- 1Food4Future (F4F), Leibniz Institute of Vegetable and Ornamental Crops (IGZ), Großbeeren, Germany
- 2Healthy Foods, Leibniz Institute for Agricultural Engineering and Bioeconomy (ATB), Großbeeren, Germany
- 3Quality and Safety of Food and Feed, Leibniz Institute for Agricultural Engineering and Bioeconomy (ATB), Potsdam, Germany
- 4Research Division Polymeric Materials and Composites PYCO, Fraunhofer-Institute for Applied Polymer Research IAP, Wildau, Germany
- 5Food Chemistry, Institute of Nutritional Science, University of Potsdam, Nuthetal, Germany
- 6Department of Agricultural and Food Sciences, University of Bologna, Cesena, Italy
- 7Department of Engineering and Natural Sciences, Technical University of Applied Sciences Wildau, Wildau, Germany
- 8Food Metabolome, Faculty of Life Science: Food, Nutrition and Health, University of Bayreuth, Kulmbach, Germany
Facing an inexorable growth of the human population along with substantial environmental changes, the assurance of food security is a major challenge of the present century. To ensure responsible food consumption and production (SDG 12), new approaches in the food system are required. Thus, environmentally controlled, sustainable production of alternative food sources are of key interest for both urban agriculture and food research. To face the current challenge of integrating food production systems within existing structures, multidisciplinary discourses are required. Here, we bring together novel technologies and indoor farming techniques with the aim of supporting the development of sustainable food production systems. For this purpose, we investigated the feasibility of 10 composite materials for their innovative use as structural support in macroalgal cultivation (settlement substrates) and cricket rearing (housing). Considering material resistance, rigidity, and direct material-organism interactions, the bio-based composite polylactic acid (PLA) was identified as a suitable material for joint farming. For macroalgae cultivation, PLA sustained the corrosive cultivation conditions and provided a suitable substrate without affecting the macroalgal physiology or nutritional composition (carotenoids and chlorophylls). For cricket rearing, PLA provided a suitable and recyclable shelter, which was quickly accepted by the animals without any observed harm. In contrast, other common composite components like phenolic resin or aramid were found to be unsuitable due to being harmful for the cultivated organisms or instable toward the applied sterilization procedure. This multidisciplinary study not only provides profound insights in the developing field of urban indoor food production from a new perspective, but also bridges material science and farming approaches to develop new sustainable and resilient food production systems.
Introduction
The human population is predicted to possibly reach about 9.7 billion people in 2050 (PRB Dataset, 2021). Thus, the issue of sustainable food security is more pressing than ever, as reflected in the Sustainable Development Goals (SDGs) (UN, 2015).
Closely connected with altered human behavior, environmental changes, such as the salinization of fertile soils, increasing loss of drinking water, and progressive pollution by industrially generated contaminants, provide a rising threat to global food security (Shukla et al., 2019). Consequently, alternative sustainable food production systems are urgently needed to mitigate these global changes (Henderson et al., 2019) and ensure responsible food consumption and production patterns (SDG 12).
Since meat and dairy consumption have been identified among the major drivers of climate change (Bailey et al., 2014), a transition toward a meat-reduced, healthy, and sustainably produced diet becomes inevitable (Shukla et al., 2019). Thus, alternative food sources, such as macroalgae and insects (e.g., crickets), are becoming of increasing interest to diversify people's diets (Overland et al., 2019; Tso et al., 2021).
The underexploited potential of macroalgae and insects for global nutrition has now been recognized (Tao and Li, 2018; Naylor et al., 2021). For example, the European algal cultivation sector is quickly developing (Araújo et al., 2021), supporting the global macroalgal market reaching about 33 · 106 tons (fresh weight) in 2016 (European Commission, 2019). In addition, the market for edible insects is also rapidly growing with a forecasted amount of 26 · 104 tons of insect-based food products alone on the European market in 2030 (IPIFF, 2020).
Considering macroalgae, the algal genera Ulva and Ectocarpus are regarded as valuable key organisms in macroalgal research: Ectocarpus is considered as a model organism for brown algae (Coelho et al., 2020), which play a key role in the global macroalgal food industry (Araújo et al., 2021). Moreover, the edible Ulva (also known as “sea lettuce” or “green laver”) (Kim et al., 2011) has been central in the development of innovative aquaculture (e.g., SeaWheat EU Cost Action CA20106). Similarly, insects in general and the house cricket (Acheta domesticus) in particular are increasingly used as a food source within several European countries (van Huis, 2013). Consequently, their risk profiles were investigated (SLU et al., 2018; Fernandez-Cassi et al., 2019; EFSA Panel on Nutrition et al., 2021) and A. domesticus has been recently accepted as novel food pursuant under Regulation (EU) 2015/2283 (EFSA Panel on Nutrition et al., 2021). In addition, also the extraction and recovery of dominant insect compounds, like chitin, has been targeted, due to its easy transformation to chitosan, a bioactive molecule with various industrial applications (Mohan et al., 2020; Psarianos et al., 2022).
Importantly, both organism groups, namely macroalgae (Ulva) and insects (crickets), provide valuable proteins (Rumpold and Schlüter, 2013; Juul et al., 2021) and a variety of different beneficial bioactive compounds like chlorophylls, carotenoids or essential fatty acids (e.g., Omega-3) (Ayieko and Orinda, 2020; Eismann et al., 2020; Leandro et al., 2020). In addition, due to the high anti-oxidative properties of these compounds, freshly harvested biomass is preferred for either direct consumption or subsequent processing, and this has led to increases in environmental controlled farming systems (Ventura et al., 2015; Jerney and Spilling, 2020). These systems foster the close or combined cultivation of different organisms—an approach that can save and/or recycle resources as well as provide additional multitrophic benefits (Pintado et al., 2017; Das et al., 2022).
The development of urban farming systems faces a wide range of different challenges, including consumer acceptance, optimization of nutrient flows, and the development of a cost-effective sustainable energy budget (Kennard and Bamford, 2020). But there have already been generated successful cases, like environmentally controlled vertical farms, contributing to urban food production by providing fresh and healthy food for the local communities with shorter supply chains (Chatterjee et al., 2020; Liu et al., 2022).
A crucial step on the way to a sustainable urban food production system lays in the construction of a viable and flexible cultivation unit, that is able to support the requirements of the different organism and is also integrable in existing structures such as roof terraces, basement rooms, and other unused rooms.
Overall, there are two main requirements for the needed structural materials. First, a high resistance to a very corrosive environment containing salt water, microorganisms, and insects. Second, a low weight, so the load capacity of floors and ceilings in pre-existing buildings is not exceeded. In this context, composite materials are promising since they are known for their higher strength and light weight compared to solid materials (Griffith, 1995). Composite materials are composed of different pliable fibers (e.g., made of glass, carbon, and various polymers), which are often embedded in a reinforcing and protecting polymetric matrix (e.g., epoxy and phenolic resin). This composition provides a variety of advantages such as low weight, corrosion resistance, high fatigue strength, and faster assembly (Jose and Joseph, 2012). Thus, composites are commonly used as construction materials for extreme environments and can be found in the aviation and automotive industry. Next to the classical petroleum-based polymers, alternative bio-based and biodegradable polymers like cellulose and polylactic acids (PLA) can be used for composites. Given the rising environmental issues of petrol use and microplastic pollution, these innovative bio-based and biodegradable materials respond to the pressing need for a sustainable alternative. Of interest is that PLA has been declared as GRAS (Generally Recognized as Safe) by the American Food and Drug Administration (Prendiz et al., 2019), and thus, there is a high application potential for these composites in the food industry. However, not all bio-based and biodegradable materials meet the properties needed to be used in cultivation units.
The choice of composite material is crucial for a successful farming. The materials need to provide chemical and physical stability, while not negatively interfering with the physiology of the respective organism (e.g., being harmful or toxic) and even supporting their cultivation (e.g., by providing suitable structures for attachment or shelter). In a multidisciplinary approach we here experimentally investigated 10 different composite materials regarding their suitability for macroalgae and insect urban farming. To this end, we addressed the following two research questions:
1) Are the composite materials resistant to the corrosive (e.g., saline, humid) cultivation environments of the alternative crops and livestocks?
2) Do the composite materials not negatively affect the physiology of the cultivated organisms and allow successful food production?
