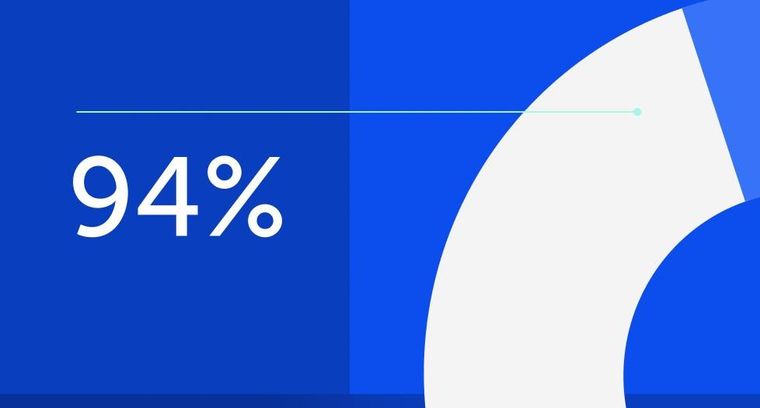
94% of researchers rate our articles as excellent or good
Learn more about the work of our research integrity team to safeguard the quality of each article we publish.
Find out more
ORIGINAL RESEARCH article
Front. Sustain. Food Syst., 29 April 2022
Sec. Agroecology and Ecosystem Services
Volume 6 - 2022 | https://doi.org/10.3389/fsufs.2022.755548
This article is part of the Research TopicEcological Nutrient Management as a Pathway to Zero HungerView all 13 articles
The UN's Sustainable Development goal of Zero Hunger encompasses a holistic set of targets that range from ending hunger by 2030, to increasing environmental sustainability and resilience of food production. Securing and managing soil nutrients remains one of the most basic challenges to growing adequate food while simultaneously protecting biodiversity and the integrity of ecosystems. To achieve these objectives, it is increasingly clear that the management of ecological processes will need to supplant reliance on non-renewable and environmentally damaging inputs. In recent years, progress has been made in developing perennial grain crops that show promise to improve on a range of ecological functions such as efficient nitrogen cycling and soil carbon accretion that tend to be well-developed in natural ecosystems but become compromised following land conversion to row crop agriculture. Here we report on a multi-faceted, 5-year experiment in which intermediate wheatgrass (IWG) (Thinopyrum intermedium), a perennial relative of wheat that is bred to produce the grain Kernza®, was intercropped in alternating rows with the perennial legume alfalfa (Medicago sativa). The performance of the unfertilized intercrop was compared to monocropped IWG treatments, with and without urea-N applications, planted at two row densities such that the intercrop could be interpreted as either an addition or substitution design. Comparisons of relative IWG yields (RYs) in the intercrop with unfertilized monocrops suggest net competitive interactions between alfalfa and IWG in the establishment year, followed by increasing degrees of facilitation over the next 4 years. Evidence from N fertilizer responsiveness, SPAD readings, net N mineralization assays, and N balance calculations suggest that alfalfa contributed to an aggrading pool of soil organic nitrogen over the course of the experiment. Comparisons of grain RYs of intercropped IWG and fertilized IWG monocultures suggest N-limitation in the first half of the experiment, and N sufficiency in the second half. Grain yields in the intercrop did not decline significantly over 5 years in contrast to all IWG monocrop treatments that did significantly decline. This study contributes to a growing literature on approaches to ecological nutrient management that incorporate diversity and perenniality to increase food security and resilience.
Input intensification characterized many of the most consequential agricultural innovations of the 20th century. For example, the invention and widespread adoption of synthetic nitrogen fertilizers was a pre-requisite for the quadrupling of the human population between 1920 and 2020 (Smil, 2001; Davidson et al., 2012). While profound in the outsized role they have played in feeding humanity, adoption of N fertilizers also resulted in outsized environmental problems as the amount of reactive N cycling in the ecosphere has exceeded what many believe to be a safe planetary boundary (Rockstrom et al., 2009; Steffen et al., 2015). A principal reason for these environmental problems is that only about half of applied N fertilizers are taken up by crops (Ladha et al., 2005; Lassaletta et al., 2016). The other half either leach into aquatic ecosystems, often inducing eutrophication, or are emitted in gaseous forms that have local to global consequences, including the formation of potent greenhouse gasses and the destruction of stratospheric ozone (Galloway et al., 2004; Howarth et al., 2005). In addition to environmental challenges, the economic consequences of fertilizer dependence for famers and ultimately consumers can be unpredictable. For example, due to the volatility of natural gas markets underlying N fertilizer production supplying sub-Saharan Africa, the average cost of urea has increased more than 300% between November 2020 and November 2021, resulting in lower fertilizer application rates and lower food production by farmers [IFDC (International Fertilizer Development Center), 2022].
Considering the social and environmental costs that have been associated with strategies of input intensification in general, and adoption of N fertilizers in particular, researchers are increasingly envisioning agroecosystems that maintain productivity through manipulation of ecological processes (Foley, 2011; Bommarco et al., 2013; Kleijn et al., 2018; Cassman and Grassini, 2020). Indeed, achieving sufficient food production through approaches like ecological nutrient management while reducing inputs that harm the environment are in close accord with the United Nation's Sustainable Development Goal of Zero Hunger by 2030 (United Nations, 2021; Target 2.4). Intercropping cereals with legumes is a time-tested approach to ecological nutrient management that is receiving renewed interest globally (Malézieux et al., 2009; Li et al., 2011; Lithourgidis et al., 2011; Pelzer et al., 2017; Daryanto et al., 2020). Much of this interest lies in the potential to improve on the synchrony between N supply and demand in cereal cropping systems (Gardner and Drinkwater, 2009), as well as shifting reliance from fossil fuel-intensive industrial sources of fixed nitrogen to biological nitrogen fixation (Crews and Peoples, 2004, 2005).
Several mechanisms have been identified to explain how diversifying cereal production with the inclusion of a compatible legume can improve on N synchrony in annual crops. While belowground transfer of N from annual legumes to cereals due to rhizodeposition of exudates (Fustec et al., 2010), root or nodule turnover (Johansen and Jensen, 1996), direct root contact (Hupe et al., 2021) or interspecific mycorrhizal bridging (Ayres et al., 2007; Meng et al., 2015) have been detected within a growing season, most evidence suggests that such transfers are minor relative to mechanisms of N partitioning (Bedoussac et al., 2015; Rodriguez et al., 2020). Annual cereals have been found to be more competitive than annual legumes in taking up available soil N, inducing greater reliance by legumes on N fixation (Rodriguez et al., 2020). Cereals are therefore thought to access more N on a per plant basis in many intercrop settings because of this shift from soil to atmospheric N sources by legumes coupled with a lower density (plants per hectare) of cereal plants in replacement intercrop designs (Rodriguez et al., 2020).
In annual intercrops, mechanisms by which legumes might contribute to improved N synchrony in cereals, are restricted to those that might occur within a growing season. Perennial cereal-legume intercrops that maintain deep roots over years represent a category of emerging cropping systems that potentially expand on the ecological nutrient management mechanisms that underlie annual cereal-legume intercrops. In this study we investigated the nitrogen economy of an intercrop consisting of perennial intermediate wheatgrass [IWG; Thinopyrum intermedium (Host) Barkworth & D.R. Dewey] that is undergoing de novo domestication to produce the grain Kernza®, with the perennial legume alfalfa (Medicago sativa, L.). We hypothesize three mechanisms that may facilitate a perennial cereal-legume intercrop such as IWG and alfalfa to achieve greater synchrony of soil N supply and crop demand.
1) Enhanced soil N uptake efficiency. Established stands of IWG have consistently reduced nitrate leaching relative to annual grains by 77–99% (Culman et al., 2013; Jungers et al., 2019; Reilly, 2021). The ability to take up greater soil N in time and space equates to more complete utilization of existing soil N resources, potentially leading to improved synchrony (Blesh and Drinkwater, 2013; Ruan et al., 2016).
2) Enhanced legume N fixation. Annual legumes, whether intercropped for grain, green manure or forage, have been found to fix less nitrogen, or fix less consistently across years compared to perennial forage legumes that begin the growing season fully established and commonly fix N for longer durations per year (Peoples et al., 2012; Schipanski and Drinkwater, 2012).
3) Enhanced soil organic N accumulation. The amount of N fixed by an annual legume that can be transferred to an associated cereal crop is limited by processes governing N enrichment of the soil organic nitrogen pool (Ayres et al., 2007; Crews et al., 2016). Perennial legumes have the potential to build SON pools through years of continued N fixation and rhizodeposition of N-rich exudates, roots and nodules (Fustec et al., 2010).
When considered together, these mechanisms suggest that perennial cereal-legume intercrops may benefit from greater N inputs from biological N fixation (BNF), reduced N losses and a resulting accumulation of larger pools of organic-N with the potential for mineralization to better synchronize with crop N demands (Drinkwater and Snapp, 2007).
The intercrop advantage in annual cereal-legume systems is weighted toward a net reduction in competition as the interaction between the two functional groups enhances legume utilization of atmospheric N and cereal utilization of soil N (Jensen et al., 2020). Under these circumstances, the yield (biomass or grain) of either individual species in the intercrop would be expected to be lower than monocropped fields planted at an equivalent density to the intercrop since the resource partitioning of N in the intercrop is enhanced but not complete; some competition for soil N remains. A net reduction in competition through resource partitioning of N has also been found to occur in perennial grass-legume intercrops (Schipanski and Drinkwater, 2012), and given that inputs from legume N fixation can accrue over years, perennial intercrops also have the potential to experience net facilitation whereby the legume both reduces reliance on and contributes to soil N reserves available to the cereal. In this case the non-legume species in the intercrop may show greater productivity than it achieves in a monocrop, as the legume contributes the limiting resource of N (Vandermeer, 1990; Bybee-Finley and Ryan, 2018).
The goal of the current study was to understand competition and facilitation as well as resource partitioning in a perennial legume-cereal intercrop with a special emphasis on N dynamics. Specifically we asked:
1. Is there evidence of resource competition or facilitation when intermediate wheatgrass is intercropped with the perennial legume alfalfa? Do these interactions change over time? Do these interactions change when the intercrop is a replacement vs. an addition design?
2. To what extent does the N uptake potential of intermediate wheatgrass synchronize with the N supplied by the intercropped legume alfalfa? What evidence is there that alfalfa is supplying N to IWG? Do alfalfa shoots and roots both contribute to enhancing soil organic N pools and in turn IWG productivity?
3. How does the mass balance of the annual and cumulative N budget of the intercrop system compare with fertilized and unfertilized single species stands of intermediate wheatgrass?
