- 1Sustainable Chemistry (Resource Efficiency), Institute of Sustainable Chemistry, Leuphana University of Lüneburg, Lüneburg, Germany
- 2Institute for Food and Environmental Research (ILU), Bad Belzig, Germany
- 3German Institute of Food Technologies – DIL e.V., Quakenbrück, Germany
Microalgae are utilized for various purposes through cell content extraction and application. Cell walls are not utilized and not studied in an extensive manner. At the same time, composition of multilayer and fibrillar structures with various chemical compositions depends on microalgae species, they present an interesting object for chromatography. However, it requires the application of novel processing technologies (such as pulsed electric fields [PEFs]), which are able to selectively permeabilize the cell walls with pores of various sizes and shapes. The current review indicates the application of potential of microalgae cell walls for separation by size exclusion, ion-exchange, and hydrophobic interaction chromatography. However, such a hypothesis should be further experimentally proven.
Introduction
The principle of chromatography is the interaction of an analyte with a stationary phase, which captures, binds, or interacts with the analyte. To mention are, for example, ion-exchange, hydrophobic interaction, size exclusion, or affinity chromatography. The stationary phase is usually made of defined resins that are packed in a column. Depending on the chemical modification of resins, the separation of certain analytes from complex mixtures can be achieved. Chromatography is not only used in analysis but also in preparation to purify molecules during downstream processing from complex mixtures of organic compounds, such as biomass hydrolysates.
Irrespective of the principle of chromatographic separation, the prerequisite is the application of chemically defined and uniform spheric resin particles. This prerequisite would usually exclude the direct use of biological material without modification and unification. Nevertheless, nature provides interesting structures with unique forms and properties. Even though unification is not given, these structures might be worth investigating as the chemical functionality might be unique as well. Cell walls of algae, for instance, can consist of modified polysaccharides, proteins, and in the case of diatoms also silicate (Domozych, 2016). Cell walls maintain the structure and protect the cells from the impact of the environment even under harsh conditions (Domozych, 2019). So far, the utilization potential of algal cells is among others connected to the carbohydrate, lipid, protein contents, and availability of pigments (Baudelet et al., 2017). What remains after the extraction of carbohydrates, lipids, proteins, and pigments is the empty cell wall with different levels of proliferation or destruction. In many cases, the cell wall is destroyed after extraction and loses its potential to be applied in chromatography.
To shed light on the application of cell walls that are obtainable after the extraction of cell compartments, the aim of this mini-review was the systematization of knowledge on the biochemical composition and use of cell walls from microalgae species. Starting with the introduction of extraction methods and particularly pulsed electric fields (PEFs) to obtain cell walls from algal cells, their chemical properties were illuminated, and the application as a solid phase in chromatography was discussed (Figure 1). The focus was laid on maintaining the stability of algal cell walls and their chemical functionality to allow an application in chromatographic separation. The outcomes of this review are expected to contribute to the development of holistic algal biomass utilization strategies, such as cell walls.
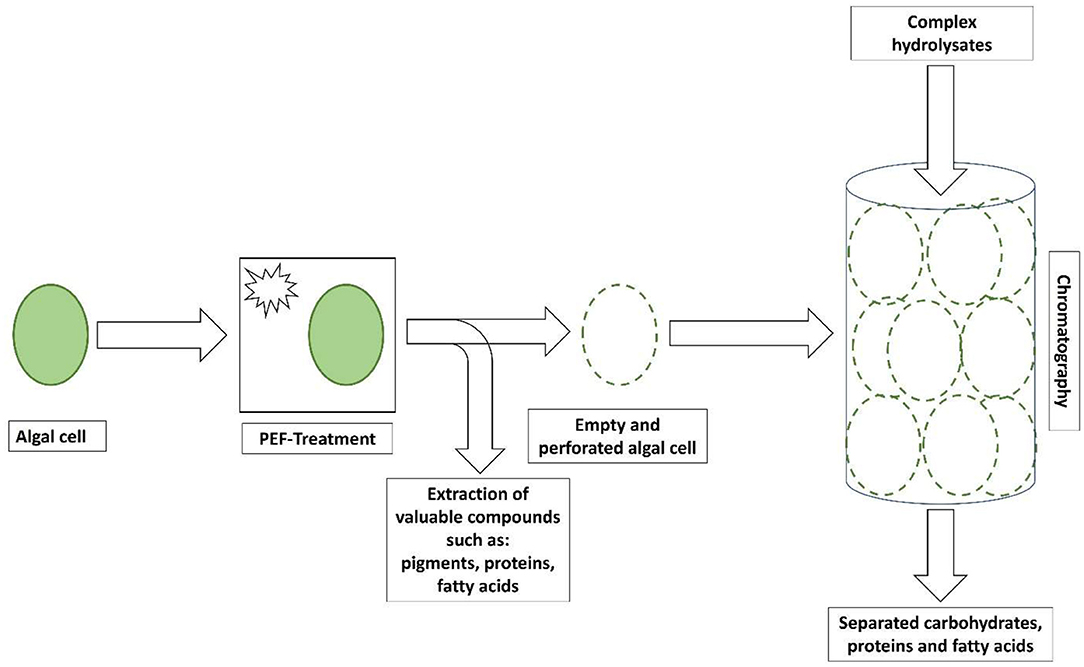
Figure 1. Illustration of the treatment of algal cells by a pulsed electric field (PEF) and proposed use of empty and perforated algal cells in the separation of carbohydrates, proteins, and fatty acids from complex hydrolysates.
