- 1Intercollege Graduate Degree Program in Ecology, The Pennsylvania State University, University Park, PA, United States
- 2Department of Ecosystem Science and Management, College of Agricultural Sciences, The Pennsylvania State University, University Park, PA, United States
- 3Department of Plant Science, The Pennsylvania State University, University Park, PA, United States
- 4USDA-ARS Pasture Systems and Watershed Management Research Unit, University Park, PA, United States
Maximizing living cover and minimizing soil disturbance with no-till are key strategies in regenerative row-crop production. Although living cover and no-till can increase beneficial soil carbon and water stable aggregates (WSA), annual crops in rotation with perennials often rely on herbicides to control weeds and terminate perennials. Integrated weed management (IWM) reduces reliance on herbicides by employing multiple weed control strategies including tillage and/or cultivation. However, many no-till growers are reluctant to implement some soil disturbance due to concerns about negative impacts on soil health. For that reason, we hypothesized that compared to continuous no-till and standard herbicides (NT-SH), a strategic inversion tillage in IWM (ST-IWM) would result in lower soil carbon and WSA in the year following the tillage event. We also hypothesized that soil carbon and WSA would not differ between the two systems when sampled after cover cropping and 2 years of perennials. We tested these hypotheses within a 6-year, diverse, dairy crop rotation initiated in 2010 in central Pennsylvania in a channery silt loam soil. The systems were compared in split-plots in a full crop entry experiment, where the six phases of the crop rotation were planted every year in a randomized complete block design, replicated four times. We compared the soil health indicators in spring 2010 prior to the start of the experiment and in 2013 and 2019 following inversion tillage (ST-IWM) or herbicide termination (NT-SH) of the perennial forage in the first year of the rotation. We also compared these indicators in the sixth year of the rotation after 3 years of annual and cover crops and 2 years of perennial forage. We sampled at two depths: 0–5 and 5–15 cm for total carbon and bulk density, 0–5 cm for labile carbon and 0–15 cm for WSA. Results indicate that despite initial smaller soil health values in the ST-IWM system following inversion tillage, all properties except labile carbon were similar to the NT-SH system in the sixth year of the rotation.
Introduction
Annual crop production that fulfills the regenerative agriculture goals of enhancing soil carbon without tillage but with continuous plant cover often relies on herbicides to terminate cover and perennial crops and to control weeds. No-till equipment places crop seeds into soil without plowing or disking, reducing soil disturbance and leaving previous crop residues on the surface, thus reducing soil erosion and maintaining soil structure (Jarecki and Lal, 2003; Baker et al., 2007). Besides saving fuel and protecting soil from erosion, no-till farming conserves soil carbon near the soil surface by slowing the decomposition of residues on or near the surface relative to soils that are mixed through tillage (Stubbs et al., 2004; Kan et al., 2021) and is therefore considered an important management tool for conserving soil and improving key components of soil health. When cover crops and perennial crops are integrated into no-till cropping systems, farmers typically apply herbicides to terminate the crops that provide continuous cover and control weeds. This reliance on herbicides for crop and weed termination can contribute to the evolution of herbicide resistant weeds (Quincke et al., 2007; Green and Siehl, 2021; Heap, 2022). Concerns that herbicide use can adversely affect humans (Sanborn et al., 2007; Zhang et al., 2019; Stradtman and Freeman, 2021), aquatic ecosystems (Hunt et al., 2017), wildlife (Freemark and Boutin, 1995), soil organisms (Gaupp-Berghausen et al., 2015) and soil health, present a conundrum for no-till farmers. No-till also increases nutrient stratification in the soil because it allows fertilizers, lime and residues from terminated crops to accumulate near the surface (Scheiner and Lavado, 1998). This stratification can be detrimental to crop production and the environment (Baker et al., 2017; Norton, 2020). These issues have led to the consideration of using strategic disturbance events, such as occasional inversion tillage and shallow cultivation to terminate perennials or cover crops, reducing the frequency and rate of herbicide applications and incorporating nutrients into the soil profile (Kettler et al., 2000; Dang et al., 2015; Summers et al., 2021).
In a 2017 survey by the USDA, 67% of crop acreage in Pennsylvania was managed with no-till and 24% with cover crops [National Agricultural Statistics Service (NASS) USDA, 2017]. Organizations such as the Pennsylvania No-Till Alliance have played an important role in advocating for these conservation practices and are often averse to using any type of tillage that may destroy the soil health benefits gained from continuous no-till (PA No-Till Alliance, 2022). Multiple studies have documented the negative effects of tillage on soil health, with soil health indictors tending to decline with increased tillage intensity (Jarecki and Lal, 2003; Bhardwaj et al., 2011; Cates et al., 2016; Nunes et al., 2020; Sprunger et al., 2021).
A nationwide meta-analysis compared the effects of moldboard plowing, chisel plowing, no-till, and perennial systems on soil organic carbon, permanganate oxidizable C (POXC or active C), soil respiration, microbial biomass C and N, soil protein, and beta-glucosidase activity (Nunes et al., 2020). The authors found that converting from moldboard to chisel plowing improved soil organic carbon, microbial biomass carbon and soil respiration in the first 0–15 cm of soil, however converting from moldboard plowing to no-till improved all seven soil health indicators at 0–15 cm. Additionally, compared to moldboard plowing, perennial systems had improved soil health indicators at all depths sampled (0–40 cm). The authors concluded that combining cover crops and minimizing crop residue removal along with no-till improved soil health indicators more than switching to no-till alone (Nunes et al., 2020).
The addition of cover crops, perennials and increased crop diversity in cropping systems also enhances soil health indicators, such as SOC and water stable aggregates (Angers and Caron, 1998; Salvo et al., 2014; Congreves et al., 2015; King and Blesh, 2017; Basche and DeLonge, 2019; Sprunger and Martin, 2020). In a Wisconsin study, particulate organic matter (POM) and aggregate C and N were the soil health indicators assessed across six cropping systems ranging from continuous maize with yearly chisel plowing to more diverse rotations with less frequent chisel plowing and perennial forages, to never-tilled perennial pasture (Cates et al., 2016). Although the authors' hypothesized that soil health would be reduced in proportion to tillage intensity they found that the systems that were tilled every year had POM and aggregate C levels similar to the crop rotation tilled every 3 years that included significant crop residues from corn stover and perennials (Cates et al., 2016). The integration of crop residues and perennials also diversifies weed control strategies and can reduce reliance on herbicides with diverse crop lifecycles that interrupt weed lifecycles and mechanical weed control via frequent harvests of perennial forages (Cavigelli et al., 2008; Davis et al., 2012; Summers et al., 2021).
