- 1Department of Microbial Ecology, Netherlands Institute of Ecology, Wageningen, Netherlands
- 2Chair Group Soil Biology, Wageningen University and Research, Wageningen, Netherlands
Chitin, a biopolymer present in fungi and arthropods, is a compound of interest for various applications, such as in the agricultural and medical fields. With the recently growing interest in the development of insect farming, the availability of chitin-containing residual streams, particularly the molting skins (exuviae), is expected to increase in the near future. For application purposes, accurate quantification of chitin in these insect sources is essential. Previous studies on chitin extraction and quantification often overlooked the purity of the extracted chitin, making the outcomes inconsistent and prone to overestimation. The present study aims to determine chitin content in the exuviae of three insect species mass-reared worldwide: black soldier fly (BSF), mealworm, and house cricket. Chitin was chemically extracted using acid and alkali treatments to remove minerals and proteins. The purity of extracted chitin was evaluated by hydrolyzing the chitin into glucosamine, followed by quantitative determination of the latter using two liquid chromatography methods: electrochemical detection (ECD) and tandem mass spectrometry (MS/MS). Both methods proved accurate and precise, without the need for labor-intensive derivatization steps. Pearson's correlation and Bland-Altman plots showed that the glucosamine determination results obtained by the two methods were comparable, and there is no consistent bias of one approach vs. the other. The chitin content in extracted residues ranged between 7.9 and 18.5%, with the highest amount found in BSF puparium. In summary, the study demonstrated that (1) the residual streams of the insect farming industry have a great potential for utilization as an alternative chitin source, and (2) both LC-ECD and LC-MS/MS are reliable for the quantitative determination of glucosamine in insect chitin.
Introduction
Chitin is a naturally occurring polysaccharide composed of N-acetyl-D-glucosamine units linked by β-(1-4) glycosidic bonds and is the second most abundant polymer on Earth after cellulose (Roberts, 1992). It is a major structural component providing rigidity of the cell wall of filamentous fungi and yeasts, as well as of the exoskeleton of arthropods such as insects and crustaceans (Cohen, 1993). Chitin and its derivatives possess many functional properties that make them useful in a broad range of applications, namely as an adsorbent in wastewater treatment (Hubert Ribeiro and dos Santos, 2019), scaffold in bioactive tissue engineering (Yang, 2011), and as a soil amendment to suppress soil-borne diseases (Andreo-Jimenez et al., 2021). Despite its widespread occurrence, so far, the primary source of commercial chitin has been the waste of the seafood processing industry.
With the increasing chitin demand on the global market (Jardine and Sayed, 2018; Silva et al., 2020), studies in the past decade have indicated the need for additional sources. In particular, the chitin component of residual streams of the insect farming industry has become increasingly important. Insects can recover valuable nutrients from agro-industrial waste and efficiently convert them into protein-rich biomass. Insect-based bioconversion offers practical solutions to waste management while at the same time presenting an opportunity to produce protein in a sustainable manner (Dossey et al., 2016; van Huis, 2021). This sector has recently witnessed rapid growth worldwide, with an expected volume of 730.000 metric tons by 2030 (Anon, 2019). Therefore, the residual streams of the insect farming industry, namely the excrements (frass) and the molting skins (exuviae), are expected to become more readily available. The latter is most interesting as a source for chitin purification because exuviae contain a considerable amount of chitin, especially in the innermost layer (procuticle) (Kramer et al., 1995; Roer et al., 2015).
Given the potential of insect exuviae as a viable chitin source, knowledge of the chitin content in this biological source is essential. The determination of chitin content has been performed for many insect species (Hahn et al., 2020b; Mohan et al., 2020; Zainol Abidin et al., 2020), most frequently by adapting a chemical extraction method for crustacean chitin. As is the case with crustacean chitin, insect chitin is tightly embedded in cuticular protein, forming a protective structure with other components such as calcium carbonate, catechol, and pigments (Muzzarelli, 2011). Chitin extraction usually involves the total elimination of these compounds using acidic and alkaline solutions, leaving behind an insoluble residue that is subsequently weighed to determine chitin content (Liu et al., 2012; Kaya et al., 2015; Purkayastha and Sarkar, 2020; Wang et al., 2020). However, because the extraction procedure is not specific to chitin, other insoluble compounds may still be present in the extracted chitin, thus affecting the determination accuracy (Smets and van der Borght, 2021). Hence, a further step to assess the purity of extracted chitin is needed to determine chitin content accurately.