4. Does efficient N cycling translate into reduced emissions of the greenhouse gas nitrous oxide?
Research plots were established in October of 2012 at The Land Institute in central Kansas, USA (38°49'20.13” N; 97°35'29.51” W). In the USDA taxonomy, soils were coarse-silty, mixed, mesic Fluventic Haplustolls in the Cozad silt loam series (Palmer et al., 1992). The site receives an average of 760 mm of precipitation per year with 70% falling as rain between April through September. The average high and low temperatures for July are 34 and 20.5°C, and in January are 4 and −7°C. The site is considered semi-arid with soil moisture deficits occurring in most years and topsoil pH values ranging from 7 to 8.
Four replicates of seven treatments were sowed into recently tilled land that was previously planted to an unfertilized perennial sorghum. Plots measuring 5.2 m (L) × 6 m (W) were sowed to single species stands of IWG consisting of 13 rows per plot, or intercrops of IWG and alfalfa consisting of seven rows of IWG alternating with 6 rows of alfalfa. A buffer row of IWG spaced at 38 cm was sowed between treatments. Intermediate wheatgrass seed was “Cycle 2,” an early breeding population from The Land Institute. Treatments were sown as follows:
1. IWG = single species, no fertilizer, sowed in 76 cm rows
2. IWG+N = single species, 75 kg urea-N ha−1 yr−1, sowed in 76 cm rows
3. IWG(2) = single species, no fertilizer, sowed in 38 cm rows
4. IWG(2)+NP = single species, 150 kg urea-N and 20 kg triple super phosphate-P ha−1 yr−1, sowed in 38 cm rows
5. IWG-ALF = alternating rows of IWG and alfalfa sowed in 38 cm rows, no fertilizer
6. IWG-ALFr = alternating rows of IWG and alfalfa sowed in 38 cm rows, no fertilizer, alfalfa shoot biomass removed with root biomass remaining.
7. IWG-ALFs = single species (IWG only), no fertilizer, sowed in 76 cm rows, mulched with ALF shoot biomass produced in the plots of treatment 6.
8. IWG-ALFu = alternating rows of IWG and alfalfa sowed in 38 cm rows, 20 kg P ha−1 yr−1 as human urine, sowed in 38 cm rows.
The seeding rate for IWG plots was 6 and 12 kg ha−1 for IWG(2) plots. In the bicultures, alfalfa was seeded at a rate of 4.5 kg ha−1. Alfalfa seed variety was “I-70” and was inoculated with rhizobia. By establishing two row spacings of single species intermediate wheatgrass plots, both fertilized and unfertilized, it was possible to interpret the design as a replacement strategy, where one row of IWG in the 38 cm row spacing was replaced with a row of alfalfa, and as an addition strategy, where alfalfa was added to the intermediate wheatgrass planted in 76 cm rows (Figure 1) (Bybee-Finley and Ryan, 2018). Triple super phosphate was included in treatment 4 as a fertilizer-based comparison to treatment 8, which consisted of urine as a P source. Treatment 8 was discontinued in 2014 due to logistical challenges. Over a decade of breeding intermediate wheatgrass had taken place at this research site and in no cases had breeding populations shown responsiveness to P fertilizer amendments although responses to N additions have been common (DeHaan, pers com). In the interpretation of results in this study, we are confident in focusing on N dynamics, although we cannot rule out P effects in the IWG(2)+NP treatment.
Figure 1. Addition and replacement designs for comparing IWG-ALF intercrops with IWG monocrop treatments.
Fertilizers were applied to the soil surface of plots between March 15 and April 25 of every year. Alfalfa produced 2–4 cuttings per year (2, 4, 3, 2, 2 cuttings from 2013 to 2017, respectively) as influenced by stand age, climate and interactions with IWG. Every year in early May initial alfalfa growth was lost to herbivory by newly emerged alfalfa weevils (Hypera postica). Thereafter, at ca. 10% flowering, alfalfa biomass was cut by hand and replaced as mulch either back on original plots (IWG-ALF) or removed from IWG-ALFr plots and placed between rows of IWG in IWG-ALFs plots. During harvest, the alfalfa biomass from two 5.2 m rows were weighed and subsampled for dry:wet conversions (see below). Each year in the third week of July, 1-m sections of aboveground biomass + seed of IWG were cut from two rows by hand to 4 cm stubble height to estimate aboveground net primary production (ANPP) in all treatments. Within days, grain was harvested with a Wintersteiger (Delta) plot combine, dried, cleaned, and weighed. In every alfalfa and IWG plot harvest, subsamples of biomass were taken directly from the field, weighed, dried at 60C until constant weight, and weighed again to obtain dry:wet weight conversion ratios for estimating ANPP on a dry weight basis. IWG and alfalfa sub-plot harvests were scaled to hectare equivalents for comparisons. After grain harvest IWG residues were swathed, baled, and removed. Determination of δ15N and %N were made on alfalfa and IWG shoot materials sampled in early June ~6 weeks before harvest. Shoots from five plants per plot of each species present were sampled and aggregated into one composite sample. At harvest, IWG stems and seeds were sampled from all treatments for %N determination. All shoot materials harvested for N analyses were dried at 60°C and knife milled to homogenize bulk plant materials. Subsamples were ball milled, weighed into tin capsules and sent to SIMSL stable isotope laboratories at Kansas State University for determination of %N and δ15N.
We calculated relative yields (RY) (Vandermeer, 1990), also referred to as partial Land Equivalent Ratios (Bybee-Finley and Ryan, 2018) to compare the relative ANPP and grain harvests of IWG in the addition and replacement intercrop designs.
IWG RY values <1 indicate that the yields of IWG in the intercrop are lower than those of a IWG monocrop grown on an equivalent land area, implying competition. RY values >1 indicate that IWG yields in the intercrop exceed those of a monocrop grown on an equivalent land area, implying facilitation (Vandermeer, 1990; Bybee-Finley and Ryan, 2018).
In 2015, we measured potential N mineralization of soils from IWG-ALF, IWG(2), and IWG(2)+NP in field and laboratory incubations after Robertson et al. (1999). To conduct field incubations, we cut schedule 80, 5 cm dia. PVC pipe into 23 cm lengths, and beveled one end. Two sets of two tubes were hammered, beveled end down, 20 cm into the soil between 12” crop rows. Two of the four tubes were immediately extracted and put on ice. The remaining two cores were capped with PVC caps and left in the field. In the laboratory, the extracted soils were transferred from the PVC tubes to plastic bags where they were homogenized. Within 24 h of sampling, a 5 g subsample was removed from each bag and placed in 50 mL of 1 M KCl, shaken for 2 min, shaken again for 1 min after 4 h, and then sampled by pipette after 20 h for and analyses. A separate ~10 g sample was removed from each plastic bag, weighed, dried at 100°C for dry weight conversion. The remaining field cores were retrieved after 25–35 days and processed the same as the initial cores.
To undertake N mineralization incubations of soil strata deeper than 20 cm, we used a hydraulic soil corer to obtain 5 cm(D) × 1 m(L) cores, which we divided into the following strata: 1–10, 10–20, 20–40, 40–60, 60–80, and 80–100 cm. Soil samples were placed in plastic bags, homogenized by hand and subsampled for KCl extractions as described above. A separate 5 g sample from each sample bag was placed in a petri dish, and based on oven-weight conversion values, each stratum's moisture content was adjusted to 40% moisture using reversed osmosis water in a spray bottle. Soils were kept in the dark and adjusted to 40% moisture on a daily basis throughout the incubation period. After 25 days, the samples were extracted with 1M KCl. KCl samples were frozen until analyzed, along with field N mineralization KCl extracts, by the Kansas State soil testing laboratory for nitrate and ammonium concentrations. Average daily potential net N mineralization was calculated as:
where Nitratef and Ammoniumf = final nitrate and ammonium N concentrations. Nitrate0 and Ammonium0 = initial nitrate and ammonium N concentrations.
We designed the experiment to employ the natural abundance of 15N method for estimating N-fixation over the course of the study (Unkovich et al., 2008). Central to the method is the calculation of percent nitrogen derived from the atmosphere (%Ndfa) as follows:
where IWG was used as the non-N2 fixing reference plant selected to approximate the spatial and temporal patterns of soil N uptake by alfalfa. There is a general consensus that the natural abundance of 15N method is only viable when the reference and legume δ15N differ by a minimum of 2 delta units (Unkovich et al., 2008). Year one (2013) was the only year this criterion was met, and thus was the only year we fully calculated %Ndfa (88%) and total shoot N fixation based on field derived 15N values. For years 2–5, we used a hybrid approach to estimate N fixation that integrated field values for shoot %N and total biomass, with a %Ndfa value of 70% (median value, n=120 alfalfa fields in Europe; Anglade et al., 2015). As a check on our approach to estimating shoot N fixation, we used correlations between alfalfa shoot dry weight and N fixation reported by Carlsson and Huss-Danell (2003) in Europe and North America (Table 1). To estimate above + belowground inputs of N derived from alfalfa N fixation we used an alfalfa-specific conversion factor based on aboveground N fixation of 1.61 (Walley et al., 1996).
Table 1. Relative Yielda (RY) ratios of IWG ANPP grain yields grown in addition replacement intercrop designs with alfalfa (IWG-ALF) relative to four monocrop IWG treatments.
In 2013 and 2014, one meter soil cores measuring 6.2 cm in diameter were removed from IWG(2), IWG(2)+NP and IWG-ALF plots to quantify root biomass with depth. Cores were partitioned with a knife into two 10 cm strata (0–10 and 10–20), and then four 20 cm strata. Four hundred grams of soil at field moisture were twice passed through a 6 mm sieve to collect coarse roots. Next the 400 g soil samples were passed through a 1 mm sieve using a gentle spray of water, leaving fine roots on the mesh for collection. Coarse and fine roots were dried at 60°C and weighed.
We used static chambers in IWG(2), IWG(2)N+P and IWG-ALF treatments to collect gas samples for nitrous oxide analyses approximately every 2 weeks from May 12-Sep 15 in year 3, and April 18-Oct 3 in year 4, with additional sampling events added following fertilization and rain events. Molded PVC chambers 25 cm in diameter were placed on PVC rings inserted 2 cm in the soil, and air was sampled from the chamber four times over a 45-min period. Chamber air samples were collected in nylon syringes and stored up to 24 h before analysis. In the laboratory N2O was analyzed using a gas chromatograph (Agilent 6890) fitted with an electron capture detector (ECD) and calibrated with certified N2O standards. N2O flux was calculated as the increase in concentration within the chamber over 45 min.
We used a Minolta SPAD-502 to measure chlorophyll content of intermediate wheatgrass in mid-June of years 2 and 3 of the experiment. Measurements were taken on 15 leaves per replicate and averaged resulting in four replicate values per treatment. The SPAD meter was positioned mid-length on the second fully expanded new leaf of sampled plants.
