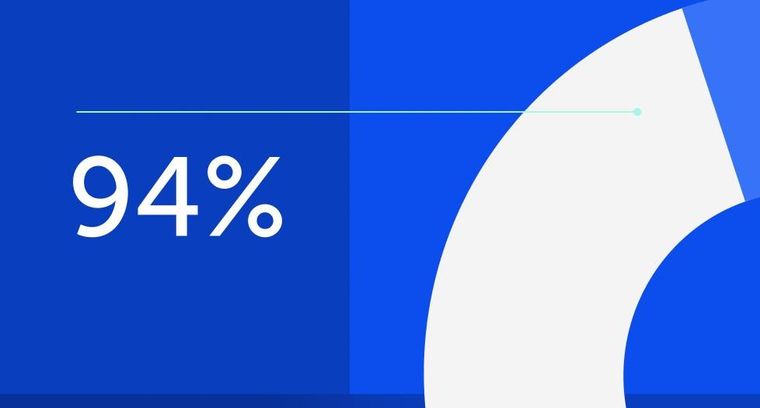
94% of researchers rate our articles as excellent or good
Learn more about the work of our research integrity team to safeguard the quality of each article we publish.
Find out more
REVIEW article
Front. Sustain. Food Syst., 15 June 2022
Sec. Climate-Smart Food Systems
Volume 6 - 2022 | https://doi.org/10.3389/fsufs.2022.891256
This article is part of the Research TopicMitigation and Adaptation Strategies Involving Nitrogen Management for Enhancing Agro-Ecosystem Productivity and Resilience to Future Climate ChangeView all 5 articles
As demand for food production continues to rise, it is clear that in order to meet the challenges of the future in terms of food security and environmental sustainability, radical changes are required throughout all levels of the global food system. Controlled Environment Agriculture (CEA) (a.k.a. indoor farming) has an advantage over conventional farming methods in that production processes can be largely separated from the natural environment, thus, production is less reliant on environmental conditions, and pollution can be better restricted and controlled. While output potential of conventional farming at a global scale is predicted to suffer due to the effects of climate change, technological advancements in this time will drastically improve both the economic and environmental performance of CEA systems. This article summarizes the current understanding and gaps in knowledge surrounding the environmental sustainability of CEA systems, and assesses whether these systems may allow for intensive and fully sustainable agriculture at a global scale. The energy requirements and subsequent carbon footprint of many systems is currently the greatest environmental hurdle to overcome. The lack of economically grown staple crops which make up the majority of calories consumed by humans is also a major limiting factor in the expansion of CEA systems to reduce the environmental impacts of food production at a global scale. This review introduces the concept of Integrated System CEA (ISCEA) in which multiple CEA systems can be deployed in an integrated localized fashion to increase efficiency and reduce environmental impacts of food production. We conclude that it is feasible that with sufficient green energy, that ISCEA systems could largely negate most forms of environmental damage associated with conventional farming at a global scale (e.g., GHGs, deforestation, nitrogen, phosphorus, pesticide use, etc.). However, while there is plenty of research being carried out into improving energy efficiency, renewable energy and crop diversification in CEA systems, the circular economy approach to waste is largely ignored. We recommend that industries begin to investigate how nutrient flows and efficiencies in systems can be better managed to improve the environmental performance of CEA systems of the future.
- CEA systems have large potential for environmentally sustainable food production
- Expansion of current CEA systems is limited by economic factors and technological constraints
- Environmental sustainability of CEA systems depends largely on availability of low or zero-carbon electricity
- Integrated system CEA (ISCEA) may be the most sustainable form of food production possible in the future
The major challenge of the 21st century will be feeding a global population of 8–11 billion in a sustainable way (UN sustainable development goals 2, 6, 11). Human activities directly associated with agriculture (including land-use change, food waste and the supply chain) account for 21–37% of all greenhouse gas (GHG) emissions each year (IPCC, 2019). Just as food demand increases, climate models predict that conditions for crop production in much of the world will deteriorate through increases in the frequency and intensity of extreme hydro-meteorological events such as extended periods of drought and flooding (e.g., Eyring et al., 2021). Furthermore, the expansion and intensification of conventional farming practices to meet global food demand may result in severe detrimental environmental consequences (e.g., deforestation, eutrophication, reduced biodiversity, and poor air and water quality) (e.g., IPCC, 2019; Malone and Newton, 2020; Wade et al., 2020). The global food system accounts for 38% of global land surface cover (Roser, 2019) and is recognized to be the primary driver of global biodiversity loss due to human activities such as land use change (LUC), pesticide use and pollution (Benton et al., 2021). Agriculture accounts for approximately 70% of global freshwater withdrawals globally, on top of water consumption after rainfall and evapotranspiration (World Bank, 2020). Taking all of these impacts into account, it is clear that, in order to meet the challenges of the future in terms of food security and environmental sustainability, radical changes are required throughout all levels of the global food system, including production, land-use and supply chain stages.
One potential option to help realize this radical change, and meet future food demand in a more sustainable way, is the application of controlled environment agriculture (CEA) systems. CEA systems cover a range of production methods, and are defined by the ability to control the environment in which crops are grown. This means that crops are typically grown within some form of fabricated structure, i.e., “indoor agriculture.” Indoor agriculture includes cultivation within a glasshouse or plastic structure (any structure that can controlled from external environmental factors) with a single layer of crops exposed to direct sunlight, or within multiple vertical stacked racks under artificial lighting with nutrient solutions delivered via automated systems (e.g., vertical farming). As well as traditional crop production, other common food production methods such as mushroom, insect and fish farming (aquaculture and aquaponics) are also typically carried out in CEA systems (indoor controlled environments). And while it is still in its infancy, lab-grown meat methods may also fall into the category of CEA as a food production industry that operates in a synthetic environment, with a large degree of separation from nature.
One major beneficial environmental factor of certain CEA systems is that the systems are “closed;” meaning that they are less susceptible to external factors such as fluctuations in precipitation and temperature, and resilience to environmental stressors is increased. Exposure to the spread of pests (i.e., insects and disease) is also decreased, thus pesticide use, and resultant environmental exposure to pesticides are both radically lower than conventional agriculture systems. Nutrients in these systems also have the potential to be controlled and recirculated, particularly where hydroponic and aeroponic systems are used (e.g., Goddek et al., 2019; Rufí-Salís et al., 2020), thus nutrient use efficiency can be increased and losses into the natural environment can be negated almost completely, significantly reducing phosphorus (P) and nitrogen (N) pollution. However, CEA systems are more complex than this vision may suggest, and their scaling-up is currently hampered by the limitations of technology, economics and societal factors.
This article aims to highlight the current understanding and gaps in knowledge surrounding the environmental sustainability of CEA systems, and assess whether these systems may allow for intensive and sustainable agriculture at a global scale. While focussing on the knowledge available regarding a variety of CEA systems in their current form, the potential for advancement in CEA systems will also be discussed in respect to development of future technology, adaptation to climate change, and integration of CEA systems to achieve a circular economy and ultimately, sustainable agriculture at a global scale.
