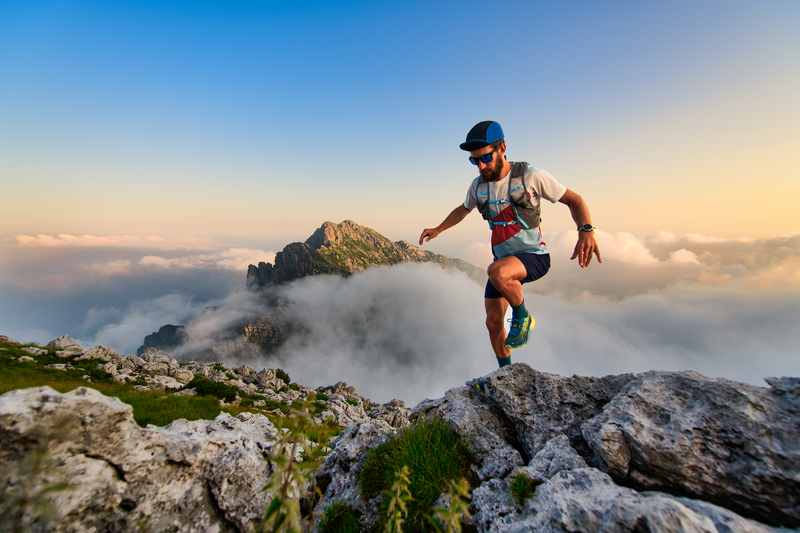
95% of researchers rate our articles as excellent or good
Learn more about the work of our research integrity team to safeguard the quality of each article we publish.
Find out more
ORIGINAL RESEARCH article
Front. Sustain. Food Syst. , 13 January 2023
Sec. Crop Biology and Sustainability
Volume 6 - 2022 | https://doi.org/10.3389/fsufs.2022.1076844
This article is part of the Research Topic Elucidating the roles of plant-microbe interactions, biological control and plant breeding in promotion of soil health and sustainable crop production systems View all 5 articles
Introduction: Maize is a major staple cereal crop grown and consumed globally. However, due to climate change, extreme heat and drought stresses are greatly affecting its production especially in sub-Saharan Africa. The use of a bio-based approach to mitigate drought stress is therefore suggested using plant growth-promoting rhizobacteria (PGPR).
Methods: This study investigated the abilities of 1-aminocyclopropane-1-carboxylate (ACC) deaminase producing PGPR Pseudomonas sp. MRBP4, Pseudomonas sp. MRBP13 and Bacillus sp. MRBP10 isolated from maize rhizosphere soil, to ameliorate the effect of drought stress in maize genotypes MR44 and S0/8/W/I137TNW//CML550 under two water regimes; mild drought stress (50% FC) and well-watered conditions (100% FC). The rhizobacterial strains were identified by 16S rRNA sequencing and biochemical tests, and evaluated for plant growth-promoting and abiotic stress tolerance traits.
Results and discussion: The synergistic effect of the bacterial strains had a highly significant (p < 0.001) effect on the total soluble sugar, soil moisture content and relative water content, which were enhanced under water-stress in the inoculated plants. Relative water content was significantly highest (p < 0.001) in maize plants co-inoculated with Pseudomonas sp. MRBP4 + Bacillus sp. MRBP10 (60.55%). Total chlorophyll content was significantly enhanced in maize seedlings sole inoculated with Pseudomonas sp. MRBP4, Pseudomonas sp. MRBP13, and co-inoculated with Pseudomonas sp. MRBP13 + Bacillus sp. MRBP10 by 15.91%, 14.99% and 15.75% respectively, over the un-inoculated control. Soil moisture content increased by 28.67% and 30.71% compared to the un-inoculated control when plants were inoculated with Pseudomonas sp. MRBP4 + Bacillus sp. MRBP10 and Pseudomonas sp. MRBP4 + Bacillus sp. MRBP10 respectively. The interactive effect of genotype × bacteria significantly enhanced biomass production. Leaf area was highest in maize plants co-inoculated with Pseudomonas sp. MRBP4 + Pseudomonas sp. MRBP13 (212.45 ± 0.87 cm2) under drought stress. Treatment of maize seeds with Pseudomonas sp. MRBP 4 + Pseudomonas sp. MRBP13 + Bacillus sp. MRBP10 significantly increased the root length (10.32 ± 0.48 cm) which enhanced survival of the maize seedlings. Bioinoculation of maize seeds with these strains could boost maize production cultivated in arid regions.
Maize (Zea mays L.) is an essential staple crop grown globally, ranking third after rice and wheat (Subbaiah et al., 2016). It is widely consumed as food in different forms in South America, Asia and sub-Saharan Africa (SSA). Maize yield in SSA dropped in western and southern Africa to below 2 t ha−1, and <1.5 t ha−1 respectively, due to changes in climatic conditions (Cairns et al., 2013). In South Africa, maize is majorly produced in the North-West and the Free State Provinces using irrigation systems, unlike other African countries that experience rains during the planting season. The impact of the El Niño induced drought of 2016 was mostly felt in South Africa with the resultant yield reductions in maize production and economic losses to the Rainbow Nation. Drought stress leads to a reduction in plant nutrient uptake which causes poor root growth and low photosynthesis resulting in low yields.
Insufficient moisture in the soil, coupled with contamination by heavy metals or hydrocarbons and environmental stresses such as elevated temperatures, heat, drought, and salinity (Chibuike and Obiora, 2014; Etesami and Maheshwari, 2018), could affect the quality of soil required for cultivation of crops as it becomes poor and ceases to support life. Researches have shown that microorganisms residing in the rhizosphere soil play major roles in enhancing soil quality and promote healthy plant growth (Ojuederie et al., 2019). Bacteria in soil aid in the recycling of nutrients (carbon and nitrogen), degradation of organic matter, and some can produce plant growth-promoting (PGP) substances even under unfavorable environmental conditions (Jacoby et al., 2017). Enrichment of bacterial communities within the rhizosphere soil by plant root exudates creates a rhizospheric effect as the exudates influences the composition of the microbiome (Yin et al., 2021), which is also dependent on the type of plant, and the genotype.
Plant growth-promoting rhizobacteria (PGPR) when inoculated on plants, could enhance plant growth using both direct and indirect mechanisms such as synthesis of 1-aminocyclopropane-1-carboxylate (ACC) deaminase, sequestration of iron, mobilization of phosphorus in soils, and induced systemic resistance (Olanrewaju et al., 2017; Etesami and Maheshwari, 2018; Ojuederie et al., 2019), synthesis of phytohormones like indole-3-acetic acid (Gujral et al., 2013; Grover et al., 2014; Dutta et al., 2015), solubilization of phosphate (Timmusk et al., 2014; Otieno et al., 2015; Zeng et al., 2016; Li et al., 2017; Saikia et al., 2018), and production of exopolysaccharides (Sandhya et al., 2010; Timmusk et al., 2014; Niu et al., 2018; Igiehon et al., 2019).
PGPR are the vital players in the quest for sustainable plant development during unfavorable environmental conditions due to the effects of climate change and anthropogenic activities (Enebe and Babalola, 2018). Some studies have shown the abilities of rhizospheric bacteria mostly of the genera Pseudomonas (Chandra et al., 2018; Niu et al., 2018), Bacillus (Vardharajula et al., 2011; Vardharajula, 2014; Dutta et al., 2015; Saleem et al., 2018), Burkholderia (Maxton et al., 2018), Enterobacter (Saleem et al., 2018), and Azospirillum (Curá et al., 2017), to ameliorate the impact of drought stress on crop plants. Besides these PGPR, other soil microorganisms such as the Arbuscular mycorrhizal fungi (Wu and Zou, 2017; Li J. et al., 2019; Posta and Duc, 2019), nitrogen-fixing bacteria (Igiehon et al., 2019), and actinobacteria (Li H. et al., 2019; Chukwuneme et al., 2020) have also been harnessed in boosting plant growth under drought stress conditions.
Mitigating the negative impact of drought stress on crop production requires a holistic approach. Utilization of drought-tolerant rhizobacteria strains with multifunctional growth-promoting traits as bio-inoculants for healthy plant growth under abiotic stress conditions is a cheap and eco-friendly approach to win the war against the detrimental effects of drought stress on crop production. This study was based on the hypotheses that (i) bioinoculation of maize genotypes with PGPR will ameliorate the effects of drought stress on maize genotypes (ii) the performance of PGPR isolated from maize rhizosphere soil is influenced by their plant growth promoting properties, and (iii) co-inoculation of PGPR strains is more effective in mitigation of drought stress than sole inoculation. Considering this, our research aimed to investigate the ability of three ACC deaminase producing rhizobacteria strains with multifunctional growth-promoting factors isolated from maize rhizosphere soil, to enhance the performance of maize genotypes under partially watered and well-watered conditions. These strains were chosen based on their ability to grow at a low water activity (0.919Aw), elevated temperature of 50°C, and ability to produce multiple plant growth-promoting substances.
Maize rhizosphere soil was collected at a depth of 5–15 cm randomly, from five locations on a maize field at the Agricultural Research Council (ARC) Potchefstroom South Africa (Latitude 26° 43' 49.2” and Longitude 27° 3' 43.3”). Large particles were removed by sieving with a 2 mm sieve, and a composite soil sample made. The physicochemical characteristic of the soil was analyzed by the Agricultural Research Council (ARC) analytical laboratory, Africa (Supplementary Table 1). Soil organic carbon and organic matter were determined using the Walkley-Black titration method (Walkley and Black, 1934; Motsara and Roy, 2008). The method of Olsen and Sommers (1982) was used for determination of available phosphorus. Available potassium was determined by ICP-OES on an ammonium acetate extraction of the soil (Toth and Prince, 1949; Motsara and Roy, 2008), while total nitrogen was determined by the Kjeldahl method (Bremner, 1960). Soil moisture was measured using the method of Motsara and Roy (2008).