We used separate repeated-measures mixed linear model analyses (PROC MIXED, SAS 9.4) to test for differences over time among total IWG ANPP and grain yields among primary treatments (IWG-ALF, IWG, IWG(2), IWG+N, IWG(2)+NP). The ANPP and grain yield were square root-transformed to provide best fit for the mixed models. Next, we used repeated-measures mixed linear models to analyze IWG yield effects over time for different avenues of alfalfa N-transfer (IWG-ALF, IWG-ALFs, IWG-ALFr, IWG). For these models ANPP was ln-transformed and grain yield was square root-transformed to provide best model fit. In both models, treatment was the fixed variable and year was the random repeated variable (subject = treatment*replicate, with an AR(1) covariance matrix).
To determine total N2O emissions from treatments IWG(2), IWG(2)+NP, and IWG+ALF in 2015 and 2016, we calculated the area under the curve (AUC) for N2O flux by treatment plot by year using the equation:
where t0 is initial sampling date, tfinal is final sampling date, xt is the N2O flux at given time t, and xt+1 is the N2O flux at the next sampling date.
Once AUCs were calculated for each plot by year, we analyzed effects of treatment, year, and treatment*year interaction on total N2O emissions using a two-way ANOVA with treatment as a fixed effect and year and treatment*year included as random effects. The AUCs were ln-transformed in order to meet the assumptions of normality and variance homogeneity. We then conducted follow-up pairwise comparisons with a Tukey-Kramer adjustment to determine differences by treatment.
Treatment effects on SPAD, net N mineralization and root biomass measurements taken in individual years were analyzed with one-way ANOVAs using JMP 11 (SAS, Cary, North Carolina, USA). When the effect of the treatments was found to be significant (F-tests, p <0.05), means were compared using Tukey's HSD test at α = 0.05.
ANPP of IWG changed significantly over time for all treatments, being greatest in the initial year and lowest in the final year of the experiment (Figure 2A, Supplementary Table S1). These yield extremes corresponded to the highest and lowest precipitation extremes, respectively, for April-July measured over the 5 years (Supplementary Figure S1). The decline in productivity between 2013 and 2017 was greatest in unfertilized IWG treatments, such that by 2017, these treatments had significantly lower ANPP than fertilized IWG treatments (Figure 2A). Decreases between 2013 and 2017 for IWG ANPP were 87% [IWG(2)], 78% (IWG), 68% (IWG(2)+NP), and 47% (IWG+N). When alfalfa was included, the ANPP of the IWG-ALF intercrop reached peak biomass in year 2, and then declined by 37% between years 2014 and 2017. In contrast to aboveground biomass production, grain yields in treatments that experienced N additions peaked in Year 2 (2014), whereas IWG and IWG(2) peaked in year 1 (2013) (Figure 2B). Mean grain yields decreased significantly over 5 years in all IWG monocrop treatments while we measured no significant decrease in yields of the intercrop over the 5-year study (Figure 2B).
Figure 2. Productivity of IWG as influenced by crop density, diversity, and fertility treatments. (A) Aboveground net primary productivity (ANPP) of IWG (kg ha−1) in monoculture and biculture treatments as well as ANPP of IWG-ALF including alfalfa (IWG+ALF total–green line) for all years, and (B) Grain yields of IWG (kg ha−1) in monoculture and biculture treatments for all years. Different lowercase letters represent significant differences within years (P < 0.05) based on Tukey pairwise comparisons.
The design of this experiment allowed us to evaluate the performance of the IWG-ALF intercrop both as a replacement and addition to IWG monocrop configurations (Figure 1). In both designs, intercrop grain and biomass productivity were intermediate between the fertilized and unfertilized IWG treatments (Figures 2A,B). When interpreted as a replacement design (IWG-ALF compared to IWG(2) or IWG(2)+NP treatments) differences between the intercrop and monocrop were strongly dependent on whether supplemental fertilizers were applied to the monocrops. From 2014 to 2017, IWG(2) demand for N and possibly P appeared to exceed the nutrients supplied through soil resources alone, as IWG(2)+NP ANPP was significantly greater than IWG(2) ANPP from 2014 to 2017. In the establishment year, N did not appear to strongly limit IWG(2) productivity. Intermediate wheatgrass ANPP in the IWG-ALF intercrops was relatively low in the establishment year, but by 2014, ANPP equaled that of IWG(2) even though the intercrop had half the intermediate wheatgrass planting density. By years 3–5, the effect of the alfalfa became more pronounced as intercrop productivity increasingly exceeded that of unfertilized IWG(2). The differences in intermediate wheatgrass ANPP between IWG(2)+NP and IWG-ALF were large in the first 2 years (between 3–4 tons ha−1 yr−1) but then narrowed over the final 3 years to where ANPP of these two treatments was equal in 2017. When the ANPP of IWG and alfalfa were summed (IWG+ALF total) the unfertilized intercrop shared the ranking of most productive treatment with the fertilized IWG(2)+NP for four of the 5 years (Figure 2A).
When interpreted as an addition intercrop experiment, the row of alfalfa in the IWG-ALF treatments represents an addition to the IWG monocrop treatments (IWG and IWG+N) planted at 76 cm row spacing. This addition of alfalfa resulted in an intercrop with double the crop density of the monocrop IWG treatments. Patterns of mean intermediate wheatgrass ANPP in the intercrop addition comparisons were similar but less exaggerated than the patterns exhibited in the replacement design. In 2013, the IWG-ALF treatment had lower ANPP and grain yield than either fertilized or unfertilized IWG and the reduced grain yield persisted through year 2 (Figure 2B). These differences in productivity occurred when N appeared to be relatively abundant, thus competition for water may have occurred. By 2015, the IWG-ALF ANPP was greater than the unfertilized IWG plots, and grain production of these two treatments was similar. The IWG+N treatment produced the highest ANPP and grain production of the wide-spaced IWG (Figure 2A).
Compared to patterns of ANPP, grain yields differed considerably when evaluated as replacement and addition intercrop designs. In the replacement comparison, grain yields of the intercrop were about 30% lower than fertilized or unfertilized IWG(2) plantings in year 1 (2013) (Figure 2B). In 2014, IWG(2)+NP grain yields peaked, but then declined precipitously in 2015, and remained low but stable from 2016 to 2017, whereas yields of unfertilized IWG(2) declined substantially after the establishment year. Grain yields in the intercrop were quite consistent at ca. 250 kg ha−1 yr−1 for four of the 5 years. In the addition comparison, both fertilized and unfertilized IWG treatments had greater mean grain yields than the intercrop in 2013 and 2014, but the trend reversed in the later years of the experiment with mean intercrop grain yields exceeding IWG monocrops in 2017 (Figure 2B).
Relative yield (RY) ratios help to clearly distinguish intercrop performance relative to fertilized and unfertilized monocrops (Table 1). In 2013, seven of the eight different IWG intercrop vs. monocrop comparisons showed that the monocrops yielded greater biomass or grain, resulting in RYs <1 (i.e., it would require less land under a monocrop to achieve the IWG yield of the intercrop). With the sole exception of the 2016 IWG(2) grain RY, the annual increase of IWG RYs was evident for ANPP and grain comparisons of unfertilized treatments in both addition and replacement intercrop designs (Table 1). RYs in the final year of the experiment were particularly high at 3.4 and 4.2 in the unfertilized addition and replacement intercrops, respectively. In contrast to comparisons with unfertilized IWG treatments, RYs involving fertilized IWG treatments changed less after 2013. ANPP RY ratios in the addition intercrop stayed almost constant between 0.8 and 0.9, with grain ratios increasing from 0.6 to 1.2. In the fertilized replacement intercrop after the establishment year, ANPP RYs dropped to 0.5 and then increased to 1.0 from 2014 to 2017, and the grain ratios dropped to 0.6 in 2014 and then gradually increased to 1.4 in 2017.
Estimations of N inputs into the IWG-ALF treatment from alfalfa N-fixation ranged between 54 and 162 kg ha−1 yr−1 (Table 2). The shoot N fixation value of 55 kg N ha−1 for 2013 that was based entirely on field measurements was similar to the value of 52 kg N ha−1 calculated using a correlation of shoot fixed N and alfalfa dry matter production reported by Carlsson and Huss-Danell (2003). N fixation estimates for 2014–2016 were lower than the correlation-based estimates whereas the two approaches produced the same value of 80 kg N fixed ha−1 in 2017. The reduced fit between field-based N fixation estimates and the correlation check can be explained by lower alfalfa shoot %N in years 2014–2016 compared to 2013 and 2017 (Table 2).
Table 2. Shoot %N, total-N and fixed-N in alfalfa from the IWG-ALF treatment between 2013–2017 based on empirical and literature values.
The addition of alfalfa roots, shoots or roots+shoots to unfertilized, wide spaced IWG rows did not result in statistically different levels of ANPP, and seed yields were only significantly different between the root + shoot and no-alfalfa treatments in the final year (Figure 3, Supplementary Table S2). Both ANPP and grain yield of the no-alfalfa treatment had the highest mean values in the first year and declined over time to the lowest mean values in the final year.
Figure 3. Influence of above and belowground alfalfa biomass on IWG productivity. (A) Mean ANPP and (B) mean grain yield of IWG planted in 76 cm rows with the following inter-row alfalfa additions: root+ shoot, root only, shoot only, none. No within year differences of ANPP between treatments were found to be statistically significant. In grain yields, different lower-case letters represent significant differences within years (P < 0.05) based on Tukey pairwise comparisons. Bars = 1 S.E.
The N balances for each year of the experiment were calculated as managed N inputs in urea or alfalfa N fixation minus N exported in harvest. Predictably, the two treatments that received neither of the N inputs, IWG and IWG(2), maintained negative N balances for all 5 years of the experiment (Figure 4A). The degree of N deficit mirrored the patterns of biomass and grain exports with the most negative N balances in 2013, and the least negative in year 2017. The IWG+N and IWG(2)+NP had small negative N balances in year one of −26 and −15 kg ha−1, respectively, but in subsequent years IWG(2)+NP maintained a greater N surplus than IWG+N ranging between 42 and 91 kg ha−1. The mean N balance of the IWG-ALF intercrop varied substantially from year to year reflecting the relative productivity and N fixation by alfalfa relative to IWG productivity through time. The intercrop experienced N deficits of 20 kg ha−1 in 2013 and 17 kg ha−1 in 2016, with N surpluses of 119, 34, and 90 kg ha−1 in years 2014, 2015 and 2017, respectively.
Figure 4. N balance comparisons of intercropped IWG-ALF with unfertilized and fertilized IWG treatments. (A) Yearly N balance calculated as managed N inputs in urea or alfalfa N fixation minus N exported in harvest, (B) Cumulative N balance over 5 years, (C) Cumulative N export in grain + biomass harvests over 5 years.
