- 1School of International Development, University of East Anglia, Norwich, United Kingdom
- 2WWF International, Gland, Switzerland
This article seeks greater clarity in the connections between water and regenerative agriculture (RA). We first review existing soil/water knowledge and argue that desired “RA and water” outcomes depend on the management and optimal levels of two key soil properties; readily available moisture and infiltration rate. Secondly, we hypothesize these help define a range of RA beneficial outcomes or “promises,” such as improved vegetative growth, reduced risk of erosive runoff, higher soil organic matter content and biological fertility, easier irrigation management, resilience to drought, better water filtration and less variable streamflow hydrology. Thirdly we show that by not fully understanding water's multiple roles for achieving desired RA outcomes, risks, contradictions and “pitfalls” can arise. With these three parts we observe that carefully explained and quantified roles of water in the existing RA literature are often missing, neglected, over-generalized or poorly explained. We also suggest that narratives and terms (e.g., “net zero” or “net positive”) for describing the benefits and aims of RA and water can lack context and situational fit, or are misleading and incorrect. Cautions also apply to water quantities additionally required for soil regeneration activities, recognizing stiff competition for scarce river basin water and its allocation. How necessary quantities overlap with local and catchment policies for water allocations and hydrology, incorporating farmers' views and choices and informed by field, farm and system water accounting, are critical to successful agricultural practices including those that are “regenerative.” Accordingly, we suggest that scientists and decisions-makers should more thoroughly interrogate how “RA and water” is being analyzed so that relevant policies develop its promises whilst avoiding pitfalls.
Introduction
Regenerative agriculture (RA) is receiving significant and growing attention from companies, food producers, academics, NGO's and consumers, as well as politicians and the mainstream media (Giller et al., 2021). Google Trends over the past 5 years shows a substantive increase in a phrase search for RA (Newton et al., 2020). RA is a term with multiple meanings, focus areas and definitions, and similarly across the RA literature, water's roles and functions are similarly diverse (Robinson et al., 2019). With a focus on soil health and carbon sequestration (Schreefel et al., 2020), it is possible to outline water's role as a set of idealized RA benefits depicted by Figure 1. As RA is currently framed and if its objectives are realized, then RA will deliver healthier soils that generate or are involved in a range of RA and water “promises.” At the farm scale, regenerated soils will absorb and retain more water in the soil profile which allows crops to productively transpire longer without rainfall or irrigation. At the catchment scale, via higher soil infiltration rates, RA soils will ameliorate rainfall-runoff hydrology, reduce runoff peak flows and erosion, and allow more water to pass through to groundwater. This in turn increases base flows of better–filtered water and helps to leach salts out of the soil profile. RA does not only create these benefits; RA depends on managing water, irrigation, rainfall and soil–water. Water supports regenerative processes to improve soil physical fertility, for example; by boosting biomass accumulation via higher plant and root growth; by sustaining soil biological activity; and by acting within desirable limits to dry then moisten soils to aid nutrient release and soil structure formation via shrinking and swelling (Bullock et al., 1985).
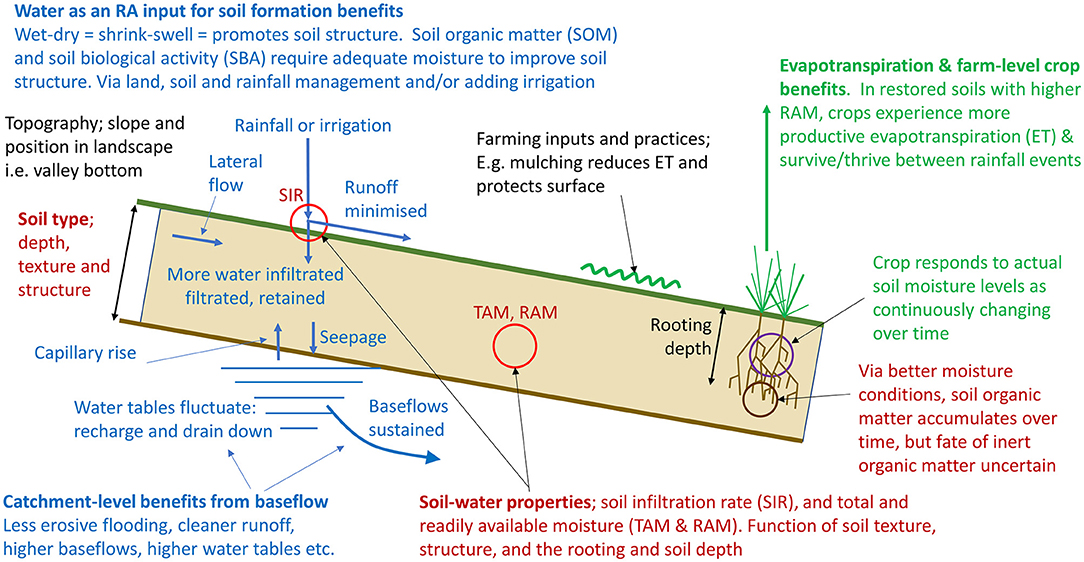
Figure 1. Benefits for regenerative agriculture arising from the soil-water properties of readily available moisture and infiltration.
Given these idealized multiple benefits—what potential problems are there with water's role within RA? By examining published papers on RA, we find two main problems. First, they do not fully explain or quantify the multiple interconnections between water and soils when articulating the benefits and promises of RA (Lal, 2020; Newton et al., 2020; Schreefel et al., 2020; Dent and Boincean, 2021; McLennon et al., 2021). Studies either omit soil-water interactions and water inputs entirely, or mainly address water quality issues or point to political-ecology connections between agriculture and water (Allan and Dent, 2021).
Similarly, we believe the literature often fails to see the “pitfalls” of RA and water. In these situations, scientists run the risk of idealizing or over-generalizing these beneficial connections, or of building potentially flawed narratives (for example RA is “net water positive”) that are insufficiently hydrologically explained. [By “narratives” we mean ideas that act as compelling explanatory beliefs and imply policy instructions (Molle, 2008)]. This is partly driven by the temptation to borrow narratives from carbon emissions accounting (Bistline, 2021) or from closed urban water systems (Crosson, 2018) which when applied to agricultural and catchment water are incomplete and misleading. Likewise, secondary effects or paradoxical outcomes are not fully acknowledged. For example the suggestion that RA soils and cropping use less water (Lal, 2020) is contradicted by other literature that questions efficiency gains (Grafton et al., 2018; Lankford et al., 2020). In short, pitfalls will occur if RA policies are drawn up by referring to idealized narratives rather than being designed to fit specific contexts, cases and factors.
We define “RA and water” as the broad subject area of multiple interconnections covering soil-water, soil physical fertility, catchment hydrological processes such as rainfall and evapotranspiration, and artificial inputs in the form of irrigation. This paper is mainly concerned with water quantity and its fluctuations rather than the water quality dimensions of RA, and is focused on cropped agriculture rather than livestock and animals. Our main geographical focus is on semi-arid, tropical and sub-tropical farming systems facing generally more seasonally variable and higher rainfall deficits, higher soil organic turnover rates and less chemically-active soil mineralogies (Six et al., 2002) than those in temperate climates. We also quantitatively examine RA and water using procedures from irrigation design and planning; expressing rainfall, evapotranspiration and soil moisture capacity in millimeters (mm) depth equivalent. Table 1 presents the main abbreviations employed.
RA and Water—An Introduction to Existing Knowledge
What Idealized Objectives Is RA Seeking?
RA and Water is defined in the literature by five main idealized objectives, or “promises.” The first objective is the optimal crop evapotranspiration of water over time to sustain the viability and growth of rainfed crops to ensure required yields alongside other inputs such as nutrients, seed management and soil husbandry (Ali and Talukder, 2008). The second is reduced surface runoff and flooding, allied to more seepage of water into the soil, resulting in better water quality, and possible reductions in soil salinity (Craggs, 2017; Rhodes, 2017; Schreefel et al., 2020). The third is to facilitate the combined management of rainfall and irrigation water at the field and farm scale (Luo et al., 2022). The fourth refers to the improved management of catchment water withdrawals and allocations enabled by managing soils, rainfall and freshwater resources in a much more coordinated way (Falkenmark and Rockström, 2006). A fifth indirect objective, discussed in later Sections, is a hoped-for increase in soil organic matter content and carbon sequestration (Amelung et al., 2020). We now discuss the first four in order.
A clear expression of the first objective is the unrestrained evapotranspiration of water from crops over a given time period to support local, regional, and global rainfed crop production. For example, in the face of intermittent rainfall, wheat normally requires about 500–600 mm depth equivalent per growing season while sugarcane normally requires about 1,000–2,000 mm of water (FAO, 1999) (N.B. Storage of water within crop biomass is also critical but volumetrically is far less than transpiration). From this objective, four observations follow: (1) volumetrically on a per hectare basis, wheat transpiration requires 5,000–6,000 m3 and sugarcane 10,000–20,000 m3 per growing season. (2) Crop evapotranspiration has to be met by drawing on stores of soil moisture—in turn met from periodic rainfall, capillary rise of moisture or irrigation. (3) If these levels of evapotranspiration are not met, yields suffer as a result. (4) The periodic addition of water and gaps between those additions are mediated and assisted by two “soil-water properties;” the ingress of water into the soil and the storage and availability of water in the soil profile. Thus, a more physically fertile soil is more likely to sustain crop growth over time because soil moisture is on average higher and is more “easily” topped up by rain or irrigation.
