- 1Food Science Department, Laval University, Quebec, QC, Canada
- 2Center for Research in Plant Innovation (CRIV), Laval University, Quebec, QC, Canada
- 3Institute on Nutrition and Functional Foods (INAF), Laval University, Quebec, QC, Canada
Hydrogen peroxide (H2O2) is a reactive oxygen species (ROS) which participates in the signal transduction responses of plants toward biotic and abiotic stresses. Therefore, the objective of this study was to link the exposure of low doses of H2O2 to the improvement of the phytochemical composition of broccoli florets, in particular the content of glucosinolates (GLS), and hydroxy-cinnamates (HCA) without affecting the quality parameters of the vegetable. A dose of 1.25 mM H2O2 applied for 180 min was effective (hormetic) in maintaining the color of broccoli florets, which was also compared with a higher dose of 5.0 mM H2O2 applied for 180 min. The intensity of the treatments was related to respiration rate, which was monitored for 21 d at 4◦C along to weight loss. The initial respiration rate of florets exposed to both doses of H2O2 was significantly (p < 0.0001) higher relative to controls and resulted in weight loss in florets treated with the hormetic dose. The antioxidant capacity of florets, measured indirectly as Oxygen Radical Absorbance Capacity (ORAC) and ascorbic acid (AA), decreased in florets exposed to both doses. The concentration of glucobrassicins, aliphatic GLS, and HCA was consistently higher in florets treated with the two doses, compared to non-exposed florets. The enhancement of these compounds was accompanied by the over expression, immediately (6 h) after treatments, of tryptophan N-hydroxylase 2 (CYP79B3), dihomomethionine N-hydroxylase (CYP79F1), and phenylalanine ammonia-lyase (PAL) genes. Overall, the tested doses of H2O2 positively influenced the augmentation of indole-type and aliphatic GLS, as well as HCA in broccoli florets.
Introduction
Hydrogen peroxide (H2O2) is a colorless, odorless, pungent non-radical reactive oxygen species (ROS) liquid and a strong oxidizer compound. Due to its small size, and relatively long half-life (1 mS) compared with other ROS, H2O2 is a key component of signal transduction responses in plants to biotic and abiotic stresses (Foyer et al., 1997; Petrov and Van Breusegem, 2012). Hydrogen peroxide is produced in plants during the electron transport chain in cell organelles including mitochondria and chloroplasts by superoxide and superoxide dismutase, as well as apoplastic oxidases (Smirnoff and Arnaud, 2019). Diffusion of H2O2 across the cell membrane is restricted; thus, evidence suggests that aquaporins can transport the molecule across these structures (Bienert et al., 2006; Bienert and Chaumont, 2014; Wang et al., 2020). Once inside the cell, H2O2 is removed by the antioxidant enzyme system consisting of ascorbate peroxidase, glutathione peroxidase, peroxiredoxin, and catalase (Smirnoff and Arnaud, 2019).
At low concentrations, H2O2 is related with downstream signaling events including calcium mobilization, protein phosphorylation and gene expression (Neill et al., 2002). In contrast, high concentrations can be toxic to the cells leading to programmed cell death (Gechev and Hille, 2005). This is due, in part, to the fact that when high concentrations of H2O2 are not scavenged, hydroxy radicals can be produced by Haber-Weiss reaction with transition metals (Asada, 1992). These compounds are among the most highly reactive molecules known (Babbs et al., 1989). The effect of hydroxy radicals includes the attack on amino acid residues in proteins, protein degradation, formation of carbonyl derivatives, -S-S-bonding and damage to membranes by lipid peroxidation (Shen et al., 1997).
Exogenous applications of H2O2 have been used to cope with other stresses in crops on the pre-harvest phase. In Soybean (Glycine max L.), water loss was alleviated, and antioxidant-related enzyme activity increased when 1 mM of H2O2 was sprayed on leaf samples (Guler and Pehlivan, 2016). Similarly, a sprayed solution of 1.5 mM H2O2 on cucumber seedlings was effective for the following development of plant resistance to drought stress, observed by the accumulation of proline, and the alleviation of membrane lipid peroxidation (Sun et al., 2016). Prior to storage, in the postharvest phase, exogenous H2O2 treatments are used for the disinfection of fresh produce as an alternative to chlorine (Abadias et al., 2011; de Siqueira Oliveira et al., 2018). The obvious advantage of H2O2 over chlorine is the absence of residues since it decomposes rapidly into oxygen and water. Therefore, H2O2 has been generally recognized as safe (GRAS) by the U.S. Food and Drug Administration (FDA) (de Siqueira Oliveira et al., 2018).
However, the impact of H2O2 on the phytochemical composition of postharvest products has been less explored. Even less explored has been the effect of H2O2 on sulfur-derived compounds. Yet, H2O2 is associated with cell signaling processes and is also an essential part of the defense-related mechanisms in plants and horticultural products (Foyer et al., 1997; Neill et al., 2002; Cheeseman, 2007; Petrov and Van Breusegem, 2012; Lin et al., 2016; Smirnoff and Arnaud, 2019). Furthermore, it has been previously observed that the expression of genes related to glucosinolate synthesis, in particular the expression of tryptophan N-hydroxylase 2 (CYP79B3), responsible for the synthesis of indole-type glucosinolates, has been increased by other oxidative abiotic stresses such as heat and ultraviolet (UV) radiation (Duarte-Sierra et al., 2017, 2019). Therefore, we hypothesize that H2O2 is a crucial element in the synthesis of secondary metabolites related to defense responses in Brassicas, and that its external application can be used to increase the concentration of glucosinolates (GLS) and hydroxycinnamic acids (HCA) in broccoli.
Thus, the aim of this study was to use low doses of H2O2 to induce bioactive compounds in broccoli florets (i.e., GLS and HCA) and to gain insight into their role in oxidative events based on the phenomenon of hormesis. That is: “an adaptive beneficial effect on any system due to the exposure to a low dose of any agent otherwise considered harmful at high dose” (Duarte-Sierra et al., 2020). To our knowledge, this is the first study to investigate the effect of low doses of H2O2 where the purpose is not disinfection, but the induction of bioactive compounds in broccoli florets.
Materials and Methods
Broccoli
Freshly harvested, commercially mature broccoli (Brassica oleracea L. var. italica “Diplomat”) heads (0.1–0.15 m diameter, 0.6 kg) were purchased from farms on Orleans Island, Quebec, Canada. The selection of plant material was previously described in detail by Duarte-Sierra et al. (2017). Briefly, florets were cut from the heads, maintaining a constant color and size (0.025–0.04 m diameter) among them by visual examination. Prior to H2O2 exposition, cut florets, including controls, were stored for 12 h in the dark at 4◦C/90–95% RH to reduce the influence of wounding and light stresses. Each experimental unit consisted of ~300 g of plant tissue, and sampling for all experiments was performed 6 h after the completion of treatments.
Determination of Hormetic Dose
The treatments were carried out by immersing broccoli flower stems in solutions of different concentrations of H2O2 at a depth of 2.0 cm: 0 mM (control) to 5 mM for 180 min (Figure 1A). The solutions of H2O2 were disposed into a plastic box of 0.1 m × 0.5 m × 0.3 m with a plastic grid inside the box to hold the stems. Later, the box was covered, and introduced to a cold chamber at 4◦C/90–95% RH. Non-exposed florets were similarly arranged and immersed in distilled water for 180 min. Once the treatments finished, florets were removed from the box and the remaining H2O2 solutions were drained. Subsequently, florets were poured in individual clean plastic containers inside the plastic boxes with distilled water (Figure 1B) in a cold chamber at 4◦C/90–95% RH for 21 d. In each box, two holes of ~0.005 m in diameter were drilled in the walls to allow O2 and CO2 exchange. The hormetic dose selection was based on the minimal total color change (ΔE) of florets (n = 9) at the end of the storage, after 27 d.