The mean cumulative N balances of managed inputs minus harvested exports over 5 years revealed an almost identical negative slope for the IWG and IWG(2) non-fertilized treatments even though row spacing and seeding rates differed by 100% (Figure 4B). IWG at both row spacings exhibited maximum productivity and thus the greatest N deficit in 2013, followed by N balances between −65 and −11 kg ha−1, corresponding to increasingly N-limited yields over the following 4 years. The three treatments that received N inputs—IWG+N, IWG(2)+NP and IWG-ALF–demonstrated positive cumulative N balances over 5 years of 63, 332, and 188 kg ha−1, respectively.
Differences between cumulative N exported between 2013 and 2017 from the unfertilized IWG and IWG(2) treatments compared to the IWG-ALF treatment were 39 and 56 kg ha−1, respectively (Figure 4C). In contrast, five-year cumulative net N balance differences between IWG-ALF and the IWG and IWG(2) unfertilized treatments were 456 and 439, respectively (Figure 4B).
The mean N exports in biomass and grain harvests from all treatments except IWG(2)+NP were similar (within 35 kg) for the first 3 years of the experiment (Figure 4C). In the final 2 years the IWG+N and IWG-ALF treatments appeared to bifurcate from the two non-fertilized IWG treatments, showing greater N exports, presumably in response to receiving N inputs. The IWG(2)+NP treatment mean N offtake was the highest of all treatments in all years, with an export of 165 kg N ha−1 in 2013, followed by 3 years of between 70 and 80 kg N ha−1 yr-1, and then a sharp decline to 29 kg N ha−1 in 2017.
By measuring the difference between the transmittance of a red (650 mn) and infrared (940 nm) light through leaves, SPAD meters calculate relative values of chlorophylla+b content which can be directly related to the N status of a crop (Yuan et al., 2016). In 2014 of the experiment, significantly different mean SPAD values of IWG were measured between the urea-N addition treatments and all others [ANOVA F(6, 26) = 13.83, P <0.0001] (Figure 5). By 2015, the mean SPAD values of IWG had differentiated to a greater extent showing significantly greater chlorophyll content of IWG-ALFr,s and IWG-ALFr treatments compared to unfertilized IWG(2) and significantly less than both fertilized IWG treatments [ANOVA F(6, 26) = 22.66, P <0.0001] (Figure 5).
Figure 5. SPAD values reflecting relative IWG leaf N status as influenced by crop density, diversity, and fertilization in 2014 and 2015. Bars with different upper and lower-case letters indicate significant differences between treatments in 2014 and 2015, respectively, at p < 0.05 (Tukey HSD). SPAD values from both years were log transformed to achieve normal distributions (not shown).
In 2015, laboratory incubations of soils sampled to 1 m depth from IWG+ALF, IWG(2) and IWG(2)+NP treatments were undertaken to compare rates of net N mineralization. The intercrop treatment IWG+ALF and the fertilized treatment IWG(2)+NP were found to support significantly greater rates of net N mineralization than the unfertilized IWG(2) treatment in the surface 20cm of soil (Figure 6, Supplementary Table S3). Below 20 cm, no significant differences were found between treatments. In the same treatments in 2015, we conducted in situ soil net N mineralization assays to 23 cm depth across 4 months from spring to late summer (Supplementary Figure S2, Supplementary Table S4). Mean net N mineralization rates were higher in the IWG-ALF treatments compared to unfertilized IWG in each of the 4 month-long assays, but none of the differences were statistically significant. Mean N mineralization in IWG-ALF and IWG(2)+NP treatments were equivalent in the first measurement of the summer, but were significantly lower than the fertilized treatment in Period 2 and exhibited a similar trend in the subsequent two periods. This finding reflects the timing of fertilization, as the first set of cores were installed on April 23, a week before urea applications to the IWG(2)+NP treatment.
Figure 6. Net N mineralization assay conducted in year three of IWG-ALF, IWG(2) and IWG(2)+NP treatments. Laboratory incubation of 1 m cores obtained in early July, which were divided into five-20 cm strata. Bars with different lower-case letters indicate significant differences at p < 0.05 (Tukey HSD). Bars = 1 S.E.
The IWG-ALF intercrops produced significantly greater mean total root biomass (fine + coarse) in the top 10 cm compared to IWG(2) and IWG(2)+NP plots in 2014 (not shown) [ANOVA F(17, 68) = 10.19, P <0.0001] and 2015 (ANOVA F(17, 71) = 26.43, P <0.0001] (Figure 7). In the 0–10 cm stratum of the intercrop treatments, the soil cores sampled from the IWG and alfalfa rows had similar total root biomass. However, in all depths below 10 cm, there was greater root biomass in the strata sampled from the alfalfa row. It was not possible to separate roots by species. We attempted to determine the proportion of alfalfa and IWG roots collected from the rows of the two species by analyzing their δ15N signatures relative to pure samples of alfalfa and IWG roots. However, the difference in δ15N values between alfalfa and IWG roots were insufficient, and intraspecific variation too great to characterize the composition of mixed roots from the cores (data not shown).
Figure 7. Mean total root biomass (coarse + fine roots) sieved from two 1 m cores (diameter 6.2 cm) per plot in 2015. In the IWG(2)+NP treatment (gray bar) and the IWG(2) treatment (black bar) 1 core was sampled from 2 separate rows. In the IWG-ALF treatment, 1 core was sampled from an alfalfa row (white) and one core sampled from an intermediate wheatgrass row (speckled). In all cases cores were taken from between plants. Cores were divided into six strata. Bars with different lowercase letters indicate significant differences within a depth at p < 0.05 (Tukey HSD). Bars = 1 S.E.
Nitrous oxide emissions were significantly greater in the IWG(2)+NP treatment that received urea-N compared to the IWG(2) and IWG-ALF treatments in both 2015 and 2016 (Figure 8). The highest N2O emissions were measured early in the growing season which corresponded to urea applications in the IWG(2)+NP treatment, as well as warmer soil temperatures and intermittent wet conditions (Supplementary Figure S3).
Figure 8. N2O emissions summed over growing season in 2015 and 2016 from IWG(2), IWG-ALF, and IWG(2)+NP treatments. Treatments with different letters indicate significant differences at p < 0.05 (Tukey HSD). Bars = 1 S.E.
Significant differences in shoot δ15N values were observed in Years 2013–2015 between alfalfa and IWG within the IWG-ALF intercrop, with IWG having higher δ15N signatures than alfalfa. The two crop species did not differ in δ15N in Years 2016 and 2017 (Figure 9, Supplementary Table S5).
Figure 9. Changes in shoot δ15N over 5 years from IWG-ALF and IWG treatments in the replacement intercrop design. Crop designations are ALF (w/IWG) = shoots from alfalfa grown with intermediate wheatgrass, IWG = shoots from intermediate wheatgrass grown in monocrop, IWG (w/ALF) = shoots from intermediate wheatgrass grown with alfalfa. Bars with different lowercase letters indicate significant differences at p < 0.05 (Tukey HSD).
Results from this 5-year study highlight important ways in which diversity and perenniality combine to influence the supply and cycling of N–two key targets of ecological nutrient management. Comparisons of IWG yields in addition and replacement intercrop designs with unfertilized IWG monocrops provided insight into a temporal shift from net competition in early years to net facilitation in later years governing IWG and alfalfa interactions. Similar comparisons of IWG intercrop yields with fertilized IWG monocrops revealed the degree to which alfalfa N fixation inputs were able to meet the N demands of associated IWG over time. These intercrop and monocrop comparisons, coupled with N balance and relative yield calculations, and indicators of N availability and crop N synchrony, provide a multi-dimensional understanding of ecological nutrient management in perennial cereal-legume intercrops.
The greater productivity measured with fertilization of low and high density IWG plantings compared to unfertilized plantings demonstrated N limitation across all 5 years of the experiment. The rapid and precipitous decrease in ANPP and grain yield in the unfertilized and densely planted IWG(2) treatment suggests the development of profound N limitation (Figure 2).
Against the backdrop of fertilized and unfertilized IWG treatments, RYs of ANPP and grain production by IWG in the intercrop reveal important patterns relevant to ecological nutrient management. Intercropping IWG with alfalfa, in both addition and replacement configurations, resulted in RYs in the establishment year that were consistently <1 (Table 1), possibly due to belowground competition for water or nutrients when alfalfa and IWG have yet to partition niches via differential rooting depths. From 2015–2017, the RYs of all intercrop comparisons with unfertilized monocrops show clear evidence of net facilitation (Table 1). In absolute terms, the addition of alfalfa appears to slow the decline of ANPP demonstrated by IWG and IWG(2), and it prevents the decline of grain yields measured in the monocrop treatments (Figures 2, 3). Yields in IWG monoculture stands have been found to decline in the first 2–3 years in other studies (Jungers et al., 2017; Hunter et al., 2020).
In response to the overarching question of whether intercropping with alfalfa results in sufficient N fixation and mineralization to meet the demands of IWG, the answer, based on yield comparisons with fertilized IWG treatments, is generally “no” early in the experiment, and generally “yes” late, but there are interesting differences between addition and replacement designs with respect to ANPP. Intercropped IWG in the addition experiment had ANPP yields similar to those of the fertilized monocrop over the 5 years of the study, suggesting weak to no N limitation in the intercrop. In contrast, the same ANPP comparison between intercropped IWG and the fertilized monocrop in the replacement design suggested N limitation to intercropped IWG in years one through four. Relatively low grain yields in the first 3 years of the intercrop implied moderate N-limitation in both the addition and replacement designs relative to fertilized monocropped IWG treatments. In contrast intercropped IWG grain yields in the second half of the experiment were equal or greater than fertilized monocropped IWG in both designs suggesting N sufficiency and a small degree of facilitation in the intercrop. It makes sense that the yields of intercropped-IWG in the replacement design might be less than fertilized monocultures, since the IWG plant density in the intercrop was half that of the monocrop. The finding that RYs in this comparison were >0.5 in the first 2 years, and >1 in the final three underscores how diversity can play a pivotal role in ecological nutrient management. It is not simply the provisioning of N by alfalfa that supports intercrop grain productivity, but also an apparent reduction in IWG intraspecific competition, or another ecological function such as pathogen suppression.
Other studies examining IWG-legume intercrop dynamics have also reported relatively lower IWG yields in intercrops compared to monocrops, especially when the intercropped legume was alfalfa or another species deemed to be competitive. Reilly (2021) found intercrops involving alfalfa and red clover (Trifolium pretense) tended to have relative yields <1 compared to unfertilized IWG. The row spacing used in Reilly's experiments was 15 cm, with 2 rows of legume for every 1 row of IWG (45 cm between IWG rows); much closer than the spacing used in the present study which may have enhanced competition and depressed RY values. Less competitive legumes such as Canada milkvetch (Astragalus canadensis) and white clover (Trifolium repens) did demonstrate positive RY values in the majority of sites and years (Reilly, 2021). When compared to fertilized IWG Reilly found IWG intercropped with six different perennial legumes grown in three sites for 3 years to have RYs <1.