While the feasibility of conventional agriculture (i.e., crop production) is directly related to local environmental conditions, the needs of CEA systems are drastically different. Commonly-used CEA systems such as hydroponics and aeroponics are able to grow crops in inert mediums (e.g., rock wool, saw dust, coconut husk and hemp) and do not require soil. Therefore, large-scale CEA operations can be carried out on land where soils are considered barren or contaminated, as the crops are not grown using the terrain itself (Khan et al., 2020). This means that CEA systems can, in theory, be built and thrive in any location, including deserts, tundra or industrial urban areas (Al-Chalabi, 2015; Saad et al., 2021). As well as this adaptability to terrain, CEA systems commonly use artificial light to grow crops. Using artificial light allows crops to be stacked in shelf-like growing conditions, thus greatly increasing the production capability of CEA systems for a given area and potentially only physically limited by the structures' housing production (Banerjee and Adenaeuer, 2014). These controlled conditions allow production of a large variety of crops to occur all year round in optimal conditions, and seasonal harvests are no longer required, thus annual production increases substantially in comparison to conventional farming (e.g., Wilson and Finlay, 1995; Barbosa et al., 2015). All-year-round production also avoids the concentration of activity and production bottlenecks experienced by conventional farming at certain times of the year, such as harvest season. While it has been reported that annual production of certain crops in CEA systems (vertical farming in particular) can exceed 100 times the yield expected from the same area of conventional farming (Banerjee and Adenaeuer, 2014; Benke and Tomkins, 2017), the practicalities of this limit are controlled by economic and structural considerations, based on the crop type and growing methods. Thus, the true potential of CEA systems is difficult to predict, as this will be determined by changing technological availability and economic contexts.
The water requirements of CEA systems are also very different to conventional agricultural methods. In contained structures (roofed, glasshouse, plasticulture, etc.) evapotranspiration is reduced significantly, while precise water application and drip irrigation also minimize water requirements (O'Connor and Mehta, 2016). Hydroponic and vertical farming methods may save up to 99% of water required to grow certain crops (e.g., Benke and Tomkins, 2017; Graamans et al., 2017; Kalantari et al., 2018). It is also possible to recycle municipal wastewater for use in vertical farm irrigation, with potential to purify water (Ellingsen and Despommier, 2008), or substitute with brackish water using desalination processes (Da Silva et al., 2018). As well as reducing water consumption (and water-related energy costs), CEA systems can operate in arid desert land at large scales where conventional agricultural practices are not feasible and land suitable for crop production is already saturated or under threat. This is an important advantage when it comes to food security for nations without adequate land in which to become self-sustainable [e.g., Singapore (Wang, 2021)] and arid regions (e.g., the Middle-East). Populations in these areas typically rely heavily on foreign trade and imports of food which are susceptible to significant uncertainty in the future due to the unpredictable impacts of climate change and trade-impacting events such as the COVID-19 pandemic (e.g., Sippel, 2015; de Anda and Shear, 2017; Abusin and Mandikiana, 2020; Woertz, 2020).
The most significant environmental disadvantage to CEA systems is the carbon footprint associated with the energy use required by the systems. CEA systems are not always reliant on electricity use (e.g., glasshouse horticulture); however, to reach full potential, artificial lighting and temperature control (heating and cooling) would be required to maximize productivity in many systems (e.g., Badiola et al., 2018; Kalantari et al., 2018). While it is possible to use solar, geothermal or waste heat from industrial processes to mitigate energy use and costs (e.g., Adams et al., 2011; Teo and Go, 2021), the world is still highly reliant on fossil fuels for electricity. Few nations are able to generate enough renewable energy to meet their needs fully without supplementing power grids with fossil fuels. The importance of this is that with renewable energy, CEA systems are able to become a net uptake of carbon (until consumption) as the process of photosynthesis will become larger than emissions (which can be theoretically zero). An example of this would be in bioenergy production, which could contribute to cutting carbon emissions where the correct systems are in place (e.g., Harris and Kountouris, 2020).
Global offsetting of N2O (and CH4 for livestock) will always be necessary to reach net-zero global warming impact (i.e., reforestation and carbon capture); however, where net carbon-uptake CEA systems can be integrated, this offsetting could remain within the agricultural sector without the need for continual land-use change or industrial CO2 removals. The future value of integrating CEA systems for carbon capture is that agricultural and industrial emissions of CO2 from other sources (e.g., fossil fuel burning, sugar refining, composting, etc…) can be redirected through and captured by crop production systems which benefit from elevated CO2 concentrations in the atmosphere. Here, emissions can be reduced drastically from exterior operations while providing a benefit to CEA crop production.
In terms of other major GHG sources from food production (i.e., CH4 and N2O), little research has been carried out in CEA systems. Any CH4 emissions from CEA systems would be dependent on the system, primarily the substrates used in which to grow crops (i.e., organic materials). While activities such as mushroom farming will inherently emit small quantities of CH4 from substrates (e.g., Dorr et al., 2021), emissions from the vast majority of CEA crop production systems would be negligible as the carbon-rich anaerobic conditions required for methanogens to thrive would not be present. One potential for CEA systems to drastically reduce GHG emissions in the food supply is by shifting meat production away from traditional ruminant livestock methods toward insect production and lab-grown meat. Emissions of all GHGs associated with insect production is extremely small compared to traditional ruminant livestock, especially that of beef (Oonincx et al., 2010). However, unlike insect farming, current technology prohibits any environmental advantage of the mass production of lab-grown meat, which currently has a higher carbon footprint than conventional meat production processes due to very high energy requirements (Lynch and Pierrehumbert, 2019). While there is potential for CEA systems such as insect farming and lab-grown meat to gradually replace the relatively highly polluting livestock industry, a significant amount of research and development into system efficiency and the long-term impacts of these changes on human health is required (van Huis and Oonincx, 2017; Specht et al., 2019; Tuomisto, 2019).
Like CH4, emissions of N2O from CEA systems will depend predominantly on substrates/growing media on which crops are planted and grown. While emissions of N2O from inert materials (e.g., rock wool or perlite) without an established microbiome (hosting microbes to perform nitrification and denitrification) could be expected to be minimal, few studies have investigated this; with mixed results for a limited number of crops (e.g., Yoshihara et al., 2014; Halbert-Howard et al., 2020). Where nitrogen and carbon-rich waste products are generated in aquatic systems, such as aquaculture, high emissions of N2O have been observed (Hu et al., 2012).
