-09461-220310
Renuka, N., Prasanna, R., Sood, A., et al. (2016). Exploring the efficacy of wastewater-grown microalgal biomass as a biofertilizer for wheat. Environ. Sci. Pollut. Res. 23, 6608–6620. doi: 10.1007/s11356-015-5884-6
Roberts, K. G., Gloy, B. A., Joseph, S., Scott, N. R., and Lehmann, J. (2010). Life cycle assessment of biochar systems: estimating the energetic, economic and climate change potential. Environ. Sci. Technol. 44, 827–833. doi: 10.1021/es902266r
Ronga, D., Biazzi, E., Parati, K., Carminati, D., Carminati, E., and Tava, A. (2019). Microalgal biostimulants and biofertilisers in crop productions. Agronomy 9:192. doi: 10.3390/agronomy9040192
Ronga, D., Rizza, F., Badeck, F. W., Milc, J., Laviano, L., Montevecchi, G., et al. (2018). Physiological responses to chilling in cultivars of processing tomato released and cultivated over the past decades in Southern Europe. Sci. Hortic. 231, 118–125. doi: 10.1016/j.scienta.2017.12.033
Roy-Lachapelle, A., Solliec, M., Bouchard, M., and Sauvé, S. (2017). Detection of cyanotoxins in algae dietary supplements. Toxins 9:76. doi: 10.3390/toxins9030076
Santini, G., Rodolfi, L., Biondi, N., Sampietro, G., Mattii, G., and Tredici, M. R. (2021). “Arthrospira-based biostimulants and their effects on different plants,” in Paper Delivered at the Virtual AlgaEurope Conference (Florence: University of Florence).
Sanyal, D., Kodgire, S., Desai, D., Saxena, N., Singh, S. D., and Dasgupta, S. (2020). “Commitment for a cleaner india: Utilization of CO2 and sewage wastewater by green algae scenedesmus sp. under laboratory conditions,” in Renewable Energy and Climate Change: Proceedings of REC 2019, eds D. Deb, A. Dixit, and L. Chandra (Singapore: Springer Nature). doi: 10.1007/978-981-32-9578-0_25
Seaweed Climate (2022). Seaweed as a Nature-Based Climate Solution: Vision Statement. Seaweed Climate. Available online at: seaweedclimatesolution.com (accessed June 1, 2022).
Shah, A. A., Totakul, P., Matra, M., Cherdthong, A., Hanboonsong, Y., and Wanapat, M. (2022). Nutritional composition of various insects and potential uses as alternative protein sources in animal diets. Anim. Biosci. 35, 317–331. doi: 10.5713/ab.21.0447
Shaw, G. R., Moore, D. P., and Garnett, C. M. (2003). “Eutrophication and algal blooms,” in Encyclopedia of Life Support Systems (EOLSS), Volume II, Environmental and Ecological Chemistry. Paris: United Nations Educational, Scientific and Cultural Organization. Available online at: http://www.eolss.net/Sample-Chapters/C06/E6-13-04-04.pdf (accessed February 1, 2022).
Siedenburg, J. (2021). Perils facing Kenyan pastoralists, livelihood innovations and wider impacts: learning from project experience. Dev. Stud. Res. 8, 218–235. doi: 10.1080/21665095.2021.1961595
Siedenburg, J., Brown, S., and Hoch, S. (2015). Voices from the field: carbon markets and rural poverty as seen from Madagascar and Mali. Clim. Dev. 8, 10–25. doi: 10.1080/17565529.2014.998602
Sills, D. L., Van Doren, L. G., Beal, C., and Raynor, E. (2021). The effect of functional unit and co-product handling methods on life cycle assessment of an algal biorefinery. Algal Res. 46, 101770. doi: 10.1016/j.algal.2019.101770
Spokas, K. A. (2010). Review of the stability of biochar in soils: predictability of O:C molar ratios. Carbon Manag. 1, 289–303. doi: 10.4155/cmt.10.32
Stabler, S. P., and Allen, R. H. (2004). Vitamin B12 eficiency as a worldwide problem. Annu. Rev. Nutr. 24, 299–232 doi: 10.1146/annurev.nutr.24.012003.132440
Suchithra, M. R., Muniswami, D. M., Sri, M. S., Usha, R., and Rasheeq, A. A. (2022). Effectiveness of green microalgae as biostimulants and biofertilizer through foliar spray and soil drench method for tomato cultivation. South Afr. J. Bot. 146 740–750. doi: 10.1016/j.sajb.2021.12.022
Thompson, T. (2021). Young peoples' climate anxiety revealed in landmark survey. Nature 597:605. doi: 10.1038/d41586-021-02582-8
Tibbetts, S. M. (2018). “The potential for ‘next-generation', microalgae-based feed ingredients for salmonid aquaculture in context of the blue revolution,” in Microalgal Biotechnology, eds E. Jacob-Lopes, L. Q. Zepka, and M. I. Queiroz (InTech Open Publishing), 151–175.
