- 1Department of Biochemistry, Microbiology and Biotechnology, University of Namibia, Windhoek, Namibia
- 2Department of Natural and Applied Sciences, Namibia University of Science and Technology, Windhoek, Namibia
- 3International Centre for Genetic Engineering and Biotechnology, Trieste, Italy
The increase in dryland agriculture elicits the need to develop sustainable practices that improve crop yield and protect soil fertility. The use of biofertilisers adapted to nutrient deficient soils and arid climates would help achieve this. In this review, the use of plant growth-promoting bacteria is explored as a possible solution to the current state of dryland agriculture and climate change threats to agriculture. Plant microbe interactions form the basis of this review as evidence has shown that these interactions often exist to improve the health of plants. This is achieved by the production of important biochemicals and enzymes like indole acetic acid and amino cyclopropane-1-carboxylate deaminase while also actively protecting plants from pathogens including fungal pathogens. Research, therefore, has shown that these plant-growth promoting bacteria may be exploited and developed into biofertilisers. These biofertilisers are both economically and environmentally sustainable while improving soil quality and crop yield. The literature presented in this review is in context of the Namibian climate and soil profiles.
Introduction
The state of food security today is an important factor given the challenges being faced by the agriculture sector across the whole world. These challenges include climate change (Cowie et al., 2011), droughts (Ibrahim et al., 2015), human conflicts (Ezemenaka and Ekumaoko, 2018) and an increase in land degradation (Prăvălie et al., 2019). Africa is vulnerable to food insecurity as more than 50% of its land mass is considered dryland (Prăvălie, 2016). Drylands, therefore, refer to regions that receive low amounts of rainfall and have limited arable land such as the horn of Africa (Prăvălie et al., 2019) and central Asia (Vicente-Serrano et al., 2015). Such drylands are characterized by abiotic stress such as water and nutrient deficiency, high and low temperatures, high salinity, and UV radiation that have a significant impact on soil fertility and consequently impose an obvious limitation on crop production which in turn affect food security (Middleton and Sternberg, 2013). Therefore, there is a need to engage economic and environmentally sustainable skills, practices and knowledge systems to improve agricultural productivity in these regions, particularly in Africa (Chimwamurombe and Mataranyika, 2021).
The use of practical knowledge systems includes the expansion of the food base. This is important in regions that also incur the burden of malnutrition as a consequence of food insecurity (Chimwamurombe et al., 2020). Nutrient-dense drought tolerant crops would adequately mitigate these challenges of food security in dryland areas. Legumes offer a prime example of such crops that offer great benefits as nutritional alternatives. Some legumes of note are chickpeas (Cicer arietinum L.), soy beans (Glycine max), and marama bean [Tylosema esculentum (Burchell) Schreiber] (Caprioli et al., 2016; Bahroun et al., 2018; Cullis et al., 2018). Furthermore, research has observed improvement in biological soil quality after the cultivation of legumes making a strong argument for including them in crop rotations (Yu et al., 2014).
With these facts in mind, it is imperative to explore the plant microbe interactions that exist between legumes and the respective microbes. Studies have identified positive plant microbe interactions in arid climate-adapted legumes that make a compelling argument for further exploration and analysis (Bhattacharyya and Jha, 2012; Bahroun et al., 2018; Bakhtiyarifar et al., 2021). All the organisms occurring in these extreme environments, including bacteria, fungi and protozoa, develop intricate survival mechanisms to mitigate abiotic stresses (Khan, et al., 2020a). They possess the ability to express and regulate only those genes necessary to adequately adapt to the physical and chemical composition of these habitats (Martínez-Hidalgo and Hirsch, 2017). Hence, exploiting the plant-microbe interactions to sustainably meet agricultural demands in these regions is important (Verma et al., 2010; Lawless et al., 2018).
Some legumes have developed the ability to successfully grow in arid climates. These legumes offer ideal sources to isolate plant growth-promoting bacteria adapted to these climates (Dudeja et al., 2012). Common legumes grown in the arid parts of southern Africa include Tylosema esculentum (Chimwamurombe et al., 2016) and Glycine max (Igiehon and Babalola, 2018). Other legumes of note include Lablab purpureus, Vigna unguiculata, and Macrotyloma uniflorum (Bhardwaj et al., 2016; Grönemeyer et al., 2016; Pranesh and Ramesh, 2019). Bacterial species isolated from these legumes are also equally important due to their ability to fix nitrogen and promote growth in different stress situations. Species associated with this include Bradyrhizobium diazoefficiens, Rhizobium etli, Sinorhizobium spp. (Lawless et al., 2018) and Mesorhizobium spp. (Verma et al., 2010).
Namibia is a country located in the southwestern region of Africa (Ahmadalipour et al., 2019). Much of the country experiences a semi-arid to an arid climate. This is perpetuated by the low rainfall all year round and high evapotranspiration rates (Muhoko et al., 2020). Average rainfall ranges from <25 mm in the desert regions to 700 mm in the north-eastern regions (Montle and Teweldemedhin, 2014). Subsequently, groundwater becomes the largest source of water across the country making (Kalola et al., 2020). Namibia is also inclined to extreme climate change vulnerability (Montle and Teweldemedhin, 2014).
This review will explore known beneficial plant microbe associations in arid and nutrient poor conditions. It will focus on these interactions with Namibia in mind. It will explore interactions between legumes and microbes due to their known arid climate tolerance. Some legumes to be considered are moth bean [Vigna aconitifolia (Jacq.) Marechal], mung bean [Vigna radiata (L.) R. Wilczek var. radiata], and cow pea (Vigna unguiculata L. Walp). However, other plant microbe interactions will also be referenced.
Abiotic stress effects on plants
Various forms of stress affect agricultural production across the world. These may be abiotic or biotic stresses. Abiotic stresses are defined as pressures that arise from the environment. These include drought, extreme temperatures (which include freezing), abnormal salt levels and nutrient abnormalities (Suzuki et al., 2014; Enebe and Babalola, 2018). Abiotic stresses may also influence the extent to which biotic stresses affect plants. The effects may include oxidative damage to plant cells which increases susceptibility to pathogenic infections and pests. A combination of both types increases the potential threat to crop yield (Haggag et al., 2015; Pandey et al., 2017).
Drought tolerance is an important feature of plant growth-promoting bacteria (PGPB) as it offers a means to improve crop production during long periods of drought. Plant associated microbes help plants tolerate drought by enhancing the plants' physiological defenses against drought and producing different types of beneficial biochemicals such as auxins and enzymes (Ngumbi and Kloepper, 2016). PGPB can induce drought tolerance by reducing the accumulation of ethylene which impedes root elongation and eventual plant growth. This is done by the production of amino cyclopropane-1-carboxylate (ACC) deaminase, an enzyme able to catalyse the ethylene precursor ACC (Vurukonda et al., 2016; Delshadi et al., 2017). Bacteria in the genera Arthrobacter, Bacillus, and Microbacterium actively produce ACC deaminase in plants during water stress (Fadiji et al., 2021).
By producing essential amino acids and hormones, PGPB increase the plants' defenses in cases of drought stress. Arthrobacter and Bacillus PGPB, for example, contribute to proline production increasing plant growth (Kumari et al., 2016). Some Bacillus species, like B. megaterium and B. subtilis, produce cytokinins which are essential in drought stress tolerance (García-fraile et al., 2015). Drought tolerance may also be induced by PGP antioxidant activity. Associated endophytes increase the concentration of antioxidants such as flavonoids in plant cells.
Furthermore, evidence has shown that plant growth-promoting rhizobacteria (PGPR) help improve root systems in the event of drought stress by inducing root elongation and increasing surface area. This improves water uptake (Ngumbi and Kloepper, 2016). Alcaligenes faecalis, Burkholderia phytofirmans (Ngumbi and Kloepper, 2016), and Azospirillum brasilense (Vurukonda et al., 2016) strains are known to facilitate root elongation in drought stress conditions. This has been similarly observed in studies of Paenibacillus polymyxa SK1 isolated from Lilium lancifolium (Khan, et al., 2020b).
The morphological effects that droughts have on plants are the main causes of the reduced productivity of crops. These effects often present as reduced germination rate and seedling growth. Stunted plant growth is also often observed with decreased leaf, root and overall plant size (Hanaka et al., 2021). Plant-water potential is a parameter measured as a reflection of water energy in plants and is negatively affected by droughts. Drought stress reduces plant water potential which affects the transport of nutrients from the soil to the leaves. Plant fresh weight and biological processes such as photosynthesis which rely on water availability and nutrient transportation are also negatively affected by water stress (Ngumbi and Kloepper, 2016). Furthermore, drought stress negatively affects the biochemical processes that function to protect the plant. This results in protein and nucleic acid degradation, and weakening of membranes (Vurukonda et al., 2016).
Diversity and factors shaping rhizospheric and plant associated bacteria
Within plant tissues, microbes exist in symbiosis with the plant without causing damage to the plant. These microbes achieve this through roots, stems and/or seeds (Reinhold-Hurek and Hurek, 2011; Suman et al., 2016). Plant microbial associations include plant growth-promoting microbiome in the rhizosphere, pathogenic microbes and opportunistic human pathogens (Iyer and Rajkumar, 2017). These associations, when not pathogenic, offer positive support to the plant and soil. These plant growth-promoting microbes are known either as rhizobia or endophytes depending on whether they colonize the rhizosphere or the inner cells of the plant. Therefore, the successful colonization by the microbes contributes to the positive growth of the plant (Verma et al., 2010).
Seed endophytic bacteria influence
Diverse endophytic microbes colonize seeds forming some of the first bacterial associations in a plant's life cycle (López et al., 2018). These microbes include both bacteria and fungi (Nair and Padmavathy, 2014; Chimwamurombe et al., 2016). Seed endophytes have been observed to contribute to seed germination and cell elongation (Verma et al., 2017; Khalaf and Raizada, 2018). In addition, they form the initial microbial association for the promotion of the overall health of plants (Khalaf and Raizada, 2016). Seed endophytes can also remain quiescent in latent seeds. This means they only become active when germination begins. Furthermore, seed endophytes may be passed through to progeny with some changes occurring in the microbiome due to pathogenic infections, environmental changes or other stresses (López et al., 2018).
Seeds endophytic bacteria contribute positively to the general health of plants. Several species and genera have been identified as plant growth-promoting endophytic bacteria. Endophytic rice seedlings analysis revealed a diverse group of bacteria including Enterobacter asburiae, Pantoea dispersa and Pseudomonas putida. These were found to produce auxins, solubilize phosphates and inhibit pathogenic fungi (Verma et al., 2017). Through nitrogen fixation (Verma et al., 2017), hormone production (Chimwamurombe et al., 2016; Khalaf and Raizada, 2018) and antimicrobial activity (Nair and Padmavathy, 2014), endophytes improve abiotic stress tolerance and increase germination rates (Suman et al., 2016). Furthermore, they are also able to regulate hormone concentration thereby improving plant adaptation to environmental strains (Asaf et al., 2017).