A switch from conventional to CEA farming requires a large initial investment in infrastructure. This investment comes in the form of building materials (e.g., steel and concrete) and a potentially large embedded carbon footprint, as well as financial cost (e.g., Forchino et al., 2018; Sarkar and Majumder, 2018; Martin and Molin, 2019). The magnitude of this carbon investment will vary depending on a number of factors, specifically the size of the facility and the materials used to construct it, but in most cases, it will be substantial, with larger facilities in excess of millions of USD. However, CEA systems can be built on barren land or within urban areas, and often utilize disused industrial buildings (urban voids) to reduce start-up costs (e.g., Dal Ri et al., 2020). This means that, while rapid expansion of CEA systems could significantly boost global food production, deforestation and large scale land-use change would not be required-which is often not the case for conventional farming. It has also been recorded that CEA systems can have a cooling effect locally due to reflectance of sunlight. Campra et al. (2008) showed that a continuous area of 26,000 ha of greenhouse agriculture in Almería (Spain) resulted in a surface air temperature trend of −0.3°C over 10 years in contradiction to rising regional and global warming trends.
While embedded carbon emissions associated with infrastructure are not trivial, it is the energy demands of CEA systems that dominate emissions in most systems (e.g., Barbosa et al., 2015; Martin and Molin, 2019; Song et al., 2022). As a result, the long-term sustainability of CEA systems (comparative to conventional farming) will depend on operational/upkeep carbon emissions, rather than initial infrastructure (same principal as electric cars). Due to high operational costs, there is a focus on optimizing energy efficiencies in these systems, predominantly through improved lighting technology and techniques (e.g., Avgoustaki and Xydis, 2020; Wong et al., 2020) and temperature control, which is also highly impacted by geographic location (Georgiou et al., 2018; Engler and Krarti, 2021).
The carbon footprint of the transportation of goods in the supply chain is also a significant factor in the sustainability of CEA systems. While this will vary for both CEA and conventional systems depending upon mode of transport, distance between production location and supplier/consumers is the main factor by which to compare the methods. Availability of food grown via conventional farming methods is limited by both seasonality (i.e., harvest timing) and storage of goods. As a result, availability of certain foods on an all-year-round basis is subject to either storage requirements (refrigeration or freezing) or international trade, both of which can have high associated carbon emissions and high loss and wastage risks (Dalin and Rodríguez-Iturbe, 2016). The potential advantage for CEA systems here is that food can be produced at a stable rate, in or near urban centers all year round, thus greatly reducing the need for long-range transportation and the associated carbon emissions. However, future development of sustainable CEA facilities will have to factor in the balance of emissions as a result of transportation against energy savings made using ideal environmental conditions and energy availability (i.e., geothermal energy and daylight). These assessments will have to be made on a case-by-case basis, and priority may change with emerging technology (i.e., electric vehicles and availability of renewable energy).
One potentially significant advantage of CEA systems over conventional farming is in the ability to drastically reduce pesticides applied. While argued that pesticides will not be required in future CEA systems due to their enclosure and separation from the environment (Despommier, 2013), it is unlikely that these systems will be capable of remaining completely pest free. However, compartmentalized systems with limited exposure to external factors will result in unique opportunities to significantly reduce both the amount of pesticides applied and exposure to the external environment. As well as having physical boundaries to prevent pathogens, there is also more scope to use technology to monitor and detect health problems within crops, and therefore prevent spread, potentially in tandem with automated robotics for real time pest detection/removal processes (e.g., Lauguico et al., 2019).
The ability to cut out pesticides allows for in-house pollinators to live within the confines of a CEA cropping system. The use of bees to pollinate crops in glasshouses is common, especially for tomato crops (Velthuis and van Doorn, 2006). While most crops currently grown in CEA systems (e.g., leafy greens) do not require pollinator interactions, future attempts to produce staple crops may require more bespoke pollination methods to account for the lack of natural ecosystem services within indoor systems. While technology is developing to account for pollination activities without the need for manual interaction with crops by using drones or automated machinery (e.g., Ohi et al., 2018; Guzman et al., 2021), insects are still by far the most effective and efficient means by which to pollinate large quantities of crops. However, where cereal crops are concerned, artificial wind may be all that is required to replicate conditions required to pollinate crops such as wheat and barley grown indoors.
Where unwanted insects (pests) infiltrate CEA systems it is likely that, with appropriate vigilance, simple bio-controls can be used in place of harmful pesticide compounds [e.g. ladybugs to combat aphid infestation (Mattson et al., 2017)]. Where bio-controls are implemented, greater control is possible when compared to conventional farming, especially for agents that can fly as they are more likely to remain within structural boundaries (Roberts et al., 2020). However, the current lack of quantitative scientific investigation into this subject means that it is not possible to say with confidence whether CEA systems of the future are likely to eliminate pesticide use in agriculture, even if the potential exists (Roberts et al., 2020).
The importance of recycling nutrients in agricultural systems is 2-fold. As well as economic inefficiencies as a result of waste, nutrient losses in conventional agriculture cause a significant amount of environmental damage at a global scale. It is estimated that over 80% of Nitrogen (N) and 25–75% of phosphorus (P) applied to agricultural fields globally is lost to the environment, as well as the energy used to produce them (Sutton et al., 2013). Losses of N and P from food systems largely end up in natural aquatic bodies due to leaching and run-off, which results in significant damage to aquatic biodiversity and water quality (Malone and Newton, 2020), as well as increasing GHG emissions from aquatic sources. An advantage of environmentally segregated CEA systems is that nutrient solutions can be recycled in a closed-loop, which can increase efficiency (Rufí-Salís et al., 2020). However, this approach is not fully circular and nutrients are still lost in current commercial/industrial scale systems. While solutions can be recycled in systems, gradual imbalances in the chemical composition of the solution will typically result in increasing salt content, and the solution will be flushed and replaced with a fresh supply. One of the major advantages that CEA systems may have in regards to nutrient management is that monitoring of nutrients available to crops can be easily measured, and in some instances dynamically manipulated (e.g., Michael et al., 2021). While conventional farming requires a myriad of technology to provide precision farming methods the capability to attempt to optimize fertilizer application, the exact amount of nutrients and the timing of their delivery of the system can be controlled with exact precision in CEA systems.
While offering many potential advantages over conventional farming methods, no CEA system is entirely efficient or sustainable on its own. However, as waste streams from CEA systems are fully controlled and predictable, there is potential to combine multiple systems to increase recycling of nutrients and materials. The concept of Integrated System Controlled Environment Agriculture (ISCEA) in its most basic form is most common in the form of aquaponics: the merging of hydroponics with aquaculture in such a way as the two systems can operate in a symbiotic fashion (e.g., Shafahi and Woolston, 2014; Goddek et al., 2015). While recycling of waste between CEA systems is not utilized commercially (as described in Figure 1), there is a large potential to integrate these systems in future and introduce a circular thinking to nutrient management (Sayadi-Gmada et al., 2019; Aznar-Sánchez et al., 2020; Rufí-Salís et al., 2020). Large quantities of nutrient solution will be flushed from hydroponic and aeroponic systems due to increasing salinity and to remove pathogens. While crops are sensitive to these conditions, industrially farmed algae or seaweed crops may benefit from these nutrients (e.g., Seghetta et al., 2016), while also stripping pollutants from waste streams (i.e., a form of low-energy/cost water treatment). Where crops are grown that produce large quantities of inedible plant material (e.g., tomatoes, potatoes, beans, etc.), these can be used as a food source for livestock or insect production. Certain insects may then be used to produce feed for fish and the aquaculture industry as well as for human consumption. Composted materials can be used in mushroom farming, and waste from aquaculture can be fed back into appropriate hydroponic systems. While food is the main output of such a circular system, composts, fertilizers and animal feed could all come as secondary external outputs of a system that would not require excessive inputs from the natural environment. External inputs such as wastewaters from sewage or urban streams may also provide crop systems with the nutrients required (e.g., Martin et al., 2019), though contamination of these streams with heavy metals may be difficult to address.