Extraction of Cell Compartments
Microalgae are known to accumulate considerable amounts of carbohydrates, proteins, and lipids and contain pigments, such as chlorophyll, carotenoids, and phycobilins. Conventionally, mechanical measures are applied to disintegrate cell walls and liberate cell compartments. To mention are ultrasound, microwave, hydrothermal liquefaction, enzymatic disruption, supercritical carbon dioxide extraction, oxidative stress, and osmotic shock (Ghasemi Naghdi et al., 2016). Furthermore, organic solvents are dominantly used to de-fat biomass and extract valuable lipids and fatty acids. Even though the measures are developed and can be considered safe, cost-effective, robust, efficient, selective, environmentally friendly, feasible for large-scale production, and free of product contamination, which are prerequisites, for instance, for biodiesel production (Ghasemi Naghdi et al., 2016), the different measures destroy the cell wall or create undefined pores to allow the organic solvent to penetrate and to improve extraction efficiency. Thus, these measures may not be appropriate for allowing extraction and maintaining cell walls as they cause non-specific leakage (Martínez et al., 2020).
Recently, PEF technology has been gaining attraction as it allows the creation of pores in the cell wall and facilitates the release of cell compartments. PEF “is based on the formation of a potential difference across a conductive biological material between two electrodes.” The created electric field depends on electric voltage, the shape of electrodes, and the gap between electrodes (Toepfl et al., 2006; Buchmann and Mathys, 2019). Martínez et al. have outlined that PEF fosters the extraction of valuable compounds not only from microalgae but also from bacteria and yeast. They emphasized the non-thermal character and low energy input and highlighted the selective release of intracellular compounds without compromising the cell's general structure. Furthermore, fresh biomass instead of dried biomass can be applied (Martínez et al., 2020). Nevertheless, it is controversially discussed whether a mechanical treatment using bead mills or the application of PEF is beneficial in terms of the release of cell compartments and the energy needed. Lam et al. found a decreased protein yield and higher energy input when PEF was used for protein extraction from Chlorella vulgaris and Neochloris oleoabundans as compared to bead milling (Lam et al., 2017a). Application of bipolar pulses (4–32 μs) and specific energy of 25–150 kJ kg−1 to Chlorella sorokiniana demonstrated a marginal extraction effect (Leonhardt et al., 2020). However, a lower energy input was reported for the release of around 70% (w/w) of the proteins from Chlamydomonas reinhardtii using PEF (Lam et al., 2017b) and Buchmann et al. were able to release 96.6% (w/w) of the proteins from C. vulgaris (Buchmann et al., 2019).
From the information about PEF and its effect on the cell permeability, it can be concluded that this technique is appropriate for the extraction of valuable compounds. To achieve a proper extraction of cell compartments without destroying the structural and chemical functionality of algal cell walls, PEF seems an appropriate technique as well. Carullo et al. stated that PEF contributes to an extraction “without the formation of cell debris, which might facilitate the subsequent downstream purification operations” (Carullo et al., 2020). Usually, without cell disruption, the extraction of lipids using solvents is a slow process that relies on the passive diffusion of solvents and lipids across the cell wall. Contrarily, cell rupture enables lipids to be rapidly recovered by solvents (Yap et al., 2014; Parniakov et al., 2015). Mechanical treatment usually destroys the cell wall and does not allow further use of it. As mentioned above, PEF creates pores in cell walls or partly opens it but keeps the wall basically intact. This is a particularly important aspect when it comes to utilization of it. Bensalem et al. found pores in the cell wall of C. reinhardtii with a radius between 0.77 and 1.59 nm for reversible (5 kV cm−1) and irreversible (7 kV cm−1) electroporation with 5 μs pulse duration. The longer the pulse the larger the radius of the pores, and 5.11 nm of radius was obtained for a pulse duration of 10 μs (Bensalem et al., 2020). The characterization of the pore size was carried out using a Confocal Laser Scanning Microscope and by fluorescein-labeled dextran molecules of different sizes. To determine the permeability of cells, the presence or absence of dextran fluorescence inside the cell was analyzed. The authors further found that in all cases, the cell wall of C. reinhardtii showed permeability toward 3 kDa large dextran molecules. The penetration of larger dextran molecules was found when cells were exposed to additional mechanical stress, such as compression. Thus, PEF not only allows the extraction of larger molecules but apparently also the creation of defined pores size by changing electric field strength and pulse duration. However, it should also be noted that even with PEF severe cell disruption can occur at high forces (Sheng et al., 2011) and the right balance needs to be found to create defined pores while keeping the overall cell wall intact.