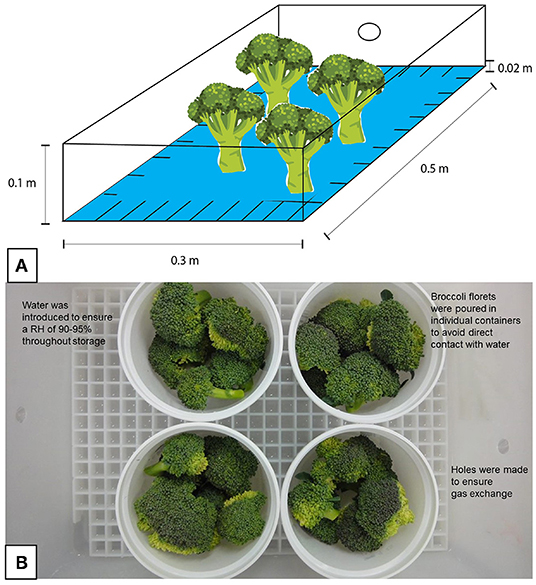
Figure 1. Arrangement and storage conditions of broccoli florets exposed to H2O2. (A) Broccoli florets were placed vertically inside a box with a grid inside, both made of plastic, and then different H2O2 solutions [0 mM (control) to 5 mM for 180 min] were poured to a depth of ~2.0 cm. (B) Once the exposure was concluded, the florets were stored in plastic containers with ~300 g of plant tissue (experimental unit), which were placed in boxes for storage. Water was introduced into these boxes to ensure high relative humidity during storage, and they were subsequently covered. To avoid CO2 accumulation, two holes were drilled in the sides of each box.
Measurements of Color, Weight Loss and Respiration Rate of Florets During Storage
Broccoli stems were placed in acrylic holders (0.02 m) to keep the florets upright during color measurements. The surface color of florets was analyzed with a colorimeter (Minolta CR200, Osaka, Japan) equipped with an 8 mm measuring head and a D 65 illuminant. Results were presented as the total color change (ΔE) from L*, a* and b* values . All measurements (n = 9) were done in nine times.
Weight loss was determined by weighing the florets (n = 9) per treatment, at the beginning, and the end of each sampling day (1, 2, 4, 7, 14, and 21 d). The rate of weight loss during storage for each treatment was calculated by the difference of the initial and final weights compared to the initial weight of the samples and expressed as a percentage. The respiration rate of broccoli florets (n = 9) expressed as nmol CO2 kg−1 s−1 was calculated from a defined mass of broccoli florets that were conditioned in airtight plastic containers of known volume and resolved by monitoring CO2 accumulation and O2 depletion using a headspace analyzer (CheckMate 9900, Cambridge, ON, Canada) under a static approach at regular intervals (0, 7, 14, and 21 d) in quadruplicates (four containers per treatment).
Chemical Analysis
The content of total phenolic compounds; total flavonoid compounds; ascorbic acid content (i.e., total, reduced, and oxidized); and Oxygen Radical Absorbance Capacity (ORAC); were analyzed from tissue powder (previously, the florets were flash frozen using liquid nitrogen, and then lyophilized) of control and exposed florets (n = 3) to both concentrations of H2O2 according to the methodology described in detail by Duarte-Sierra et al. (2017). All assays were determined spectrophotometrically on 96-well microplates. The total content of phenolic compounds was determined by the Folin-Ciocalteau method reported by Ainsworth and Gillespie (2007). Total flavonoid content was calculated using quercetin as standard by the colorimetric aluminum chloride method described by Lin and Tang (2007). Assay of reduced and total ascorbic acid was determined in accordance to the protocol established by Gillespie and Ainsworth (2007). ORAC was assayed by fluorescence spectroscopy, using Trolox as internal standard by the protocol established by Gillespie et al. (2007). The measurements were performed in triplicates and expressed as g kg−1 per equivalent of gallic acid, quercetin, ascorbic acid, or Trolox.
Glucosinolates and Hydroxycinnamic Acid Analysis
The extraction, separation, and quantification steps for the analysis of GLS and HCA from broccoli florets exposed to water (control) or H2O2 at 1.25 mM or 5.0 mM for 180 min were performed following the protocols outlined by Nadeau et al. (2012) and described in detail by Duarte-Sierra et al. (2017). In brief, extraction was done from freeze-dried broccoli samples (n = 3) mixed with an aqueous methanol solution (700 mL L−1) containing 800 μg of sinigrin as internal standard (samples were analyzed without sinigrin to determine its absence). Compounds in the extracts were separated by LC-MS (HP 1100 Series LC/MSD System, Palo Alto, CA) using a Phenomenex Synergi Hydro-RP column (250 mm × 2 mm, 80 Å) at 30◦C, with 10 mM ammonium acetate/formic acid pH 4.4 as mobile phase A; and acetonitrile as mobile phase B. The separation of the compounds of both classes was performed by retention times and molecular weights. The internal standard sinigrin corresponded to 8.3 min and 358.9 M, while the aliphatic type glucosinolate glucoraphanin started at 9.9 min and 437 M. Indole-type glucosinolate separation was achieved at 23–50.5 min, with molecular weights of 464–478 M. Separation of hydroxycinnamic acids (HCA) occurred from 51 to 55 min with molecular weights of 694–960 M. Finally, quantification of GLS and HCA was achieved at 224 nm and 330 nm, respectively, by a diode array detector.
Gene Expression Analysis
The description of primers used in this study, along with their corresponding accession numbers, and the methods used for the extraction and cleaning of RNA, as well as the synthesis of cDNA are given in detail by Duarte-Sierra et al. (2017). The expression was quantified (n = 3) on genes coding for enzymes related to the phenylpropanoid biosynthesis branch: chalcone synthase (CHS); flavonoid monooxygenase (F3H1); and phenylalanine ammonia-lyase (PAL). As well as those genes related to the biosynthesis of GLS compounds: tryptophan N-hydroxylase (CYP79B3); dihomo-methionine N-hydroxylase (CYP79F1); and phenylalanine N-hydroxylase (CYP79A2). Relative expression was quantified using a housekeeping gene (actin). The quantification of the expression was completed 6 h after treatments were completed.
Statistical Analysis
The experiment was based on a randomized complete design, analyzed by ANOVA at a significance level of 0.05, using the Statistical Analysis System version 9.3 (SAS Institute Inc. 2011. Base SAS® 9.3 Procedures Guide. Cary, NC, USA) which has been described by Duarte-Sierra et al. (2017). Comparisons for all pairs in hormetic dose determination, spectrophotometric analyses and gene experimentation were performed using Tukey-Kramer HSD. Least Significant Differences (LSD) at 0.05 were used to evaluate significant differences during storage. Statistical analysis for total phenols, total flavonoids, oxidized, reduced and total ascorbic acid, as well as ORAC were determined only at the end of the storage (i.e., 21 d).
Results and Discussion
Hormetic Dose of H2O2
The hormetic dose for H2O2 treatment was found to be 1.25 mM for 180 min (Figure 2A), although no significant differences on ΔE were found with 5 mM concentration for 180 min, which was selected as the high dose for subsequent experiments.