The second objective is a reduced risk of erosive flooding. This benefits the local scale “on farm” by retaining soil on slopes which helps maintain soil depth. Connected to this, RA will generate catchment-level benefits when rapid runoff is reduced, in turn leading to more water entering groundwater giving higher base flows fed by groundwater (Zipper et al., 2022). Higher base flows assist with riverine ecosystem services and their management whilst a flashier river regime (high immediate runoff followed by low base flows) will likely harm or stress ecosystems and other water users. Furthermore, associated with slow or less runoff and more baseflow is better water quality. The opposite case also applies; rapid runoff from unprotected degraded soils usually witnesses reduced water quality in surface runoff, streams, rivers, and lakes (Moore et al., 2019).
The third objective sees improved soils retain more rainfall which produces two benefits for irrigation management. First, greater rainfall capture lowers the need for irrigation inputs, in turn reducing demand for water in water-stressed areas. The second is that irrigation scheduling does not need to be so accurate if soils are healthier and hold more water. Using rainfall more effectively to reduce irrigation requirements can be demonstrated quantitatively. If a farmer requires a 700 mm of water for crop evapotranspiration, and 200 mm of that is via capture of rainfall into the soil profile, then 500 mm net irrigation water is required. If improvements in soil-water holding capacity and infiltration rates mean that, for the same annual rainfall, an extra 50 mm of rainfall is effectively captured, then a lower amount of 450 mm net irrigation water is required. However, as discussed below, possible pitfalls can occur with this argument.
Widening our scale to the fourth objective, RA will help manage agricultural water consumption within available water allocations for irrigated agriculture. In order to meet an average 500–900 mm depth of evapotranspiration for all crops per season requires either rainfall or irrigation. It is this water requirement that, in the face of rainfall deficits, results in more than 70% of all water abstracted globally taken by irrigated agriculture (water from rivers, lakes and groundwater) (FAO, 2021). This can be as high as 95% in semi-arid countries such as in Morocco. On ~340–350 million hectares of irrigated land globally, this water use accounts for daily withdrawals of about 7–8 km3 of water (Lankford et al., 2016).
However, the global consensus on food demands by 2050 leans strongly toward an increase in water consumed by agriculture. According to the International Water Management Institute and other observers, without additional improvements to current levels of water productivity or major changes to current production patterns, the volume of water consumption through agricultural evapotranspiration will increase by between 70 and 90% by 2050 (Falkenmark and Rockström, 2006; CAWMA, 2007; Mancosu et al., 2015).
In addition, climate change will bring additional stresses to agriculture and agricultural water demand. Higher transpiration rates from higher temperatures, more erratic precipitation patterns and increased variability in river flows and aquifer recharge are expected to negatively impact crop production. Thus, although rainfed agriculture accounts for the largest proportion of water use in agriculture and provides the world with over 60% of global food supply (Rockström et al., 2007), rainfed farming is more impacted by weather within a variable climate, affecting crop growth, timing of planting and harvesting, and yields. Therefore, food security objectives facing climate change will bring further incentives to equip yet more rainfed land with irrigation (Malabo Montpellier Panel, 2018).
However, the outlook for irrigation to be the solution to climate-framed food insecurity is nonetheless bleak. This is because in many tropical and sub-tropical basins, insufficient water for additional irrigation-based water withdrawals and depletion exists within current water allocations (Mekonnen and Hoekstra, 2020). For example in many parts of the world, the withdrawal of freshwater already exceeds natural renewal capacities leading to increasing incidences of river basin closure (WWAP, 2006; Falkenmark and Molden, 2008). This means irrigation and water allocation in river basins must be co-managed, looking at competing uses and trade-offs, all increasingly within the constraints posed by climate variability (Speed et al., 2013).
Summarizing the above, there is a 3-way problem; (1) the need to sustain or boost food production which means the same or more crop evapotranspiration of water from irrigated (as well as rainfed) agriculture; (2) the reduction of water consumed by irrigation to allocate water to other sectors; and (3) the limited availability of water within the broad parameters of the hydrological cycle. The contribution that RA can play here is that if irrigated soils are regenerated, more rainfall can be captured in situ by the soil to maintain crop production simultaneously reducing the withdrawal of water from river basins. Falkenmark and Rockström (2006, p. 131) similarly conclude their analysis of the same conundrum; “capturing more local rainwater on current farmers' fields.” Thus, an extra 50 mm of effective rainfall lowers irrigation needs by the same, allowing 50 mm less irrigation water to be withdrawn for the same crop and irrigated area. On paper in this idealized calculation, RA potentially helps to manage tight water allocations in water-scarce basins. However, as discussed below, pitfalls exist which means regenerated soils may not have this mitigating effect.
The Two Key Soil-Water Properties Explained
We argue that two key linked soil-water properties are central to RA. These are the readily available levels of water in the soil (Rosenzweig et al., 2020) and the base infiltration rate of the soil within its land cover and geomorphological context (White, 2020). Higher rather than lower soil moisture levels benefit root penetration, vegetative growth, soil processes such as organic matter accumulation, and biological soil activity such as earthworms. Higher rather than lower soil infiltration result in more water penetrating the soil during a given rainfall or irrigation event of a given intensity. This means less water escapes as runoff which means more water tops up soil moisture levels, and/or passes through the underlying groundwater to sustain river base flows and recharge aquifers. The two soil-water properties are discussed in turn.
Readily Available Soil Moisture
Figure 2 contrasts soil moisture levels in two soil types. On the left a degraded soil and on the right a “regenerated” physically fertile soil. The upper line is field capacity (FC) and the lower line is permanent wilting point (PWP). The blue line in both graphs is the extant soil moisture content that increases with rainfall and irrigation but decreases over time due to its consumption from crop and soil evapotranspiration (ET). Drainage of water out of the soil profile is not playing a major role between FC and PWP as it occurs when moisture levels are above field capacity. With the same rainfall regime in both graphs, crops growing in the healthier soil spend more time drawing on more moisture in the soil and, importantly, accessing the more readily available moisture. Therefore, crop ET, growth and yields are higher in the right-hand graph than for the crops growing in the left-hand degraded soil. In addition to rainfall, irrigation and the rate of ET consumption, what establishes or “controls” the actual soil moisture content and its impact on crop growth are the soil properties of total available moisture (TAM) and the readily available moisture (RAM).
TAM reflects the capacity of the soil to hold water for crop transpiration and is set by the upper limit of “field capacity” (FC), the lower limit of “permanent wilting point” (PWP) and plant rooting depth (Using soil water tension units, FC is normally found between −10 and −33 kPa suction and PWP occurs at −1,500 kPa suction). A TAM of 40 mm is very low and denotes a soil that is shallow or sandy, whereas a soil with 200 mm TAM contains a lot of water and denotes a soil that is deep-rooted and has a heavier texture (e.g., clay loam or clay). TAM is vital for crop production as it means that, if daily depletions from crop ET are 5 mm/day, a 40 mm TAM soil lasts “only” 8 days before drying out, whereas the 200 mm TAM soil lasts 40 days. The latter soil can be thought of as “more drought resilient” as crops can last longer between rainfall events.
Readily available moisture (RAM) is the part of the TAM which is readily available to plants to allow “easier and more productive transpiration” which helps maximize growth and yield (In soil water tension terms, RAM occurs between −10 and −100 to −200 kPa, reflecting water held in meso-sized pores). So, while a clay soil holds a lot of water (high TAM), much of that water might be very tightly held in lots of very small soil pores under high tension and not be readily available (low RAM). RAM rather than TAM is the key metric for the physical health of a soil under RA because RAM reflects changes in soil structure and aggregation over time and because a restored soil will have a crumb-size and pore-size distribution that “produces” greater porosity, soil water storage and hydraulic conductivity in this RAM/meso-pore zone (Sasal et al., 2006; Rhodes, 2012) (Note both TAM and RAM can be increased if the crop rooting depth can be increased).
Thus, the same soil over time will witness a relative drop in RAM if the soil is degrading and a higher relative RAM if the soil is recuperating. If 40–70% of TAM is RAM, then the soil is very favorable from the point of view of plant leaf transpiration as it means most of the TAM is readily available to the plant's roots. A soil with a relatively high RAM is physically fertile and could be thought of as “regenerated.” If RAM is low, say <40% of TAM, then we can say the soil is degraded and consequently crops will both temporarily and permanently wilt sooner as RAM and then TAM is depleted. Figure 2 also shows that a higher RAM will be more forgiving for irrigation scheduling as irrigation doses can be less frequent and do not need to be so precise in mm depth application.
Soil Infiltration Rate
The soil infiltration rate (SIR) determines how much water from rainfall and irrigation penetrates the soil. Furthermore, the soil structure of the top 1–5 cm of the soil is a significant determinant of infiltration. Figure 3 contrasts a lower SIR in a less healthy soil on the left with a higher SIR in a regenerated soil. Both soils start with a higher initial infiltration rate which declines to give a lower terminal (base) infiltration rate; a phenomenon influenced by the swelling of soil colloidal and organic material under wetting. The less healthy “degenerated” soil has a more compact soil structure with a lower porosity and its surface may be capped offering few or no large or medium pores where water can enter. Thus, the degraded capped soil in Figure 3, with its terminal infiltration rate of <5 mm/h, will slow the amount of water passing into it leading to more runoff. A “regenerated” clay soil with the same texture but better structure might see this SIR increase to 15 mm/hr which means more water ends up in the soil and/or passing through to groundwater.