Figure 1. Theoretical Integrated System Controlled Environment Agriculture (ISCEA) approach to achieving a circular economy by recycling waste products generated in each system.
Where waste treatment is not considered or given priority in CEA systems, it can lead to issues. The large amounts of organic waste generated (rooting media and plant materials) can take up large amounts of space, and are often industrially composted (Aznar-Sánchez et al., 2020). While it is also possible to turn some waste plant material into animal feed, there are limitations on the economic feasibility of long-range transport of these materials to livestock farms, which are typically not integrated with large greenhouse facilities. The fate of inorganic waste is potentially more environmentally damaging than organic waste due to its longevity and poor economic return of recycling (Sayadi-Gmada et al., 2019). Large quantities of plastic waste material can be produced from industrial CEA systems that can overwhelm local recycling capabilities and end up in landfill (e.g., Castillo-Díaz et al., 2021). Rooting media (inert materials used to replicate soil in CEA systems) can be made of recycled organic or inorganic materials, and is discarded after each harvest. The fate of these materials varies, and while organic materials can be composted, inorganic materials like rock wool can be recycled for re-use or for materials such as loft insulation.
While adopting an ISCEA approach has the potential to drastically reduce waste, improving environmental efficiencies of nutrient use management is not seen as a priority in the commercial CEA industry as pollution (N and P) has no associated business cost beyond wasted inputs. Research in the CEA sector is largely dominated by system specific economic factors, such as productivity, energy use and staff requirements. While improving efficiencies here will provide real environmental benefits (e.g., improved land-use-efficiency and lower carbon emissions), these outcomes are largely achieved as a consequence of cost saving rather than deliberate attempts to improve sustainability of systems. With the exception of aquaponics systems (which are typically not economically feasible at large scales), there is a real lack of peer-reviewed research into circular economy approaches within CEA systems. To reach their full environmental potential, food producers of the future will need to consider how CEA systems carried out at large scale can integrate to improve both cost and nutrient efficiency. In order for ISCEA systems to be cost effective, dedicated research would be required to improve understanding of nutrient flows and how best to integrate crop types to improve maximum outputs. However, it is uncertain who might carry out this research as nutrient flows are variable to crop types, system designs and controlled environment conditions. Thus, this particular topic of research falls between the cracks of system developers and growers for which environmental performance is not currently a primary focus.
As well as addressing concerns with waste management on Earth, highly efficient ISCEA methods may be the only realistic way in which sustainable off-world food production may be feasible in the early colonization of inhospitable terrains. Environments such as that of the moon and Mars do not have the soil ecosystems required to break down organic materials, and conventional farming techniques would not be possible. Although off-planet colonization is still many years away from reality, we may already have much of the technology required to produce food in controlled environments, as demonstrated by the short-lived cotton plants grown on the moon by the Chang'E-4 craft (Jones et al., 2021). The main weakness in the long-term sustainability of this concept remains in the closed cycling of nutrients and elements between waste streams, replicating the finely balanced natural pathways of the ecosystem services on Earth. Should ISCEA be utilized effectively by commercial growers to feed the global population, the lessons learned will likely play an important role in any off-planet expansion in the centuries to come.
Sustainable CEA systems of the future have the potential to replace some conventional food production systems without having major impacts on availability of foods. For most of the EU and wealthier arid nations, the change from conventional to CEA has already happened with salad crops (e.g., lettuce, tomatoes, bell peppers, etc.) which are now predominantly grown in CEA systems in Europe. Here, there has been little objection to the crossover from conventional to CEA production as the majority of the public has not perceived any difference over time and is mostly unaware of the source of their food. Where questioned on the appeal of CEA systems, the environmental benefits are positively welcomed by the public; however, there is still a perception of unnatural and unsustainable practice surrounding CEA systems (Jürkenbeck et al., 2019). While the technological aspects such as vertical farming and robotics of CEA alters human perception to food production (e.g., Ares et al., 2021), in some cultures, the placement of large CEA facilities themselves will cause concern, particularly in communities where conventional farming is culturally dominant. Where communities face extreme food security issues and have limited options (e.g., drought and crop failure), CEA systems are popular (e.g., Besthorn, 2013).
While CEA systems are able to produce similar crops as those grown using conventional farming methods, meat production is more difficult. Global meat consumption increased by 58% between 2008 and 2018, predominantly driven by population growth, but also as a result of increasing preference and income growth (Whitnall, 2019). If this trend continues, the meat industry would need to increase production by an estimated 50–73% by 2050 to keep up with demand (Bonny et al., 2017). The future of meat (or protein) production in CEA systems that can challenge conventional methods comes in a variety of forms. While mushroom, fungal and algal feed stocks are already viable, it is mass-scale insect production and lab-grown meat that are seen as having the most realistic prospect in terms of competing with conventional livestock production methods in a more sustainable way (Alexander et al., 2017). By absorbing some of the future demand for meat products, these industries may provide a vital means by which to limit environmental damage.
In terms of economic and environmental sustainability, geographic location will be a major driver in the feasibility of CEA development. In areas with high biodiversity or pristine natural beauty, it does not make sense to build intensive CEA facilities, nor is there a need to do so. CEA facilities are best suited to areas where conventional productivity is low, and where sustainable energy is easily available and cheap. The use of geothermal energy to heat indoor agriculture in colder regions (e.g., Barbaresi et al., 2020; Sakai et al., 2022) or cooling in hotter regions (e.g., Al-Helal et al., 2022) can significantly reduce power requirements and subsequent GHG emissions associated with CEA systems. While water requirements are significantly lower than conventional agricultural needs, the impacts of large-scale facilities on soil moisture and natural water flow (i.e., soil impermeabilization and infiltration after rainfall) should still be considered to avoid destructive interference with hydrological dynamics. In the example of the Almería region in Spain (> 26,000 ha of glasshouse coverage, the largest concentration of greenhouses in the world), energy consumption is relatively small due to the natural temperature and sunlight available, which was reported as 1.5% of the total production costs by (Mendoza-Fernández et al. 2021). While the expansion of the Almería glasshouse farming sector initially led to over exploitation of groundwater, adoption of desalination and water recycling has improved aquifers in recent years (Mendoza-Fernández et al., 2021), showing that pre-empting and prioritizing environmental concerns in regards to CEA is key to creating sustainable food security in the future.