Plant growth-promoting bacteria and nodulation
Root nodules are small structures typically found on legume roots. These nodules are small ranging between 2 and 5 mm containing up to 109 bacterial cells (Downie, 2014). Root nodule formation is triggered by simultaneous correlations between plants and their soil environment. The release of Nod factors into the soil by rhizobia temporarily activates plant genes that code for specific hormones (Spaink, 2000; Poehlman et al., 2019). Peptide hormones, for example, together with signal receptors and low levels of nitrogen in soil induce nodule formation with close association with nitrogen fixing bacteria (Taleski et al., 2018). However, nodule formation may be negatively affected by the absence of specific strains, low quorum and failure to colonize the rhizosphere (Prasanna et al., 2017). Though root nodules are mostly colonized by nitrogen fixing rhizobia, other microorganisms may also be found present in the nodules (Martínez-Hidalgo and Hirsch, 2017).
The formation of root nodules with the eventual colonization by bacteria is not fully understood however, it is known that nitrogen fixation is a result of this process. The process of nodulation is triggered by nitrogen levels in the soil with low levels initiating hormone signaling in the form of C-terminally encoded peptides (Verma et al., 2010; Taleski et al., 2018). Nod factors are produced by the bacteria as a response to signal molecules from the plant. These chemical signals include flavonoids which trigger the activation of Nod factor regulatory genes in bacteria (Spaink, 2000). This begins the process of infection with the rhizobial bacteria attached to root hairs. Once plant cell membranes detect the Nod factors, root hair deformation follows. A process that results in the nodule structure (Downie, 2014). Microbial interactions with roots tend to be location specific. Figure 1 below illustrates the specificity of different bacteria with the root system.
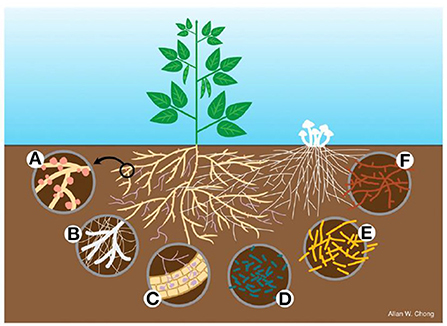
Figure 1. Root-nodule interactions with microbes. (A) Root nodules on plant roots. (B) Ectomycorrhizal associations with legume tree roots. (C) Arbuscular mycorrhizal interactions with root cells. (D) Gram negative rhizospheric bacteria that may influence nodule formation. (E) Gram positive bacteria colonize both the rhizosphere and the nodules. (F) Free living actinomycetes influence plant growth by nitrogen fixation among others. Adapted from Martínez-Hidalgo and Hirsch (2017).
Bacteria associated with root nodules include Mesorhizobium, Rhizobium and Sinorhizobium (Verma et al., 2010). In addition, species from the Bacillus, Bradyrhizobium and Leifsonia genera have been isolated from legume nodules in semiarid regions. Microbacterium endophytic isolates have also been isolated from root nodules (Nunes et al., 2018; Muresu et al., 2019). The symbioses have the advantage of promoting plant growth by increasing nitrogen uptake and assisting in disease tolerance and resistance. The bacteria may also solubilize phosphate or produce plant hormones which increase plant growth (Busby et al., 2017; Muresu et al., 2019). Plants consequently take advantage of the symbiotic relationship with bacteria present in the soil facilitating the formation of root nodules (Lawless et al., 2018).
Rhizospheric influence on plant growth promotion
The rhizosphere is described as the soil region closest to the roots. It acts as a platform for close interaction within the biosphere around the roots of plants (Jha and Saraf, 2015) and is largely influenced by the plant roots themselves (Ai et al., 2011; Semenov et al., 2020). Therefore, bacteria that colonize the rhizosphere are known as rhizobacteria (Haiyambo et al., 2015).
Through the action of root exudates and essentially chemotaxis (Figure 2) the rhizosphere is a microbe-rich zone (Orozco-Mosqueda et al., 2018; Swarnalakshmi et al., 2020). Also referred to as inter-kingdom signaling, chemotaxis forms the basis for the initial colonization of the rhizosphere by microbes (Venturi and Keel, 2016). As a result, it is a site for biological functions including microbial activity (Fernández Lópeza et al., 2013) and water regulation (Zhang et al., 2020). Both fungal and bacterial organisms form the population of microbes that occupy the rhizosphere (Bui and Franken, 2018; Liu et al., 2019; Leontidou et al., 2020; Sharma et al., 2020).
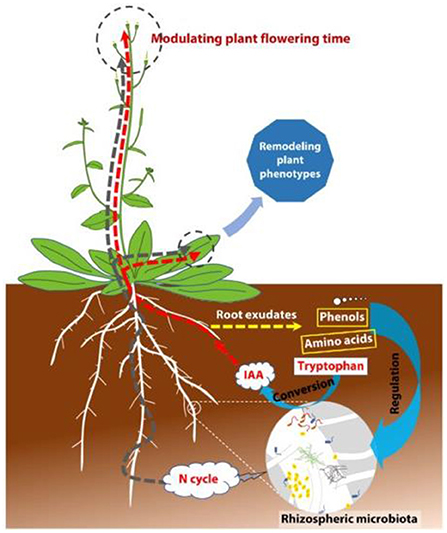
Figure 2. Rhizospheric interactions between the environment, microbes, and plants. Adapted from Lu et al. (2018).
Rhizobacteria possess the unique ability to influence plant systems both directly and indirectly (Enebe and Babalola, 2018). They offer positive support and influence the crops by performing or facilitating various biological processes. These include solubilisation of inorganic forms of essential compounds (Kaushal and Kaushal, 2015; Puri et al., 2020), biological nitrogen fixation (Tamagno et al., 2018) and antimicrobial activity (Qiu et al., 2012; Martínez-Hidalgo and Hirsch, 2017) among others. The microbial community of the rhizosphere, as such, is heavily influenced by microbes present in the general soil mass (Mendes et al., 2014).
The rhizosphere forms the primary stage for the exchange of nutrients and compounds between the plants and rhizobacteria. This is made possible by carbon rich root exudates that make the rhizosphere a nutrient rich region. This favors microbial growth (Orozco-Mosqueda et al., 2018; Semenov et al., 2020). The physical characteristics of the rhizosphere also create a suitable environment to accommodate both aerobic and anaerobic bacteria among others (Jha and Saraf, 2015; Chawngthu et al., 2020).
One important role played by the rhizosphere is the contribution it makes to water uptake from the bulk soil into plant roots. The uptake of water by plants from the bulk soil is a well understood process, however, the influence of the rhizosphere is often overlooked. Through an intricate interaction between the plant and rhizosphere, the water uptake is regulated (Carminati et al., 2010). This is initiated by plant roots that have been observed to produce a gel like substance (mucilage) that is held within the rhizosphere. Mucilage modifies rhizospheric soil properties resulting in improved water storage (Zeppenfeld et al., 2017; Zhang et al., 2020). Mucilage also has an additional function of inducing hydrophobicity in the event of reduced water availability. This allows for biophysical protection of the plant from drought (Kroener et al., 2016).
In addition, research strongly suggests that rhizospheric influence may differ depending on the age of the roots. This implies, therefore, that distal (younger) roots experience a greater mucilage occurrence to improve water uptake compared to proximal (older) roots (Carminati, 2013). Therefore, the hydraulic properties of the rhizosphere together with root exudates play a crucial regulatory role in water uptake by plants.
Root exudates are nutrient rich carbon sources ideal for microbial communities. They also offer a certain degree of influence on the microbiome (Semenov et al., 2020). Due to this influence and its physical properties, the rhizosphere creates an ideal environment for microbes. With this, the rhizosphere is able to house a wide variety of microbes whose composition is often influenced by plant roots (Essel et al., 2019). Distinct differences in microbiomes between the bulk soil and rhizosphere exist, however, the multiplicity decreases around the rhizosphere (Cui et al., 2019). In addition, the rhizospheric microbiome is more functionally structured compared to the bulk soil. This strongly points toward ecological stability within the rhizosphere (Zhang et al., 2017; Tian et al., 2022).
The rhizospheres of all plants are characterized by bacteria from several different genera. These include Bacillus, Enterobacter and Pseudomonas (Haiyambo et al., 2015). Some of the most abundant bacterial genera that have been identified within the rhizosphere are Lactococcus, Nocardioides, Pseudarthrobacter, Rhizobium and Streptomyces (Essel et al., 2019). The rhizosphere of legumes also includes a similar microbial profile. Rhizobacteria isolated from the chickpea rhizosphere include Azotobacter chroococcum, Bacillus pumilis, Bacillus subtilis and Pseudomonas aeruginosa (Pandey et al., 2019). Hence, other legumes like dolichos bean (L. purpureus) that have formed beneficial symbioses with bacteria become ideal candidates for sustainable intercropping practices.
Use of bioinoculants in crop improvement
Bioinoculants or biofertilisers are microorganisms developed for application to the surface of plants, seeds or mixed with the soil with eventual colonization of the rhizosphere or endosphere of the plants. They promote plant growth and improve nutrient use and uptake by the plant (Singh, 2013). The identification of PGPB and eventual growth-promoting traits has led to the use of bacteria strains as bioinoculants. These associations may be used in sustainable agriculture to substitute the use of chemical fertilizers.
Inoculation of soil or seeds with bioinoculants improves plant growth of plants. Root length, for example, may be influenced by inoculation of seeds with Azospirillum brasilence and Pseudomonas putida which are both known to encourage plant growth due to their ability to produce IAA (Shahab et al., 2009). Further evidence indicates plant growth improvement by the production of bioactive metabolites of PGPB isolated from the roots of Salvia miltiorrhiza. These contribute toward pathogen inhibition and improved disease tolerance and resistance (Duan et al., 2013). The use of bioinoculants has been assessed in Namibia on the growth of cowpea varieties. The study observed increases in yield of approximately 30% (Luchen et al., 2018).
The use of bioinoculants is further motivated by their environmental benefits. Unlike chemical fertilizers, biofertilisers do not leach into the soil and water nearby, a process known as eutrophication (Wimalawansa and Wimalawansa, 2015; Ouyang et al., 2018). However, this may be negatively affected by the chemical composition of the soil. Long-term exposure to fertilizers, for example, impacts the rhizospheric microbiome often reducing the diversity of PGP bacteria (Semenov et al., 2020).