It is worth noting that if rainfall is prolonged, the base infiltration rate will control ingress of water into the soil more than the initial infiltration rate. A low base infiltration rate means that for any given rainfall, less water enters the soil leading to surface ponding and/or runoff. For example, rain falling at 30 mm/h exceeds the capacity of the soil's infiltration rate of 15 mm/h to “absorb” that water resulting in a likely 50% of surface runoff. This runoff carries a risk of erosion and flooding, and does not “top up” the soil's moisture levels for crop use. Also, soils in fields with flat gradients witness prolonged surface ponding and low oxygen levels in the soil, harming plant roots and soil organisms.
Actual Levels vs. Soil Properties
We furthermore distinguish “actual levels of soil moisture and soil infiltration,” changing day-to-day or week-to-week, from longer term soil properties changing over months and years. Taking soil moisture as an example, RAM and TAM in the short term can be seen as continuously fluctuating. This asks “what is today's actual or extant moisture level?” which is important determining when to irrigate. In the long-term, RAM and TAM can also be seen as soil properties which asks “how physically healthy is the soil?” This is important, for example, in deciding whether to add organic matter or rest the soil in order to increase RAM and TAM. Importantly, while “short term actual levels” and “long term properties” are related and both can be managed, the crop only senses the former; the actual levels of moisture. In other words, as long as moisture levels are kept high via say artificial irrigation means (and crop nutrients are also sustained), a crop does not “know” it is growing in a soil with a low RAM/TAM as a soil property. This is important for RA because in the short-term, irrigation and its efficiency of field application should match the capacity of the soil to absorb irrigation dosage and sustain extant soil moisture levels. But in the long term total RAM/TAM as a ratio needs to be built up as a measure of soil health and indicator of resilience to drought or intermittent irrigation scheduling.
RA and Water: Idealized Promises
Building on the introduction above, we now hypothesize several ways in which RA and water can be seen as idealized “promises;” particularly as interconnected and recursive beneficial properties and outcomes.
Soil-Water Properties Connect Together
Soil porosity, moisture-levels and soil infiltration rates mutually connect together (Pagliai et al., 2004). They can function to improve each other and RA outcomes. Both readily available soil moisture and infiltration are controlled by the pore size distribution of meso and macro pores, especially in the topsoil. Soil moisture content will not be raised if the soil infiltration rate remains low as no or little water can enter the soil. Similarly, the soil infiltration rate is more likely to reduce over time if soil moisture levels remain consistently low. This “mutual decline” occurs when consistently dry soils; (1) do not undergo drying and wetting (shrink-swell) forces that encourage soil structure formation; and (2) start to lose their organic matter content via oxidation and thus organic binding agents to sustain soil structure diminish. Soil desiccation will also reduce biotic and root activity in the soil. Ideally therefore, RA seeks to create and build soil conditions whereby a greater proportion of total soil moisture is more readily available (higher RAM) and where, via higher infiltration, more soil moisture is refilled adequately when rainfall and irrigation occurs. When these two mechanisms function “ever more mutually,” regenerated soils can be characterized as being more supportive of crop, root and soil biomass growth, and more resilient to breaks in rainfall or lack of irrigation.
Soil Organic Matter Benefits
Higher soil moisture levels and soil infiltration rates connect to two key RA outcomes of higher crop growth and the combined catchment benefits of higher water quality and reduced risk of erosive runoff. However, these two properties can also be linked to RA via its emphasis on soil carbon content and biotic activity (Farrell et al., 2021; Prescott et al., 2021). The RA literature commonly refers to activities associated with improving carbon/organic properties—such as zero till agriculture and mulching—as well as the benefits from higher carbon content and biotic activity—such as better rooting and moisture holding/retention properties (Nunes et al., 2018; Lal, 2020). Raising the content of soil carbon also mitigates climate change if this sequesters atmospheric carbon dioxide through the incorporation of vegetation into soil inert organic matter (IOM) (Coleman and Jenkinson, 1996; Amelung et al., 2020). Soil moisture content and infiltration are connected here because higher moisture levels give rise to higher organic matter inputs and better living conditions for micro-, meso- and macro-organisms and plant roots (Prescott et al., 2021). Improved biotic activity and diversity in turn results in improved soil structure formation from soil turning and movement, and stability from soil organic cements, as well as benefiting nutrient status by increasing ion exchange buffering, soil carbon accumulation and bio-chemical nutrient cycling in turn supporting crop production and drought resilience (Bot and Benites, 2005; Blanco-Canqui et al., 2013).
Resilience Benefits
Adding sufficient water to soils in a timely way via irrigation increases crop production. However, “production maximization” is not the same as “production resilience” able to face shortfalls in rainfall or irrigation water that might occur during drought (Rey et al., 2017). By this we mean resilience as the capacity to absorb a “lack of water” shock. This argument picks up on the discussion in the Section ‘Actual Levels vs. Soil Properties' which showed that a crop growing at a moment in time does not “know” if its roots are growing in a soil with a high TAM (as a soil property) or is growing in a shallow thin soil with a low TAM constantly topped up by micro-dosing with irrigation.
Yet take the two soils described in the Section ‘Readily Available Soil Moisture' and imagine both encounter a drought whereby water cannot be supplied artificially. When ET is 5 mm/day, the 40 mm TAM soil dries up after 8 days and its crops permanently wilt. However, the 200 mm TAM soil continues to provide a crop with moisture for 40 days. Likewise, a crop growing in a soil with a higher RAM draws on readily available moisture for longer than when growing in a low RAM soil. With this thought experiment we argue that the higher TAM/RAM soil is more resilient to drought than the lower TAM/RAM soil, reinforcing the message that a set of mutually beneficial recursive connections exist between soil water content, soil water properties, resilience and regenerated soils (Bot and Benites, 2005). This illustration also emphasizes the resilience benefits, rather than “efficiency” tasks, of RA (Gosnell et al., 2019).
Water as Recursive Inputs and Outputs
RA and water are recursive in that water supports the task of regenerating soils and agriculture, and in turn regenerated soils benefit water and hydrology. Through this recursive lens, we can also see water is both an input and a contributor to RA, as well as being an outcome of or beneficiary of RA. Yet despite this circularity being “tight,” it is also important to be realistic and numerate about what is going on with water as an input and output. To address this, calculations of the very large amounts of water needed to “create RA” in a water stressed catchment are assessed below. Furthermore, given other environmental stresses on soil such as farming practices, drought, erosive flooding, fire and wind erosion, this additional moistness is not guaranteed to create a healthy soil.
An Interconnected System
Building on the above, we introduce the many system factors that interconnect RA and water. Table 2 and Figure 4 present the recursive and circular interconnections between soils, water and RA processes and outcomes operating at the farm, catchment, and policy scales (Robinson et al., 2019). With this table and figure we argue that RA and water is not a linear system which has a single origin and single outcome.
In the top three rows of Table 2 are a number of natural environmental factors that set the context for RA and water. Soil type is important as this establishes and influences the distribution of soil particle sizes (whether clay, sand, or loam etc.) plus natural processes (e.g., chemical weathering, shrink-swell, freeze-thaw) both form soil and attendant soil properties (e.g., cation exchange capacity, porosity, bulk density). With our focus on RA and water, soil physical fertility is key; a soil that was once physically fertile becomes, via extractive intensive farming, physically infertile and via regeneration should return again to being physically fertile. Since the texture of the soil is not that easy to change, the physical fertility of the soil is primarily determined via changes to its structure revealed by a changing RAM. While chemical fertility is important as RA might “leach” nutrients out of the soil, soils with a good physical fertility allow good root penetration and from the perspective of the plant, will have a higher nutrient fertility. Soil structure can be compacted by land preparation and traffic, loss of organic matter and biotic activity, or rainfall impact, and movement of soil fines (silts and clays) down the soil to create dense layers. Ideally a soil must drain to field capacity to allow the roots and soil organisms to breath, but not drain out too much as happens in a sandy soil. Soil organic matter (SOM) and soil biological activity (SBA) are vital in farming because under sustained mono-cropping, intensive plowing and use of chemicals, micro- and macro-organisms (such as beetles and worms) become either depleted in quantity or narrow in diversity. When reversed, a rested regenerated soil should recover SOM and SBA levels.
Factors also include overall agrometeorological and hydrological conditions recognizing for example that temperate agriculture and catchments are wholly different to arid and semi-arid agriculture and catchments. Soil types and their distribution differ in soil texture, depth and soil salinity as well as the topography, relief and position of a farm in the landscape and its geology in turn dictate the gradients and drainage lines and the presence of and depth to a water table (see Figure 1). These factors determine overall moisture levels in the soil, meaning that a deep clay loam in a flat field in a more humid cooler climate is likely to hold more water for longer than a sandier soil on sloping ground in an arid region. Similarly, a high water table replenished by lateral flow will lead to more water in the soil profile through capillary rise. In summary, there will be farms where benign soil-moisture conditions allow farmers to relatively quickly build up SOM and regenerate soils. On the other hand, there will be situations where soils are prone to dryness where creating a biotically rich soil ecology will be difficult.
The bottom three rows of Table 2 briefly explore the human and technical inputs that help control soil moisture levels and infiltration rates. Interpreting their environmental conditions, farmers and other actors adopt, adapt, and drop agricultural and catchment practices. Examples of farming practices include cropping patterns, planting density, mulching, adding extra organic matter (e.g., manure) cover crops, etc., and whether the farm is wholly rainfed or has access to irrigation. With irrigation, the artificial top-up of water puts more water in the soil and sees water as an RA input. The farmer is interested in the efficacy with which rainfall and applied irrigation water is converted to productive crop transpiration. Yet irrigation brings its own challenges such as under- or over-irrigation, increased water consumption, and the risk of increased salinity. The addition of drainage, either surface or subsurface drainage, also helps control water in the soil profile.