Bacteria strains were obtained using a pour plate method on nutrient agar (NA; Merck, South Africa) according to the method of Abiala et al. (2015), and incubated for 24 h at 28 ± 2°C. Pure cultures of strains were obtained which were subsequently characterized using morphological features and biochemical tests. The method of Cheesbrough (2006) was used for Gram stain, catalase activity, oxidase, nitrate reduction and starch hydrolysis tests. Hydrogen cyanide (HCN) was determined using the method of Kavamura et al. (2013). Development of orange color after 48–72 h at 28°C incubation was indicative of HCN production. Ammonia production was also determined using the method of Kavamura et al. (2013) by inoculating freshly grown cultures (18–24 h) of each isolate in 10 mL of peptone water and then incubated at 28°C for 48 h. Ammonia production was assessed by the development of faint yellow to deep yellow color by the strains in different proportions in 1.5 μL Eppendorf tubes containing 50 μL of Nessler's reagent (Dey et al., 2004).
Three bacterial strains Pseudomonas sp. MRBP4, Pseudomonas sp. MRBP13, and Bacillus sp. MRBP10 were selected for greenhouse study based on their plant growth-promoting traits and ability to withstand low water activity in vitro. 1-aminoccylopropane-1-carboxylate (ACC) deaminase activity was determined in accordance with the method of Ali et al. (2013) using tryptone soy broth.
The method of Kavamura et al. (2013) was used to determine indole-3-acetic acid (IAA) production by culturing the strains in triplicate in tryptophan nutrient broth (Oxoid Chemicals). The cultures were incubated with constant shaking for 48 h at 28 ± 2°C. After centrifugation of turbid cultures for 20 min at 10,000 rpm, 2 mL of the supernatant was mixed with 4 mL of Salkowsky reagent. After 20 min of the mixture kept in the dark, light absorption at 530 nm was measured using a spectrophotometer (Themospectronic Merck SA). The IAA production by each isolate in mg L−1 was determined by comparing the light absorption to a standard curve prepared from pure IAA solutions (5, 10, 20, 50, and 100 mg L−1).
Strains were grown in TSB medium amended with sucrose (10%) at pH 7.5 containing discs of sterile 5 mm filter paper in petri plates. Each disc was inoculated with 2 mL culture of each isolate for EPS production and incubated at 28°C for 48 h. The presence of EPS was confirmed from the precipitation observed after mixing a part of the mucoid substance formed around the discs in 2 mL of absolute ethanol (Paulo et al., 2012; Kavamura et al., 2013).
Each isolate was screened for phosphate solubilization on Pikovskaya's agar (PKA) medium according to the method of Paul and Sinha (2017). The strains were obtained using pour plate method and incubated for 48 h at 28 ± 2°C. Distinct colonies with halo zones were identified and the halo zone surrounding the colonies measured. Phosphate solubilization index was calculated using the formula of Premono et al. (1996).
The rhizobacteria strains were tested in vitro for the ability to grow on a medium with low water activity (0.919Aw; Kavamura et al., 2013). This was achieved by inoculating TSA medium (10%) supplemented with 450 g L−1 D. sorbitol (Sigma, France), with 24 h culture of each isolate, incubated at 40°C. Strains were also tested on their ability to withstand high temperature of 50°C by inoculating 10 mL of sterilized TSB with 24 h culture of each isolate. The inoculated test tubes were incubated at 120 rpm for 24 h. Subsequently the optical density (OD) was measured at 600 nm using a UV spectrophotometer (Thermo Spectronic; Merck, South Africa). The strains were also evaluated for water-deficit stress tolerance by inoculating each isolate in 10 mL of sterilized TSB broth containing 20% polyethylene glycol (PEG) 8000 and subsequently incubated for 24 h at 28°C on a rotatory shaker. The OD was measured at 600 nm using a UV spectrophotometer (Thermo Spectronic; Merck, South Africa).
A ZR Soil Bacterial DNA MiniPrep extraction kit (Zymo Research, Irvine, CA, USA) was used for DNA extraction in accordance with the manufacturer's instructions. Inqaba Biotechnical Industrial (Pty) Ltd, Pretoria, South Africa sequenced the purified PCR products using PRISMTM Ready Reaction Dye Terminator Cycle Sequencing Kit. The partial 16S rRNA gene of each bacterial isolate was amplified Universal bacteria primers 341F and 907R (Fukuda et al., 2016). A 25-μl volume cocktail was made composed of 12.5 μL of 2x PCR Master Mix (0.05 U/μl Taq DNA polymerase, 4 mM MgCl2 and 0.4 mM dNTPs (Fermentas), 0.5 μL of forward primer (341F), 0.5 μL of reverse primer (907R), 2.0 μL of template DNA, and 9.5 μL of nuclease free water. The PCR cocktail was subjected to 35 cycles in a Bio-Rad C-1000 thermocylcer beginning with an initial denaturation temperature of 94°C for 2 min, then 94°C for 30 s followed by an annealing temperature of 59°C for 1 min, extension at 72°C for 2 min and a final extension at 72°C for 8 min.
The chromatographs obtained were subjected to quality check using Chromas Lite version 6.5 (Technelysium, 2012). The cleaned chromatographs were edited using Bio Edit Sequence Alignment Editor (Hall, 1999). Subsequently, consensus sequences were generated and a Blast search made on the NCBI database (www.ncbi.nlm.nih.gov/Blast.cgi), using the Basic Alignment Search Tool (BLASTn) in order to identify the closest representative strains of the bacterial strains. Sequences of the strains were deposited in the GenBank and accession numbers given by the GenBank (Supplementary Table 2). The evolutionary history was inferred using the Neighbor-Joining method (Saitou and Nei, 1987) and evolutionary distances computed using the Tamura-Nei method (Tamura and Nei, 1993). Evolutionary analyses was conducted in MEGA11 (Tamura et al., 2021).
A pot experiment was conducted in the greenhouse at the North West University to assess the effectiveness of the rhizobacterial strains for enhancement of maize growth promotion under water-deficit stress. Field soil was used for the experiment with two maize varieties: a drought tolerant genotype MR44 and a recombinant inbreed line S0/8/W/I137TNW//CML550. The seeds of both genotypes were surface sterilized using 70% ethanol for 5 min, treated with 2% sodium hypochlorite for 10 min with a drop of Tween 20, and rinsed at least thrice with sterile distilled water. The bacteria strains MRBP4, MRBP10, and MRBP13 were prepared by growing each isolate in 500 mL of sterilized TSB on an orbital shaker at a speed of 120 rpm for 72 h at 28°C. Subsequently, bacterial cells were harvested by centrifugation for 15 min at 10,000 rpm, and the pellets washed twice with sterile distilled water and re-suspended in phosphate buffer (0.01 M, pH 7.0). The OD was adjusted to an absorbance of 1.4 at 600 nm using a spectrophotometer (Thermo Spectronic, Merck, SA). Pre-germinated maize seeds of each genotype were inoculated by placing them in a suspension of each bacterium alone and co-inoculated (108 CFU mL−1) for 12 h. Afterwards, the inoculated seeds were air dried in a laminar flow cabinet for 1 h. Control seeds were suspended in sterile distilled water for the same period, and air dried. Three inoculated and control seeds were sown in 36 cm pots containing 10 kg of sterile soil and thinned to one plant per pot 2 weeks after germination. The experiment was conducted between October and December 2018 and 2019. The average maximum day temperature ranged between 32 and 40°C as this was the summer period. The experiment was set up as a 2 × 2 × 8 factorial in a completely randomized design with three replications (Figure 1). The factors were:
Figure 1. Maximum Likelihood phylogenetic tree based on 16S rRNA gene sequence, using the neighbor-joining (NJ) algorithm. It shows the evolutionary relationships of the 3 rhizobacteria strains and their closest representative species obtained from the GenBank with Aeribacillus composti (NR159152) as the outgroup. The numbers at the nodes represent the levels of bootstrap support based on 1,000 resampled data sets. Bootstrap confidence levels >50% are indicated at the internode.
Two maize genotypes-M1-Genotype S0/8/W /I137TNW/ /CML550 an inbreed line, M2-Genotype MR44 a drought tolerant variety.
Two levels of water field capacity partially watered at 50% FC (450 mL of water daily), and well-watered at 100% FC (900 mL of water daily).
Eight levels of bacterial inoculation-B0, No inoculation; B1, inoculation with Pseudomonas sp. MRBP4; B2, inoculation with Pseudomonas sp. MRBP13; B3, inoculation with Bacillus sp. MRBP10; B1B2, co-inoculation with Pseudomonas sp. strain MRBP4 and Pseudomonas sp. strain MRBP13. B1B3, co-inoculation with Pseudomonas sp. MRBP4 and Bacillus sp. MRBP10; B2B3, co-inoculation with Pseudomonas sp. MRBP13 and Bacillus sp. MRBP10; and B1B2B3, inoculation with the three strains Pseudomonas sp. MRBP4 + Pseudomonas sp. MRBP13 + Bacillus sp. MRBP10. Two weeks after germination, water stress was imposed. 50 mL of each bacteria inoculum was used for root inoculation of seedlings at the 2nd and 4th week after commencement of water stress.