Plant growth-promoting traits
1-aminocyclopropane-1-carboxylate (ACC) deaminase
Ethylene is a phytohormone with a regulatory role necessary for plant growth when in low concentrations. However, abiotic and biotic stresses trigger a different response (Ghosh et al., 2018). Stress events such as drought and higher temperatures induce the production of plant growth limiting compounds such as ethylene (Gupta and Pandey, 2019a). During drought stress, a frequent problem in arid and semi-arid regions, ethylene is produced as a stress signal. The increased water stress accelerates the oxidation of 1-aminocyclopropane-1-carboxylic acid from S-adenosyl methionine. A reaction that results in the production of ethylene (Danish and Zafar-Ul-Hye, 2020). An unregulated increase in “stress ethylene” results in the death of shoots and roots leading to the plant eventually failing to thrive (Singh et al., 2015). The presence of the enzyme ACC deaminase regulates the amount of ethylene in the plant. This is done by the hydrolysis of ACC to ammonium and α-ketobutyrate (Penrose and Glick, 2003). Studies have noted that ACC deaminase can effectively eliminate drought stress effects and this has been observed in pea crops (Ghosh et al., 2018).
ACC deaminase is also especially useful in increasing plant stress tolerance in events of high salinity and pathogenic infections (Bhattacharyya and Jha, 2012). Furthermore, the presence of ACC deaminase promotes nodule formation supporting plant growth. Some bacterial species produce ACC deaminase that actively breaks down ACC to ammonium and α-ketobutyrate (Belimov et al., 2001; Tsukanova, 2017). It has been noted, however, that ACC deaminase activity is higher in phosphorous deficient environments than in phosphorous abundance (Alemneh et al., 2020). Essentially ACC deaminase, by reducing the amount of ethylene, can influence an increase in root length (in the event of water stress) and improved nutrient uptake (in situations of nutrient deficiency) (Alemneh et al., 2020).
To determine the presence of ACC deaminase, bacterial isolates are tested for their ability to utilize ACC as the sole source of nitrogen (in the form of ammonium) (Penrose and Glick, 2003). This is achieved by inoculating the bacterial samples onto augmented Dworkin Foster minimal salt media with added ACC. Growth on these plates would indicate the presence of active ACC deaminase. An additional step measures the activity by determining the amount of α-ketobutyrate and ammonium produced (Ali et al., 2014). The process of the production of ammonia and α-ketobutyrate via ACC deaminase activity is shown in Figure 3 below. Molecular analysis of the isolates via 16S rRNA primers provides their identities. Some known bacteria species which are capable of hydrolyzing ACC include Pseudomonas putida strain Am2, P. brassicacearum strain Am3, Variovorax paradoxus strain Bm2, P. putida strain Bm3 (Belimov et al., 2001), P. fluorescens strain FPG3 (Ali et al., 2014), Paenibacillus sp. strain SG_AIOA2 and Aneurinibacillus aneurinilyticus (Gupta and Pandey, 2019a).
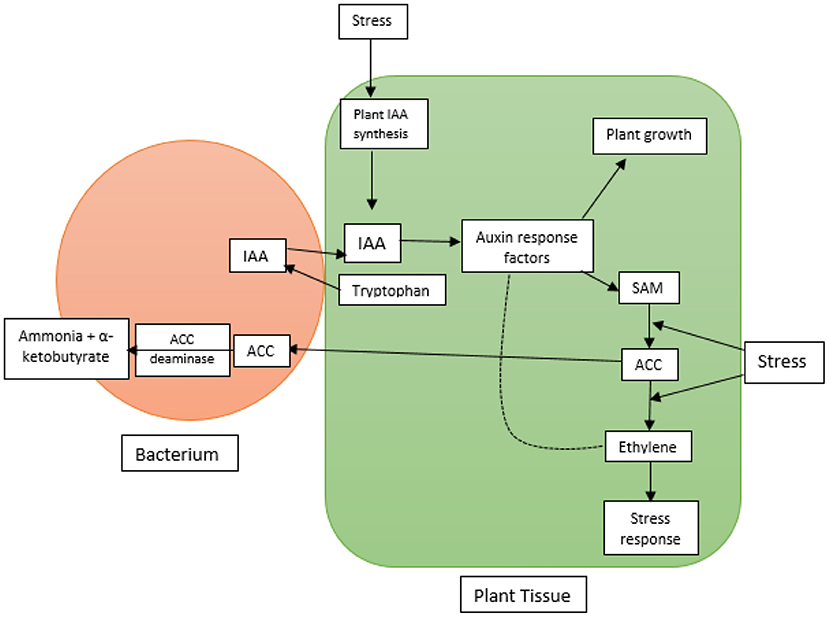
Figure 3. The image above [as described by Glick (2014)] shows the bacteria-assisted production of ammonia and α-ketobutyrate through the action of ACC deaminase as a response to stress on plants. Abbreviations: ACC - 1-aminocyclopropane-1-carboxylate; IAA - indole acetic acid; SAM - S-adenosyl methionine.
Phosphate solubilization
Minerals in insoluble forms cannot be taken up and utilized by plants, hence the need for chemical fertilizers. Phosphorous is one such mineral (Khandare et al., 2020). Phosphate solubilizing bacteria convert inorganic phosphate (Pi or ) into more soluble forms ( or H2PO4) that can be taken up and utilized by the plant. Bacteria achieve this by secreting acids that facilitate solubilization. Succinic acid is one such acid produced by several strains of Bacillus megaterium (Suleman et al., 2018; Zheng et al., 2018).
Phosphorous is an essential nutrient required for the growth and development of plants. It is a crucial element in DNA and RNA, adenosine triphosphate (ATP) and phospholipids (Daneshgar et al., 2018). Thereby positively contributing to photosynthesis, root elongation and nitrogen fixation (Matse et al., 2020). The availability of phosphorous to plants is crucial in soils with low concentrations of biologically available phosphates (Khandare et al., 2020). Furthermore, by using phosphate solubilizing PGPR in agriculture the use of environmentally damaging phosphate fertilizers is avoided. These phosphate fertilizers are known to leach heavy metals into water sources (Bhattacharyya and Jha, 2012).
To characterize bacteria for phosphate solubilization, isolates are grown on Pikovaskya's agar plates with 2% inorganic Tricalcium phosphate (Ca3(PO4)2 (Pandey et al., 2019) or a tris-minimal medium with added zinc phosphate (Shahab et al., 2009) and monitored. A molecular technique may also be employed in the identification and characterization of phosphate solubilising bacteria. This method entails the identification of phosphate solubilising genes in bacterial isolates. Using gene specific primers, genes may be identified (Zheng et al., 2018). This, however, is an inconclusive technique as it only indicates the ability of the bacteria to solubilise phosphates but does not reveal the level of expression of the genes.
Bacteria known to solubilize inorganic phosphate include P. fluorescens, P. putida, Xanthomonas maltophilia (Gupta et al., 2014), Enterobacter agglomerans and Rhizobium leguminosarum (Bhattacharyya and Jha, 2012). Some studies have identified bacterial strains in co-inoculation studies that improve phosphorus uptake. Improved phosphorous content was observed when Rhizobium spp strains (CHB1120 and CHB1121) were inoculated with Azotobacter vinelandii (strain G31) and Bacillus aryabhattai (strain Sb) (Matse et al., 2020).
Siderophore production
Iron is one of the most crucial elements for plant growth and is essential for plants to maintain ion homeostasis. It is also an essential component as plants are the main source of iron for humans. Iron deficiency in plants, therefore, is a serious problem (Rai et al., 2021). Some PGPR can produce siderophores that improve the uptake of iron by plants. These siderophores, by forming chelating complexes, promote plant growth by improving the availability of iron to plants and microbes. Siderophores are low molecular weight compounds released by organisms that have a high chelating affinity for ferric iron (Dudeja and Giri, 2014). These compounds solubilise ferric iron into more soluble forms (Fe3+ complexes) that are more easily taken up by plant cells (Gamit and Tank, 2014).
The functions of the siderophores promote plant health. As previously mentioned, nitrogen is an essential nutrient required by all plants. For nitrogen to be fixed, bacteria require the enzyme nitrogenase which contains iron. Therefore, sufficient amounts of iron are required (Singh et al., 2018). Iron is also an essential mineral required by plants for growth and development. Using iron-chelating siderophores, PGPR improve the uptake of iron in iron-deprived soils (Dastager et al., 2011). Siderophores also play a secondary role in biocontrol. By chelating ferric iron, they reduce the availability of free living iron in the soil which is required by phytopathogenic microbes (Bhattacharyya and Jha, 2012; Majeed et al., 2015). This has been observed in the control of pathogenic fungi by reducing the availability of iron (Penrose and Glick, 2003; Goswami et al., 2014).
Ligands that chelate iron (III) are used to classify and identify siderophores, these include carboxylates, catecholates and hydroxamates (Louden et al., 2011). Chrome azurol S (CAS) agar, with a pH indicator, is often used as a universal identifier for siderophore production tests. Isolates are inoculated onto CAS agar and observed for color change. The presence of a yellow halo around inoculated isolates indicates siderophore production (Schwyn and Neilands, 1987; Batista et al., 2017). Siderophore producing rhizobacteria in the genera Azadirachta, Azotobacter, Bacillus, Pseudomonas and Rhizobium contribute positively to plant growth and improvement of chlorophyll content (Gamit and Tank, 2014; Gupta et al., 2015). Pseudomonas sp. strain GRP3 from V. radiata supports iron uptake because of efficient siderophore production (Glick, 2012). Some siderophore producing species include Bradyrhizobium japonicum, Rhizobium leguminosarum and Sinorhizobium meliloti (Bhattacharyya and Jha, 2012).
Indole-3-acetic acid production
Indole-3- acetic acid (IAA), a growth-promoting auxin, stimulates root elongation and root hair growth. It is synthesized from tryptophan (Lu et al., 2018). However, previous studies have also identified bacteria that can produce IAA without the use of a tryptophan precursor (Kumari et al., 2016). It is an essential plant growth-promoting compound that offers positive support during drought stress, nutrient deficiency, and high salinity.
Extended periods without water (drought stress) mean the amount of water available to plants decreases continuously. However, IAA creates a metabolic reaction that improves water and nutrient uptake (Etesami, 2018). IAA stimulates root elongation and increases root hairs during drought stress. Furthermore, by increasing cell-water uptake efficiency and protein synthesis, IAA promotes embial activity. This in turn promotes increased nutrient uptake, (by longer roots) and induces flowering and fruiting (by delayed abscission) (Mohite, 2013).