Chitin can be hydrolyzed into glucosamine, an amino sugar building block that can be used to estimate the chitin content in the analyzed material. High-performance liquid chromatography (HPLC) is a powerful instrument for glucosamine measurement, but the detection of glucosamine can be limited due to its poor UV absorbance and lack of fluorescent properties (Harvey, 2011). In order to enhance the detectability, many analytical approaches rely on the prior formation of derivatives aided by reagents such as 9-fluorenylmethoxycarbonyl (Fmoc) (Zhou et al., 2005; Zhu et al., 2005; Han and Heinonen, 2021) and 6-aminoquinolyl-N-hydroxysuccinimidyl carbamate (AQC) (Wang et al., 2008). However, the derivatization procedure is labor-intensive and time-consuming. This step can be avoided when HPLC with electrochemical (ECD) or mass spectrometry (MS) detection is used since both methods allow for the quantitative analysis of carbohydrates in their native, underivatized form. ECD is a conventional method that detects carbohydrate analytes via anion-exchange mechanism, which takes advantage of the weakly acidic nature of carbohydrates (Masuda et al., 2002). This method has been widely used for direct glucosamine determination in biological samples, such as human plasma (Pashkova et al., 2009). Recently, a tandem mass spectrometry (MS/MS) method was established to determine glucosamine in insect chitin samples (D'Hondt et al., 2020). MS/MS is highly selective and suitable for analyzing complex sample matrices (Olofsson and Bylund, 2016), but it has higher investment costs and skill requirements to operate (Brandhorst et al., 2012; Adaway et al., 2015).
The present study aims to compare the ECD with an established MS/MS method and discuss the applicability of the methods to determine chitin content in insect sources. Chitin was chemically extracted from raw exuvial waste of the insect farming industry. The purity of extracted chitin was evaluated by performing acidic hydrolysis of chitin into its monomers (glucosamine, N-acetylglucosamine, acetic acid) followed by their quantification. The determination was performed on the exuviae of yellow mealworm (Tenebrio molitor), black soldier fly (Hermetia illucens), and house cricket (Acheta domesticus). The insects were mass-reared in the Netherlands to produce protein for food and feed and belong to the seven insect species approved for use as aquaculture feed (EU, 2017). Yellow mealworm was also recently approved for human consumption by the European Food Safety Authority (EFSA Panel on Nutrition et al., 2021).
Materials and Methods
Materials
D-(+)-Glucosamine hydrochloride, N-acetylglucosamine, and acetic acid, used as reference standards, were purchased from Sigma Aldrich (St Louis, MO, USA). Theophylline, an internal standard to correct for possible ion suppression in the MS source, was purchased from Sigma Aldrich (St Louis, MO, USA). HPLC-grade water was prepared using a Milli-Q water purification system (Millipore Corp., Bedford, MA, USA). Analytical grade chemicals used for chitin extraction, such as hydrochloric acid (HCl) and sodium hydroxide (NaOH), were obtained from Merck (Darmstadt, Germany). Commercially available purified shrimp shell chitin (Sigma, St. Louis, MO, USA) was used as a reference substrate for the acid hydrolysis process without further purification.
Raw Materials and Pretreatments
Exuviae used for chitin extraction were obtained from three commercially reared insects: yellow mealworm (Tenebrio molitor), black soldier fly (Hermetia illucens), and house cricket (Acheta domesticus) (Table 1). Samples of each insect species were kindly provided by Nijenkamp (Hellendoorn, NL), Bestico (Berkel en Rodenrijs, NL), and Fair Insects (Dongen, NL), respectively, where the insects were reared on various types of agro-industrial waste. Collected exuvial samples were rinsed repeatedly with distilled water to eliminate soluble impurities that may adhere to the external surface. Cleaned samples were oven-dried at 60°C for 24–48 h until a constant weight was obtained. Samples were pulverized into a fine powder using a TissueLyser II stainless steel ball mill (QIAGEN, CA, USA) to increase the accessibility for chemical solvents into the particle. Powdered samples were stored in an airtight container in a desiccator until extraction.
Chitin Extraction
Defatting and Demineralization
As a preliminary step, exuvial samples were defatted according to a previously described method (Choi et al., 2017) to remove lipid fractions which may cause hydrophobic repulsion that prevents the interaction of exuviae with chemical reagents. First, 10 g of each powdered sample was weighed on a precision scale in triplicate. The samples were treated with n-hexane in shaking flasks at room temperature for 48 h, and the solvent was replaced every 12 h. Defatted samples were separated from the liquid fraction by vacuum filtration (VWR cellulose filter paper 413, particle retention 5–13 μm) and dried overnight under a fume hood.