As well as farm-scale benefits, regenerated soil-water properties also benefit catchment-scale hydrology. Regenerated soils witness higher infiltration which in turn means the soil profile and its underlying geology both function to filter water and reduce sediment loads. Probably the most understandable connection between RA and water is via water quality. That is, a healthier landscape having a more resilient soil structure with better infiltration properties will have an improved water filtering function for water seeping through to aquifers, and, for a given rainfall event, will reduce runoff carrying a sediment load. Runoff water will not necessarily result in poor water quality but there is an added risk of soil material being carried into waterways with consequences for drinking water quality and eutrophication (Issaka and Ashraf, 2017).
Soil properties also mediate the rainfall-runoff response at the catchment scale. For any given rainfall (or irrigation) event, with its total depth equivalent (mm) and its rate (mm/hour), the soil infiltration rate and capacity to hold water will divide rainfall into that which runs off and that which infiltrates. This in turn determines whether a farm or catchment is prone to rapid runoff from a rainfall event carrying the risk of erosion, sediments, and less water topping up soil RAM.
Figure 4 should be seen recursively and iteratively; that water is influenced by, but is also a requirement of, regenerated soils and agriculture. As Figure 4 principally moves clockwise through the dimensions (from policy through to practices to soil processes and properties to agricultural and catchment outcomes), in reality these dimensions contain feedback loops and are therefore highly interconnected. Working in an anti-clockwise direction, “effects” shape “causes.”
Managing Water and RA “Upwards”
Building on the “systems” view of RA and water, the significant priority of RA can be interpreted as the task of sustaining and improving the two key properties of readily available moisture levels and soil infiltration rates. Thus, Figure 5 has these both depicted in the blue line “increasing” over time following efforts to regenerate soil and landscape. This intends to put soils in a virtuous cycle of continuing to improve the infiltration and retention of water which help with biotic activity and organic matter accumulation which help with vegetative growth and farm and catchment hydrology in the form of the rainfall-runoff response (Nunes et al., 2018; Lal, 2020). In Figure 5, a number of sliding factors are presented which assist with this task, pushing moisture and infiltration rates upwards over time. For example, in the text at the top of the diagram, farming inputs such as well-managed tillage, crop cover and rotations assist in this task. The opposite can also occur; that with adverse environmental and management factors, the two key soil properties can degrade over time.
RA and Water: Risks and Pitfalls
Having connected two soil properties of soil moisture and infiltration to a range of promised benefits in the previous section, what challenges are there in generalizing about the science of RA and water for policy at the farm, basin and global scales? In this Section we hypothesize a number of pitfalls about RA and water exist that often simplify or rely on the above beneficial connections without realizing the nuances or contextual factors involved.
Not Quantifying the Volumes of Water in RA and Water
The first pitfall considers that the amount of water stored in a soil is very different from the amount of water required to keep a soil moister (in order to restore it) in the face of on-going consumption by plants. We therefore explore the volumes of water in RA and water via three calculations, employing TAM and RAM expressed as millimeters and use the conversion of one millimeter on one hectare equating to 10 cubic meters.
The first calculation examines the extra water stored within a regenerated soil across a landscape when it holds more water. For example, an extra 50 mm of readily available moisture (RAM) created by a healthier soil would store 1,000 million cubic meters (MCM) on a landscape unit of 20,000 km2, which is approximately the size of Wales. This may sound a lot, but to fill this extra storage in purely volume terms only needs an effective rainfall of 50 mm. Therefore, we conclude that in purely soil water storage terms, RA soils do not put extra pressure on water resources at the farm and catchment scale.
The second calculation (top of Table 3) asks how much extra water is needed in the form of irrigation to regenerate 10,000 ha of soils to keep them moister (above the RAM threshold) to be more habitable for plants and soil organisms. Table 3 works by comparing a current situation called “degraded soil” with a soil that has been regenerated and as such has required extra water to achieve this. The calculation assumes three levers or magnifiers are in operation; the longer time (an extra 100 days above a season's crop length of 120 days) that a soil is kept moist to promote biological activity; the 10% higher crop ET from more moist soil; and the effect of a 10% lower effective irrigation efficiency which magnifies these net water requirements to give gross depleted demand. The drop in irrigation efficiency to regenerate the soil is explained thus: drip irrigation (ordinarily seen as efficient when only watering the rooting zone) witnesses a lower efficiency when keeping a greater field area moist in turn losing more water from non-beneficial soil evaporation as compared to beneficial crop transpiration. The results in Table 3 indicate that more than a doubling (129%) of water depletion occurs if irrigation is applied to keep soils moist for regenerative purposes above the desired water requirements for crop growth.
Building on the second calculation, the lower part of Table 3 also determines whether the extra water required for regenerating soils can be allocated for RA in a water-scarce catchment. Although hypothetical, results show extra water to keep soils moist (above and beyond purely meeting crop water requirements) would put a huge strain on already water-scarce catchments in semi-arid and semi-humid areas. The water balance in Table 3 switches from a surplus (+ 41 MCM) when farms are drip irrigated “precisely” to meet crop water requirements to a deficit (minus 23 MCM) when irrigation demand increases to regenerate soils. Crucially, this additional depletion is “only” for 10,000 ha and not for watering the whole 20,000 km2 catchment (the area in the first calculation).
We believe this indicative calculation reveals some significant challenges for over-idealized portrayals of RA. It highlights the considerable volumes of water required to actively manage soil moisture for soil regeneration. Indeed the water accounting of the effect of RA-induced demand on farm- and catchment-level water resources is often missing (McLennon et al., 2021). It questions whether in quantity terms water is a “weak link” in soil restoration aiming to capture atmospheric carbon. Returning to the relevance of soil RAM and SIR, this volume of irrigation water has to be managed accurately and effectively if it is to regeneratively function within the soil profile as envisaged rather than “lost” via evaporation, sub-surface, and surface drainage.
Not All Soil-Water Is the Same When Managing Water
When water is seen in general or idealized terms, water inputs to crop growth and soil health might not be sufficiently analyzed. Take for example, a soil with a TAM of 120 mm and a RAM of 60 mm (RAM is 50% of TAM). The same volume of water is required when topping up from zero TAM to say 60 mm (i.e., from PWP to RAM) as it is to top up from 60 mm to full 120 mm (now above RAM). But the pitfall for management is that not all soil-water is the same. The degree to which soil-water is less or more “readily available” depends on changes in the soil moisture content. Thus, water for crop roots as measured by soil-water levels oscillating in the lower dry portion of Figure 1 between the RAM threshold and PWP is “not readily available.” A soil that is “not fully dry” but still below the RAM threshold, represents a harsh environment for soil organisms and crops not accustomed to such desiccation. This is why rainfed farmers growing “normal” field crops such as wheat and maize, hope for soils that in moisture terms sit above the RAM threshold However, the implication of this for crop production and regenerating soils is that there is a considerable step-up in the challenge of managing soil-water if water levels are to be kept above RAM, almost certainly requiring the addition of irrigation which cannot easily or quickly be bolted on to rainfed systems.
Another issue occurs when generalizing the role of organic matter content in benefitting water retention. While higher SOM generally improves water retention overall (meaning TAM), this is not always true. It is more likely that higher SOM benefits the pore-size distribution above RAM and that this effect is more pronounced in coarser-grain soils such as sandy silts (Rawls et al., 2003). This effect reinforces the point that it is readily available moisture rather than total moisture content that is a key soil parameter for RA.
Irrigated RA Is Categorically Different to Rainfed RA
The worked examples of hydrological volumes in RA reveal the need to clearly distinguish between rainfed and irrigated RA. Under rainfed agriculture, soil moisture is managed indirectly by acting on soil and land properties and processes (via cropping, plowing, soil ridges, and so on). With irrigation, soil moisture levels are directly managed via adjusting the irrigation scheduling parameters (depth per dose, frequency, placement, duration etc.). While there are real challenges for both rainfed and irrigated agriculture, to adjust the irrigation parameters so that wasteful or over-depletive irrigation (or indeed under-irrigation) does not occur requires an array of scale-related technical, social, political and economic problems to be solved (Lankford et al., 2020). This is because in irrigation, water is withdrawn, conveyed, distributed, applied, consumed, drained away, slowed down and contaminated with salts or agrochemicals. At each stage there are errors, opportunity costs for, and perspectives on, that water.
To exemplify why soil moisture under rainfed is categorically different to soil moisture under irrigated (or hybrid irrigated/rained farming), let us imagine the actual soil moisture levels for a group of 25 farmers as neighbors on a farming cooperative of 50 hectares. If these farmers are fully rainfed, a cessation of rainfall for 30 days means they watch their crop wilt as extant soil moisture depletes. Their options to bring water to the crop's roots are extremely limited because even adding 1 mm of water weighs 500 tons (although in the long-term they can manage soil TAM/RAM upwards by adding soil organic matter or resting the soil). If, however, the 25 farmers share a canal irrigation system, they can start to respond to the drought; mostly likely after day 10 when they realize their irrigation system cannot water their soils as fast as moisture is being depleted without the help of rainfall. But they have irrigation scheduling options that the rainfed farmers can only dream of. They can; decide which crops in their 2-hectare farms can manage with no water; delay irrigation for other crops; irrigate every other row; and attempt to irrigate only the topsoil by applying a smaller dose. Alongside these technical options are institutional changes; how to monitor who gets what water; how to ensure farmers pass on their water supply sooner; and how to alter the irrigation system to convey smaller flows of water more transparently.