It is an asset that PEF does not result in the destruction of the cell wall and the creation of cell debris. This does not only ease the downstream processing for the separation of proteins and lipids as concluded by Carullo et al. (2020) but may also ease the separation of perforated and empty cell walls. Algal cells are usually harvested using flocculation, floatation, filtration, and centrifugation (Zhu et al., 2018). Filtration is an easy measure to separate solvent and extracted compounds from cell walls. Another separation method is centrifugation, which relies on the density of substances and their separation by gravitational force. Using centrifugation, a solid concentration of up to 10–20% (w/w) can be achieved. However, the energy demand can be high (Najjar and Abu-Shamleh, 2020). Nevertheless, centrifugation would allow the separation of “empty” from “full” cells due to the different densities but similar sizes. It is an asset as compared to filtration, flocculation, and floatation.
Characterization of Cell Walls
The potential of algal cell walls as solid-state material in chromatography depends on the size and on the composition. Domozych stated that a typical algal cell wall “consists of crystalline fibrillar polysaccharides (e.g., cellulose) that interact with a surrounding matrix of polysaccharides and proteoglycan” (Domozych, 2019). An algal cell wall is usually formed by an inner and an outer cell walls and has biological functions, such as structural function, intercellular communication (Kinnaert et al., 2017), defense, absorption of key ions, water, and biochemicals (Domozych, 2019). The characterization of the composition of algal cell walls was already under investigation almost 60 years ago. Punnett and Derrenbaker identified various amino acids and amino sugars in the walls of Chlorella sp., Scendesmus sp., Lyngby sp., and Nostoc sp. (Punnett and Derrenbacker, 1966). Their major finding was the presence of eight amino acids (aspartic acid, glutamic acid, glycine, alanine, serine, valine, isoleucine, and leucine) in the composition of the walls. This led to the conclusion that “there is a common basal structure in the walls of algae.”
Over the years with improving analytical equipment, more detailed information regarding the composition could be revealed. In Velazquez-Lucio et al. (2018) and other studies, the cell wall of algae is described as a combination of “cellulose, pectin (polygalacturonic acid), and sulfated polysaccharides, that can be impregnated with inorganic substances, such as calcium carbonate, silica, and magnesium” (Chen et al., 2013; Vahabisani and An, 2021). A detailed analysis chemical composition of the C. vulgaris highlighted the presence of chitin or chitin-like polymers (glucosamine found in strong alkali extracts) and starch or cellulose (glucose detected in strong acidic extracts) (Weber et al., 2022). The composition is considered particularly important as a cell wall is in contact with the molecules to be separated. However, algal cells are highly diverse. Most obvious is not only the range of size from micrometers to tens of micrometers (Mata et al., 2012) but also the wall structure and shape. Structures, such as scales, theca, fibrillar composites, frustule, or amphiesma, have been described for the different algal taxon (Table 1). The shape can be oval, round, or even like a needle.
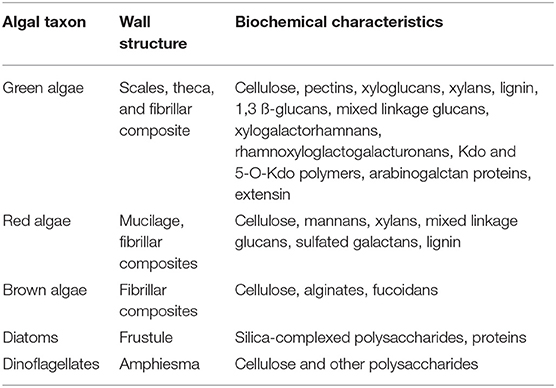
Table 1. The structure and biochemical characteristics of cell walls of selected algal taxon after (Domozych, 2016, 2019) and references therein (Canelli et al., 2021).
Relatively hidden is the biochemical composition (Table 1). Scholz et al. investigated the cell wall of Nannochloropsis gaditana and found around 79% (w/w) carbohydrates, 6% (w/w) proteins, and 1% (w/w) minerals. In total, they were able to characterize 86% (w/w) of the cell wall. The general composition is made of polysaccharides, such as pectin, agar, alginate, and algaenan polymers; fucans, hemicellulose, and glycoproteins in a microfibrils matrix of cellulose; and traces of fucose, xylose, rhamnose, arabinose, and galactose (Scholz et al., 2014). Similar results are indicated in another study; however, with other microalgae species, the chemical and structural compositions are different (Rashidi and Trindade, 2018). This composition can vary between algal species (Table 1). Common for green, red, and brown algae and dinoflagellates is cellulose in combination with other polysaccharides in cell walls. Diatoms are special as they contain silica, which is complexed with polysaccharides. Examples of polysaccharides found in a selection of microalgae are shown in Table 1. A detailed explanation of polysaccharides found in various algal cell walls is out of the scope of this mini-review and can be seen in Voigt et al. (2014), Domozych (2016), Baudelet et al. (2017), Domozych (2019), and references therein.