Testing the resistance of materials toward common sanitation procedures and bringing them to direct contact with the cultivated organisms, we investigated potential material stability, toxicity, as well as acceptance as settlement (macroalgae) and shelter (crickets) substrates for the cultivated organisms. The findings show that bio-based composites are suitable materials for the farming of the studied macroalgae and crickets. To our knowledge, this is the first study testing novel bio-based composite lightweight materials for habitat formation of seaweeds and insects. Given the rising interest and need of combined environmentally controlled cultivation systems for food production and other industrial purposes (e.g., pharmacy, cosmetics), the present findings support new developments in urban agriculture and will help to strengthen food security in the future.
Materials and methods
Materials
Ten different composite materials were considered and tested for their potential in macroalgae cultivation and cricket rearing (materials developed by InnoMat GmbH, Germany). The materials were chosen because of their macrostructure, known as nap core. This three-dimensionally shaped material provides an enlarged surface, which is critical for the cultivation of algae spores, and can also create many cavities that can serve as hiding places for small insects. Five of the materials (M1, M2, M5, M6, M7) were composites based on various matrices, namely phenolic resin, epoxy resin, and wood glue, in which different types of textiles, cellulose, glass, as well as para- or met-aramid were embedded. The remaining five consisted of different thermoplastic textiles, made of a combination of polyester, polyamide, elastane, thermoplastic special yarns, or PLA. Given the requirements for sustainability, the bio-based materials M2 (regenerated cellulose) and M3 (PLA) were of main interest. An overview of the materials is presented in Table 1.
Material durability and compatibility
To determine the suitability of the 10 different materials for the application in closed co-cultivation systems for macroalgae and insects, material durability and compatibility investigations were carried out in a three-step approach, which tested both material resistance to operating conditions and potential material-organism interactions. The three-step testing phases were (i) sterilization by autoclaving the raw materials, (ii) testing for algae compatibility under exposure to marine (3.5% salinity) long-term conditions, and (iii) testing for insect compatibility under short-term exposure to adult crickets under tropic conditions (31°C, 70% relative humidity). To investigate potential structural deformations and superficial changes, subsamples of materials were analyzed microscopically prior to and post experimental treatments using a scanning electron microscope (Phenom electron microscope, Phenom-World BV, Netherlands). For preparation, samples were air dried, glued on the sample holder, and directly analyzed at 250–350 times magnification. Since the surface area of the materials directly depends on their fiber diameter, these values were also examined during microscopy.
Sterilization: Autoclave resistance
Using pressurized steam to destroy microorganisms, autoclaves provide a common and widespread sterilization method applied for surfaces, media, reagents, or waste, and thus, are often applied to avoid contamination or for decontamination. In this respect, autoclave resistance is an essential property for materials used for sustainable farming systems, and thus, this was considered as a main exclusion criterion in the present work. The 10 materials (Table 1) were exposed to high pressure and temperature using an autoclave (Systec VX-75, Systec GmbH, Germany, for 20 min at 121°C and 2.1 bar). Based on the outcome, only dimensionally stable materials were considered for further investigations.
Macroalgal assay: Experimental organisms, material exposure, and physiological investigation
The green algal genus Ulva is considered as a model organism for the developing innovative aquaculture (e.g., SeaWheat EU Cost Action CA20106) and the foliose growing Ulva fenestrata (Ulvaceae, Ulvales; formerly considered as Ulva lactuca) is already commercially farmed for food production in European waters (Steinhagen et al., 2021). In addition, the brown macroalgae Ectocarpus sp. (Ectocarpaceae, Ectocarpales) is widely cultivated in many laboratories (Coelho et al., 2020). Both algal cultivars (U. fenestrata and Ectocarpus sp.) were isolated from field material collected in the intertidal area of the North Sea island Helgoland, Germany, in November 2019. The algal material was cultivated under artificial conditions at 16°C water temperature and an 8 h photoperiod with 35 μmol m2 s−1 PPFD in artificial seawater (Tropic Marine, 3.5% salinity, Supplementary Table 1).
Two different macroalgal assays were conducted: (i) a material seeding approach to test the feasibility of different materials as substrates for macroalgae cultivation and (ii) a material exposure approach to investigate the potential impact of material chemistry on algal physiology and nutritional composition.
Material seeding approach
In the material seeding approach, different materials (M1–M4) were seeded with spores of U. fenestrata and thalli of Ectocarpus sp. for U. fenestrata freshly released spores. These spores, derived from field material were collected via pipette and 1 ml of the solution was transferred to each material unit. For Ectocarpus sp., developing sporophyte basal patches (<1 mm) showing a high potential for attachment and biofouling (Bringloe et al., 2020) were selected and individually transplanted on the materials via forceps. Material tests were conducted in 24-well plates, where each well served as an experimental unit filled with cultivation media (Tropic Marine, 3.5% salinity, Supplementary Table 1), selected material, and corresponding macroalgae (n = 4). Wells with autoclaved oyster shill and empty wells served as procedural controls. To evaluate cultivation times of a 1-month period, commonly applied to evaluate macroalgae growth cycles (Carl et al., 2014; Sebok et al., 2020; Schmitz and Kraft, 2022), as well as longer periods needed for environmental controlled recirculating cultivation systems, experiments were conducted over 28 days and up to 632 days. To support the algal physiology, available water was fertilized with enriched sealife medium (ESL) at an early stage and the commercial green algal medium Algal (Aqualgae, Portugal) at later stages (>200 days) provided at a concentration of 1.25 ml L−1 served as the medium to support algal growth (Supplementary Table 1). Experimental units were investigated under dissection microscope (Axioscope, Zeiss, Germany) at different times (data shown in Table 2).
Six different two-level parameters (P1–P6) were considered to evaluate the physiological stage and potential interaction of macroalgae with the different materials: P1 = vitality (1-cells pigmented, 0-not pigmented) and P2 = germling presence: (1-visible, 0-not visible) were both determined directly. To evaluate P3 = thalli growth, relative growth was calculated for Ectocarpus sp. by size comparison with the control unit (1-grown, 0-not grown), whereas for U. fenestrata, lengths of randomly selected germlings (n = 10 per replicate) were determined from photographs using ImageJ software and the average thallus lengths were calculated for 31, 76, and 83 days exposure. In addition, daily growth rates were calculated for the last week (76–83 days) of the experiment. To test P4 = attachment (1-organism attached on material, 0-not attached), thalli were carefully moved using forceps. The investigation of P5 = thallus morphology (1-upright, 0-creeping/crustose) and P6 = fertility (1-presence of sporangia, 0-immature), both only done for Ectocarpus sp., required additional disruptive analyses at higher magnifications and were conducted at the end of experiment. Further investigated parameters and growth conditions of the experiments are provided in Table 2.
Material exposure approach
In the material exposure approach, the potential impact of the most suitable material, identified during the material seeding approach, was tested on the nutritional composition of the cultivated U. fenestrata. For this purpose, germlings (about 2 mm in length) of U. fenestrata were exposed to material samples at 18°C under a 10 h photoperiod with LED illumination of 75 μmol m−2 s−1 PPFD at 2.9 % salinity (Tropic Marine). To additionally study the potential impact of material r, an important parameter for construction purposes, three different level of rigidity were selected for the exposed PLA material (soft-PLA1, flexible-PLA2, firm-PLA3) (n = 4). Empty wells served as the procedural control, resulting in 16 wells filled with cultivation media, corresponding material samples, and germlings. After a period of 260 days, the pre-conditioned thalli were weighted and transferred to opaque plastic dishes equipped with the corresponding materials (cut stripes of 2 × 7 cm) and 50 ml cultivation media for a period of 74 days. To support the algal growth over this time, the thalli were fertilized with Algal (Aqualgae, Portugal) three times per week. At the end of experiments, thalli weights and specific growth rates (SGRs) were calculated over the final 74 days, expressed as % increase per day according to the formula: , whereas x1 and x2 are fresh weights measured at time 1 and time 2, expressed as days. Collected biomass was snap freezed with liquid nitrogen, lyophilized, and stored until further investigation at −60°C. Chlorophylls and carotenoids were determined following the protocol described in Fitzner et al. (2021) with slight modifications. In brief, 10 mg of the macroalgae material was extracted with 500 ml THF/MeOH (1:1, v/v) and analysis was performed with an Agilent Technologies 1290 Infinity UHPLC coupled with a ToF (Agilent Technologies Sales and Services, GmbH & Co. KG, Waldbronn, Germany). The column temperature was set to 15°C.