One-time tillage, also referred to as strategic tillage, occasional tillage, and single inversion tillage, etc., is a potential alternative to continuous no-till; however, disagreement exists as to the efficacy of one-time tillage on otherwise no-till land. Some studies report little to no effect of one-time tillage on soil health (Salvo et al., 2014; Dang et al., 2015; Blanco-Canqui and Wortmann, 2020), whereas others report a persistent decrease in soil health following tillage (Wortmann et al., 2010; Stavi et al., 2011). For instance, one-time tillage was effective at reducing herbicide dependence and controlling downy brome (Bromus tectorum L.), an annual grassy weed, in a 20 year NT winter wheat (Triticum aestivum L.)-fallow system in Nebraska (Kettler et al., 2000). The authors compared a one-time tillage using a moldboard plow with secondary tillage (disking, chisel) and rod weeding to a no-till control treatment. Tillage reduced the downy brome densities and wheat yield increased; although 5 years after the tillage event SOC at 0–7.5 cm depth was still 20% less than in continuous no-till. However, in the 7.5–15 cm depth, SOC was 15% greater compared to the continuous no-till treatment, suggesting that carbon was redistributed through tillage rather than lost from the soil profile (Kettler et al., 2000). In grain-crop systems in Northeastern Australia, Dang et al. (2015) also evaluated strategic or occasional shallow tillage in 14 sites and found weed populations were reduced the year following tillage, without reducing soil TOC. Although bulk density and soil water availability decreased for the first 12 months at some sites, most appeared to recover after 24 months except in high clay soils (Dang et al., 2015).
By contrast, integrating perennials into an annual crop rotation with some tillage has been reported to maintain SOC that was similar to an annual crop rotation with no-till and some tillage (Cates et al., 2016) or 100% no-till management of the same crop rotation of perennials and annuals (Salvo et al., 2014). For instance, Salvo et al. (2014) found that at the end of 9 years of integrating a few years of perennial pasture into annual crop rotations with tillage maintained total SOC at levels similar to no-tillage with the same crop rotation. A review by Blanco-Canqui and Wortmann (2020) examined the impacts of occasional tillage on SOC and physical properties. Although results varied from study to study, they concluded that occasional tillage generally does not reduce overall SOC content but can affect its vertical distribution, with effects lasting up to 2 years following tillage. Additionally, occasional tillage was effective at reducing nutrient stratification and suppressing weed populations for several years. Blanco-Canqui and Ruis (2020) concluded that the benefits of occasional tillage depend on the type, timing, depth, and frequency of the tillage and that the ideal type of one-time tillage will vary. These findings explain the variability in the results of other studies but are encouraging for the use of one-time tillage when occasionally integrated. Though many studies include one-time tillage or cover crops and perennials as a factor, a research gap exists concerning the interaction between one-time tillage and continuous cover with cover and perennial crops (Osterholz et al., 2021).
We undertook this study because we wanted to assess the effects of strategic tillage on soil health indicators. Although farmer cooperators frequently point out that increased water infiltration is a major benefit of no-till, a recent meta-analysis of 89 field trial studies reported that no-till had no significant effect (5.7 ± 9.7%) on infiltration rates even with residue retention (Basche and DeLonge, 2019). Instead, the use of either perennials or cover crops across those studies was found to increase mean infiltration rates by 59.2 ± 20.9 and 34.8 ± 7.7%, respectively. Moreover, recognition is growing that no-till management by itself is not a consistently effective means to increase soil carbon, because soil carbon accrual can vary, due to differences in climate, soil texture, organic mineralization rates, and carbon saturation (Ogle et al., 2012; Powlson et al., 2014; Daryanto et al., 2020). Thus, farmers are more likely to recognize the utility of weed control provided by strategic tillage combined with the integration of perennials and cover crops that can be more effective for sequestering soil carbon than no-till alone (Mary et al., 2020).
In this study, we assessed the effects of one-time tillage in a 6-year annual and perennial crop rotation with continuous cover by measuring soils across crop rotation years for total organic carbon (TOC), permanganate oxidizable carbon (POXC), and water-stable soil aggregates (WSA). We chose POXC as an indicator of soil carbon dynamics because it is a good proxy for labile C (i.e., readily available to soil microorganisms) and has been reported to be more responsive to soil management than TOC (Culman et al., 2012; Hurisso et al., 2016). We also chose WSA as a sensitive indicator of changes in soil structure due to management (Haynes and Swift, 1990). To those ends, we hypothesized that (1) in the spring following an inversion tillage event, the three soil health indicators would be smaller compared to those for continuous no-till soils; and (2) indicator values would return to similar levels observed in no-till soils after returning to no-till with cover crops and 2 years of perennial crops in the sixth year of the rotation.
Materials and methods
The experiment was conducted as part of the Dairy Cropping Systems (DCS) project established in 2010 at the Pennsylvania State University Russell E. Larson Agronomy Research Farm near Pennsylvania Furnace, PA (40.72°N, −77.92°W). The project aimed to simulate a confinement 97-ha dairy farm at 1/20th the scale (4.86 ha) that could produce all forage and grain needed for a simulated 65-cow dairy herd while minimizing off-farm inputs and environmental impacts. We sampled soils from a 6-year crop rotation of annual and perennial crops comparing two weed control systems: (i) continuous no-till with standard herbicides (NT-SH) and (ii) strategic tillage and integrated weed management that reduced herbicide applications (ST-IWM). The crop sequence (Figure 1) consisted of: (1) winter canola (Brassica napus L.) or canola plus oats followed by a rye cover crop (2) soybean [Glycine max (L.) Merr.] followed by a rye (Secale cereale L.) cover crop (3) corn grain or corn silage (Zea mays L.) followed by (4–6) 3 years of perennial forage. The perennial forage in the ST-IWM system was alfalfa (Medicago sativa L.) and orchard grass (Dactylis glomerata L.) planted with a companion small grain (for species over the 9 years, see Summers et al., 2021) (Table 1), while the NT-ST system was alfalfa as the perennial crop until 2016 when orchardgrass was added so that the perennial forage systems would have the same species that were harvested for hay and silage. The practice of harvesting perennial forages grown in rotation with annual crops to feed a total mixed ration to cattle in confinement is typical for dairy farms of this size in Pennsylvania (Holly et al., 2019).

Figure 1. Six-year crop rotation sequence with continuous no-till and standard herbicides (NT-SH) and strategic tillage within integrated weed management (ST-IWM).
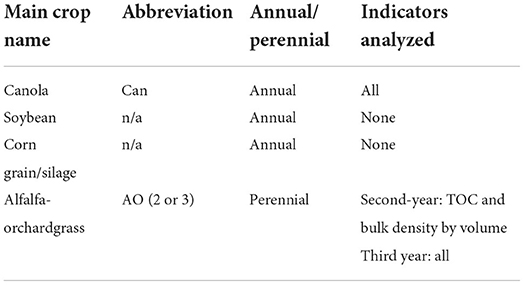
Table 1. The main crops in the rotation sequence, the abbreviation used within the paper, and which soil analyses was conducted in each crop.