In a separate Minnesota study, Tautges et al. (2018) compared yields of unfertilized and N-fertilized IWG monocrops with a replacement design intercrop where every other row of IWG was replaced with alfalfa. In year 2 of the study, grain yields of the intercrop were lower in 3 of 5 locations compared to unfertilized monocrops, and 4 of 5 locations compared to fertilized treatments. The lower intercrop yields appeared to be the result of lower IWG density and in some cases, suppression of intermediate wheatgrass by alfalfa. Patterns of productivity changed by year 4 and yields of the intercrop were greater than unfertilized monocrop treatments in 4 of 5 locations and fertilized monocrop treatments in 3 of 5 locations (Tautges et al., 2018). Similar to the present study, the authors suggested the intercrop performance may have been tied to improved N fertility provided by the legume as alfalfa biomass was correlated positively with grain yield, harvest index and nutrient uptake in the year 4 intercrop.
Casamitjana (2021) recently reported on a project in which IWG productivity was evaluated as a function of row spacing, N fertility and legume intercrop in Wisconsin USA. She found that when legumes, including alfalfa, were added to IWG stands in addition intercrop designs, grain and ANPP either did not change or decreased. However, Casamitjana was only able to study wheatgrass-legume intercropped plots for 3 years which is before facilitation effects of alfalfa were detected in the present study and by Tautges et al. (2018). In SE Australia, Hayes et al. (2016) found replacement intercrop designs of perennial wheats and subterranean clover (Trifolium subterraneum) to yield less than addition designs. Replacing cereal rows with legumes resulted in greater N fixation inputs but the reduction in cereal stand density lowered intercrop yields compared to monocultures. The Australian experiment only lasted for 3 years and the authors acknowledged that the relative performance of the intercrop could change in later years.
The results from the experiment in which we partitioned the influence of above and belowground alfalfa inputs were not significant (Figure 3). In other studies, evidence increases that belowground inputs from root death and turnover have a greater influence than aboveground inputs on soil organic matter accretion (Rasse et al., 2005; Schmidt et al., 2011; Poirier et al., 2018), but we did not see that in this study. The relative roles of above and belowground inputs are relevant because a range of strategies are being considered to manage IWG-alfalfa intercrops. One involves removal of aboveground alfalfa biomass by hand or machine for animal forage, while another involves cutting the alfalfa and leaving it in place as an organic mulch for weed control and N addition. More research would be useful to determine whether legume shoot biomass removal affects IWG grain and biomass productivity and soil carbon accumulation.
N synchrony has been defined as the matching of crop N demand with N supply (Robertson, 1997; Drinkwater and Snapp, 2007). The two common types of asynchronies that occur are when N supply falls short of or exceeds crop N demand (Crews and Peoples, 2005). Annual N balance and cumulative N balance calculations of the IWG monocrop and intercrop plots suggest that N demand in unfertilized plots strongly exceeded N supply. In contrast, N supply likely strongly exceeded crop demand in the IWG(2)+NP treatment where in years 2014–2016, about half of the 150 kg N ha-1yr−1 applied was not exported in crop biomass, increasing to 80% in 2017 (Figure 4A). While the annual N balance of the intercrop treatment fluctuated substantially between positive and negative across years, the cumulative mean N balance of the IWG+N, IWG-ALF and IWG(2)+NP treatments were positive by 63, 188, and 332 kg ha−1, respectively. However, we know that estimated N fixation by a living perennial legume does not equate to “N supply” in the same time frame as applications of readily available N in the form of urea fertilizer. Biological processes such as root turnover and mineralization regulate the rate of legume-N release for cereal uptake, thus restricting the potential for N losses that negatively impact the environment. In contrast, fertilizer-N forms lack mechanisms of biological regulation, and thus surpluses are vulnerable to loss pathways that commonly result in ecosystem disservices such as eutrophication or enhanced N2O emissions (Crews et al., 2016).
Our N balance calculations do not include N sinks associated with microbial immobilization in the short term, and net soil organic matter accumulation in the longer term that is predicted to take place with the conversion of annual cropping systems to perennial vegetation (Post and Kwon, 2000; Sprunger et al., 2017; King and Blesh, 2018). The duration of this experiment fell short of the time commonly considered necessary to detect significant differences in soil organic carbon stocks following a change in crop vegetation (Necpálová et al., 2014; McGowan et al., 2019). However, reviews and meta-analyses that examined soil carbon sequestration rates following the conversion of annual to perennial grasslands report a range of 0.3–1 ton C ha−1 yr−1 (Crews and Rumsey, 2017). Most stable soil organic matter (SOM) has C:N ratios of between 10 and 15:1, and thus the N required to accumulate 0.3–1 ton C ha−1 would fall between 20 and 100 kg N ha−1 yr−1 or 100–500 kg ha−1 over 5 years. If we assume this range of organic matter accumulation rates is applicable to perennial grain crops, then this potential N sink could account for the 5-year positive N balances of the IWG+N, IWG-ALF, and IWG(2)+NP treatments. Positive N balances on croplands that are in approximate equilibrium with SOM accumulation and loss rates may have greater potential to lose N, resulting in negative environmental impacts. However, agroecosystems undergoing successional changes such as the shift from annual to perennial grains will likely need to maintain positive N balances for up to decades until SOM pools achieve new equilibria (Crews et al., 2016).
The relative stability of ANPP and grain yields of the IWG-ALF intercrop compared to fertilized and unfertilized IWG monocrops across 5 years is noteworthy (Figure 2), especially when soil N dynamics appeared to change throughout the same period of time. It seems clear that when a perennial cereal and legume are sowed simultaneously, the plant and soil processes involved in N fixation and transfer from the legume to cereal require more than one growing season to develop (Louarn et al., 2015). Plant establishment, cycles of shoot cutting and residue decomposition, root and nodule turnover, and stand thinning all likely contribute to a gradually aggrading pool of soil organic nitrogen (Gault et al., 1995; Peoples et al., 2009; Louarn et al., 2015). In our study, several lines of evidence suggest that a consequential pool of organic N had accumulated by year 3 (2015) in the IWG-ALF treatment, and continued to facilitate IWG productivity through 2017. First, changes in SPAD meter readings from 2014 to 2015 (Figure 5) suggest an increase in IWG-ALF leaf chlorophyll content and N content by proxy relative to the unfertilized IWG treatments. Second, the net N mineralization lab assay conducted in 2015 suggests greater N supply from microbial mineralization in the intercrop soils compared to soils from IWG(2) (Figure 6). Third, cumulative N exports from the intercrop treatment fell below those of the unfertilized IWG treatments between 2013 and 2015, consistent with N limitation (Figure 4C), while during the same time, the cumulative N balance of the IWG-ALF intercrop was very similar to IWG(2)+NP, the treatment that received the greatest fertilizer inputs (Figure 4B). Only after 2015 did the cumulative N exports from the intercrop exceed those of the unfertilized treatments. That N inputs to the intercrop were high, but N availability was low for the first half of the experiment is consistent with the idea that N inputs were decoupled from N supply to IWG, resulting in an accumulation of soil organic nitrogen in the intercrop.
Other studies have reported on a similar decoupling of N inputs from perennial legumes and N supply to proximate perennial grasses. In a ryegrass (Lolium perenne) white clover (Trifolium repens) intercrop in Denmark, Hogh-Jensen and Schjoerring (1997) used 15N enrichment and natural abundance methods to estimate that only 3% of the N fixed by clover was accessible to ryegrass in the first year of production. In the second and third years, as the soil organic nitrogen pool grew larger and N mineralization increased proportionately, this percentage increased to 17 and 22%, respectively. In another study involving N transfer from red clover (Trifolium pratense) to Kentucky bluegrass (Poa pratensis), Thilakarathna et al. (2016a) found that N fixation in red clover was greatest in the first production season, but seasonal N-transfer doubled in the second production year over the first. They attributed the increase in N transfer from legume to grass with stand age to increases in root mass and surface area, root exudates, senescence and decay of roots and nodules, defoliation induced N release by legume root systems, and development of mycorrhizal hyphae networks (Thilakarathna et al., 2016a,b). Decoupling of N inputs from perennial legumes has also been documented in alley cropping systems involving tree and/or shrub hedgerows bordering narrow fields of annual grains. Okonkwo et al. (2008) found single applications of prunings from Gliricidia sepium and Leucaena leucocephala to maize significantly increased net soil N mineralization by 2–3-fold over controls for three growing seasons in Nigeria. In a detailed review of the N budgets of similar alley cropping systems in tropical regions, Sanginga et al. (1995) estimated that N inputs in hedgerow prunings commonly exceeded 300 kg N ha−1 yr−1, while only ~30% or less appeared to be taken up by the cereal in the first year of the growing season. The authors surmised that the remaining N added to an aggrading pool of soil organic nitrogen, as we found in the IWG-ALF intercrop, or was lost to the environment, given windows of compromised N synchrony inherent in the annual cropping cycles. Although they acknowledged belowground N transfers are poorly understood and characterized, Sanginga et al. (1995) estimated that 25–102 kg N ha−1 season−1 were contributed by roots and nodule turnover of hedgerow legume species to the annual cereal.
Natural abundance of 15N values from alfalfa and IWG in the intercrop and the unfertilized IWG treatment over 5 years suggests a shift from soil organic matter as the primary source of available N early in the experiment to more depleted inputs later in the experiment (Figure 9). In 2013, the mean δ15N value of IWG shoots grown in the intercrop was relatively enriched at 3.3, and then declined over the next years converging on a δ15N signature similar to that of the N-fixing alfalfa in 2017. This simple 15N pool dilution interpretation of converging legume and grass δ15N values cannot be used to demonstrate with certainty that soil pools of legume-fixed N are responsible for the change in intermediate wheatgrass δ15N (Peoples et al., 2015). Indeed, this example is complicated because the δ15N signature of the unfertilized monocropped IWG also declined over the course of the study even though it is not influenced by the less enriched δ15N of alfalfa-N. We speculate that the relatively enriched δ15N signatures of IWG from both monocrop and intercrop treatments in the establishment year (2013) reflected relatively high rates of soil N mineralization stimulated by the tillage employed to establish the experiment. The similar ANPP in 2013 of unfertilized IWG and IWG(2) treatments relative to their fertilized counterparts also supports the interpretation that endogenous N was sufficient early (Figure 2). In subsequent years, it is clear that the IWG(2) treatment became increasingly N limited exacerbated by annual removal of N in harvests, and reduced soil N mineralization with the cessation of soil disturbance. The δ15N values of vegetation experiencing profound N limitation frequently reflect the δ15N values of inputs as opposed to enriched soil δ15N driven by N losses (e.g., Vitousek et al., 1989). In the case of central Kansas, δ15N of nitrate and ammonium deposition has been measured at 2 to −15 (Townsend et al., 2003), thus N deposition is frequently strongly depleted in 15N as compared to atmospheric N2. We suggest that the decline in δ15N in the IWG treatment may reflect a shift in relative dependence from mineralized soil-N to atmospheric deposition-N. It is likely that the intercrop IWG δ15N also reflects atmospheric deposition, however the greater ANPP and grain yields produced by the intercrop compared to the unfertilized IWG treatments in years 4–5 imply that the δ15N signature of legume N inputs likely contributed to the depleted δ15N of intercrop IWG as well (Figure 2).