Insect assay: Experimental organism and substrate for shelter and rearing
Known for their agility and partly aggressive behavior, crickets (A. domesticus) were chosen for direct material stress tests. Adult house crickets, purchased from the Tropic Shop (Nordhorn, Germany), were placed inside 22 L transparent plastic boxes (n = 10 per box) with a metallic mesh-covered lid for ventilation and reared in a climatic chamber (Polyklima, Freising, Germany) at 32°C under an 8 h photoperiod with 150 μmol m−2 s−1 PPFD and a 70% relative humidity (Fernandez-Cassi et al., 2019). Crickets were fed with commercial pellets (Tropic Shop, Nordhorn, Germany, Supplementary Table 1) and water fused with a hydrogel to avoid drowning. The materials brought in contact with the crickets were aimed to serve as shelter and to replace the egg carton, a disposable waste product commonly used in cricket production that is not recyclable (Mellberg and Wirtanen, 2018).
In this respect, the surface area of the egg carton (pyramid shape) and experimental substrates (cylindrical shape) were calculated to provide comparable amounts. Compared to the environmental influences during algae cultivation, no damaging effects on the material can be assumed when insects are reared in a conventional air atmosphere and at temperatures of 32°C. Preliminary tests have indicated that the egg cartons show insect-related damage after only 3 days and that adult animals are responsible for main damaging effects. Potential material toxicity was another important aspect considered during the present study. House crickets are reported to be highly sensitive toward contact to inappropriate materials or growing conditions, reacting with death after few days (Vaga et al., 2020) or even few hours (McCluney and Date, 2008). To detect material-related elevated mortality and avoid interferences with other potential detrimental parameters like overpopulation, nymphal stage, or competition among insects, adult animals were chosen and brought in direct contact with the autoclavable materials for 3 days. As it has been suggested that an individual cricket requires a minimum of 2.5 cm2 surface (Orinda et al., 2021), a surface of 160 cm2 substrate/cricket was provided to ensure that cannibalism and competition among crickets would not affect their response and dying rate.
In two subsequent experimental runs, crickets were exposed for a period of 72 h to the different materials, namely M1, M2 (first run), and M3 (second run), whereas egg carton served as a procedural control unit. All experiments were conducted in triplicate. To investigate the potential impact on the cricket's physiology, three different parameters were calculated before and after each experimental run using the following equation (Mole and Zera, 1993): P7 = average weight: maver.(g/cricket) = , P8 = percentage survival: Survival (%) = (where Ni is the number of crickets on day i and N0 the number of crickets).
Statistical analyses
Differences in the parameters between different treatments were compared as one- or two-way analyses of variances (ANOVAs) using the Statistica software (version 13.5.0.17, TIBCO, USA). Data were checked for homogeneity using the Cochran test. In cases where homogeneity was not detected, the significance of inter-group differences was tested with a non-parametrical Kruskall Wallis test. Significant differences (p < 0.05) were corrected a posteriori using the post-hoc Tukey test.
Results
Material properties and resistance
Of the 10 tested materials, four (M1–M4) provided good dimensional stability during the initial sterilizing autoclaving process (Figure 1). None of the aramid-containing materials (M5–M7) was found to be stable enough having observable cracks and surface roughness (Supplementary Figure 1). This observation is in line with the material's known high water absorption (Yin et al., 2017). Moreover, the matrix-free polyamide (M8–M9) and polyester (M10) materials were shown to be instable, which may be due to the thermal exposure. The effect is recognizable by the loosening of the fiber structures (Supplementary Figure 1).
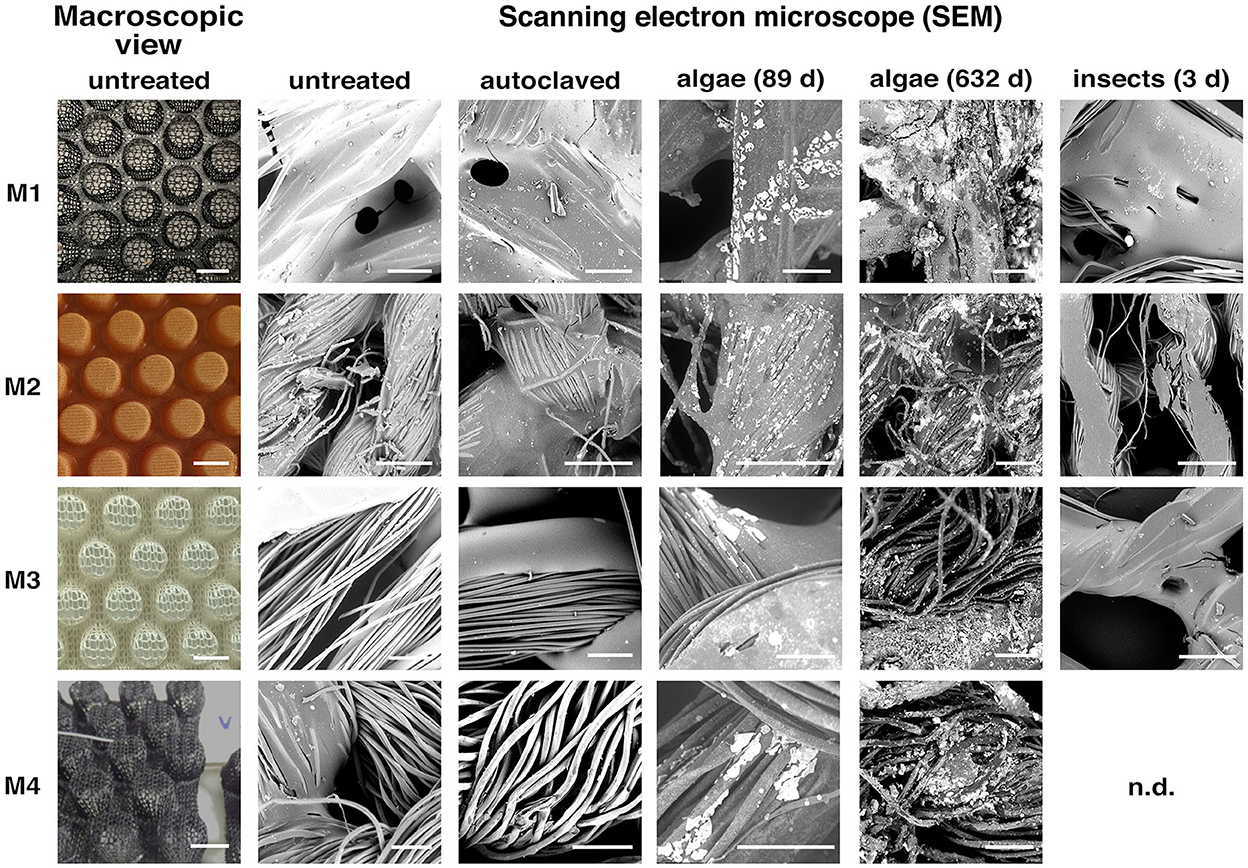
Figure 1. SEM images of experimental materials (M1–M4). Microscopic images taken with a scanning electron microscope (SEM) at a magnification of X1000 at high dynamic range. Showing material before (untreated) and after physical pressure (autoclaved) and exposure to the marine macroalgae Ulva fenestrata (Algae) for a period of 89 days (left) and 632 days (right) at 3.5% salinity at 16°C and crickets Acheta domesticus (Insect) for a period of 3 days at 32°C and 70% relative humidity. Scale bar size in macroscopic views of materials (first column) is 1 cm length and in detail view of materials is 200 μm length. n.d. indicate missing material in insect treatment.
The remaining PLA (M3), cellulose (M2), and polyester-based (M1, M4) materials were considered in further organism exposure experiments. Some material durability issues were directly observable during the experimental runs: M1 showed agglutinations between the fibers, M2 floated in the algal cultivation media and M4 disintegrated by losing fibers, makes it the least suitable. Consequently only material M1–M3 were exposed to the crickets.
Scanning electron microscopy (SEM) revealed a high similarity of M2–M4 in their textile fiber structure. These fibers were 10–20 μm in diameter and formed a loose yarn. In contrast, M1 consisted of significantly thicker fibers of about 75 μm in diameter (Figure 1—untreated). The SEM images also showed that the thick fibers were a compound of melted thinner fibers of different materials. In the SEM images, the matrix resin was visible for materials M1 and M2. While for M1, the resin was mainly between the thick fiber compounds, for M2, the yarn was fully infiltrated with the phenolic resin.