To accurately manage tighter irrigation scheduling parameters, simultaneously minimizing errors and costs while using more water for RA, might suggest that efficient “precision irrigation” is the solution (McLennon et al., 2021). Setting aside the cultural and ethical differences between the application of a modern industrial technology (drip irrigation) within a more holistic approach embodied by RA [see Codur and Watson's (2018) discussion on this], precision irrigation brings significant management problems. For example, the technology confronts smallholder farmers with the responsibility of sharing high operation and maintenance costs that exceed those of gravity/canal systems (Venot et al., 2017). Second, precision irrigation is invariably pumped and pressurized, raising energy needs which, if not met from renewable sources, adds to carbon emissions (Rothausen and Conway, 2011). Third, drip irrigation is more suited to spaced row crops (i.e., fruit trees), not densely packed field “broadacre” crops such as wheat and rice. Fourth, “efficient” irrigation runs the risk of raising basin water depletion by increasing the area under irrigation or switching to other crops (Ahmad et al., 2007; Ward and Pulido-Velázquez, 2008). Thus, promoting precision irrigation requires a considerable parallel effort on farmer support, subsidies, energy and water regulation.
Further complicating the ideal of precision irrigation, the category-type differences between rainfed and irrigated agriculture cannot be diminished or wished away by suggesting that irrigation need only be a little bit supplementary to existing rainfall (Rockström et al., 2010). In other words, in the face of temporal and spatial variations in rainfall (and even with a very well-designed and operated irrigation system) it is extremely difficult to “precisely” administer a small single irrigation dose (say 50 mm) of water to thousands of farmers across an irrigation scheme or irrigated catchment facing an unexpected delay in rainfall. So, while across the world's many agricultural systems we find statistically a continuum of fully rainfed to fully irrigated agriculture, this continuum is not a technological lens to bring “a little bit of irrigation” to rainfed agricultural systems. This irrigation caution applies to both (a) water to meet basic crop needs (the purpose of the Rockström et al., 2010 paper) or (b) water to regenerate soils by filling in rainfall gaps to sustain high levels of soil moisture for soil biological activity.
Furthermore, irrigation brings its own problems to soil health–which need to be managed and mitigated. Soils can be further degraded by various means; (1) leaching of nutrients and soil silt and clay particles from the top soil; (2) raised salinity and/or waterlogging levels which can lead to soil infertility; (3) increased intensity of farming simply by having water available to crop (whereas in rainfed farming, soils might be rested more). Even when farmers have a choice regarding which of the three types of irrigation (flooding, sprinkler, and drip) they use, each will bring different physical impacts to soil depending on how they are designed and managed.
Thus, for RA to benefit from the application of precision “regenerative irrigation” (McLennon et al., 2021) across thousands of hectares of canal-irrigated and/or rainfed agriculture would require an extraordinary level of adaptation and regulation of those systems to switch from being what they currently do (crop-protecting, crop-optimizing, farmer/livelihood optimizing, and system optimizing) to being optimized for regenerative dynamics of soil moisture within the constraints of available water in catchments already facing multiple demands from other sectors.
Reversals Caused by Environmental Disturbances
In Figure 5 we presented an idealized linear development path of the recursive benefits of RA, water, soil health and soil carbon. However, real-world soil and farm ecologies are non-linear and are presented with significant stresses that can disturb this progression toward higher organic matter content, water infiltration and water retention (Sietz et al., 2017; Robinson et al., 2019; Anderegg et al., 2020). These stresses can reverse the gains made and cause loss of soil and soil organic matter (the latter via rapid oxidation). Some stresses are short-lived and exogenous, examples being drought, fire, extreme heat events, and flood- or wind-induced erosion. Other stresses take longer to express themselves and are a result of farming practices. Examples of these include soil salinisation, over-irrigation, or preparing soil in ways that expose soil to erosion such excessive plowing, regular depth plowing (thought to create soil pans) and land preparation (e.g., ridging and seeding) running up and down instead of across the slope (Basic et al., 2004). Even when farmers aim to minimize erosion, chance events can cause erosion, such as intense rain when fields are not covered at the start and end of a cropping season. Other long-term stresses on vegetation, litter incorporation and soil biodiversity arise from excessive use of agro-chemicals (Ganguly et al., 2021) and from biological vectors—for example when pests and diseases cause leaf canopy loss (van Lierop et al., 2015).
The Weak Link in Carbon Sequestration?
Articles draw attention to the climate change benefits of regenerated soils (Amelung et al., 2020; Bossio et al., 2020; UNCCD, 2022). Here, climate change mitigation occurs because plant/crop capture of atmospheric carbon dioxide is incorporated into labile short-term soil organic matter (SOM) and then part of this transfers into stable longer-term inert organic matter (IOM). Beyond the practicability and efficacy of this process in social and economic terms (Amundson and Biardeau, 2018), we are concerned that research in this field does not fully explain how water functions in mediating the multiple pathways of sequestering plant and algal material into IOM in the face of ongoing soil respiration of plant material back into carbon dioxide (Falloon et al., 2011).
While discussions on the role of soils in climate mitigation refer to the co-benefits for water management (Lal, 2016, 2018; Bossio et al., 2020), there is less certainty and clarity regarding soil science explanations for how soil texture, water, soil wetting and drying, pH, salinity, organic carbon and biotic activity interact over time to produce IOM (Błońska and Lasota, 2017; Ramesh et al., 2019). Thus, although optimal soil moisture levels support higher biomass production and soil incorporation, soil moisture's role in converting plant/crop input to long-term IOM is less certain. This is because the production of IOM is reduced when soil respiration increases which occurs under optimal soil moisture conditions (Jones et al., 2005). Although much more research is required, it is not safe to generalize that optimizing soil moisture on farms via rainfall harvesting and irrigation achieves both higher biomass production and long-term carbon sequestration.
Another concern regarding the literature extends to practical interventions for managing soil moisture levels in order to raise soil carbon levels above and beyond that influenced by natural means (e.g., temperature, slope, rainfall, drainage). For these interventions, we believe it is not sufficient for scientists to argue locking up carbon is managed by implementing technologies such as sub-irrigation, drip irrigation and water harvesting without these being fully costed in water accounting, social and economic terms (Rumpel et al., 2020). Even then, there will be many thousands of square kilometers of land where such technologies cannot be economically or technically justified, where reversals in SOM sequestration will occur or where net gains for permanent IOM over a longer time period will be limited due to on-going cropping and biomass harvesting (Post and Kwon, 2000).
Net Zero or Net Positive Water
The terms “net zero” or “net positive” (NP) are used to describe desirable outcomes for carbon (Bistline, 2021) and have also been used to define preferred outcomes in other realms such as urban water (Crosson, 2018). Emissions can be NP if one activity that creates carbon dioxide is “offset” by another that sequesters carbon. In urban water a closed loop is sought; where losses in one part of the system are cleaned, reused and recycled. Here, a negative in one place is canceled out by the plus in another place to arrive at “net zero.” The mirror idealized version for RA and water would see the consumption of water in rainfed or irrigated agriculture “canceled out” by activities that generate water or offset that water consumption elsewhere.
In the real world, such idealized thinking requires caution. RA sits within an open hydrological cycle nested at the catchment and regional scale whereby “units of water use” such as an irrigation system experience this hydrological cycle only “partially” and not fully. This means water for an irrigation system arrives and exits imperfectly or insufficiently. In other words, a 5,000 hectare irrigation system is not its own closed hydrological cycle whereby outflows recycle seamlessly back into inflows.
In this way, RA differs to a managed or closed system such as a municipal water network or a factory site. NP has been tested through water replenishment programs (Coca Cola, 2012) but measured reductions in consumption are restricted to agro-processing factory or bottling sites which typically accounts for 2–3% of total water use when agriculture is considered (Rudebeck, 2019). Therefore, notions that RA water can be “NP” at a basin level are untested and unrealistic. Instead, it is more relevant and useful to employ a local framing of the hydrological cycle when accounting for water. This would mean that water “ins” (rainfall, irrigation) are accounted for and disposed of in ways that in that local frame are “outs” (such as evaporation or seepage). In other words, water cannot be “created” in situ hydrologically, or by expected to flow uphill (or re-used without additional pumping), or by conveniently expanding the frame of reference for that use, or by assuming that water flows through systems without timing effects and that delays do not exist (Lankford, 2012).
Similarly, the source of the irrigation water (groundwater, river, check dam, lake etc.) all respond to differing opportunity costs and social and legal requirements, and to hydrologic realities and timings (dry and wet seasons). One would not tell a farmer in the middle of a drought not to be concerned about a lack of water because the ongoing evapotranspiration from her field was balanced by rainfall occurring 100 miles away. Secondly even if one argued that the distant rainfall was canceling out the evaporation, or that a water offsetting project was “putting water back,” these creative water accounts hold no relevance for a real farmer. From a volumetric perspective, the amount of water used in irrigation or rainfed agriculture simply cannot be de facto “offset” or “replenished,” let alone in ways that genuinely remove the opportunity or consumptive cost of that water in its original “pre-project” use.
Thus, instead of attempting to verify NP idealisations regarding water volumes, it might be better to think of a regenerated soil as a healthier “gate” that switches water away from being “immediate runoff and a few days of evaporation” to being “slower infiltration, seepage flow and a few weeks of transpiration.” Slowing water can be critical as water systems change under a warmer climate capable of holding and releasing larger volumes of water. The restored soil acts more of a buffer or battery, absorbing intense rainfall and re-releasing it slowly to groundwater flows and to crops.
Does “RA Use Less Water” or the Same or More Water?