The presence of IAA has also been attributed to increased salt tolerance by plants. By improving and maintaining the homeostasis of auxins and phytohormones, IAA supports salt tolerance. This is of importance as high salinity affects hormone production and balance (Saleem et al., 2021). Plants infected with IAA producing PGPB have been found to contain higher levels of antioxidant enzymes which increase salt tolerance (Viscardi et al., 2016). However, salt tolerance may also be enhanced with physical modifications induced by IAA. Khalid and Aftab (2020) observed salt tolerance samples with IAA. They attributed this tolerance to a possible increased salinity tolerance threshold made possible by the improved root length and cell extension.
IAA production may be assessed from bacterial isolates and quantified using different methods. Microbial analysis of IAA production often follows the growth of isolates in Luria-Bertani (LB) broth with tryptophan and incubated while shaking. Samples will thereafter be centrifuged and supernatant extracted for quantification using a spectrophotometer (Rajendran et al., 2012). Isolates can also be grown in yeast malt dextrose broth and quantification of IAA can be done using thin layer chromatography (Mohite, 2013). Some IAA producing genera include Azotobacter, Azospirillum, Bacillus, Kocuria, Pseudomonas, and Rhizobia (Bhattacharyya and Jha, 2012; Goswami et al., 2014).
Antifungal activity
Biotic stresses are major threats to crop production and yield and often results from fungal, bacterial, or viral infections. These infections cause great losses. Sub-Saharan Africa has recorded losses of more than 220, 000 tons due to fungal infections in common beans. The result of this on a global scale is approximately 800 million people being undernourished (Burke, 2010; Rajendran et al., 2012). Therefore, the antifungal activity of biofertilisers is an important characteristic.
One important fungal pathogen to legumes is Colletotrichum lindemuthianum. It affects L. purpureus (dolichos bean) and causes anthracnose disease which often results in yield loss. V. radiata (mung bean) is also susceptible to anthracnose infection with losses sometimes reaching up to 60% of planted crops (Bhutani et al., 2018). Other important fungal species are in the genus Fusarium. These include F. oxysporum and F. solani which are common pathogens that affect legumes (Burke, 2010; Eid and Fouda, 2021). Antifungal activity of plants by endophytic bacteria, therefore, is beneficial and contributes to plant growth-promoting activities (Haiyambo et al., 2015).
The antifungal activity of endophytic bacteria may be determined by molecular analysis or microbiological techniques. Molecular analysis of bacterial endophytes with primers allows for the detection of genes for antifungal compounds. Previous studies have identified the following genes phzC-phzD, prnD, pltc, phz, phlD and hcnAB to code for the production of antifungal compounds such as phenazine, phenazine-1-carboxylic acid and pyrrolnitrin (Bahroun et al., 2018). Metagenomics may also be used to detect antifungal clones in isolates, however, this method often results in low detection (Burke, 2010).
Antifungal compounds produced by endophytic bacteria actively inhibit the growth of pathogenic fungi. Microbial analysis of antifungal activity follows the concept of the inhibitory potential of isolates (Bhattacharyya and Jha, 2012). Isolates from V. radiata have been found to produce hydrogen cyanide which actively inhibits pathogenic fungi (Bhutani et al., 2018). To determine antifungal activity, fungal isolates are grown on potato dextrose agar (PDA) plates co-inoculated with bacterial isolates with antifungal abilities (Rajendran et al., 2012). Zones of inhibition indicate the degree of efficacy of antifungal compounds produced.
PGPB with antifungal activity can be isolated from different plants. An endophytic bacterium (Paenibacillus polymyxa SK1) isolated from bulbs of the Lilium lancifolium was found to possess significant antifungal activity. P. polymyxa SK1 was shown to actively inhibit Botrytis cinerea, Botryosphaeria dothidea, Fusarium fujikuroi and F. oxysporum, all detrimental fungal pathogens (Khan, et al., 2020b). Some Staphylococcus strains have been found to reduce drought stress but also inhibit fungal infections in plants (Eid and Fouda, 2021). Streptomyces murinus is a well-studied endophyte with antifungal activity. The most significant activity has been observed against Gibberella fujikuroi, Aspergillus niger and Aspergillus fumigatus all-important plant pathogens (Sun et al., 2013).
Nitrogen fixation
One of the most beneficial characteristics of plant growth is nitrogen fixation. Biological nitrogen fixation (BNF) is the process of supplying available nitrogen to the plant through microbial action. This is a trait that has been observed more often in legumes. L. purpureus and Cajanus cajan (pigeon pea) are examples of such legumes (Mendonça et al., 2017). This can be facilitated by bacteria (also referred to as diazotrophs) that fix atmospheric nitrogen (N2) to more biologically available ammonium form (). This reaction typically occurs in root nodules (Chidebe et al., 2018). This characteristic is especially crucial for plants growing in nitrogen poor soils. The chemical equation and Figure 4 below represent the process of nitrogen fixing. Studies have found that the enzyme nitrogenase catalyses the reaction below (Das and Microbial, 2018; Saiz et al., 2019).
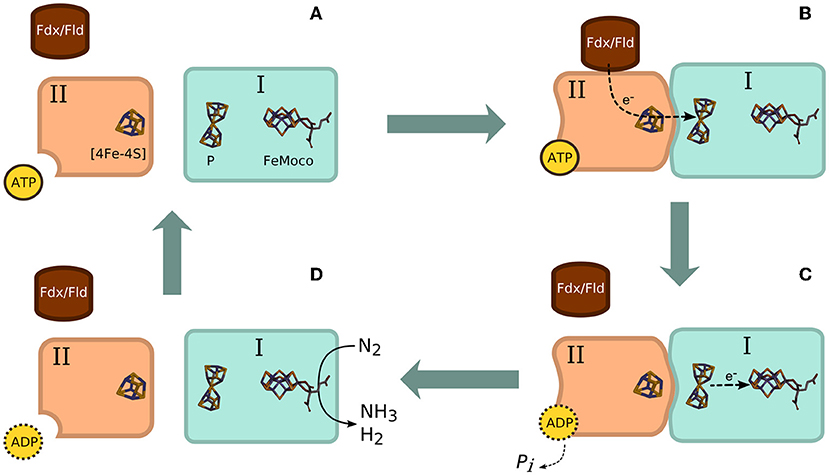
Figure 4. Schematic presentation of nitrogen fixation via nitrogenase facilitation. (A) Detached nitrogenase components I (dinitrogenase; MoFe protein) and II (dinitrogen reductase; Fe protein) show II awaiting reduction by ATP. (B) ATP binds to component II initiating electron transfer from donor [Fdx (ferredoxin) or Fld (flavodoxin)]. ATP binding triggers an allosteric structural change which leads to the components attaching. A flow of electrons occurs from the [4Fe-4S] cluster on II to the P cluster on I. (C) Electrons are further shuttled to the cofactor-iron-molybdenum cofactor (FeMoco) while ATP is hydrolysed to adenosine diphosphate (ADP). (D) The two components detach and produce ammonia and H2 via the reduction catalysed by nitrogenase (Seefeldt et al., 2009). Image by R Patrícia.
For nitrogen content, BNF plays a crucial role in improving soil fertility. In addition, it has been documented that close to 80% of all BNF occurrences are through symbiotic bacteria while non-symbiotic activity also contributes significantly (Gothwal et al., 2008; Das and Microbial, 2018). Non-symbiotic bacteria also referred to as free living nitrogen fixing (FLNF) bacteria can occur throughout the soil. However, they are often restricted to the rhizosphere due to the availability of carbon from the plant (Smercina et al., 2019).
The rate of nitrogen fixation is measured to determine the nitrogen fixing abilities of microbes. This is done in one of two ways, acetylene reduction assay (ARA) or the 15N2 incorporation method (Smercina et al., 2019). ARA is based on the reduction activity of nitrogenase enzyme on acetylene to ethylene (Saiz et al., 2019). To assess nitrogen fixing activity, isolates are grown on nitrogen free medium with an indicator. Isolates that show growth are thereafter inoculated into nitrogen free broth. This is followed by inoculation and growth in enriched cultures in vials allowing to produce ethylene. The ethylene produced is then measured by gas chromatography (Gothwal et al., 2008; Baldani et al., 2014).
However, ARA requires the use of a conversion factor to estimate the biological nitrogen fixation rate based on the number of moles of ethylene produced. The conversion factor is often approximately 4:1 (Saiz et al., 2019). The latter method, on the other hand, is more accurate as it measures nitrogen fixation based on the differences in 15N isotope abundance when exposed to 15N2 standard samples. However, this method carries a higher risk of contamination (Smercina et al., 2019). In addition to these two methods, a microbial bioassay may also be used. In this method, isolates are grown on a nitrogen free medium before growth on Jensen's medium plates under N2 atmosphere. Colony growth is then monitored and measured using a haemocytometer. A published equation is then used to calculate the rate of BNF (Das and Microbial, 2018).
There exists a catalog of nitrogen fixing bacteria that play an important role in plant growth promotion. Many of them have been isolated from legume species from roots, rhizosphere, and nodule endosphere. These include, among many others, Phaseolus vulgaris, V. angularis, V. subterranea, and L. purpureus (Andrews and Andrews, 2017). Within that list of bacteria are Bacillus pumilis and B. subtilis which have been isolated from the rhizosphere of cauliflower plants. Studies found strains from both species to positively influence plant growth (Kaushal and Kaushal, 2015). Rhizobium larrymoorei, Rhizobium oryzae and Rhizobium undicola are known to fix nitrogen in association with the legume Tylosema esculentum locally known as marama bean (Chimwamurombe et al., 2016). Other genera identified include Bradyrhizobium, Mesorhizobium, Ensifer and Azorhizobium (Wasai and Minamisawa, 2018). Table 1 below summarizes some of the most important species and genera for plant growth-promoting bacteria.
Concluding remarks
The semi-arid to arid climate of Namibia makes it vulnerable to the increasing threat of climate change affecting the world over. This further threatens subsistence farming which rural populations rely heavily on. Therefore, the development and use of plant growth-promoting bacteria as bioinoculants favors farmers, the population, and the environment. Research on plant microbial associations of arid-adapted crops like legumes would help facilitate more environmentally sustainable practices in agriculture with the Namibian climate and soil profiles in mind. We recommendation that work be put into developing plant growth-promoting bacteria associated with legumes that are currently grown in Namibia into bioinoculants for use in Namibia and other dryland regions across the globe. Furthermore, it is also recommended that subsistence farmers be included in developmental stages as crucial stakeholders of the developed bioinoculants.
