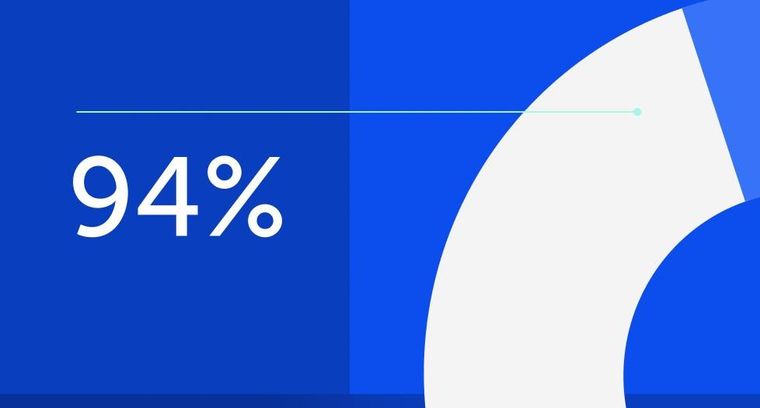
94% of researchers rate our articles as excellent or good
Learn more about the work of our research integrity team to safeguard the quality of each article we publish.
Find out more
ORIGINAL RESEARCH article
Front. Sustain. Food Syst., 04 February 2022
Sec. Agroecology and Ecosystem Services
Volume 6 - 2022 | https://doi.org/10.3389/fsufs.2022.824750
This article is part of the Research TopicPollen as Food for Bees: Diversity, Nutrition, and ContaminationView all 9 articles
With 2,000 species currently recorded in Europe, bees are a highly diversified and efficient group of pollinating insects. They obtain their nutrients from nectar and pollen of flowers. However, the chemical composition of these resources, especially of pollen (e.g., protein, lipid, amino acids, fatty acids, or sterol content), is highly variable among plant species. While it is well-known that bees show interspecific variation in their floral choices, there is a lack of information on the nutritional requirements of different bee species. We therefore developed original experiments in laboratory conditions to evaluate the interspecific variations in bee nutritional requirements. We analyzed the chemical content of eight pollen blends, different in terms of protein, lipid, amino acids, and sterols total concentration and profiles. Each pollen blend was provided to four different bee model species: honey bees (Apis mellifera), bumblebees (Bombus terrestris), mason bees (Osmia bicornis and Osmia cornuta). For each species, specific protocols were used to monitor their development (e.g., weight, timing, survival) and resource collection. Overall, we found that the nutritional requirements across those species are different, and that a low-quality diet for one species is not necessarily low-quality for another one. While honey bees are negatively impacted by diets with a high protein content (~40%), bumblebees and mason bees develop normally on these diets but struggle on diets with a low total amino acid and sterol content, specifically with low concentrations of 24-methylenecholesterol and β-sitosterol. Overall, our study supports the need of conserving and/or introducing plant diversity into managed ecosystems to meet the natural nutritional preferences of bees at species and community level.
With more than 2,000 species recorded in Europe (Rasmont et al., 2017), bees represent a highly diverse group of pollinators (Michener, 2007; Danforth et al., 2013). These species show a wide variability in various traits such as body size (i.e., from 0.3 mm to 4.5 cm in Europe), social behavior (e.g., cleptoparasitic, solitary, eusocial), nesting behavior (e.g., cavity- or soil-nesting), foraging strategies (e.g., pollen generalist or specialist), or phenology (e.g., uni- or bivoltine) (Michener, 2007; Michez et al., 2019). This diversity is crucial for the successful sexual reproduction of wild and domesticated plants, but it is also critical to understand this variability to implement efficient conservation programs (Nieto et al., 2014). Indeed, bees are the dominant pollinators of crop and wild plants in most ecosystems, visiting more than 90% of crop varieties (Potts et al., 2016). Some generalist bee species have been domesticated and are now used for crop pollination like the western honey bee (Apis mellifera), the buff-tailed bumblebee (Bombus terrestris) (Banda and Paxton, 1991; Velthuis and Doorn, 2006), and a few solitary species (Gruber et al., 2011; Pitts-Singer and Cane, 2011). However, unmanaged species are still key pollinators as there are many genus-specific plant-pollinator interactions, linking wild plant diversity to wild bee diversity (Ollerton, 2017). Moreover, wild bees have been shown to increase crop production by up to twice as much as honey bees, underlining the importance of wild bees even in agro-ecosystems (Garibaldi et al., 2013; Weekers et al., 2022).
Losses and declines in managed and wild bee populations have been reported worldwide (Cameron et al., 2011; Goulson et al., 2015; Duchenne et al., 2020). Habitat loss and agricultural intensification, resulting in landscape simplification have been identified as important drivers of pollinator decline (Winfree, 2010; Persson et al., 2015; Vray et al., 2019). These factors can directly or indirectly affect the quality, the quantity and the diversity of floral resources and thus the food sources of bees (e.g., Roger et al., 2017b). This makes the abundance, distribution/availability, quality and diversity of these resources potentially a main proximal pressure explaining bee population trends (Roulston and Goodell, 2011; Vaudo et al., 2015).
Bees obtain their carbohydrate nutrient intake mainly from nectar, and their protein and lipid from pollen (Roulston and Cane, 2000; Nicolson, 2011). The chemical composition of pollen is highly variable across floral species, between 2–60 and 1–20% for protein and lipid contents, respectively (Roulston and Cane, 2000; Vaudo et al., 2020). Field and semi-field studies showed that this chemical composition can be related to bee health (e.g., honey bee A. mellifera: Alaux et al., 2010; Brodschneider and Crailsheim, 2010; Di Pasquale et al., 2013, mason bee Osmia bicornis, Bukovinszky et al., 2017). Generalist bees seem able to assess pollen chemical quality and balance multiple macronutrient resources when making foraging decisions (Vaudo et al., 2016, 2018; Kraus et al., 2019; Ruedenauer et al., 2020). Based on a large quantity and diversity of samples, Vaudo et al. (2020) showed that honey bees collected pollens between 1:1 and 2:1 protein to lipid (P:L) ratio. This species appears to occupy a different nutritional space compared to Bombus impatiens and Osmia cornifrons, which collect at P:L ratios of 4:1 and 2:9, respectively. Furthermore, to satisfy the food intake of colonies with numerous individuals, honey bees must collect large amounts of pollen. Therefore, honey bees collect pollen from generalist, open floral morphologies such as mass blooming trees (e.g., Quercus sp., Salix sp., Prunus sp.) and wild herbs with high production of pollen (e.g., Asteraceae), which may have a nutritional make up that falls in the lower P:L values (i.e., 1–3:1 P:L) (Vaudo et al., 2020). Bumblebees look much more picky in their choices, since many species mainly forage on Fabaceae pollen showing a high P:L ratio value (3.8 ± 0.5) (Leonhardt and Blüthgen, 2012; Wood et al., 2021). In contrast to honey bees and bumblebees, Osmia cornifrons, a solitary foraging bee with a short flight period, has mixed preferences for Rosaceae and Fabaceae pollen (Haider et al., 2014; Nagamitsu et al., 2018), with average P:L ratios of 1.6 ± 0.3 and 3.8 ± 0.5, respectively.
Regarding chemical profiles, particular lipids and proteins seem more also important in bee nutritional requirement. For example, sterols (e.g., β-sitosterol) are essential to synthetize ecdysteroid, involved in the molting of the larvae and the maturation of the ovaries of female imago. In case of sterol deficiency, a delay in molting can be observed (Regali, 1996). Additionally, a good amino acid balance is also crucial for the bee development (Moerman et al., 2016). They are involved in growth, survival, flight ability or in immunity (Regali, 1996; Carter et al., 2006; Moerman et al., 2016). Some amino-acids (methionine, lysine, threonine, histidine, leucine, isoleucine, valine, phenylalanine, tryptophan) and sterols (24-methylenecholestrol and β-sitosterol) cannot be synthetised by the bee and are therefore considered as essential, meaning that it is necessary to obtain them through pollen consumption (De Groot, 1953; Svoboda et al., 1978; Behmer and Nes, 2003).
Experimental studies in controlled conditions have confirmed that the nutritional quality of pollen (e.g., the concentration of protein, and lipids, sterols, and amino acids) can have an impact on the development and mortality of bumblebees (e.g., Taseï and Aupinel, 2008a; Vanderplanck et al., 2014; Moerman et al., 2016, 2017; Barraud et al., 2020; Carnell et al., 2020) and mason bees (Sedivy et al., 2011; Eckhardt et al., 2014). The floral diversity of pollen diet does not seem to be the major factor of quality, as bumblebees develop better on high-quality monofloral diets compared to low-quality polyfloral diets (Moerman et al., 2017; Carnell et al., 2020). The pattern for honey bees appears to be similar at an individual level, with pollen quality (reflected by protein content) having an impact on the physiology and survival of adult honey bees (Brodschneider and Crailsheim, 2010; Di Pasquale et al., 2013; Frias et al., 2016; Omar et al., 2017; Li et al., 2019).