Experimental design
Every crop phase of the 6-year rotation was present every year in randomized main plots (37 by 27 m) replicated four times, and the two weed control systems were split-plots (18 × 27 m) within each crop entry. A winter rye cover crop was present on the entire experiment when the experimental plots were established in spring 2010. Agronomic production details (ex. crop varieties, seeding rates, planting dates, herbicides, etc.) are described in Summers et al. (2021). Most relevant to this study, the NT-SH system received standard herbicide application rates to control weeds and terminate perennial crops without any tillage. To terminate the perennial alfalfa or alfalfa-orchardgrass in fall 2018 prior to planting winter canola, 0. 9 kg ae ha−1 of glyphosate (N-[phosphonomethyl]glycine), 0.5 kg ae ha−1 2–4-D LV4, and 0.3 kg ae ha−1 dicamba was applied in late August. Pre- and post-emergence herbicides were broadcast as part of the NT-SH system weed management strategy (see Summers et al., 2021).
By contrast, in ST-IWM prior to fall planting or winter canola, the perennial crops (alfalfa-orchardgrass) were terminated with a moldboard plow followed by secondary tillage (disk, a S-tine chisel and cultimulcher) in late August followed by planting winter canola. For the corn and soybean row crops, pre-emergent herbicide was banded over only corn and soybean rows, a high residue shallow-disk cultivator was used to control weeds between the row crops twice early in the season, instead of postemergence herbicide from 2010 to 2012 (Summers et al., 2021). From 2013 to 2018 the corn and soybean crop plots were split into two nested split-split (9 by 27 m) plots, one nested split-plot received the shallow-disk cultivation, the other nested split plot received a post-herbicide application instead of the high residue cultivation as described in Summers et al. (2021). Soil samples from both of these nested split-split plots were combined in 2019 for the ST-IWM. Herbicide reduction in the ST-IWM varied over the 9 years and by crop entries, with the exception of when the perennial forage was terminated with tillage and herbicide was eliminated or reduced 100%. For the other crops, burndown herbicide applications were the same in both systems, and the STM-IWM system herbicide reductions occurred in the pre-emergent and post-emergent herbicide applications and were calculated as kilograms per hectare of active ingredient. Compared to the NT-SH system, over the 9 years the STM-IWM herbicide reduction averaged 18% in soybean, 37% in corn and 37% in the establishment year of the perennials. Herbicides were not applied to either treatment in the second and third years of the perennial forages or when the winter canola was planted (Summers et al., 2021).
The predominant soil series at the experimental site is Murrill (Fine-loamy, mixed, semiactive, mesic Typic Hapludults). Manure management practices were chosen to reflect best on-farm practices. When soil was managed without tillage (NT-SH), manure was injected following perennial termination prior to planting winter canola, and before planting a rye cover crop (Figure 1). Manure was broadcast and incorporated by the tillage in the ST-IWM system prior to planting canola.
Soil sampling
Prior to any experimental field operations, soils were sampled in spring 2010 to establish baseline values. In spring 2013 and 2019, soils were sampled from plots containing winter canola (planted the previous fall) and the third year of the perennial crop. In the ST-IWM system, the canola plots followed the full fall tillage event, while the third year perennial plots were in the sixth year after tillage. In the NT-SH system, neither the canola nor perennial crops had received any tillage. In 2013, the NT-SH system had been in only alfalfa, while the ST-IWM had alfalfa and orchardgrass. In 2019, both the NT-SH and ST-IWM plots were planted in alfalfa and orchardgrass in 2016. For simplicity, we therefore refer to this crop as alfalfa + orchardgrass (AO3). In 2019, we also sampled soil from the second-year alfalfa orchardgrass (AO2) crop (5 years after tillage) from both the NT-SH and ST-IWM systems for TOC and bulk density measurements.
Ten to 15 soil cores were randomly collected between 4 and 20 of April 2010, on 9 and 10 of April 2013 and 19 March, 2019, when winter canola and perennials had begun greening-up after winter dormancy. Soil cores were split into 0–5 and 5–15 cm depths for separate composite samples. After being air dried and passed through a 2 mm sieve, these samples were measured for TOC and POXC.
Additional soil cores were sampled to 15 cm depth and composited on 20 and 21 May of 2010; on 17, 20 and 21 May, 2013; and on 3 June, 2019, when the canola and the perennials were flowering or beginning to flower. These samples were measured for WSA after storage in cool, airtight containers to minimize microbial activity.
Bulk density
Soil bulk density was sampled on 17 November, 2019, which also allowed the calculation of TOC on a volumetric basis in 2019. Ten to 15 bulk density samples were collected from canola, and the second and third years of alfalfa and orchardgrass (AO2 and AO3, respectively) using a tractor mounted Giddings soil probe (7.5 cm diameter). Following a modified bulk density procedure described by Blake and Hartge (1986), cores were collected in a thin plastic sheath, cut open in the laboratory, and soil was separated into 0–5 and 5–15 cm depths. Soils were air dried, weighed, and passed through a 2 mm sieve. Material collected on the sieve was weighed, washed, and volume determined by water displacement to allow correction of bulk density values for stone content.
Total organic carbon
TOC concentrations at 0–5 and 5–15 cm depths were measured by combustion with a 2,400 CHNS/O Series II Analyzer (Perkin Elmer, Waltham, MA, USA) in 2010 and 2013 and a Vario Max elemental analyzer (Elementar, Langenselbold, Hesse, Germany) in 2019.
Labile carbon (POXC) and water-stable aggregates
Samples (0–5 cm) were tested for POXC using the Weil et al. (2003) method. Briefly, 2.5 g of air-dried soils were mixed with 2 mL stock solution (0.2 M KMnO4 in 1 M CaCl2, pH 7.2) and brought to 20 mL volume with water. Cleared soil suspensions were diluted 1/10 before measuring absorbances at 550 nm in a colorimeter (Hach, Loveland, CO). Standard curves of absorbance values for known KMnO4 concentrations provided the y-intercept (a) and slope (b) in the following equation to calculate soil POXC concentration::
For water-stable aggregates, field moist soils sampled from 0 to 15 cm were sieved through 2 and 1-mm sieves, with material remaining on top of the 1-mm sieve retained and air-dried. A modified version (Grover, 2008) of the Kemper and Rosenau (1986) method was used with a dispersing solution (2 g of sodium hexametaphosphate in 1 L of deionized water). Four grams of air-dried soils were added to sieve-bottom cups in an 8-cup wet-sieving apparatus (Eijkelkamp Soil & Water Giesbeek, Netherlands). Tins containing deionized water were placed under the sieve-bottom cups, which were lowered to completely submerge the soils. After submersion without disturbance for 5 min, the cupholder was raised and lowered at a rate of 33 times per minute for another 5 min. Tins containing water were replaced with tins containing dispersing solution, and the process was repeated.
Once raised out of the solution, samples in the sieve-bottom cups were gently rubbed with a rubber-tipped rod for 20 s each. Samples were lowered again into dispersing solution and raised and lowered for a final 5 min. The two sets of tins, one containing water and unstable soil fraction and the other containing dispersing solution and the stable fraction, were removed and placed in a drying oven at 110°C for 2 days. Any sand, rock, or particulate organic matter remaining in the sieve bottom cup was discarded.
WSA percentage was measured using the equation:
where stable aggregate refers to the fraction of the soil slaked off in dispersing solution and unstable aggregate refers to the fraction of the soil that slaked off in water.