Next, demineralization was conducted to eliminate minerals (mainly calcium carbonate) deposited in insect cuticles. This was done by treating the exuviae with acid, based on a procedure previously tested on various insect species (Marei et al., 2016). The defatted samples were treated with 1 M HCl [solid-to-solvent ratio of 1:20 (w/v)] in shaking flasks at room temperature for 1 h. The acid treatment resulted in slightly discolored biomass. The insoluble precipitate was separated from the salts by vacuum filtration (VWR cellulose filter paper 413, particle retention 5–13 μm), washed thoroughly with distilled water until neutral, and dried overnight at 60°C.
Deproteinization
The removal of proteins was carried out based on a previously suggested method (Sagheer et al., 2009; Marei et al., 2016). Samples were treated with 1 M NaOH in shaking flasks [solid-to-solvent ratio of 1:20 (w/v)] at 80°C for 1 h. The alkali treatment was done several times (9–13 times) with intermediate filtration (VWR cellulose filter paper 413, particle retention 5–13 μm) until the filtered NaOH solution was colorless to ensure that all proteins were solubilized. Light brown-colored chitin precipitate was obtained by the end of the successive treatments (Supplementary Figure 1). Chitin was washed thoroughly with distilled water until neutral, dried overnight at 60°C, and weighed. The end product was stored in a desiccator at room temperature until further analysis.
Acid Hydrolysis
Each biological chitin sample (20.0 mg) was precisely weighed and transferred into heat-resistant glass vials containing 10 mL 6 M HCl. The reaction mixture was briefly homogenized by vortex and sonicated for 15 min to improve the digestibility of the chitin sample. Acid hydrolysis was carried out by incubating the reaction mixture in a high-temperature thermostat at 110°C for 4 h (HT200S, Hach-Lange, Dusseldorf, Germany). After the reaction, the vials were immediately cooled down to room temperature under a fume hood. The resulting hydrolysate was diluted with Milli-Q, filtered through 0.2 μm PTFE membrane filters (VWR International, Atlanta, GA), and used for subsequent chromatographic analysis.
Chromatographic Analysis
After the acid hydrolysis step, the resulting hydrolysate was analyzed for its constituents: glucosamine, N-acetylglucosamine, and acetic acid. Glucosamine and N-acetylglucosamine were analyzed with ECD and MS/MS, and acetic acid was analyzed with LC-UV. The amount of hydrolyzed chitin from biological samples was calculated according to the formulation described by D'Hondt et al. (2020) by taking into account the concentration of chitin constituents and the weight of the extracted insect chitin from each biological source (Equation 1 and 2).
LC-ECD
The concentrations of glucosamine and N-acetylglucosamine were determined using HPLC BioInert 1260 Infinity Agilent (Agilent Technologies Inc., California, USA) with electrochemical detection (ECD, Decade elite Antec). The filtered hydrolysate was diluted (50-fold) in ultrapure water. The chromatographic separation was achieved with a Dionex™ Guard column CarboPac PA1 2 × 50 mm, main column CarboPac PA1 2 × 250 (Thermo Fisher Scientific) at 20°C, employing an isocratic mobile phase consisting of 100% NaOH 0.1 M at a 0.25 ml/min flow rate. A carry-over test was performed by alternately measuring the samples with blanks (MilliQ) to test for substances in the preceding sample that may interfere with the measurement of the subsequent sample. The retention time was 4.2 and 4.5 min for glucosamine and N-acetylglucosamine, respectively. The calibration curves were determined using different concentrations of glucosamine (0.5, 1, 2.5, 5, 7.5 and 10 mg/L) in triplicate. The following validation parameters were determined: linearity (expressed as coefficient of determination, R2), intraday and interday precisions (expressed as coefficient of variation, CV%), limit of detection (LOD), and limit of quantification (LOQ). The interday precisions were studied by measuring three levels of glucosamine in triplicate on three separate days. LOD and LOQ were calculated using a signal-to-noise criterion of 3 and 10, respectively.