Overall, these results suggest that a loss of a part of the plant community, especially the families covering specific physiological requirement (e.g., Fabaceae), is more likely to affect bumblebees and solitary bees than honey bees (Leonhardt and Blüthgen, 2012). The more generalized the foraging behavior of a particular bee species, the more likely it is to be able to switch to alternative host plants and persist in an area, even if those host plants are of a lower nutritional quality (Roger et al., 2017b). However, there are multiple studies evaluating and comparing the development of various generalist bee species in controlled conditions on the same pollen diets (Moerman et al., 2016), and no study considering a broad diversity of bee clades (e.g., different bee tribes or bee families).
To address these knowledge gaps, we evaluated the effect of 8 pollen mixes of different qualities on key life-history traits regulated by pollen consumption in four European bee species (2 Apidae species: Apis mellifera (Apini) and Bombus terrestris (Bombini); 2 Megachilidae species: Osmia bicornis and O. cornuta). We first conducted palynological and chemical analysis (total protein, total lipid, amino acid, and sterol content) on these pollen blends to characterize their quality composition. We then developed experiments in controlled conditions and monitored the key life-history traits in bees fed with these pollen diets (e.g., survival for honey bees, brood production for bumblebees, and larva development for mason bees). We finally investigated which nutritional factors better explain bee health and development across the four species. Our hypothesis was that bee nutritional requirements are different across species.
This study was conducted on four common pollen generalist bee species recorded in Europe which are foraging in the same habitat for part of the year (Michez et al., 2019). We selected the Western honey bee Apis mellifera (Hymenoptera, Apidae, Apini), a domesticated eusocial species; the buff tailed bumblebee Bombus terrestris (Hymenoptera, Apidae, Bombini), a wild social species (Rasmont et al., 2008); and two mason bees (Osmia bicornis and O. cornuta; Hymenoptera, Megachilidae, Osmiini), wild solitary species. They are commonly used as model species because of their easy management in laboratory conditions. Bumblebee colonies were provided by Biobest NV (Westerlo, Belgium); honey bees were obtained from local apiaries at the “Institut National de la Recherche pour l'Agriculture, l'Alimentation et l'Environnement” (INRAE) in Avignon (France) and the mason bees were provided by Wildbiene + Partner (Switzerland).
Eight organic blends of honeybee-collected pollen were purchased from the company “Abeille heureuse” (France). Each pollen blend was gamma irradiated to avoid parasite infection, homogenized to reduce the risk of variation in palynological composition in each pollen treatment, and stored at −80°C before the experiment. In addition, a fraction of each pollen diet was lyophilized and stored at −20°C for palynological and chemical analyses (see below). Each pollen mix was named based on their palynological analysis, using the first letter of dominant pollen species (see Table 1).
For each pollen diet, the presence of pesticide residues was determined by liquid chromatography–tandem mass spectrometry (LC-MS/MS) with a limit of quantification of 0.01 mg/kg and a limit of detection of 0.005 mg/kg following the EN 15662:2018 procedure. Residues of 2,4 dimethylformamidine (DMF, degradation products of amitraz) and tau-fluvalinate were detected in all pollen blends but were below the limit of quantification. These compounds used as chemical treatments against the honeybee parasite Varroa destructor are consistently found in pollens (47.4 and 88.3% of trapped pollens for amitraz and tau-fluvalinate, respectively; Mullin et al., 2010; Calatayud-Vernich et al., 2019) and are considered as relatively safe for honeybees with an oral LD50 of 75 μg/bee for amitraz (contact exposure) and 45 μg/bee for tau-fluvalinate (oral exposure) (US EPA, 2021).
One gram of pollen sample was inserted and centrifuged in a 50 ml centrifuge tube and then dissolved in 20 ml of distilled water. Using a Pasteur pipette, a drop of sediment was placed on a microscope slide and spread out over an area of about 18 × 18 mm. After drying, the sediment was included in one drop of glycerine jelly and covered with the cover slip. Examination under the microscope were performed with 400X magnification. After a first general check to identify all the pollen types in the slide, a second read of the slide was carried out until 500 pollen grains were counted. Abortive, irregular, or broken pollen grains were still counted if they could be identified.
Recognition of pollen type was based on comparison between the observed pollen forms and those present in the CREA-AA collection of reference slides (built from anthers of identified plants). For each pollen type, the percentage of each species with respect to the total number of counted pollen grains was calculated.
Pollen protein concentration was measured using the Bradford assay according to Vaudo et al. (2020). We added 1.5 mL of 0.1 M NaOH to ~1 mg of pollen sample (dry weight), and conducted the Bradford assay with the Bio-Rad Protein Assay Kit microassay 300 μL microplate protocol using bovine γ-globulin as the protein standard (Bio-Rad Laboratories, Inc., Hercules, CA). We used three technical replications for each biological replication and measured absorbance at 595 nm using a SpectraMax 190 spectrophotometer (Molecular Devices, LLC, Sunnyvale, CA). Protein concentrations were calculated using polynomial 2nd analysis from the protein standards.
Pollen lipid concentrations were determined using a modified protocol from Van Handel and Day (1988). In 2.0 mL microcentrifuge tubes, we added 200 μL 2% sodium sulfate and 1.6 mL chloroform/methanol to ~1 mg of each pollen sample (dry weight) before a 5 min centrifugation. Supernatant was transferred to a clean glass tube with 600 μL deionised water, and centrifuged for 5 min. We separated the top carbohydrate/water/methanol fraction and the remaining chloroform fraction was used for lipid analysis. The lipid/chloroform fraction was left overnight in a fume hood to completely evaporate the solvent. We added 200 μL sulfuric acid to the sample and heated at 100°C for 10 min. Then, 5 mL vanillin/phosphoric acid reagent was added. We used three 300 μL technical replications for each biological replication and measured absorbance at 525 nm. Lipid concentrations were calculated using polynomial 2nd analysis from vegetable oil standards. Pollen concentrations of protein and lipids are reported as μg nutrient/mg pollen, and subsequent P:L ratios were determined for each diet.
For the analysis of total amino acids, 1 mL of hydrolysis solution (6N HCl, 0.1% phenol and 500 μM norleucine) was added to 3–5 mg (dry weight) of pollen (Vanderplanck et al., 2014) and then incubated for 24 h at 110°C. The hydrolysate was evaporated until dryness under vacuum in a boiling bath at 100°C. Afterwards, 1 mL of the sodium citrate buffer pH 2.2 was added into the tube. The sample solution was poured in an HPLC vial after filtration (0.2 μm filter), and each amino acid was measured separately with an ion-exchange chromatograph. A post-column ninhydrin reaction produced colored derivatives, which was monitored via a UV detector. For amino acid quantification, norleucine was used as internal standard. This analysis includes essential amino acids that bee cannot synthesize, as well as the non-essential ones. The essential amino acids were established by De Groot (1953) for honey bees; namely arginine, histidine, isoleucine, leucine, lysine, methionine, phenylalanine, threonine, tryptophan and valine.
Before each analysis, pollen samples were divided into a minimum of three samples (i.e., 20 mg (dry weight) per analytical replicate). Sterols were quantified by GC-FID after extraction and purification according to the method described by Vanderplanck et al. (2011). The multi-step procedure can be summarized as follows: (i) saponification with 2M methanolic potassium hydroxide, (ii) extraction of the unsaponifiable portion with diethylether and several water washings, (iii) solvent evaporation, (iv) fractionation of the unsaponifiable portion by TLC, (v) trimethylsilylation of the sterols (scraped from the silicagel), and (vi) separation by GC. The total sterol content was determined considering all peaks above the limit of quantification [(LOQ); LOQ = 9.6 ng/1.2 μl injected] whose retention time were between cholesterol and betulin (internal standard). Individual sterols were quantified on the basis of peak areas from analyses. Under the present analytical conditions applied, campesterol and 24-methylenecholesterol co-eluted. Therefore, the results are pooled for these two compounds. Compounds were identified according to their retention times by comparison with those of sunflower oil as reference. The identifications were corroborated by GC-FID (Vanderplanck et al., 2011).
As the three genera (i.e., Apis, Bombus, and Osmia) show very different life cycles and behavior, they could not be tested following the same protocol in laboratory conditions. Thus, we developed different experimental setup for each of the three bee genera.