Two replicates were performed for each plot and a percent difference between replicates was determined using the equation:
If the percent difference between replicates was greater than 29%, a third replicate was performed. This was only necessary for one sample.
Statistical analysis
Because the WSA procedure was carried out by different individuals in 2010, 2013, and 2019, potential variation in individuals' techniques called for separate analyses of 2010, 2013 and 2019 results, and standardized scores were generated for water stable aggregate data within each year. Standardized scores were calculated by subtracting the population mean (all blocks and both systems) from each WSA percentage of each sampled plot and dividing this value by the population standard deviation.
Data were analyzed with PROC MIXED for a split-plot design in JMP Pro 15 by SAS (SAS Institute, Inc. Cary, North Carolina). For TOC, labile carbon, WSA, and standardized WSA, fixed effects were year, system, crop, and the two and three-way interactions between these terms with block, block × crop, and block × year as random effects, with fixed effects separated by the Personality Standard Least Squares test, equivalent to the Satterthwaite approximation. Bulk density and total organic carbon by volume was analyzed for 2019, which was the only year with sufficient bulk density data. Crop and system and the interaction between them were fixed effects and block and the interaction between block and crop were random. The SLICE test, which analyzes simple effects to separate LSmeans within an interaction, was used to test the pre-planned hypotheses, comparing the system within the same crop and year. Means were considered significantly different at p < 0.05. We also conducted the SLICE test when there was a significant interaction, with the exception of testing the Year effect. We only conducted the SLICE test to compare 2013 and 2019, the years when crops were planted in the same plots or soil and appropriate to compare between those years.
Results and discussion
Total organic carbon concentration
For TOC at the 0–5 cm depth, “system” showed the only significant effect (p = 0.00089), where ST-IWM averaged 14% lower TOC than the NT-SH average. Canola TOC concentration in ST-IWM was 21% less in 2013 (p = 0.0197) and 29% less in 2019 (p = 0.0013), compared to the NT-SH system (Table 2). Others have also reported a reduction in soil TOC in the upper soil layer following inversion tillage compared to no-till (Kettler et al., 2000; Jarecki and Lal, 2003; Mary et al., 2020).
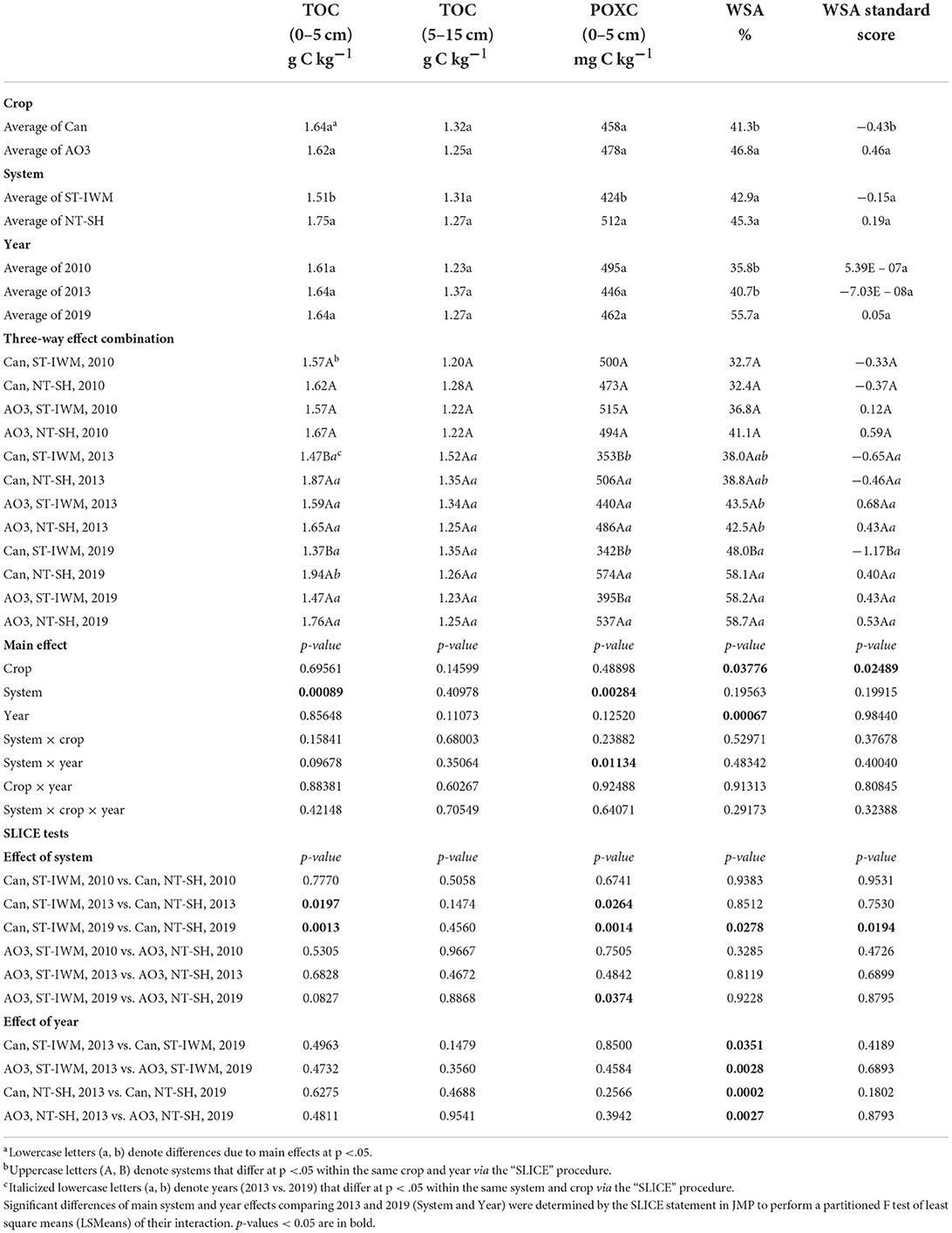
Table 2. Total organic carbon (g C kg soil−1) at 0–5cm and 5–15 cm, labile carbon by POXC (mg C kg soil−1) at 0–5 cm, water stable aggregates at 0–15 cm and water stable aggregate standard scores, calculated using the means and standard deviation of within-year score compared in 2010, 2013, and 2019.
By contrast, there were no significant differences between systems in the AO3 in any year (Table 2). In 2013 the ST-IWM AO3 had not yet been exposed to tillage since the start of the experiment, but in 2019 the ST-IWM AO3 had experienced inversion tillage 6 years earlier. The similar TOC concentrations indicated that levels in the ST-IWM system had recovered to those observed in the NT-SH system (Table 2). In Uruguay, Salvo et al. (2014) also found that when rotated to perennial pastures following tillage, SOC was not reduced relative to no-till. In addition, in 14 grain crop locations that were occasionally tilled in Australia, Dang et al. (2015) found that soil organic carbon in the top 0–10 cm did not differ from paired no-till systems three to 24 months after tillage.
