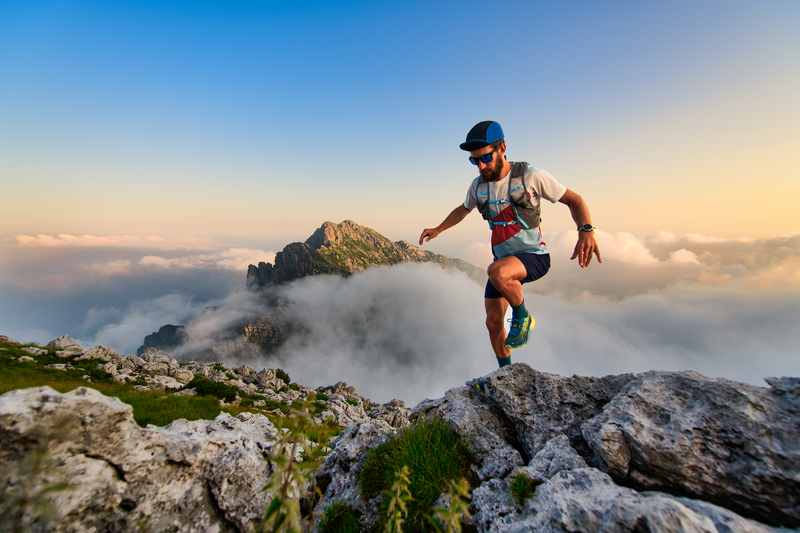
95% of researchers rate our articles as excellent or good
Learn more about the work of our research integrity team to safeguard the quality of each article we publish.
Find out more
ORIGINAL RESEARCH article
Front. Sustain. Food Syst. , 24 May 2022
Sec. Water-Smart Food Production
Volume 6 - 2022 | https://doi.org/10.3389/fsufs.2022.889830
This article is part of the Research Topic The Performance of Smallholder Irrigation and its Potential to Contribute to Food Security and Sustainability in Africa View all 4 articles
With climate change, maize production is becoming more constrained by limited water availability especially in rainfed production systems. Climate Smart Agriculture (CSA) practices have potential to enhance water availability and water use efficiency in rainfed production systems, but their efficiencies have not been adequately investigated. The study evaluated the performance of permanent planting basins (PPB), mulching (M), and halfmoon pits (HM) on soil moisture storage, maize yield, and water use efficiency in a maize cropping system for the sub-humid areas of Uganda for three cropping seasons in Albert region. The control treatment consisted of bare soil as the existing conventional farming practice without any CSA practice. Maize growth parameters and soil moisture storage were monitored and evaluated in each cropping season and CSA treatment. The maize yield, water use efficiency, and evapotranspiration (ET), were determined in each CSA treatment. Results showed that CSA practices significantly increased (P < 0.05) total soil water storage (1–12%) than the control treatment. It was also noted that; the use of M, PPB, and HM increased the water use efficiency by 9 – 68% and 8 – 66% of grain yield compared to the control in the three growing seasons. Our results indicate that even under unreliable and limited precipitation in sub-humid regions, the studied CSA practices indicate a high possibility to increase maize productivity than conventional farming practices (control). These findings are critical as climate change continues to affect maize productivity in rainfed farming systems where there limited supplemental water alternative sources for smallholder farmers. The adoption of CSA practices will enhance the resilience of maize production in sub-humid regions.
Globally, water stress arising from unreliable rainfall and rising temperatures is a major constraint on agricultural production (Rockström et al., 2007; Bhattacharya, 2019; Zizinga et al., 2022). Sub-humid zones are considered dryland farming regions, occupying 27–41% of the earth's land surface area and produce food for a third of world's population (Feng and Fu, 2013). Agriculture production in sub-humid regions is mostly rainfed, yet the adoption of irrigation is still low (Tilman et al., 2011; Wheeler and Von Braun, 2013 and Xie et al., 2018).
Recent studies have predicted decline in rainfall over East Africa (Souverijns et al., 2016; Ongoma et al., 2018; Wainwright et al., 2019) and 5.8°C increase in temperatures for Uganda (Epule et al., 2017). In Uganda, most smallholder farmers (76%) rely on rainfed agriculture that contributes 24% of gross domestic product Uganda Bureau of Statistics, 2019; World Bank, 2019). Given the ever-increasing droughts, extremely low and erratic rainfall with absence of effective adaptation and mitigation strategies, Uganda's climate change adaptation efforts remain a challenge. This is likely to affect agricultural production in the region, especially maize (Zea mays L.) (Gornall et al., 2010; Zizinga et al., 2022), and lead to food insecurity.
Climate smart agriculture (CSA) practices have been recommended as an effective tool for increasing and sustaining agriculture productivity in rainfed production systems, amidst climate change (Lipper et al., 2014; Tambo and Kirui, 2021). Climate smart agriculture (CSA) practices refers to farm management technologies which sustainably increases productivity, resilience, reduces greenhouse gases to enhance the achievement of national food security and development goals (FAO, 2016). It also contributes to resource use efficiency and environmental conservation in the context of low and erratic rainfall and offers alternatives to mitigate greenhouse gases (FAO, 2013; Nagargade et al., 2017).
Mulching, halfmoon pits and permanent planting basins have been used across the tropics as climatic change adaptation and mitigation strategies to increase soil moisture storage in rainfed maize production systems (Biazin et al., 2012; Partey et al., 2018). In southern Africa, adoption of permanent planting basins (PPB) increased water capture and storage, boosted maize yield and enhanced resilience to drought compared to conventional tillage practices (Twomlow and Bruneau, 2000; Mwenge Kahinda et al., 2009).
In Uganda, CSA practices such as mulching with straw dry grass, digging various basins and pits to harvest rain water (permanent planting basins and halfmoon pits) are being promoted to increase the resilience of cropping systems through improved soil and water conservation (FAO, 2013; MAAIF, 2017; Zizinga et al., 2022). However, the adoption of CSA practices in Uganda is still low, majorly due to lack of adequate research to generate the information necessary for integration into existing farming practices and extension services to transfer research products and innovations to farmers. For example, quantitative scientific data on the effects of mulching, halfmoon pits and permanent planting basins on soil moisture storage, maize growth, yield and water use efficiency is still lacking, yet crucial for adoption and implementation. In addition, based on the sole reliance of natural rainfall for agricultural production in Uganda, the production systems are prone to the unprecedented shifts in rainfall seasons which has adversely affected the critical stages of crop production due to water stress. Therefore, experiments to evaluate the performance of the selected CSA practices on maize production in a sub-humid climate of Uganda were conducted to bridge this gap. Specifically, the objectives of this study are; (1) assessing the effect of the CSA practices (i.e., PPB, M, and HM) on soil water storage and (2) analyzing the effect of CSA on maize growth, yield and water use efficiency, as compared with the conventional farming practices.