In honeybees, pollen is mostly consumed by young adult bees. Its consumption enables the development of mandibular glands (Camilli et al., 2020) and especially hypopharyngeal glands (Crailsheim et al., 1992), where jelly is produced to feed larvae, the queen and drones (Crailsheim, 1992). We therefore tested the influence of pollen quality at the individual level on the fresh weight of individual heads, which is highly correlated to the volume of acini from the hypopharyngeal glands (Hrassnigg and Crailsheim, 1998). We also measured the survival rate of bees. Experiments were performed in the spring. To obtain 1-day-old bees, brood frames containing late-stage pupae were taken from eight healthy colonies normally treated against the parasitic mite Varroa destructor (Apis mellifera ligustica × Apis mellifera mellifera), and were placed overnight into an incubator under controlled conditions [34°C, 50–70% of relative humidity (RH)]. The next day, newly-emerged bees (<1 day old) were collected, mixed and groups of 40 bees were placed in cages (10.5 × 7.5 × 11.5 cm) (Pain, 1966). Caged bees, kept in an incubator (30°C and 50–70% RH), were provided ad libitum with water, candy (Apifonda® + powdered sugar) and one of the pollen diets (n = 10 cages per experimental group) (Figure 1A). Pollen diets were replaced every day for 10 days. To simulate as much as possible colony rearing conditions, caged bees were provided with a Beeboost® (Ickowicz, France), releasing one queen-equivalent of queen mandibular pheromone per day. Each day, pollen diets were weighed to determine the amount of pollen consumed per day and per bee. Pollen collection was corrected for evaporation, which was estimated by placing two samples of each pollen mixture in the same incubator for 24 h. Bee mortality was recorded every day for 44 days by counting and removing dead bees from cages. On day 7, 9 bees were sampled from each cage and stored at −80°C. The fresh weight of heads was then measured on individual bees (n = 9 bees per cage giving a total of 90 bees per experimental group).
Figure 1. Experimental set-ups for (A) honeybees, (B) bumblebees and (C) mason bees. Caged honeybees were provided with water, candy, and one of the pollen diets. Five bumblebees (workers) were placed in plastic boxes provided with pollen and syrup. Eggs of mason bees developed in cell culture plates of 48 wells filled with 400 mg of pollen.
We tested the impact of pollen on bumblebees at micro-colony level. Such a method to test the nutritive value of pollen diets has been shown to be a good estimate of queenright colony development at least under laboratory conditions with food ad libitum (Taseï and Aupinel, 2008b). A total of five queen-right colonies of 100 Bombus terrestris workers were used to build up 80 queen-less micro-colonies of five workers, placed in plastic boxes (8 × 16 × 16 cm) (Figure 1B). This number of individuals per micro-colony has been repeatedly used and is assumed to be the most reliable for assessing diet effects (Gradish et al., 2013; Moerman et al., 2016; Roger et al., 2017a; Vanderplanck et al., 2018; Klinger et al., 2019). Micro-colonies were then distributed in the different conditions (n = 10 micro-colonies for each experimental treatment). All micro-colonies were maintained in the same room in constant darkness at 26 ± 2°C with a relative humidity of 60–65%. They were manipulated under red light to minimize disturbance (Sadd, 2011) for a period of 28 days. Pollen diets were provided ad libitum to the micro-colonies as candies (mixed pollen with sugar syrup). New pollen candies were provided every 2 days, while the previous ones were weighed to assess the pollen collection. Pollen collection was corrected for evaporation by monitoring the weight of two samples of each diet placed in the rearing room for 48 h. To estimate the performance and development of bumblebee micro-colonies, we measured: (i) the total pollen and syrup collections, which can impact brood production and development (e.g., Plowright et al., 2008; Sutcliffe and Plowright, 2008); (ii) colony growth after 28 days of development [i.e., mass of individuals from all brood stages (eggs, larvae, pupae, non-emerged, and emerged males)] (Vanderplanck et al., 2014, 2018). For each micro-colony, all the measured parameters were divided by the total mass of the five workers to standardize the results and avoid potential effect of worker activities related to their size (i.e., consumption and brood care) (Cnaani and Hefetz, 1994). Additionally, we calculated the pollen efficacy as the mass of total offspring divided by the total pollen collection to estimate the colony performance.
We tested the impact of the pollen diet on the two species of mason bees at the larval stage. Standard mason bee nesting plates were installed close to the laboratory on the campus of the University of Mons (Belgium). A total of 1,000 individuals were released next to the nests. At regular time intervals, nests were opened and investigated for brood cell production. After 3 weeks, offspring were collected at the egg stage to avoid the consumption of the original pollen supply by the freshly emerged larvae. In the laboratory, cell culture plates of 48 wells were filled with 400 mg of prepared pollen (mixed pollen with sugar syrup) (Figure 1C). A fine brush was used to pick the egg from its original brood cell, and a single egg was placed cautiously onto each pollen provision (n = 35–40 eggs per treatment group). Plates were then placed into an incubator under controlled conditions (23°C, 60% RH). Developmental stage of larvae was assessed every day for 1 month and categorized into egg, larvae, feeding larvae, feeding and defecating larvae, spinning larvae, light cocoon, and cocoon. The time required to reach cocoon stage was used for the analyses. On average 90 days after cocoon development, each of them was taken out of the brood cells and weighed. Plates were then kept at 12°C for 4 days and at 4°C for ~120 days to mimic hibernation. After 140 days, all cocoons were again kept at 12°C for 4 days before moving them into an incubator (25°C, 60% RH) to elicit emergence. After emergence, adults were weighed (fresh weight) and determined as male or female.
Statistical analyses were run using statistical software R version 3.3.3 (R Core Team, 2017). To analyse the influence of pollen diets on honey bee survival, the number of dead bees per day and cage throughout the experiments were transformed into a survival table. A Cox proportional-hazards regression model was then used to compare the different diets, with R functions (coxph) and the package survival (Cox, 1970), considering the censored data of the bees that were alive at the end of the study. Pollen consumption and fresh weight of heads were analyzed using Kruskal–Wallis followed by Dunn's multiple comparison tests since data were not normally distributed.
For statistical analyses on bumblebees and Osmia data, two-way crossed analyses of variance (Two-Way crossed ANOVA) were conducted to evaluate the effect of diet. Since it is a parametric test, homoscedasticity (Bartlett test) and normality of the residuals (Shapiro test) were checked prior to the analyses. When violation occurred, data were log- or z-transformed to normality of residuals (“ztransform”function, R-package “GenABEL,” Lenth, 2009) prior to the test. Multiple pairwise comparisons were conducted using Tukey HSD post-hoc tests when ANOVA detected significant difference between pollen diets (p < 0.05).
Differences in nutritional content were assessed using either Kruskal–Wallis followed by Dunn's multiple comparison tests for proteins, lipids and P:L ratios, or perMANOVA for sterols and amino acids (Euclidean distance, 999 permutations, “adonis” command) after testing for multivariate homogeneity (“betadisper” command) (R-package vegan, Oksanen et al., 2020). Given the number of replicates, it was not possible to run a multiple pairwise comparisons on amino acids and sterol data. Differences were then visually assessed on UPGMA clusters using Euclidean distance and multiscale bootstrap resampling to calculate p-values for uncertainty in hierarchical cluster (R- package pvclust, Suzuki et al., 2019). Indicator compound analyses were performed to identify nutrients that were indicative of the groups defined based on the hierarchical cluster (“indval” command) (R-package labdsv, Roberts, 2019). All these analyses were conducted using data expressed as mg/g.
We analyzed the influence of each macro-nutrient in diets (i.e., proteins and lipids) on species performance using Response Surface Models (RSM). As it is standard for geometric analyses of nutrition, the models included the linear and quadratic components for protein and lipid intake as well as the interaction term between proteins and lipids as explanatory variables. Regarding the response variable, we used the proportion of individuals that survived for each diet treatment for Apis mellifera, the pollen efficacy for Bombus terrestris and the adult mass for Osmia bicornis and O. cornuta. As our response variables were measured in different units, we standardized each response variable to a mean of zero and a standard deviation of one using a Z transformation prior to analysis (≪ ztransform ≫ function from the GenABEL R-package; Ronnegard et al., 2016). We performed these analyses using the ≪ rsm ≫ function from the rsm R-package (Lenth, 2009), first considering lipid and protein content (RSM 1), then sterol and amino acid content (RSM 2).
The pollen mixtures were analyzed to verify the indication of the dominant pollen (Table 1). Analyses confirmed the palynological origin indicated by the seller only for two out of eight samples (samples C, S). Only four out of eight samples showed the presence of a dominant pollen (samples C, MS, BQ, SP); the other samples were found to be more or less heterogeneous mixtures of three or more pollen (see Supplementary Figure S1).
Pollen mixes had different chemical composition (Table 2, perMANOVA, p < 0.001). Protein content varies from 209.61 to 397.66 mg/g of pollen (p < 0.001). C and S pollen blends had the significantly lowest protein content compared to other mixes (209.61 ± 9.13 and 214.86 ± 10.5 mg/g, respectively, all p < 0.03), whereas TSo pollen mix had the highest protein content (397.66 ± 11.5 mg/g, all p < 0.001). SP pollen mix had the lowest lipid content (45.72 ± 2.42 mg/g, all p < 0.001) while ST and QS pollen mixes had the significantly highest lipid content (89.93 and 82.87 mg/g, respectively, all p < 0.03). P:L ratio ranged from 2.93 to 3.44 for ST, C and S pollen mixes, to 6.01–6.19 for SP and TSo diets (all p < 0.001).