For TOC concentration at 5–15 cm depth, none of the fixed effects were significant. And the hypothesis that the systems would differ after tillage in Canola was not true in any year (Table 2), indicating tillage did not have an effect at this depth. It is possible that some TOC was redistributed to a deeper depth than what was sampled in our study, as Kettler et al. (2000) noted a redistribution of carbon to deeper depths following tillage. Because the plow depth is closer to 30 cm, increased TOC from buried residue could be buried in the 15–30 cm layer. Due to channery soils, sampling past 15 cm was not feasible on a wide scale and this possibility was not investigated. The potential for redistribution of a portion of the carbon, rather than loss of carbon due to tillage, would support the idea that occasional tillage does not reverse the benefits of no-till.
Bulk density and calculation of total organic C by volume
In the 2019 bulk density analysis, only crop showed a significant effect in the 0–5 cm depth (p = 0.00418), where AO3 had 9% lower average bulk density than the canola and AO2 was not significantly different from either of the other crops (Table 3). Overall, bulk density values at both depths were lower than 1.55 g cm−3, which is the bulk density considered to be root- restrictive for silt loam soils (Kaufmann et al., 2010). Nevertheless, lowered bulk densities are an indication of higher organic matter content, greater porosity, and improved soil hydraulic function (Kaufmann et al., 2010; Bagnal et al., 2022). The lower bulk density after 2 years of perennial forage, regardless of tillage, thus suggested that soils had better structure in support of root growth. As with the TOC concentration results, there were no significant effects observed in the 5–15 cm depth for bulk density (Table 3).
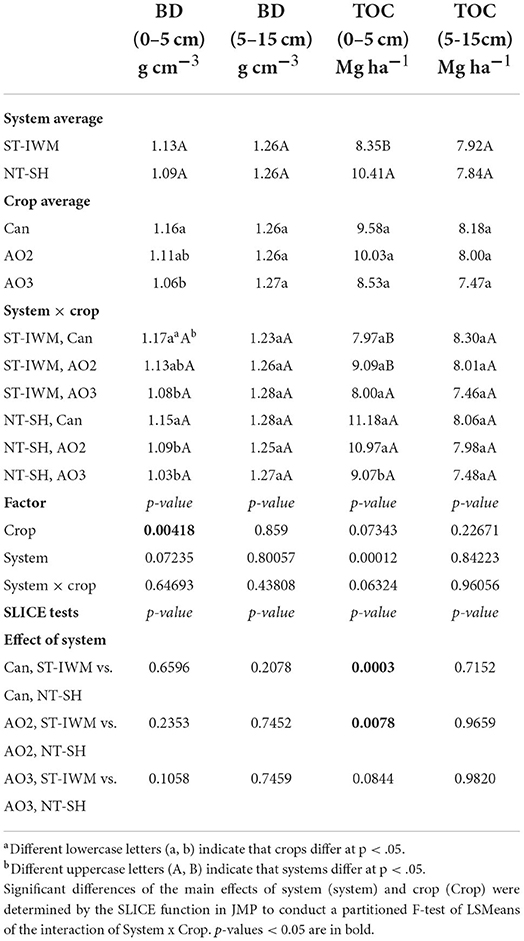
Table 3. Bulk density and TOC by volume averages at 0–5 and 5–15 cm depths for strategic tillage within integrated weed management (ST-IWM) and continuous no-till with standard herbicide (NT-SH) in canola (Can), second-year alfalfa orchardgrass (AO2) and third-year alfalfa orchardgrass (AO3) crops sampled in 2019.
Decreased bulk density following perennial crops was expected as the perennial roots are likely to add organic matter to the soil, as well as improving fungal hyphae and biological activity that promotes soil porosity, making the soil less dense (Angers and Caron, 1998; Blanco-Canqui and Ruis, 2020) and reducing the potential for compaction. This result supports our hypothesis that the lack of disturbance during perennial growth would lead to improvement in soil health indicators. Interestingly, the bulk density did not increase significantly following tillage as there was no difference between the two systems in the canola crop. This did not support our first hypothesis that strategic tillage would cause an initial decrease in soil quality. Tillage has been shown to decrease bulk density in the short term but increase it in the long-term (Logsdon and Karlen, 2004; Dang et al., 2015). However, we did not detect a difference in systems or following tillage in the canola.
The TOC by volume (Mg/ha) showed similar trends to the TOC concentration in both sampled depths. System had a significant effect in the 0–5 cm depth (p = 0.00012), with TOC by volume 20% smaller in ST-IWM compared to the NT-SH. The canola had 29% less (p = 0.0003) and AO2 had 17% less TOC by volume (p = 0.0078) in ST-IWM compared to NT-SH (Table 3). There were no significant differences however, between the two systems in AO3 (Table 3), indicating that following strategic tillage, 2 years of the perennial forage were required for TOC to increase to the same level as the no-till system. At the 5–15 cm depth, there were no significant differences in TOC by volume between systems or crops (Table 3). In summary, TOC by volume decreased significantly following tillage, but was similar between systems following 3 years of annuals and cover crops and 2 years of perennials.
Labile carbon
In the analysis of POXC, system showed a significant effect (p = 0.00079), where ST-IWM averaged 17% lower than NT-SH. Compared to NT-SH, canola POXC in ST-IWM was 30% less in 2013 (p = 0.0264) and 40% less in 2019 (p = 0.0014, Table 2). However in 2019, POXC in AO3 of the ST-IWM system was 26% less than in the NT-SH system (p = 0.0374), indicating that POXC had not increased to similar levels in ST-IWM 6 years after tillage. In 2013, AO3 in the ST-IWM system hadn't yet experienced tillage, while in 2019, the AO3 in that system had been tilled 6 years before. Quincke et al. (2007) also noted a significant decrease in labile carbon following tillage in the top few centimeters of soil, but found increased labile carbon in deeper layers that essentially offset the carbon lost from the more superficial layer, suggesting that labile carbon had been redistributed by moldboard plow rather than lost. In our study, POXC was only analyzed at the 0–5 cm depth, and it may have been possible that a similar redistribution of carbon occurred at deeper soil depth. Kettler et al. (2000) and Mary et al. (2020) also noted a redistribution of carbon to deeper depths following tillage.
Water stable aggregates
In the analysis of the WSA and standardized WSA scores, only year showed a significant effect on WSA (p = 0.00067) while crop had significant effects on both scores (p = 0.03776 and p = 0.02489, respectively, Table 2). Compared to 2010, the average WSA was 37% smaller than the averages for 2013 and 2019. The larger average WSA values in 2013 and 2019 may be attributed to the combination of cover crops, perennials and lack of disturbance in most years of both systems. The role of different people conducting the WSA analysis also likely explains some of the WSA differences among years. Year-to-year variation was why we used a standardization procedure for raw WSA scores. Compared to the canola, WSA and the standardized score in AO3 averaged 11.8% greater and 1.9 fold greater, respectively (Table 2). Others have also reported that perennial roots and their associated fungal hyphae and soil microorganisms stabilized soil aggregates regardless of tillage (Angers and Caron, 1998).