The experimental site was located in Bulindi Zonal Agriculture Research and Development Institute (1° 00′-2° 00′ N and 30° 30′-31°45′ E), the western Graben of Albert region, a high altitude region in Uganda (Wortmann and Eledu, 1999; Kikoyo and Nobert, 2016) (Figure 1). The annual rainfall received in the study area ranges between 800 and 1,300 mm and highly variable across the production seasons. The altitude of the study area ranges between 621 and 1,158 m above sea level with the mean annual temperature of 29°C.
Figure 1. Location of the study area, Bulindi station in gray color were the experiment was conducted. The red color illustrate a catchment area and river Kiha of Uganda.
During the study period, the weather station was installed to record weather data (rainfall and temperature). The rainfall received was 529, 416, and 405 mm for seasons 1, 2, and 3, respectively, while average temperatures of 21.5, 24.4, and 20.9°C were recorded during the same seasons (Figure 2). The site was characterized at the beginning of the experiment as indicated in Table 1. The soil type in the study area is Acric Ferralsols characterized by predominantly clayey texture in subsurface according to FAO soil classification (Legrain et al., 2018). The soil also has an impervious layer between 40 and 50 cm. Therefore, the maize roots could not exceed 40 cm. Consequently, this was considered as the rooting depth during experiments and the maximum length of the access tubes was chosen to be 40 cm. The experiments were established in a uniform sloping area with a slope of <5%.
Figure 2. Daily precipitation and temperature during the three maize growing seasons (S1, S2, S3) and (a), (b) are off seasons at the Bulindi Zonal Agriculture Research Development Research Institute station.
The experiments were setup in 2019–2020 following the FAO CSA manual (FAO, 2013), and consisted of four treatments that included three CSA practices namely halfmoon pits (HM), permanent planting basins (PPB), straw mulch of 2 cm thickness (M), and the control (C) treatment. The C plots comprised a bare surface field, which is a typical common conventional cultivation practice in the study area without any soil water management technique (Figure 3A). The previous seasons before the experiment at the study site had been plowed using a tractor. The HM treatment was established by digging six halfmoon shaped pits measuring 30 cm deep, 50 cm wide and 100 cm circumference using a hand hoe at a spacing of 30 cm to capture rainwater (Figure 3B).
Figure 3. Schematic illustration of treatments of rainfed maize production at the experimental station; (A) control, (B) halfmoon pits, (C) permanent planting basin 30 cm deep, and (D) mulch 2 cm thick.
The PPB practice involved excavation of circular pits of 15 cm diameter at depths of 30 cm in a plot. Both PPB and HM were established 1 day before planting (Figure 3C). For mulch treatment, the soil in each plot was covered with dry grass materials of 2 cm thickness (Figure 3D), and this was applied at planting.
The completely randomized block design (CRBD) experiment was set up with eight replicates per treatment (Figures 3A–D). Maize (Longe 9H variety) which is tolerant to moisture stress was used as a test crop (MAAIF, 2009).
Maize planting was done on March 31st, 2019 (first season), October 5th, 2019 (second season) and March 17th, 2020 (third season) at spacing of 75 × 30 cm. Plots of 5 × 5 m with borders of 1 m between plots and 2 m between blocks were used in this experiment. Thinning was done at 2 weeks after planting to maintain one plant per hill, 111 plants per plot and 44, 444 plants per hectare for each treatment replicate. Prior to planting, soil properties were determined (Table 1). Based on the low values of available phosphorus (Av. P) and potassium (K), the plots were amended to cater for crop nutrient requirements and soil homogeneity, with a blanket basal application of di-ammonium phosphate (60 kg P ha−1) and muriate of potash (60 kg ha−1). At 8 weeks after planting, urea fertilizer was applied at a blanket rate of 90 kg N ha−1. After the first season, the soil in CSA treated plots was not tilled for the consecutive growing seasons, therefore, land preparation during season 2 and 3 was by slashing. In comparison however, land preparation in the control treated plots (conventional practice) was done by hand hoeing.
Above ground biomass was determined at vegetative (44 days after sowing), tasseling (59 days after sowing), silking (87 days after sowing) and maturity (140 days after sowing) stages using four randomly selected plants from the two outside rows and this was done to retain enough plants for yield determination across the sampling growth stages. The selected plants were cut and their fresh weight measured. Maize yield was determined at maturity stage from a subplot of 2 × 2 m. The maize shoot, stovers, and grain samples were weighed for each plot in the field to obtain fresh weight and thereafter packed and taken to Makerere University laboratory. The samples were oven dried at 60°C for 48 h up to constant dry weight and expressed as Kg ha−1.
To determine soil moisture within each maize growing season, soil water content was measured at 0–10 cm, 10–20 cm, 20–30 cm, and 30–40 cm, using the principle of Frequency Domain Reflectometry (FDR) moisture probe-type PR2/4 (Delta-T Devices Ltd., 2006). The maximum length of the access tubes was 40 cm which was taken as the maximum rooting depth with 10 cm increment intervals used in the measurement of soil moisture content. Soil moisture was measured using 26 access tubes installed randomly at the start of the experiment in each treatment plot. In each replicate of the CSA practice and control treatment, one access tube was installed to measure soil moisture content. For control treatment plots, a total of five (access tubes were installed, for HM eight access tubes, seven for PPB and six for the mulched plots. For data analysis, soil moisture data from five access tubes were considered for each treatment to ensure uniformity with the control treatment.
Volumetric soil moisture readings were manually recorded daily along the four soil depths with a handheld moisture meter connected to a profile probe sensor. Seasonal evapotranspiration (ET, mm) for each CSA treatment was determined based on soil water budget (Bu et al., 2013; Lin et al., 2016; Equation 1):
Where, P = total precipitation (mm) and ΔSWS = difference in soil moisture storage (mm) between planting and harvesting stages. The water-use efficiency (WUE) was calculated by dividing grain yield (kg ha−1) with total ET (mm) over the growing seasons.