It is likely that CEA systems will continue to expand rapidly, predominantly in wealthier nations (especially those with arid climates), and in doing so, these nations may be the first to realize the environmental benefits of large-scale industrial food processing in controlled systems. This expansion is likely to accelerate in the future if CEA is recognized to play a positive role in reaching climate goals in these nations, which is becoming a time-sensitive political concern in itself. However, arguably it is the less developed nations that are more in need of the expansion of CEA systems and the stability of food production that it offers, especially those that will face the worst impacts of climate change this century (i.e., Sub-Saharan Africa). Here, while CEA is viable and in demand (e.g., Gumisiriza et al., 2020) there are many barriers to the expansion of systems that require expensive investment, skilled workers and access to renewable energy. While establishing CEA systems may be relatively expensive in these regions, arguably, local government and external investment may provide lasting solutions to tackle food security issues. While CEA systems of the future may allow for wealthier nations to improve the sustainability of their agricultural systems, they may be the only way to achieve food security in nations that will face the worst of climate change in the coming decades.
Time is a significant factor in preventing the worst of the environmental damage to come over the next few decades. The available industrial expertise and lack of regulation in the CEA industry are both barriers that will slow development on top of economic issues (Al-Kodmany, 2018). The greatest weakness of the CEA industry at this point in time is the lack of economically viable food products available to growers. While salad crops, some fruits, mushrooms, fish and insects are viable and are already produced in large quantities in commercially available systems, staple crops such as rice, maize and wheat are not considered viable. These three foods provide 60% of the world's food energy intake. While wheat (e.g., Asseng et al., 2020) and potatoes (e.g., Tunio et al., 2020) are possible to grow in CEA systems, the limitation is the cost of production, as is for lab-grown meat. This is a major limitation, specifically in poorer nations in which there are greater sensitivities to food prices than in wealthier nations where consumers can more readily absorb these costs. This is expected to change as technology progresses and costs are reduced, but significant investment in this research would be required, including technological advances, and likely significant genetic modification of crops to maximize crop production in CEA systems.
Further investment in renewable (or green) energy would also be required to expand CEA systems in a way that would allow environmental sustainability to be achieved in the future. While not all CEA systems require the use of additional energy, it is the vertical stacking and high productivity aspects of CEA systems that are most environmentally attractive when comparing with conventional farming methods. Thus, where staple crops are grown, there will likely be the need for large amounts of artificial lighting and extremely large energy requirements. As with the electrification of the transport sector (e.g., electric vehicles), the shift from conventional to CEA farming would require to some extent the electrification of the agricultural sector. This increase in energy consumption could be expected to be at least an order of magnitude larger than that required by the transport sector, due to the scale of energy required to grow crops. Expansion of sustainable agriculture through CEA systems would therefore be tied closely with the availability of green energy and the industry may need to consider integrating facility design with the capture of renewable (or carbon neutral) energy sources in the future (i.e., wind turbines, solar arrays, wave power, nuclear, etc.) This energy requirement is perhaps the greatest challenge facing the expansion of CEA at the scale required to achieve environmental sustainability at a global scale.
Currently, CEA systems have a wide array of advantages and disadvantages associated with their use, both in terms of economic and environmental sustainability. While CEA systems such as glasshouse production or vertical farming are expanding rapidly in competition with conventional farming for some food types (predominantly salad crops), the majority food production, primarily staple crops and meat, will remain economically unfeasible in these systems for the foreseeable future. While some environmental savings may be possible with current CEA systems (especially water saving and minimizing of pesticide use), the carbon footprint of many systems (construction and operations) is significantly higher than that of conventional systems, and availability of renewable energy is not sufficient to power systems in most regions (especially poorer nations) to counter this.
However, the true strength of CEA lies in the technological development of the systems that is likely to come in the next few decades. While we have already reached the threshold in which CEA systems can begin to improve the sustainability of food production via the use of renewable energy, it is in the development of economically sustainable mass production of staple crops in which the true potential will be realized. With climate change likely to disrupt conventional farming at a global scale, numerous regions will turn to CEA technologies to adapt to changing environmental conditions such as droughts and desertification. Eventually, CEA systems and specialized (e.g., GM) crops could be developed that will enable cheap and intensive production of staple foods. With access to plentiful renewable (carbon-neutral) energy and the appropriate systems in place, it is feasible that CEA systems of the future could largely negate most forms of environmental damage associated with conventional farming at a global scale (e.g., GHGs, deforestation, nitrogen, phosphorus, pesticide use, etc.).
To achieve the advances in sustainability that CEA potentially offers, future development should focus on integrating economic and environmental aspects of system design. As well as basic aspects such as energy and water use, quantitative assessments of nutrient flows in these systems are required, which may allow for advanced circular waste management. Integrating this research in the development stages of CEA systems now will lead to more sustainable food production in the future, that in time, could be expected to provide the industry with more economically efficient systems, lower operating costs, allowing more competitive food prices and developing a more positive image in the public domain.
NC primary author and manuscript lead. LF carried out primary literature review and assessment. BS contributed to text with global nutrient use and pollution expertise. JD contributed to text with greenhouse gas and nitrogen pollution expertise. DR contributed to text with global carbon and agricultural sustainability expertise. US contributed to text with expertise on agricultural pollution and sustainability. All authors listed have made a substantial, direct, and intellectual contribution to the work and approved it for publication.
The authors declare that the research was conducted in the absence of any commercial or financial relationships that could be construed as a potential conflict of interest.
All claims expressed in this article are solely those of the authors and do not necessarily represent those of their affiliated organizations, or those of the publisher, the editors and the reviewers. Any product that may be evaluated in this article, or claim that may be made by its manufacturer, is not guaranteed or endorsed by the publisher.
We acknowledge contribution from UKSCAPE Programme, funded by the Natural Environment Research Council as National Capability (award number NE/R016429/1).