There are claims suggesting that RA is supposed to experience “less use of water” (Lal, 2020, p. 123). This point is potentially a pitfall if it is not explained and therefore it could be undermined by unstated complexity and nuance, or by a lack of quantification within specific contexts. The following bullet points of “less,” “same,” and “more” use of water by regenerated irrigated soils demonstrate how the expression can be misleading:
• It can be argued that a healthy RA soil under supplementary irrigation will apply and consume less water and that a degraded soil will both require higher applications and deplete more water. A healthier regenerated soil characterized by a high SIR and RAM will likely capture more rainfall and need fewer irrigations. A shallower/lower RAM soil will likely capture less rainfall requiring a greater total application of irrigation water to make up the crop water deficit.
• In addition, the degraded lower RAM soil will need more frequent irrigation of smaller doses. The consequence of this for depletion arises from the technical difficulty of irrigating degraded soils which leads to lower field-level irrigation efficiencies and more water losses, a proportion of which will be non-beneficial and not recovered. Thus, the more degraded lower RAM soil which is more frequently irrigated is “using more water” in numbers of irrigation doses and in terms of total applied and depleted volumes.
• One could argue crops growing in either degraded or regenerated soils that are well-watered “use the same amount of water.” This is because under these “non-stressed” conditions, the transpiration of moisture from leaf surfaces and therefore crop use of soil water is determined by crop and agrometeorological variables such as stomatal resistance, radiation, windspeed, and humidity (Steduto et al., 2007) and not by soil-water properties such as porosity and pore size distribution.
• It is also possible to argue that when not well-watered, a regenerated soil per hectare or per field might “use more water” than a degraded soil. This is because a crop in a healthier soil will be transpiring and consuming more water because it experiences more soil-water as “freely available soil moisture.” The corollary also applies; degraded soils with lower RAM are more likely to present crops and farmers with higher soil moisture stress and poor hydraulic conductivity that together reduce plant transpiration (Bodner et al., 2015; Gonzalez-Dugo et al., 2018).
• Additionally, considering the whole irrigated system, interventions to “use less water” at the field or farm scale can result in an overall increase in basin water consumption (Ward and Pulido-Velázquez, 2008; Grafton et al., 2018). For example, instead of the earlier case whereby healthier soils allow for 50 mm more rainfall capture in order to reduce irrigation withdrawals, the same withdrawal of irrigation continues as before which paradoxically expands the area under irrigation resulting in the same consumption of withdrawn water as before. Furthermore, this “rainfall-capture” induced expansion of the area might then lead to a greater depletive demand for irrigation water when rainfall deficits subsequently occur in those newly expanded areas.
Summarizing, whether RA uses less, the same or more water should not be prefigured on the basis of idealized promises. “Using less water” will depend on scale, context, likely differ between rainfed and irrigated conditions, and have impacts and feedback loops that relate to scale (field, river or basin). Accurate water accounting, tracking empirical data over time, at the relevant local-to-basin scales is required (Lankford et al., 2020; Uhlenbrook et al., 2022).
Emphasizing Local Conditions and Local Voices
Guarding against the pitfall of applying blueprint solutions, we should recognize the paramount importance of local conditions and site-specific RA dynamics and also hear the voices of the farmers (Luján Soto et al., 2021). Policy instruments should be constantly questioned for how they support or undermine the use of water in RA as seen by farmers contextualized within farmer livelihoods situated in a mosaic of soils, weather, land tenure systems, river basins, and landscapes (Guijt and Thompson, 1994; Calo, 2020). Therefore, in small mixed subsidized farms on the humid side of the UK having other income options such as tourism, farmers can entertain switching to other activities, relying on rainfall to rest their land in order to raise soil health. Other farmers, e.g., on drier slopes in Ethiopia, are very much on the margins, both in terms of water access and quantities, but also regarding tenure security, income and lack of subsidies. When livelihoods mainly come from farming, marginal farmers have a narrow bandwidth to rest their land, build up biodiversity and improve soil carbon levels (Pittelkow et al., 2015).
Concluding Remarks
The water requirements and regimes for crop production and soil regeneration in both rainfed and irrigated fields, and the related hydrological functioning of farms and catchments, need to be acknowledged and understood. We argue that water could be better explained by the RA literature at present ranging from a more nuanced understanding of physical factors (including soil-water dynamics) to a fuller exposition of policy and practice factors. RA and water can be seen through the lens of a range of interconnections related to two key soil properties; readily available moisture and soil infiltration rate. With this lens, we also see water is both an input and contributor to RA, as well as being an outcome or beneficiary of RA. Thus, RA and water should be viewed as people-mediated, systems-based, complex, recursive, cyclical, non-linear, and often containing opportunity costs and unstated impacts and feedback loops (Robinson et al., 2019). The corollary also applies; the science of RA and water is far from linear, uni-directional, mono-dimensional, or formulaic.
Yet to only focus on the idealized connections between RA and water, or to assume these are always working in virtuous or vicious circles is not sufficient given the complex task at hand. There are significant challenges with water's role in RA. For example, key roles and processes are rarely distinguished. In addition, with one of these roles (“water as an input to restoring soils”) there is often little quantification of the considerable volumes of water required if applied via irrigation. Furthermore, finding this water in water-scarce basins on top of other priority demands will be challenging. Simply put, there is not enough extra water in some water scarce catchments to boost moisture levels to help regenerate soils. This conclusion implies there is the need to evaluate the wider water-related impacts of RA, accounting for context. Other water-related claims related to RA, especially regarding “net positive” and “net zero” type claims, need to be tempered to match reality. RA could slow down water, and lend itself to improved filtration, but unless very carefully explained and measured, this may not result in “more water” nor require “less water.” Moreover, RA framings that expect considerable soil carbon capture or that directly draw on the carbon cycle, or that skirt over complex soil moisture and soil chemistry regimes, may miss the dynamics, externalities, weak links, and trade-offs that we have drawn attention to.
Improved water and soil-water knowledge is critical to bringing rigor to the RA debate. We therefore ask for greater clarity and quantitative detail to accompany our aspirations and narratives regarding the many connections between “RA and water” and its wider context. In particular, policy-makers should carefully situate RA aspirations inside real world contexts and options. Until such clarity can be established, water-related policy measures, especially those that are “blue-print phrased” or linearly conceived to incentivize RA may need to be rethought. In conclusion, we have transposed this article into a set of key questions that can be asked when discussing both the science and policy of RA and water (Table 4).
Data Availability Statement
The original contributions presented in the study are included in the article/supplementary material, further inquiries can be directed to the corresponding author.
Author Contributions
SO initiated the paper by setting out an array of science and policy questions regarding regenerative agriculture and water. BL provided text and diagrams on soils and water analyses to respond to these questions. All authors completed the paper by drafting, reading, finalizing, and approving the text. Both authors contributed to the article and approved the submitted version.
Funding
This work was supported by funding from WWF International.
Conflict of Interest
SO was employed by WWF International.
The remaining author declares that the research was conducted in the absence of any commercial or financial relationships that could be construed as a potential conflict of interest.
Publisher's Note
All claims expressed in this article are solely those of the authors and do not necessarily represent those of their affiliated organizations, or those of the publisher, the editors and the reviewers. Any product that may be evaluated in this article, or claim that may be made by its manufacturer, is not guaranteed or endorsed by the publisher.
Acknowledgments
The authors would like to thank Brian Richter and Alexis Morgan and the three reviewers for their comments that strengthened our paper. Thank also to those who commented on a brief presentation of this paper at the Soil Health meeting held at the John Innes Center, Norwich, on 3rd May 2022.
References
Ahmad, M.-D., Turral, H., Masih, I., Giordano, M., and Masood, Z. (2007). Water Saving Technologies: Myths and Realities Revealed in Pakistan's Rice-Wheat Systems. Colombo: International Water Management Institute.
Ali, M. H., and Talukder, M. S. U. (2008). Increasing water productivity in crop production—a synthesis. Agric. Water Manage. 95, 1201–1213. doi: 10.1016/j.agwat.2008.06.008
Allan, T., and Dent, D. (2021). “The cost of food: consequences of not valuing soil and water and the people who manage them,” in Regenerative Agriculture, eds D. Dent, and B. Boincean (Cham: Springer). doi: 10.1007/978-3-030-72224-1_1
Amelung, W., Bossio, D., de Vries, W., Kögel-Knabner, I., Lehmann, J., Amundson, R., et al. (2020). Towards a global-scale soil climate mitigation strategy. Nat. Commun. 11, 5427. doi: 10.1038/s41467-020-18887-7
Amundson, R., and Biardeau, L. (2018). Soil carbon sequestration is an elusive climate mitigation tool. Proc. Natl. Acad. Sci. U.S.A. 115, 11652–11656. doi: 10.1073/pnas.1815901115
Anderegg, W. R. L., Trugman, A. T., Badgley, G., Anderson, C. M., Bartuska, A., Ciais, P., et al. (2020). Climate-driven risks to the climate mitigation potential of forests. Science 368, eaaz7005. doi: 10.1126/science.aaz7005
Basic, F., Kisic, I., Mesic, M., Nestroy, O., and Butorac, A. (2004). Tillage and crop management effects on soil erosion in central Croatia. Soil Tillage Res. 78, 197–206. doi: 10.1016/j.still.2004.02.007
Bistline, J. E. T. (2021). Roadmaps to net-zero emissions systems: Emerging insights and modeling challenges. Joule 5, 2551–2563. doi: 10.1016/j.joule.2021.09.012
Blanco-Canqui, H., Shapiro, C. A., Wortmann, C. S., Drijber, R. A., Mamo, M., Shaver, T. M., et al. (2013). Soil organic carbon: the value to soil properties. J. Soil Water Conserv. 68, 129A−134A. doi: 10.2489/jswc.68.5.129A
Błońska, E., and Lasota, J. (2017). Soil organic matter accumulation and carbon fractions along a moisture gradient of forest soils. Forests 8, 448. doi: 10.3390/f8110448
Bodner, G., Nakhforoosh, A., and Kaul, H.-P. (2015). Management of crop water under drought: a review. Agron. Sustain. Dev. 35, 401–442. doi: 10.1007/s13593-015-0283-4
Bossio, D. A., Cook-Patton, S. C., Ellis, P. W., Fargione, J., Sanderman, J., Smith, P., et al. (2020). The role of soil carbon in natural climate solutions. Nat. Sustain. 3, 391–398. doi: 10.1038/s41893-020-0491-z
Bot, A., and Benites, J. (2005). The Importance of Soil Organic Matter: Key to Drought-Resistant Soil and Sustained Food Production. Rome: FAO Food and Agriculture Organisation.