Plants were harvested 49 days after sowing. Weekly data was collected on the leaf area, number of leaves and shoot length. The shoot and root fresh weights and the shoot and root lengths were measured at the end of the 5th week of drought imposition. Shoots of each plant were cut at the base and dried at 72°C for 48 h, and dry weight taken using an electronic scale. The roots of the same plants with soil were harvested, washed carefully to separate roots, dried at 72°C for 48 h, and weighed using an electronic scale.
The leaf relative water content (RWC) for each treatment was measured according to the method described by Naveed et al. (2014) using the Flag leaves. The fresh weights of cut leaves from each treatment were measured and placed in distilled water in a 50 mL tube for 24 h at 4°C in the dark. Subsequently, the soaked leaves were carefully blotted with tissue paper and fully turgid weight measured. The dry weight was measured after the leaf samples were oven dried at 72°C for 24 h (Naveed et al., 2014). RWC was computed using the equation described by Naveed et al. (2014).
The total soluble sugar content was estimated by the method of Dey (1990). The absorbance was read at 485 nm using a spectrophotometer (Thermo Spectronic, Merck, SA) and the equivalent concentration of total soluble sugar determined against a standard curve prepared by using pure glucose solution. The amount of sugar was expressed as mg g−1 DW−1.
A dimethyl sulphoxide (DMSO) method was used to determine the chlorophyll pigment of the leave samples after harvest following the method of Hiscox and Israelstam (1979). 0.5 g leaf tissue of each sample was cut into little pieces and placed in test tubes containing 7 mL DMSO. The test tubes were incubated in a water bath at 65°C for 20 min. The supernatant obtained was decanted into 15 mL graduated tubes and made up to 10 mL with 3 mL DMSO and assayed immediately. The optical density was read at 480, 645, and 663 nm using DMSO as blank. Chlorophyll a, b, total chlorophyll, and carotenoid contents were determined according to the method of Arnon (1949).
Data collected were checked for test of normality using the “proc univariate” program of SAS software 9.4 (SAS, 2014) and square-root transformed if not in a normal distribution curve before being subjected to analysis of variance (ANOVA). Significantly different means were separated using Tukey's Studentized Range (HSD) and Duncan Multiple Range post-hoc test (p ≤ 0.05). Data were analyzed using the Statistical Analysis System software version 9.4 (SAS, 2014).
The rhizobacteria strains used in this study were identified using biochemical and morphological traits and RNA sequencing. Two of the strains were gram-negative rod-shaped bacteria of the genus Pseudomonas (MRBP4 and MRBP13) while the third MRBP10 was of the genus Bacillus (Table 1). Ammonia production was high in MRBP4 while MRBP10 had a very high catalase response (Table 1). Hydrogen cyanide was only produced by MRBP13. The Maximum Likelihood method used for phylogenetic tree reconstruction based on the Tamura-Nei method (Tamura and Nei, 1993) corresponded with the BLAST results obtained from the National Center for Biotechnology Information (NCBI) database. The Strains had a very high evolutionary relationship with the reference strains, with a bootstrap value of 100% (Figure 1). MRBP4 had a very high similarity with Pseudomonas sp. (KX254973, KJ831556, and MH156648), MRBP13 had extremely high similarity with Pseudomonas sp. (MN94409) as well as Pseudomonas mediterranea (MN 712327) and Pseudomonas lini (MK883138). The third strain MRBP10 had high similarity with Bacillus sp. (HM566589).
The strains were screened in vitro for PGP traits. Exopolysaccharide (EPS) production was highest in Bacillus sp. MRBP10 (0.87 ± 0.03 mm) but was not significantly different from EPS production by Pseudomonas sp. MRBP4 and Pseudomonas sp. MRBP13 (Table 2). However, the ability to solubilize phosphate in the form of tricalcium phosphate, synthesize indole-3-acetic acid and ACC deaminase activity were highest in Pseudomonas sp. MRBP13 (Table 2).
The three strains Pseudomonas sp. MRBP4 (MG953559), Pseudomonas sp. MRBP13 (MG953568), and Bacillus sp. MRBP10 (MG953564) were evaluated for their ability to grow on a medium amended with 20% PEG 8000 and could grow in nutrient broth incubated at temperatures of 28, 45, and 50°C with 24 h bacteria cultures. The strains grew on medium containing 405 g L−1 sorbitol with lower water activity of 0.919Aw incubated at 40°C. Bacillus sp. MRBP10 had the highest growth in medium amended with 20% PEG (0.62 ± 0.01 nm) and also had the highest growth at a temperature of 50°C (Table 3). Since the strains were able to grow with an OD > 0.5 on medium with 20% PEG and grew at a high temperature of 50°C with OD > 0.1, they were regarded as zero-tolerant and temperature tolerant bacteria strains.
From the combined analysis of variance for the 2-year evaluation of maize genotypes under drought stress (2018 and 2019), the main effect of bacterial inoculation had a significant effect (p < 0.05) on the root fresh weight (RFW) of maize seedlings under mild drought stress but a non-significant effect on the shoot fresh weight (SFW) as presented in Figure 2A. Nevertheless, SFW was highest in maize seedlings co-inoculated with Pseudomonas sp. MRBP4 + Pseudomonas sp. MRBP 13 (6.68 ± 0.09 g). Inoculation with Pseudomonas sp. MRBP4 produced the highest RFW which was 15.98% higher than the un-inoculated control. Likewise, co-inoculation of maize seedlings with Pseudomonas sp. MRBP 4 + Pseudomonas sp. MRBP 13 increased the RFW by 6.97% over the un-inoculated control. Root dry weight (RDW) and total plant biomass (TPB) were also significantly highest in maize seedlings inoculated with Pseudomonas sp. MRBP 4 which was increased by 44.19 and 17.69% respectively, over the un-inoculated control. An increment of RDW and TPB by 37.21 and 10.47% was also observed over the un-inoculated control by co-inoculating the seedlings with Pseudomonas sp. MRBP 4 + Pseudomonas sp. MRBP 13 (Figure 2B). Nonetheless, the shoot dry weight (SDW) was highest in maize seedlings inoculated with Pseudomonas sp. MRBP 13 (3.17 ± 0.07 g) which was not significantly different from the co-inoculated plants (3.15 ± 0.07 g).
Figure 2. Effect of bacterial inoculation on (A) shoot and root FW (B) root FW, shoot FW, and total plant biomass of maize plants. Values presented as means ± standard error (n = 24). Means with different letters are significantly different from each other [Tukeys Honest Significant Difference (p < 0.05)]. B0, un-inoculated control; B1, Pseudomonas sp. MRBP4; B2, Pseudomonas sp. MRBP13; B3, Bacillus sp. MRBP10; B1B2, Pseudomonas sp. MRBP4 + Pseudomonas sp. MRBP13; B1B3, Pseudomonas sp. MRBP4 + Bacillus sp. MRBP10; B2B3, Bacillus sp. MRBP10 + Pseudomonas sp. MRBP13; B1B2B3, Pseudomonas sp. MRBP4 + Pseudomonas sp. MRBP13 + Bacillus sp. MRBP10.
Table 4 shows the interactive effect of the treatments on biomass production of maize seedlings under mild drought stress (50% FC) and well-watered condition (100% FC). At 50% FC, the co-inoculated treatments had lower SFWs. SFW was significantly higher (p ≤ 0.05) in genotype MR44 sole inoculated with Pseudomonas sp. MRBP4 and then MR44 inoculated with Pseudomonas sp. MRBP13 which were significantly higher than the un-inoculated control by 59.86 and 43.81%, respectively. Similarly, the RFW was highest in maize genotype MR44 sole inoculated with Pseudomonas sp. MRBP4 followed by MR44 inoculated with Pseudomonas sp. MRBP 13. This was higher than the un-inoculated control of MR44 by 80.97 and 45.11%, respectively (Table 4). Co-inoculation with the three rhizobacteria strains; Pseudomonas sp. MRBP 4 + Pseudomonas sp. MRBP13 + Bacillus sp. MRBP 10 (BIB2B3) increased the SFW under well-watered condition in CML550 by 10.02% while co-inoculation with Pseudomonas sp. MRBP 4 + Pseudomonas sp. MRBP13 increased it by 9.98% over the un-inoculated control.
Table 4. Effect of treatments on biomass production of maize genotypes under drought stress (50% FC) and well-watered (100% FC) conditions.
A similar trend was observed in the SDW, RDW and TPB in both genotypes CML550 and MR44 under mild drought stress. RDW and TPB were significantly highest in Pseudomonas sp. MRBP4 under drought stress which was greater than the un-inoculated control by 84.87 and 85.24%, respectively (Table 4). Treatment MR44+B2 (MR44 inoculated with Pseudomonas sp. MRBP13 under drought stress) also had high SDW (3.23 g), RDW (1.72 g), and TPB (3.08 g). SFW was significantly higher in genotype MR44 by 7.43% compared to CML 550 (Figure 3A). Likewise, the SDW and RDW were also higher in genotype MR44 by 6.29 and 8.39%, respectively over CML550 (Figure 3B).