LC-MS/MS
Determination of glucosamine and N-acetylglucosamine was also performed on MS/MS system, based on the method adapted from D'Hondt et al. (2020). The filtered hydrolysate was dried with N2 to eliminate HCl, which may influence the retention and pH of the mobile phase, and re-dissolved in 1:1 (v/v) acetonitrile + 0.1% formic acid and MilliQ + 0.1% formic acid. Each sample was diluted (50-fold) in ultrapure water and injected on Agilent 1290 Infinity II UPLC-MS/MS systems (Agilent Technologies Inc., California, USA) equipped with Cogent Diamond Hydride 100 Å column (150 mm x 2.1, 4 μm) and triple quadrupole mass spectrometer (6460C, Agilent Technologies Inc., California, USA). The standard concentration range was 1–10 mg/L. The mobile phase gradient consisted of solvent A (20% MilliQ + 0.1% formic acid) and solvent B (80% acetonitrile + 0.1% formic acid) supplied at a flow rate of 0.5 mL/min. The column temperature was 30°C. The gradient settings were 20–70% A (0–5 min), 70% A (5–7 min), 70–20% A (7–8 min), 20% A (8–15 min). Quantification was performed on protonated ions using Multiple Reaction Monitoring (MRM) mode. After electron spray ionization, the following transitions were monitored: m/z 180 → 162 and 180 → 72 for glucosamine, 181→ 124 for internal standard theophylline, and m/z 222→ 138 for N-acetylglucosamine in positive ion mode. The presence of carry-over substances was checked. The retention time was 3.9 and 1.4 min for glucosamine and N-acetylglucosamine, respectively. Validation parameters such as linearity, precisions, LOD, and LOQ were evaluated and compared with those obtained with LC-ECD.
LC-UV
The acetic acid concentration was determined by injecting filtered, undiluted hydrolysate into HPLC Dionex Ultimate 3000 systems (Thermo Fisher, Agawam, USA) equipped with 340U UV-VIS Detector (UVD) (Thermo Scientific). Separation was achieved by the Hi-Plex H column (300 x 7.7 mm, 8 μm) at 50°C. Isocratic elution was used to separate the target analysis using 100% sulfuric acid 0.01 M. The flow rate was maintained at 0.6 mL/min, and the UV detector was set at 210 nm. The retention time was 15.5 min. The standard concentration range was 0.25–10 mg/L. The presence of carry-over substances was checked.
Data Analysis
Chromatographic data were measured in triplicate and expressed as the mean ± standard deviation. Normality and homogeneity of the variances were verified using the Shapiro–Wilk and Levene's tests, respectively. The student's t-test assessed the statistical differences between two groups. Pearson's correlation (r) was used to measure the degree of a linear relationship between the concentrations of glucosamine measured by ECD and MS/MS. Bland-Altman plot was analyzed to evaluate the difference (bias) between the mean difference and the agreement interval (95%) between ECD and MS/MS in determining glucosamine. Statistical analysis was performed using GraphPad Prism 9.1 (GraphPad Prism software, La Jolla, CA, USA).
Results and Discussion
In the present study, we performed acid-base extraction to purify chitin from the exuviae of different insect species. Next, the purity of extracted chitin was evaluated by hydrolyzing the chitin into its constituents (glucosamine, N-acetylglucosamine, and acetic acid), followed by quantifying the latter with liquid chromatography methods. To our knowledge, this was the first time that the LC-ECD method was adapted for glucosamine and N-acetylglucosamine determination in hydrolyzed insect chitin. The results were compared with a recently established LC-MS/MS method, which has been proven successful in determining chitin content in pure and impure insect chitin samples (D'Hondt et al., 2020).
Comparison of LC-ECD and LC-MS/MS Methods
The chromatograms obtained from HPLC analysis with MS/MS and ECD methods showed distinct separation of glucosamine and N-acetylglucosamine peaks (Supplementary Figures 2A,B, 3). No interfering peaks with similar retention times were observed. A blank check performed on all instruments showed that none of the tested chitin samples contained carry-over substances. All calibration curves presented good linearity with determination coefficients (R2) of 0.991–0.999 (Table 2). After every 10–15 samples in the analytical sequences, the standards were analyzed to check for possible drifts; no drifts were observed throughout the study period. Both analyses require very low sample volumes (5 μL of the 50-fold diluted sample). For both methods, the LOQs (< 0.1 mg/L) were lower than the lowest standard concentration (0.5 mg/L) (Table 2).
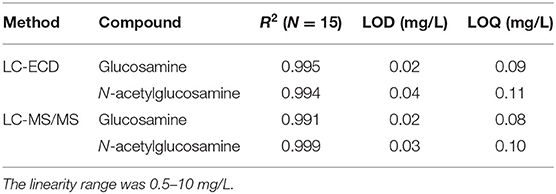
Table 2. Linearity (expressed as coefficient of determinations, R2), limit of detection (signal-to-noise ratio of 3) and limit of quantification (signal-to-noise ratio of 10) of glucosamine and N-acetylglucosamine as assessed by LC-ECD and LC-MS/MS.