Total and essential amino acid content ranged from 109.73 mg/g (SP diet) and 233.28 mg/g (SP diet) to 52.57 mg/g (C diet) and 125.04 mg/g (C diet), respectively. UPGMA analyses identified two clusters: one composed of C diet only (Cluster A, Supplementary Figure S1A) with a higher proline concentration (p = 0.018), and a second cluster composed of all other diets (Cluster B, Supplementary Figure S1A) with a higher content of every amino acid except proline (all p < 0.017). The different diets displayed concentrations of total sterol from 4.54 mg/g (C diet) to 14.38 mg/g (ST diet). UPGMA analyses identified two clusters: one composed of ST and SP diets (Cluster B, Supplementary Figure S1B) with a higher 24-Methylenecholesterol concentration compared to the second cluster composed of all other diets (Cluster A, Supplementary Figure S1B, p = 0.021). Other significant differences out of these clusters have been identified: higher concentrations of Cholesterol and δ7-Stigmasterol in TSo diet (p = 0.024 and 0.01, respectively), a higher concentration of Stigmasterol in BQ diet (p = 0.007) and higher concentration of δ7-Avenasterol in ST diet (p = 0.007). Despite the low concentrations of 24-Methylenecholesterol and δ5-Avenasterol observed in C diet compared to other diets (Table 2), they were not defined as significant by our analytical model, probably due to the lack of replicates. Those differences were therefore considered as tendencies.
Significant differences in bee survival between pollen diets were observed with the following order from the least to the most beneficial pollen: TSo < MS – BQ – C < SP < ST - QS – S (Figure 2A). Pollen diets were not consumed equally (p < 0.01, Figure 2B). Bees consumed significantly more of the QS and BQ than TSo and S pollen mixes. The head weight was also affected by the type of pollen (p < 0.001, Figure 2C). Bees fed with TSo pollen diet had a lighter head than bees fed with S, QS, BQ, or ST pollen mixes. After normalization to the amount of consumed pollen, head weights were the lowest for QS and BQ pollens and the highest for TSo and S pollen mixes (p < 0.001; Supplementary Figure S2).
Figure 2. Influence of pollen diets on Apis mellifera. (A) Survival probability (n = 30 bees per cage and 10 cages per pollen regime), (B) pollen collection and (C) head weight. Boxes indicate the 1st and 3rd interquartile range with line denoting median (n = 30 pools of 3 bees per pollen). Whiskers include 90% of the individuals, beyond which each outlier are represented by circles. Different letters indicate significant differences between pollen diets (Kruskal-Wallis tests followed by Dunn's multiple comparison tests: p < 0.01).
The brood production was impacted by the diet (p < 0.01). Microcolonies fed with MS and S diets were more developed, in term of total brood mass after 28 days, compared to those fed with C diet (p = 0.026 and p = 0.022, respectively, Figure 3A). Pollen efficacy was also influenced by the different pollen diets. Microcolonies with bumblebees fed with MS, TSo, SP, S, and ST pollen diets produced more brood per gram of pollen consumed in 28 days compared to those fed with C pollen diet (all p < 0.04, Figure 3B). The different pollen mixes had a limited impact on resources collection. Bumblebees consume more of the TSo diet than the SP diet (p = 0.024, Figure 3C). No significant differences were observed between other diets nor regarding syrup collection (p > 0.05, Figures 3C,D).
Figure 3. Influence of pollen diets on Bombus terrestris. (A) Brood mass production (g), (B) pollen efficacy (ratio between brood mass and pollen collection), (C) pollen collection (g), and (D) syrup collection. Boxes indicate the 1st and 3rd interquartile range with line denoting median (n = 10 micro-colonies with 5 workers per pollen diet). Different letters indicate significant differences between pollen diets (ANOVA tests followed by Tukey's multiple comparison tests: p < 0.05).
Osmia bicornis developed differently depending of their diet (p < 0.001). Bees fed with C diet had a significantly higher development time compared to any other diets (all p < 0.027), with ~25 and 31 days required to reach cocoon stage for females and males, respectively (Figure 4A). In contrast, bees fed with BQ diet had the lowest development time (all p < 0.004), with 20 and 23 days required to reach cocoon stage for females and males, respectively (Figure 4A). Development time on other diets range from 22 to 27 days. Cocoons of larvae fed with C diet were significantly lighter compared to any other diets (all p < 0.002), with a mean weight of 0.118 ± 0.011 and 0.095 ± 0.012 g for females and males, respectively. Cocoons of larvae fed with MS diet were ~29, 12, and 11% heavier compared to those fed with C, TSo or ST diet (all p < 0.02), respectively (Figure 4C). Adult weight showed similar differences (Figure 4E). The time required to reach cocoon stage for Osmia cornuta was significantly lower when fed with BQ diet compared to any other diets (all p < 0.02, Figure 4B). No significant differences were observed among the other diets (all p > 0.05). Cocoons of larvae fed with C diet were significantly lighter compared to any other diets (all p < 0.021), with a mean weight of 0.102 ± 0.005 and 0.094 ± 0.011 g for females and males, respectively. Cocoons of larvae fed with SP diet were ~27% heavier compared to those fed with C diet, with a mean weight of 0.135 ± 0.012 and 0.115 ± 0.021 g for females and males, respectively. They were also significantly heavier than cocoons of larvae fed with QS, BQ, ST, and TSo diet (all p < 0.003, Figure 4D). Adult weight showed similar differences, but with no significant differences between C, Tso, and ST diets (all p > 0.07, Figure 4F).
Figure 4. Influence of pollen diets on (left) Osmia bicornis and (right) Osmia cornuta. (A,B) Time required for larvae to reach the cocoon stage (days), (C,D) cocoon weight (g) and (E,F) adult weight (g). Boxes indicate the 1st and 3rd interquartile range with line denoting median (n = 40 per pollen diet). Different letters indicate significant differences between pollen diets (ANOVA tests followed by Tukey's multiple comparison tests: p < 0.05).
RSM analyses showed that A. mellifera survival variations between diets were not explained by total amino acids (AAT) or sterols (RSM 2, Supplementary Table S2), but rather by protein and lipid content (RSM 1, Supplementary Table S2). On the other hand, observed differences between diet-dependant pollen efficacies in B. terrestris were not explained by protein or lipid content (RSM 1, Supplementary Table S2), but rather by AAT and sterols (RSM 2, Supplementary Table S2). O. bicornis female mass variations were explained by the protein content only, while male mass was explained by proteins, AAT and sterols (RSM 1, Supplementary Table S2). Finally, O. cornuta adult mass variations between diets were explained in the same way as for O. bicornis: proteins for females (RSM 1, Supplementary Table S2); proteins, AAT and sterols for males (RSM 2, Supplementary Table S2).
Chemical composition of the tested diets was different, despite some observed similarities. First, none of the pollen diets had a protein content lower than 21%, which is already an average amount: ~25 plant genera contain <15% of protein in pollen (Roulston and Cane, 2002). On the other hand, the protein-richest diet of our experiments contained nearly 40% of proteins (TSo diet). With more than 60 plant genera containing more than 40% of protein in pollen (Roulston and Cane, 2002), our diets are in the range of what can be find in natura, but no pollen with a particularly low or high protein content was tested here. As we used pollen blends and not monofloral diets, it was expected that no extreme values would be observed (Roger et al., 2017b). Lipids analyses also showed differences between the diets. Previous analyses showed that lipids can range from ~1 to 20% in total content (Roulston and Cane, 2000; Roulston et al., 2000). With a variation from 4.6 to 9% of total lipid content, our diets are again in range with no extreme values. Note that the lipid analysis method may not completely lyse the pollen grains. There may therefore be a slight difference between the results of the analysis and what can be assimilated by the bee.
Amino acids analyses showed similar amino acids profiles were similar between pollen blends. These results are in line with previous studies, suggesting that amino acid profiles are conserved among plants (Roulston and Cane, 2002; Weiner et al., 2010; Vanderplanck et al., 2014). However, differences between our diets have been observed in term of total and essential amino acids, and the amount of specific amino acids. In this regard, we have observed that C diet contains 54–72% less amino acids and 48–61% less total and essential amino acids, compared to other diets.
Finally, among our different diets, total sterol concentration and sterolic profiles were different with a lower total sterol content in the C diet. Those results corroborate with previous studies that showed a high variability in sterolic compounds concentrations across plant species (Vanderplanck et al., 2014, 2018).
B.terrestris, O.bicornis and O.cornuta worst performances were observed when fed with C diet, whereas a different result was recorded for A.mellifera, for which worst performance was observed when fed with TSo diet. A.mellifera performances fed with C diet were average, while TSo diet was good and average for B.terrestris and Osmia species, respectively. Diet effects were similar between O.bicornis and O.cornuta. As both species are included in the same genus, it can be expected that nutritional preferences are conserved.