Figure 3. Effect of genotype on (A) shoot and root fresh weight (B) root dry weight, shoot dry weight, and total plant biomass. Values presented as means ± standard error (n = 96). Means with different letters are significantly different from each other [Tukey's Studentized Range (HSD) test (p < 0.05)]. B0, un-inoculated control; B1, Pseudomonas sp. MRBP4; B2, Pseudomonas sp. MRBP13; B3, Bacillus sp. MRBP10; B1B2, Pseudomonas sp. MRBP4 + Pseudomonas sp. MRBP13; B1B3, Pseudomonas sp. MRBP4 + Bacillus sp. MRBP10; B2B3, Bacillus sp. MRBP10 + Pseudomonas sp. MRBP13; B1B2B3, Pseudomonas sp. MRBP4 + Pseudomonas sp. MRBP13 + Bacillus sp. MRBP10.
Bacterial inoculation had no significant effect on the number of leaves, number of roots and shoot length of inoculated plants. However, it had a significant effect (p ≤ 0.05) on the root length of inoculated plants (Figure 4A). Root length was significantly highest in maize genotypes co-inoculated with Pseudomonas sp. MRBP 4 + Pseudomonas sp. MRBP13 + Bacillus sp. MRBP10 (B1B2B3-which was 5.63% higher than the un-inoculated control. No significant difference was observed in the growth parameters in both genotypes (Figure 4B). Nonetheless, CML550 had higher root length (9.56 cm), number of roots (16.40), and shoot length (44.70 cm) compared to MR44 (9.54, 15.54, and 44.67 cm), respectively. Figure 5 shows the leaf area of inoculated plants which ranged from 150.69 ± 0.77 cm2 (B1B3- Pseudomonas sp. MRBP4 + Bacillus sp. MRBP10) to 212.45 ± 0.87 cm2 (B1B2- Pseudomonas sp. MRBP4 + Pseudomonas sp. MRBP13).
Figure 4. (A) Effect of bacterial inoculation on growth parameters of maize genotypes; (B) Effect of genotype on growth parameters. Values are presented as means ± standard error [(A) n = 24, (B) n = 96]. Means with different letters are significantly different from each other [Tukey's Studentized Range (HSD) test (p ≤ 0.05)]. B0, un-inoculated control; B1, Pseudomonas sp. MRBP4; B2, Pseudomonas sp. MRBP13; B3, Bacillus sp. MRBP10; B1B2, Pseudomonas sp. MRBP4 + Pseudomonas sp. MRBP13; B1B3, Pseudomonas sp. MRBP4 + Bacillus sp. MRBP10; B2B3, Bacillus sp. MRBP10 + Pseudomonas sp. MRBP13; B1B2B3, Pseudomonas sp. MRBP4 + Pseudomonas sp. MRBP13 + Bacillus sp. MRBP10.
Figure 5. Leaf area of inoculated maize plants. Values are presented as means ± standard error (n = 24). Means with different letters are significantly different from each other according to Duncan Multiple Range test (p ≤ 0.05).
The combined analysis of variance for both years (2018 and 2019) revealed that bacterial inoculation had a highly significant (p < 0.001) effect on relative water content (Figure 6A). Inoculation of maize seedlings with Bacillus sp. MRBP 10 (B3) and its co-inoculation with Pseudomonas sp. MRBP4 (B1B3) and Pseudomonas sp. MRBP13 (B1B2B3) had higher relative water contents than any of the other treatments. Relative water content increased under drought stressed condition (58.63%) compared to well-watered condition (49.96%). A similar trend was observed for the total soluble sugar (TSS) produced in maize plants inoculated with the rhizobacteria strains. Bacterial inoculation had a significant (p ≤ 0.05) effect on TSS production (Figure 6B). Bacillus sp. MRBP10 (B3) had the highest production of total soluble sugar which exceeded that produced by the un-inoculated control by 26.13% (Figure 6B). The TSS produced was not significantly different from the co-inoculated treatments B1B3 (Pseudomonas sp. MRBP 4 + Bacillus sp. MRBP10) and B1B2B3 (Pseudomonas sp. MRBP 4+ Pseudomonas sp. MRBP 13+ Bacillus sp. MRBP 10). Bacillus sp. MRBP 10 significantly increased the RWC and the TSS in inoculated plants under drought stress (Figures 6A, B).
Figure 6. Effect of bacterial inoculation on (A) relative water content, (B) total soluble sugar, and (C) soil moisture content of inoculated maize plants. Values are presented as means ± standard error (n = 24). Means with different letters are significantly different from each other according to Tukey's Studentized Range (HSD) test (p ≤ 0.05). B0, un-inoculated control; B1, Pseudomonas sp. MRBP4; B2, Pseudomonas sp. MRBP13; B3, Bacillus sp. MRBP10; B1B2, Pseudomonas sp. MRBP4 + Pseudomonas sp. MRBP13; B1B3, Pseudomonas sp. MRBP4 + Bacillus sp. MRBP10; B2B3, Bacillus sp. MRBP10 + Pseudomonas sp. MRBP13; B1B2B3, Pseudomonas sp. MRBP4 + Pseudomonas sp. MRBP13 + Bacillus sp. MRBP10.
Bacterial inoculation had a significant effect (p ≤ 0.01) on the soil moisture content (SMC) of inoculated maize plants (Figure 6C). SMC was significantly (p ≤ 0.05) highest in the soil of maize plants co-inoculated (B1B2B3) with Pseudomonas sp. MRBP4 + Pseudomonas sp. MRBP13 + Bacillus sp. MRBP10 (10.21%) which was higher than the un-inoculated control by 37.05%. Sole inoculation with Bacillus sp. MRBP10 (B3) also had high SMC of 10.13% (Figure 6C).
Combined analysis of variance for the 2018 and 2019 planting season revealed significant differences in the chlorophyll content of inoculated maize plants (Figure 7). Bacterial inoculation had a significant effect (p ≤ 0.05) on the carotenoid contents of the maize plants. Carotenoid content ranged from 0.97 ± 0.09 mg g −1 fresh weight (Pseudomonas sp. MRBP4 + Pseudomonas sp. MRBP13) to 1.33 ± 0.09 mg g −1 fresh weight (Pseudomonas sp. MRBP13). Carotenoid content increased by 18.75 and 15.19% more than the un-inoculated control in maize seedlings inoculated with Pseudomonas sp. MRBP13, and co-inoculated with Pseudomonas sp. MRBP13 + Bacillus sp. MRBP10, respectively. Likewise, co-inoculation with Pseudomonas sp. MRBP13 + Bacillus sp. MRBP10 produced significantly (p ≤ 0.01) higher chlorophyll a content in maize plants which was 25.54% higher than the un-inoculated control, but not significantly different from the chlorophyll a produced by maize plants inoculated with Pseudomonas sp. MRBP13 (15.30 ± 0.13 mg g−1 dry weight) or co-inoculated with the three strains; Pseudomonas sp. MRBP4 + Pseudomonas sp. MRBP13 + Bacillus sp. MRBP10 -B1B2B3 (14.59 ± 0.14 mg g−1 dry weight). Chlorophyll b content ranged from 4.05 ± 0.14 mg g−1 dry weight in plants co-inoculated with Pseudomonas sp. MRBP4 + Pseudomonas sp. MRBP13 to 5.66 ± 0.09 mg g−1 dry weight in maize plants inoculated with Pseudomonas sp. MRBP4. The total chlorophyll (Tchl) content significantly increased by 18.92% in maize plants inoculated with Pseudomonas sp. MRBP4 and by 17.57 and 18.62% in plants inoculated with Pseudomonas sp. MRBP13 and that co-inoculated with Pseudomonas sp. MRBP13 + Bacillus sp. MRBP10, respectively (Figure 7).
Figure 7. Effect of bacterial inoculation on chlorophyll content of maize plants. Values are presented as means ± standard error (n = 24). Means with different letters are significantly different from each other according to Tukey's Studentized Range test (p ≤ 0.05). B0, un-inoculated control; B1, Pseudomonas sp. MRBP4; B2, Pseudomonas sp. MRBP13; B3, Bacillus sp. MRBP10; B1B2, Pseudomonas sp. MRBP4 + Pseudomonas sp. MRBP13; B1B3, Pseudomonas sp. MRBP4 + Bacillus sp. MRBP10; B2B3, Bacillus sp. MRBP10 + Pseudomonas sp. MRBP13; B1B2B3, Pseudomonas sp. MRBP4 + Pseudomonas sp. MRBP13 + Bacillus sp. MRBP10.
Evaluation of the physiological response of inoculated maize seeds at 50% FC (mild drought stress) and 100% FC (well-watered) revealed significant differences in the production of TSS and the chlorophyll contents (Table 5). Under drought stress, TSS was significantly increased in treatments CML550 + B3 inoculated with Bacillus sp. MRBP10, and CML550 + B1B3 co-inoculated with Pseudomonas sp. MRBP4 and Bacillus sp. MRBP10, by 48.93 and 54.13% respectively, over the un-inoculated control. Treatment CML550 + B1B2 co-inoculated with Pseudomonas sp. MRBP4 and Pseudomonas sp. MRBP13 had the lowest TSS production (130.51 mg g−1 dw−1). Under well-watered conditions TSS was increased in treatments CML550 + B1B2B3 and CML550 + B3 by 47.72 and 45.65% respectively, over the un-inoculated control.
Table 5. Effect of treatments on total soluble sugar and chlorophyll content production under drought stress (50% FC) and well-watered (100% FC) conditions.