After mid- and long-term exposure to salt water (3.5% salinity, 89 and 632 days), the fiber structure was retained in all materials. Even after long-term exposure, there was no measurable change of the fiber thicknesses. In contrast, optical changes were found in all four materials. The diffuse reflections indicate that the surface of the materials was heavily roughened during the treatment. This can be caused by either erosive processes or particle deposition.
The deposits of the algae cultivation are recognizable by their bright appearance on the SEM images. After 89 days of algae cultivation (mid-term exposure), they could be found on two of the three examined materials (M3, M4). Both M3 and M4 had small particles (<20 μm) between the fibers and few superficial deposits on them. The extent of the deposits was higher in M3 than in M4. Material M2 did not significantly change and the degree of contamination was found to be just slightly higher than before the experiment (Figure 1—Algae 89 days).
Notably, after 632 days of algae cultivation (long-term exposure), all the specimens (including M1 and M2) showed a high level of superficial fouling. The highest amount of deposit was found on M1, where almost the entire fiber surface was covered with additional material. The fibers of M2 and M4 were also partially covered with deposits. Only M3 did not show much difference compared to the mid-term examined specimens. This observation may be due to the already high degree of deposition in the mid-term exposed specimens (Figure 1—Algae 632 days).
Changes of the fiber texture were observed in areas without any deposits for all materials after exposure to algae and salt water, especially after 632 days. A roughened fiber surface was visible for all specimens, which is also why the fibers appeared to be very dark in the SEM images. The superficial changes were most likely due to the corrosive properties of the salt water and the growth of algae. As a rougher surface was found to be a better support for the algae cultivation (Fletcher and Callow, 1992), the suitability of the examined materials may be increased over exposure time. Thus, since there was no measurable reduction of the fiber cross-sections all tested materials were found to be suitable for long-term use in a saline environment (>600 days).
Macroalgal–material interactions
No loss in vitality (P1), measured as loss in pigmentation, was observed during the study, thereby indicating that there were no severe effects on the algal survival (Table 3). Consequently, P1 was excluded from further statistics. From the four tested materials, the phenolic resin-based M1 and M2 had the largest effect on the macroalgal development (Table 3).
M1 showed a strong biological fouling with 100 % attachment reached within the first 4 days (Figure 2B), and thus, was more supportive for Ectocarpus sp. than the control unit (maximum of 75 % attachment) (Table 3, Supplementary Table 2). Furthermore, this material affected the brown algae by reducing growth and suppressing the thallus morphology since no erect growing filaments were observed even after 28 days of exposure (Figure 2A). In contrast, neither a reduction in growth nor a change in thallus morphology was measured for U. fenestrata—the observation of which was hampered due to the dark material coloration.
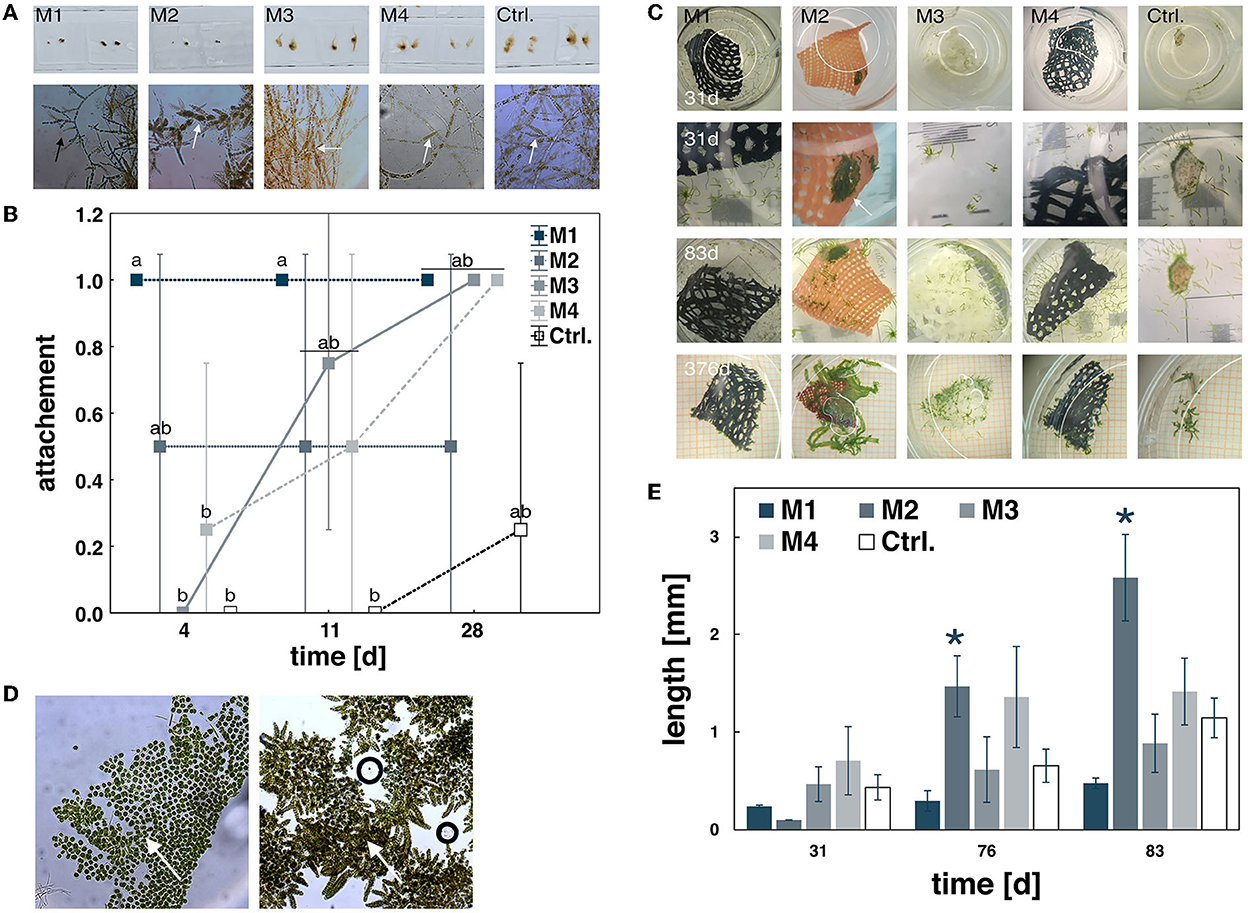
Figure 2. Material tests with the brown alga Ectocarpus sp. and Ulva fenestrata. Thalli of Ectocarpus sp. after 28 days of exposure showing upright and creeping growth (black arrow) and fertile sporangia (marked by white arrows) (A) and differences in biofouling measured by % thallus attachment over 28 days (B). Data are expressed as mean ± SD; significant differences (p < 0.001) are indicated by lower case letters. The green alga Ulva fenestrata showing differences in germling development over time (C), observed as crustose (left, arrow) and normal erect growth (right, arrow) at 31 days of exposure (D), and differences at further increased lengths growth after 31 days (E). Data expressed as mean ± SD; significant differences (p < 0.001) are indicated by asterisks.
M2 was least supportive for Ectocarpus, with a strong negative impact on thallus growth (Table 3, Supplementary Table 2, Figure 2A). Moreover, U. fenestrata germlings were found to be strongly affected by the material and having a changing impact over the exposure time. After 4 days, settled spores were clearly visible on the material. After 18 days, Ulva germlings started to become visible in the control unit and spores developed to cellular crusts, growing on the material and sides of the well. No germlings were visible within the first 31 days (Figures 2C,D). Surprisingly, after 76 days of exposure, this pattern completely changed as germlings rapidly developed and reached growth rates of about 0.16 mm d−1, which is 2.28 times higher on average than the control unit (Figure 2E).
M4 had no influence on the growth of U. fenestrata and Ectocarpus sp. and allowed only limited adherence. The material was also frayed and the dark color made it difficult to see the seedlings. Thus, this material was deemed less suitable for cultivation compared to M1, M2, and M3 (Table 3, Supplementary Table 2, Figure 2).
Compared to the other materials, M3 was identified as the most suitable for algal cultivation. For the brown algae Ectocarpus sp., this material supported its attachment and normal fertile development (Table 3, Supplementary Table 2, Figure 2). For the green alga U. fenestrata, this material supported the development of a large number of seedlings, which were easy to identify macroscopically due to their light color. Reaching growth rates of 4.23 ± 0.81% days by the end of the study, no significant differences were found for either biomass or growth. To evaluate the potential impact on the nutritional composition of the cultivated macroalgae, the carotenoid and chlorophyll contents were determined. Here, there was no significant differences between M3 and the control treatments (Table 4). Thus, these observations suggest that M3 is a suitable material for the cultivation of macroalgae, including in the context of the construction of for food production systems.