Several studies pointed out protein and/or protein:lipid ratio as an indicator for pollen quality regarding developmental performances (Roulston and Cane, 2002; Smeets and Duchateau, 2003; Alaux et al., 2010; Nicolson, 2011). However, observed results and RSM analyses on Bombus terrestris showed that performance cannot be explained by protein, lipid or P:L ratio, while adult female mass of Osmia species is slightly affected by protein content. Some good diets (see S pollen mix) and bad diets (see C pollen mix) share the same protein, lipid, content and P:L ratio. Those results indicate a difference in nutritional abilities compared to A. mellifera for which RSM analyses highlighted an importance of protein and lipids for their survivability. Despite the common assumption that proteins are positively related to performances (Regali and Rasmont, 1995; Génissel et al., 2002; Roulston and Cane, 2002; Smeets and Duchateau, 2003; Alaux et al., 2010; Nicolson, 2011; Stabler et al., 2015), in our case, the only observed effects that can be related to protein content were a negative one on Apis mellifera when fed with a protein-rich diet (39.8%, TSo pollen mix) and a slight one on Osmia species that is difficult to identify as positive or negative, as both pollen with the highest (TSo) and lowest (C) protein content resulted in lighter adults compared to other diets. Interestingly, Roulston and Cane (2002) observed a higher mortality rate when Lasioglossum zephyrum individuals were fed with a diet containing more than 39% of total protein content. Moreover, it has already been shown that in laboratory conditions, mortality and ovary development in A.mellifera workers were negatively impacted when fed with pollen containing 32% of proteins compared to bees fed with 15% of proteins (Human et al., 2007). Other authors observed similar mortality results with protein-rich diet (Standifer, 1967; Herbert et al., 1977), suggesting that high levels of proteins can lead to deleterious effect.
Differences in amino acids content between the different diets did not impact A. mellifera, but seems to explain results observed with B. terrestis and Osmia species, with more than 3 times less pollen efficacy for B. terrestris, and up to −22 and −26% adult weight for O.bicornis and O.cornuta, respectively, when fed with C diet, which had the lowest amount of total and essential amino acids. These results are similar to those of Archer et al. (2021), who concluded that amino acid intake was positively correlated with bumblebee body mass, and underlined that the effects of total amino acids intake may depend on the blend of individual amino acids. Interestingly, bumblebees can perceive some amino acids via chemotactile antennal stimulation and distinguish different concentrations without being able to differentiate each amino acid (Ruedenauer et al., 2019). This way, bumblebees could therefore assess the overall quantity of some amino acids and adapt their foraging decisions. However, such strategy could also lead wrong decisions, i.e., a rich pollen in non-favorable amino acids proportions.
Finally, sterols analyses could explain observed results regarding B. terrestris and O. cornuta measured performance traits. Some sterolic compounds have already been reported to be positively correlated with larval growth (Vanderplanck et al., 2014). 24-methylenecholesterol is known to influence molting and ovary development (Svoboda et al., 1978, 1983; Human et al., 2007), while β-sitosterol and δ5-avenasterol are supposedly involved in metabolic pathways of B. terrestris or have a phagostimulant effect (Regali, 1996). Sterols in the C diet did not affect A. mellifera performances. However, these results could be explained by the performance traits tested in our experiment, which did not take into account reproductive performances. In this regard, it could be interesting to test the effect of diet on larval development and not only on adults, to better evaluate the sterols effect, known to have a positive effect on the larval development on other bee species. Nevertheless, TSo diet, which caused the lowest survival rate in A. mellifera, contained relatively high concentrations of cholesterol and δ7-stigmasterol. If no negative effect has been reported for cholesterol in the literature, maybe this compound was in excess for A. mellifera. On the other hand, δ7-stigmasterol have already been reported as not beneficial for bumblebees (Vanderplanck et al., 2018). A. mellifera could therefore be more sensitive to the presence of this compound compared to other species. Finally, O. bicornis reached cocoon stage quicker when fed with BQ pollen mix. This diet has a relatively high concentration of stigmasterol, suggesting a potential role in the larval development of this species. It has recently been reported by Ruedenauer et al. (2021) that bees can't taste sterols, limiting the bee ability to forage on plants with beneficial sterol composition or to avoid detrimental ones. Moreover, this work and previous studies showed that pollen consumption is not adjusted to compensate low nutritional quality of a diet (see Vanderplanck et al., 2014 for B. terrestris and Corby-Harris et al., 2018 for A. mellifera), suggesting that a diet with a detrimental sterol composition could be regularly consumed by the bees without them perceiving it and being able to adapt their consumption.
Our results show that, if the 8 diet effects are different among species, similarities can be noted. Because the best performances of each species were shared across different diets, we could not define only one specific favorable diet. However, a global profile could be defined by comparing the nutritional factors of these diets. Based on this and previous studies, a generally good diet would require a high concentration of amino acids, and of 24-methylenecholesterol, β-sitosterol and δ5-avenasterol (Vanderplanck et al., 2014). P:L ratio did not impact measured parameters of this study. While Vaudo et al. (2016) have shown an impact of the P:L ratio on foraging behavior, the effects of this ratio on colony development remain relatively unclear. More studies should be done to see if the foraging strategy in favor of the P:L ratio results in effects on colony development, by artificially modifying the protein and lipid content of the same pollen without affecting the other compounds. Low protein content did not affect bees in this study. However, as all diets contained more than 20% of protein, we cannot conclude that proteins are not playing a role in diet quality. Regarding the literature, we hypothesize that protein content is important up to a certain threshold that can be close to 20% depending on the species (Regali and Rasmont, 1995; Génissel et al., 2002; Taseï and Aupinel, 2008b; Alaux et al., 2010), and can become detrimental if in excess (Standifer, 1967; Herbert et al., 1977; Roulston and Cane, 2002; Human et al., 2007). Once the minimum protein requirements are met, this work suggest that amino acid and sterol compositions are playing a key role in reproductive performances.
This study does did consider every element that can alter the quality of a pollen, such as pollenkit digestibility or secondary metabolites (alkaloids, lactones, diterpenes, or cyanogenic glycosides) (Peng et al., 1985; Detzel and Wink, 1993; London-Shafir et al., 2003; Williams, 2003; Gunduz et al., 2008; Kempf et al., 2010; Sedivy et al., 2012; Gosselin et al., 2013), and did not evaluate directly the sterols effect on A. mellifera due to measured parameters (only adults evaluated). Further research is therefore needed to fully comprehend the importance of each pollen-quality driver and interspecific differences. However, our study overall supports the need of conserving/introducing plant diversity into agro-ecosystems to meet the nutritional preferences of different bee species.
The original contributions presented in the study are included in the article/Supplementary Material, further inquiries can be directed to the corresponding author/s.
AB and LB wrote the manuscript and conducted experiments on the bees. VL did all nutrient analyses. DS was involved in Apis experiments. YL and CA supervised Apis experiments and redaction. F-VG, FC, GS, and CC perfomed the palynological/pesticide residues analyses and wrote results about it. MV and DM supervised the whole work. All authors contributed to the article and approved the submitted version.
This research has received funding from the European Union's Horizon 2020 Research and Innovation Programme under grant agreement N° 773921 for the POSHBEE project.
The authors declare that the research was conducted in the absence of any commercial or financial relationships that could be construed as a potential conflict of interest.
All claims expressed in this article are solely those of the authors and do not necessarily represent those of their affiliated organizations, or those of the publisher, the editors and the reviewers. Any product that may be evaluated in this article, or claim that may be made by its manufacturer, is not guaranteed or endorsed by the publisher.
We acknowledge all people that helped us to carry out this experiment especially K. Przybyla, M. Folschweiller, E. Zambra, M. Gerard, D. Evrard, and M. Bonneville for their help during colony dissection. We thank also D. Evrard (University of Mons) for the maintenance of bumblebee breeding room.