Soil water storage (mm) was determined using Equation 2.
Where,
SWS is soil water storage,
is volumetric available soil water content at a given depth (mm cm−1),
L is the rooting depth (cm).
The effect of CSA treatments on the selected soil properties were assessed at the end of the third season. At harvesting, 128 disturbed composite soil samples (32 from each treatment) were randomly collected using a soil auger for soil texture and soil organic carbon (SOC) determination. In each plot, four (4) soil samples were collected, one (1) sample from each soil depth (0–10 cm, 10–20 cm, 20–30 cm, and 30–40 cm). This was mixed to make a composite sample through quarter sampling technique. Soil texture was analyzed in the laboratory using the hydrometer method while for SOC, the wet oxidation method of Blackley-Walkley (Okalebo et al., 2002) was applied and thereafter converted into soil organic matter (SOM) using a factor of 1.724.
Conversely, a total of 128 undisturbed core samples (100 cm3; 32 from each treatment) were collected using a core method to determine soil bulk density (BD) and saturated hydraulic conductivity (Ksat). The Ksat was analyzed using a laboratory water permeameter with the constant head method (Eijkelkamp Soil Water, 2017).
Data analysis was done using R statistical tool version 3.6.0 (R Core Team, 2019). Analysis of variance was performed on soil moisture using a linear mixed-effect model with “lmer” function from the package “lme4”. The CSA treatments, soil depth and the time (days after sowing) factor were maintained as fixed effects and the replication was a random effect. The CSA practices on soil moisture content and storage, yield, water use efficiency and maize grain yield was analyzed using one-way analysis of variances. The “lsmeans” package, was used to compute means while post-hoc comparisons were performed using the Tukey's HSD for separation of significant means at P < 0.05 and this was achieved using “cld” function from the “multicompView” package.
The different CSA treatments caused significant differences in maize biomass accumulation in the three growing seasons at the various maize growth stages. However, the interactions of growth stages and CSA treatments and that of growth stages, CSA treatments and seasons were not (P = 0.990; Table 2).
Overall, mulch consistently produced the highest biomass (16,389 kg ha−1, 31, 801 kg ha−1, and 20,293 kg ha−1) followed by PPB (15,885 kg ha−1, 19,810 kg ha−1, and 20,293 kg ha−1) and HM (15,002 kg ha−1, 29,283 kg ha−1, and 18,569 kg ha−1) in season one, two and three, respectively, at all the growth stages (Figure 4).
Figure 4. Biomass for the CSA practices in the vegetative (44 days after sowing), tasseling (59 days after sowing), silking (87 days after sowing) and maturity (140 days after sowing) growth stages for seasons 1 (A), 2 (B), and 3 (C). C, control; M, mulch 2 cm thickness; PPB, permanent planting basins; HM, halfmoon pits; M, mulch 2 cm thickness. The vertical bars represent ± the standard error of the mean and (P < 0.05). Means ± standard error followed by the same letters are not significantly different (p < 0.05).
The application of mulch increased biomass by 18%, 62%, 25% and HM treatment by 8%, 49%, and 14% in season one, two and three, respectively, compared to control treatment. While, the PPB treatment increased biomass by 1–14% in seasons one and two. The M treatment biomass was 3–61% higher from PPB and HM in the three growing seasons (Figure 4). Also, drastic loss of biomass in season three was attributed to crop damage at maturity stage and low rainfall (Figure 4C).
At the end of third season, soil properties showed high variability across the CSA practices and soil depths within the different CSA practices (Figure 5). At all soil depths, the CSA practices did not significantly (P > 0.05) influence Ksat. At soil depths of 0–10 cm and 10–20 cm, all CSA practices had higher soil organic matter (P < 0.05) (SOM) than control (C) treatment. At the same soil depths, the permanent planting basins (PPB) had significantly higher SOM than mulch (M). For the soil depth 20–30 cm, PPB and half-moon pits (HM) had significantly higher SOM than M and C whereas, CSA practices significantly enhanced SOM better than the control at 30–40 cm (Figure 5B). At soil depth, 0–10 cm, PPB had significantly lower bulk density than other treatments. However, bulk density did not vary significantly among treatments at 10–20 cm and 20–30 cm due to higher effects of non-mechanised tillage in the plots. Also, at 30–40 cm, PPB had significantly lower bulk density than other treatments while control had significant difference with mulch (Figure 5C).
Figure 5. Variability in selected soil properties across the CSA practices and soil depths. (A) Ksat-saturated hydraulic conductivity, (B) organic matter, and (C) Bulk density. C, control; HM, halfmoon pits; PPB, permanent planting basins; M, mulch 2 cm thickness. Different letters on each panel of the graph illustrate significant differences across soil depth (P < 0.05).
The rainfall concentration in season one also enhanced soil water storage compared to seasons two and three. The soil water storage varied significantly in 0–40 cm soil layers due to CSA treatments, seasons, and growth stages. However, the interaction effect of growth stages, season and CSA treatments (P = 0.660; Table 3).
The changes in water during the maize growth affect yield significantly. In the three growing seasons, soil water storage in 0–40 cm soil layers caused significant (P < 0.001) differences in seasons for different treatments (Figure 6). The application of CSA practices increased soil water storage by 4–12%, 2–5%, and 1–6% in seasons one, two, and three, respectively relative to control treatment. The highest soil water storage (66.2 mm) was produced under HM in season one, mulch in season two (77.1 mm), and PPB (78.1 mm) for season three (Figures 6A–C). Soil water storage from mulch, permanent planting basins and halfmoon pits was higher in all three growing seasons than in the control treatment. In season one, the rainfall was higher by 113 mm than in season two and 14 mm for season three (Figure 2). The increase in rainfall affected soil water storage and this was observed after sowing in seasons one and three which led to the highest soil water storage and retention in the CSA practices (Figure 6A).
Figure 6. Total soil moisture storage in different CSA treatments for seasons 1 (A), 2 (B), and 3 (C). C, control; M, mulch 2 cm thickness; PPB, permanent planting basins; HM, halfmoon pits; M, mulch 2 cm thickness. The vertical bars represent the ± the standard error of the mean and (P < 0.05).