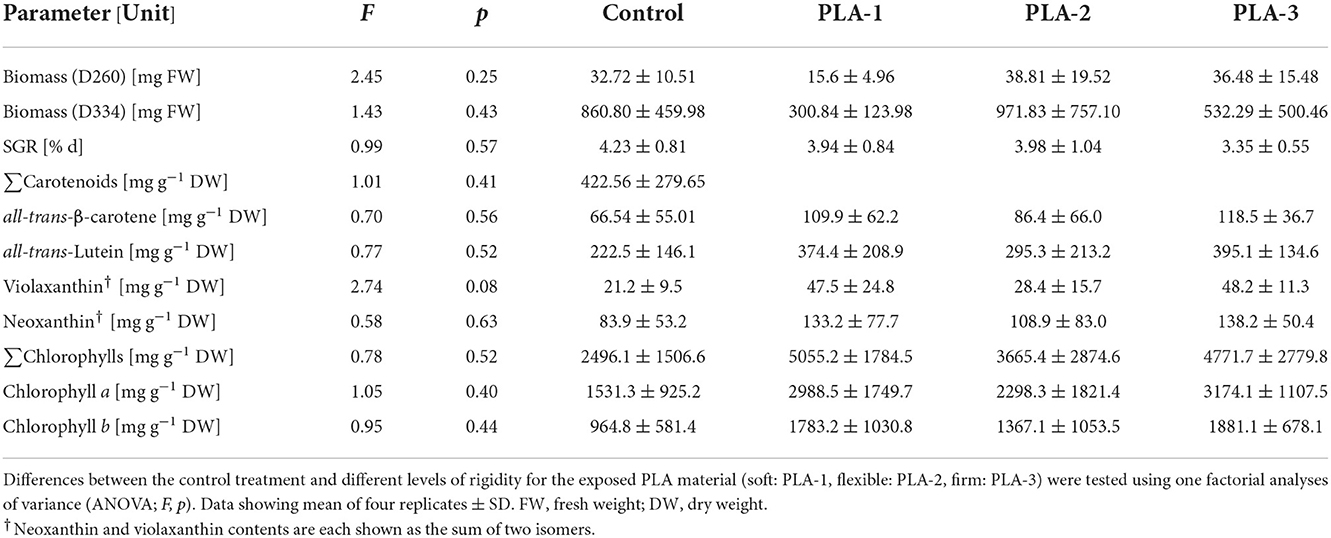
Table 4. Differences in biomass, specific growth rates (SGRs), carotenoid and chlorophyll concentrations of Ulva fenestrata grown exposed to different PLA materials (types 1–3) under a 10 h photoperiod with artificial LED illumination (75 μmol m2 s−1 PPFD) at 18°C in artificial seawater (tropic marine) at 2.9 % salinity.
Insect: Material interactions
No significant differences in cricket physiology were found between egg carton (control) and material-treated crickets (Figure 3). In fact, all tested parameters of the crickets (P7–P9) did not show any significant differences (p < 0.05; Table 3, Supplementary Table 2). At the end of each experimental period, crickets showed a survival rate that ranged from approximately 82–93% for all tested materials without significant differences among the different treatments. The crickets reared under the artificial LED conditions with the standard egg carton had a weight of 0.34 ± 0.01 g per cricket on the first run and 0.33 ± 0.01 g per cricket on the second.
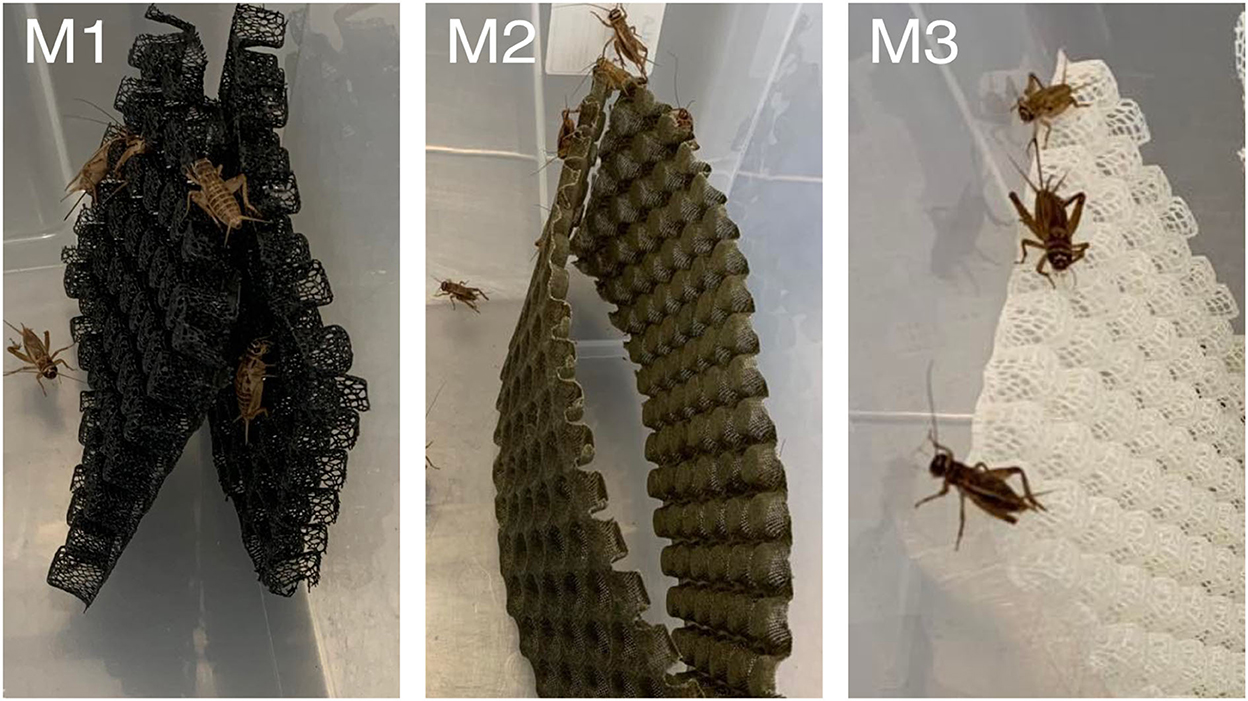
Figure 3. Material test with Acheta domesticus. Adult crickets in direct contact with different materials M1–M3.
Discussion
Polylactic acid (PLA, M3) was identified as the most suitable material for cultivation in the experiments since it supported both macroalgae and crickets used in this study, which otherwise are known to be highly sensitive toward their cultivation conditions (Clifford et al., 1977; Dominguez and Loret, 2019).
In fact, the macroalgae responded to exposure to unsuitable materials in a short time, showing alterations in their growth and morphological development within days. A reduction in growth and alteration in morphology in these macroalgae could be related to an uptake of pollutants from the surrounding media as shown for heavy metal exposure (Vecchia et al., 2012; Roncarati et al., 2015). Apart from the toxic nature of the incorporated pollutants as regards human consumption, the observed morphological changes could also affect the algal metabolism, e.g., due to shifted surface to volume ratios (Israel et al., 1995; Taylor et al., 1998), which in turn would also affect the quality of the crop.
Polylactic acid was shown not to affect algal development or nutritional composition (carotenoid and chlorophyll concentrations). Moreover, PLA not only helped to increase the visibility of the early stages of macroalgae development and growth, but also even supported its cultivation. In addition, the crickets accepted PLA as a shelter substrate, which did not disturb their physiology, and they did not affect the material themselves by, e.g., biting. Notably, PLA also provided form constancy even under exposure to corrosive aquatic or humid environments as well as was resistant to the necessary sterilization procedure. Since PLA is considered as a bioplastic as it is made from renewable sources, e.g., corn starch, it has become one of the most promising components—a fact indicated by a global increase in use from about 2.11 million in 2020 to about a predicted 2.87 million tons in 2025 (Wellenreuther et al., 2021). Polylactic acid also has a wide application potential, including as a filament in 3D printing (Prendiz et al., 2019). Since PLA is regarded as a feasible and sustainable alternative for fossil-based packaging, this material has become one of the most used biopolymers in the agriculture industry (Taib et al., 2022). Despite PLA being considered to be biodegradable, different studies have reported its stability and long durability and that it is compostable only under higher temperatures and industrial conditions (Prendiz et al., 2019; McKeown and Jones, 2020). This study therefore underlines that PLA is a valuable and stable construction material. Nevertheless, due to the observation of some slight erosions on the PLA material, its potential effect on surrounding biota and the environment needs further evaluation under marine conditions.