Abusin, S. A. A., and Mandikiana, B. W. (2020). Towards sustainable food production systems in Qatar: assessment of the viability of aquaponics. Glob. Food Sec. 25, 100349. doi: 10.1016/j.gfs.2020.100349
Adams, S. R., Valdés, V. M., Langton, F. A., and Hamer, P. J. C. (2011). Reducing carbon emissions from greenhouse production through the use of temperature integration and alternative sources of heat. Acta Hortic. 95−101. doi: 10.17660/ActaHortic.2011.893.5
Al-Chalabi, M. (2015). Vertical farming: Skyscraper sustainability? Sustain. Cities Soc. 18, 74–77. doi: 10.1016/j.scs.2015.06.003
Alexander, P., Brown, C., Arneth, A., Dias, C., Finnigan, J., Moran, D., et al. (2017). Could consumption of insects, cultured meat or imitation meat reduce global agricultural land use? Glob. Food Sec. 15, 22–32. doi: 10.1016/j.gfs.2017.04.001
Al-Helal, I., Alsadon, A., Marey, S., Ibrahim, A., Shady, M., and Abdel-Ghany, A. (2022). Geothermal energy potential for cooling/heating greenhouses in hot arid regions. Atmosphere. 13, 105. doi: 10.3390/atmos13010105
Al-Kodmany, K. (2018). The Vertical Farm: A Review of Developments and Implications for the Vertical City. Buildings. 8, 24. doi: 10.3390/buildings8020024
Ares, G., Ha, B., and Jaeger, S. R. (2021). Consumer attitudes to vertical farming (indoor plant factory with artificial lighting) in China, Singapore, UK, and USA: A multi-method study. Food Res. Int. 150, 110811. doi: 10.1016/j.foodres.2021.110811
Asseng, S., Guarin, J. R., Raman, M., Monje, O., Kiss, G., Despommier, D. D., et al. (2020). Wheat yield potential in controlled-environment vertical farms. Proc. Natl. Acad. Sci. USA. 117, 19131–19135. doi: 10.1073/pnas.2002655117
Avgoustaki, D. D., and Xydis, G. (2020). How energy innovation in indoor vertical farming can improve food security, sustainability, and food safety? Advances in Food Security and Sustainability. 5, 1−51. doi: 10.1016/bs.af2s.2020.08.002
Aznar-Sánchez, J. A., Velasco-Muñoz, J. F., García-Arca, D., and López-Felices, B. (2020). Identification of opportunities for applying the circular economy to intensive agriculture in Almería (South-East Spain). Agronomy. 10, 1499. doi: 10.3390/agronomy10101499
Badiola, M., Basurko, O. C., Piedrahita, R., Hundley, P., and Mendiola, D. (2018). Energy use in recirculating aquaculture systems (RAS): a review. Aquac. Eng. 81, 57–70. doi: 10.1016/j.aquaeng.2018.03.003
Banerjee, C., and Adenaeuer, L. (2014). Up, up and away! the economics of vertical farming. JAS. 2, 40. doi: 10.5296/jas.v2i1.4526
Barbaresi, A., Maioli, V., Bovo, M., Tinti, F., Torreggiani, D., and Tassinari, P. (2020). Application of basket geothermal heat exchangers for sustainable greenhouse cultivation. Renew. Sust. Energ. Rev. 129, 109928. doi: 10.1016/j.rser.2020.109928
Barbosa, G., Gadelha, F., Kublik, N., Proctor, A., Reichelm, L., Weissinger, E., et al. (2015). Comparison of land, water, and energy requirements of lettuce grown using hydroponic vs. conventional agricultural methods. IJERPH. 12, 6879–6891. doi: 10.3390/ijerph120606879
Benke, K., and Tomkins, B. (2017). Future food-production systems: vertical farming and controlled-environment agriculture. Sustain.: Sci. Pract. Policy. 13, 13–26. doi: 10.1080/15487733.2017.1394054
Benton, T. G., Bieg, C., Harwatt, H., Pudasaini, R., and Wellesley, L. (2021). Food system impacts on biodiversity loss: Three levers for food system transformation in support of nature; Energy, Environment and Resources Programme. Available online at: https://www.chathamhouse.org/sites/default/files/2021-02/2021-02-03-food-system-biodiversity-loss-benton-et-al_0.pdf
Besthorn, F. H. (2013). Vertical farming: social work and sustainable urban agriculture in an age of global food crises. Aust. Soc. Work. 66, 187–203. doi: 10.1080/0312407X.2012.716448
Bonny, S. P. F., Gardner, G. E., Pethick, D. W., and Hocquette, J.-.F. (2017). Artificial meat and the future of the meat industry. Anim. Prod. Sci. 57, 2216. doi: 10.1071/AN17307
Campra, P., Garcia, M., Canton, Y., and Palacios-Orueta, A. (2008). Surface temperature cooling trends and negative radiative forcing due to land use change toward greenhouse farming in southeastern Spain. J. Geophys. Res. 113, D18109. doi: 10.1029/2008JD009912
Castillo-Díaz, F. J., Belmonte-Ureña, L. J., Camacho-Ferre, F., and Tello-Marquina, J. C. (2021). The Management of agriculture plastic waste in the framework of circular economy. Case of the almeria greenhouse (Spain). IJERPH. 18, 12042. doi: 10.3390/ijerph182212042
Da Silva, J. S., Paz, V., Soares, T. M., Almeida, W. F., and Fernandes, J. (2018). Production of lettuce with brackish water in NFT hydroponic system. SCA. 39, 947. doi: 10.5433/1679-0359.2018v39n3p947
Dal Ri, S., Favargiotti, S., and Albatici, R. (2020). The role of vertical farming in re-thinking and re-designing cities within a circular perspective. Tema. 6, 96–106. doi: 10.30682/tema0601i
Dalin, C., and Rodríguez-Iturbe, I. (2016). Environmental impacts of food trade via resource use and greenhouse gas emissions. Environ. Res. Lett. 11, 035012. doi: 10.1088/1748-9326/11/3/035012
de Anda, J., and Shear, J. H. (2017). Potential of vertical hydroponic agriculture in Mexico. Sustainability. 9, 140. doi: 10.3390/su9010140
Despommier, D. (2013). Farming up the city: the rise of urban vertical farms. Trends Biotechnol. 31, 388–389. doi: 10.1016/j.tibtech.2013.03.008
Dorr, E., Koegler, M., Gabrielle, B., and Aubry, C. (2021). Life cycle assessment of a circular, urban mushroom farm. J. Clean. Prod. 288, 125668. doi: 10.1016/j.jclepro.2020.125668
Ellingsen, E. C., and Despommier, D. D. (2008). The Vertical Farm-The origin of a 21st century Architectural Typology. CTBUH Journal 2008 Issue III
Engler, N., and Krarti, M. (2021). Review of energy efficiency in controlled environment agriculture. Renew. Sust. Energ. Rev. 141, 110786. doi: 10.1016/j.rser.2021.110786
Eyring, V., Gillett, N. P., Achuta Rao, K. M., Barimalala, R., Barreiro Parrillo, M., Bellouin, N., et al. (2021). “Human influence on the climate system.” In: Climate Change 2021: The Physical Science Basis. Contribution of Working Group I to the Sixth Assessment Report of the Intergovernmental Panel on Climate Change, eds V. Masson-Delmotte, P. Zhai, A. Pirani, S. L. Connors, C. Péan, S. Berger, et al. (Cambridge: Cambridge University Press. In Press).