CSA practices caused significant differences (P < 0.05) in soil moisture content at different soil depths in maize growth period in all the three growing seasons (Figure 7). The dynamic changes in soil moisture content was also affected by rainfall (Figure 7). The soil moisture trend was different across the soil depth, CSA practices and the maize growth stages. There was both increasing and decreasing trends in all CSA practices and the control treatments across the respective soil depths and seasons. At all depths, the soil moisture content increased from planting to 44 days after sowing (Vegetative stage) and at 87 days after sowing (Silking stage) with the control treatment having the lowest soil moisture content across seasons and soil depths (Figure 7).
Figure 7. Seasons 1 (A–D), 2 (E–H), and 3 (I–L) effects of CSA practices on soil moisture content at soil depths of 0–10 cm, 10–20 cm, 20–30 cm and 30–40 cm during maize growth, respectively. C, control; M, mulch 2 cm thickness; PPB, permanent planting basins; HM, halfmoon pits; M, mulch 2 cm thickness. The vertical bars represent the ± the standard error of the mean and (P < 0.05).
At soil depth of 0–10 cm, HM soil moisture content was relatively higher by 21–74% than other treatments from the vegetative to silking stage in season one (Figure 7A). Mulch also increased soil moisture content by 9–47% and PPB from 4 to 25%. At 0–20 cm soil depth, only PPB increased soil moisture content in all maize growth stages (Figure 7B). In the 0–30 cm soil depth, mulch increased moisture by 8–18% from planting to maturity stage (Figure 5C) and at 40 cm depth, it was 7–14% and 9–24% for half-moon pits (Figure 7D).
In season two, there was fluctuation of soil moisture content from planting to maturity in the M, PPB and HM across all the growth stages at the 10 cm soil depth. The soil moisture increase was observed at maturity in mulch by 33–48% (Figure 7E). At 20 cm depth, there were significant differences at 44 and 59 days after sowing in all the treatments due to reduction in the rainfall and soil moisture did not increase above 5% (Figure 7F). While at 30 and 40 cm soil depth, the differences are also observed in the CSA practices after 59 and 87 days of sowing (Figure 7G). At the 30 cm depth, soil moisture increased in mulch by 1–18%, 1–23% for PPB and HM to 16% (Figure 7H).
Furthermore, season three soil moisture content increased higher (78%) at 30 cm soil depth compared to 10 cm (18%), 20 cm (32%), and 40 cm (8%) soil depths in all the CSA practices (Figures 7I–L). In the whole maize growing period, the soil moisture content in the CSA practices was higher than the control treatment with M, HM and PPB having the highest soil moisture content across the maize growth stages.
CSA practices caused significant differences in maize grain yield and water use efficiency in all seasons (Table 4). The maize grain yield in CSA practices was significantly (P < 0.05) higher than control treatment by 28–66%, 38–57%, and 8–56% in seasons one, two and three, respectively (Table 2). The PPB and M treatments produced significantly higher (P < 0.05) grain yield than HM treatment by 30, 14, 44% for PPB and 18, 1, 44% for M in seasons one, two and three, respectively. Similarly, the water use efficiency (WUE) increase was higher for PPB, M and HM by 68, 54, 30% for season one, 57, 40, 38% for season two while season three M had the highest WUE of 58, 57% for PPB and HM by 9%.
The PPB and M treatments also enhanced water use efficiency better than HM in all the maize growing seasons (Table 4). Use of PPB, M and HM significantly reduced evapotranspiration in all the three maize growing seasons (Table 4).
The improvement in soil moisture storage, soil organic matter levels, bulk density and water infiltration achieved using the various CSA practices indicate the potential for improving soil moisture retention and crop productivity in rainfed systems. The significantly higher soil organic matter under PPB practice for the topsoil (0–20 cm) could be related to root biomass and crop residues within the permanent planting basins. This is related to findings of Campbell et al. (2001) who report higher soil organic matter levels in plots where residues were retained under minimum tillage. Similarly, soil organic matter increase is a result of microbial decomposition from crop residues as reported in maize fields treated with permanent planting basins and mulching practices, which is in agreement with previous studies (Thierfelder and Wall, 2012; Mupangwa et al., 2013; Mloza-Banda et al., 2014). The retention of crop residues in intermediate seasons in mulch and permanent planting basins in the present study is a major attribute for improvements in soil properties studied.
The higher soil organic matter in CSA plots compared to the control treatments could be the benefits of minimum tillage and crop residual decomposition which improved the soil structure. Studies earlier conducted (Sharma et al., 2011; Lin et al., 2016; Dixit et al., 2019), found a significant reduction in soil moisture, biological diversity and microbial activity in topsoil layers and increased rates of organic matter oxidation in soils under conventional practices. The topsoil organic matter levels of >4% achieved in CSA treated plots are within the values recommended for maize production in low-input tropical soils of sub-humid climates (Okalebo et al., 2002).
Low soil bulky density in the permanent planting basins could be attributed to high soil organic matter in topsoil layer (0–20 cm) as discussed in the past studies (Nyamadzawo et al., 2008), the relationship with soil organic matter and bulk density existed in the field trials. However, the slight increases in soil bulk densities at depths beyond 20 cm could be attributed to compaction arising a from the previous field site management of plowing with a method of land preparation (Thomas et al., 2007; Verhulst et al., 2010; Marumbi et al., 2020). Although, results from this present study agree with Moreira et al. (2016) who reported improvements in bulk density and saturated hydraulic conductivity of maize fields in conservation farming for subtropical soils in Brazil.
The absence of soil water management techniques limit maize productivity in most regions of tropics which are solely rainfed. This situation is exacerbated by low rainfall in prolonged droughts that result into soil water depletion (Fan et al., 2016). The higher moisture in 20–30 cm soil depth for mulch (M) and permanent planting basin (PPB) treatments compared to HM and C practices may be attributed to higher water storage arising from improved water infiltration and reduction in surface water runoff since the study area was relatively flat. This also directly relates to the rainfall variability in the maize growing seasons (Figure 2), the higher rain received in season one, the higher soil water storage compared to season two and three in the present study. Mulching reduces soil evaporation by protecting the soil surface from direct solar heat and preventing surface runoff while increasing water infiltration (Bu et al., 2013; Wang et al., 2019). Permanent planting basins (PPB) and halfmoon (HM) pits capture runoff and increase water infiltration, while the control treatment consisted of bare soil surfaces that was susceptible to soil erosion and evaporation. The soil surface is covered in M and low tillage for HM and PPB corroborates with previous findings (Twomlow and Bruneau, 2000; Nyakudya et al., 2014), of non-mechanized tillage systems in Southern Africa. Given the observed rainfall (Figure 2) in the growing seasons and the dimensions (sizes) of HM and PPB for this study, the rain drop effect was reduced through water capture in the pits which increased water retention.