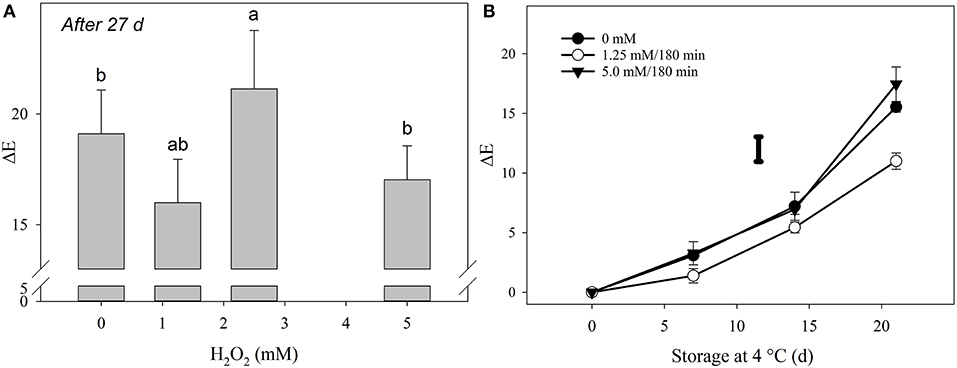
Figure 2. Determination of the total color change in broccoli florets exposed to H2O2. (A) Hormetic dose determination based on total color change (ΔE) of florets stored at 27 d at 4◦C/90–95% using four different H2O2 doses with 9 replicates per treatment (n = 9). Comparisons for all pairs using Tukey-Kramer HSD are shown with different letters. (B) Total color change (ΔE) of (•) untreated florets; treated with (◦) 1.25 mM/180 min; and treated with (▾) 5 mM/180 min during 21 d at 4◦C/RH of 90–95%. Bars are the means of 9 repetitions per treatment (n = 9) with ±SD. The individual bar represents the LSD value (0.05) = 2.08. Sampling on day 0 was performed 6 h after the end of the treatments.
The selection of the beneficial dose of H2O2 based on the color retention of broccoli florets was performed as a simple approach to anticipate beneficial responses on the phytochemical composition of the vegetable. Moreover, this strategy is also justified by the fact that visual appearance is one of the most important aspects of commodity marketing. However, it was subsequently found that the differences in GLS and HCA contents were not strikingly different in florets exposed to the hormetic and high doses. Therefore, dose selection based on quality parameters to predict phytochemical enhancement in vegetables may not be the best approach; at least didn't work for broccoli florets exposed to the doses employed in this experiment. Moreover, the data obtained, related to other physiological responses (i.e., respiration and weight loss) were also not sufficient to relate them to GLS or HCA contents.
In a review paper Duarte-Sierra et al. (2020) highlight the importance of categorizing doses (i.e., treatment intensity by application time) to establish the characteristic effects of an abiotic stressor on plant tissue. Obviously, this can be achieved by analyzing a large spectrum of doses, so it is important to continue research on the influence of applied doses of H2O2 to increase the concentration of phytochemical compounds related to human health. In addition, it is essential to continue the exploration for easily measurable parameters to predict the phytochemical content of plant products, since their determination is very demanding.
Physiological Characteristics
Color Evolution
Yellowing of broccoli florets was also successfully delayed by 1.25 mM/180 min of H2O2, but not with the most intense dose (Figure 2B). The literature regarding the application of H2O2 treatments to produce prior to postharvest storage is sparse and opposite effects on product quality in response to this molecule have been reported. Application of glycolic acid (1 μM) as a substrate for H2O2 synthesis by the action of glycolate oxidase in pear fruit tissue resulted in accelerated ripening, as denoted by increased softening and ethylene production (Brennan and Frenkel, 1977). Instead, the application of H2O2 in the vapor phase to grapes at 1.1 mg L−1 did not affect their visual appearance (Forney et al., 1991). Likewise, the application of 15 mM of H2O2 improved the overall visual appearance, ascorbic acid content, and increased the activity of antioxidant enzymes in white pepper fruits (Bayoumi, 2008).
Besides yellowing, a common defect affecting the visual appearance of products is browning. For instance, in longan fruits exposed to H2O2 (1.96 M for 20 min at 15◦C) an increase in the rate of superoxide radical production due to a decrease in the active oxygen-scavenging capacity of the fruit was documented (Lin et al., 2014). This radical flux could have damaged the cell membrane with the concomitant alteration of cell compartmentalization, resulting in the encounter of phenolic substrates with O2 and the formation of brown polymers. Although cellular damage to the floret membrane may have occurred in this report, as indicated by ORAC values and the oxidized form of ascorbic acid (Table 1), browning is not the primary visual concern in broccoli. Instead, chlorophyll bleaching due to lower doses of H2O2 might have been the main problem in this work.
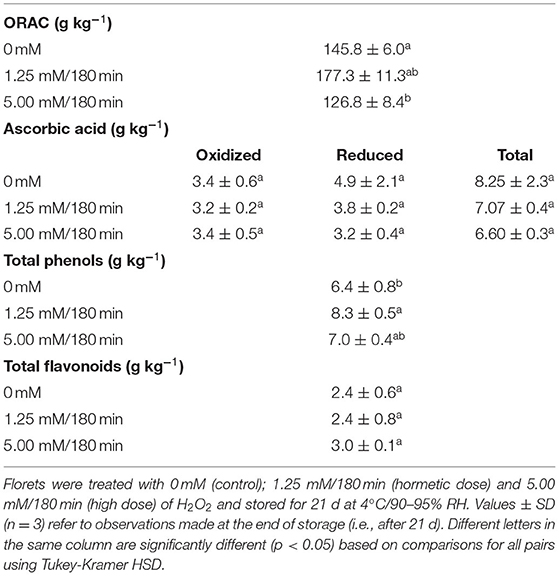
Table 1. Oxygen radical absorbance capacity (ORAC, Trolox equivalents), ascorbic acid (oxidized, reduced, total), total phenols (gallic acid equivalents), and total flavonoids (quercetin equivalents), contents in broccoli florets exposed to H2O2.
Indeed, the addition of 16.3 mM of H2O2, led to bleaching of chlorophyll a in spinach leaves (Chen et al., 2006). The latter has also been confirmed in other tissues. Chlorophyll extracted from orange (Citrus sinensis) was bleached by H2O2 at a concentration of 222 μM, pH 5.9 and the presence of resorcinol (a phenolic compound) (Huff, 1982). Therefore, it could be assumed that the high concentration of H2O2 at 5 mM/180 min was affecting chlorophyll a, which was probably not the case for the hormetic dose, but this was not confirmed. Nevertheless, it remains unclear why the florets treated with the hormetic dose of H2O2 retained more color compared to non-exposed plant material. As with UV-B radiation, it is possible that H2O2 may suppress chlorophyll-degrading enzymes, as low (i.e., nmol) concentrations of this molecule can increase susceptibility to proteolysis of enzymes (Fligiel et al., 1984). In addition, methionine can be degraded by H2O2 (Matsuo, 1953), which could be related to the low levels of endogenous ethylene in the tissues. Thus, although both doses could have affected proteins, amino acids, and ethylene, only the high dose could have bleached chlorophyll.
Respiration and Weight Loss
Hydrogen peroxide treatments affected the respiration rate of the florets. The highest CO2 production was recorded with the high dose of 5 mM/180 min at 1,148.2 nmol kg−1 s−1, followed by the hormetic dose of 1.25 mM/180 min at 1,110 nmol kg−1 s−1 at day 0. These values were significantly different (p < 0.0001) compared to the untreated florets, where the CO2 production was of 337 nmol CO2 kg−1 s−1 (Figure 3A). However, after 7 days of storage at 4◦C, similar values were observed among the three treatments groups (224.6–250 nmol kg−1 s−1) with no significant differences (p > 0.05) among them.