Forchino, A. A., Gennotte, V., Maiolo, S., Brigolin, D., Mélard, C., and Pastres, R. (2018). Eco-designing aquaponics: a case study of an experimental production system in Belgium. Procedia CIRP. 69, 546–550. doi: 10.1016/j.procir.2017.11.064
Georgiou, S., Acha, S., Shah, N., and Markides, C. N. (2018). A generic tool for quantifying the energy requirements of glasshouse food production. J. Clean. Prod. 191, 384–399. doi: 10.1016/j.jclepro.2018.03.278
Goddek, S., Delaide, B., Mankasingh, U., Ragnarsdottir, K., Jijakli, H., and Thorarinsdottir, R. (2015). Challenges of Sustainable and Commercial Aquaponics. Sustainability. 7, 4199–4224. doi: 10.3390/su7044199
Goddek, S., Joyce, A., Kotzen, B., and Burnell, G. M, Eds., (2019). Aquaponics Food Production Systems. New York, NY: Springer International Publishing. doi: 10.1007/978-3-030-15943-6
Graamans, L., van den Dobbelsteen, A., Meinen, E., and Stanghellini, C. (2017). Plant factories; crop transpiration and energy balance. Agric. Syst. 153, 138–147. doi: 10.1016/j.agsy.2017.01.003
Gumisiriza, M. S., Ndakidemi, P. A., and Mbega, E. R. (2020). Memoir and farming structures under soil-less culture (hydroponic farming) and the applicability for Africa: a review. AG. 139–145. doi: 10.18805/ag.R-137
Guzman, S. D., Henspeter, D., Taylor, M., and Duan, S. (2021). Drone Pollination of Flowering Vegetation for Agricultural Applications. Volume 4: Advances in Aerospace Technology.
Halbert-Howard, A., Häfner, F., Karlowsky, S., Schwarz, D., and Krause, A. (2020). Evaluating recycling fertilizers for tomato cultivation in hydroponics, and their impact on greenhouse gas emissions. Environ Sci Pollut Res. 28, 59284–59303. doi: 10.1007/s11356-020-10461-4
Harris, Z. M., and Kountouris, Y. (2020). Vertical farming as a game changer for BECCS technology deployment. Sustainability. 12, 8193. doi: 10.3390/su12198193
Hu, Z., Lee, J. W., Chandran, K., Kim, S., and Khanal, S. K. (2012). Nitrous oxide (N2O) emission from aquaculture: a review. Environ. Sci. Technol. 46, 6470–6480. doi: 10.1021/es300110x
IPCC. (2019). Climate Change and Land: an IPCC special report on climate change, desertification, land degradation, sustainable land management, food security, and greenhouse gas fluxes in terrestrial ecosystems, Eds P. R. Shukla, J. Skea, E. Calvo Buendia, V. Masson-Delmotte, H.-O. Pörtner, D. C. Roberts, et al. (Cambridge: Cambridge University Press).
Jones, A., Patel, P., Waltz, E., and Bajema, N. (2021). The Latest Developments in Technology, Engineering, and Science: News 58, 6–13. IEEE Spectr. doi: 10.1109/MSPEC.2021.9563955
Jürkenbeck, K., Heumann, A., and Spiller, A. (2019). Sustainability matters: consumer acceptance of different vertical farming systems. Sustainability. 11, 4052. doi: 10.3390/su11154052
Kalantari, F., Tahir, O. M., Joni, R. A., and Fatemi, E. (2018). Opportunities and challenges in sustainability of vertical farming: a review. J. Landsc. Ecol. 11, 35–60. doi: 10.1515/jlecol-2017-0016
Khan, S., Purohit, A., and Vadsaria, N. (2020). Hydroponics: current and future state of the art in farming. J. Plant Nutr. 44, 1515–1538. doi: 10.1080/01904167.2020.1860217
Lauguico, S. C., Concepcion, R. S., Macasaet, D. D., Alejandrino, J. D., Bandala, A. A., and Dadios, E. P. (2019). Implementation of Inverse Kinematics for Crop-Harvesting Robotic Arm in Vertical Farming. 2019 IEEE International Conference on Cybernetics and Intelligent Systems (CIS) and IEEE Conference on Robotics, Automation and Mechatronics (RAM). doi: 10.1109/CIS-RAM47153.2019.9095774
Lynch, J., and Pierrehumbert, R. (2019). Climate impacts of cultured meat and beef cattle. Front. Sustain. Food Syst. 3, 5. doi: 10.3389/fsufs.2019.00005
Malone, T. C., and Newton, A. (2020). The globalization of cultural eutrophication in the coastal ocean: causes and consequences. Front. Mar. Sci. 7. doi: 10.3389/fmars.2020.00670
Martin, M., and Molin, E. (2019). Environmental assessment of an urban vertical hydroponic farming system in Sweden. Sustainability. 11, 4124. doi: 10.3390/su11154124
Martin, M., Poulikidou, S., and Molin, E. (2019). Exploring the environmental performance of urban symbiosis for vertical hydroponic farming. Sustainability. 11, 6724. doi: 10.3390/su11236724
Mattson, N., Sanderson, J., Lamb, E., Eshenaur, B., Lobdell, E., Catlin, N., et al. (2017). Effective aphid management in greenhouse crops by optimizing biological control and nutrient inputs. New York State Integrated Pest Management Program. Available online at: https://ecommons.cornell.edu/bitstream/handle/1813/57156/2017mattson.pdf?sequence=1andisAllowed=y
Mendoza-Fernández, A. J., Peña-Fernández, A., Molina, L., and Aguilera, P. A. (2021). The role of technology in greenhouse agriculture: towards a sustainable intensification in campo de dalías (Almería, Spain). Agronomy. 11, 101. doi: 10.3390/agronomy11010101
Michael, G. W., Tay, F. S., and Then, Y. L. (2021). Development of automated monitoring system for hydroponics vertical farming. J. Phys.: Conf. Ser 1844, 012024. doi: 10.1088/1742-6596/1844/1/012024
O'Connor, N., and Mehta, K. (2016). Modes of greenhouse water savings. Procedia Engineering. 159, 259–266. doi: 10.1016/j.proeng.2016.08.172
Ohi, N., Lassak, K., Watson, R., Strader, J., Du, Y., Yang, C., et al. (2018). Design of an autonomous precision pollination robot. 2018 IEEE/RSJ International Conference on Intelligent Robots and Systems (IROS). Madrid: IEEE. doi: 10.1109/IROS.2018.8594444
Oonincx, D. G. A. B., van Itterbeeck, J., Heetkamp, M. J. W., van den Brand, H., van Loon, J. J. A., and van Huis, A. (2010). An exploration on greenhouse gas and ammonia production by insect species suitable for animal or human consumption. PLoS ONE. 5, e14445. doi: 10.1371/journal.pone.0014445
Roberts, J. M., Bruce, T. J. A., Monaghan, J. M., Pope, T. W., Leather, S. R., and Beacham, A. M. (2020). Vertical farming systems bring new considerations for pest and disease management. Ann Appl Biol. 176, 226–232. doi: 10.1111/aab.12587
Roser, M. (2019). Our world in data: Yields and land use in agriculture [WWW Document]. Available online at: https://ourworldindata.org/yields-and-land-use-in-agriculture#breakdown-of-global-land-area-today
Rufí-Salís, M., Petit-Boix, A., Villalba, G., Sanjuan-Delmás, D., Parada, F., Ercilla-Montserrat, M., et al. (2020). Recirculating water and nutrients in urban agriculture: An opportunity towards environmental sustainability and water use efficiency? J. Clean. Prod. 261, 121213. doi: 10.1016/j.jclepro.2020.121213
Saad, M. H. M., Hamdan, N. M., and Sarker, M. R. (2021). State of the art of urban smart vertical farming automation system: advanced topologies, issues and recommendations. Electronics. 10, 1422. doi: 10.3390/electronics10121422
Sakai, P., Hoffmann, F., and Carver, B. (2022). Using geothermal mine water energy for food production in Leeds. University of Leeds.