The higher soil water storage observed in PPB, M, and HM treatments in the three growing seasons compared to the control can be a result of high harvest of rainwater and movement to deeper soil layers compared to the control treatment (Sawadogo, 2011; Kuotsu et al., 2014). The HM, PPB, and M treatments also created a soil surface buffer to insulate the ground surface and traped running water in rain seasons. For example, studies of Wang et al. (2019), showed that mulch cover can strongly reduce soil surface heat flux and soil water evaporation, and this corroborates to the present study of M relatively increasing soil moisture content compared to other treatments. However, the observed reductions in soil moisture in the mulch treatment compared to other CSA practices could also be largely attributed to termite activity which degraded the grass straw, leading to decreased mulch thickness and exposure of soil surface to evaporative water loss (Mando et al., 1996; Gregory et al., 2002; Iqbal et al., 2020). It is also imperative to note that, soil water storage and moisture content availability is dependent on rainfall and soil water conservation especially in the rainfed agriculture systems (Famiglietti et al., 1998; Gao et al., 2013), hence controlling mulch infestation maintains the thickness level, and occurrence of rainfall and its interception in CSA practices relatively improved soil water storage in the three growing seasons.
With climate change, crop yield, especially that of maize will decrease drastically in rainfed production systems, and consequently lead to increased hunger and poverty in the sub-humid regions. Moreover, maize is a high water use crop (500–800 mm per season), hence often affected by water shortages at critical growth stages (Steduto et al., 2012; Song et al., 2019). For Uganda, the wake of the growing population growth (3.4% per annum) and high demand for food in Uganda justifies promotion and adoption of CSA practices to improve crop productivity (Uganda Bureau of Statistics, 2019). The increase in growth of maize, yield and water use efficiency achieved using climate smart agriculture (CSA) practices imply that adoption of these technologies is a critical step toward improving food security in rainfed agriculture through improved soil water storage in growing seasons. The higher growth and water use efficiency of maize in plots amended using CSA practices could be attributed to reduced soil evaporation, and increase in soil moisture storage available for the crop use as discussed in the above sections. Previous studies (Gicheru et al., 2004; Rahman et al., 2005), have reported higher maize yield and WUE using minimum tillage practices.
Biomass accumulation is a key physiological determinant for maize growth and yield for maize productivity (Gifford et al., 1984; Andrade et al., 2005). The significantly higher grain yield and WUE for maize grown in plots amended with M, HM and PPB treatments in the three maize growing seasons would be a result of increase in soil water availability for optimum maize growth and yield (Monneveux et al., 2006). In comparison to the control treatment, the higher maize productivity achieved using M, PPB and HM practices could be mostly attributed to increased water capture and assimilation to higher yield and this corroborate previous studies (Yang et al., 2004; Edreira et al., 2014; European Commission, 2014; Balugani et al., 2018). Thus, the improved soil water storage in CSA practices reduced risks of crop failure due to erratic rainfall corroborating the findings of Mupangwa et al. (2006), FAO (2013), and Nyakudya and Stroosnijder (2015) who reported improved soil moisture storage in the maize rainfed production systems using permanent planting basins and mulches.
In the present study, the maize grain yields achieved under the different CSA practices are above those reported from farmer-managed maize fields in the study area (2,500–3,800 Kg ha−1) (Uganda Bureau of Statistics, 2019). This suggests that adoption of CSA practices, especially PPB, M and HM could drastically improve maize productivity, due to high efficiency in soil water storage and conservation as demonstrated in the present study. Adoption of these CSA practices would require farmer training and more evidence-based data from mid-long-term trials in different agroecological zones, to refine the technologies for wider application. Our results also are in line with conclusions and recommendations made by The Montpellier Panel (2013) and FAO (2013) on adoption of CSA practices and a suite of on-farm technologies to increase resilience and agriculture productivity amidst the climate change conditions.
The study has revealed for the first time in the sub-humid regions of Uganda that Climate smart agriculture practices relatively improve soil moisture storage, maize growth, yield, and water use efficiency. Its integration with the existing conventional farming practices in rainfed agriculture production systems could be a solution to water shortages in the agriculture production seasons and a potential in increasing resilience for maize growth hence higher final maize biomass for smallholder farmers. Therefore, the adoption of CSA practices in the rainfed agricultural systems increases crop yields and water use efficiency compared to the conventional farming practices amidst the progressive climate change. This could accelerate implementation efforts of climate change adaptation for food production by providing cost-effective and sustainable strategies for soil water management, which is a critical step toward improving food security. Mulching and Permanent planting basins were the most effective at improving soil moisture retention, maize yield and water use efficiency compared to control treatment. Rolling out these technologies in different areas requires to consider availability of mulching materials, costs of establishment, landscape characteristics and labor requirements. More field studies would be necessary to further investigate the effectiveness of these climate smart agriculture practices for improved maize production in different agroecological zones, and their effects on soil nutrient cycles at both mid- to long-term basis.
The raw data supporting the conclusions of this article will be made available by the authors, without undue reservation.
AZ led the writing of the manuscript and experimental data collection, with contributions of J-GM, BT, BB, GG, and KL. All authors contributed to the article and approved the submitted version.
The authors declare that the research was conducted in the absence of any commercial or financial relationships that could be construed as a potential conflict of interest.
All claims expressed in this article are solely those of the authors and do not necessarily represent those of their affiliated organizations, or those of the publisher, the editors and the reviewers. Any product that may be evaluated in this article, or claim that may be made by its manufacturer, is not guaranteed or endorsed by the publisher.
We acknowledge the Center of Excellence Project: IDA Credit 5794-ET under the frame of Africa Center of Excellence for supporting a Ph.D., study in Climate Smart Agriculture and Biodiversity Conservation. We also thank Bulindi Zonal Agriculture Research Development Institute for allocating land for the field trials, the reviewers and the research assistants (Henry Alinda, Geofrey Kairagura, Christine Najjuma, and Ephraim Nabong) for supporting data collection.