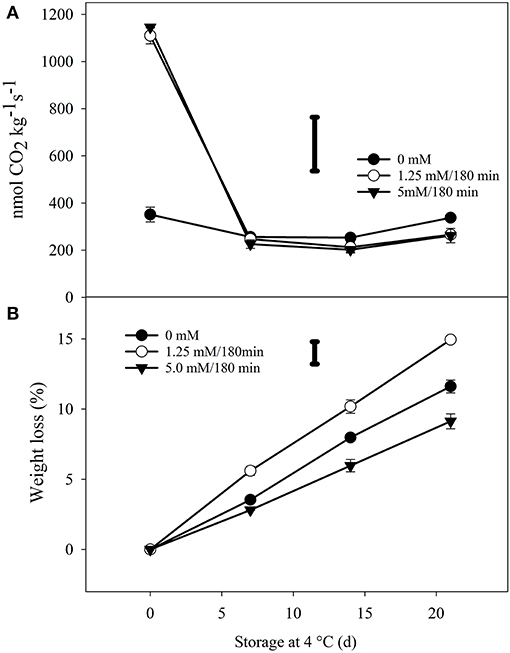
Figure 3. Progress of respiration rate and weight loss of H2O2 treated broccoli florets during storage of 21 d in the dark at 4◦C/90–95% RH. (A) The average production of carbon dioxide (CO2) of florets treated with three H2O2 doses: (•) untreated (0 mM); (◦) hormetic dose (1.25 mM/180 min); and (▾) high (5 mM/180 min) dose. Each point is the mean of 4 replicates per treatment (n = 4) and vertical bars represents standard deviation. (B) Weight loss (%) of florets during storage. Bars are the means of 9 replicates per treatment (n = 9) with ±SD. The bars in each graph represents the LSD value (0.05) = 228 (A) and 1.59 (B). Sampling on day 0 was performed 6 h after the end of the treatments.
The respiration rate can be used as an indicator for the metabolic activity of produce, and can be applied as a tool to maximize the longevity of produce during the postharvest storage (Mathooko, 1996). The respiration rate of broccoli at 5◦C, without any treatment, is considerably high (i.e., 374 nmol CO2 kg−1 s−1) compared to other commodities at the same temperature, hence postharvest storage has to be limited to short periods of time (Kader and Saltveit, 2002). In contrast, a rapid increase in respiration rate is normally a general adaptive process, where protective mechanisms are established by the depletion of energy (i.e., ATP) in produce (Duarte-Sierra et al., 2017). A transient increase in respiration rates has already been reported in produce exposed to other abiotic stresses. In blueberry fruit exposed to 0.2 μL L−1 of O3 for 1–2 d, increased respiration was complemented by ethylene production (Song et al., 2003). Similarly, transient increase in respiration has been observed in broccoli exposed to moist hot air at 41◦C/180 min, as well as in florets treated with 1.2 kJ m−2 UV-C (Duarte-Sierra et al., 2017, 2019). In all cases, the respiration rate of fresh produce returned to basal levels and no significant differences were observed during cold storage.
The transient increase in the rate of respiration of plant products imposed by oxidative stress not only depletes ATP in cells. It has also been found that high doses of H2O2 (1.96 M/20 min at 15◦C) applied to longan fruits for example, increased the enzymatic activity of cytochrome C oxidase as well as ascorbic acid oxidase, which contrasted with the decreased activity of NAD kinase and the low amounts of NADP and NADPH (Lin et al., 2016). This association of lower NADPH levels in H2O2-exposed fruit compared to non-exposed fruit, as well as the accumulation of NADH could have affected some metabolic pathways such as the Meyerhof pathway (EMP), as well as the tricarboxylic acid cycle (TCA) related to respiratory metabolism (Lin et al., 2016). An example of this was reported on Jiashi muskmelon (Cucumis melo L.), where relatively high doses of H2O2 (3–5% v/v), inhibited respiration (Chen et al., 2015).
In addition to the transient increase in respiration rates, weight loss was observed in florets exposed to H2O2. A greater weight loss was recorded with the hormetic dose of H2O2 (14.9%) compared to unexposed florets (11.6%). On the other hand, less weight loss was reported with the highest dose (9.1%) (Figure 3B). This suggests that the high dose of H2O2 probably causes tolerance to osmotic stress. Previously, this has been observed in maize (Zea mays L.) leaves exposed to low concentration of H2O2 (10 mM). Along with the increase in endogenous H2O2 concentration, polyamines, absicic acid (ABA), soluble sugars, and proline induced the osmotic stress resistance of detached leaves (Terzi et al., 2014). Furthermore, according to Bright et al. (2006) there is a strong relationship between endogenous H2O2, nitric oxide (NO), ABA and stomatal closure in Arabidopsis. Although not quantified, it is possible that the concentration of ABA increased with both doses of H2O2, since the concentration of indole-type glucosinolates also increased, and this plant signal is known to induce them (Frerigmann and Gigolashvili, 2014). Moreover, the highest dose of H2O2 might also have been able to promote osmotic stress resistance, and thus lessen the weight loss of florets exposed to 5 mM for 180 min.
Antioxidant Capacity of Florets
Hydrogen peroxide at 5.00 mM/180 min significantly (p < 0.05) reduced the total antioxidant capacity (i.e., ORAC) of treated florets compared to unexposed florets, as well as to florets exposed to the hormetic dose, after 21 days of storage (Table 1). This reduction was 13% compared to non-exposed florets (Table 1). By contrast, a 22% increase on ORAC was detected in the florets exposed to the hormetic dose, again with reference to the unexposed florets (Table 1). No significant differences related to total, reduced, or oxidized ascorbic acid (AA) were observed among treatments. However, a non-significant (p > 0.05) reduction of 34% in the amount of reduced AA, compared to non-exposed florets, was observed when these were exposed to the highest dose of H2O2 (Table 1). The amount of total phenols, on the other hand, increased significantly (p < 0.05) by 9% in florets exposed to the highest dose of 5 mM/180 min, and by 29% in florets exposed to the hormetic dose, relative to the control group (Table 1). However, no differences were found between the total flavonoid contents in the florets treated or not with H2O2.
In the context of this experiment, H2O2 was an abiotic stress and a stable ROS. Hydrogen peroxide can potentially generate free radicals in the presence of metal ions such as Fe2+ or metal ion-containing molecules via the Fenton reaction (Cheeseman, 2007). In general, the accumulation of ROS is accompanied by modifications in enzymatic and non-enzymatic mechanisms in cells that can lead to oxidative damage as well as other signaling responses (Janicka et al., 2019).
The most abundant class of antioxidants quantified in this experiment corresponded to total phenolic content (6.4–8.3 g kg−1), followed by total AA (6.6–8.25 g kg−1), and total flavonoids (2.4–3.0 g kg−1) (Table 1). Although, a higher concentration of total phenols was quantified compared to the total AA content in the tissue, this does not necessarily mean that the former has a higher antioxidant capacity. In fact, intracellular concentrations in the millimolar range are sufficient for AA to act as an electron donor/acceptor to react directly with ROS. (i.e., , 1O2, and ·OH) and its function of reducing H2O2 to H2O makes it one of the most relevant compounds for maintaining cell redox state (Hodges and Forney, 2003; Fisher, 2013). Moreover, AA depletion might be correlated with the intensity of the stress. For instance, in a different experiment, the amount of total AA on florets exposed to 5 μL L−1 of O3 for 180 min was significantly reduced at the end of the treatment (unpublished data). If this is the case, the reduction of total phenolics observed in this work could be related to their efficiency as electron donors, as well as to a probable quenching specificity of ROS produced by H2O2. Indeed, the ability of phenolic acids to scavenge free radicals produced by H2O2 is correlated with the number and position of hydroxyl groups within the aromatic ring (Sroka and Cisowski, 2003). However, the determination of specific phenols with this activity requires further investigation.