Author contributions
PM: conceptualization and writing–original draft. PC, VV, and JU: writing–review and editing and supervision. All authors contributed to the article and approved the submitted version.
Acknowledgments
The work presented in this chapter forms part of a Ph.D. Thesis undertaken at the University of Namibia.
Conflict of interest
The authors declare that the research was conducted in the absence of any commercial or financial relationships that could be construed as a potential conflict of interest.
Publisher's note
All claims expressed in this article are solely those of the authors and do not necessarily represent those of their affiliated organizations, or those of the publisher, the editors and the reviewers. Any product that may be evaluated in this article, or claim that may be made by its manufacturer, is not guaranteed or endorsed by the publisher.
References
Ahmadalipour, A., Moradkhani, H., Castelletti, A., and Magliocca, N. (2019). Future drought risk in Africa: integrating vulnerability, climate change, and population growth. Sci Total Environ. 662, 672–86. doi: 10.1016/j.scitotenv.2019.01.278
Ai, C., Liang, G., Sun, J., Wang, X., and Zhou, W. (2011). Responses of extracellular enzyme activities and microbial community in both the rhizosphere and bulk soil to long-term fertilization practices in a fluvo-aquic soil. Geoderma. (2012) 173–174, 330–8. doi: 10.1016/j.geoderma.2011.07.020
Alemneh, A. A., Zhou, Y., Ryder, M. H., and Denton, M. D. (2020). Mechanisms in plant growth-promoting rhizobacteria that enhance legume–rhizobial symbioses. J. Appl. Microbiol. 129, 1133–1156. doi: 10.1111/jam.14754
Ali, S. Z., Sandhya, V., and Rao, L. V. (2014). Isolation and characterization of drought-tolerant ACC deaminase and exopolysaccharide-producing fluorescent Pseudomonas sp. Ann. Microbiol. 64, 493–502. doi: 10.1007/s13213-013-0680-3
Andrews, M., and Andrews, M. E. (2017). Specificity in legume-rhizobia symbioses. Int. J. Mol. Sci. 18 doi: 10.3390/ijms18040705
Asaf, S., Khan, M. A., Khan, A. L., Waqas, M., Shahzad, R., Kim, A. Y., et al. (2017). Bacterial endophytes from arid land plants regulate endogenous hormone content and promote growth in crop plants: an example of Sphingomonas sp. and Serratia marcescens. J. Plant Interact. 12, 31–38. doi: 10.1080/17429145.2016.1274060
Bahroun, A., Jousset, A., Mhamdi, R., Mrabet, M., and Mhadhbi, H. (2018). Anti-fungal activity of bacterial endophytes associated with legumes against Fusarium solani: Assessment of fungi soil suppressiveness and plant protection induction. Agric. Ecosyst. Enviro,. Appl. Soil Ecol. 124, 131–140. doi: 10.1016/j.apsoil.2017.10.025
Bakhtiyarifar, M., Enayatizamir, N., and Mehdi Khanlou, K. (2021). Biochemical and molecular investigation of non-rhizobial endophytic bacteria as potential biofertilisers. Arch Microbiol. 203, 513–21. doi: 10.1007/s00203-020-02038-z
Baldani, J. I., Reis, V. M., Videira, S. S., Boddey, L. H., and Baldani, V. L. D. (2014). The art of isolating nitrogen-fixing bacteria from non-leguminous plants using N-free semi-solid media: a practical guide for microbiologists. Plant Soil. 384, 413–431. doi: 10.1007/s11104-014-2186-6
Batista, B. D., Lacava, P. T., Ferrari, A., Teixeira-Silva, N. S., Bonatelli, M. L., Tsui, S., et al. (2017). Screening of tropically derived, multi-trait plant growth- promoting rhizobacteria and evaluation of corn and soybean colonization assay for siderophore detection ability. Microbiol Res. 206, 33–42. doi: 10.1016/j.micres.2017.09.007
Belimov, A. A., Safronova, V. I., Sergeyeva, T. A., Egorova, T. N., Matveyeva, V. A., Tsyganov, V. E., et al. (2001). Characterization of plant growth promoting rhizobacteria isolated from polluted soils and containing 1-aminocyclopropane-1-carboxylate deaminase. Can. J. Microbiol. 47, 642–652. doi: 10.1139/w01-062
Bhardwaj, J., Gangwar, I., Panzade, G., Shankar, R., and Yadav, S. K. (2016). Global de novo protein-protein interactome elucidates interactions of drought-responsive proteins in horse gram (macrotyloma uniflorum). J. Proteome Res. 15, 1794–1809. doi: 10.1021/acs.jproteome.5b01114
Bhattacharyya, P. N., and Jha, D. K. (2012). Plant growth-promoting rhizobacteria (PGPR): Emergence in agriculture. World J. Microbiol. Biotechnol. 28, 1327–1350. doi: 10.1007/s11274-011-0979-9
Bhutani, N., Maheshwari, R., and Suneja, P. (2018). Isolation and characterization of plant growth promoting endophytic bacteria isolated from Vigna radiata. Indian J. Anim. Res. 52, 596–603.
Bui, V. C., and Franken, P. (2018). Acclimatization of rhizophagus irregularis enhances zn tolerance of the fungus and the mycorrhizal plant partner. Front. Microbiol. 9, 1–13. doi: 10.3389/fmicb.2018.03156
Burke, C. (2010). A metagenomic analysis of the epiphytic bacterial community from the green macroalga Ulva australis. New South Wales: The University of New South Wales. doi: 10.1038/ismej.2010.164
Busby, P. E., Soman, C., Wagner, M. R., Friesen, M. L., Kremer, J., Bennett, A., et al. (2017). Research priorities for harnessing plant microbiomes in sustainable agriculture. PLoS Biol. 15, 1–14. doi: 10.1371/journal.pbio.2001793
Caprioli, G., Giusti, F., Ballini, R., Sagratini, G., Vila-Donat, P., Vittori, S., et al. (2016). Lipid nutritional value of legumes: evaluation of different extraction methods and determination of fatty acid composition. Food Chem. 192, 965–971. doi: 10.1016/j.foodchem.2015.07.102
Carminati, A. (2013). Rhizosphere wettability decreases with root age: a problem or a strategy to increase water uptake of young roots? Front. Plant Sci. 4, 1–9. doi: 10.3389/fpls.2013.00298
Carminati, A., Moradi, A. B., Vetterlein, D., Vontobel, P., Lehmann, E., Weller, U., et al. (2010). Dynamics of soil water content in the rhizosphere. Plant Soil. 332, 163–176. doi: 10.1007/s11104-010-0283-8
Chawngthu, L., Hnamte, R., and Lalfakzuala, R. (2020). Isolation and characterization of rhizospheric phosphate solubilizing bacteria from wetland paddy field of Mizoram, India. Geomicrobiol. J. 37, 366–75. Available from: doi: 10.1080/01490451.2019.1709108
Chidebe, I. N., Jaiswal, S. K., and Dakora, F. D. (2018). Distribution and phylogeny of microsymbionts associated with cowpea (Vigna unguiculata) nodulation in three agroecological regions of Mozambique. Appl. Environ. Microbiol. 84, 1–25. doi: 10.1128/AEM.01712-17
Chimwamurombe, P. M., Grönemeyer, J. L., and Reinhold-Hurek, B. (2016). Isolation and characterization of culturable seed-associated bacterial endophytes from gnotobiotically grown Marama bean seedlings. FEMS Microbiol. Ecol. 92, 1–11. doi: 10.1093/femsec/fiw083
Chimwamurombe, P. M., Luchen, C. C., and Mataranyika, P. N. (2020). Redefining global food security: do we really have a global food crisis? Agric. Food Sci. Res. 7, 105–112. doi: 10.20448/journal.512.2020.71.105.112
Chimwamurombe, P. M., and Mataranyika, P. N. (2021). Factors influencing dryland agricultural productivity. J. Arid Environ. 189, 104489. doi: 10.1016/j.jaridenv.2021.104489
Cowie, A. L., Penman, T. D., Gorissen, L., Winslow, M. D., Lehmann, J., Tyrrell, T. D., et al. (2011). Towards sustainable land management in the drylands: Scientific connections in monitoring and assessing dryland degradation, climate change and biodiversity. L. Degrad. Dev. 22, 248–260. doi: 10.1002/ldr.1086
Cui, Y., Bing, H., Fang, L., Wu, Y., Yu, J., Shen, G., et al. (2019). Diversity patterns of the rhizosphere and bulk soil microbial communities along an altitudinal gradient in an alpine ecosystem of the eastern Tibetan Plateau. Geoderma. 338, 118–27. doi: 10.1016/j.geoderma.2018.11.047
Cullis, C., Kunert, K., Vorster, J., Chimwamurombe, P., and Barker, N. (2018). Orphan legumes growing in dry environments: marama bean as a case study. Front. Plant Sci. 9, 1199. doi: 10.3389/fpls.2018.01199
Daneshgar, S., Callegari, A., Capodaglio, A. G., and Vaccari, D. (2018). The potential phosphorus crisis: resource conservation and possible escape technologies: a review. Resources. 7 doi: 10.3390/resources7020037
Danish, S., and Zafar-Ul-Hye, M. (2020). Combined role of ACC deaminase producing bacteria and biochar on cereals productivity under drought. Phyton (B Aires). 89, 217–227. doi: 10.32604/phyton.2020.08523
Das, S., and Microbial, D. (2018). Assay of N2 fixation rate, a simple alternate for acetylene reduction assay. MethodsX. 5, 909–914. doi: 10.1016/j.mex.2017.11.010
Dastager, S. G., Deepa, C. K., and Ashok, P. (2011). Potential plant growth-promoting activity of Serratia nematodiphila NII-0928 on black pepper (Piper nigrum L.). World J. Microbiol. Biotechnol. 27, 259–265. doi: 10.1007/s11274-010-0454-z
Delshadi, S., Ebrahimi, M., and Shirmohammadi, E. (2017). Effectiveness of plant growth promoting rhizobacteria on Bromus tomentellus Boiss seed germination, growth and nutrients uptake under drought stress. South African J. Bot. 113, 11–18. doi: 10.1016/j.sajb.2017.07.006
Duan, J. L., Li, X. J., Gao, J. M., Wang, D. S., Yan, Y., Xue, Q. H., et al. (2013). Isolation and identification of endophytic bacteria from root tissues of Salvia miltiorrhiza Bge. and determination of their bioactivities. Ann. Microbiol. 63, 1501–1512. doi: 10.1007/s13213-013-0614-0
Dudeja, S. S., and Giri, R. (2014). Beneficial properties, colonization, establishment and molecular diversity of endophytic bacteria in legumes and non legumes. African J. Microbiol Res. 8, 1562–1572. doi: 10.5897/AJMR2013.6541
Dudeja, S. S., Giri, R., Saini, R., Suneja-madan, P., and Kothe, E. (2012). Interaction of endophytic microbes with legumes. J. Basic Microbiol. 52, 248–260. doi: 10.1002/jobm.201100063
Eid, A. M., and Fouda, A. (2021). Abdel-rahman MA, Salem SS, Elsaied A, Oelmüller R, et al. Harnessing Bacterial Endophytes for Promotion of Plant Growth and Biotechnological Applications : An Overview. Plants. 10, 1–33. doi: 10.3390/plants10050935
Enebe, M. C., and Babalola, O. O. (2018). The influence of plant growth-promoting rhizobacteria in plant tolerance to abiotic stress: a survival strategy. Appl. Microbiol. Biotechnol. 102, 7821–7835. doi: 10.1007/s00253-018-9214-z
Essel, E., Xie, J., Deng, C., Peng, Z., Wang, J., Shen, J., et al. (2019). Bacterial and fungal diversity in rhizosphere and bulk soil under different long-term tillage and cereal/legume rotation. Soil Tillage Res. 194 doi: 10.1016/j.still.2019.104302
Etesami, H. (2018). Agriculture, ecosystems and environment can interaction between silicon and plant growth promoting rhizobacteria bene fi t in alleviating abiotic and biotic stresses in crop plants? Agric. Ecosyst Environ. 253:98–112. Available from: doi: 10.1016/j.agee.2017.11.007
Ezemenaka, K. E., and Ekumaoko, C. E. (2018). Contextualising Fulani- Herdsmen Conflict in Nigeria. Cent. Eur. J. Int. Secur. Stud. 12, 30–35.