Following tillage in 2019, WSA of canola in the ST-IWM system was 17% smaller than in NT-SH (p = 0.0278), and the standardized WSA score was also significantly smaller in the ST-IWM system (p = 0.0194). However, WSA scores for canola in 2013 did not differ between the two systems when the NT-SH system had not included orchardgrass in the perennial forage prior to herbicide termination. In 2019, by contrast, AO3 in the systems did not differ for either WSA or the standardized scores, indicating that less disturbance with cover crops and 2 years of perennial alfalfa and orchardgrass significantly enhanced the physical soil stability in the ST-IWM. The lack of significant difference following tillage in the 2013 canola, however, may also be explained by the presence of orchardgrass in addition to alfalfa in the ST-IWM system when the NT-SH was planted to only alfalfa. The presence of orchardgrass in the ST-IWM system may have promoted and protected WSA enough to counter the tillage impact. Because perennial grasses have more fibrous roots, it has been suggested that they promote greater aggregate stability than other types of perennials (Miller and Jastrow, 1990; Angers and Caron, 1998; Rachman et al., 2003). In 2019, the NT-SH system also had orchardgrass planted with alfalfa, and the benefits of both no-till and perennial grass roots may have assisted in the formation of stable aggregates.
Other studies that compared occasional tillage to continuous no-till have also reported that occasional tillage often did not reduce soil aggregation, or that it recovered within a year with return to no-till (Dang et al., 2015; Blanco-Canqui and Wortmann, 2020). A 2010 study by Wortmann et al. found no significant differences in water stable aggregates across several tillage treatments, including no-till and moldboard plow tillage, 5 years following tillage. It is possible that ST-IWM might have returned to levels similar to NT-SH after several years without the aid of perennial roots. However, Dougherty et al. (2022) have suggested that only slight differences in soil health indicators can be expected within short timeframes, which may explain the lack of significant differences in the Wortmann et al. (2010) study. Additionally, the Wortmann et al. (2010) study used the water stable aggregate sampling method by Cambardella and Elliott (1994), which involves wet sieving soil and combining weights of three aggregate size classes, which could have accounted for different sensitivities.
Conclusion
Herbicides are typically used to terminate cover and perennial crops and control weeds during no-till management of annual and perennial crop rotations. Reliance on herbicides can lead to herbicide-resistant weeds and negative environmental impacts that conflict with the goals of regenerative agriculture. Integrated weed management employs multiple weed control practices but the use of occasional tillage is often dismissed in minimal tillage systems because of its association with reduced soil health. In this study, we found that most of the negative effects of strategic tillage on soil health indicators (soil carbon at 0–5 cm, water stable aggregates and bulk density) were mitigated in no-till annual and perennial cropping systems after 3 years' growth of annuals and cover crops and 2 years of perennial forages. We also found evidence that integrating perennial forage grasses with legumes is beneficial for promoting water stable aggregates. When compared to the continuous NT system, labile carbon was the only soil indicator that was lower in the ST-IWM system when measured in the third year of alfalfa-orchardgrass (AO3). Further research on the significance of labile carbon for total soil carbon accumulation and the long-term impacts of strategic tillage in these systems would elucidate the implications of this small reduction in labile carbon. Additionally, this study found no effects of the strategic tillage on soil carbon and bulk density at the 5–15 cm depth, implying that tillage did not impact soil health below the 0–5 cm range in this study, although an investigation of soil carbon at lower depths would be worth pursuing.
Data availability statement
The raw data supporting the conclusions of this article will be made available by the authors, without undue reservation.
Author contributions
DM, HK, and MB contributed to the conception and design of the study. CD advised on data collection. DM generated and organized data. DM and HK performed statistical analysis. DM wrote the first draft of the manuscript. All authors contributed to manuscript revision, read, and approved the submitted version.
Funding
This work was supported by National Institute of Food and Agriculture, Grant/Award Numbers: LNE09-291, LNE13-329, and LNE16-354, Hatch Appropriations and Grant/Award Numbers: 1009362, PEN04600, PEN04425, PEN04764, and Accession #1025969, The Pennsylvania State University College of Agriculture Sciences, LTAR ARS USDA 58-8070-8-008, USDANIFA Grant 1009145, and USDA Hatch Project PEN04710.
Acknowledgments
We thank Tom Adams, Kaleb Wolfe, Glenna Malcolm, Emad Jahanzad, and members of the Northeast Sustainable Agriculture Research and Education (NESARE) Dairy Cropping Systems research team who helped with field operations, soil sampling and analyses over the years. We also thank Kyle Elkin who conducted soil carbon analyses and the PSU Agronomy Farm research team.
Conflict of interest
The authors declare that the research was conducted in the absence of any commercial or financial relationships that could be construed as a potential conflict of interest.
Publisher's note
All claims expressed in this article are solely those of the authors and do not necessarily represent those of their affiliated organizations, or those of the publisher, the editors and the reviewers. Any product that may be evaluated in this article, or claim that may be made by its manufacturer, is not guaranteed or endorsed by the publisher.
References
Angers, D. A., and Caron, J. (1998). Plant-induced changes in soil structure: processes and feedbacks. Biogeochemistry 42, 55–72. doi: 10.1007/978-94-017-2691-7_3
Bagnal, D.K., Morgan, C.L.S., Bean, G.M., Liptzin, D., Cappellazzi, S.B., et al. (2022). Selecting soil hydraulic properties as indicators of soil health: measurement responses to management and site characteristics. Soil Sci. Soc. Am. J. 86, 1206–1226. doi: 10.1002/saj2.20428
Baker, C. J., Justice, S., Saxton, K., Hobbs, P., Ritchie, W., Charmen, W., et al. (2007). No-Tillage Seeding in Conservation Agriculture, 2nd ed. CABI Publishing, FAO. Available online at: http://www.fao.org/docrep/012/Al298e/al298e.pdf doi: 10.1079/9781845931162.0000