Carotenoid content increased by 156.25 and 155.20% in maize genotype CML550 inoculated with Bacillus sp. MRBP10 (B3) and co-inoculated with Pseudomonas sp. MRBP13 + Bacillus sp. MRBP10 (B2B3) respectively, over the un-inoculated control under drought stress (Table 5). As observed for the TSS production, treatment CML550 + B1B2 co-inoculated with Pseudomonas sp. MRBP4 and Pseudomonas sp. MRBP13 had the lowest carotenoid content (0.33 mg g−1 DW). Under well-watered condition, carotenoid content was significantly higher than other treatments, and 76.10% higher than the un-inoculated control in treatment CML550 + B2 sole inoculated with Pseudomonas sp. MRBP13. Likewise, treatment CML550 + B3 produced the highest chlorophyll a (19.26 mg g−1 DW) and total chlorophyll (24.97 mg g−1 DW) contents compared to the other treatments, and were significantly higher than the un-inoculated control plants by 24.74 and 25.60% respectively, under drought stress. Treatment CML550 + B2B3 also had increased levels of chlorophyll a and total chlorophyll by 20.47 and 20.12% respectively, over the un-inoculated control under drought stress (Table 5). Under well-watered conditions, treatment CML550 + B2 consistently gave the highest level of chlorophyll a, chlorophyll b, and total chlorophyll contents compared to the other treatments. Genotype CML550 was more responsive than genotype MR44.
Drought is a prominent abiotic stress that is affecting global food production leading to economic losses in crops especially cereals such as wheat, rice and maize. This study investigated the abilities of three rhizobacterial strains; Bacillus sp. MRBP10, Pseudomonas sp. MRBP4, and Pseudomonas sp. MRBP13 to alleviate the effects of drought stress on inoculated maize varieties MR44 and S0/8/W/I137TNW//CML550 as well as to enhance their growth. Understanding the architecture and functions of the activities of the rhizosphere, will aid the utilization of plant-microbe interactions in promoting plant growth, while mitigating the negativities of climate change on crop production. Due to the richness of the exudates released by plants, the rhizosphere forms a niche for abundant microbial activities. Utilization of beneficial microbes especially the rhizobacteria within the rhizosphere of plants possessing multifunctional growth promoting factors, and ability to tolerate abiotic stresses, is therefore a cheap and alternative means of augmenting plant growth under abiotic stresses. Previous researchers indicated that application of PGPR lessens drought stress in plants (Yasmin et al., 2017; Chandra et al., 2018; Raheem et al., 2018; Khan et al., 2019).
The rhizobacterial strains used in this study had the ability to produce plant growth promoting traits most importantly, indole-3-acetic acid, 1-aminocyclopropane-1-carboxylate deaminase, exopolysaccharide as well as the ability to solubilize phosphate in the form of tricalcium phosphate in vitro. ACC deaminase producing rhizobacteria possessing the ACC gene could lessen water stress in plants by reducing the levels of stress ethylene in plant tissues through decomposition to ammonia and α-ketobutyrate MRBP13 isolated from maize rhizosphere (Gupta and Pandey, 2019; Misra and Chauhan, 2020). This study revealed the potentials of Bacillus sp. MRBP10, Pseudomonas sp. MRBP4, and Pseudomonas sp. MRBP13 isolated from maize rhizosphere soil after the winter season, to promote plant growth and biomass of maize under drought stress and well-watered conditions. Our findings agrees with the study of Magnucka and Pietr (2015) and Chandra et al. (2018), who indicated that inoculation with PGPR possessing ACC deaminase activity significantly increased root elongation, coleoptile length, and shoot biomass of wheat (Magnucka and Pietr, 2015) and improved the growth performance of finger millet (Chandra et al., 2018), respectively. Pseudomonas sp. MRBP13 exhibited a higher level of ACC deaminase activity which may have reduced ethylene stress in inoculated plants and resulted in enhanced growth of the plants under drought stress. P. fluorescens strains with ACC deaminase ability isolated from arid regions exhibited drought tolerance at −0.30 MPa (15% PEG 6000) and also had other PGP abilities such as synthesis of IAA, siderophore, hydrogen cyanide, and the ability to solubilize phosphate (Ali et al., 2013). Increasing the root system caused by the reduction of ethylene, enhanced plant access to water, and nutrients from the depths of soil (Etesami and Maheshwari, 2018), which subsequently enabled the plants to survive drought stress conditions. The occurrence of ACC deaminase producing bacteria in the rhizosphere of plants enables them to resist desiccation of plant roots by affecting the ethylene signaling pathway. PGPR Achromobacter piechaudii with ACC deaminase activity enabled tomato and pepper plants to resist water stress and led to improvement in the biomass (Kumar et al., 2019).
Reports have shown that augmenting PGPR Pseudomonas sp. with L-tryptophan significantly enhanced the ability of the bacterium to produce higher concentrations of indole 3-acetic acid and gibberellic acid which alleviated water stress in the rhizosphere soil and leaves of inoculated maize plants (Yasmin et al., 2017). In our study, Pseudomonas sp. strain MRBP13 produced higher levels of IAA and in synergism with MRBP4, must have led to improved root architecture and extended root lengths which enabled the inoculated plants to endure water stress. In the study of Kaur et al. (2015), co-inoculation of Mesorhizobium sp. and Pseudomonas sp. strain PGPR3 with high IAA (43.18 and 66.79 μg mg−1) and phosphate solubilizing (4.40 and 13.34 mg 100 mL−1 abilities respectively, significantly improved the yield of two chickpea varieties desi and kabuli by 7 and 5.3%, respectively over sole inoculation with Mesorhizobium sp.
Indole-3-acetic acid and ACC deaminase also play a major role in relieving abiotic stress in plants by increasing the RWC of the leaves. In this study, co-inoculation of maize seedlings with Bacillus sp. MRBP10 and Pseudomonas sp. MRBP4 significantly enhanced RWC of the leaves of the maize plants by 10.67% and sole inoculation with Bacillus sp. MRBP10 by 8.76% over the un-inoculated control. The leaf RWC acts as an essential indicator of water status in plants as it gives the stability between water supply to the leaf tissue and transpiration rate (Soltys-Kalina et al., 2016). Azospirillum brasilense have also been reported to have enhanced RWC of maize seedlings when inoculated with the bacterium compared to un-inoculated plants (Kumar et al., 2019). Inoculation of drought stress tolerant bacteria augments the water potential of plants under water-deficit stress conditions. In a related study, inoculation of two varieties of Mustard, NPJ-124 (drought tolerant) and Pusa Karishma LES-39 (drought susceptible) with osmo-tolerant rhizobacteria Bacillus cereus strain NA D7 and Bacillus sp. strain MR D17 significantly enhanced plant physiological status, stabilized membranes and improved RWC of the inoculated plants under drought stress condition (Bandeppa et al., 2019). From all indications, inoculation of plants with a consortium of beneficial microbes with growth promoting abilities often gives better growth response than inoculation with individual strains due to their synergistic effect.
Lack of adequate water in the soil causes water-deficit stress which affects the growth of plants and also stresses the microbes found in the soil. This leads healthy soils to lose their basic functions. In such drought conditions, the microorganisms in the soil try to adjust their osmotic conditions in order to maintain their hydration by taking up solutes for retaining water in their cells (Shirinbayan et al., 2019; Ahmad et al., 2022). Combined inoculation of maize seeds with Pseudomonas sp. MRBP4, Pseudomonas sp. MRBP13, and Bacillus sp. MRBP10 (10.21%) as well as sole inoculation with Bacillus sp. MRBP10 enhanced plant growth under drought stress conditions by increasing the soil moisture content. This was made possible by the ability of the rhizobacterial strains to produce exopolysaccharides which keeps the soil hydrated by increasing its water holding capacity thereby protecting the bacteria and plant roots against desiccation (Ahmad et al., 2022). Thus, EPS production by these microbes may have augmented the ability of the soil to balance its water potential and maintain soil aggregation which enhanced nutrient uptake with the resultant growth of the maize plants and protection from dehydration (Subramaniam et al., 2020; Ahmad et al., 2022). In terms of mitigating abiotic stresses such as drought, EPS producing microbes are indispensable as they increase the water holding capacity of the soil, thereby relieving the plants from stress (Ojuederie et al., 2019).
Chlorophyll concentration is reduced under water-deficit stress conditions, which may be due to the fact that chlorophyll degradation exceeds chlorophyll synthesis (Zarei et al., 2020). Chlorophyll a concentration was generally higher than that of chlorophyll b and carotenoid under drought stress and well-watered conditions. This could be attributed to the fact that Chlorophyll a is the primary pigment while others pigments are mainly accessory pigment. Sole inoculation of maize with Pseudomonas sp. MRBP4, Pseudomonas sp. MRBP13, and co-inoculation with Pseudomonas sp. MRBP13 + Bacillus sp. MRBP10 significantly enhanced total chlorophyll contents in comparison with the un-inoculated control treatment. Singh N. B. et al. (2015), reported significant increase in total chlorophyll content in Sunflower plants under water stress when co-inoculated with Azotobacter chroococcum and Bacillus polymyxa, compared to sole inoculation and control treatments. Likewise Saikia et al. (2018) reported significant improvement in the leaf chlorophyll contents of chlorophylls a, b, and a + b in consortium-treated stressed plants in black gram plants by 106, 100, 120% respectively, and in garden pea plants by 283, 132, and 159% respectively, when compared with the un-inoculated stressed plants This indicates the efficacy of the these bacterial strains in the maintenance of chlorophyll content under water-stress conditions. Drought stress significantly reduced carotenoid content as well as that of chlorophyll a, chlorophyll b and total chlorophyll content in maize plants subjected to water-deficit stress in this study. Inoculation of seeds with both Pseudomonas strains MRBP4 and MRBP13 (B1B2) had the lowest production of Chlorophyll a, Chlorophyll b, carotenoid and total chlorophyll under drought stress. However, inoculation of the seeds with Bacillus sp. MRBP10 enhanced the concentration of carotenoid, Chlorophyll a, and total chlorophyll pigments in the maize plants. This was more pronounced in drought sensitive genotype CML550 sole inoculated with Bacillus sp. MRBP10 and that co-inoculated with Pseudomonas sp. MRBP13 and Bacillus sp. MRBP10 compared to genotype MR44.