The Supplementary Material for this article can be found online at: https://www.frontiersin.org/articles/10.3389/fsufs.2022.824750/full#supplementary-material
Alaux, C., Ducloz, F., Crauser, D., and Le Conte, Y. (2010). Diet effects on honeybee immunocompetence. Biol. Lett. 45, 562–565. doi: 10.1098/rsbl.2009.0986
Archer, C. R., Fähnle, J., Pretzner, M., Üstüner, C., Weber, N., Sutter, A., et al. (2021). Complex relationship between amino acids, fitness and food intake in Bombus terrestris. Amino Acids 53, 1545–1558. doi: 10.1007/s00726-021-03075-8
Banda, H. J., and Paxton, R. J. (1991). Pollination of greenhouse tomatoes by bees. Acta Horticult. 288, 191–198. doi: 10.17660/ActaHortic.1991.288.28
Barraud, A., Vanderplanck, M., Nadarajah, S., and Michez, D. (2020). The impact of pollen quality on the sensitivity of bumblebees to pesticides. Acta Oecol. 105:103552. doi: 10.1016/j.actao.2020.103552
Behmer, S. T., and Nes, W. D. (2003). Insect sterol nutrition and physiology: a global overview. Adv. Insect. Physiol. 31, 1–72. doi: 10.1016/S0065-2806(03)31001-X
Brodschneider, R., and Crailsheim, K. (2010). Nutrition and health in honey bees. Apidologie 41, 278–294. doi: 10.1051/apido/2010012
Bukovinszky, T., Rikken, I., Evers, S., Wäckers, F. L., Biesmeijer, J. C., Prins, H. H. T., et al. (2017). Effects of pollen species composition on the foraging behaviour and offspring performance of the mason bee Osmia bicornis (L.). Basic Appl. Ecol. 18, 21–30. doi: 10.1016/j.baae.2016.11.001
Calatayud-Vernich, P., Calatayud, F., Simó, E., Pascual Aguilar, J. A., and Picó, Y. (2019). A two-year monitoring of pesticide hazard in-hive: high honey bee mortality rates during insecticide poisoning episodes in apiaries located near agricultural settings. Chemosphere 232, 471–480. doi: 10.1016/j.chemosphere.2019.05.170
Cameron, S. A., Lozier, J. D., Strange, J. P., Koch, J. B., Cordes, N., Solter, L. F., et al. (2011). Patterns of widespread decline in North American bumble bees. Proc. Natl. Acad. Sci. U.S.A. 108, 662–667. doi: 10.1073/pnas.1014743108
Camilli, M. P., de Barros, D. C. B., Justulin, L. A., Tse, M. L. P., Orsi, R., and de, O. (2020). Protein feed stimulates the development of mandibular glands of honey bees (Apis mellifera). J. Apic. Res. 60, 165–171. doi: 10.1080/00218839.2020.1778922
Carnell, J. D., Hulse, R. A., and Hughes, W. O. H. (2020). A review of nutrition in bumblebees: the effect of caste, life-stage and life history traits. Adv. Insect. Phys. 59, 71–129. doi: 10.1016/bs.aiip.2020.09.003
Carter, C., Shafir, S., Yehonatan, L., Palmer, R. G., and Thornburg, R. (2006). A novel role for proline in plant floral nectars. Naturwissenschaften 93, 72–79. doi: 10.1007/s00114-005-0062-1
Cnaani, J., and Hefetz, A. (1994). The effect of workers size frequency distribution on colony development in Bombus terrestris. Insect. Sociaux 41, 301–307. doi: 10.1007/BF01242301
Corby-Harris, V., Snyder, L., Meador, C., and Ayotte, T. (2018). Honey bee (Apis mellifera) nurses do not consume pollens based on their nutritional quality. PLoS One 13:e0191050. doi: 10.1371/journal.pone.0191050
Cox, D. R. (1970). Regression models and life-tables. J. R. Stat. Soc. 34, 187–220. doi: 10.1111/j.2517-6161.1972.tb00899.x
Crailsheim, K. (1992). The flow of jelly within a honeybee colony. J. Comp. Physiol. B 162, 681–689. doi: 10.1007/BF00301617
Crailsheim, K., Schneider, L. H. W., Hrassnigg, N., Bühlmann, G., Brosch, U., Gmeinbauer, R., et al. (1992). Pollen consumption and utilization in worker honeybees (Apis mellifera carnica): dependence on individual age and function. J. Insect Physiol. 38, 409–419. doi: 10.1016/0022-1910(92)90117-V
Danforth, B. N., Cardinal, S., Praz, C., Almeida, E. A. B., and Michez, D. (2013). The impact of molecular data on our understanding of bee phylogeny and evolution. Annu. Rev. Entomol. 58, 57–78. doi: 10.1146/annurev-ento-120811-153633
De Groot, A. P. (1953). Protein and amino acid requirements of the honey bee (Apis mellifera L.). Physiol. Comp. Oecol. 3, 197–285.
Detzel, A., and Wink, M. (1993). Attraction, deterrence or intoxication of bees (Apis mellifera) by plant allelochemicals. Chemoecology 4, 8–18. doi: 10.1007/BF01245891
Di Pasquale, G., Salignon, M., Le Conte, Y., Belzunces, L. P., Decourtye, A., Kretzschmar, A., et al. (2013). Influence of pollen nutrition on honey bee health: do pollen quality and diversity matter? PLoS One 8:e72016. doi: 10.1371/journal.pone.0072016
Duchenne, F., Thébault, E., Michez, D., Gérard, M., Devaux, C., Rasmont, P., et al. (2020). Long-term effects of global change on occupancy and flight period of wild bees in Belgium. Glob. Chang. Biol. 26, 6753–6766. doi: 10.1111/gcb.15379
Eckhardt, M., Haider, M., Dorn, S., and Mu, A. (2014). Pollen mixing in pollen generalist solitary bees: a possible strategy to complement or mitigate unfavourable pollen properties? J. Anim. Ecol. 83, 588–597. doi: 10.1111/1365-2656.12168
Frias, B. E. D., Barbosa, C. D., and Lourenço, A. P. (2016). Pollen nutrition in honey bees (Apis mellifera): impact on adult health. Apidologie 47, 15–25. doi: 10.1007/s13592-015-0373-y
Garibaldi, L. A., Steffan-Dewenter, I., Winfree, R., Aizen, M. A., Bommarco, R., Cunningham, S. A., et al. (2013). Wild pollinators enhance fruit set of crops regardless of honey bee abundance. Science 339, 1608–1611. doi: 10.1126/science.1230200
Génissel, A., Aupinel, P., Bressac, C., Tasei, J. N., and Chevrier, C. (2002). Influence of pollen origin on performance of Bombus terrestris micro-colonies. Entomol. Exp. Appl. 104, 329–336. doi: 10.1046/j.1570-7458.2002.01019.x
Gosselin, M., Michez, D., Vanderplanck, M., Roelants, D., Glauser, G., and Rasmont, P. (2013). Does Aconitum septentrionale chemically protect floral rewards to the advantage of specialist bumblebees? Ecol. Entomol. 38, 400–407. doi: 10.1111/een.12032
Goulson, D., Nicholls, E., Botías, C., and Rotheray, E. L. (2015). Bee declines driven by combined stress from parasites, pesticides, and lack of flowers. Science 347:6229. doi: 10.1126/science.1255957
Gradish, A. E., Scott-Dupree, C. D., McFarlane, A. D., and Frewin, A. J. (2013). Too much work, not enough tarsi: group size influences Bombus impatiens (Hymenoptera: Apidae) worker reproduction with implications for sublethal pesticide toxicity assessments. J. Econ. Entomol. 106, 552–557. doi: 10.1603/EC12154
Gruber, B., Eckel, K., Everaars, J., and Dormann, C. F. (2011). On managing the red mason bee (Osmia bicornis) in apple orchards. Apidologie 42, 564–576. doi: 10.1007/s13592-011-0059-z
Gunduz, A., Turedi, S., Russell, R. M., and Ayaz, F. A. (2008). Clinical review of grayanotoxin/mad honey poisoning past and present. Clin. Toxicol. 46, 437–442. doi: 10.1080/15563650701666306
Haider, M., Dorn, S., Sedivy, C., and Müller, A. (2014). Phylogeny and floral hosts of a predominantly pollen generalist group of mason bees (Megachilidae : Osmiini). Biol. J. Linn. Soc. 111, 78–91. doi: 10.1111/bij.12186
Herbert, E. W., Shimanuki, J. H., and Caron, D. (1977). Optimum protein levels required by honey bees (Hymenoptera, Apidae) to initiate and maintain brood rearing. Apidologie 8, 141–146. doi: 10.1051/apido:19770204
Hrassnigg, N., and Crailsheim, K. (1998). Adaptation of hypopharyngeal gland development to the brood status of honeybee (Apis mellifera L.) colonies. J. Insect Physiol. 44, 929–939. doi: 10.1016/S0022-1910(98)00058-4
Human, H., Nicolson, S. W., Strauss, K., Pirk, C. W. W., and Dietemann, V. (2007). Influence of pollen quality on ovarian development in honeybee workers (Apis mellifera scutellata). J. Insect Physiol. 53, 649–655. doi: 10.1016/j.jinsphys.2007.04.002
Kempf, M., Reinhard, A., and Beuerle, T. (2010). Pyrrolizidine alkaloids (PAs) in honey and pollen-legal regulation of PA levels in food and animal feed required. Mol. Nutr. Food Res. 54, 158–168. doi: 10.1002/mnfr.200900529
Klinger, E. G., Camp, A. A., James, S. P., Cox-foster, D., and Lehmann, D. M. (2019). Bombus (Hymenoptera: Apidae) microcolonies as a tool for biological understanding and pesticide risk assessment. Environ. Entomol. 48, 1249–1259. doi: 10.1093/ee/nvz117
Kraus, S., Gomez-Moracho, T., Pasquaretta, C., Latil, G., Dussutour, A., and Lihoreau, M. (2019). Bumblebees adjust protein and lipid collection rules to the presence of brood. Curr. Zool. 65, 437–446. doi: 10.1093/cz/zoz026
Lenth, R. V. (2009). Response-surface methods in R, using RSM. J. Stat. Softw. 32, 1–17. doi: 10.18637/jss.v032.i07
Leonhardt, S. D., and Blüthgen, N. (2012). The same, but different: pollen foraging in honeybee and bumblebee colonies. Apidologie 43, 449–464. doi: 10.1007/s13592-011-0112-y
Li, J., Heerman, M. C., Evans, J. D., Rose, R., Li, W., Rodr, C., et al. (2019). Pollen reverses decreased lifespan, altered nutritional metabolism and suppressed immunity in honey bees (Apis mellifera) treated with antibiotics. J. Exp. Biol. 222:jeb202077. doi: 10.1242/jeb.202077
London-Shafir, I., Shafir, S., and Eisikowitch, D. (2003). Amygdalin in almond nectar and pollen - facts and possible roles. Plant Syst. Evol. 238, 87–95. doi: 10.1007/s00606-003-0272-y
Michener, C. D. (2007). The Bees of the World, second edition. Baltimore, MD: Johns Hopkins University Press.