Fadiji, A. E., Ayangbenro, A. S., and Babalola, O. O. (2021). Unveiling the putative functional genes present in root-associated endophytic microbiome from maize plant using the shotgun approach. J. Appl. Genet. 62, 339–351. doi: 10.1007/s13353-021-00611-w
Fernández Lópeza, M., Ramirez-Saad, H. C., Martínez-Abarca, F., Aguirre-Garrido, F. J., and Toro, N. (2013). Rhizosphere metagenomics. Encycl Metagenomics. (2017).
Gamit, D. A., and Tank, S. K. (2014). Effect of siderophore producing microorganism on plant growth of Cajanus cajan (Pigeon pea). Int. J. Res. Pure Appl. Microbiol. 4, 20–27.
García-fraile, P., Menéndez, E., and Rivas, R. (2015). Role of bacterial biofertilizers in agriculture and forestry. Bioengineering. 2, 183–205. doi: 10.3934/bioeng.2015.3.183
Ghosh, P. K., De, T. K., and Maiti, T. K. (2018). “Role of ACC deaminase as a stress ameliorating enzyme of plant growth-promoting rhizobacteria useful in stress agriculture: A review,” in Role of Rhizospheric Microbes in Soil: Stress Management and Agricultural Sustainability. 57–106. doi: 10.1007/978-981-10-8402-7_3
Glick, B. R. (2012). Plant growth-promoting bacteria: mechanisms and applications. Scientifica (Cairo). 2012, 1–15. doi: 10.6064/2012/963401
Glick, B. R. (2014). Bacteria with ACC deaminase can promote plant growth and help to feed the world. Microbiol Res. 169, 30–9. doi: 10.1016/j.micres.2013.09.009
Goswami, D., Pithwa, S., Dhandhukia, P., and Thakker, J. N. (2014). Delineating Kocuria turfanensis 2M4 as a credible PGPR: A novel IAA-producing bacteria isolated from saline desert. J. Plant Interact. 9, 566–76. doi: 10.1080/17429145.2013.871650
Gothwal, R. K., Nigam, V. K., Mohan, M. K., Sasmal, D., and Ghosh, P. (2008). Screening of nitrogen fixers from rhizospheric bacterial isolates associated with important desert plants. Appl Ecol Environ Res. 6, 101–109. doi: 10.15666/aeer/0602_101109
Grönemeyer, J. L., Hurek, T., Bünger, W., and Reinhold-Hurek, B. (2016). Bradyrhizobium vignae sp. nov., a nitrogen-fixing symbiont isolated from effective nodules of Vigna and Arachis. Int J Syst Evol Microbiol. 66, 62–69. doi: 10.1099/ijsem.0.000674
Gupta, G., Parihar, S. S., Ahirwar, N. K., Snehi, S. K., and Singh, V. (2015). Plant Growth Promoting Rhizobacteria (PGPR): Current and Future Prospects for Development of Sustainable Agriculture. J Microb Biochem Technol. 7, 96−102.
Gupta, G. N., Srivastava, S., Khare, S. K., and Prakash, V. (2014). Role of phosphate solubilizing bacteria in crop growth and disease management. J. Pure Appl. Microbiol. 8, 461–474.
Gupta, S., and Pandey, S. (2019b). Unravelling the biochemistry and genetics of ACC deaminase-An enzyme alleviating the biotic and abiotic stress in plants. Plant Gene. 18. doi: 10.1016/j.plgene.2019.100175
Gupta, S., and Pandey, S. A. C. C. (2019a). Deaminase producing bacteria with multifarious plant growth promoting traits alleviates salinity stress in French Bean (Phaseolus vulgaris) plants. Front. Microbiol. 10, 1–17. doi: 10.3389/fmicb.2019.01506
Haggag, W. M., Abouziena, H. F., Abd-El-Kreem, F., and El Habbasha, S. (2015). Agriculture biotechnology for management of multiple biotic and abiotic environmental stress in crops. J. Chem. Pharm. Res. 7, 882–889.
Haiyambo, D. H., Chimwamurombe, P. M., and Reinhold-Hurek, B. (2015). Isolation and screening of rhizosphere bacteria from grasses in east kavango region of namibia for plant growth promoting characteristics. Curr. Microbiol. 71, 566–571. doi: 10.1007/s00284-015-0886-7
Hanaka, A., Ozimek, E., Reszczyńska, E., Jaroszuk- Sciseł, J., and Stolarz, M. (2021). Plant tolerance to drought stress in the presence of supporting bacteria and fungi: an efficient strategy in horticulture. Horticulturae. 7, 1–21. doi: 10.3390/horticulturae7100390
Ibrahim, Y. Z., Balzter, H., Kaduk, J., and Tucker, C. J. (2015). Land degradation assessment using residual trend analysis of GIMMS NDVI3g, soil moisture and rainfall in Sub-Saharan West Africa from 1982 to 2012. Remote Sens. 7, 5471–5494. doi: 10.3390/rs70505471
Igiehon, N. O., and Babalola, O. O. (2018). Below-ground-above-ground plant-microbial interactions: focusing on soybean, rhizobacteria and mycorrhizal fungi. Open Microbiol. J. 12, 261–279. doi: 10.2174/1874285801812010261
Iyer, B., and Rajkumar, S. A. (2017). “Metagenomic approach to identify distinct rhizospheric and endophytic bacterial communities from roots and root nodules of vigna radiata,” in Understanding Host-Microbiome Interactions - An Omics Approach: Omics of Host-Microbiome Association, Singh, R. P., Kothari, V., Koringa, P. G., Singh, S. P. (eds). Springer Nature. p. 173–91. doi: 10.1007/978-981-10-5050-3_11
Jha, C. K., and Saraf, M. (2015). Plant growth promoting rhizobacteria (PGPR): a review. J Agric Res Dev. 5, 108–119.
Kalola, M. S., Kgabi, N. A., and Mukendwa, H. M. (2020). Geochemical assessment of groundwater in the Kuiseb River basin in Namibia. Phys Chem Earth. 102956. doi: 10.1016/j.pce.2020.102956
Kaushal, M., and Kaushal, R. (2015). Acetylene reductase activity and molecular characterization of plant growth promoting rhizobacteria to know efficacy in integrated nutrient management system. Indian J. Biotechnol. 14, 221–227.