Andrade, F. H., Sadras, V. O., Vega, C. R. C., and Echarte, L. (2005). Physiological determinants of crop growth and yield in maize, sunflower and soybean: their application to crop management, modeling and breeding. J. Crop Improv. 14, 51–101. doi: 10.1300/J411v14n01_05
Balugani, E., Lubczynski, M. W., van der Tol, C., and Metselaar, K. (2018). Testing three approaches to estimate soil evaporation through a dry soil layer in a semi-arid area. J. Hydrol. 567, 405–419. doi: 10.1016/j.jhydrol.2018.10.018
Bhattacharya, A.. (2019). Water-Use Efficiency Under Changing Climatic Conditions, Changing Climate and Resource Use Efficiency in Plants. London: Academic Press.
Biazin, B., Sterk, G., Temesgen, M., Abdulkedir, A., and Stroosnijder, L. (2012). Rainwater harvesting and management in rainfed agricultural systems in sub-Saharan Africa - a review. Phys. Chem. Earth 47–48, 139–151. doi: 10.1016/j.pce.2011.08.015
Bu, L. D., Liu, J. L., Zhu, L., Luo, S. S., Chen, X. P., Li, S. Q., et al. (2013). The effects of mulching on maize growth, yield and water use in a semi-arid region. Agric. Water Manag. 123, 71–78. doi: 10.1016/j.agwat.2013.03.015
Campbell, C. A., Selles, F., Lafond, G. P., and Zentner, R. P. (2001). Adopting zero tillage management: impact on soil C and N under long-term crop rotations in a thin Black Chernozem. Can. J. Soil Sc. 81, 139–148. doi: 10.1016/S0167-8809(00)00215-2
Delta-T Devices Ltd. (2006). User Manual for the Profile Probe Type PR2, Vol. 104 (2006). Available online at: https://www.delta-t.co.uk/product/pr2/ (accessed September 10, 2018).
Dixit, A. K., Agrawal, R. K., Das, S. K., Sahay, C. S., Choudhary, M., Rai, A. K., et al. (2019). Soil properties, crop productivity and energetics under different tillage practices in fodder sorghum + cowpea–wheat cropping system. Arch. Agron. Soil Sci. 65, 492–506. doi: 10.1080/03650340.2018.1507024
Edreira, J. I. R., Mayer, L. I., and Otegui, M. E. (2014). Heat stress in temperate and tropical maize hybrids: Kernel growth, water relations and assimilate availability for grain filling. Field Crops Res. 166, 162–172. doi: 10.1016/j.fcr.2014.06.018
Eijkelkamp Soil Water (2017). User Manual, Soil and Water, 1–14. Available online at: https://en.eijkelkamp.com/products/laboratory-equipment/soil-water-permeameters.htmlLaboratorypermeameters (accessed November 10, 2018)
Epule, T. E., Ford, J. D., and Lwasa, S. (2017). Projections of maize yield vulnerability to droughts and adaptation options in Uganda. Land Use Policy 65, 154–163. doi: 10.1016/j.landusepol.2017.04.013
European Commission (2014). Study on Soil and Water in a Changing Environment. Paris: European Commission.
Famiglietti, J. S., Rudnicki, J. W., and Rodell, M. (1998). Variability in surface moisture content along a hillslope transect: Rattlesnake Hill, Texas. J. Hydrol. 210, 259–281. doi: 10.1016/S0022-1694(98)00187-5
Fan, J., Wang, Q., Jones, S. B., and Shao, M. (2016). Soil water depletion and recharge under different land cover in China's Loess Plateau. Ecohydrology 9, 396–406. doi: 10.1002/eco.1642
FAO (2013). Climate Smart Agriculture Sourcebook. Rome: FAO. Available online at: www.fao.org/docrep/018/i3325e/i3325e.pdf (accessed July 23, 2020).
Feng, S., and Fu, Q. (2013). Expansion of global Drylands Under a Warming Climate. Atmos. Chem. Phys. 13, 10081–10094. doi: 10.5194/acp-13-10081-2013
Gao, X., Wu, P., Zhao, X., Wang, J., Shi, Y., Zhang, B., et al. (2013). Estimation of spatial soil moisture averages in a large gully of the Loess Plateau of China through statistical and modeling solutions. J. Hydrol. 486, 466–478. doi: 10.1016/j.jhydrol.2013.02.026
Gicheru, P., Gachene, C., Mbuvi, J., and Mare, E. (2004). Effects of soil management practices and tillage systems on surface soil water conservation and crust formation on a sandy loam in semi-arid Kenya. Soil Tillage Res. 75, 173–184. doi: 10.1016/S0167-1987(03)00161-2
Gifford, R. M., Thorne, J. H., Hitz, W. D., and Giaquinta, R. T. (1984). Crop productivity and photoassimilate partitioning. Science. 225, 801–808. doi: 10.1126/science.225.4664.801
Gornall, J., Betts, R., Burke, E., Clark, R., Camp, J., Willett, K., et al. (2010). Implications of Climate Change for Agricultural Productivity in the Early Twenty-First Century. London: The Royal Society Publishing.
Gregory, P., Ingram, J., Andersson, A., Betts, R., and Brovkin, V. (2002). Environmental consequences of alternative practices for intensifying crop production. Agric. Ecosyst. Environ. 88, 279–90. doi: 10.1016/S0167-8809(01)00263-8
Iqbal, R., Raza, M. A. S., Valipour, M., Saleem, M. F., Zaheer, M. S., Ahmad, S., et al. (2020). Potential agricultural and environmental benefits of mulches—a review. Bull. Natl. Res. Cent. 44, 1–16. doi: 10.1186/s42269-020-00290-3
Kikoyo, D. A., and Nobert, J. (2016). Assessment of impact of climate change and adaptation strategies on maize production in Uganda. Phys. Chem. Earth 93, 37–45. doi: 10.1016/j.pce.2015.09.005
Kuotsu, K., Das, A., Lal, R., Munda, G. C., Ghosh, P. K., and Ngachan, S. V. (2014). Land forming and tillage effects on soil properties and productivity of rainfed groundnut (Arachis hypogaea L.)-rapeseed (Brassica campestris L.) cropping system in northeastern India. Soil Tillage Res. 142, 15–24. doi: 10.1016/j.still.2014.04.008
Legrain, X., Berding, F., Dondeyne, S., Schad, P., and Chapelle, J. (2018). World Reference Base for Soil Resources 2014: International Soil Classification System for Naming Soils and Creating Legends for soil maps. Rome: FAO.