From the review published by Kinnaert et al., it is impressive to see how complicated synthetic routes can be to create the complex structures of macromolecules present in plant cell walls (Kinnaert et al., 2017). They further concluded that there is “clearly no lack of unexplored structures to serve as inspiration for synthetic chemists, especially within the marine polysaccharides.” New molecules are continuously found in micro- and macroalgal cells and cell walls. Pankiewicz et al. isolated the polysaccharide ulvan from the green macroalgae Ulva flexusa and Cladophora glomerata (Pankiewicz et al., 2016). In accordance with the authors, this was the first time that sulfated polysaccharides were isolated from freshwater macroalgae. In 2009, Robic et al. extracted ulvan from the cell wall of the marine green alga Ulva rotundata (Robic et al., 2009). Thus, it is assumed here that the chemical potential and many interesting molecules are still to be discovered, which may foster the use of algal cell walls in special applications.
Application of Cell Walls in Chromatography
Commercially defined resins are applied as a stationary phase for the chromatographic separation of molecules. Molecules that interact strongly with the stationary phase migrate through the resin slowly, whereas molecules that interact weakly migrate through it quickly. As mentioned in the introduction, various principles of chromatographic separation exist. Depending on the principle, the resin and its modification need to be selected accordingly. Ion-exchange chromatography is used to separate charged molecules, such as anions and cations, amino acids, peptides, and proteins. Depending on the charge, anion and cation resins exist. To mention are resins with quaternary ammonium or diethylaminoethyl groups as anion exchanger, or sulfopropyl or carboxymethyl groups as a cation exchanger. Hydrophobic interaction chromatography separates molecules based on their hydrophobicity. This technique is usually applied to separate proteins under less denaturing conditions and to maintain their activity. The resin for hydrophobic interaction chromatography can contain t-butyl and/or carboxyl groups interfering with the molecule of interest.
Size exclusion chromatography separates molecules based on their size by filtration through a gel of spherical beads that contain pores of a specific size. Separation occurs when molecules of different sizes are included or excluded from the pores. Small molecules diffuse into the pores and their flow through the column is retarded according to their size, while large molecules do not enter the pores and are eluted. Consequently, molecules are separated based on their size as they pass through the column and are eluted in order of decreasing molecular weight. For instance, bead sizes between <45 and 180 μm are available. Affinity chromatography is a separation method based on a specific binding interaction between an immobilized ligand and its binding partner, such as antibody/antigen, enzyme/substrate, and enzyme/inhibitor interactions (Bio-Rad, 2022).
It needs to be shown to what extent an empty algal cell can contribute to a chromatographic separation process. The success of chromatography is based on the specific resins, and thus it needs to be shown whether PEF-treated algal cells and their walls can be applied as a stationary phase. As outlined, algal cells exhibit an interesting biochemical composition, and the different molecules at the outer surface of the walls might be appropriate for the interaction with various molecules. Thus, algal cells have been tested for the adsorption of ions at their walls, which has basically the same result as ion-exchange chromatography but is based on adsorption of ions rather than an exchange. For instance, Gu and Lan have shown the biosorption of Pb(II), Hg(II), Zn(II), Cd(II), and Cu(II). The adsorption capacities were between 1.03 and 1.23 mmol per gram biomass of N. oleoabundans (Gu and Lan, 2021). Heavy metal adsorption occurred on the cell surface. The authors concluded that peptide-containing molecules and non-cellulosic polysaccharides on the cell wall are primary sites of adsorption. In 50 ppm metal ion solutions to which 0.3 g dry N. oleoabundans was added and adsorption was carried out at pH 7, 25°C for 30 min around 90% of Pb was removed. Pb thereby did reveal an almost three-times higher removal than Zn, Cd, and Cu, while Hg was removed by around 70%. Gu and Lan further reported that heavy metal removal is influenced by biomass dosage, pH value, contact time, and ion concentration. Cu(II) caused cell aggregation and reduced the apparent adsorption capacity. Pb(II) revealed the largest affinity to the cell surface (Gu and Lan, 2021). Spain et al. (2021) described, based on Javanbakht et al. (2014), that various functional groups, such as carboxyl, hydroxyl, sulfate, sulfhydryl (thiol), phosphate, amino, amide, imine, thioether, phenol, and carbonyl (ketone, imidazole, phosphonate, and phosphodiester), at the outer layer of the cell wall have the potential to be involved in metal binding. Andrade et al. reported that the brown alga Padina gymnospora modifies its polysaccharides when exposed to heavy metals, such as Cd and Pb. Polysaccharides, sulfate groups, uronic acids, fucose, mannose, and galactose were found in higher amounts as compared to control cultures. These compounds were responsible for metal sorption. From the initial 351.3 and 547.5 mg L−1, 39.5 and 60.8% remained of Cd and Pb, respectively. The authors concluded that adoption of extracellular compounds might be a defense mechanism to avoid an accumulation in the cells (Andrade et al., 2010). This is of interest as it might imply that the specificity to bind certain molecules can be created by exposing living algal cells to respective compounds. However, it should be highlighted that the use of dead, PEF-treated cells is superior to living cells, as the last ones tend to bioaccumulate metals (Spain et al., 2021) or modify their biochemical characteristics.