Glucosinolates and Hydroxy-Cinnamic Acids
A significant increase in total glucoraphanin and glucobrassicins content was observed in florets exposed to 1.25 and 5.0 mM H2O2/180 min compared to the control group (Figure 4). For glucoraphanin (aliphatic-type GLS), the analyzed concentration reached a maximum on the third day of storage for both treatments and remained constant thereafter. However, in the non-exposed florets, the concentration of this molecule decreased steadily at a rate of 0.05 g kg−1 per day. In addition, from day 3 to the end of storage, significant differences were observed in glucoraphanin titers of florets treated with 1.25 or 5.0 mM H2O2 both for 180 min with respect to non-exposed florets. For the hormetic dose, the increase was 27%, while at the highest dose, the induction was 29% (Figure 4A).
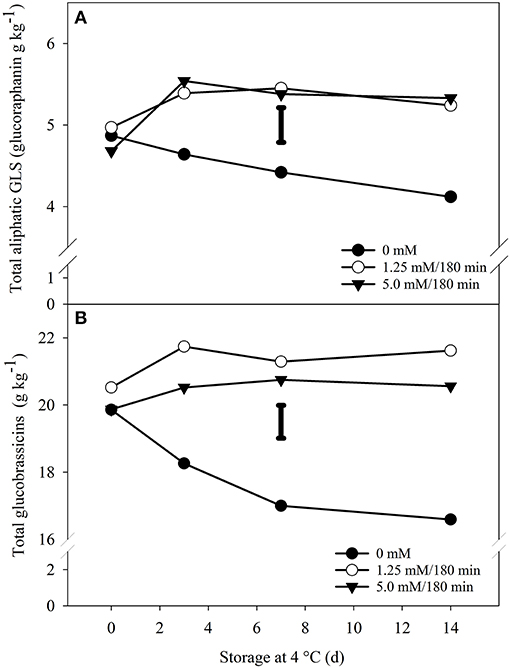
Figure 4. Effect of H2O2exposure on total aliphatic and indole-type glucosinolates of broccoli florets. Total aliphatic (glucoraphanin) (A) and indole-type (B) glucosinolates in broccoli florets exposed to three doses of H2O2, 0 mM (•), 1.25 mM/180 min (◦) and 5 mM/180 min (▾) during storage in the dark for 14 d at 4◦C. Values are g equivalents of sinigrin on a dry weight basis and represent the mean of 3 replicates per treatment (n = 3). The bars in each graph represents the LSD value (0.05) = 0.42 (A) and 0.98 (B). Sampling on day 0 was performed 6 h after the end of the treatments.
On the other hand, the levels of total glucobrassicins were more abundant than those of glucoraphanin (Figure 4), due to the addition of several indole-type glucosinolates. Nevertheless, the profile of this class of GLS was like that of glucoraphanin described above. That is, the concentration decreased in the control florets, although not in a linear fashion, and the concentrations in the exposed florets reached a maximum after 14 days of cold storage. For the hormetic dose, the increase was 30% compared to the control group in the same period (i.e., 14 d). While for the highest concentration, the stimulation was 23% (Figure 4B).
However, if the values of non-exposed broccoli at the beginning of storage (day 0) are compared to the values of the treatments at the end of storage (day 14), the induction percentages are more modest. For example, with glucoraphanin, these induction levels vary between 8 and 9%, while with total glucobrassicins, the levels vary between 8 and 4% (Figure 4). Following this approach, an exception was observed with 4-methoxyglucobrassicin, where the induction percentages were found to be more significant (Figure 5). Thus, the percentage of induction considering the unexposed control group as a reference, were of 12 and 13% for the hormetic and high doses, respectively. However, if we consider the difference that existed on day 0, with respect to the control group and the high dose of 5.0 mM, the increase would be 20% (Figure 5).
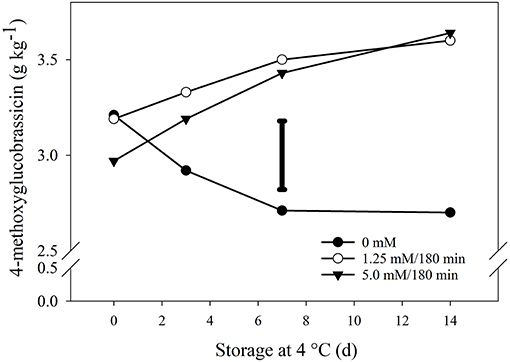
Figure 5. Impact of hydrogen peroxide on 4-methoxyglucobrassicin. Broccoli florets were exposed to three doses of H2O2, 0 mM (•), 1.25 mM/180 min (◦) and 5 mM/180 min (▾) during storage in the dark for 14 d at 4◦C. Values are g equivalents of sinigrin on a dry weight basis and represent the mean of 3 replicates per treatment (n = 3). The individual bar represents the LSD value (0.05) = 0.35. Sampling on day 0 was performed 6 h after the end of the treatments.
Total contents of HCA (1-sinapoyl-2-feruloyl gentibiose + 1,2-disynalpoyl-2-feruloyl gentibiose + 1,2,2-trisinalpoyl gentibiose + 1,2-disinapoyl gentibiose) on florets were also significantly altered by H2O2 treatments, although at a more conservative manner compared to GLS (Figure 6). After 14 days of storage, the concentration of total HCA in the florets treated with 1.25 and 5.0 mM H2O2/180 min was 3 and 8%, respectively (Figure 6). In fact, no significant differences were observed between broccoli exposed at the hormetic dose and those not exposed during this period. Moreover, total HCA contents also increased in the control group, which differs from that observed for glucosinolates, and contrasts with the decrease of this group in the florets exposed to the hormetic dose (Figure 6). Even so, there was a considerable and significant increase in the concentration of total HCA up to day 7 in the plant material exposed to both doses.
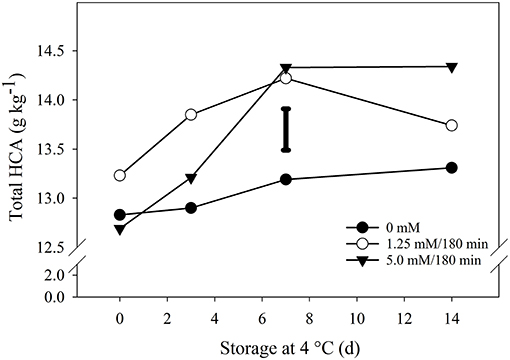
Figure 6. Impact of hydrogen peroxide on total hydroxy-cinnamates. Broccoli florets were exposed to 0 mM; 1.25 mM/180 min (hormetic dose); and 5.0 mM/180 min (high dose) H2O2, and then stored at 4◦C/90–95% RH for 14 d. Contents of 1,2-diferuloyl gentiobiose; 1,2-disinapoyl gentiobiose; 1,2,2-trisinalpoyl gentiobiose; 1,2-disynalpoyl-2-feruloyl gentiobiose; and 1-sinapoyl-2-feruloyl gentiobiose were quantified and combined as g equivalents of sinigrin on a dry and represent the mean of 3 replicates per treatment (n = 3). The individual bar represents the LSD value (0.05) = 0.43. Sampling on day 0 was performed 6 h after the end of the treatments.