Tibbetts, S. M., Whitney, C. G., MacPherson, M. J., Bhatti, S., Banskota, A. H., Stefanova, R., et al. (2015). Biochemical characterization of microalgal biomass from freshwater species isolated in Alberta, Canada for animal feed applications. Algal Res. 11, 435–447. doi: 10.1016/j.algal.2014.11.011
Trading Economics (2022). EU Carbon Permits - 2022 Data - 2005-2021 Historical - 2023 Forecast - Price - Quote. Available online at: tradingeconomics.com (accessed June 12, 2022).
Tredici, M. R. (2010). Photobiology of microalgae mass cultures: understanding the tools for the next green revolution. Biofuels 1, 143–162. doi: 10.4155/bfs.09.10
Trejo, A., De-Bashan, L. E., Hartmann, A., et al. (2012). Recycling waste debris of immobilized microalgae and plant growth-promoting bacteria from wastewater treatment as a resource to improve fertility of eroded desert soil. Environ. Exp. Bot. 75, 65–73. doi: 10.1016/j.envexpbot.2011.08.007
Tzachor, A., Richards, C. E., and Holt, L. (2021). Future foods for risk-resilient diets. Nat. Food 2021, 326–329. doi: 10.1038/s43016-021-00269-x
United Nations (2021a). Food Systems Summit 2021. United Nations. Available online at: https://www.un.org/en/food-systems-summit/summit (accessed June 18, 2022).
United Nations (2021b). Nationally Determined Contributions Registry. Available online at: https://unfccc.int/NDCREG (accessed September 30, 2021).
UNRIC (2021). Guterres: The IPCC Report Is a Code Red for Humanity. United Nations in Western Europe. Available online at: https://unric.org/en/guterres-the-ipcc-report-is-a-code-red-for-humanity/ (accessed June 19, 2022).
Uribe-Wandurraga, Z. N., Igual, M., García-Segovia, P., and Martínez-Monzó, J. (2019). Effect of microalgae addition on mineral content, colour and mechanical properties of breadsticks. Food Funct. 10, 4685–4692. doi: 10.1039/C9FO00286C
Uysal, O., Ozdemir, F. O., and Ekinci, K. (2015). Evaluation of microalgae as microbial fertilizer. Eur. J. Sustain. Dev. 4, 77–82. doi: 10.14207/ejsd.2015.v4n2p77
Van Emon, M. L., Loy, D. D., and Hansen, S. L. (2015). Determining the preference, in vitro digestibility, in situ disappearance, and grower period performance of steers fed a novel algae meal derived from heterotrophic microalgae. J. Anim. Sci. 93, 3121–3129. doi: 10.2527/jas.2014-8654
Van Huis, A., Dicke, M., and Van Loon, J. J. A. (2015). Insects to feed the world. J. Insects Food Feed. 1, 3–5. doi: 10.3920/JIFF2015.x002
Van Oosten, M. J., Pepe, O., Pascale, S. D., Silletti, S., and Maggio, A. (2017). The role of biostimulants and bioeffectors as alleviators of abiotic stress in crop plants. Chem. Biol. Technol. Agric. 4:5. doi: 10.1186/s40538-017-0089-5
Vernieri, P., Borghesi, E., Ferrante, A., and Magnani, G. (2005). Application of biostimulants in floating system for improving rocket quality. J. Food Agric. Environ. 33, 86–88. Available online at: https://typeset.io/papers/application-of-biostimulants-in-floating-system-for-1gbibzaype
Viegas, C., Gouveia, L., and Goncalves, M. (2021a). Evaluation of microalgae as bioremediation agent for poultry effluent and biostimulant for germination. Environ. Technol. Innov. 24:102048. doi: 10.1016/j.eti.2021.102048
Viegas, C., Gouveia, L., and Gonçalves, M. (2021b). Aquaculture wastewater treatment through microalgal, biomass potential applications on animal feed, agriculture, and energy. J. Environ. Manag. 286:112187. doi: 10.1016/j.jenvman.2021.112187
Walsh, B. J., Rydzak, F., Palazzo, A., Kraxner, F., Herrero, M., Schenk, P. M., et al. (2015). New feed sources key to ambitious climate targets. Carbon Balance Manag. 10:26. doi: 10.1186/s13021-015-0040-7
Wang, K., Brown, R., Homsy, S., Martinez, L., and Sidhu, S. S. (2013). Fast pyrolysis of microalgae remnants in a fluidized bed reactor for bio-oil and biochar production. Bioresour. Technol. 127, 494–499. doi: 10.1016/j.biortech.2012.08.016