An example of the hydrophobic interaction might be the biosorption of organic compounds by microalga. Using living cells, an accumulation and adsorption may take place. Wang et al. used the four microalgal species, i.e., Phaeocystis globosa, Nannochloropsis oculata, Dunaliella salina, and Platymonas subcordiformis, to remove nonylphenol. They found “a sharp reduction of nonylphenol in medium containing the four microalgal species during the first 24 h of incubation, and the four species exhibited the greatest capacity for nonylphenol adsorption and absorption within 24 h of culture” (Wang et al., 2019). The aspect of accumulation and even degradation or formation by the respective cells emphasized the use of dead cells without intact metabolism. Furthermore, the advantages of using deadly over living cells are avoidance of toxicity-related problems, no maintenance is required, storage of biomass for long periods without loss of effectiveness, regeneration is more feasible, and the possibility to work on a greater range of environmental variables (Torres, 2020). Torres concluded in his review that biosorption “is a technology that presents a great diversity of options and combinations, demonstrating great flexibility for its application.” However, Torres also concluded that “the amount of possible materials (living or dead) is enormous.” This is certainly correct, however, with increasing algal biomass production and increasing cases where PEF is applied, sufficient prepared cells might be available for chromatography. Nevertheless, the new biosorbent needs to be compared with commercially available sorbents to illuminate its advantages and disadvantages.
A drawback that may hinder the application of PEF-treated algal cells in chromatography is the fact that biosorption does not only rely on a selective binding but may also rely on chelation and electrostatic attraction (Vahabisani and An, 2021). As long the binding is irreversible, it will not be possible to selectively desorb molecules from algal cells. However, also using commercial resins, desorption needs to be carried out to selectively release molecules from a chromatography column, and thus there is most likely a chance to achieve selective desorption. Spain et al. stated that it is possible to recover biomass and heavy metals (Spain et al., 2021), and thus defined desorption should function.
Whether compounds form the cell wall or are a part of the outer layer and exposed to the surrounding environment is of relevance for chromatographic applications. The molecules at the outer layer interact with a molecule and can bind it, while the compounds found in walls may not interfere with molecules. Thus, every characterization of compounds, such as sulfonated polysaccharides or proteins, at the outer layer is of relevance to develop novel applications. What is most likely to work is size exclusion chromatography when pores of defined sizes are created using PEF in cell walls. The possibility to create different pore sizes by longer or shorter PEF treatments has been shown already (Bensalem et al., 2020). Assuming that the functional groups on the cell wall surface are still intact after PEF treatment, cell wall-based stationary phases can enable a separation not even by size exclusion but also by ion-exchange or hydrophobic interaction chromatography. Another aspect, which may increase the performance, is the interaction of molecules with the inner structural compounds of cell walls. Structural compounds are usually not exposed to or in contact with the environment. However, once a molecule passes through PEF-created pores, it may interact with the compounds inside.
Economic Aspects
The cost of PEF application depends on the applied field strengths and specific energy input per unit of the treated product. While investment costs depend more on the scale and capacity of applications (field strength), operation costs are more related to the daily operation needs (specific energy inputs) (Töpfl, 2006). It is expected that total PEF costs for the application to microalgae in the scope of 14–20 Euros per ton of treated biomass are lower than similar thermal treatments. Investment costs for the cell disintegration equipment at the scale of 20 tons per hour of liquid with the accounting of the inflation should account for capital investment up to 630,000 Euro. Despite the high initial investment costs, lysing of microalgae cells for extraction of valuable components is pointed to be in the scope of 2.69 USD (3.47 USD with the accounting of inflation) per barrel of extracted lipids (Roth, 2011). It corresponds well with the recent estimates on the cost of PEF application for the extraction of proteins from C. vulgaris, when protein costs are indicated to be in the scope of 4.16 USD per 1 kg (4.58 USD with the accounting of inflation) (Soto-Sierra et al., 2020).
The mass of an algal cell wall is different between algae species. Even if only 1% (w/w) of the biomass is made of the cell walls and under consideration that 14–20 Euros are needed to treat 1 ton of biomass (Töpfl, 2006), then 1,400–2,000 Euros are needed to produce 1 ton of perforated cell walls. This seems relatively cheap as compared to the cost of synthetic resins. For instance, 100 g of commercially available hydroxylamine wang resin costs around 3,000 Euros. However, a direct comparison of perforated cell walls with synthetic resin is challenging as it depends on the performance and purity. Synthetic resins are highly effective for a specific task and highly pure. This needs to be shown for perforated algal cell walls.