In contrast to PLA, the phenolic resin materials M1 and M2 showed the strongest impact on the algae. Considered as a versatile precursor to a large array of drugs and an essential commodity chemical, phenol is widely applied in different industries (e.g., pharmaceuticals, cosmetics, chemicals, construction, etc.). However, phenol is a known toxic pollutant, and thus, is regarded as unsuitable for food production (Arumugam et al., 2018). The observed alterations in algal growth exposed to M2 (regenerated cellulose) could indicate a potential loss of the phenolic compounds over time—potentially due to dilution in the surrounding media and uptake by the algae. For example, the genus Ulva is known for its high bio-sorption potential of phenolic compounds (Abbas et al., 2019). A potential incorporation of diluted phenolic compounds would consequently alter the quality and quantity of harvested macroalgal biomass. Nevertheless, to evaluate in which way and to what extent such a potential uptake could affect the nutritional composition, further studies are required.
Of note is that after a period of 28 days, this harmful effect vanished, suggesting that the macroalgae had degraded the phenol (Klekner and Kosaric, 1992). The interesting strong increase in Ulva growth observed for material M2 after a period of about 83 days could be the result of a direct interaction with the cellulose textile, which seemed to serve as a feasible carbon source. Indeed, the observed strong erosion of M2, observed over 632 days, supports this hypothesis. This result therefore endorses the use of cellulose textile, potentially without or in combination with a less harmful binder as an effective material for macroalgal cultivation for food production, e.g., as a settlement substrate. However, its use should be restricted for longer lasting cultivation purposes due to its limited life span.
Regarding the crickets, the phenolic-containing materials, namely M1 and M2, did not have any visible toxic impact or stress effects on the growth performance of the crickets. The measured comparable survival rate and growth performance of the crickets indicated that all three tested materials (M1–3) provided a suitable replacement for the egg carton as a shelter in the rearing of A. domesticus. Compared to the egg carton the further use of these materials is bi-fold, as they can be sterilized and thus re-used and allow additional further construction possibilities. That crickets can be successfully raised using alternative materials and shapes for their hideouts was shown in the proposed bamboo based system by Ng'ong'a et al. (2021).
Crickets are nocturnal insects and require a shelter to hide in to prevent stress and cannibalism as well as to support their growth and development of the immune system. Usually the provided shelter is either egg carton per trays that are placed vertically to assist the movements of the crickets. It is noted that the shelter can collect waste, such as frass, exuviae, or dead insects, and is also difficult to keep clean (Orinda et al., 2021). However, egg cartons or egg trays require several cleaning procedures, such as brushing, air-drying in the sun, or even baking at mild heating conditions, to keep them clean (Hanboonsong et al., 2013). In addition, such cleaning procedures generate a considerable amount of solid waste since the egg carton per trays can be damaged or contaminated with mold (Hanboonsong and Durst, 2020), attract predators such as spiders (Orinda et al., 2021), and also cannot be reused for insect farming (Mellberg and Wirtanen, 2018). To reduce waste, reusable alternatives are in high demand. In detail, bamboo stems have been proposed to be used as hideouts for crickets. However, they still needed to be cleaned with water and dried in the sun weekly (Charles et al., 2021), but given their stability toward more effective sterilization procedures M1–3 could be better alternatives. Moreover, since harmful bacteria like Listeria monocytogenes have been found in biofilm on the floor of cricket rearing systems (Mellberg and Wirtanen, 2018), maintaining hygienic conditions at a high level is crucial to maintain food safety. To sufficiently sanitize the used materials and reduce biofilm formation, the addition of certain anti-microbial agents, such as phenol to the building materials, may be helpful. In this respect, further work is required to identify suitable additives able to control harmful biofilm formers without negatively affecting the food safety.
Conclusion
Based on the findings of the present work, both bio-based and biodegradable composite materials, namely PLA and cellulose, can be considered as alternative materials for the rearing/cultivation of both macroalgae and crickets, and thus, applicable in the construction of resilient urban food production systems. Indeed, based on its stability and acceptance, PLA has considerable potential as a future construction material, whereas, cellulose could be used as a handling material for shorter exposure times, e.g., settlement substrate. Due to the high global interest and demand in bio-based composite materials, recent efforts in the fabrication of these two materials also included the use of food waste and macroalgal components (Helmes et al., 2018; Ögmundarson et al., 2020; Wahlström et al., 2020). Such research will not only lower the fabrication costs and provide a more sustainable alternative, but could even enable a further development of cascade utilization in the food sector. Given the novelty of the approach, the variety of materials, and the diversity of upcoming edible cultivable organisms, further studies are now required to reveal potential impacts on organism physiology and chemistry, that potentially not only affect the final product quantity, but also the overall quality. Moreover, before starting to apply the investigated materials in a commercial farms, further aspects like life cycle assessments need to be taken in account, aiming to compile cost-effective, sustainable, and innovative farming systems. Considering the current technological developments and ever increasing interest in bio-based and biodegredable composite materials, we are confident that the presented approach will have an important impact on the further development of sustainable environmental controlled and resilient food production systems. And hence, contribute to the SDG 12.
Data availability statement
The raw data supporting the conclusions of this article will be made available by the authors, without undue reservation.
Author contributions
AF: investigation, conceptualization, methodology, and writing—original draft. MP, JS, and RR: investigation, methodology, and writing—original draft. MF and JV: methodology and writing—original draft. OS, CD, and MS: validation, resources, project administration, and writing—review and editing. SB: validation, resources, writing—review and editing, and supervision. All authors contributed to the article and approved the submitted version.
Funding
The work was performed in the frame of the BMBF Project food4future (Funding code IGZ: 031B0730A, ATB: 031B0730I, PYCO: 031B0730F) within the Funding Line Agricultural Systems of the Future.
Acknowledgments
We thank Prof. Bauer from Innomat GmbH for her support. We also thank Ute Kieb from the Biological Institute Helgoland (BAH) of the Alfred Wegener Institute, Helmholtz Centre for Polar and Marine Research for collecting and sending algal field material and Dr. Florian Weinberger from the GEOMAR Helmholtz Centre for Ocean Research Kiel for support in Ulva identification.
Conflict of interest
The authors declare that the research was conducted in the absence of any commercial or financial relationships that could be construed as a potential conflict of interest.
Publisher's note
All claims expressed in this article are solely those of the authors and do not necessarily represent those of their affiliated organizations, or those of the publisher, the editors and the reviewers. Any product that may be evaluated in this article, or claim that may be made by its manufacturer, is not guaranteed or endorsed by the publisher.
Supplementary material
The Supplementary Material for this article can be found online at: https://www.frontiersin.org/articles/10.3389/fsufs.2022.1001769/full#supplementary-material
References
Abbas, M. N., Mohammed Al-Hermizy, S. M., Abudi, Z. N., and Ibrahim, T. A. (2019). Phenol biosorption from polluted aqueous solutions by Ulva lactuca alga using batch mode unit. J. Ecol. Eng. 20, 225–235. doi: 10.12911/22998993/109460
Araújo, R., Vázquez Calderón, F., Sánchez López, J., Azevedo, I. C., Bruhn, A., Fluch, S., et al. (2021). Current status of the algae production industry in Europe: an emerging sector of the blue bioeconomy. Front. Mar. Sci. 7:626389. doi: 10.3389/fmars.2020.626389
Arumugam, N., Chelliapan, S., Kamyab, H., Thirugnana, S., Othman, N., and Nasri, N. S. (2018). Treatment of wastewater using seaweed: a review. Int. J. Environ. Res. Public Health 15:2851. doi: 10.3390/ijerph15122851
Ayieko, M. A., and Orinda, M. A. (2020). “Production, nutrient composition, and bioactive components of crickets (Gryllidae) for human nutrition,” in African Edible Insects As Alternative Source of Food, Oil, Protein and Bioactive Components, ed A. Adam Mariod (Cham: Springer International Publishing), 213–224. doi: 10.1007/978-3-030-32952-5_14
Bailey, R., Froggatt, A., and Wellesley, L. (2014). Livestock–Climate Change's Forgotten Sector. Chatham House.