Evaluations of intra- and interday precisions for glucosamine determination, expressed as coefficient of variation (CV%), are shown in Table 3. The interday precision, obtained by measuring three levels of glucosamine in triplicate on three separate days, was within the range of 0.6–3% and 1.6–4% for ECD and MS/MS, respectively. With respect to intraday precision, lower CV values of 0.1–0.8% and 0.2–1.7% were obtained for ECD and MS/MS, respectively, indicating good repeatability of both methods for glucosamine determination. For both methods, the overall time needed for setting up and running the samples is generally similar. However, ECD allows faster preparation since the samples can be immediately analyzed without any additional step. In comparison, preparing samples for MS/MS analysis requires extra time to dry out the HCl that may interfere with the analysis and resuspend the dried sample in a suitable solvent.
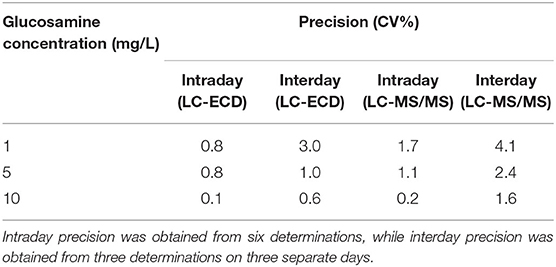
Table 3. Intra- and interday precisions (expressed as coefficient of variation, CV%) for quantifying glucosamine reference solution measured by LC-ECD and LC-MS/MS.
The result of glucosamine and N-acetylglucosamine determination in hydrolyzed commercial chitin and chitin extracts of different insect exuviae is summarized in Table 4. As depicted in the correlation plot (Figure 1), a strong positive relationship was observed between glucosamine concentrations measured by ECD and MS/MS (r = 0.994, y = 0.996x + 0.0151). The paired t-test showed that the glucosamine measurements obtained by the two methods were not significantly different (p = 0.6). The Bland-Altman plot showed a mean bias of −0.01 ± 0.09, and the 95% limits of agreement were −0.17 to 0.19 (Figure 2). The scattered distribution of the sample points indicated no systematic trend in variation, and the variation was not dependent on the use of different detection principles.
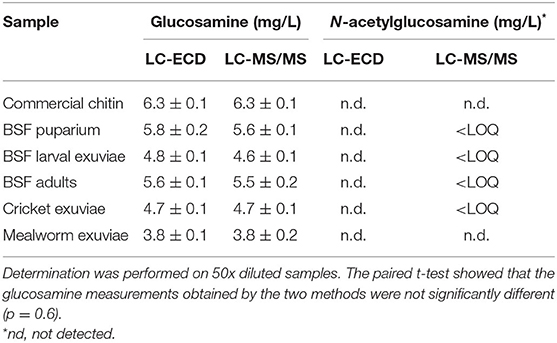
Table 4. Concentrations (mg/L) of glucosamine and N-acetylglucosamine in hydrolyzed commercial chitin and chitin extracts of different insect exuviae as measured by LC-ECD and LC-MS/MS.
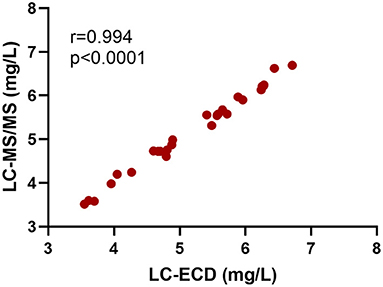
Figure 1. Positive relationship of glucosamine concentrations in hydrolyzed commercial chitin and chitin extracts of different insect exuviae measured by LC-ECD and LC-MS/MS (Pearson's correlation, r = 0.994, p < 0.0001, N = 27). The samples were diluted 50x in both measurements.
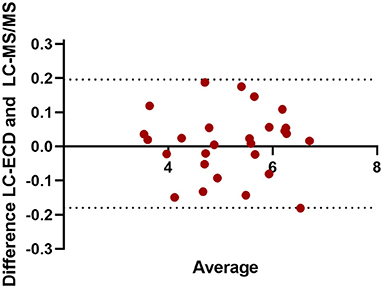
Figure 2. Bland-Altman plot of glucosamine concentrations in hydrolyzed commercial chitin and chitin extracts of different insect exuviae measured by LC-ECD and LC-MS/MS (N = 27). The x-axis shows the mean of the results, and the y-axis shows the absolute glucosamine difference in mg/L between LC-ECD and LC-MS/MS measurement. The mean bias was −0.01 ± 0.09, and the 95% limits of agreement were −0.17 to 0.19.