Conclusions
Microalgae content extraction methods vary in their approaches, efficiency, and selectivity not only for the cell content separation but also for further utilization of cell walls. PEF technology demonstrates to be one of the most tender techniques for the permeabilization of microalgae cell walls, selective extraction of cell components, and preservation of cell wall components and their integrity. Such an approach is useful for further potential uses, e.g., the use of cell walls in chromatography. Different microalgae species demonstrate various cell wall structures, most of them are of multilayer and fibrillar nature. Such “onion-like” structures and variations in chemical composition make them an interesting object for chromatographic applications. PEF technology, being a flexible technique, can deliver pulses of various intensities, duration, and shapes to microalgae cells. Such selective permeabilization allows to preserve the functional groups on different levels of cells and enables separation by size exclusion and by ion-exchange or hydrophobic interaction chromatography. The advantage that may foster an application of PEF-treated cells in chromatography is the presence of various functionalized molecules on the surface of algal cells. This diverse functionality can result in the chromatographic separation of various organic compounds. This potential may be used for the separation of proteins, peptides, amino acids, carbohydrates, oligosaccharides, and sugars from complex organic waste streams. However, the efficiency might be not comparable to synthetic resins, which are defined to separate a certain class of organic compounds. The hypothesis on the potential of microalgae cell wall application for chromatography should be thoroughly tested in experiments and biochemical studies.
Author Contributions
DP and SS: writing—original draft preparation and reviewing and editing. All authors contributed to the article and approved the submitted version.
Conflict of Interest
The authors declare that the research was conducted in the absence of any commercial or financial relationships that could be construed as a potential conflict of interest.
Publisher's Note
All claims expressed in this article are solely those of the authors and do not necessarily represent those of their affiliated organizations, or those of the publisher, the editors and the reviewers. Any product that may be evaluated in this article, or claim that may be made by its manufacturer, is not guaranteed or endorsed by the publisher.
Acknowledgments
The authors acknowledge the German Federal Ministry of Education and Research (BMBF) for providing funding within the Era-Net Cofund FACCE SURPLUS (Project UpWaste 031B0934) and the Era-Net Cofund FOSC-ERA (Project Climaqua 2821ERA12) Programs.
References
Andrade, L. R., Leal, R. N., Noseda, M., Duarte, M. E., Pereira, M. S., Mourão, P. A., et al. (2010). Brown algae overproduce cell wall polysaccharides as a protection mechanism against the heavy metal toxicity. Mar. Pollut. Bull. 60, 1482–1488. doi: 10.1016/j.marpolbul.2010.05.004
Baudelet, P.-H., Ricochon, G., Linder, M., and Muniglia, L. (2017). A new insight into cell walls of Chlorophyta. Algal Res. 25, 333–371. doi: 10.1016/j.algal.2017.04.008
Bensalem, S., Pareau, D., Cinquin, B., Français, O., Le Pioufle, B., and Lopes, F. (2020). Impact of pulsed electric fields and mechanical compressions on the permeability and structure of Chlamydomonas reinhardtii cells. Sci. Rep. 10, 2668. doi: 10.1038/s41598-020-59404-6
Bio-Rad (2022). Bio-rad. Available online at: www.bio-rad.com (accessed January 1, 2022).