Baker, D. B., Johnson, L. T., Confesor, R. B., and Crumrine, J. P. (2017). Vertical stratification of soil phosphorus as a concern for dissolved phosphorus runoff in the Lake Erie Basin. J. Environ. Qual. 46, 1287–1295 doi: 10.2134/jeq2016.09.0337
Basche, A. D., and DeLonge, M. S. (2019). Comparing infiltration rates in soils managed with conventional and alternative farming methods: A meta-analysis. PLoS ONE. 14, e0215702. doi: 10.1371/journal.pone.0215702
Bhardwaj, A. K., Jasrotia, P., Hamilton, S. K., and Robertson, G. P. (2011). Ecological management of intensively cropped agro-ecosystems improves soil quality with sustained productivity. Agric. Ecosys. Environ. 140, 419–429. doi: 10.1016/j.agee.2011.01.005
Blake, G. R., and Hartge, K. H. (1986) “Bulk density,” in Methods of Soil Analysis, Part 1—Physical Mineralogical Methods, 2nd Edition ed. Klute, A. Agronomy Monograph 9 American Society of Agronomy—Soil Science Society of America, Madison, 363–382. doi: 10.2136/sssabookser5.1.2ed.c13
Blanco-Canqui, H., and Ruis, S. J. (2020). Cover crop impacts on soil physical properties: a review. Soil Sci. Soc. Am. J. 84, 1527–1576. doi: 10.1002/saj2.20129
Blanco-Canqui, H., and Wortmann, C. S. (2020). Does occasional tillage undo the ecosystem services gained with no-till? A review. Soil Tillage Res., 198:104534. doi: 10.1016/j.still.2019.104534
Cambardella, C. A., and Elliott, E. T. (1994). Carbon and nitrogen dynamics of soil organic matter fractions from cultivated grassland soils. Soil Sci. Soc. Am. J. 58:123–130. doi: 10.2136/sssaj1994.03615995005800010017x
Cates, A. M., Ruark, M. D., Hedtcke, J. L., and Posner, J. L. (2016). Long-term tillage, rotation and perennialization effects on particulate and aggregate soil organic matter. Soil Tillage Res. 155, 371–380. doi: 10.1016/j.still.2015.09.008
Cavigelli, M. A., Teasdale, J. R., and Conklin, A. E. (2008). Long-term agronomic performance of organic and conventional field crops in the mid-atlantic region. Agron. J. 100, 785–94. doi: 10.2134/agronj2006.0373
Congreves, K. A., Hayes, K., Verhallen, E. A., and Van Eerd, L. L. (2015). Long-term impact of tillage and crop rotation on soil health at four temperate agroecosystems. Soil Tillage Res., 152, 17–28. doi: 10.1016/j.still.2015.03.012
Culman, S. W., Snapp, S. S., Freeman, M. A., Schipandki, M. E., Beniston, J., Lal, R., et al. (2012). Permanganate oxidizable carbon reflects a processed soil fraction that is sensitive to management. Soil Sci. Soc. Am. J. 76, 494–504. doi: 10.2136/sssaj2011.0286
Dang, Y. P., Moody, P. W, Bell, M. J., Seymour, N. P., Dalal, R. C., and Freebairn, D. M. (2015). Strategic tillage in no-till farming systems in Australia's northern grains-growing regions: II. Implications for Agronomy, Soil and Environment. Soil and Tillage Research. Elsevier.152, 115–123 doi: 10.1016/j.still.2014.12.013
Daryanto, S., Wang, L., and Jacinthe, P.A. (2020). No-till is challenged: complementary management is crucial to improve its environmental benefits under a changing climate. Geogr. Sustain., 1:229–232. doi: 10.1016/j.geosus.2020.09.003
Davis, A. S., Hill, J. D., Chase, C. A., Johanns, A. M., and Liebman, M. (2012). Increasing cropping system diversity balances productivity, profitability and environmental health. PLOS ONE, 7: 1–13. doi: 10.1371/journal.pone.0047149
Dougherty, B. W., Andersen, D. S., and Helmers, M. J. (2022). Evaluating the impact of midwestern cropping systems on soil health and soil carbon dynamics. J. Soil Water Conserv. 77, 78–87. doi: 10.2489/jswc.2022.00056
Freemark, K., and Boutin, C. (1995). Impacts of agricultural herbicide use on terrestrial wildlife in temperate landscapes: a review with special reference to North America. Agric. Ecosys. Environ., 52, 7–91. doi: 10.1016/0167-8809(94)00534-L
Gaupp-Berghausen, M., Hofer, M., Rewald, B., and Zaller, J. G. (2015). Glyphosate-Based herbicides reduce the activity and reproduction of earthworms and lead to increased soil nutrient concentrations. Sci. Rep., 5, 12886. doi: 10.1038/srep12886
Green, J. M., and Siehl, D. L. (2021). History and outlook for glyphosate-resistant crops. Reviews of Environmental Contamination and Toxicology Volume 255:67–91. Cham: Springer International Publishing. doi: 10.1007/398_2020_54
Grover, K. K. (2008). Long-Term Cropping Systems Effects on Soil Aggregate Stability, Corn Grain Yields, and Yield Stability. Ph. D. diss. Dep. of Crop and Soil Sci. The Pennsylvania State Univ. University Park.
Haynes, R. J., and Swift, R. S. (1990). Stability of soil aggregates in relation to organic constituents and soil water content. J. Soil Sci. 41, 73–83. doi: 10.1111/j.1365-2389.1990.tb00046.x
Heap, I. (2022). The International survey of herbicide resistant weeds. Online. Internet. Available online at: www.weedscience.org (accessed July 26, 2022).
Holly, M. A., Gunn, K. M., Rotz, C. A., and Kleinman, P. J. A. (2019). Management characteristics of Pennsylvania dairy farms. Appl. Anim. Sci. 35, 325–338. doi: 10.15232/aas.2018-01833
Hunt, N. D., Hill, J. D., and Liebman, M. (2017). Reducing freshwater toxicity while maintaining weed control, profits, and productivity: effects of increased crop rotation diversity and reduced herbicide usage. Environ. Sci. Technol., 51, 1707–17. doi: 10.1021/acs.est.6b04086
Hurisso, T. T., Culman, S. W., Horwath, W. R., Wade, J., Cass, D., Beniston, J. W., Bowles, T. M., Grandy, A. S., Franzluebbbers, A. J, Schipanski, M. E., Lucas, S. T., and Ugarte, C. M. (2016). Comparison of permanganate-oxidizable carbon and mineralizable carbon for assessment of organic matter stabilization and mineralization. Soil Sci. Soc. Am. J. 80, 1352–1364. doi: 10.2136/sssaj2016.04.0106
Jarecki, M. K., and Lal, R. (2003). Crop management for soil carbon sequestration. Crit. Rev. Plant Sci., 22, 471–502. doi: 10.1080/713608318
Kan, Z. R., Liu, Q. Y., Virk, A. L., He, C., Qi, J. Y., Dang, Y. P., et al. (2021). Effects of experiment duration on carbon mineralization and accumulation under no-till. Soil Tillage Res., 209, 104939. doi: 10.1016/j.still.2021.104939
Kaufmann, M., Tobias, S., and Schulin, R. (2010). Comparison of critical limits for crop plant growth based on different indicators for the state of soil compaction. J. Plant Nutr. Soil. 173,573–583. doi: 10.1002/jpln.200900129
Kemper, W., and Rosenau, R (1986). “Aggregate stability and size distribution”, in, Methods of Soil Analysis, Part 1. Physical and Mineralogical Methods, Agronomy Monograph, American Society of Agronomy and Soil Science Society of America, Madison, Klute, A., ed. 425–442. doi: 10.2136/sssabookser5.1.2ed.c17