Phytochemical enhancement in florets correlated with overexpression in genes encoding key enzymes in the GLS and HCA pathways. Overall, the relative gene expression of florets exposed to either the hormetic dose or the higher dose of H2O2 increased after 6 h of storage. The highest overexpression was observed in tryptophan N-hydroxylase 2 (CYP79B3) from florets exposed to the hormetic dose ≈4-fold, and to the highest dose ≈3.8-fold (Figure 7). Likewise, ≈3- and 3.5-fold overexpression of dihomeothionine N-hydroxylase (CYP79F1) was determined in florets exposed to hormetic and higher doses of H2O2, respectively. Furthermore, the overexpression of phenylalanine by-products was similar. The expression of genes encoding phenylalanine N-hydroxylase (CYP79A2) and phenylalanine ammonia lyase (PAL) was increased ≈2-fold with both doses of H2O2. Finally, more conservative overexpression levels were observed in flavanone 3-hydroxylase (F3H1), and chalcone synthase (CS) (Figure 7).
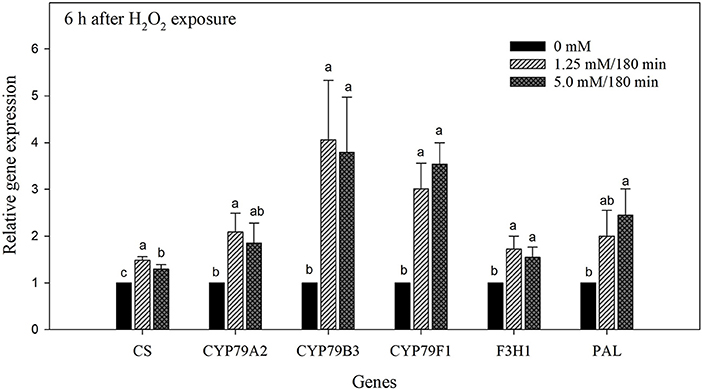
Figure 7. Gene expression analysis of broccoli florets exposed to H2O2 on day 0. Florets were treated with H2O2 at 0; 1.25 mM/180 min (hormetic dose); and 5 mM/180 min (high dose) stored at 4◦C/90–95% RH and the values were normalized against actin. Gene expression in broccoli florets exposed to H2O2 was measured after each treatment on chalcone synthase (CHS); phenylalanine N-hydroxylase (CYP79A2); tryptophan N-hydroxylase 2 (CYP79B3); dihomomethionine N-hydroxylase (CYP79F1); flavanone 3-hydroxylase (F3H1); and phenylalanine ammonia-lyase (PAL). Values were averaged and the standard deviation is presented with vertical bars, representing 3 replicates per treatment (n = 3). Comparisons for all pairs using Tukey-Kramer HSD are shown with different letters.
Hydrogen peroxide, at non-toxic levels, can be used as a tool to increase the tolerance of vegetables to other abiotic stresses by priming and regulating, through gentle control of production and scavenging, multiple pathways and gene expression (Hossain et al., 2015). In this study, H2O2 was used to induce the synthesis of secondary metabolites, especially glucosinolates. Although the extent of penetration of H2O2 into the broccoli stems submerged in this experiment is unknown, it can be anticipated that some volume was absorbed. This is probably due to its small size and long lifetime (among ROS), which allows the molecule to probably diffuse into different cellular structures (Petrov and Van Breusegem, 2012). However, this diffusion could be reduced by the different antioxidant compounds inside the cell (Neill et al., 2002). But once inside the apoplast, a series of sensors including redox-sensitive transcription factors (TFs), as well as the oxidation of methionine to methionine sulfoxide can sense H2O2 to initiate a series of downstream signals (Petrov and Van Breusegem, 2012).
One of the most important interdependent downstream signal reactions includes H2O2 and Ca2+ which is a crucial signaling element in guard cell responses (Hossain et al., 2015). In addition, this interaction is important for ABA-induced stomatal closure (Neill et al., 2002; Bright et al., 2006; Quan et al., 2008). The water retention observed with the higher dose of H2O2 applied to florets would probably lead to the accumulation of ABA (Figure 8). This hormone is commonly induced by abiotic stress, especially to drought stress, but may also mediate tolerance to ROS stress (Hossain et al., 2015). In addition, there is evidence that ABA can affect the concentration of secondary metabolites in Brassicas. For example, secondary metabolites (SM) including GLS, and flavonoids were induced in Brassica napus plants exposed to 10 μM of ABA. Among the GLS, 7-methylthioheptyl glucosinolate and 8-methylthiooctyl glucosinolate were significantly increased. Similarly, the flavonoids quercetin-3-(6″-malonyl)-glucoside and quercetin were strongly stimulated by the hormone (Zhu and Assmann, 2017).
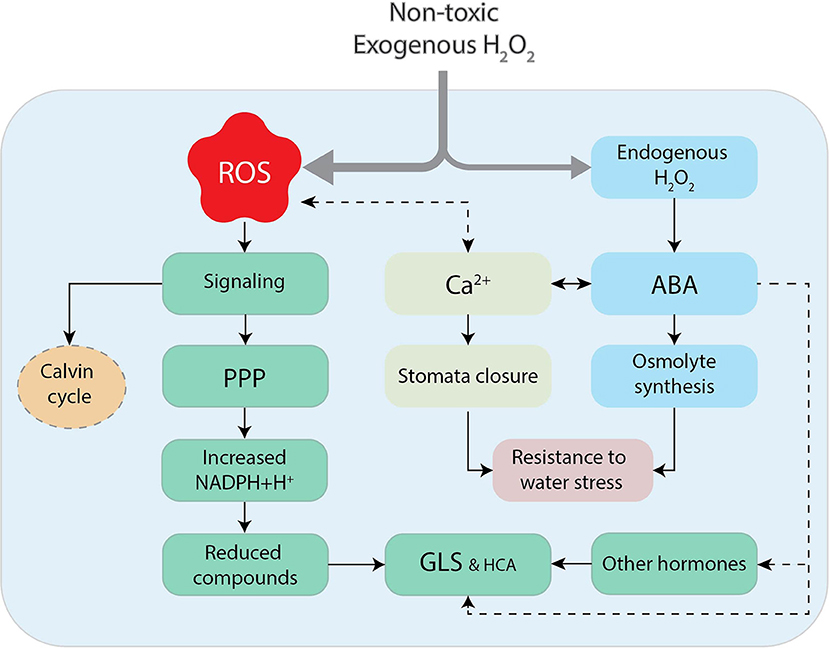
Figure 8. The hypothetical oversimplification of nontoxic exogenous application of H2O2 to broccoli florets. The exposure of florets to H2O2 could derive into the apparition of reactive oxygen species (ROS), serving as signals to reduce the Calvin cycle, favoring the accumulation reductive power (NADPH+H+) by the pentose phosphate pathway (PPP) and the concomitant production of reduced compounds such as glucosinolates (GLS) and hydroxycinnamates (HCA). Exogenous H2O2 could also produce the synthesis of endogenous H2O2, which in conjunction with intracellular Ca2+ and abscisic acid (ABA), led to stomata closure and the production of osmolytes, protecting the cell from water loss. The interaction of ABA with other plant signals (JA?) is another possibility for the enhancement of glucosinolates in broccoli exposed to abiotic stresses.
The hypothetical increase in ABA levels, and the concomitant closure of stomata, could also have decreased the consumption of reducing equivalents for CO2 fixation in the Calvin cycle. This could significantly increase the amount of reducing equivalents for the biosynthesis of reduced compounds (Selmar and Kleinwachter, 2013). Thus, the observed enhancement of GLS and HCA, could have been the result of NADPH+H+ accumulation (Figure 8).
Conclusions
Low doses of H2O2 can maintain the color of florets, as well as being used effectively to improve their phytochemical composition, especially indole-type GLS, and more specifically 4-methoxyglucobrassicin levels. Moreover, from a commercial point of view, the application of hormetic doses of H2O2 could be a simple form of disinfection, which also offers two significant benefits: (1) the delay of senescence, and (2) the possibility of obtaining functional horticultural products related to beneficial effects on human health.