The synergistic effect of the bacterial strains could be responsible for the increased root and shoot dry weights and total plant biomass obtained in the co-inoculated plants, compared to the control and other treatments. In a bid to reduce water loss, maize leaves get reduced in size and undergo leaf folding, reducing the transpiration rate in the leaves. Water stress substantially reduced leaf area in maize plants by 41.16% when compared to well-watered plants. Leaf area was significantly increased in maize plants co-inoculated with Pseudomonas sp. MRBP4 + Pseudomonas sp. MRBP13 by 23.12% compared to the un-inoculated control. Parallel to the microbial interaction during unfavorable conditions, plant systems also respond to drought stress by stimulating osmolyte production thereby sustaining the osmotic potential inside their cellular environment (Farooq et al., 2009; Sarma and Saikia, 2013). Under drought stress, plants produce osmolytes such as proline, total soluble sugars, total protein as well as glycine betaine in order to mitigate the effects of oxidative stress arising from reactive oxygen species (ROS). However, inoculation of plants with multifunctional PGP traits greatly enhances osmolyte production in plants. In terms of the total soluble sugar production, its concentration was significantly increased in inoculated plants compared to the control under drought stress. Nevertheless, sole inoculation with Bacillus sp. MRBP10 gave significantly higher TSS in the leaves of maize plants and then co-inoculation with Pseudomonas sp. MRBP 4 + Bacillus sp. MRBP10, and Pseudomonas sp. MRBP4+ Pseudomonas sp. MRBP 13+ Bacillus sp. MRBP 10, respectively. Inoculation of the sensitive maize genotype CML550 with Bacillus sp. MRBP10 significantly increased total soluble sugar under drought stress by 56.23%, and well-watered conditions by 45.65% compared to the un-inoculated controls. Higher accumulation of compatible osmolytes in plants inoculated with PGPR have been reported, which aided in sustaining cell membrane integrity and water status, and prevented protein degradation under stress (Gagné-Bourque et al., 2016; Zhou et al., 2016; Mohammadi et al., 2018; Bandeppa et al., 2019), by acting as molecular chaperons. Our findings revealed the relationship between bacterial inoculation and production of TSS in inoculated plants under drought stress. Such observations could be used as an indication of the drought stress mitigation ability of bacterial inoculants in tested host plants.
Thus, this study established the synergistic effect of Bacillus sp. MRBP10, Pseudomonas sp. MRBP4, and Pseudomonas sp. MRBP13 isolated from maize rhizosphere soil, in ameliorating the negative effects of water-deficit stress in maize plants. Seeds treated with PGPR significantly enhanced plant biomass, Tchl content and increased production of total soluble sugar, in addition to improving the growth parameters of maize. These strains in addition to tolerating abiotic stress also had the ability to produce significant amounts of plant growth promoting substances. Beneficial microbes should be protected by the use of technologies such as nano-encapsulation which would still maintain the efficacy in promoting plant growth under harsh environmental conditions.
Multifaceted PGPR have the ability to mitigate abiotic stresses in plants. In this study, Pseudomonas sp. MRBP4, Pseudomonas sp. MRBP13, and Bacillus sp. MRBP10 when sole or co-inoculated on seeds of two maize genotypes MR44 and S0/8/W/I137TNW//CML550, ameliorated the effect of drought stress. The presence of ACC deaminase activity and other growth promoting traits such as indole-3-aetic acid, phosphate solubilization, and exopolysaccharide production, enabled the rhizobacteria strains to mitigate the effect of induced drought stress on the maize plants by increasing the relative water content of the leaves of inoculated plants, and the soil moisture content when co-inoculated with Pseudomonas sp. MRBP4+Pseudomonas sp. MRBP13+Bacillus sp. MRBP10 (10.21%). Inoculation also improved the total chlorophyll content when sole inoculated (Pseudomonas sp. MRBP4 (20.30 ± 0.13 mg g−1 DW−1) or co-inoculated (Pseudomonas sp. MRBP13 + Bacillus sp. MRBP10 (20.25 ± 0.15 mg g−1 DW−1). The osmolyte total soluble sugar was significantly increased in seeds sole inoculated with Bacillus sp. MRBP10 (478.84 ± 1.22 mg g−1 DW−1). The presence of EPS in the three rhizobacteria strains improved the water holding capacity of the soil while ACC deaminase and indole-3-acetic acid subsequently alleviated water-deficit in maize genotypes by increasing the root lengths. Evaluation of the mitigating effects of the rhizobacterial strains on the field is needed to confirm their performances under natural conditions with the indigenous soil microorganisms.
The datasets presented in this study can be found in online repositories. The name of the repository and accession number(s) can be found at: https://www.ncbi.nlm.nih.gov/genbank/, MG953559, MG953564, and MG953568.
OO and OB: conceptualization, draft, and final report. OO: conduct of the experiment and data analysis. Both authors read and approved the final manuscript.
This work was supported by the North-West University Postdoctoral Fellowship awarded to OO. Research in the Microbial Biotechnology Laboratory of OB was supported by the National Research Foundation of South Africa (UID105248, UID123634, and UID132595). The funding body was not involved in the design of the study, sample collection, analysis, interpretation of data, and in writing the manuscript.
The authors declare that the research was conducted in the absence of any commercial or financial relationships that could be construed as a potential conflict of interest.
All claims expressed in this article are solely those of the authors and do not necessarily represent those of their affiliated organizations, or those of the publisher, the editors and the reviewers. Any product that may be evaluated in this article, or claim that may be made by its manufacturer, is not guaranteed or endorsed by the publisher.
The Supplementary Material for this article can be found online at: https://www.frontiersin.org/articles/10.3389/fsufs.2022.1076844/full#supplementary-material
Abiala, M., Odebode, A., Hsu, S., and Blackwood, C. (2015). Phytobeneficial properties of bacteria isolated from the rhizosphere of maize in southwestern Nigerian soils. Appl. Environ. Microbiol. 81, 4736–4743. doi: 10.1128/AEM.00570-15
Ahmad, H. M., Fiaz, S., Hafeez, S., Zahra, S., Shah, A. N., Gul, B., et al. (2022). Plant growth-promoting rhizobacteria eliminate the effect of drought stress in plants: A review. Front. Plant Sci. 13, 875774. doi: 10.3389/fpls.2022.875774
Ali, S. Z., Sandhya, V., and Rao, L. V. (2013). Isolation and characterization of drought-tolerant ACC deaminase and exopolysaccharide-producing fluorescent Pseudomonas sp. Annal. Microbiol. 64, 493–502. doi: 10.1007/s13213-013-0680-3
Arnon, D. I. (1949). Copper enzymes in isolated chloroplasts. Polyphenoloxidase in Beta vulgaris. Plant Physiol. 24, 1. doi: 10.1104/pp.24.1.1
Bandeppa, S., Paul, S., Thakur, J. K., Chandrashekar, N., Umesh, D. K., Aggarwal, C., et al. (2019). Antioxidant, physiological and biochemical responses of drought susceptible and drought tolerant mustard (Brassica juncea L) genotypes to rhizobacterial inoculation under water deficit stress. Plant Physiol. Biochem. 143, 19–28. doi: 10.1016/j.plaphy.2019.08.018
Bremner, J. (1960). Determination of nitrogen in soil by the Kjeldahl method. J. Agric. Sci. 55, 11–33. doi: 10.1017/S0021859600021572
Cairns, J. E., Hellin, J., Sonder, K., Araus, J. L., Macrobert, J. F., and Thierfelder, C. (2013). Adapting maize production to climate change in sub-Saharan Africa. Food Secur. 5, 345–360. doi: 10.1007/s12571-013-0256-x
Chandra, D., Srivastava, R., Glick, B. R., and Sharma, A. K. (2018). Drought-tolerant Pseudomonas spp. improve the growth performance of finger millet [Eleusine coracana (L.) Gaertn.] under non-stressed and drought-stressed conditions. Pedosphere 28, 227–240. doi: 10.1016/S1002-0160(18)60013-X
Cheesbrough, M. (2006). District Laboratory Practice in Tropical Countries. Cambridge: Cambridge University Press. doi: 10.1017/CBO9780511543470
Chibuike, G. U., and Obiora, S. C. (2014). Heavy metal polluted soils: Effect on plants and bioremediation methods. Appl. Environ. Soil Sci. 2014, 752708. doi: 10.1155/2014/752708
Chukwuneme, C. F., Babalola, O. O., Kutu, F. R., and Ojuederie, O. B. (2020). Characterization of actinomycetes strains for plant growth promoting traits and their effects on drought tolerance in maize. J. Plant Interact. 15, 93–105. doi: 10.1080/17429145.2020.1752833
Curá, J., Franz, D., Filosofía, J., Balestrasse, K., and Burgueño, L. J. M. (2017). Inoculation with Azospirillum sp. and Herbaspirillum sp. bacteria increases the tolerance of maize to drought stress. Microorganisms 5, 41. doi: 10.3390/microorganisms5030041
Dey, P. M. (1990). “Oligosaccharides,” in Methods in Plant Biochemistry, Vol. 2, Carbohydrates, ed P. M. (London: Academic Press), 189–218.