Lin, W., Liu, W., and Xue, Q. (2016). Spring maize yield, soil water use and water use efficiency under plastic film and straw mulches in the Loess Plateau. Sci. Rep. 6, 995. doi: 10.1038/srep38995
Lipper, L., Thornton, P., Campbell, B. M., Baedeker, T., Braimoh, A., Bwalya, M., et al. (2014). Climate-smart agriculture for food security. Nat. Clim. Change 4, 1068–1072. Available online at: https://doi.org/10.1038/nclimate2437
MAAIF (2009). Statistical Abstracts. Ministry of Agriculture, Animal Industry and Fisheries. ENTEBBE.
MAAIF (2017). The Republic of Uganda Ministry of Agriculture, Animal Industries and Fisherise: Performance Review Report. MAAIF, 150.
Mando, A., Stroosnijder, L., and Brussaard, L. (1996). Effects of termites on infiltration into crusted soil. Geoderma 74, 107–113. doi: 10.1016/S0016-7061(96)00058-4
Marumbi, R., Nyamugafata, P., Wuta, M., Tittonell, P., and Torquebiau, E. (2020). Influence of planting basins on selected soil quality parameters and sorghum yield along an agro-ecological gradient in South Eastern Zimbabwe. South. Africa J. Educ. Sci. Technol. 5, 26–52. doi: 10.4314/sajest.v5i1.39821/sajest.2020.001
Mloza-Banda, M. L., Cornelis, W. M., Mloza-Banda, H. R., Makwiza, C. N., and Verbist, K. (2014). Soil properties after change to conservation agriculture from ridge tillage in sandy clay loams of mid-altitude Central Malawi. Soil Use Manag. 30, 569–578. doi: 10.1111/sum.12139
Monneveux, P., Sánchez, C., Beck, D., and Edmeades, G. O. (2006). Drought tolerance improvement in tropical maize source populations: evidence of progress. Crop Sci. 46, 180–191. doi: 10.2135/cropsci2005.04-0034
Moreira, W. H., Tormena, C. A., Karlen, D. L., da Silva, A. P., Keller, T., and Betioli, E. (2016). Seasonal changes in soil physical properties under long-term no-tillage. Soil Tillage Res. 160, 53–64. doi: 10.1016/j.still.2016.02.007
Mupangwa, W., Love, D., and Twomlow, S. (2006). Soil – water conservation and rainwater harvesting strategies in the semi-arid Mzingwane Catchment, Limpopo Basin. Zimbabwe 31, 893–900. doi: 10.1016/j.pce.2006.08.042
Mupangwa, W., Twomlow, S., and Walker, S. (2013). Cumulative effects of reduced tillage and mulching on soil properties under semi-arid conditions. J. Arid Environ. 91, 45–52. doi: 10.1016/j.jaridenv.2012.11.007
Mwenge Kahinda, J., Taigbenu, A. E., Sejamoholo, B. B. P., Lillie, E. S. B., and Boroto, R. J. (2009). A GIS-based decision support system for rainwater harvesting (RHADESS). Phys. Chem. Earth 34, 767–775. doi: 10.1016/j.pce.2009.06.011
Nagargade, M., Tyagi, V., and Singh, M. K. (2017). Climate smart agriculture: an option for changing climatic situation. Plant Eng. 143–165. doi: 10.5772/intechopen.69971
Nyakudya, I. W., and Stroosnijder, L. (2015). Conservation tillage of rainfed maize in semi-arid Zimbabwe: a review. Soil Tillage Res. 145, 184–197. doi: 10.1016/j.still.2014.09.003
Nyakudya, I. W., Stroosnijder, L., and Nyagumbo, I. (2014). Infiltration and planting pits for improved water management and maize yield in semi-arid Zimbabwe. Agric. Water Manag. 141, 30–46. doi: 10.1016/j.agwat.2014.04.010
Nyamadzawo, G., Chikowo, R., Nyamugafata, P., Nyamangara, J., and Giller, K. E. (2008). Soil organic carbon dynamics of improved fallow-maize rotation systems under conventional and no-tillage in Central Zimbabwe. Nutr. Cycl. Agroecosyst. 81, 85–93. doi: 10.1007/s10705-007-9154-y
Okalebo, J. R., Gathua, K. W., and Woomer, P. L. (2002). Laboratory Methods of Soil and Plant Analysis: A Working Manual. 2nd Edn. Nairobi: Sacred African Publishers.
Ongoma, V., Chen, H., Gao, C., Nyongesa, A. M., and Polong, F. (2018). Future changes in climate extremes over Equatorial East Africa based on CMIP5 multimodel ensemble. Nat. Hazards 90, 901–920. doi: 10.1007/s11069-017-3079-9
Partey, S. T., Zougmoré, R. B., Ouédraogo, M., and Campbell, B. M. (2018). Developing climate-smart agriculture to face climate variability in West Africa: challenges and lessons learnt. J. Clean. Prod. 187, 285–295. doi: 10.1016/j.jclepro.2018.03.199
R Core Team (2019). R: A Language and Environment for Statistical Computing. R Foundation for Statistical Computing, Vienna. Available online at: https://www.R-project.org/ (accessed May 20, 2020).