Data Availability Statement
The original contributions presented in the study are included in the article/supplementary materials, further inquiries can be directed to the corresponding authors. Data set can be found at: doi.org/10.17632/wntkdkx2rb.1.
Author Contributions
MT contributed in the performance of experiments related to chemical analysis and gene expression. PA contributed to the experimental design and revision of the manuscript. JA supervised the research activities as well as the review of the publication. AD-S participated in the experimental design, experimental performance, drafting of the manuscript, and supervision of MT. All authors contributed to the article and approved the submitted version.
Funding
This study was supported by the Natural Science and Engineering Research Council (NSERC) and the Quebec Ministry of Agriculture, Fisheries and Food (MAPAQ) project 810335. AD-S acknowledges the award of scholarship (211363) from the National Council of Science and Technology of Mexico (CONACYT).
Conflict of Interest
The authors declare that the research was conducted in the absence of any commercial or financial relationships that could be construed as a potential conflict of interest.
Publisher's Note
All claims expressed in this article are solely those of the authors and do not necessarily represent those of their affiliated organizations, or those of the publisher, the editors and the reviewers. Any product that may be evaluated in this article, or claim that may be made by its manufacturer, is not guaranteed or endorsed by the publisher.
References
Abadias, M., Alegre, I., Usall, J., Torres, R., and Viñas, I. (2011). Evaluation of alternative sanitizers to chlorine disinfection for reducing foodborne pathogens in fresh-cut apple. Postharvest Biol. Technol. 59, 289–297. doi: 10.1016/j.postharvbio.2010.09.014
Ainsworth, E. A., and Gillespie, K. M. (2007). Estimation of total phenolic content and other oxidation substrates in plant tissues using Folin-Ciocalteu reagent. Nat. Protoc. 2, 875–877. doi: 10.1038/nprot.2007.102
Asada, K (1992). Ascorbate peroxidase – a hydrogen peroxide-scavenging enzyme in plants. Physiol. Plant. 85, 235–241. doi: 10.1111/j.1399-3054.1992.tb04728.x
Babbs, C. F., Pham, J. A., and Coolbaugh, R. C. (1989). Lethal hydroxyl radical production in paraquat-treated plants. Plant Physiol. 90, 1267–1270. doi: 10.1104/pp.90.4.1267
Bayoumi, Y. A (2008). Improvement of postharvest keeping quality of white pepper fruits (Capsicum annuum L.) by hydrogen peroxide treatment under storage conditions. Acta Biol. Szegediensis 52, 7–15. Available online at: http://abs.bibl.u-szeged.hu/index.php/abs/article/view/2572
Bienert, G. P., and Chaumont, F. (2014). Aquaporin-facilitated transmembrane diffusion of hydrogen peroxide. Biochim. Biophys. Acta 1840, 1596–1604. doi: 10.1016/j.bbagen.2013.09.017
Bienert, G. P., Schjoerring, J. K., and Jahn, T. P. (2006). Membrane transport of hydrogen peroxide. Biochim. Biophys. Acta Biomembr. 1758, 994–1003. doi: 10.1016/j.bbamem.2006.02.015
Brennan, T., and Frenkel, C. (1977). Involvement of hydrogen peroxide in the regulation of senescence in pear. Plant Physiol. 59, 411–416. doi: 10.1104/pp.59.3.411
Bright, J., Desikan, R., Hancock, J. T., Weir, I. S., and Neill, S. J. (2006). ABA-induced NO generation and stomatal closure in Arabidopsis are dependent on H2O2 synthesis. Plant J. 45, 113–122. doi: 10.1111/j.1365-313X.2005.02615.x
Cheeseman, J. M (2007). Hydrogen peroxide and plant stress: a challenging relationship. Plant Stress 1, 4–15.
Chen, G., Chen, J., Feng, Z., Mao, X., and Guo, D. (2015). Physiological responses and quality attributes of Jiashi muskmelon (Cucurbitaceae, Cucumis melo L.) following postharvest hydrogen peroxide treatment during storage. Eur. J. Hortic. Sci. 80, 288–295. doi: 10.17660/eJHS.2015/80.6.4
Chen, X.-B., Zhao, X.-H., Zhu, Y., Gong, Y.-D., Li, L.-B., Zhang, J.-P., et al. (2006). Hydrogen peroxide-induced chlorophyll a bleaching in the cytochrome b6f complex: a simple and effective assay for stability of the complex in detergent solutions. Photosyn. Res. 90, 205–214. doi: 10.1007/s11120-006-9118-0
de Siqueira Oliveira, L., Eça, K. S., de Aquino, A. C., and Vasconcelos, L. B. (2018). “Hydrogen peroxide (H2O2) for postharvest fruit and vegetable disinfection,” in Postharvest Disinfection of Fruits and Vegetables, ed M.W. Siddiqui (London: Academic Press), 91–99.
Duarte-Sierra, A., Forney, C. F., Michaud, D., Angers, P., and Arul, J. (2017). Influence of hormetic heat treatment on quality and phytochemical compounds of broccoli florets during storage. Postharvest Biol. Technol. 128, 44–53. doi: 10.1016/j.postharvbio.2017.01.017
Duarte-Sierra, A., Nadeau, F., Angers, P., Michaud, D., and Arul, J. (2019). UV-C hormesis in broccoli florets: preservation, phyto-compounds and gene expression. Postharvest Biol. Technol. 157, 110965. doi: 10.1016/j.postharvbio.2019.110965
Duarte-Sierra, A., Tiznado-Hernández, M. E., Jha, D. K., Janmeja, N., and Arul, J. (2020). Abiotic stress hormesis: an approach to maintain quality, extend storability, and enhance phytochemicals on fresh produce during postharvest. Comprehens. Rev. Food Sci. Food Safety 19, 3659–3682. doi: 10.1111/1541-4337.12628
Fisher, M. L. A (2013). Hydrogen peroxide treatment of fresh-cut spinach (Spinicia oleracea L.) and its effects on microbiological and quality parameters (M.Sc. Thesis). Acadia University, Wolfville, Nova Scotia, Canada.
Fligiel, S. E., Lee, E. C., McCoy, J. P., Johnson, K. J., and Varani, J. (1984). Protein degradation following treatment with hydrogen peroxide. Am. J. Pathol. 115, 418–425.