Sarkar, A., and Majumder, M. (2018). Economic of a six-story stacked protected farm structure. Environ. Dev. Sustain. 21, 1075–1089. doi: 10.1007/s10668-018-0088-0
Sayadi-Gmada, S., Rodríguez-Pleguezuelo, C. R., Rojas-Serrano, F., Parra-López, C., Parra-Gómez, S., García-García, M., et al. (2019). Inorganic waste management in greenhouse agriculture in almeria (SE Spain): towards a circular system in intensive horticultural production. Sustainability. 11, 3782. doi: 10.3390/su11143782
Seghetta, M., Tørring, D., Bruhn, A., and Thomsen, M. (2016). Bioextraction potential of seaweed in Denmark—an instrument for circular nutrient management. Sci. Total Environ. 563–564, 513–529. doi: 10.1016/j.scitotenv.2016.04.010
Shafahi, M., and Woolston, D. (2014). Aquaponics: A Sustainable Food Production System. Volume 3: Biomedical and Biotechnology Engineering. doi: 10.1115/IMECE2014-39441
Sippel, S. R. (2015). Food security or commercial business? gulf state investments in australian agriculture. J. Peasant Stud. 42, 981–1001. doi: 10.1080/03066150.2014.990448
Song, S., Hou, Y., Lim, R. B. H., Gaw, L. Y. F., Richards, D. R., and Tan, H. T. W. (2022). Comparison of vegetable production, resource-use efficiency and environmental performance of high-technology and conventional farming systems for urban agriculture in the tropical city of Singapore. Sci. Total Environ. 807, 150621. doi: 10.1016/j.scitotenv.2021.150621
Specht, K., Zoll, F., Schümann, H., Bela, J., Kachel, J., and Robischon, M. (2019). How will we eat and produce in the cities of the future? from edible insects to vertical farming—a study on the perception and acceptability of new approaches. Sustainability. 11, 4315. doi: 10.3390/su11164315
Sutton, M. A., Bleeker, A., Howard, C. M., Bekunda, M., Grizzetti, B., Vries, d. e., et al. (2013). Our Nutrient World: The challenge to produce more food and energy with less pollution. Global Overview of Nutrient Management. Centre for Ecology and Hydrology, Edinburgh on behalf of the Global Partnership on Nutrient Management and the International Nitrogen Initiative.
Teo, Y. L., and Go, Y. I. (2021). Techno-economic-environmental analysis of solar/hybrid/storage for vertical farming system: a case study, Malaysia. Renew. Energy Focus. 37, 50–67. doi: 10.1016/j.ref.2021.02.005
Tunio, M. H., Gao, J., Shaikh, S. A., Lakhiar, I. A., Qureshi, W. A., Solangi, K. A., et al. (2020). Potato production in aeroponics: an emerging food growing system in sustainable agriculture forfood security. Chil. J. Agric. Res. 80, 118–132. doi: 10.4067/S0718-58392020000100118
Tuomisto, H. L. (2019). Vertical farming and cultured meat: immature technologies for urgent problems. One Earth. 1, 275–277. doi: 10.1016/j.oneear.2019.10.024
van Huis, A., and Oonincx, D. G. A. B. (2017). The environmental sustainability of insects as food and feed. A review. Agron. Sustain. Dev. 37, 421–451. doi: 10.1007/s13593-017-0452-8
Velthuis, H. H. W., and van Doorn, A. (2006). A century of advances in bumblebee domestication and the economic and environmental aspects of its commercialization for pollination. Apidologie. 37, 421–451. doi: 10.1051/apido:2006019
Wade, C. M., Austin, K. G., Cajka, J., Lapidus, D., Everett, K. H., Galperin, D., et al. (2020). What is threatening forests in protected areas? a global assessment of deforestation in protected areas, 2001–2018. Forests. 11, 539. doi: 10.3390/f11050539
Wang, J. (2021). The sprouting farms: you are what you grow. Humanities. 10, 27. doi: 10.3390/h10010027
Whitnall, T. (2019). Global trends in meat consumption. Agric. Commod. 9, 96–99. doi: 10.3316/informit.309517990386547
Wilson, D. P., and Finlay, A. R. (1995). Hydroponic system for the production of all year round chrysanthemums. Acta Hortic. 401, 185−192. doi: 10.17660/ActaHortic.1995.401.22
Woertz, E. (2020). Wither the self-sufficiency illusion? food security in Arab Gulf States and the impact of COVID-19. Food Sec. 12, 757–760. doi: 10.1007/s12571-020-01081-4
Wong, C. E., Teo, Z. W. N., Shen, L., and Yu, H. (2020). Seeing the lights for leafy greens in indoor vertical farming. Trends Food Sci. Technol. 106, 48–63. doi: 10.1016/j.tifs.2020.09.031
World Bank. (2020). Water Data. World Development Indicators. The World Bank Group. Available online at: https://wbwaterdata.org/
Keywords: agriculture, hydroponics, greenhouse gases, climate change, vertical farming
Citation: Cowan N, Ferrier L, Spears B, Drewer J, Reay D and Skiba U (2022) CEA Systems: the Means to Achieve Future Food Security and Environmental Sustainability? Front. Sustain. Food Syst. 6:891256. doi: 10.3389/fsufs.2022.891256
Received: 07 March 2022; Accepted: 09 May 2022;
Published: 15 June 2022.
Edited by:
Dibyendu Chatterjee, National Rice Research Institute (ICAR), IndiaReviewed by:
Joanna Marie Cloy, Scotland's Rural College, United KingdomCopyright © 2022 Cowan, Ferrier, Spears, Drewer, Reay and Skiba. This is an open-access article distributed under the terms of the Creative Commons Attribution License (CC BY). The use, distribution or reproduction in other forums is permitted, provided the original author(s) and the copyright owner(s) are credited and that the original publication in this journal is cited, in accordance with accepted academic practice. No use, distribution or reproduction is permitted which does not comply with these terms.
*Correspondence: Nicholas Cowan, bmljd2FuMTFAY2VoLmFjLnVr
Disclaimer: All claims expressed in this article are solely those of the authors and do not necessarily represent those of their affiliated organizations, or those of the publisher, the editors and the reviewers. Any product that may be evaluated in this article or claim that may be made by its manufacturer is not guaranteed or endorsed by the publisher.
Research integrity at Frontiers
Learn more about the work of our research integrity team to safeguard the quality of each article we publish.