Bullock, P., Newman, A. C. D., and Thomasson, A. J. (1985). Porosity aspects of the regeneration of soil structure after compaction. Soil Tillage Res. 5, 325–341. doi: 10.1016/S0167-1987(85)80001-5
Calo, A. (2020). “Who has the power to adapt?” Frameworks for resilient agriculture must contend with the power dynamics of land tenure. Front. Sustain. Food Syst. 4, 555270. doi: 10.3389/fsufs.2020.555270
CAWMA (2007). Water for Food, Water for Life: Comprehensive Assessment of Water Management in Agriculture (CAWMA). London; Colombo: Earthscan; IWMI.
Coca Cola (2012). Global Water Stewardship and Replenish Report. Atlanta, GA: The Coca Cola Company.
Codur, A.-M., and Watson, J. (2018). “Climate smart or regenerative agriculture? Defining climate policies based on soil health,” in GDAE Climate Policy Brief (Boston, MA: Tufts University: Global Development and Environment Institute). Available online at: https://sites.tufts.edu/gdae/files/2019/10/ClimatePolicyBrief9.pdf.
Coleman, K., and Jenkinson, D.S. (1996). “RothC-26.3 - A Model for the turnover of carbon in soil,” in Evaluation of Soil Organic Matter Models, eds D. S. Powlson, P. Smith, and J. U. Smith (Berlin: Springer), 237–246. doi: 10.1007/978-3-642-61094-3_17
Craggs, G. (2017). The Role of Water in Ensuring Healthy Soils. Dalkeith, WA: Future Directions International Pty Ltd.
Crosson, C. (2018). Innovating the urban water system: achieving a net zero water future beyond current regulation. Technol. Archit. Des. 2, 68–81. doi: 10.1080/24751448.2018.1420966
Dent, D., and Boincean, B, (eds.). (2021). Regenerative Agriculture: What's Missing? What Do We Still Need to Know? Cham: Springer Nature. doi: 10.1007/978-3-030-72224-1
Falkenmark, M., and Molden, D. (2008). Wake up to realities of river basin closure. Int. J. Water Resourc. Dev. 24, 201–215. doi: 10.1080/07900620701723570
Falkenmark, M., and Rockström, J. (2006). The new blue and green water paradigm: breaking new ground for water resources planning and management. J. Water Resourc. Plan. Manage. 132, 129–132. doi: 10.1061/(ASCE)0733-9496(2006)132:3(129)
Falloon, P., Jones, C. D., Ades, M., and Paul, K. (2011). Direct soil moisture controls of future global soil carbon changes: an important source of uncertainty. Glob. Biogeochem. Cycles 25, GB3010. doi: 10.1029/2010GB003938
FAO (1999). Crop Evapotranspiration, FAO Irrigation and Drainage Paper No. 56. Rome: Food and Agriculture Organisation of the United Nations (FAO).
FAO (2021). World Food and Agriculture - Statistical Yearbook 2021. Rome: Food and Agriculture Organisation.
Farrell, M., Richardson, A. E., Cavagnaro, T. R., Wilson, B. R., Glanville, H. C., and Beare, M. (2021). Soil organic matter in a stressed world. Soil Res. 59, i–iv. doi: 10.1071/SRv59n6_ED
Ganguly, R. K., Mukherjee, A., Chakraborty, S. K., and Verma, J. P. (2021). “Chapter 2 - Impact of agrochemical application in sustainable agriculture,” in New and Future Developments in Microbial Biotechnology and Bioengineering, eds J. P. Verma, C. A. Macdonald, V. K. Gupta, and A. R. Podile (Cambridge, MA: Elsevier), 15–24. doi: 10.1016/B978-0-444-64325-4.00002-X
Giller, K. E., Hijbeek, R., Andersson, J. A., and Sumberg, J. (2021). Regenerative agriculture: an agronomic perspective. Outlook Agric. 50, 13–25. doi: 10.1177/0030727021998063
Gonzalez-Dugo, V., Ruz, C., Testi, L., Orgaz, F., and Fereres, E. (2018). The impact of deficit irrigation on transpiration and yield of mandarin and late oranges. Irrigat. Sci. 36, 227–239. doi: 10.1007/s00271-018-0579-7
Gosnell, H., Gill, N., and Voyer, M. (2019). Transformational adaptation on the farm: Processes of change and persistence in transitions to ‘climate-smart' regenerative agriculture. Glob. Environ. Change 59, 101965. doi: 10.1016/j.gloenvcha.2019.101965
Grafton, R. Q., Williams, J., Perry, C. J., Molle, F., Ringler, C., Steduto, P., et al. (2018). The paradox of irrigation efficiency. Science 361, 748–750. doi: 10.1126/science.aat9314
Guijt, I., and Thompson, J. (1994). Landscapes and livelihoods : environmental and socioeconomic dimensions of small-scale irrigation. Land Use Policy 11, 294–308. doi: 10.1016/0264-8377(94)90055-8
Issaka, S., and Ashraf, M. A. (2017). Impact of soil erosion and degradation on water quality: a review. Geol. Ecol. Landsc. 1, 1–11. doi: 10.1080/24749508.2017.1301053
Jones, C., McConnell, C., Coleman, K., Cox, P., Falloon, P., Jenkinson, D., et al. (2005). Global climate change and soil carbon stocks; predictions from two contrasting models for the turnover of organic carbon in soil. Glob. Change Biol. 11, 154–166. doi: 10.1111/j.1365-2486.2004.00885.x
Lal, R. (2016). Beyond COP 21: Potential and challenges of the “4 per thousand” initiative. J. Soil Water Conserv. 71, 20A–25A. doi: 10.2489/jswc.71.1.20A
Lal, R. (2018). Digging deeper: a holistic perspective of factors affecting soil organic carbon sequestration in agroecosystems. Glob. Change Biol. 24, 3285–3301. doi: 10.1111/gcb.14054
Lal, R. (2020). Regenerative agriculture for food and climate. J. Soil Water Conserv. 75, 123A. doi: 10.2489/jswc.2020.0620A
Lankford, B. (2012). Fictions, fractions, factorials and fractures; on the framing of irrigation efficiency. Agric. Water Manage. 108, 27–38. doi: 10.1016/j.agwat.2011.08.010
Lankford, B., Closas, A., Dalton, J., López Gunn, E., Hess, T., Knox, J. W., et al. (2020). A scale-based framework to understand the promises, pitfalls and paradoxes of irrigation efficiency to meet major water challenges. Glob. Environ. Change 65, 102182. doi: 10.1016/j.gloenvcha.2020.102182
Lankford, B., Makin, I., Matthews, N., McCornick, P. G., Noble, A., and Shah, T. (2016). A compact to revitalise large-scale irrigation systems using a leadership-partnership-ownership'theory of change'. Water Alternat. 9, 1–32. Available online at: http://www.water-alternatives.org/index.php/alldoc/articles/302-a9-1-1/file.
Luján Soto, R., Cuéllar Padilla, M., Rivera Méndez, M., Pinto-Correia, T., Boix-Fayos, C., and de Vente, J. (2021). Participatory monitoring and evaluation to enable social learning, adoption, and out-scaling of regenerative agriculture. Ecol. Soc. 26, 29. doi: 10.5751/ES-12796-260429
Luo, W., Chen, M., Kang, Y., Li, W., Li, D., Cui, Y., et al. (2022). Analysis of crop water requirements and irrigation demands for rice: implications for increasing effective rainfall. Agric. Water Manage. 260, 107285. doi: 10.1016/j.agwat.2021.107285
Malabo Montpellier Panel (2018). Water-wise: Smart Irrigation Strategies for Africa. Washington, DC: International Food Policy Research Institute.
Mancosu, N., Snyder, R. L., Kyriakakis, G., and Spano, D. (2015). Water scarcity and future challenges for food production. Water 7, 975–992. doi: 10.3390/w7030975
McLennon, E., Dari, B., Jha, G., Sihi, D., and Kankarla, V. (2021). Regenerative agriculture and integrative permaculture for sustainable and technology driven global food production and security. Agron. J. 113, 4541–4559. doi: 10.1002/agj2.20814
Mekonnen, M. M., and Hoekstra, A. Y. (2020). Sustainability of the blue water footprint of crops. Adv. Water Resourc. 143, 103679. doi: 10.1016/j.advwatres.2020.103679
Molle, F. (2008). Nirvana concepts, narratives and policy models: insights from the water sector. Water Alternat. 1, 131–156. Available online at: https://www.water-alternatives.org/index.php/allabs/20-a-1-1-8/file.