Bringloe, T. T., Starko, S., Wade, R. M., Vieira, C., Kawai, H., De Clerck, O., et al. (2020). Phylogeny and evolution of the brown algae. CRC. Crit. Rev. Plant Sci. 39, 281–321. doi: 10.1080/07352689.2020.1787679
Carl, C., de Nys, R., Lawton, R. J., and Paul, N. A. (2014). Methods for the induction reproduction in a species of filamentous Ulva. PLoS ONE 9:e97396. doi: 10.1371/journal.pone.0097396
Charles, A. N., Christopher, O. G., Erick, O. A. O., and Kevin., O. O. (2021). Economic efficiency of cricket production reared under improvised cage system for improved food production. Afr. J. Agric. Res. 17, 1453–1462. doi: 10.5897/AJAR2021.15649
Chatterjee, A., Debnath, S., and Pal, H. (2020). “Implication of urban agriculture and vertical farming for future sustainability,” in Urban Horticulture, eds S. S. Solankey, S. Akhtar, A. I. L. Maldonado, H. Rodriguez-Fuentes, J. A. V. Contreras, and J. M. M. Reyes (London, UK: IntechOpen). doi: 10.5772/intechopen.91133
Clifford, C., Roe, R., and Woodring, J. (1977). Rearing methods for obtaining house crickets, Acheta domesticus, of known age, sex, and instar. Ann. Entomol. Soc. Am. 70, 69–74. doi: 10.1093/aesa/70.1.69
Coelho, S. M., Peters, A. F., Muller, D., and Cock, J. M. (2020). Ectocarpus: an evo-devo model for the brown algae. Evodevo 11:19. doi: 10.1186/s13227-020-00164-9
Das, R. R., Sarkar, S., Saranya, C., Esakkiraj, P., Aravind, R., Saraswathy, R., et al. (2022). Co-culture of indian white shrimp, Penaeus indicus and seaweed, Gracilaria tenuistipitata in amended biofloc and recirculating aquaculture system (RAS). Aquaculture 548:737432. doi: 10.1016/j.aquaculture.2021.737432
Dominguez, H., and Loret, E. P. (2019). Ulva lactuca, a source of troubles and potential riches. Mar. Drugs 17:357. doi: 10.3390/md17060357
EFSA Panel on Nutrition, N. F., Allergens, F., Turck, D., Bohn, T., Castenmiller, J., De Henauw, S., et al. (2021). Safety of frozen and dried formulations from whole house crickets (Acheta domesticus) as a novel food pursuant to regulation (EU) 2015/2283. EFSA J. 19:e06779. doi: 10.2903/j.efsa.2021.6779
Eismann, A. I., Perpetuo Reis, R., Ferreira da Silva, A., and Negrão Cavalcanti, D. (2020). Ulva spp. carotenoids: responses to environmental conditions. Algal Res. 48:101916. doi: 10.1016/j.algal.2020.101916
European Commission Joint Research Centre. (2019). Brief on Algae Biomass Production. Luxembourg: Publications Office of the European Union.
Fernandez-Cassi, X., Supeanu, A., Vaga, M., Jansson, A., Boqvist, S., and Vagsholm, I. (2019). The house cricket (Acheta domesticus) as a novel food: a risk profile. J. Insects Food Feed 5, 1–22. doi: 10.3920/JIFF2018.0021
Fitzner, M., Fricke, A., Schreiner, M., and Baldermann, S. (2021). Utilization of regional natural brines for the indoor cultivation of Salicornia europaea. Sustainability 13:12105. doi: 10.3390/su132112105
Fletcher, R. L., and Callow, M. E. (1992). The settlement, attachment and establishment of marine algal spores. Brit. Phycol. J. 27, 303–329. doi: 10.1080/00071619200650281
Hanboonsong, A., and Durst, P. (2020). Insects as Food; Gryllidae; Insect Farming; Feeding; Disease Prevention; Waste Management; Sustainability; Farmers; Agricultural Extension; Best Practices; Training Materials. Bangkok: FAO. p. 84. Available online at: https://www.fao.org/documents/card/en/c/cb2446en/
Hanboonsong, Y., Jamjanya, T., and Durst, P. D. (2013). Six-Legged Livestock: Edible Insect Farming, Collection and Marketing in Thailand. Bangkok: Food and Agriculture Organization of the United Nations.
Helmes, R., López-Contreras, A., Benoit, M., Abreu, M., Maguire, J., Moejes, F., et al. (2018). Environmental impacts of experimental production of lactic acid for bioplastics from Ulva spp. Sustainability 10:2462. doi: 10.3390/su10072462
Henderson, B., Lankoski, J., and Giner, C. (2019). Enhancing Climate Change Mitigation through Agriculture. Paris: OECD.
IPIFF. (2020). IPIFF Questionnaire on the EU Market - March 2020. Available online at: https://ipiff.org/wp-content/uploads/2020/06/10-06-2020-IPIFF-edible-insects-market-factsheet.pdf
Israel, A. A., Friedlander, M., and Neori, A. (1995). Biomass yield, photosynthesis and morphological expression of Ulva lactuca. Bot. Mar. 38, 297–302. doi: 10.1515/botm.1995.38.1-6.297
Jerney, J., and Spilling, K. (2020). “Large scale cultivation of microalgae: open and closed systems,” in Biofuels from Algae: Methods and Protocols, ed K. Spilling (New York, NY: Springer New York), 1–8. doi: 10.1007/7651_2018_130
Jose, J., and Joseph, K. (2012). “Advances in polymer composites: macro- and microcomposites - state of the art, new challenges, and opportunities,” in Polymer Composites, Vol. 1, Macro- and Microcomposites, eds S. Thomas, J. Kuruvilla, S. K. Malhotra, K. Goda, and M. S. Sreekala (Wiley), 1–16. doi: 10.1002/9783527645213.ch1
Juul, L., Danielsen, M., Nebel, C., Steinhagen, S., Bruhn, A., Jensen, S. K., et al. (2021). Ulva fenestrata protein – comparison of three extraction methods with respect to protein yield and protein quality. Algal Res. 60:102496. doi: 10.1016/j.algal.2021.102496
Kennard, N. J., and Bamford, R. H. (2020). “Urban agriculture: opportunities and challenges for sustainable development,” in Zero Hunger, eds. W. Leal Filho, A.M. Azul, L. Brandli, P.G. Özuyar and T. Wall. (Cham: Springer International Publishing), 929–942. doi: 10.1007/978-3-319-95675-6_102
Kim, S.-K., Pangestuti, R., and Rahmadi, P. (2011). “Chapter 5 - sea lettuces: culinary uses and nutritional value,” in Advances in Food and Nutrition Research, ed S.-K. Kim (Academic Press), 57–70. doi: 10.1016/B978-0-12-387669-0.00005-3
Klekner, V., and Kosaric, N. (1992). Degradation of phenols by algae. Environ. Technol. 13, 493–501. doi: 10.1080/09593339209385176
Leandro, A., Pacheco, D., Cotas, J., Marques, J., Pereira, L., and Gonçalves, A. (2020). Seaweed's bioactive candidate compounds to food Industry and global food security. Life 10:140. doi: 10.3390/life10080140
Liu, J., Oita, A., Hayashi, K., and Matsubae, K. (2022). Sustainability of vertical farming in comparison with conventional farming: a case study in Miyagi Prefecture, Japan, on nitrogen and phosphorus footprint. Sustainability 14:1042. doi: 10.3390/su14021042
McCluney, K. E., and Date, R. C. (2008). The effects of hydration on growth of the house cricket, Acheta domesticus. J. Insect Sci. 8, 1–9. doi: 10.1673/031.008.3201
McKeown, P., and Jones, M. (2020). The chemical recycling of PLA: a review. Sustain. Chem. 1, 1–22. doi: 10.3390/suschem1010001
Mellberg, S., and Wirtanen, G. (2018). Clean and Easy Cricket Rearing : A Guide on Hygienic Building Design in Rearing Facilities. Helsingin Yliopisto Ruralia-Instituutti.