Buchmann, L., Brändle, I., Haberkorn, I., Hiestand, M., and Mathys, A. (2019). Pulsed electric field based cyclic protein extraction of microalgae towards closed-loop biorefinery concepts. Bioresour. Technol. 291, 121870. doi: 10.1016/j.biortech.2019.121870
Buchmann, L., and Mathys, A. (2019). Perspective on fulsed electric field treatment in the bio-based industry. Front. Bioeng. Biotechnol. 7, 265. doi: 10.3389/fbioe.2019.00265
Canelli, G., Murciano Martínez, P., Austin, S., Ambühl, M. E., Dionisi, F., Bolten, C. J., et al. (2021). Biochemical and morphological characterization of heterotrophic Crypthecodinium cohnii and Chlorella vulgaris cell walls. J. Agric. Food Chem. 69, 2226–2235. doi: 10.1021/acs.jafc.0c05032
Carullo, D., Pataro, G., Dons,ì, F., and Ferrari, G. (2020). Pulsed electric fields-assisted extraction of valuable compounds from Arthrospira platensis: effect of pulse polarity and mild heating. Front. Bioeng. Biotechnol. 8, 551272. doi: 10.3389/fbioe.2020.551272
Chen, C.-Y., Zhao, X.-Q., Yen, H.-W., Ho, S.-H., Cheng, C.-L., Lee, D.-J., et al. (2013). Microalgae-based carbohydrates for biofuel production. Biochem. Eng. J. 78, 1–10. doi: 10.1016/j.bej.2013.03.006
Domozych, D. (2016). “Biosynthesis of the cell walls of the algae,” in The Physiology of Microalgae. Developments in Applied Phycology, eds M. Borowitzka and R. J. Beardall (Cambridge: Springer), 47–63. doi: 10.1007/978-3-319-24945-2_2
Ghasemi Naghdi, F., González González, L. M., Chan, W., and Schenk, P. M. (2016). Progress on lipid extraction from wet algal biomass for biodiesel production. Microb. Biotechnol. 9, 718–726. doi: 10.1111/1751-7915.12360
Gu, S., and Lan, C. Q. (2021). Biosorption of heavy metal ions by green alga Neochloris oleoabundans: effects of metal ion properties and cell wall structure. J. Hazard. Mater. 418, 126336. doi: 10.1016/j.jhazmat.2021.126336
Javanbakht, V., Alavi, S. A., and Zilouei, H. (2014). Mechanisms of heavy metal removal using microorganisms as biosorbent. Water Sci. Technol. 69, 1775–1787. doi: 10.2166/wst.2013.718
Kinnaert, C., Daugaard, M., Nami, F., and Clausen, M. H. (2017). Chemical synthesis of oligosaccharides related to the cell walls of plants and algae. Chem. Rev. 117, 11337–11405. doi: 10.1021/acs.chemrev.7b00162
Lam, G. P., Postma, P. R., Fernandes, D. A., Timmermans, R. A.H., Vermuë, M., Barbosa, M. J., et al. (2017a). Pulsed electric field for protein release of the microalgae Chlorella vulgaris and Neochloris oleoabundans. Algal Res. Biomass Biofuels Bioprod. 24, 181–187. doi: 10.1016/j.algal.2017.03.024
Lam, G. P., Van Der Kolk, J. A., Chordia, A., Vermuë, M., Olivieri, G., Eppink, M. H. M., et al. (2017b). Mild and selective protein release of cell wall deficient microalgae with pulsed electric field. ACS Sustain. Chem. Eng. 5, 6046–6053. doi: 10.1021/acssuschemeng.7b00892
Leonhardt, L., Käferböck, A., Smetana, S., De Vos, R., Toepfl, S., and Parniakov, O. (2020). Bio-refinery of Chlorella sorokiniana with pulsed electric field pre-treatment. Bioresour. Technol. 301, 122743. doi: 10.1016/j.biortech.2020.122743
Martínez, J. M., Delso, C., Álvarez, I., and Raso, J. (2020). Pulsed electric field-assisted extraction of valuable compounds from microorganisms. Compr. Rev. Food Sci. Food Saf. 19, 530–552. doi: 10.1111/1541-4337.12512
Mata, T. M., Martins, A. A., and Caetane, N. S. (2012). “Microalgae processing for biodiesel production,” in Advances in Biodiesel Production, eds R. Luque and J. A. Melero (Sawston: Woodhead Publishing), 204–231. doi: 10.1533/9780857095862.2.204
Najjar, Y. S. H., and Abu-Shamleh, A. (2020). Harvesting of microalgae by centrifugation for biodiesel production: a review. Algal Res. 51, 102046. doi: 10.1016/j.algal.2020.102046
Pankiewicz, R., Łeska, B., Messyasz, B., Fabrowska, J., Sołoducha, M., and Pikosz, M. (2016). First isolation of polysaccharidic ulvans from the cell walls of freshwater algae. Algal Res. 19, 348–354. doi: 10.1016/j.algal.2016.02.025
Parniakov, O., Barba, F. J., Grimi, N., Marchal, L., Jubeau, S., Lebovka, N., et al. (2015). Pulsed electric field assisted extraction of nutritionally valuable compounds from microalgae Nannochloropsis spp. using the binary mixture of organic solvents and water. Innov. Food Sci. Emerg. Technol. 27, 79–85. doi: 10.1016/j.ifset.2014.11.002
Punnett, T., and Derrenbacker, E. C. (1966). The amino acid composition of algal cell walls. Microbiology 44, 105–114. doi: 10.1099/00221287-44-1-105
Rashidi, B., and Trindade, L. M. (2018). Detailed biochemical and morphologic characteristics of the green microalga Neochloris oleoabundans cell wall. Algal Res. 35, 152–159. doi: 10.1016/j.algal.2018.08.033
Robic, A., Rondeau-Mouro, C., Sassi, J. F., Lerat, Y., and Lahaye, M. (2009). Structure and interactions of ulvan in the cell wall of the marine green algae Ulva rotundata (Ulvales, Chlorophyceae). Carbohydr. Polym. 77, 206–216. doi: 10.1016/j.carbpol.2008.12.023
Roth, I. (2011). Application of Pulsed Electric Fields to the Extraction of Oil From Microalgae. Final Report for IIP-1013814. Bedford, MA: National Science Foundation SBIR.