Moore, K. J., Anex, R. P., Elobeid, A. E., Fei, S., Flora, C. B., Goggi, A. S., et al. (2019). Regenerating agricultural landscapes with perennial groundcover for intensive crop production. Agronomy 9, 458. doi: 10.3390/agronomy9080458
Newton, P., Civita, N., Frankel-Goldwater, L., Bartel, K., and Johns, C. (2020). What is regenerative agriculture? A review of scholar and practitioner definitions based on processes and outcomes. Front. Sustain. Food Syst. 4, 577723. doi: 10.3389/fsufs.2020.577723
Nunes, M. R., van Es, H. M., Schindelbeck, R., Ristow, A. J., and Ryan, M. (2018). No-till and cropping system diversification improve soil health and crop yield. Geoderma 328, 30–43. doi: 10.1016/j.geoderma.2018.04.031
Pagliai, M., Vignozzi, N., and Pellegrini, S. (2004). Soil structure and the effect of management practices. Soil Tillage Res. 79, 131–143. doi: 10.1016/j.still.2004.07.002
Pittelkow, C. M., Liang, X., Linquist, B. A., van Groenigen, K. J., Lee, J., Lundy, M. E., et al. (2015). Productivity limits and potentials of the principles of conservation agriculture. Nature 517, 365–368. doi: 10.1038/nature13809
Post, W. M., and Kwon, K. C. (2000). Soil carbon sequestration and land-use change: processes and potential. Glob. Change Biol. 6, 317–327. doi: 10.1046/j.1365-2486.2000.00308.x
Prescott, C. E., Rui, Y., Cotrufo, M. F., and Grayston, S. J. (2021). Managing plant surplus carbon to generate soil organic matter in regenerative agriculture. J. Soil Water Conserv. 76, 99A–104A. doi: 10.2489/jswc.2021.0920A
Ramesh, T., Bolan, N. S., Kirkham, M. B., Wijesekara, H., Kanchikerimath, M., Srinivasa Rao, C., et al. (2019). “Chapter one - soil organic carbon dynamics: impact of land use changes and management practices: a review,” in Advances in Agronomy, ed D. L. Sparks (Amsterdam: Academic Press), 1–107. doi: 10.1016/bs.agron.2019.02.001
Rawls, W. J., Pachepsky, Y. A., Ritchie, J. C., Sobecki, T. M., and Bloodworth, H. (2003). Effect of soil organic carbon on soil water retention. Geoderma 116, 61–76. doi: 10.1016/S0016-7061(03)00094-6
Rey, D., Holman, I. P., and Knox, J. W. (2017). Developing drought resilience in irrigated agriculture in the face of increasing water scarcity. Reg. Environ. Change 17, 1527–1540. doi: 10.1007/s10113-017-1116-6
Rhodes, C. J. (2012). Feeding and healing the world: through regenerative agriculture and permaculture. Sci. Prog. 95, 345–446. doi: 10.3184/003685012X13504990668392
Rhodes, C. J. (2017). The imperative for regenerative agriculture. Sci. Prog. 100, 80–129. doi: 10.3184/003685017X14876775256165
Robinson, D. A., Hopmans, J. W., Filipovic, V., van der Ploeg, M., Lebron, I., Jones, S. B., et al. (2019). Global environmental changes impact soil hydraulic functions through biophysical feedbacks. Glob. Change Biol. 25, 1895–1904. doi: 10.1111/gcb.14626
Rockström, J., Hatlbu, N., Owels, T. Y., and Wani, S. P. (2007). “Managing water in rainfed agriculture,” in Water for Food, Water for Life: A Comprehensive Assessment of Water Management in Agriculture, ed D. Molden (International Water Management Institute, Earthscan), 315–352.
Rockström, J., Karlberg, L., Wani, S. P., Barron, J., Hatibu, N., Oweis, T., et al. (2010). Managing water in rainfed agriculture—the need for a paradigm shift. Agric. Water Manage. 97, 543–550. doi: 10.1016/j.agwat.2009.09.009
Rosenzweig, S. T., Carolan, M. S., and Schipanski, M. E. (2020). A dryland cropping revolution? Linking an emerging soil health paradigm with shifting social fields among wheat growers of the high plains. Rural Sociol. 85, 545–574. doi: 10.1111/ruso.12304
Rothausen, S. G. S. A., and Conway, D. (2011). Greenhouse-gas emissions from energy use in the water sector. Nat. Clim. Change 1, 210–219. doi: 10.1038/nclimate1147
Rudebeck, T. (2019). Corporations as Custodians of the Public Good? Exploring the Intersection of Corporate Water Stewardship and Global Water Governance. Cham: Springer. doi: 10.1007/978-3-030-13225-5
Rumpel, C., Amiraslani, F., Chenu, C., Garcia Cardenas, M., Kaonga, M., Koutika, L.-S., et al. (2020). The 4p1000 initiative: opportunities, limitations and challenges for implementing soil organic carbon sequestration as a sustainable development strategy. Ambio 49, 350–360. doi: 10.1007/s13280-019-01165-2
Sasal, M. C., Andriulo, A. E., and Taboada, M. A. (2006). Soil porosity characteristics and water movement under zero tillage in silty soils in argentinian pampas. Soil Tillage Res. 87, 9–18. doi: 10.1016/j.still.2005.02.025
Schreefel, L., Schulte, R. P. O., de Boer, I. J. M., Schrijver, A. P., and van Zanten, H. H. E. (2020). Regenerative agriculture – the soil is the base. Glob. Food Sec. 26, 100404. doi: 10.1016/j.gfs.2020.100404
Sietz, D., Fleskens, L., and Stringer, L. C. (2017). Learning from non-linear ecosystem dynamics is vital for achieving land degradation neutrality. Land Degrad. Dev. 28, 2308–2314. doi: 10.1002/ldr.2732
Six, J., Feller, C., Denef, K., Ogle, S., Joao Carlos De Moraes, S.a, and Albrecht, A. (2002). Soil organic matter, biota and aggregation in temperate and tropical soils—effects of no-tillage. Agronomie 22, 755–775. doi: 10.1051/agro:2002043
Speed, R., Yuanyuan, L., Zhiwei, Z., Le Quesne, T., and Pegram, G. (2013). Basin Water Allocation Planning: Principles, Procedures and Approaches for Basin Allocation Planning. Paris: Asian Development Bank, GIWP, UNESCO, and WWF-UK.
Steduto, P., Hsiao, T. C., and Fereres, E. (2007). On the conservative behavior of biomass water productivity. Irrigat. Sci. 25, 189–207. doi: 10.1007/s00271-007-0064-1
Uhlenbrook, S., Yu, W., Schmitter, P., and Smith, D. M. (2022). Optimising the water we eat—rethinking policy to enhance productive and sustainable use of water in agri-food systems across scales. Lancet Planet. Health 6, e59–e65. doi: 10.1016/S2542-5196(21)00264-3
UNCCD (2022). The Global Land Outlook, 2nd Edn. Bonn: United Nations Convention to Combat Desertification.
van Lierop, P., Lindquist, E., Sathyapala, S., and Franceschini, G. (2015). Global forest area disturbance from fire, insect pests, diseases and severe weather events. For. Ecol. Manage. 352, 78–88. doi: 10.1016/j.foreco.2015.06.010
Venot, J.-P., Kuper, M., and Zwarteveen, M., (eds.). (2017). Drip Irrigation for Agriculture: Untold Stories of Efficiency, Innovation, and Development. Abingdon, VA: Routledge. doi: 10.4324/9781315537146
Ward, F. A., and Pulido-Velázquez, M. (2008). Water conservation in irrigation can increase water use. Proc. Natl. Acad. Sci. U.S.A. 105, 18215–18220. doi: 10.1073/pnas.0805554105
White, C. (2020). Why regenerative agriculture? Am. J. Econ. Sociol. 79, 799–812. doi: 10.1111/ajes.12334
World Water Assessment Programme (2006). Water: A Shared Responsibility; The United Nations World Water Development Report 2. UNESCO World Water Assessment Programme. Paris: UNESCO.
Zipper, S. C., Farmer, W. H., Brookfield, A., Ajami, H., Reeves, H. W., Wardropper, C., et al. (2022). Quantifying streamflow depletion from groundwater pumping: a practical review of past and emerging approaches for water management. JAWRA J. Am. Water Resourc. Assoc. 58, 289–312. doi: 10.1111/1752-1688.12998
Keywords: allocation, catchments, crops, hydrology, irrigation, rainfed, soils
Citation: Lankford B and Orr S (2022) Exploring the Critical Role of Water in Regenerative Agriculture; Building Promises and Avoiding Pitfalls. Front. Sustain. Food Syst. 6:891709. doi: 10.3389/fsufs.2022.891709
Received: 08 March 2022; Accepted: 12 May 2022;
Published: 13 June 2022.
Edited by:
Luuk Fleskens, Wageningen University and Research, NetherlandsReviewed by:
Kathy Boomer, Foundation for Food and Agriculture Research (FFAR), United StatesAna Iglesias, Polytechnic University of Madrid, Spain
Luigi Piemontese, University of Florence, Italy
Copyright © 2022 Lankford and Orr. This is an open-access article distributed under the terms of the Creative Commons Attribution License (CC BY). The use, distribution or reproduction in other forums is permitted, provided the original author(s) and the copyright owner(s) are credited and that the original publication in this journal is cited, in accordance with accepted academic practice. No use, distribution or reproduction is permitted which does not comply with these terms.
*Correspondence: Bruce Lankford, Yi5sYW5rZm9yZEB1ZWEuYWMudWs=