Rahman, M. A., Chikushi, J., Saifizzaman, M., and Lauren, J. G. (2005). Rice straw mulching and nitrogen response of no-till wheat following rice in Bangladesh. F. Crop. Res. 91, 71–81. doi: 10.1016/j.fcr.2004.06.010
Rockström, J., Lannerstad, M., and Falkenmark, M. (2007). Assessing the water challenge of a new green revolution in developing countries. Proc. Natl. Acad. Sci. U. S. A. 104, 6253–6260. doi: 10.1073/pnas.0605739104
Sawadogo, H.. (2011). Using soil and water conservation techniques to rehabilitate degraded lands in Northwestern Burkina Faso. Int. J. Agric. Sustain. 9, 120–128. doi: 10.3763/ijas.2010.0552
Sharma, P., Abrol, V., and Sharma, R. K. (2011). Impact of tillage and mulch management on economics, energy requirement and crop performance in maize-wheat rotation in rainfed subhumid inceptisols, India. Eur J Agron. 34, 46–51. doi: 10.1016/j.eja.2010.10.003
Song, L., Jin, J., and He, J. (2019). Effects of severe water stress on maize growth processes in the field. Sustain. 11, 86. doi: 10.3390/su11185086
Souverijns, N., Thiery, W., Demuzere, M., and Lipzig, N. P. M. V. (2016). Drivers of future changes in East African precipitation. Environ. Res. Lett. 11, 1–9. doi: 10.1088/1748-9326/11/11/114011
Steduto, P., Hsiao, T. C., Fereres, E., and Raes, D. (2012). Crop Yield Response to Water, Vol. 1028. Rome: Food and Agriculture Organization of the United Nations. FAO Irrigation and Drainage Paper, 6–13.
Tambo, J. A., and Kirui, O. K. (2021). Yield effects of conservation farming practices under fall armyworm stress: the case of Zambia. Agric. Ecosyst. Environ. 321, 107618. doi: 10.1016/j.agee.2021.107618
The Montpellier Panel (2013). Sustainable Intensification: A New Paradigm for African Agriculture. London: Agriculture for Impact.
Thierfelder, C., and Wall, P. C. (2012). Effects of conservation agriculture on soil quality and productivity in contrasting agro-ecological environments of Zimbabwe. Soil Use Manag. 28, 209–220. doi: 10.1111/j.1475-2743.2012.00406.x
Thomas, G. A., Dalal, R. C., and Standley, J. (2007). No-till effects on organic matter, pH, cation exchange capacity and nutrient distribution in a Luvisol in the semi-arid subtropics. Soil Tillage Res. 94, 295–304. doi: 10.1016/j.still.2006.08.005
Tilman, D., Balzer, C., Hill, J., and Befort, B. L. (2011). Global food demand and the sustainable intensi fi cation of agriculture. Proc. Natl. Acad. U. S. A. 108, 20260–4. doi: 10.1073/pnas.1116437108
Twomlow, S. J., and Bruneau, P. M. C. (2000). The influence of tillage on semi-arid soil-water regimes in Zimbabwe. Geoderma 95, 33–51. doi: 10.1016/S0016-7061(99)00071-3
Uganda Bureau of Statistics (UBOS). (2019). 2017 Statistical Abstract. Kampala: Uganda Bureau of Statistics 2017. Available online at: http://www.ubos.org/onlinefiles/uploads/ubos/pdfdocuments/abstracts/StatisticalAbstract2013.pdf (accessed July 10, 2018).
Verhulst, N., Govaerts, B., Verachtert, E., Castellanos-Navarrete, A., Mezzalama, M., Wall, P., et al. (2010). Conservation Agriculture, Improving Soil Quality for Sustainable Production Systems. Advances in Soil Science: Food Security and Soil Quality. Boca Raton, FL: CRC Press. p. 137–208.
Wainwright, C. M., Marsham, J. H., Keane, R. J., Rowell, D. P., Finney, D. L., Black, E., et al. (2019). ‘Eastern African Paradox' rainfall decline due to shorter not less intense Long Rains. NPJ Clim. Atmos. Sci. 2, 1–9. doi: 10.1038/s41612-019-0091-7
Wang, X., Fan, J., Xing, Y., Xu, G., Wang, H., Deng, J., et al. (2019). The effects of mulch and nitrogen fertilizer on the soil environment of crop plants. Adv. Agronom. 153, 121–173. doi: 10.1016/bs.agron.2018.08.003
Wheeler, T., and Von Braun, J. (2013). Climate change impacts on global food security. Science. 341, 508–513. doi: 10.1126/science.1239402
World Bank (2019). Toward Scaled-Up and Sustainable Agriculture Finance and Insurance in Uganda, Toward Scaled-Up and Sustainable Agriculture Finance and Insurance in Uganda. New York, NY: World Bank.
Wortmann, C. S., and Eledu, C. S. (1999). Uganda's Agroecological Zones: A Guide for Planners and Policy Marker. Kampala: CIAT, Nairobi.
Xie, H., Perez, N., Anderson, W., Ringler, C., and You, L. (2018). Can Sub-Saharan Africa feed itself? The role of irrigation development in the region's drylands for food security. Water Int. 43, 796–814. doi: 10.1080/02508060.2018.1516080
Yang, H. S., Dobermann, A., Lindquist, J. L., Walters, D. T., Arkebauer, T. J., and Cassman, K. G. (2004). Hybrid-maize - a maize simulation model that combines two crop modeling approaches. F. Crop. Res. 87, 131–154. doi: 10.1016/j.fcr.2003.10.003
Keywords: climate smart agriculture, soil moisture storage, maize, water use efficiency, rainfed agriculture
Citation: Zizinga A, Mwanjalolo J-GM, Tietjen B, Bedadi B, Gabiri G and Luswata KC (2022) Impacts of Climate Smart Agriculture Practices on Soil Water Conservation and Maize Productivity in Rainfed Cropping Systems of Uganda. Front. Sustain. Food Syst. 6:889830. doi: 10.3389/fsufs.2022.889830
Received: 04 March 2022; Accepted: 02 May 2022;
Published: 24 May 2022.
Edited by:
Olcay I. Unver, Arizona State University, United StatesReviewed by:
Margarita Ruiz-Ramos, Polytechnic University of Madrid, SpainCopyright © 2022 Zizinga, Mwanjalolo, Tietjen, Bedadi, Gabiri and Luswata. This is an open-access article distributed under the terms of the Creative Commons Attribution License (CC BY). The use, distribution or reproduction in other forums is permitted, provided the original author(s) and the copyright owner(s) are credited and that the original publication in this journal is cited, in accordance with accepted academic practice. No use, distribution or reproduction is permitted which does not comply with these terms.
*Correspondence: Alex Zizinga, YXppemluZ2FAZ21haWwuY29t
Disclaimer: All claims expressed in this article are solely those of the authors and do not necessarily represent those of their affiliated organizations, or those of the publisher, the editors and the reviewers. Any product that may be evaluated in this article or claim that may be made by its manufacturer is not guaranteed or endorsed by the publisher.
Research integrity at Frontiers
Learn more about the work of our research integrity team to safeguard the quality of each article we publish.