Michez, D., Rasmont, P., Terzo, M., and Vereecken, N. J. (2019). Bees of Europe. Hymenoptera of Europe, Vol. 1. Paris, 552.
Moerman, R., Roger, N., De Jonghe, R., Michez, D., and Vanderplanck, M. (2016). Interspecific variation in bumblebee performance on pollen diet: New insights for mitigation strategies. PLoS ONE 11:e0168462. doi: 10.1371/journal.pone.0168462
Moerman, R., Vanderplanck, M., Fournier, D., Jacquemart, A. L., and Michez, D. (2017). Pollen nutrients better explain bumblebee colony development than pollen diversity. Insect Conserv. Divers. 10, 171–179. doi: 10.1111/icad.12213
Mullin, C. A., Frazier, M., Frazier, J. L., Ashcraft, S., Simonds, R., vanEngelsdorp, D., et al. (2010). High levels of miticides and agrochemicals in North American apiaries: implications for honey bee health. PLoS ONE 5:e9754. doi: 10.1371/journal.pone.0009754
Nagamitsu, T., Suzuki, M. F., Mine, S., Taki, H., Shuri, K., Kikuchi, S., et al. (2018). Effects of forest loss and fragmentation on pollen diets and provision mass of the mason bee, Osmia cornifrons, in central Japan. Ecol. Entomol. 43, 245–254. doi: 10.1111/een.12494
Nicolson, S. W. (2011). Bee food: the chemistry and nutritional value of nectar, pollen and mixtures of the two. African Zool. 46, 197–204. doi: 10.1080/15627020.2011.11407495
Nieto, A., Roberts, S. P. M., Kemp, J., Rasmont, P., Kuhlmann, M., García Criado, M., et al. (2014). European Red List of Bees. Luxembourg: Publication Office of the European Union.
Oksanen, J., Guillaume, F. B., Friendly, M., Kindt, R., Legendre, P., McGlinn, D., et al. (2020). vegan: Community Ecology Package. Available online at: https://cran.r-project.org/package=vegan (accessed October 2021).
Ollerton, J. (2017). Pollinator diversity: distribution, ecological function, and conservation. Annu. Rev. Ecol. Evol. Syst. 48, 353–376. doi: 10.1146/annurev-ecolsys-110316-022919
Omar, E., Abd-Ella, A. A., Khodairy, M. M., Moosbeckhofer, R., Crailsheim, K., and Brodschneider, R. (2017). Influence of different pollen diets on the development of hypopharyngeal glands and size of acid gland sacs in caged honey bees (Apis mellifera). Apidologie 48, 425–436. doi: 10.1007/s13592-016-0487-x
Pain, J. (1966). Note technique nouveau modèle de cagettes expérimentales pour le maintien d'abeilles en captivité. Les Ann. l'Abeille, INRA Ed. 9, 71–76. doi: 10.1051/apido:19660106
Peng, Y., Nasr, M. E., Marston, J. M., and Fang, Y. (1985). The digestion of dandelion pollen by adult worker honeybees. Physiol. Entomol. 10, 75–82. doi: 10.1111/j.1365-3032.1985.tb00021.x
Persson, A. S., Rundlöf, M., Clough, Y., and Smith, H. G. (2015). Bumble bees show trait-dependent vulnerability to landscape simplification. Biodivers. Conserv. 24, 3469–3489. doi: 10.1007/s10531-015-1008-3
Pitts-Singer, T. L., and Cane, J. H. (2011). The Alfalfa Leafcutting Bee, Megachile rotundata : the world's most intensively managed solitary bee. Annu. Rev. Entomol. 56, 221–237. doi: 10.1146/annurev-ento-120709-144836
Plowright, R. C., Thomson, J. D., Lefkovitch, L. P., and Plowright, C. M. S. (2008). An experimental study of the effect of colony resource level manipulation on foraging for pollen by worker bumble bees (Hymenoptera: Apidae). Can. J. Zool. 71, 1393–1396. doi: 10.1139/z93-192
Potts, S. G., Imperatriz-Fonseca, V., Ngo, H. T., Aizen, M. A., Biesmeijer, J. C., Breeze, T. D., et al. (2016). Safeguarding pollinators and their values to human well-being. Nature 540, 220–229. doi: 10.1038/nature,20588
R Core Team (2017). R: A Language and Environment for Statistical Computing. Vienna: R Foundation for Statistical Computing. Available online at: https://www.R-project.org/ (accessed October 2021).
Rasmont, P., Coppee, A., Michez, D., and De Meulemeester, T. (2008). An overview of the Bombus terrestris (L. 1758) subspecies (Hymenoptera: Apidae). Ann. La Soc. Entomol. Fr. 44, 243–250. doi: 10.1080/00379271.2008.10697559
Rasmont, P., Devalez, J., Pauly, A., Michez, D., and Radchenko, V. G. (2017). Addition to the checklist of IUCN European wild bees (Hymenoptera: Apoidea). Ann. La Soc. Entomol. Fr. 53, 17–32. doi: 10.1080/00379271.2017.1307696
Regali, A. (1996). Contribution à l'étude des besoins alimentaires en stéroïdes de Bombus terrestris (L.). PhD thesis, Université de Mons, Mons.
Regali, A., and Rasmont, P. (1995). Nouvelles méthodes de test pour l'évaluation du régime alimentaire chez des colonies orphelines de Bombus terrestris (L.) (Hymenoptera, Apidae). Apidologie 26, 273–281. doi: 10.1051/apido:19950401
Roberts, D. W. (2019). labdsv: Ordination and Multivariate Analysis for Ecology. Available online at: https://cran.r-project.org/package=labd (accessed October 2021).
Roger, N., Michez, D., Wattiez, R., Sheridan, C., and Vanderplanck, M. (2017a). Diet effects on bumblebee health. J. Insect Physiol. 96, 128–133. doi: 10.1016/j.jinsphys.2016.11.002
Roger, N., Moerman, R., Carvalheiro, L. G., Aguirre-Guitiérrez, J., Jacquemart, A. L., Kleijn, D., et al. (2017b). Impact of pollen resources drift on common bumblebees in NW Europe. Glob. Chang. Biol. 23, 68–76. doi: 10.1111/gcb.13373
Ronnegard, L., McFarlane, S. E., Husby, A., Kawakami, T., Ellegren, H., and Qvarnstrom, A. (2016). Increasing the power of genome wide association studies in natural populations using repeated measures: evaluation and implementation. Methods Ecol. Evol. 7, 792–799. doi: 10.1111/2041-210X.12535
Roulston, T. H., and Cane, J. H. (2000). Plant systematics pollen nutritional content and digestibility for animals. Plant Syst. Evol. 222, 187–209. doi: 10.1007/BF00984102
Roulston, T. H., and Cane, J. H. (2002). The effect of pollen protein concentration on body size in the sweat bee Lasioglossum zephyrum (Hymenoptera: Apiformes). Evol. Ecol. 16, 49–65. doi: 10.1023/A:1016048526475
Roulston, T. H., Cane, J. H., and Buchmann, S. L. (2000). What governs protein content of pollen: pollinator preferences, pollen–pistil interactions, or phylogeny? Ecol. Monogr. 70, 617–643. doi: 10.1890/0012-9615(2000)070[0617:WGPCOP]2.0.CO;2
Roulston, T. H., and Goodell, K. (2011). The role of resources and risks in regulating wild bee populations. Annu. Rev. Entomol. 56, 293–312. doi: 10.1146/annurev-ento-120709-144802
Ruedenauer, F. A., Biewer, N. W., Nebauer, C. A., Scheiner, M., Spaethe, J., and Leonhardt, S. D. (2021). Honey bees can taste amino and fatty acids in pollen, but not sterols. Front. Ecol. Evol. 9:684175. doi: 10.3389/fevo.2021.684175
Ruedenauer, F. A., Leonhardt, S. D., Lunau, K., and Spaethe, J. (2019). Bumblebees are able to perceive amino acids via chemotactile antennal stimulation. J. Comp. Physiol. A 205, 321–331. doi: 10.1007/s00359-019-01321-9
Ruedenauer, F. A., Raubenheimer, D., Kessner-Beierlein, D., Grund-Mueller, N., Noack, L., Spaethe, J., et al. (2020). Best be(e) on low fat: linking nutrient perception, regulation and fitness. Ecol. Lett. 23, 545–554. doi: 10.1111/ele.13454
Sadd, B. M. (2011). Food-environment mediates the outcome of specific interactions between a bumblebee and its trypanosome parasite. Evolution 65, 2995–3001. doi: 10.1111/j.1558-5646.2011.01345.x
Sedivy, C., Andreas, M., and Dorn, S. (2011). Closely related pollen generalist bees differ in their ability to develop on the same pollen diet: evidence for physiological adaptations to digest pollen. Funct. Ecol. 25, 718–725. doi: 10.1111/j.1365-2435.2010.01828.x
Sedivy, C., Piskorski, R., Müller, A., and Dorn, S. (2012). Too low to kill: concentration of the secondary metabolite ranunculin in buttercup pollen does not affect bee larval survival. J. Chem. Ecol. 38, 996–1002. doi: 10.1007/s10886-012-0153-3
Smeets, P., and Duchateau, M. J. (2003). Longevity of Bombus terrestris workers (Hymenoptera: Apidae) in relation to pollen availability, in the absence of foraging. Apidologie 34, 333–337. doi: 10.1051/apido:2003026
Stabler, D., Paoli, P. P., Nicolson, S. W., and Wright, G. A. (2015). Nutrient balancing of the adult worker bumblebee (Bombus terrestris) depends on the dietary source of essential amino acids. J. Exp. Biol. 218, 793–802. doi: 10.1242/jeb.114249
Standifer, L. (1967). A comparison of the protein quality of pollens for growth-stimulation of the hypopharyngeal glands and longevity of honey bees, Apis mellifera L. (Hymenoptera: Apidae). Insectes Soc. 14, 415–426. doi: 10.1007/BF02223687
Sutcliffe, G. H., and Plowright, R. C. (2008). The effects of pollen availability on development time in the bumble bee Bombus terricola K. (Hymenoptera: Apidae). Can. J. Zool. 68, 1120–1123. doi: 10.1139/z90-166
Suzuki, R., Terada, Y., and Shimodaira, H. (2019). pvclust: Hierarchical Clustering with P-values Via Multiscale Bootstrap Resampling. Available online at: https://cran.r-project.org/package=pvclust (accessed October 2021).