Kettler, T. A., Lyon, D. J., Doran, J. W., Powers, W. L., and Stroup, W. W. (2000). Soil quality assessment after weed-control tillage in a no-till wheat-fallow cropping system. Soil Sci. Soc. Am. J. 64, 339–346. doi: 10.2136/sssaj2000.641339x
King, A. E., and Blesh, J. (2017). Crop rotations for increased soil carbon: perenniality as a guiding principle. Ecol. Appl. 28, 249–261. doi: 10.1002/eap.1648
Logsdon, S. D., and Karlen, D. L. (2004). Bulk density as a soil quality indicator during conversion to no-tillage. Soil Tillage Res. 78, 143–149. doi: 10.1016/j.still.2004.02.003
Mary, B., Clivot, H., Blaszczyk, N., Labreuche, J., and Ferchaud, F. (2020). Soil carbon storage and mineralization rates are affected by carbon inputs rather than physical disturbance: evidence from a 47-year tillage experiment. Agric. Ecosyst. Environ., 299, 106972. doi: 10.1016/j.agee.2020.106972
Miller, R. M., and Jastrow, J. D. (1990). Hierarchy of root and mycorrhizal fungal interactions with soil aggregation. Soil Biol. Biochem., 22, 579–584. doi: 10.1016/0038-0717(90)90001-G
National Agricultural Statistics Service (NASS) USDA (2017). Census of Agriculture, Pennsylvania. Chpt. 1 Table 47. Available online at: https://www.nass.usda.gov/Publications/AgCensus/2017/Full_Report/Volume_1,_Chapter_1_State_Level/Pennsylvania/st42_1_0047_0047.pdf
Norton, R. M. (2020). “Challenges and opportunities in fertilizer placement in no-till farming systems”, in, No-till Farming Systems for Sustainable Agriculture, Dang, Y., Dalal, R., Menzies, N. (eds.). Springer, Cham. doi: 10.1007/978-3-030-46409-7_5
Nunes, M. R., Karlen, D. L., Veum, K. S., Moorman, T. B., and Cambardella, C. A. (2020). Biological soil health indicators respond to tillage intensity: a US meta-analysis. Geoderma, 369:114335. doi: 10.1016/j.geoderma.2020.114335
Ogle, S. M., Swan, A., and Paustian, K. (2012). No-till management impacts on crop productivity, carbon input and soil carbon sequestration. Agric. Ecosys. Environ. 149, 37–49. doi: 10.1016/j.agee.2011.12.010
Osterholz, W. R., Culman, S. W., Herms, C., Joaquim De Oliveira, F., Robinson, A., Doohan, D., et al. (2021). Knowledge gaps in organic research: understanding interactions of cover crops and tillage for weed control and soil health. Org. Agr, 11, 13–25. doi: 10.1007/s13165-020-00313-3
PA No-Till Alliance. 4 September. (2022). https://panotill.org/about/
Powlson, D. S., Stirling, C. M., Jat, M. L., Gerard, B. G., Palm, C. A., Sanchez, P. A., et al. (2014). Limited potential of no-till agriculture for climate change mitigation. Nat. Clim. Chang., 4, 678–683. doi: 10.1038/nclimate2292
Quincke, J. A., Wortmann, C. S., Mamo, M., Franti, T. G., Drijber, R. A., and Garcia, J. (2007). One-time tillage of no-till systems: soil physical properties, phosphorus runoff, and crop yield. Agron. J., 99, 1104–1110. doi: 10.2134/agronj2006.0321
Rachman, A., Anderson, S. H., Gantzer, C. J., and Thompson, A. L. (2003). Influence of Long-term Cropping Systems on Soil Physical Properties Related to Soil Erodibility. Soil Sci. Soc. Am. J., 67: 637–644. doi: 10.2136/sssaj2003.6370
Salvo, L., Hernandez, J., and Ernst, O. (2014). Soil organic carbon dynamics under different tillage systems in rotations with perennial pastures. Soil Tillage Res., 135, 41–48. doi: 10.1016/j.still.2013.08.014
Sanborn, M., Kerr, K. J., Sanin, L. H., Cole, D. C., Bassil, K. L., and Vakil, C. (2007). Non-cancer health effects of pesticides: systematic review and implications for family doctors. Can. Fam. Physician. 53, 1712–1720.
Scheiner, J. D., and Lavado, R. S. (1998). The role of fertilization on phosphorus stratification in no-till soils. Commun. Soil Sci. Plant Anal. 29, 2705–2711.
Sprunger, C. D., Culman, S. W., Deiss, L., Brock, C., and Jackson-Smith, D. (2021). Which management practices influence soil health in midwest organic corn systems? Agron. J. 113, 4201–4219. doi: 10.1002/agj2.20786
Sprunger, C. D., Martin, T., and Mann, M. (2020). Systems with greater perenniality and crop diversity enhance soil biological health. Agric. Environ. Lett., 5, 1. doi: 10.1002/ael2.20030
Stavi, I., Lal, R., and Owens, L. B. (2011). On-farm effects of no-till vs. occasional tillage on soil quality and crop yields in eastern Ohio. Agron. Sustain. Develop., 31, 475–482. doi: 10.1007/s13593-011-0006-4
Stradtman, S. C., and Freeman, J. L. (2021). Mechanisms of neurotoxicity associated with exposure to the herbicide atrazine. Toxics, 9, 207. doi: 10.3390/toxics9090207
Stubbs, T. L., Kennedy, A. C., and Schillinger, W. F. (2004). Soil ecosystem changes during the transition to no-till cropping. J. Crop. Improv. 11, 105–135. doi: 10.1300/J411v11n01_06
Summers, H., Karsten, H. D., Curran, W., and Malcolm, G. M. (2021). Integrated weed management with reduced herbicides in a no-till dairy rotation. Agron. J., 113, 3418–3433. doi: 10.1002/agj2.20757
Weil, R. R., Islam, K. R., Stine, M. A., Gruver, J. B., and Samson-Liebig, S. E. (2003). Estimating active carbon for soil quality assessment: a simplified method for laboratory and field use. Am. J. Altern. Agric. 18:3–17. doi: 10.1079/AJAA2003003
Wortmann, C. S., Drijber, R. A., and Franti, T. G. (2010). One-time tillage of no-till crop land five years post-tillage. Agron. J. 102, 1302–1307. doi: 10.2134/agronj2010.0051
Keywords: tillage, no-till, soil health, perennial, alfalfa, soil aggregate, soil carbon, bulk density
Citation: McPheeters D, Bruns MA, Karsten HD and Dell CJ (2022) Integrated weed management with strategic tillage can maintain soil quality in continuous living cover systems. Front. Sustain. Food Syst. 6:907590. doi: 10.3389/fsufs.2022.907590
Received: 19 July 2022; Accepted: 17 October 2022;
Published: 02 November 2022.
Edited by:
Jose G. Franco, Agricultural Research Service (USDA), United StatesReviewed by:
Patrick Hatfield, Retired, Bozeman, MT, United StatesEmerson Nafziger, University of Illinois at Urbana-Champaign, United States
Copyright © 2022 McPheeters, Bruns, Karsten and Dell. This is an open-access article distributed under the terms of the Creative Commons Attribution License (CC BY). The use, distribution or reproduction in other forums is permitted, provided the original author(s) and the copyright owner(s) are credited and that the original publication in this journal is cited, in accordance with accepted academic practice. No use, distribution or reproduction is permitted which does not comply with these terms.
*Correspondence: Heather D. Karsten, aGRrMyYjeDAwMDQwO3BzdS5lZHU=