Khalaf, E. M., and Raizada, M. N. (2016). Taxonomic and functional diversity of cultured seed associated microbes of the cucurbit family. BMC Microbiol. 16, 1–16. doi: 10.1186/s12866-016-0743-2
Khalaf, E. M., and Raizada, M. N. (2018). Bacterial seed endophytes of domesticated cucurbits antagonize fungal and oomycete pathogens including powdery mildew. Front. Microbiol. 9, 1–18. doi: 10.3389/fmicb.2018.00042
Khalid, A., and Aftab, F. (2020). Effect of exogenous application of IAA and GA3 on growth, protein content, and antioxidant enzymes of Solanum tuberosum L. grown in vitro under salt stress. Vitr. Cell Dev. Biol Plant. 56, 377–389. doi: 10.1007/s11627-019-10047-x
Khan, M. S., Gao, J., Chen, X., Zhang, M., Yang, F., Du, Y., et al. (2020b). Isolation and characterization of plant growth-promoting endophytic bacteria paenibacillus polymyxa SK1 from lilium lancifolium. Biomed. Res. Int. 2020. doi: 10.1155/2020/8650957
Khan, N., Ali, S., Tariq, H., Latif, S., Yasmin, H., Mehmood, A., et al. (2020a). Water conservation and plant survival strategies of rhizobacteria under drought stress. Agronomy. 10, 1–23. doi: 10.3390/agronomy10111683
Khandare, R. N., Chandra, R., Pareek, N., and Raverkar, K. P. (2020). Carrier-based and liquid bioinoculants of Azotobacter and PSB saved chemical fertilizers in wheat (Triticum aestivum L.) and enhanced soil biological properties in Mollisols. J. Plant Nutr. 43, 36–50. doi: 10.1080/01904167.2019.1659333
Kroener, E., Zarebanadkouki, M., Bittelli, M., and Carminati, A. (2016). Simulation of root water uptake under consideration of nonequilibrium dynamics in the rhizosphere. Water Resour. Res. 52, 5755–5770. doi: 10.1002/2015WR018579
Kumari, S., Vaishnav, A., Jain, S., Varma, A., and Choudhary, D. K. (2016). Induced drought tolerance through wild and mutant bacterial strain Pseudomonas simiae in mung bean (Vigna radiata L.). World J. Microbiol. Biotechnol. 32, 1–10. doi: 10.1007/s11274-015-1974-3
Lawless, C., Zedonek, M., Curtis, D., Scharringhausen, J., Bryant, J., Dustmn, W. A., et al. (2018). Identification and characterization of nitrogen fixing bacteria associated with kudzu root nodules. Georg J Sci Vol. 76, 2. Available online at: https://digitalcommons.gaacademy.org/gjs/vol76/iss2/7
Leontidou, K., Genitsaris, S., Papadopoulou, A., Kamou, N., Bosmali, I., Matsi, T., et al. (2020). Plant growth promoting rhizobacteria isolated from halophytes and drought-tolerant plants: genomic characterisation and exploration of phyto-beneficial traits. Sci. Rep. 10, 1–15. Available from: doi: 10.1038/s41598-020-71652-0
Liu, F., Ma, H., Peng, L., Du, Z., Ma, B., Liu, X., et al. (2019). Effect of the inoculation of plant growth-promoting rhizobacteria on the photosynthetic characteristics of Sambucus williamsii Hance container seedlings under drought stress. AMB Express. 9. Available from: doi: 10.1186/s13568-019-0899-x
López, S. M. Y., Pastorino, G. N., Franco, M. E. E., Medina, R., Lucentini, C. G., Saparrat, M. C. N., et al. (2018). Microbial endophytes that live within the seeds of two tomato hybrids cultivated in Argentina. Agronomy. 8 doi: 10.3390/agronomy8080136
Louden, B. C., Haarmann, D., and Lynne, A. M. (2011). Use of blue agar CAS. J. Microbiol. Biol. Educ. 12, 51–53. doi: 10.1128/jmbe.v12i1.249
Lu, T., Ke, M., Lavoie, M., Jin, Y., Fan, X., Zhang, Z., et al. (2018). Rhizosphere microorganisms can influence the timing of plant flowering. Microbiome. 6, 1–13. doi: 10.1186/s40168-018-0615-0
Luchen, C. C., Uzabikiriho, J.-D., Chimwamurombe, P. M., and Reinhold-Hurek, B. (2018). Evaluating the Yield Response to Bio-Inoculants of Vigna unguiculata in the Kavango Region in Namibia. J. Plant Pathol. Microbiol. 9 doi: 10.4172/2157-7471.1000456
Majeed, A., Kaleem Abbasi, M., Hameed, S., Imran, A., and Rahim, N. (2015). Isolation and characterization of plant growth-promoting rhizobacteria from wheat rhizosphere and their effect on plant growth promotion. Front. Microbiol. 6, 1–10. doi: 10.3389/fmicb.2015.00198
Martínez-Hidalgo, P., and Hirsch, A. M. (2017). The nodule microbiome: N2fixing rhizobia do not live alone. Phytobiomes J. 1, 70–82. doi: 10.1094/PBIOMES-12-16-0019-RVW
Matse, D. T., Huang, C. H., Huang, Y. M., and Yen, M. Y. (2020). Effects of coinoculation of Rhizobium with plant growth promoting rhizobacteria on the nitrogen fixation and nutrient uptake of Trifolium repens in low phosphorus soil. J. Plant Nutr. 43, 739–52. doi: 10.1080/01904167.2019.1702205
Mendes, L. W., Kuramae, E. E., Navarrete, A. A., Van Veen, J. A., and Tsai, S. M. (2014). Taxonomical and functional microbial community selection in soybean rhizosphere. ISME J. 8, 1577–1587. doi: 10.1038/ismej.2014.17
Mendonça, E. d. e,. S, De Lima, P. C., Guimarães, G. P., Moura, W. d. e,. M., and Andrade, F. V. (2017). Biological nitrogen fixation by legumes and N uptake by coffee plants. Rev Bras Cienc do Solo. 41, 1–10. doi: 10.1590/18069657rbcs20160178
Middleton, N. J., and Sternberg, T. (2013). Climate hazards in drylands: a review. Earth-Science Rev. 126, 48–57. doi: 10.1016/j.earscirev.2013.07.008
Mohite, B. (2013). Isolation and characterization of indole acetic acid (IAA) producing bacteria from rhizospheric soil and its effect on plant growth. J Soil Sci Plant Nutr. 13, 638–649. doi: 10.4067/S0718-95162013005000051
Montle, B. P., and Teweldemedhin, M. Y. (2014). Small-scale irrigation farming system situational analysis in namibia: case study from ndonga linena green scheme, Kavango West Region. Int. J. Agric. Innov. Res. 3, 2319–1473.
Muhoko, E., de Wassseige, C., and Cauwer, D. (2020). Assessing land cover change in Namibia's Kavango East region: a multi-date object approach. Bois Forets Des Trop. 344, 17–32. doi: 10.19182/bft2020.344.a31897
Muresu, R., Porceddu, A., Sulas, L., and Squartini, A. (2019). Nodule-associated microbiome diversity in wild populations of Sulla coronaria reveals clues on the relative importance of culturable rhizobial symbionts and co-infecting endophytes. Microbiol. Res. 221, 10–4. Available from: doi: 10.1016/j.micres.2019.01.004
Nair, D. N., and Padmavathy, S. (2014). Impact of endophytic microorganisms on plants, environment and humans. Sci. World J. 2014. doi: 10.1155/2014/250693
Ngumbi, E., and Kloepper, J. (2016). Bacterial-mediated drought tolerance : current and future prospects. Agric,. Ecosyst. Environ,. Appl. Soil Ecol. 105, 109–125. doi: 10.1016/j.apsoil.2016.04.009
Nunes, G. F., d. e, O., Menezes, K. A. S., Sampaio, A. A., Leite, J., Fernandes-Júnior, P. I., et al. (2018). Polyphasic characterization of forage legumes root nodule bacteria isolated from semiarid region in Brazil. Rev Ciências Agrárias. 41, 612–624. doi: 10.19084/RCA17339
Olanrewaju, O. S., Glick, B. R., and Babalola, O. O. (2017). Mechanisms of action of plant growth promoting bacteria. World J. Microbiol. Biotechnol. 33, 1–16. doi: 10.1007/s11274-017-2364-9
Oleńska, E., Małek, W., Wójcik, M., Swiecicka, I., Thijs, S., Vangronsveld, J., et al. (2020). Beneficial features of plant growth-promoting rhizobacteria for improving plant growth and health in challenging conditions: A methodical review. Sci. Total Environ. 743. doi: 10.1016/j.scitotenv.2020.140682
Orozco-Mosqueda, M., del C, Rocha-Granados M del, C., Glick, B. R., and Santoyo, G. (2018). Microbiome engineering to improve biocontrol and plant growth-promoting mechanisms. Microbiol. Res. 208, 25–31. doi: 10.1016/j.micres.2018.01.005
Ouyang, W., Wang, Y., Lin, C., He, M., Hao, F., Liu, H., et al. (2018). Heavy metal loss from agricultural watershed to aquatic system: a scientometrics review. Sci. Total Environ. 637–638.208–20. doi: 10.1016/j.scitotenv.2018.04.434
Pandey, P., Irulappan, V., Bagavathiannan, M. V., and Senthil-Kumar, M. (2017). Impact of combined abiotic and biotic stresses on plant growth and avenues for crop improvement by exploiting physio-morphological traits. Front. Plant Sci. 8, 1–15. doi: 10.3389/fpls.2017.00537
Pandey, S., Gupta, S., and Ramawat, N. (2019). Unravelling the potential of microbes isolated from rhizospheric soil of chickpea (Cicer arietinum) as plant growth promoter. 3, 9. doi: 10.1007/s13205-019-1809-2
Penrose, D. M., and Glick, B. R. (2003). Methods for isolating and characterizing ACC deaminase-containing plant growth-promoting rhizobacteria. Physiol. Plant. 118, 10–15. doi: 10.1034/j.1399-3054.2003.00086.x
Poehlman, W. L., Schnabel, E. L., Chavan, S. A., Frugoli, J. A., and Feltus, F. A. (2019). Identifying temporally regulated root nodulation biomarkers using time series gene co-Expression network analysis. Front. Plant Sci. 10, 1–13. doi: 10.3389/fpls.2019.01409
Pranesh and Ramesh, S.. (2019). Variability for grain protein content among germplasm accessions and advanced breeding lines in dolichos bean (Lablab purpureus L. Sweet var. Lignosus Prain). Plant Genet Resour Characterisation Util. 17, 289–92. doi: 10.1017/S1479262118000424
Prasanna, R., Ramakrishnan, B., Simranjit, K., Ranjan, K., Kanchan, A., Hossain, F., et al. (2017). Cyanobacterial and rhizobial inoculation modulates the plant physiological attributes and nodule microbial communities of chickpea. Arch. Microbiol. 199, 1311–1323. doi: 10.1007/s00203-017-1405-y
Prăvălie, R. (2016). Drylands extent and environmental issues. A global approach. Earth-Science Rev. 161, 259–278. doi: 10.1016/j.earscirev.2016.08.003
Prăvălie, R., Bandoc, G., Patriche, C., and Sternberg, T. (2019). Recent changes in global drylands: evidences from two major aridity databases. Catena. 178, 209–31. doi: 10.1016/j.catena.2019.03.016
Puri, A., Padda, K. P., and Chanway, C. P. (2020). In vitro and in vivo analyses of plant-growth-promoting potential of bacteria naturally associated with spruce trees growing on nutrient-poor soils. Appl. Soil Ecol. 149, 103538. doi: 10.1016/j.apsoil.2020.103538
Qiu, M., Zhang, R., Xue, C., Zhang, S., Li, S., Zhang, N., et al. (2012). Application of bio-organic fertilizer can control Fusarium wilt of cucumber plants by regulating microbial community of rhizosphere soil. Biol. Fertil. Soils. 48, 807–816. doi: 10.1007/s00374-012-0675-4
Rai, S., Singh, P. K., Mankotia, S., Swain, J., and Satbhai, S. B. (2021). Iron homeostasis in plants and its crosstalk with copper, zinc, and manganese. Plant Stress. 1. Available from: doi: 10.1016/j.stress.2021.100008
Rajendran, G., Patel, M. H., and Joshi, S. J. (2012). Isolation and characterization of nodule-associated Exiguobacterium sp. from the root nodules of fenugreek (Trigonella foenum-graecum) and their possible role in plant growth promotion. Int. J. Microbiol. 2012. doi: 10.1155/2012/693982
Reinhold-Hurek, B., and Hurek, T. (2011). Living inside plants: bacterial endophytes. Curr. Opin. Plant Biol. 14, 435–443. doi: 10.1016/j.pbi.2011.04.004
Saiz, E., Sgouridis, F., Drijfhout, F. P., and Ullah, S. (2019). Biological nitrogen fixation in peatlands: comparison between acetylene reduction assay and 15 N 2 assimilation methods. Soil Biol Biochem. 131, 157–65. doi: 10.1016/j.soilbio.2019.01.011
Saleem, S., Iqbal, A., Ahmed, F., and Ahmad, M. (2021). Saudi Journal of Biological Sciences Phytobeneficial and salt stress mitigating efficacy of IAA producing salt tolerant strains in Gossypium hirsutum. Saudi J. Biol. Sci. 28, 5317–24. doi: 10.1016/j.sjbs.2021.05.056
Schwyn, B., and Neilands, J. B. (1987). Universal chemical assay for the detection and determination of siderophores. Anal. Biochem. 160, 47–56. doi: 10.1016/0003-2697(87)90612-9
Seefeldt, L. C., Hoffman, B. M., and Dean, D. R. (2009). Mechanism of mo-dependent nitrogenase. Annual Rev. Biochem. 78. doi: 10.1146/annurev.biochem.78.070907.103812
Semenov, M. V., Krasnov, G. S., Semenov, V. M., and van Bruggen, A. H. C. (2020). Long-term fertilization rather than plant species shapes rhizosphere and bulk soil prokaryotic communities in agroecosystems. Appl. Soil Ecol. 154, 103641. doi: 10.1016/j.apsoil.2020.103641
Shahab, S., Ahmed, N., and Khan, N. S. (2009). Indole acetic acid production and enhanced plant growth promotion by indigenous PSBs. African J Agric Res. 4, 1312−1316.