Wang, L., Yuan, D., Li, Y., Ma, M., Hu, Q., and Gong, Y. (2016). Contaminating microzooplankton in outdoor microalgal mass culture systems: an ecological viewpoint. Algal Res. 20, 258–266. doi: 10.1016/j.algal.2016.10.013
Watanabe, F., Takenaka, S., Kittaka-Katsura, H., Ebara, S., and Miyamoto, E. (2002). Characterization and bioavailability of vitamin B12- compounds from edible algae. J. Nutr. Sci. Vitaminol. 48, 325–331. doi: 10.3177/jnsv.48.325
Watt, R. (2021). The fantasy of carbon offsetting. Env. Polit. 30, 1069–1088. doi: 10.1080/09644016.2021.1877063
Wells, M. L., Potin, P., Craigie, J. S., Raven, J. A., Merchant, S. S., Helliwell, K. E., et al. (2017). Algae as nutritional and functional food sources: revisiting our understanding. J. Appl. Phycol. 29, 949–982. doi: 10.1007/s10811-016-0974-5
Whitman, T., Nicholson, C. F., Torres, D., and Lehmann, J. (2010). Climate change impact of biochar cook stoves in Western Kenyan farm households: system dynamics model analysis. Environ. Sci. Technol. 45, 3687–3694. doi: 10.1021/es103301k
Wise, T. A. (2020). Failing Africa's Farmers: An Impact Assessment of the Alliance for a Green Revolution in Africa. Global Development and Environment Institute. Available online at: https://sites.tufts.edu/gdae/files/2020/10/20-01_Wise_FailureToYield.pdf
Wong, C. Y., Teoh, M. L., Phang, S. M., Lim, P. E., and Beardall, J. (2015). Interactive effects of temperature and UV radiation on photosynthesis of Chlorella strains from polar, temperate and tropical environments: differential impacts on damage and repair. PLoS ONE 10:e139469. doi: 10.1371/journal.pone.0139469
Wood, A. (2022). Tweet from Alaina Wood. Available online at: https://twitter.com/alainamwood/status/1520040921034805251 (accessed April 29, 2022).
Wuang, S. C., Khin, M. C., Chua, P. Q. D., and Luo, Y. D. (2016). Use of Spirulina biomass produced from treatment of aquaculture wastewater as agricultural fertilizers. Algal Res. 15, 59–64. doi: 10.1016/j.algal.2016.02.009
Xu, X., Sharma, P., Shu, S., Lin, T. S., Ciais, P., Tubiello, F. N., et al. (2021). Global greenhouse gas emissions from animal-based foods are twice those of plant-based foods. Nat Food 2, 724–732. doi: 10.1038/s43016-021-00358-x
Yu, K. L., Show, P. L., Ong, H. C., Ling, T. C., Chen, W. H., and Salleh, M. A. M. (2018). Biochar production from microalgae cultivation through pyrolysis as a sustainable carbon sequestration and biorefinery approach. Clean Technol. Environ. Policy 20, 2047–2055. doi: 10.1007/s10098-018-1521-7
Keywords: climate change, food supply, small-scale farmers, agri-food technologies, future foods, microalgae, climate resilience, climate change mitigation
Citation: Siedenburg J (2022) Could microalgae offer promising options for climate action via their agri-food applications? Front. Sustain. Food Syst. 6:976946. doi: 10.3389/fsufs.2022.976946
Received: 23 June 2022; Accepted: 20 July 2022;
Published: 15 August 2022.
Edited by:
Liming Ye, Ghent University, BelgiumReviewed by:
Mostafa Gouda, National Research Centre, EgyptSean Tibbetts, National Research Council Canada (NRC-CNRC), Canada
Copyright © 2022 Siedenburg. This is an open-access article distributed under the terms of the Creative Commons Attribution License (CC BY). The use, distribution or reproduction in other forums is permitted, provided the original author(s) and the copyright owner(s) are credited and that the original publication in this journal is cited, in accordance with accepted academic practice. No use, distribution or reproduction is permitted which does not comply with these terms.
*Correspondence: Jules Siedenburg, ai5zaWVkZW5idXJnJiN4MDAwNDA7dWVhLmFjLnVr