The finding of significantly greater root biomass in the 0–10 cm stratum of the IWG-alfalfa intercrop compared to fertilized or unfertilized monocropped IWG(2) contributes to a growing body of literature showing a positive relationship between diversity and belowground productivity in experiments involving wild and domesticated perennial species (Figure 7). Yang et al. (2019) measured approximately twice the root mass in plots with two vs. one perennial species in 5 years after establishment. The combination of functional groups they found to produce the greatest root biomass were C4 grasses and legumes. In a comparison of perennial monocultures of bioenergy crops such as miscanthus and switchgrass and diverse grassland systems, Sprunger et al. (2017) consistently found greater fine root production in the diverse grassland ecosystems. In the present study, greater total root biomass produced in the intercrop replacement design compared to IWG(2) monoculture treatments suggests that the alfalfa and IWG are avoiding competition through resource partitioning and/or there is facilitation of belowground productivity between species. More studies will be required to determine whether perennial cereal-legume intercrops consistently maintain higher root biomass compared to monocrop perennial cereals. The question is highly relevant to predicting rates and amounts of soil carbon accumulation and resulting nutrient retention in a transition from annual to perennial species (Sprunger et al., 2020).
In many respects it is not surprising that the IWG(2)+NP treatment sustained high N2O emissions over two growing seasons in 2015 and 2016 compared to the unfertilized IWG(2) treatment. Fertilizer additions frequently result in enhanced N2O loss pathways (Robertson et al., 2012). It is notable, however, that the N2O emissions in the intercrop treatment were essentially the same as the unfertilized IWG(2) even though the difference in cumulative N balances between these two treatments was >300 kg ha−1 in both years 3 and 4 (Figure 4B). We predict that N2O emissions from perennial legume-cereal intercrops that are closer to equilibrium with respect to soil organic matter may be greater than those emitted in agroecosystems actively aggrading SOM. Even if N2O emissions are found to increase some in an intercrop that is less limited by N, the improved synchrony demonstrated in this diverse, perennial agroecosystem may be a viable ecological nutrient management strategy for reducing N2O emissions.
While the IWG-ALF intercrop may have several promising attributes worth considering in future intercrop designs, the insufficient N asynchrony that appears to have limited intercrop productivity compared to fertilized monocrops in years 1–3 deserves attention. As demonstrated in this study, substantial inputs of N through BNF and yet only a small fraction of these inputs became available to IWG. Nevertheless, results here suggest that small improvements in N availability may significantly improve yields. This will be increasingly important as the yield potential of IWG continues to increase through intensive breeding efforts (Crain et al., 2021a,b). We propose several design or management innovations that have the potential to relax intercrop N limitation in the first years following stand establishment.
1. Add clover to alfalfa to improve N synchrony. Alfalfa is widely recognized for maintaining very high rates of N fixation over many years, and has deep tap roots that may facilitate water resource partitioning with IWG and other cereals (Thilakarathna et al., 2016b; Corentin et al., 2022). However, compared to white and red clovers, a much lower percentage of alfalfa fixed-N becomes available to intercropped species within a 1–2 year timeframe (Louarn et al., 2015; Thilakarathna et al., 2016b). By diversifying the legume component of the intercrop, such as co-sowing red clover and alfalfa with IWG, it may be possible to take advantage of more rapid cycling N from clover, while benefiting from the slower N build-up and subsequent N mineralization that we saw in this study with alfalfa after 2015.
2. Add modest N amendments in years 1–2 to improve N synchrony. In a recent pot experiment in which IWG was intercropped with white clover in different plant density ratios and N fertilization regimes, Li et al. (2021) reported how the %Ndfa of clover was 60% when an IWG-clover intercrop was fertilized with the equivalent of 225 kg N ha−1. This relatively high %Ndfa in the face of large N inputs suggests that IWG is able to take up most mineral N in the soil avoiding suppression of N fixation. If this result is transferable to the field, then it may be possible to fertilize an IWG-ALF intercrop with manure, urine, or synthetic fertilizer while still benefitting from alfalfa N fixation and accumulation.
3. Improve synchrony with a “rotational intercrop.” Given the 2–3 year lag shown in this study between the onset of alfalfa N fixation inputs and adequate levels of net N mineralization to IWG in the intercrop, we suggest a cropping system that combines elements of a rotation and an intercrop to enhance N synchrony. The design involves planting and harvesting forage from a solid stand of alfalfa, effectively frontloading organically-bound N into the soil. After 2 or 3 years, terminate strips of alfalfa using an undercutter implement or herbicides, and sow intermediate wheatgrass, while leaving strips of living alfalfa intact. In this design, newly established wheatgrass could take advantage of a potentially elevated rates of N mineralization resulting from alfalfa termination, while also benefitting from ongoing N inputs from living, intercropped alfalfa.
This study is one of the first involving a detailed evaluation of how the N economy of a perennial cereal-legume intercrop functions with respect to sustaining productivity and improving on ecosystem services. Relative to unfertilized IWG treatments, IWG grain and biomass yields showed clear evidence of net facilitation by alfalfa after the establishment year. However, comparisons with fertilized and unfertilized IWG monocrops suggested that N inputs from alfalfa N fixation gradually relax but do not eliminate N limitation in the intercrop. In spite of N limitation, the intercrop was the only treatment that did not experience significant declines in IWG grain yields over the 5-year experiment. Estimated cumulative N inputs from alfalfa N fixation in the intercrop substantially exceeded N exports in grain and biomass, suggesting a legacy of good soil fertility for the crop following the IWG-ALF intercrop. Intercropping IWG with alfalfa had the surprising effect of almost doubling root biomass in surface horizons, and the synchrony of N supply and demand appeared to be high as N2O emissions in the intercrop were similar to the unfertilized IWG monocrop and significantly lower than the fertilized monocrop. Taken as a whole, the IWG-alfalfa intercrop encompasses a wide range of ecological nutrient management functions—reliance on legume BNF, improvement in N fertility as a consequence of cropping, reduced N2O emissions, and potential to increase SOM with greater root biomass. Research to bolster N availability in the early years of the intercrop, while alfalfa builds soil organic nitrogen for sustaining later years of productivity would be worthwhile.
We believe these findings will be useful as work continues to expand opportunities for ecological nutrient management in the ongoing effort to achieve zero hunger. In the near-term, IWG along with other perennial grains undergoing de novo domestication will most meaningfully contribute to food production through dual use management in which grain yields compliment forage for livestock (Ryan et al., 2018). Perennial grains can also be integrated into rotations with annual crops, enhancing yields and other services through ecological intensification of entire cropping systems (Ryan et al., 2018). Both the dual-use and rotation near term uses of perennial grains will be significantly enhanced by intercropping with a perennial legume. As investments and intensive breeding efforts continue to expand and improve on perennial grain yields, yield stability and other traits, this new crop “hardware” is expected to provide novel opportunities for increasing food security while improving soil health and contributing to climate change mitigation and adaptation (Crews et al., 2018).
The raw data supporting the conclusions of this article will be made available by the authors, without undue reservation.
EM conducted mixed linear model statistical analyses, as well as all statistical analyses of nitrous oxide emissions. LK, JB, and TC maintained and sampled the field experiment and carried out laboratory analyses. TC was lead author on the article, but all authors contributed to it and approved the final version.
Patagonia Provisions provided partial funding for 2 years of this research project including technician time and expenses related to N2O sampling. The Perennial Agriculture Project receives funding from The Malone Family Land Preservation Foundation. This operating foundation paid for all leaf tissue and soil analyses that were conducted at Kansas State University. Patagonia Provisions was not involved in the study design, collection, analysis, interpretation of data, the writing of this article or the decision to submit it for publication.
The authors declare that the research was conducted in the absence of any commercial or financial relationships that could be construed as a potential conflict of interest.
All claims expressed in this article are solely those of the authors and do not necessarily represent those of their affiliated organizations, or those of the publisher, the editors and the reviewers. Any product that may be evaluated in this article, or claim that may be made by its manufacturer, is not guaranteed or endorsed by the publisher.
We thank Mark Peoples for valuable advice, Madeline DuBois for assistance with data management, Westen Gehring for assistance with manuscript preparation and field technicians Ron Kinkelaar and Adam Gorrell. We also thank the reviewers of this manuscript for their perceptive and productive comments. We gratefully acknowledge Patagonia Provisions and the Perennial Agriculture Project, a joint project between The Land Institute and the Malone Family Land Preservation Foundation, for financial support of this research.
The Supplementary Material for this article can be found online at: https://www.frontiersin.org/articles/10.3389/fsufs.2022.755548/full#supplementary-material
Anglade, J., Billen, G., and Garnier, J. (2015). Relationships for estimating N2 fixation in legumes: incidence for N balance of legume-based cropping systems in Europe. Ecosphere 6, 37. doi: 10.1890/ES14-00353.1
Ayres, E., Dromph, K. M., Cook, R., Ostle, N., and Bardgett, R. D. (2007). The influence of below-ground herbivory and defoliation of a legume on nitrogen transfer to neighbouring plants. Funct. Ecol. 21, 256–263. doi: 10.1111/j.1365-2435.2006.01227.x
Bedoussac, L., Journet, E. P., Hauggaard-Nielsen, H., Naudin, C., Corre-Hellou, G., Jensen, E. S., et al. (2015). Ecological principles underlying the increase of productivity achieved by cereal-grain legume intercrops in organic farming. Agron. Sustain. Dev. 35, 9211–9935. doi: 10.1007/s13593-014-0277-7
Blesh, J., and Drinkwater, L. E. (2013). The impact of nitrogen source and crop rotation on nitrogen mass balances in the Mississippi River Basin. Ecol. Applicat. 23, 1017–1035. doi: 10.1890/12-0132.1
Bommarco, R., Kleijn, D., and Potts, S. G. (2013). Ecological intensification: harnessing ecosystem services for food security. Trends Ecol. Evol. 28, 230–238. doi: 10.1016/j.tree.2012.10.012
Bybee-Finley, K. A., and Ryan, M. R. (2018). Advancing intercropping research and practices in industrialized agricultural landscapes. Agriculture 8, 80. doi: 10.3390/agriculture8060080
Carlsson, G., and Huss-Danell, K. (2003). Nitrogen fixation in perennial forage legumes in the field. Plant Soil 253, 353–372. doi: 10.1023/A:1024847017371
Casamitjana, S. C. (2021). Kernza perennial grain and legume dual purpose polycultures in Wisconsin: effects of row spacing, fertilization, weed management, and legume intercrops. Master's thesis, Madison, WI: University of Wisconsin.