Shahzad, R., Khan, A. L., Bilal, S., Asaf, S., and Lee, I. J. (2017). Plant growth-promoting endophytic bacteria versus pathogenic infections: An example of Bacillus amyloliquefaciens RWL-1 and Fusarium oxysporum f. sp. lycopersici in tomato. PeerJ. 2017, 1–21. doi: 10.7717/peerj.3107
Sharma, S., Compant, S., Ballhausen, M. B., Ruppel, S., and Franken, P. (2020). The interaction between Rhizoglomus irregulare and hyphae attached phosphate solubilizing bacteria increases plant biomass of Solanum lycopersicum. Microbiol. Res. 240, 126556. Available from: doi: 10.1016/j.micres.2020.126556
Singh, J. S. (2013). Plant growth promoting rhizobacteria. Reson J Sci Educ. 275–81. doi: 10.1007/s12045-013-0038-y
Singh, K., Gera, R., and Kumar, R. (2018). Isolation and characterization of siderophore producing rhizobia from Sesbania sesban using different types of Indian soils. Int. J. Chem. Stud. 6, 797–800. doi: 10.21746/aps.2017.6.10.7
Singh, R. P., Shelke, G. M., Kumar, A., and Jha, P. N. (2015). Biochemistry and genetics of ACC deaminase: a weapon to “stress ethylene” produced in plants. Front. Microbiol. 6, 1–14. doi: 10.3389/fmicb.2015.00937
Smercina, D. N., Evans, S. E., Friesen, M. L., and Tiemann, L. K. (2019). Optimization of the 15N2 incorporation and acetylene reduction methods for free-living nitrogen fixation. Plant Soil. 445, 595–611. doi: 10.1007/s11104-019-04307-3
Spaink, H. P. (2000). Root nodulation and infection factors produced by rhizobial bacteria. Annu. Rev. Microbiol. 54, 257–288. doi: 10.1146/annurev.micro.54.1.257
Suleman, M., Yasmin, S., Rasul, M., Yahya, M., Atta, B. M., Mirza, M. S., et al. (2018). Phosphate solubilizing bacteria with glucose dehydrogenase gene for phosphorus uptake and beneficial effects on wheat. PLoS ONE. 13, 1–28. doi: 10.1371/journal.pone.0204408
Suman, A., Yadav, A. N., and Verma, P. (2016). “Endophytic microbes in crops: diversity and beneficial impact for sustainable agriculture,” in Microbial Inoculants in Sustainable Agricultural Productivity: Research Perspectives, Singh, D. P., Singh, H. B., Prabha, R. (eds). New Delhi: Springer India. p. 117–43. doi: 10.1007/978-81-322-2647-5_7
Sun, H., He, Y., Xiao, Q., Ye, R., and Tian, Y. (2013). Isolation, characterization, and antimicrobial activity of endophytic bacteria from Polygonum cuspidatum. African J Microbiol Res Full. 7, 1496–1504. doi: 10.5897/AJMR12.899
Suzuki, N., Rivero, R. M., Shulaev, V., Blumwald, E., and Mittler, R. (2014). Abiotic and biotic stress combinations. New Phytol. 203, 32–43. doi: 10.1111/nph.12797
Swarnalakshmi, K., Yadav, V., Tyagi, D., Dhar, D. W., Kannepalli, A., Kumar, S., et al. (2020). Significance of plant growth promoting rhizobacteria in grain legumes: Growth promotion and crop production. Plants. 9, 1–25. doi: 10.3390/plants9111596
Taleski, M., Imin, N., and Djordjevic, M. A. C. E. P. (2018). Peptide hormones: key players in orchestrating nitrogen-demand signalling, root nodulation, and lateral root development. J. Exp. Bot. 69, 1829–1836. doi: 10.1093/jxb/ery037
Tamagno, S., Sadras, V. O., Haegele, J. W., Armstrong, P. R., and Ciampitti, I. A. (2018). Interplay between nitrogen fertilizer and biological nitrogen fixation in soybean: implications on seed yield and biomass allocation. Sci. Rep. 8, 1–11. doi: 10.1038/s41598-018-35672-1
Tian, G., Qiu, H., Li, D., Wang, Y., Zhen, B., Li, H., et al. (2022). Little environmental adaptation and high stability of bacterial communities in rhizosphere rather than bulk soils in rice fields. Appl. Soil Ecol. 169, 104183. doi: 10.1016/j.apsoil.2021.104183
Tsukanova, K. A. (2017). Chebotar VKm, Meyer JJM, Bibikova TN. Effect of plant growth-promoting Rhizobacteria on plant hormone homeostasis. South African J Bot. 113, 91–102. doi: 10.1016/j.sajb.2017.07.007
Venturi, V., and Keel, C. (2016). Signaling in the rhizosphere. Trends Plant Sci. 21, 187–98. doi: 10.1016/j.tplants.2016.01.005
Verma, J. P., Yadav, J., Tiwari, K. N., and Singh, V. (2010). Impact of plant growth promoting rhizobacteria on crop production. Int J Agric Res. 5, 954–983. doi: 10.3923/ijar.2010.954.983
Verma, S. K., Kingsley, K., Irizarry, I., Bergen, M., Kharwar, R. N., White, J. F., et al. (2017). Seed-vectored endophytic bacteria modulate development of rice seedlings. J. Appl. Microbiol. 122, 1680–1691. doi: 10.1111/jam.13463
Vicente-Serrano, S. M., Cabello, D., Tomás-Burguera, M., Martín-Hernández, N., Beguería, S., Azorin-Molina, C., et al. (2015). Drought variability and land degradation in semiarid regions: Assessment using remote sensing data and drought indices (1982-2011). Remote Sens. 7, 4391–4423. doi: 10.3390/rs70404391
Viscardi, S., Ventorino, V., Duran, P., Maggio, A., De Pascale, S., Mora, M. L., et al. (2016). Assessment of plant growth promoting activities and abiotic stress tolerance of Azotobacter chroococcum strains for a potential use in sustainable agriculture. J. Soil Sci. Plant Nutr. 16, 848–863. doi: 10.4067/S0718-95162016005000060
Vurukonda, S. S. K. P., Vardharajula, S., and Shrivastava, M. (2016). SkZ A. Enhancement of drought stress tolerance in crops by plant growth promoting rhizobacteria. Microbiol. Res. 184, 13–24. doi: 10.1016/j.micres.2015.12.003
Wasai, S., and Minamisawa, K. (2018). Plant-associated microbes: From rhizobia to plant microbiomes. Microbes Environ. 33, 1–3. doi: 10.1264/jsme2.ME3301rh
Wimalawansa, S. A., and Wimalawansa, S. J. (2015). Protection of watersheds, and control and responsible use of fertiliser to prevent phosphate eutrophication of reservoirs. Int J Res Environ Sci. 1, 1–18.
Yu, Y., Xue, L., and Yang, L. (2014). Winter legumes in rice crop rotations reduces nitrogen loss, and improves rice yield and soil nitrogen supply. Agron. Sustain. Dev. 34, 633–640. doi: 10.1007/s13593-013-0173-6
Zeppenfeld, T., Balkenhol, N., Kóvacs, K., and Carminati, A. (2017). Rhizosphere hydrophobicity: A positive trait in the competition for water. PLoS ONE. 12, 1–18. doi: 10.1371/journal.pone.0182188
Zhang, K., Adams, J. M., Shi, Y., Yang, T., Sun, R., He, D., et al. (2017). Environment and geographic distance differ in relative importance for determining fungal community of rhizosphere and bulk soil. Environ. Microbiol. 19, 3649–3659. doi: 10.1111/1462-2920.13865
Zhang, X. X., Whalley, P. A., Ashton, R. W., Evans, J., Hawkesford, M. J., Griffiths, S., et al. (2020). A comparison between water uptake and root length density in winter wheat: effects of root density and rhizosphere properties. Plant Soil. 451, 345–356. doi: 10.1007/s11104-020-04530-3
Keywords: arid adaption, bioinoculants, legumes, plant growth-promoting bacteria, sustainable agriculture
Citation: Mataranyika PN, Chimwamurombe PM, Venturi V and Uzabakiriho JD (2022) Bacterial bioinoculants adapted for sustainable plant health and soil fertility enhancement in Namibia. Front. Sustain. Food Syst. 6:1002797. doi: 10.3389/fsufs.2022.1002797
Received: 25 July 2022; Accepted: 23 August 2022;
Published: 09 September 2022.
Edited by:
Cosmas Parwada, Zimbabwe Open University, ZimbabweReviewed by:
Ayansina Segun Ayangbenro, North-West University, South AfricaBartholomew Saanu Adeleke, Olusegun Agagu University of Science and Technology, Nigeria
Copyright © 2022 Mataranyika, Chimwamurombe, Venturi and Uzabakiriho. This is an open-access article distributed under the terms of the Creative Commons Attribution License (CC BY). The use, distribution or reproduction in other forums is permitted, provided the original author(s) and the copyright owner(s) are credited and that the original publication in this journal is cited, in accordance with accepted academic practice. No use, distribution or reproduction is permitted which does not comply with these terms.
*Correspondence: Jean D. Uzabakiriho, anV6YWJha2lyaWhvJiN4MDAwNDA7dW5hbS5uYQ==
†These authors have contributed equally to this work and share senior authorship