Forney, C. F., Rij, R. E., Denis-Arrue, R., and Smilanick, J. L. (1991). Vapor phase hydrogen peroxide inhibits postharvest decay of table grapes. HortScience 26, 1512–1514. doi: 10.21273/HORTSCI.26.12.1512
Foyer, C. H., Lopez-Delgado, H., Dat, J. F., and Scott, I. M. (1997). Hydrogen peroxide- and glutathione-associated mechanisms of acclimatory stress tolerance and signalling. Physiol. Plant. 100, 241–254. doi: 10.1111/j.1399-3054.1997.tb04780.x
Frerigmann, H., and Gigolashvili, T. (2014). MYB34, MYB51, and MYB122 distinctly regulate indolic glucosinolate biosynthesis in Arabidopsis thaliana. Mol. Plant 7, 814–828. doi: 10.1093/mp/ssu004
Gechev, T. S., and Hille, J. (2005). Hydrogen peroxide as a signal controlling plant programmed cell death. J. Cell Biol. 168, 17–20. doi: 10.1083/jcb.200409170
Gillespie, K. M., and Ainsworth, E. A. (2007). Measurement of reduced, oxidized and total ascorbate content in plants. Nat. Protoc. 2, 871–874. doi: 10.1038/nprot.2007.101
Gillespie, K. M., Chae, J. M., and Ainsworth, E. A. (2007). Rapid measurement of total antioxidant capacity in plants. Nat. Protoc. 2, 867–870. doi: 10.1038/nprot.2007.100
Guler, N. S., and Pehlivan, N. (2016). Exogenous low-dose hydrogen peroxide enhances drought tolerance of soybean (Glycine max L.) through inducing antioxidant system. Acta Biol. Hung. 67, 169–183. doi: 10.1556/018.67.2016.2.5
Hodges, D. M., and Forney, C. F. (2003). Postharvest ascorbate metabolism in two cultivars of spinach differing in their senescence rates. J. Am. Soc. Horticult. Sci. 128, 930–935. doi: 10.21273/JASHS.128.6.0930
Hossain, M. A., Bhattacharjee, S., Armin, S.-M., Qian, P., Xin, W., Li, H.-Y., et al. (2015). Hydrogen peroxide priming modulates abiotic oxidative stress tolerance: insights from ROS detoxification and scavenging. Front. Plant Sci. 6, 420. doi: 10.3389/fpls.2015.00420
Huff, A (1982). Peroxidase-catalysed oxidation of chlorophyll by hydrogen peroxide. Phytochemistry 21, 261–265. doi: 10.1016/S0031-9422(00)95247-6
Janicka, M., Reda, M., Napieraj, N., and Kabała, K. (2019). “Plant abiotic stress: function of nitric oxide and hydrogen peroxide,” in Nitric oxide and hydrogen peroxide signaling in higher plants, eds D. Gupta, J. Palma, and F. Corpas (Cham: Springer), 201–219. doi: 10.1007/978-3-030-11129-8_10
Kader, A. A., and Saltveit, M. (2002). “Respiration and gas exchange,” in Postharvest Physiology and Pathology of Vegetables, eds J. A. Bartz and J. K. Brecht (New York, NY: CRC Press), 7–32.
Lin, J.-Y., and Tang, C.-Y. (2007). Determination of total phenolic and flavonoid contents in selected fruits and vegetables, as well as their stimulatory effects on mouse splenocyte proliferation. Food Chem. 101, 140–147. doi: 10.1016/j.foodchem.2006.01.014
Lin, Y., Lin, H., Zhang, S., Chen, Y., Chen, M., and Lin, Y. (2014). The role of active oxygen metabolism in hydrogen peroxide-induced pericarp browning of harvested longan fruit. Postharvest Biol. Technol. 96, 42–48. doi: 10.1016/j.postharvbio.2014.05.001
Lin, Y.-X., Lin, Y.-F., Chen, Y.-H., Wang, H., Shi, J., and Lin, H.-T. (2016). Hydrogen peroxide induced changes in energy status and respiration metabolism of harvested longan fruit in relation to pericarp browning. J. Agric. Food Chem. 64, 4627–4632. doi: 10.1021/acs.jafc.6b01430
Mathooko, F. M (1996). Regulation of respiratory metabolism in fruits and vegetables by carbon dioxide. Postharvest Biol. Technol. 9, 247–264. doi: 10.1016/S0925-5214(96)00019-1
Matsuo, Y (1953). Degradation of methionine by hydrogen peroxide. Nature 171, 1021–1022. doi: 10.1038/1711021a0
Nadeau, F., Gaudreau, A., Angers, P., and Arul, J. (2012). Changes in the level of glucosinolates in broccoli florets (Brassica oleracea var. Italica) during storage following postharvest treatment with UV-C. Acta Horticult. 945, 145–148. doi: 10.17660/ActaHortic.2012.945.19
Neill, S., Desikan, R., and Hancock, J. (2002). Hydrogen peroxide signalling. Curr. Opin. Plant Biol. 5, 388–395. doi: 10.1016/S1369-5266(02)00282-0
Petrov, V. D., and Van Breusegem, F. (2012). Hydrogen peroxide-a central hub for information flow in plant cells. AoB Plants 2012, pls014. doi: 10.1093/aobpla/pls014
Quan, L. J., Zhang, B., Shi, W. W., and Li, H. Y. (2008). Hydrogen peroxide in plants: a versatile molecule of the reactive oxygen species network. J. Integr. Plant Biol. 50, 2–18. doi: 10.1111/j.1744-7909.2007.00599.x
Selmar, D., and Kleinwachter, M. (2013). Stress enhances the synthesis of secondary plant products: the impact of stress-related over-reduction on the accumulation of natural products. Plant Cell Physiol. 54, 817–826. doi: 10.1093/pcp/pct054
Shen, B., Jensen, R. G., and Bohnert, H. J. (1997). Mannitol protects against oxidation by hydroxyl radicals. Plant Physiol. 115, 527–532. doi: 10.1104/pp.115.2.527
Smirnoff, N., and Arnaud, D. (2019). Hydrogen peroxide metabolism and functions in plants. New Phytol. 221, 1197–1214. doi: 10.1111/nph.15488
Song, J., Fan, L., Forney, C. F., Jordan, M. A., Hildebrand, P. D., Kalt, W., et al. (2003). Effect of ozone treatment and controlled atmosphere storage on quality and phytochemicals in highbush blueberries. Acta Hortic. 417–423. doi: 10.17660/ActaHortic.2003.600.62
Sroka, Z., and Cisowski, W. (2003). Hydrogen peroxide scavenging, antioxidant and anti-radical activity of some phenolic acids. Food Chem. Toxicol. 41, 753–758. doi: 10.1016/S0278-6915(02)00329-0
Sun, Y., Wang, H., Liu, S., and Peng, X. (2016). Exogenous application of hydrogen peroxide alleviates drought stress in cucumber seedlings. South Afr. J. Bot. 106, 23–28. doi: 10.1016/j.sajb.2016.05.008
Terzi, R., Kadioglu, A., Kalaycioglu, E., and Saglam, A. (2014). Hydrogen peroxide pretreatment induces osmotic stress tolerance by influencing osmolyte and abscisic acid levels in maize leaves. J. Plant Interact. 9, 559–565. doi: 10.1080/17429145.2013.871077
Wang, H., Schoebel, S., Schmitz, F., Dong, H., and Hedfalk, K. (2020). Characterization of aquaporin-driven hydrogen peroxide transport. Biochim. Biophys. Acta Biomembr. 1862, 183065. doi: 10.1016/j.bbamem.2019.183065
Keywords: broccoli florets, hydrogen peroxide (H2O2), oxidative stress, glucosinolates (GLS), hydroxy-cinnamic acids (HCA)
Citation: Duarte-Sierra A, Thomas M, Angers P and Arul J (2022) Hydrogen Peroxide Can Enhance the Synthesis of Bioactive Compounds in Harvested Broccoli Florets. Front. Sustain. Food Syst. 6:812123. doi: 10.3389/fsufs.2022.812123
Received: 09 November 2021; Accepted: 14 February 2022;
Published: 22 March 2022.
Edited by:
Nigel Gapper, The New Zealand Institute for Plant and Food Research Ltd., New ZealandReviewed by:
Franziska Clara Doerflinger, The New Zealand Institute for Plant and Food Research Ltd., New ZealandMarian Jane McKenzie, The New Zealand Institute for Plant and Food Research Ltd., New Zealand
Copyright © 2022 Duarte-Sierra, Thomas, Angers and Arul. This is an open-access article distributed under the terms of the Creative Commons Attribution License (CC BY). The use, distribution or reproduction in other forums is permitted, provided the original author(s) and the copyright owner(s) are credited and that the original publication in this journal is cited, in accordance with accepted academic practice. No use, distribution or reproduction is permitted which does not comply with these terms.
*Correspondence: Arturo Duarte-Sierra, YXJ0dXJvLmR1YXJ0ZS1zaWVycmFAZnNhYS51bGF2YWwuY2E=