Dey, R., Pal, K., Bhatt, D., and Chauhan, S. (2004). Growth promotion and yield enhancement of peanut (Arachis hypogaea L.) by application of plant growth-promoting rhizobacteria. Microbiol. Res. 159, 371–394. doi: 10.1016/j.micres.2004.08.004
Dutta, J., Handique, P. J., and Thakur, D. (2015). Assessment of culturable tea rhizobacteria isolated from tea estates of Assam, India for growth promotion in commercial tea cultivars. Front. Microbiol. 6, 1252. doi: 10.3389/fmicb.2015.01252
Enebe, M. C., and Babalola, O. O. (2018). The influence of plant growth-promoting rhizobacteria in plant tolerance to abiotic stress: A survival strategy. Appl. Microbiol. Biotechnol. 102, 7821–7835. doi: 10.1007/s00253-018-9214-z
Etesami, H., and Maheshwari, D. K. (2018). Use of plant growth promoting rhizobacteria (PGPRs) with multiple plant growth promoting traits in stress agriculture: Action mechanisms and future prospects. Ecotoxicol. Environ. Saf. 156, 225–246. doi: 10.1016/j.ecoenv.2018.03.013
Farooq, M., Wahid, A., Kobayashi, N., Fujita, D., and Basra, S. (2009). “Plant drought stress: effects, mechanisms and management,” in Sustainable Agriculture (Dordrecht: Springer Netherlands), 153–188. doi: 10.1007/978-90-481-2666-8_12
Fukuda, K., Ogawa, M., Taniguchi, H., and Saito, M. (2016). Molecular approaches to studying microbial communities: Targeting the 16S ribosomal RNA gene. J. UOEH 38, 223–232. doi: 10.7888/juoeh.38.223
Gagné-Bourque, F., Bertrand, A., Claessens, A., Aliferis, K. A., and Jabaji, S. (2016). Alleviation of drought stress and metabolic changes in timothy (Phleum pratense L.) colonized with Bacillus subtilis B26. Front. Plant Sci. 7, 584. doi: 10.3389/fpls.2016.00584
Grover, M., Madhubala, R., Ali, S. Z., Yadav, S., and Venkateswarlu, B. (2014). Influence of Bacillus spp. strains on seedling growth and physiological parameters of sorghum under moisture stress conditions. J. Basic Microbiol. 54, 951–961. doi: 10.1002/jobm.201300250
Gujral, M. S., Agrawal, P., Khetmalas, M. B., and Pandey, R. (2013). Colonization and plant growth promotion of Sorghum seedlings by endorhizospheric Serratia sp. Acta Biol. Indica 2, 343–352.
Gupta, S., and Pandey, S. (2019). ACC deaminase producing bacteria with multifarious plant growth promoting traits alleviates salinity stress in French Bean (Phaseolus vulgaris) plants. Front. Microbiol. 10, 1506. doi: 10.3389/fmicb.2019.01506
Hall, T. A. (1999). BioEdit: A user-friendly biological sequence alignment editor and analysis program for windows 95/98/NT. Nucleic Acids Symposium Series. 41, 95–98.
Hiscox, J., and Israelstam, G. (1979). A method for the extraction of chlorophyll from leaf tissue without maceration. Canad. J. Bot. 57, 1332–1334. doi: 10.1139/b79-163
Igiehon, N. O., Babalola, O. O., and Aremu, B. R. J. B.M. (2019). Genomic insights into plant growth promoting rhizobia capable of enhancing soybean germination under drought stress. BMC Microbiol. 19, 159. doi: 10.1186/s12866-019-1536-1
Jacoby, R., Peukert, M., Succurro, A., Koprivova, A., and Kopriva, S. (2017). The role of soil microorganisms in plant mineral nutrition—current knowledge and future directions. Front. Plant Sci. 8, 1617. doi: 10.3389/fpls.2017.01617
Kaur, N., Sharma, P., and Sharma, S. (2015). Co-inoculation of Mesorhizobium sp. and plant growth promoting rhizobacteria Pseudomonas sp. as bio-enhancer and bio-fertilizer in chickpea (Cicer arietinum L.). Leg. Res. 38, 6780. doi: 10.18805/lr.v0iOF.6780
Kavamura, V. N., Santos, S. N., Da Silva, J. L., Parma, M. M., Ávila, L. A., Visconti, A., et al. (2013). Screening of Brazilian cacti rhizobacteria for plant growth promotion under drought. Microbiol. Res. 168, 183–191. doi: 10.1016/j.micres.2012.12.002
Khan, N., Bano, A., Rahman, M. A., Guo, J., Kang, Z., and Babar, M. A. (2019). Comparative physiological and metabolic analysis reveals a complex mechanism involved in drought tolerance in chickpea (Cicer arietinum L.) induced by PGPR and PGRs. Sci. Rep. 9, 2097. doi: 10.1038/s41598-019-38702-8
Kumar, A., Patel, J. S., Meena, V. S., and Srivastava, R. (2019). Recent advances of PGPR based approaches for stress tolerance in plants for sustainable agriculture. Biocatal. Agric. Biotechnol. 20, 101271. doi: 10.1016/j.bcab.2019.101271
Li, H., Guo, Q., Jing, Y., Liu, Z., Zheng, Z., Sun, Y., et al. (2019). Application of Streptomyces pactum Act12 enhances drought resistance in wheat. J. Plant Growth Regul. 39, 122–132. doi: 10.1007/s00344-019-09968-z
Li, J., Meng, B., Chai, H., Yang, X., Song, W., Li, S., et al. (2019). Arbuscular mycorrhizal fungi alleviate drought stress in C3 (Leymus chinensis) and C4 (Hemarthria altissima) grasses via altering antioxidant enzyme activities and photosynthesis. Front Plant Sci. 10, 499. doi: 10.3389/fpls.2019.00499
Li, Y., Liu, X., Hao, T., and Chen, S. (2017). Colonization and maize growth promotion induced by phosphate solubilizing bacterial strains. Int. J. Mol. Sci. 18, 1253. doi: 10.3390/ijms18071253
Magnucka, E. G., and Pietr, S. J. (2015). Various effects of fluorescent bacteria of the genus Pseudomonas containing ACC deaminase on wheat seedling growth. Microbiol. Res. 181:112–119. doi: 10.1016/j.micres.2015.04.005
Maxton, A., Singh, P., and Masih, S. A. (2018). ACC deaminase-producing bacteria mediated drought and salt tolerance in Capsicum annuum. J. Plant Nutri. 41, 574–583. doi: 10.1080/01904167.2017.1392574
Misra, S., and Chauhan, P. S. (2020). ACC deaminase-producing rhizosphere competent Bacillus spp. mitigate salt stress and promote Zea mays growth by modulating ethylene metabolism. 3 Biotech. 10, 119. doi: 10.1007/s13205-020-2104-y
Mohammadi, H., Esmailpour, M., Ghorbi, S., and Hatami, M. (2018). Physiological and biochemical changes in Matricaria chamomilla induced by Pseudomonas fluorescens and water deficit stress. Acta Agric. Slov. 111, 63–72. doi: 10.14720/aas.2018.111.1.07
Motsara, M., and Roy, R. N. (2008). Guide to Laboratory Establishment for Plant Nutrient Analysis. Rome: Food and Agriculture Organization of the United Nations Rome.
Naveed, M., Mitter, B., Reichenauer, T. G., Wieczorek, K., and Sessitsch, A. (2014). Increased drought stress resilience of maize through endophytic colonization by Burkholderia phytofirmans PsJN and Enterobacter sp. FD17. Environ. Exp. Bot. 97, 30–39. doi: 10.1016/j.envexpbot.2013.09.014
Niu, X., Song, L., Xiao, Y., and Ge, W. (2018). Drought-tolerant plant growth-promoting rhizobacteria associated with foxtail millet in a semi-arid agroecosystem and their potential in alleviating drought stress. Front. Microbiol. 8, 2580. doi: 10.3389/fmicb.2017.02580
Ojuederie, O. B., Olanrewaju, O. S., and Babalola, O. O. (2019). Plant growth promoting rhizobacterial mitigation of drought stress in crop plants: Implications for sustainable agriculture. Agronomy 9, 712. doi: 10.3390/agronomy9110712
Olanrewaju, O. S., Glick, B. R., and Babalola, O. O. (2017). Mechanisms of action of plant growth promoting bacteria. World J. Microbiol. Biotechnol. 33, 1–16. doi: 10.1007/s11274-017-2364-9
Olsen, S., and Sommers, L. (1982). “Phosphorus,” in Methods of Soil Analysis. Part 2, Chem. Microbiol. Prop, ed A. L. Page (New York, NY: John Wiley & Sons, Inc.), 403–430. doi: 10.2134/agronmonogr9.2.2ed.c24
Otieno, N., Lally, R. D., Kiwanuka, S., Lloyd, A., Ryan, D., Germaine, K. J., et al. (2015). Plant growth promotion induced by phosphate solubilizing endophytic Pseudomonas strains. Front. Microbiol. 6, 745. doi: 10.3389/fmicb.2015.00745
Paul, D., and Sinha, S. N. (2017). Isolation and characterization of phosphate solubilizing bacterium Pseudomonas aeruginosa KUPSB12 with antibacterial potential from river Ganga, India. Annal. Agrar. Sci. 15, 130–136. doi: 10.1016/j.aasci.2016.10.001
Paulo, E. M., Vasconcelos, M. P., Oliveira, I. S., Affe, H. M. D. J., Nascimento, R., Melo, I. S. D., et al. (2012). An alternative method for screening lactic acid bacteria for the production of exopolysaccharides with rapid confirmation. Food Sci. Technol. 32, 710–714. doi: 10.1590/S0101-20612012005000094
Posta, K., and Duc, N. H. (2019). “Benefits of arbuscular mycorrhizal fungi application to crop production under water scarcity,” in Drought - Detection and Solutions, ed G. Ondrasek (London: IntechOpen). doi: 10.5772/intechopen.86595
Premono, M. E., Moawad, A., and Vlek, P. (1996). Effect of phosphate-solubilizing Pseudomonas putida on the growth of maize and its survival in the rhizosphere. Indones. J. Crop Sci. 11, 13.