Cassman, K. G., and Grassini, P. (2020). A global perspective on sustainable intensification research. Nat. Sustain. 3, 262–268. doi: 10.1038/s41893-020-0507-8
Corentin, C., Sleiderink, J., Svane, S. F., Smith, A. G., Diamantopoulos, E., Desbroll, D. B., et al. (2022). Comparing the deep root growth and water uptake of intermediate wheatgrass (Kernza®) to alfalfa. Plant Soil. doi: 10.1007/s11104-021-05248-6
Crain, J., DeHaan, L., and Poland, J. (2021b). Genomic prediction enables rapid selection of high-performing genets in an intermediate wheatgrass breeding program. Plant Genome 14, e20080. doi: 10.1002/tpg2.20080
Crain, J., Haghighattalab, A., DeHaan, L., and Poland, J. (2021a). Development of whole-genome prediction models to increase the rate of genetic gain in intermediate wheatgrass (Thinopyrum intermedium) breeding. Plant Genome 14, e20089. doi: 10.1002/tpg2.20089
Crews, T. E., Blesh, J., Culman, S. W., Hayes, R. C., Jensen, E. S., Mack, M. C., et al. (2016). Going where no grains have gone before: from early to mid-succession. Agric. Ecosyst. Environ. 223, 223–238. doi: 10.1016/j.agee.2016.03.012
Crews, T. E., Carton, W., and Olsson, L. (2018). Is the future of agriculture perennial? Imperatives and opportunities to reinvent agriculture by shifting from annual monocultures to perennial polycultures. Glob. Sustain. 1, e11, 1-18. doi: 10.1017/sus.2018.11
Crews, T. E., and Peoples, M. B. (2004). Legume versus fertilizer sources of nitrogen: ecological tradeoffs and human needs. Agric. Ecosyst. Environ. 102, 279–297. doi: 10.1016/j.agee.2003.09.018
Crews, T. E., and Peoples, M. B. (2005). Can the synchrony of nitrogen supply and crop demand be improved in legume and fertilizer-based agroecosystems? A review. Nutr. Cycl. Agroecosyst. 72, 101–120. doi: 10.1007/s10705-004-6480-1
Crews, T. E., and Rumsey, B. E. (2017). What agriculture can learn from native ecosystems in building soil organic matter: a review. Sustainability 9, 578. doi: 10.3390/su9040578
Culman, S. W., Snapp, S. S., Ollenburger, M., Basso, B., and DeHaan, L. R. (2013). Soil and water quality rapidly responds to the perennial grain Kernza wheatgrass. Agron. J. 105, 735–744. doi: 10.2134/agronj2012.0273
Daryanto, S., Fu, B., Zhao, W., Wang, S., Jacinthe, P. A., and Wang, L. (2020). Ecosystem service provision of grain legume and cereal intercropping in Africa. Agric. Syst. 178, 102761. doi: 10.1016/j.agsy.2019.102761
Davidson, E. A., David, M. B., Galloway, J. N., Goodale, C. L., Haeuber, R., Harrison, J. A., et al. (2012). Excess nitrogen in the U.S. Environment: Trends, Risks, and Solutions. Issues Ecol. 15, 1–16.
Drinkwater, L. W., and Snapp, S. S. (2007). Nutrients in agroecosystems: rethinking the management paradigm. Adv. Agron. 92, 163–186. doi: 10.1016/S0065-2113(04)92003-2
Foley, J. A. (2011). Can we feed the world and sustain the planet? Sci. Am. 305, 61–65. doi: 10.1038/scientificamerican1111-60
Fustec, J., Lesuffleur, F., Mahieu, S., and Cliquet, J. B. (2010). Nitrogen rhizodeposition of legumes. a review. Agron. Sustain. Dev. 30, 57–66. doi: 10.1051/agro/2009003
Galloway, J. N., Dentener, F. J., Capone, D. G., Boyer, E. W., Howarth, R. W., Seitzinger, S. P., et al. (2004). Nitrogen cycles: past, present, and future. Biogeochemistry 70, 153–226. doi: 10.1007/s10533-004-0370-0
Gardner, J. B., and Drinkwater, L. E. (2009). The fate of nitrogen in grain cropping systems: a meta-analysis of 15N field experiments. Ecol. Applic. 19, 2167–2184. doi: 10.1890/08-1122.1
Gault, R. R., Peoples, M. B., Turner, G. L., Lilley, D. M., Brockwell, J., and Bergersen, F. J. (1995). Nitrogen fixation by irrigated lucerne during the first three years after establishment. Aust. J. Agri. Res. 46, 1401–1425. doi: 10.1071/AR9951401
Hayes, R. C., Newell, M. T., Crews, T. E., and Peoples, M. B. (2016). Perennial cereal crops: an initial evaluation of wheat derivatives grown in mixtures with a regenerating annual legume. Renew. Agric. Food Syst. 32, 276–290. doi: 10.1017/S1742170516000260
Hogh-Jensen, H., and Schjoerring, J. K. (1997). Interactions between white clover and ryegrass under contrasting nitrogen availability: N2 fixation, N fertilizer recovery, N transfer and water use efficiency. Plant Soil 197, 187–199. doi: 10.1023/A:1004289512040
Howarth, R., Ramakrishna, K., Choi, E., Elmgren, R., Martinelli, L., Mendoza, A., et al. (2005). “Nutrient management” in Millennium Ecosystem Assessment, eds K. Chopra, R. Leemans, K. Pushpam, and H. Simons (Washington, DC: World Resources Inst.), 295–311.
Hunter, M. C., Sheaffer, C. C., Culman, S. W., Lazarus, W. F., and Jungers, J. M. (2020). Effects of defoliation and row spacing on intermediate wheatgrass II: forage yield and economics. Agron. J. doi: 10.1002/agj2.20124
Hupe, A., Naether, F., Haase, T., Bruns, C., He, B. J., Dyckmans, J., et al. (2021). Evidence of considerable C and N transfer from peas to cereals via direct root contact but not via mycorrhiza. Nat. Portfolio 11, 11424. doi: 10.1038/s41598-021-90436-8
IFDC (International Fertilizer Development Center) (2022). Soaring Fertilizer Prices: A Threat to Food Security in Sub-Saharan Africa. Available online at: https://ifdc.org/2021/12/20/soaring-fertilizer-prices-a-threat-to-food-security-in-sub-saharan-africa/ (accessed January 22, 2022).
Jensen, E. S., Carlsson, G., and Hauggaard-Nielsen, H. (2020). Intercropping of grain legumes and cereals improves the use of soil N resources and reduces the requirement for synthetic fertilizer N: a global-scale analysis. Agron. Sustain. Dev. 40:5. doi: 10.1007/s13593-020-0607-x
Johansen, A., and Jensen, E. S. (1996). Transfer of N and P from intact or decomposing roots of pea to barley interconnected by an arbuscular mycorrhizal fungus. Soil Biol. Biochem. 28, 73–81. doi: 10.1016/0038-0717(95)00117-4
Jungers, J. M., DeHaan, L. H., Mulla, D. J., Sheafer, C. C., and Wyse, D. L. (2019). Reduced nitrate leaching in a perennial grain crop compared to maize in the Upper Midwest, USA. Agric. Ecosyst. Environ. 272, 63–73. doi: 10.1016/j.agee.2018.11.007
Jungers, J. M., DeHaan, L. R., Betts, K. J., Sheaffer, C. C., and Wyse, D. L. (2017). Intermediate wheatgrass grain and forage yield responses to nitrogen fertilization. Agron. J. 109, 462–472. doi: 10.2134/agronj2016.07.0438
King, A. E., and Blesh, J. (2018). Crop rotations for increased soil carbon: perenniality as a guiding principle. Ecol. Appl. 28, 249–261. doi: 10.1002/eap.1648
Kleijn, D., Bommarco, R., Fijen, T. P. M., Garibaldi, L. A., Potts, S. G., and van der Putten, W. H. (2018). Ecological intensification; Bridging the gap between science and practice. Trends Ecol. Evol. 34, 154–166. doi: 10.1016/j.tree.2018.11.002
Ladha, J. K., Pathak, H., Krupnik, T. J., Six, J., and van Kessel, C. (2005). Efficiency of fertilizer nitrogen in cereal production: retrospects and prospects. Adv. Agron. 87, 85–151. doi: 10.1016/S0065-2113(05)87003-8
Lassaletta, L., Billen, G., Garnier, J., Bouwman, L., Velazquez, E., Mueller, N., et al. (2016). Nitrogen use in the global food system: past trends and future trajectories of agronomic performance, pollution, trade, and dietary demand. Environ. Res. Lett. 11, 095007. doi: 10.1088/1748-9326/11/9/095007
Li, Q. Z., Sun, J. H., Wei, X. J., Christie, P., Zhang, F. S., and Li, L. (2011). Overyielding and interspecific interactions mediated by nitrogen fertilization in strip intercropping of maize with faba bean, wheat and barley. Plant Soil 339, 147–161. doi: 10.1007/s11104-010-0561-5
Li, S., Steen Jensen, E., Liu, N., Zhang, Y., and Dimitrova Mårtensson, L. M. (2021). Species interactions and nitrogen use during early intercropping of intermediate wheatgrass with a white clover service crop. Agronomy 11, 388. doi: 10.3390/agronomy11020388
Lithourgidis, A. S., Dordas, C. A., Damalas, C. A., and Vlachostergios, D. N. (2011). Annual intercrops: an alternative pathway for sustainable agriculture. Aust. J. Crop Sci. 5, 396–410.
Louarn, G., Pereira-Lopés, E., Fustec, J., Mary, B., Voisin, A., Cesar de Faccio Carvalho, P., et al. (2015). The amounts and dynamics of nitrogen transfer to grasses differ in alfalfa and white clover-based grass-legume mixtures as a result of rooting strategies and rhizodeposit quality. Plant Soil 389, 289–305. doi: 10.1007/s11104-014-2354-8
Malézieux, E., Crozat, Y., Dupraz, C., Laurans, M., Makowski, D., Ozier-Lafontaine, H., et al. (2009). Mixing plant species in cropping systems: concepts, tools and models: a review. Sustain. Agric. 29, 43–62. doi: 10.1007/978-90-481-2666-8_22
McGowan, A. R., Nicoloso, R. S., Diop, H. E., Roozeboom, K. L., and Rice, C. W. (2019). Soil organic carbon, aggregation and microbial community structure in annual and perennial biofuel crops. Agron. J. 111, 128–142. doi: 10.2134/agronj2018.04.0284
Meng, L., Zhang, A., Wang, F., Han, X., Wang, D., and Li, S. (2015). Arbuscular mycorrhizal fungi and rhizobium facilitate nitrogen uptake and transfer in soybean/maize intercropping system. Front. Plant Sci. 6, 339. doi: 10.3389/fpls.2015.00339
Necpálová, M., Anex, R. P. Jr., Kravchenko, A. N., Abendroth, L. J., Del Grosso, S. J., Dick, W. A., et al. (2014). What does it take to detect a change in soil carbon stock? A regional comparison of minimum detectable difference and experiment duration in the north central United States. J. Soil Water Conserv. 69, 517–531. doi: 10.2489/jswc.69.6.517
Okonkwo, C. I., Mbagwu, J. S. C., and Egwu, S. O. (2008). Nitrogen mineralization from prunings of three multipurpose legume and maize uptake in alley cropping system. Agro-Science 7, 143–148. doi: 10.4314/as.v7i2.1596
Palmer, C. D., Hamilton, V. L., Hoffman, B. R., Fahnestock, P. B., and Barker, W. L. (1992). Soil Survey of Saline County, Kansas. Washington, DC: USDA Soil Conservation Service.