Svoboda, J. A., Herbert, E. W., and Thompson, M. J. (1983). Definitive evidence for lack of phytosterol dealkylation in honey bees. Experientia 39, 1120–1121. doi: 10.1007/BF01943139
Svoboda, J. A., Thompson, M. J., Robbins, W. E., and Kaplanis, J. N. (1978). Insect steroid metabolism. Lipids 13, 742–753. doi: 10.1007/BF02533755
Taseï, J., and Aupinel, P. (2008a). Nutritive value of 15 single pollens and pollen mixes tested on larvae produced by bumblebee workers (Bombus terrestris, Hymenoptera: Apidae). Apidologie 39, 397–409. doi: 10.1051/apido:2008017
Taseï, J., and Aupinel, P. (2008b). Validation of a method using queenless Bombus terrestris micro-colonies for testing the nutritive value of commercial pollen mixes by comparison with queenright colonies. J. Econ. Entomol. 101, 1737–1742. doi: 10.1603/0022-0493-101.6.1737
US EPA (2021). ECOTOX Database. Available online at: https://cfpub.epa.gov/ecotox/ (accessed October 2021).
Van Handel, E., and Day, J. F. (1988). Assay of lipids, glycogen and sugars in individual mosquitoes: correlations with wing length in field-collected Aedes vexans. J. Am. Mosq. Control Assoc. 4, 549–550.
Vanderplanck, M., Decleves, S., Roger, N., Decroo, C., Caulier, G., Glauser, G., et al. (2018). Is non-host pollen suitable for generalist bumblebees? Insect Sci. 25, 259–272. doi: 10.1111/1744-7917.12410
Vanderplanck, M., Michez, D., Vancraenenbroeck, S., and Lognay, G. (2011). Micro-quantitative method for analysis of sterol levels in honeybees and their pollen loads. Anal. Lett. 44, 1807–1820. doi: 10.1080/00032719.2010.526271
Vanderplanck, M., Moerman, R., Rasmont, P., Lognay, G., Wathelet, B., Wattiez, R., et al. (2014). How does pollen chemistry impact development and feeding behaviour of polylectic bees? PLoS ONE 9:e86209. doi: 10.1371/journal.pone.0086209
Vaudo, A. D., Farrell, L. M., Patch, H. M., Grozinger, C. M., and Tooker, J. F. (2018). Consistent pollen nutritional intake drives bumble bee (Bombus impatiens) colony growth and reproduction across different habitats. Ecol. Evol. 8, 5765–5776. doi: 10.1002/ece3.4115
Vaudo, A. D., Patch, H. M., Mortensen, D. A., Tooker, J. F., and Grozinger, C. M. (2016). Macronutrient ratios in pollen shape bumble bee (Bombus impatiens) foraging strategies and floral preferences. Proc. Natl. Acad. Sci. U.S.A. 113, E4035–E4042. doi: 10.1073/pnas.1606101113
Vaudo, A. D., Tooker, J. F., Grozinger, C. M., and Patch, H. M. (2015). Bee nutrition and floral resource restoration. Curr. Opin. Insect Sci. 10, 133–141. doi: 10.1016/j.cois.2015.05.008
Vaudo, A. D., Tooker, J. F., Patch, H. M., Biddinger, D. J., Coccia, M., Crone, M. K., et al. (2020). Pollen protein: lipid macronutrient ratios may guide broad patterns of bee species floral preferences. Insects 11:132. doi: 10.3390/insects11020132
Velthuis, H. H. W., and Doorn, A. van. (2006). A century of advances in bumblebee domestication and the economic and environmental aspects of its commercialization for pollination. Apidologie 37, 421–451. doi: 10.1051/apido:2006019
Vray, S., Rollin, O., Rasmont, P., Dufrêne, M., Michez, D., and Dendoncker, N. (2019). A century of local changes in bumblebee communities and landscape composition in Belgium. J. Insect Conserv. 23, 489–501. doi: 10.1007/s10841-019-00139-9
Weekers, T., Marshall, L., Leclercq, N., Wood, T. J., Cejas, D., Drepper, B., et al. (2022). Dominance of honey bees is negatively associated with wild bee diversity in commercial apple orchards regardless of management practices. Agric. Ecosyst. Environ. 323:107697. doi: 10.1016/j.agee.2021.107697
Weiner, C. N., Hilpert, A., Werner, M., Linsenmair, K. E., and Blüthgen, N. (2010). Pollen amino acids and flower specialisation in solitary bees. Apidologie 41, 476–487. doi: 10.1051/apido/2009083
Williams, N. M. (2003). Use of novel pollen species by specialist and generalist solitary bees (Hymenoptera: Megachilidae). Oecologia 134, 228–237. doi: 10.1007/s00442-002-1104-4
Winfree, R. (2010). The conservation and restoration of wild bees. Ann. N. Y. Acad. Sci. 1195, 169–197. doi: 10.1111/j.1749-6632.2010.05449.x
Keywords: bees, pollen, nutrition, bumble bee, honey bee, Osmia, nutrients
Citation: Barraud A, Barascou L, Lefebvre V, Sene D, Le Conte Y, Alaux C, Grillenzoni F-V, Corvucci F, Serra G, Costa C, Vanderplanck M and Michez D (2022) Variations in Nutritional Requirements Across Bee Species. Front. Sustain. Food Syst. 6:824750. doi: 10.3389/fsufs.2022.824750
Received: 29 November 2021; Accepted: 11 January 2022;
Published: 04 February 2022.
Edited by:
Kimberly Ann Stoner, Connecticut Agricultural Experiment Station, United StatesReviewed by:
T'Ai Roulston, University of Virginia, United StatesCopyright © 2022 Barraud, Barascou, Lefebvre, Sene, Le Conte, Alaux, Grillenzoni, Corvucci, Serra, Costa, Vanderplanck and Michez. This is an open-access article distributed under the terms of the Creative Commons Attribution License (CC BY). The use, distribution or reproduction in other forums is permitted, provided the original author(s) and the copyright owner(s) are credited and that the original publication in this journal is cited, in accordance with accepted academic practice. No use, distribution or reproduction is permitted which does not comply with these terms.
*Correspondence: Alexandre Barraud, YWxleGFuZHJlLmJhcnJhdWRAdW1vbnMuYWMuYmU=; Lena Barascou, bGVuYS5iYXJhc2NvdUBpbnJhZS5mcg==
†These authors have contributed equally to this work
‡These authors have equally supervised this work
Disclaimer: All claims expressed in this article are solely those of the authors and do not necessarily represent those of their affiliated organizations, or those of the publisher, the editors and the reviewers. Any product that may be evaluated in this article or claim that may be made by its manufacturer is not guaranteed or endorsed by the publisher.
Research integrity at Frontiers
Learn more about the work of our research integrity team to safeguard the quality of each article we publish.