Raheem, A., Shaposhnikov, A., Belimov, A. A., Dodd, I. C., and Ali, B. (2018). Auxin production by rhizobacteria was associated with improved yield of wheat (Triticum aestivum L.) under drought stress. Arch. Agron. Soil Sci. 64, 574–587. doi: 10.1080/03650340.2017.1362105
Saikia, J., Sarma, R. K., Dhandia, R., Yadav, A., Bharali, R., Gupta, V. K., et al. (2018). Alleviation of drought stress in pulse crops with ACC deaminase producing rhizobacteria isolated from acidic soil of Northeast India. Sci. Rep. 8, 3560. doi: 10.1038/s41598-018-21921-w
Saitou, N., and Nei, M. (1987). The neighbor-joining method: A new method for reconstructing phylogenetic trees. Mol. Biol. Evol. 4, 406–425.
Saleem, A. R., Brunetti, C., Khalid, A., Della Rocca, G., Raio, A., Emiliani, G., et al. (2018). Drought response of Mucuna pruriens (L.) DC. inoculated with ACC deaminase and IAA producing rhizobacteria. PLoS ONE 13, e0191218. doi: 10.1371/journal.pone.0191218
Sandhya, V., Ali, S. Z., Grover, M., Reddy, G., and Venkateswarlu, B. (2010). Effect of plant growth promoting Pseudomonas spp. on compatible solutes, antioxidant status and plant growth of maize under drought stress. Plant Growth Regul. 62, 21–30. doi: 10.1007/s10725-010-9479-4
Sarma, R. K., and Saikia, R. (2013). Alleviation of drought stress in mungbean by strain Pseudomonas aeruginosa GGRJ21. Plant Soil 37, 111–126. doi: 10.1007/s11104-013-1981-9
Shirinbayan, S., Khosravi, H., and Malakouti, M. J. (2019). Alleviation of drought stress in maize (Zea mays) by inoculation with Azotobacter strains isolated from semi-arid regions. Appl. Soil Ecol. 133, 138–145. doi: 10.1016/j.apsoil.2018.09.015
Singh, N. B., Singh, D., and Singh, A. (2015). Biological seed priming mitigates the effects of water stress in sunflower seedlings. Physiol. Mol. Biol. Plants 21, 207–214. doi: 10.1007/s12298-015-0291-5
Soltys-Kalina, D., Plich, J., Strzelczyk-Zyta, D., Sliwka, J., and Marczewski, W. (2016). The effect of drought stress on the leaf relative water content and tuber yield of a half-sib family of “Katahdin”-derived potato cultivars. Breed. Sci. 66, 328–331. doi: 10.1270/jsbbs.66.328
Subbaiah, L. V., Prasad, T. N. V. K.V., Krishna, T. G., Sudhakar, P., Reddy, B. R., and Pradeep, T. (2016). Novel effects of nanoparticulate delivery of zinc on growth, productivity, and zinc biofortification in maize (Zea mays L.). J. Agric. Food Chem. 64, 3778–3788. doi: 10.1021/acs.jafc.6b00838
Subramaniam, G., Thakur, V., Saxena, R. K., Vadlamudi, S., Purohit, S., Kumar, V., et al. (2020). Complete genome sequence of sixteen plant growth promoting. Streptomyces strains. Sci. Rep. 10, 10294. doi: 10.1038/s41598-020-67153-9
Tamura, K., and Nei, M. (1993). Estimation of the number of nucleotide substitutions in the control region of mitochondrial DNA in humans and chimpanzees. Mol. Biol. Evol. 10, 512–526.
Tamura, K., Stecher, G., and Kumar, S. (2021). MEGA11: Molecular evolutionary genetics analysis version 11. Mol. Biol. Evol. 38, 3022–3027. doi: 10.1093/molbev/msab120
Timmusk, S., El-Daim, I. a. A., Copolovici, L., Tanilas, T., Kännaste, A., Behers, L., et al. (2014). Drought-tolerance of wheat improved by rhizosphere bacteria from harsh environments: Enhanced biomass production and reduced emissions of stress volatiles. PLoS ONE 9, e96086. doi: 10.1371/journal.pone.0096086
Toth, S., and Prince, A. (1949). Estimation of cation-exchange capacity and exchangeable Ca, K, and Na contents of soils by flame photometer techniques. Soil Sci. 67, 439–446. doi: 10.1097/00010694-194906000-00003
Vardharajula, S. (2014). Exopolysaccharide production by drought tolerant Bacillus spp. and effect on soil aggregation under drought stress. J. Microbiol. Biotechnol. Food Sci. 4, 51. doi: 10.15414/jmbfs.2014.4.1.51-57
Vardharajula, S., Zulfikar Ali, S., Grover, M., Reddy, G., and Bandi, V. (2011). Drought-tolerant plant growth promoting Bacillus spp.: Effect on growth, osmolytes, and antioxidant status of maize under drought stress. J. Plant Interact. 6, 1–14. doi: 10.1080/17429145.2010.535178
Walkley, A., and Black, I. A. (1934). An examination of the Degtjareff method for determining soil organic matter, and a proposed modification of the chromic acid titration method. Soil Sci. 37, 29–38. doi: 10.1097/00010694-193401000-00003
Wu, Q.-S., and Zou, Y.-N. (2017). “Arbuscular mycorrhizal fungi and tolerance of drought stress in plants,” in Arbuscular Mycorrhizas and Stress Tolerance of Plants (Berlin/?Heidelberg?: Springer), 25–41. doi: 10.1007/978-981-10-4115-0_2
Yasmin, H., Nosheen, A., Naz, R., Bano, A., and Keyani, R. (2017). l-tryptophan-assisted PGPR-mediated induction of drought tolerance in maize (Zea mays L.). J. Plant Interact. 12, 567–578. doi: 10.1080/17429145.2017.1402212
Yin, C., Casa Vargas, J. M., Schlatter, D. C., Hagerty, C. H., Hulbert, S. H., and Paulitz, T. C. (2021). Rhizosphere community selection reveals bacteria associated with reduced root disease. Microbiome 9, 86. doi: 10.1186/s40168-020-00997-5
Zarei, T., Moradi, A., Kazemeini, S. A., Akhgar, A., and Rahi, A. A. (2020). The role of ACC deaminase producing bacteria in improving sweet corn (Zea mays L. var saccharata) productivity under limited availability of irrigation water. Sci. Rep. 10, 20361. doi: 10.1038/s41598-020-77305-6
Zeng, Q., Wu, X., and Wen, X. (2016). Identification and characterization of the rhizosphere phosphate-solubilizing bacterium Pseudomonas frederiksbergensis JW-SD2, and its plant growth-promoting effects on poplar seedlings. Annal. Microbiol. 66, 1343–1354. doi: 10.1007/s13213-016-1220-8
Keywords: bioinoculation, chlorophyll content, PGPR, relative water content, water-deficit stress, Zea mays
Citation: Ojuederie OB and Babalola OO (2023) Growth enhancement and extenuation of drought stress in maize inoculated with multifaceted ACC deaminase producing rhizobacteria. Front. Sustain. Food Syst. 6:1076844. doi: 10.3389/fsufs.2022.1076844
Received: 22 October 2022; Accepted: 28 December 2022;
Published: 13 January 2023.
Edited by:
Mohamed Ait-El-Mokhtar, University of Hassan II Casablanca, MoroccoReviewed by:
Raja Ben-Laouane, Cadi Ayyad University, MoroccoCopyright © 2023 Ojuederie and Babalola. This is an open-access article distributed under the terms of the Creative Commons Attribution License (CC BY). The use, distribution or reproduction in other forums is permitted, provided the original author(s) and the copyright owner(s) are credited and that the original publication in this journal is cited, in accordance with accepted academic practice. No use, distribution or reproduction is permitted which does not comply with these terms.
*Correspondence: Olubukola Oluranti Babalola, b2x1YnVrb2xhLmJhYmFsb2xhQG53dS5hYy56YQ==
Disclaimer: All claims expressed in this article are solely those of the authors and do not necessarily represent those of their affiliated organizations, or those of the publisher, the editors and the reviewers. Any product that may be evaluated in this article or claim that may be made by its manufacturer is not guaranteed or endorsed by the publisher.
Research integrity at Frontiers
Learn more about the work of our research integrity team to safeguard the quality of each article we publish.