Pelzer, E., Bourlet, C., Carlsson, G., Lopez-Bellido, R. J., Jensen, E. S., and Jeuffroy, M.-H. (2017). Design, assessment and feasibility of legume-based cropping systems in three European regions. Crop Pasture Sci. 68, 902–914. doi: 10.1071/CP17064
Peoples, M. B., Brockwell, J., Herridge, D. F., Rochester, I. J., Alves, B. J. R., Urquiaga, S., et al. (2009). The contributions of nitrogen-fixing crop legumes to the productivity of agricultural systems. Symbiosis 48, 1–17. doi: 10.1007/BF03179980
Peoples, M. B., Brockwell, J., Hunt, J. R., Swan, A. D., Watson, L., Hayes, R., et al. (2012). Factors affecting the potential contributions of N2 fixation by legumes in Australian pasture systems. Crop Pasture Sci. 63, 759–786. doi: 10.1071/CP12123
Peoples, M. B., Chalk, P. M., Unkovich, M. J., and Boddey, R. M. (2015). Can differences in 15N natural abundance be used to quantify the transfer of nitrogen from legumes to neighboring non-legume plant species? Soil Biol. Biochem. 87, 97–109. doi: 10.1016/j.soilbio.2015.04.010
Poirier, V., Roumet, C., and Munson, A. D. (2018). The root of the matter: linking root traits and soil organic matter stabilization processes. Soil Biol. Biochem. 120, 246–259. doi: 10.1016/j.soilbio.2018.02.016
Post, W. M., and Kwon, K. C. (2000). Soil carbon sequestration and land-use change: processes and potential. Glob. Chang. Biol. 6, 317–327. doi: 10.1046/j.1365-2486.2000.00308.x
Rasse, D. P., Rumpel, C., and Dignac, M. F. (2005). Is soil carbon mostly root carbon? Plant Soil 269, 341–356. doi: 10.1007/s11104-004-0907-y
Reilly, E. C. (2021). Intermediate wheatgrass nitrogen dynamics: Nitrate leaching prevention and nitrogen supply via legume intercrops. M.S. Thesis, University of Minnesota, Minneapolis, MN.
Robertson, G. P. (1997). “Nitrogen use efficiency in row-crop agriculture: crop nitrogen use and soil nitrogen loss,” in Ecology in Agriculture, ed L. Jackson (New York, NY: Academic Press), 347–365. doi: 10.1016/B978-012378260-1/50011-7
Robertson, G. P., Bruulsema, T. W., Gehl, R. J., Kanter, D., Mauzerall, D. L., Rotz, C. A., et al. (2012). Nitrogen-climate interactions in US agriculture. Biogeochemistry. 114, 41–70. doi: 10.1007/s10533-012-9802-4
Robertson, G. P., Wedin, D., Groffman, P. M., Blair, J. M., Holland, E. A., Nadelhoffer, K. J., et al. (1999). “Soil carbon and nitrogen availability: nitrogen mineralization, nitrification, and soil respiration potentials,” in Standard Soil Methods for Long-Term Ecological Research, eds. G. P. Robertson, D. C. Coleman, C. S. Bledsoe, and P. Sollins (New York, NY: Oxford University Press), 258–271.
Rockstrom, J., Steffen, W., Noone, K., Persson, A., Chapin, F. S. III., Lambin, E. F., et al. (2009). A safe operating space for humanity. Nature 461, 472–475. doi: 10.1038/461472a
Rodriguez, C., Carlsson, G., Englund, J. E., Flöhr, A., Pelzer, E., Jeuffroy, M. H., et al. (2020). Grain legume-cereal intercropping enhances the use of soil-derived and biologically fixed nitrogen in temperate agroecosystems. Europ. J. Agron. 118, 1–11. doi: 10.1016/j.eja.2020.126077
Ruan, L., Bhardwaj, A. K., Hamilton, S. K., and Robertson, G. P. (2016). Nitrogen fertilization challenges the climate benefit of cellulosic biofuels. Environ. Res. Lett. 11, 064007. doi: 10.1088/1748-9326/11/6/064007
Ryan, M. R., Crews, T. E., Culman, S. W., DeHaan, L. R., Hayes, R. C., Jungers, J. M., et al. (2018). Managing for multifunctionality in perennial grain crops. Bioscience 68, 294–304. doi: 10.1093/biosci/biy014
Sanginga, N., Vanlauwe, B., and Danso, S. K. A. (1995). Management of biological N2 fixation in alley cropping systems: Estimation and contribution to N balance. Plant Soil 174, 119–141. doi: 10.1007/BF00032244
Schipanski, M. E., and Drinkwater, L. E. (2012). Nitrogen fixation in annual and perennial legume-grass mixtures across a fertility gradient. Plant Soil 357, 147–159. doi: 10.1007/s11104-012-1137-3
Schmidt, M. W. I., Torn, M. S., Abiven, S., Dittmar, T., Guggenberger, G., Janssens, I. A., et al. (2011). Persistence of soil organic matter as an ecosystem property. Nature 478, 49–56. doi: 10.1038/nature10386
Sprunger, C. D., Martin, T., and Mann, M. (2020). Systems with greater perenniality and crop diversity enhance soil biological health. Agric. Environ. Lett. 5:e20030. doi: 10.1002/ael2.20030
Sprunger, C. D., Oates, L. G., Jackson, R. D., and Robertson, G. P. (2017). Plant community composition influences fine root production and biomass allocation in perennial bioenergy cropping systems of the upper Midwest, USA. Biomass Bioenergy 105, 248–258. doi: 10.1016/j.biombioe.2017.07.007
Steffen, W., Richardson, K., Rockstrom, J., Cornell, S. E., Fetzer, I., Bennett, E. M., et al. (2015). Planetary boundaries: guiding human development on a changing planet. Science 347, 1259855. doi: 10.1126/science.1259855
Tautges, N. E., Jungers, J. M., DeHaan, L. R., Wyse, D. L., and Scheaffer, C. C. (2018). Maintaining grain yields of the perennial cereal intermediate wheatgrass in monoculture v. bi-culture with alfalfa in the Upper Midwestern USA. J. Agric. Sci. 156, 758–773. doi: 10.1017/S0021859618000680
Thilakarathna, M. S., McElroy, M. S., Chapagain, T., Papadopoulos, Y. A., and Raizada, M. N. (2016b). Belowground nitrogen transfer from legumes to non-legumes under managed herbaceous cropping systems. a review. Agron. Sustain. Dev. 36, 58. doi: 10.1007/s13593-016-0396-4
Thilakarathna, M. S., Papadopoulos, Y. A., Rodd, A. V., Grimmett, M., Fillmore, S. W. E., Crouse, M., et al. (2016a). Nitrogen fixation and transfer of red clover genotypes under legume-grass forage based production systems. Nutr. Cycl. Agroecosyst. 106, 233–247. doi: 10.1007/s10705-016-9802-1
Townsend, M. A., Young, D. P., and Macko, S. A. (2003). Kansas case study applications of nitrogen-15 natural abundance method for identification of nitrate sources. J. Hazardous Substance Res. 4, 1–22. doi: 10.4148/1090-7025.1029
United Nations (2021). Sustainable Development Goals, Goal 2: Zero Hunger. Available online at: https://www.un.org/sustainabledevelopment/hunger/ (accessed November 24, 2021).
Unkovich, M., Herridge, D., Peoples, M., Cadisch, G., Boddey, B., Giller, K., et al. (2008). Measuring Plant-associated Nitrogen Fixation in Agricultural Systems. Canberra: ACIAR.
Vandermeer, J. H. (1990). “Intercropping,” in Agroecology, eds C. R. Carroll, J. H. Vandermeer, and P. M. Rosset (New York, NY: MacGraw Hill), 481–516.
Vitousek, P. M., Shearer, G., and Kohl, D. H. (1989). Foliar 15N natural abundance in Hawaiian rainforest: patterns and possible mechanisms. Oecologia 78, 383–388. doi: 10.1007/BF00379113
Walley, F. L., Tomm, G. O., Matus, A., Slinkard, A. E., and van Kessel, C. (1996). Allocation and cycling of nitrogen in an alfalfa-bromegrass sward. Agron. J. 88, 834–843. doi: 10.2134/agronj1996.00021962008800050025x
Yang, Y., Tilman, D., Furey, G., and Lehman, C. (2019). Soil carbon sequestration accelerated by restoration of grassland biodiversity. Nat. Commun. 10, 718. doi: 10.1038/s41467-019-08636-w
Keywords: alfalfa, intermediate wheatgrass, Kernza®, perennial grain, UN Sustainable Development Goal, zero hunger, ecological nutrient management
Citation: Crews TE, Kemp L, Bowden JH and Murrell EG (2022) How the Nitrogen Economy of a Perennial Cereal-Legume Intercrop Affects Productivity: Can Synchrony Be Achieved? Front. Sustain. Food Syst. 6:755548. doi: 10.3389/fsufs.2022.755548
Received: 09 August 2021; Accepted: 11 February 2022;
Published: 29 April 2022.
Edited by:
Jennifer Blesh, University of Michigan, United StatesReviewed by:
Alison Nord, University of Michigan, United StatesCopyright © 2022 Crews, Kemp, Bowden and Murrell. This is an open-access article distributed under the terms of the Creative Commons Attribution License (CC BY). The use, distribution or reproduction in other forums is permitted, provided the original author(s) and the copyright owner(s) are credited and that the original publication in this journal is cited, in accordance with accepted academic practice. No use, distribution or reproduction is permitted which does not comply with these terms.
*Correspondence: Timothy E. Crews, Y3Jld3NAbGFuZGluc3RpdHV0ZS5vcmc=
Disclaimer: All claims expressed in this article are solely those of the authors and do not necessarily represent those of their affiliated organizations, or those of the publisher, the editors and the reviewers. Any product that may be evaluated in this article or claim that may be made by its manufacturer is not guaranteed or endorsed by the publisher.
Research integrity at Frontiers
Learn more about the work of our research integrity team to safeguard the quality of each article we publish.