Scholz, M. J., Weiss, T. L., Jinkerson, R. E., Jing, J., Roth, R., Goodenough, U., et al. (2014). Ultrastructure and composition of the Nannochloropsis gaditana cell wall. Eukaryot Cell 13, 1450–1464. doi: 10.1128/EC.00183-14
Sheng, J., Vannela, R., and Rittmann, B. E. (2011). Evaluation of cell-disruption effects of fulsed-electric-field treatment of Synechocystis PCC 6803. Environ. Sci. Technol. 45, 3795–3802. doi: 10.1021/es103339x
Soto-Sierra, L., Kulkarni, S., Woodard, S. L., and Nikolov, Z. L. (2020). Processing of permeabilized Chlorella vulgaris biomass into lutein and protein-rich products. J. Appl. Phycol. 32, 1697–1707. doi: 10.1007/s10811-020-02055-x
Spain, O., Plöhn, M., and Funk, C. (2021). The cell wall of green microalgae and its role in heavy metal removal. Physiol. Plant. 173, 526–535. doi: 10.1111/ppl.13405
Toepfl, S., Heinz, V., and Knorr, D. (2006). “Applications of pulsed electric fields technology for the food Industry,” in Pulsed Electric Fields Technology for the Food Industry: Fundamentals and Applications, eds J. Raso and V. Heinz (Boston, MA: Springer US), 197–221. doi: 10.1007/978-0-387-31122-7_7
Töpfl, S. (2006). Pulsed Electric Fields (PEF) for Permeabilization of Cell Membranes in Food- and Bioprocessing – Applications, Process and Equipment Design and Cost Analysis. Berlin: Technische Universität Berlin.
Torres, E. (2020). Biosorption: a review of the latest advances. Processes 8, 1584. doi: 10.3390/pr8121584
Vahabisani, A., and An, C. (2021). Use of biomass-derived adsorbents for the removal of petroleum pollutants from water: a mini-review. Environ. Syst. Res. 10, 25. doi: 10.1186/s40068-021-00229-1
Velazquez-Lucio, J., Rodríguez-Jasso, R. M., Colla, L. M., Sáenz-Galindo, A., Cervantes-Cisneros, D. E., Aguilar, C. N., et al. (2018). Microalgal biomass pretreatment for bioethanol production: A review. Biofuel Res. J. 5, 780–791. Available online at: https://www.biofueljournal.com/article_58098_a44c290fe9d5f8d9f1baf7c6a46132d7.pdf
Voigt, J., Stolarczyk, A., Zych, M., Malec, P., and Burczyk, J. (2014). The cell-wall glycoproteins of the green alga Scenedesmus obliquus. The predominant cell-wall polypeptide of Scenedesmus obliquus is related to the cell-wall glycoprotein gp3 of Chlamydomonas reinhardtii. Plant Sci. 215–216, 39–47. doi: 10.1016/j.plantsci.2013.10.011
Wang, L., Xiao, H., He, N., Sun, D., and Duan, S. (2019). Biosorption and biodegradation of the environmental hormone nonylphenol by four marine microalgae. Sci. Rep. 9, 5277. doi: 10.1038/s41598-019-41808-8
Weber, S., Grande, P. M., Blank, L. M., and Klose, H. (2022). Insights into cell wall disintegration of Chlorella vulgaris. PLoS ONE 17, e0262500. doi: 10.1371/journal.pone.0262500
Yap, B. H. J., Crawford, S. A., Dumsday, G. J., Scales, P. J., and Martin, G. J. O. (2014). A mechanistic study of algal cell disruption and its effect on lipid recovery by solvent extraction. Algal Res. 5, 112–120. doi: 10.1016/j.algal.2014.07.001
Keywords: chromatography, algal cell, wall, stationary phase, biosorbent
Citation: Pleissner D and Smetana S (2022) Can Pulsed Electric Fields Treated Algal Cells Be Used as Stationary Phase in Chromatography? Front. Sustain. Food Syst. 6:860647. doi: 10.3389/fsufs.2022.860647
Received: 23 January 2022; Accepted: 23 March 2022;
Published: 29 April 2022.
Edited by:
Pankaj B. Pathare, Sultan Qaboos University, OmanReviewed by:
Christine Jeyaseelan, Amity University, IndiaJayesh Jagannath Ahire, Unique Biotech Limited, India
Copyright © 2022 Pleissner and Smetana. This is an open-access article distributed under the terms of the Creative Commons Attribution License (CC BY). The use, distribution or reproduction in other forums is permitted, provided the original author(s) and the copyright owner(s) are credited and that the original publication in this journal is cited, in accordance with accepted academic practice. No use, distribution or reproduction is permitted which does not comply with these terms.
*Correspondence: Daniel Pleissner, ZGFuaWVsLnBsZWlzc25lckBsZXVwaGFuYS5kZQ==