Mohan, K., Ganesan, A. R., Muralisankar, T., Jayakumar, R., Sathishkumar, P., Uthayakumar, V., et al. (2020). Recent insights into the extraction, characterization, and bioactivities of chitin and chitosan from insects. Trends Food Sci. Technol. 105, 17–42. doi: 10.1016/j.tifs.2020.08.016
Mole, S., and Zera, A. J. (1993). Differential allocation of resources underlies the dispersal-reproduction trade-off in the wing-dimorphic cricket, Gryllus rubens. Oecologia 93, 121–127. doi: 10.1007/BF00321201
Naylor, R. L., Hardy, R. W., Buschmann, A. H., Bush, S. R., Cao, L., Klinger, D. H., et al. (2021). A 20-year retrospective review of global aquaculture. Nature 591, 551–563. doi: 10.1038/s41586-021-03308-6
Ng'ong'a, C., Gor, C., Okuto, E., and Ayieko, M. (2021). Growth performance of Acheta domesticus and Gryllus bimaculatus production reared under improvised cage system for increased returns and food security. J. Insects Food Feed 7, 301–310. doi: 10.3920/JIFF2020.0082
Ögmundarson, Ó., Sukumara, S., Laurent, A., and Fantke, P. (2020). Environmental hotspots of lactic acid production systems. GCB Bioenergy 12, 19–38. doi: 10.1111/gcbb.12652
Orinda, M., Oloo, J., Magara, H., Ayieko, M., and Roo, N. (2021). Cricket Rearing Handbook. SSE-UK. doi: 10.14738/eb.86.2020
Overland, M., Mydland, L. T., and Skrede, A. (2019). Marine macroalgae as sources of protein and bioactive compounds in feed for monogastric animals. J. Sci. Food Agric. 99, 13–24. doi: 10.1002/jsfa.9143
Pintado, J., Ruiz, P., Cremades, J., Masaló, I., Jiménez, P., and Baradad, J. (2017). “Co-culting Ulva ohnoi with antogonistic Phaeobacter bacteria as a strategy to protect fish-algae IMTA-RAS cultures from Vibriosis,” in EAS2017: Cooperation for Growth, Aquaculture Europe 17: Abstracts (Dubrovnic), 914–915.
PRB Dataset. (2021). World Population Data Sheet. Available online at: https://www.prb.org/news/2021-world-population-data-sheet-released/ (accessed August 17, 2021).
Prendiz, J., Vega Baudrit, J., and Mena, M. (2019). Polylactic acid (PLA) as a bioplastic and its possible applications in the food industry. Food Sci. Nutr. 5:48. doi: 10.24966/FSN-1076/100048
Psarianos, M., Ojha, S., Schneider, R., and Schlüter, O. K. (2022). Chitin isolation and chitosan production from house crickets (Acheta domesticus) by environmentally friendly methods. Molecules 27:5005. doi: 10.3390/molecules27155005
Roncarati, F., Sáez, C. A., Greco, M., Gledhill, M., Bitonti, M. B., and Brown, M. T. (2015). Response differences between Ectocarpus siliculosus populations to copper stress involve cellular exclusion and induction of the phytochelatin biosynthetic pathway. Aquat. Toxicol. 159, 167–175. doi: 10.1016/j.aquatox.2014.12.009
Rumpold, B. A., and Schlüter, O. K. (2013). Nutritional composition and safety aspects of edible insects. Mol. Nutr. Food Res. 57, 802–823. doi: 10.1002/mnfr.201200735
Schmitz, T. A., and Kraft, E. (2022). Pilot scale photobioreactor system for land-based macroalgae cultivation. J. Appl. Phycol. 34, 507–516. doi: 10.1007/s10811-021-02617-7
Sebok, S., Brockhagen, B., Storck, J. L., Post, I. B., Bache, T., Korchev, R., et al. (2020). Growth of marine macroalgae Ectocarpus sp. on various textile substrates. Environ. Technol. 43, 1340–1351. doi: 10.1080/09593330.2020.1829086
Shukla, R. S., Skea, J., Calvo Buendia, E., Masson-Delmotte, V., Pörtner, H.-O., Roberts, D. C., et al. (2019). IPCC 2019: Climate Change and Land: an IPCC Special Report on Climate Change, Desertification, Land Degradation, Sustainable Land Management, Food Security, and Greenhouse Gas Fluxes in Terrestrial Ecosystems. IPCC.
SLU Swed ish University of Agricultural Sciences, Department of Biomedical Sciences Veterinary Public Health, Sweden, Fernandez-Cassi, X., Supeanu, A., and Jansson, A.. (2018). Novel foods: a risk profile for the house cricket (Acheta domesticus). EFSA J. 16:e16082. doi: 10.2903/j.efsa.2018.e16082
Steinhagen, S., Enge, S., Larsson, K., Olsson, J., Nylund, G. M., Albers, E., et al. (2021). Sustainable large-scale aquaculture of the northern hemisphere sea lettuce, Ulva fenestrata, in an off-shore seafarm. J. Mar. Sci. Eng. 9:615. doi: 10.3390/jmse9060615
Taib, N.-A., Rahman, M., Huda, D., Kuok, K., Hamdan, S., Bakri, M. K., et al. (2022). A review on poly lactic acid (PLA) as a biodegradable polymer. Polymer Bull. 1, 1–35. doi: 10.1007/s00289-022-04160-y
Tao, J., and Li, Y. O. (2018). Edible insects as a means to address global malnutrition and food insecurity issues. Food Qual. Saf. 2, 17–26. doi: 10.1093/fqsafe/fyy001
Taylor, R., Peek, J., and Rees, A. (1998). Scaling of ammonium uptake by seaweeds to surface area: volume ratio: geographical variation and the role of uptake by passive diffusion. Mar. Ecol. Prog. Ser. 169, 143–148. doi: 10.3354/meps169143
Tso, R., Lim, A. J., and Forde, C. G. (2021). A critical appraisal of the evidence supporting consumer motivations for alternative proteins. Foods 10:24. doi: 10.3390/foods10010024
Vaga, M., Berggren, Å., and Jansson, A. (2020). Growth, survival and development of house crickets (Acheta domesticus) fed flowering plants. J. Insects Food Feed 7, 151–161. doi: 10.3920/JIFF2019.0038
van Huis, A. (2013). Potential of insects as food and feed in assuring food security. Annu. Rev. Entomol. 58, 563–583. doi: 10.1146/annurev-ento-120811-153704
Vecchia, F., Marzocchi, M., Maistro, S., and Moro, I. (2012). Morpho-physiological effects of cadmium on two Ulva species. Arch. Hydrobiol. Suppl. Algol. Stud. 138, 13–25. doi: 10.1127/1864-1318/2012/0003
Ventura, Y., Eshel, A., Pasternak, D., and Sagi, M. (2015). The development of halophyte-based agriculture: past and present. Ann. Bot. 115, 529–540. doi: 10.1093/aob/mcu173
Wahlström, N., Edlund, U., Pavia, H., Toth, G., Jaworski, A., Pell, A. J., et al. (2020). Cellulose from the green macroalgae Ulva lactuca: isolation, characterization, optotracing, and production of cellulose nanofibrils. Cellulose 27, 3707–3725. doi: 10.1007/s10570-020-03029-5
Wellenreuther, C., Wolf, A., and Zander, N. (2021). Cost Structure of Bio-Based Plastics: A Monte-Carlo-Analysis for PLA. HR papers. Hamburg Institute of Economics (HWWI).
Keywords: polylactic acid (PLA), Ulva fenestrata, Acheta domesticus (house cricket), composite light-weight materials, urban aquaculture, controlled environment agriculture (CEA)
Citation: Fricke A, Psarianos M, Sabban J, Fitzner M, Reipsch R, Schlüter OK, Dreyer C, Vogt JH-M, Schreiner M and Baldermann S (2022) Composite materials for innovative urban farming of alternative food sources (macroalgae and crickets). Front. Sustain. Food Syst. 6:1001769. doi: 10.3389/fsufs.2022.1001769
Received: 24 July 2022; Accepted: 21 November 2022;
Published: 09 December 2022.
Edited by:
Dipayan Sarkar, North Dakota State University, United StatesReviewed by:
Abdullah-Al Mamun, Noakhali Science and Technology University, BangladeshDarja Dobermann, Independent Researcher, Utrecht, Netherlands
Copyright © 2022 Fricke, Psarianos, Sabban, Fitzner, Reipsch, Schlüter, Dreyer, Vogt, Schreiner and Baldermann. This is an open-access article distributed under the terms of the Creative Commons Attribution License (CC BY). The use, distribution or reproduction in other forums is permitted, provided the original author(s) and the copyright owner(s) are credited and that the original publication in this journal is cited, in accordance with accepted academic practice. No use, distribution or reproduction is permitted which does not comply with these terms.
*Correspondence: Anna Fricke, ZnJpY2tlJiN4MDAwNDA7aWd6ZXYuZGU=