It was assumed that chitin could be effectively depolymerized by treating with concentrated acid at high temperatures. During the initial phase of acid hydrolysis, the glycosidic linkage of chitin is cleaved, leading to the release of smaller polymeric units and -N-acetylglucosamine (Hackman, 1962; Einbu and Vårum, 2008). Next, the N-acetylglucosamine is deacetylated into glucosamine and acetic acid (Hackman, 1962; Einbu and Vårum, 2008). According to the results obtained with the ECD method, N-acetylglucosamine was not detected in any of the samples. However, with the highly sensitive MS/MS detection, trace amounts of N-acetylglucosamine (below LOQ) were detected in several insect chitin samples, but not in mealworm exuviae chitin and commercial chitin. This indicates that, while the hydrolysis condition was optimum for commercial chitin, the condition was not completely optimal for most insect chitin samples, since not all of the N-acetylglucosamine monomers were deacetylated after undergoing the hydrolysis procedure. Temperature, duration, and concentration of HCl solution are important factors affecting the optimum hydrolysis of chitin (Crespo et al., 2006; Einbu and Vårum, 2008; D'Hondt et al., 2020). Inadequate acid treatment may result in incomplete chitin hydrolysis, whereas excessive treatments may lead to monomer degradation and lower recovery. Even though the N-acetyglucosamine concentrations detected in this study were below the quantification limit, further study could be conducted to optimize the acid hydrolysis conditions for chitin obtained from different insect materials.
Application in Determining Chitin Content in Insect Residual Streams
Based on the hydrolysis condition and determination methods used in our study, the chitin content measured in pure commercial chitin was 88–89% (Table 5), indicating that the commercial shrimp chitin might contain impurities. This finding is in agreement with the observation of a previous liquid chromatography-based study, in which the chitin standard was shown to contain 82–87% chitin (Crespo et al., 2006; Smets and van der Borght, 2021). The exoskeleton of hexapods and decapods are heavily cross-linked with compounds such as amino acids, catechols, and pigments (Suderman et al., 2006; Andersen, 2010; Roer et al., 2015), and it is possible that some residues remained after chitin purification (Smets and van der Borght, 2021). Further evaluations should be performed to verify the presence of residues in pure commercial chitin.
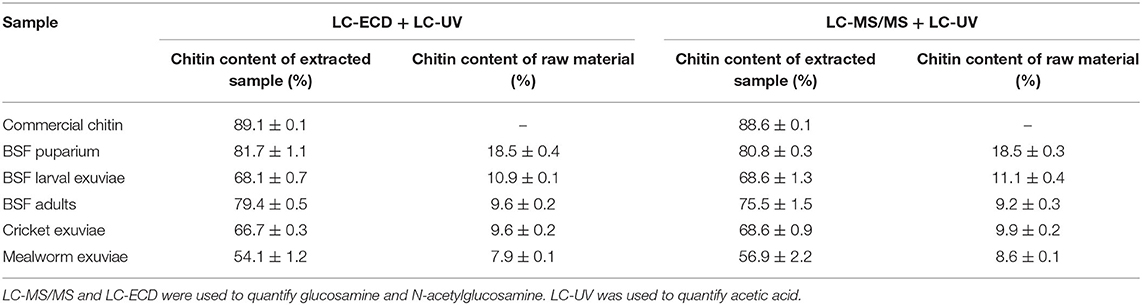
Table 5. Chitin content of the extracted samples (%) and the estimated chitin content of the raw materials (%) based on the obtained LC data.
Chitin extraction on insect exuviae was performed using a method adapted for crustacean chitin, which was achieved by treating the samples with acid and base to remove minerals and proteins. Insect exuviae are known to contain considerably lower calcium carbonate deposits than crustacean shells (Tolaimate et al., 2003; Roer et al., 2015). Thus, demineralization of this material can be accomplished under mild conditions, and in some cases, this step is excluded from the procedure (D'Hondt et al., 2020; Jantzen da Silva Lucas et al., 2021). In contrast, deproteinization is crucial since chitin is compactly bound with cuticular protein in the chitin-protein matrix. This step is challenging since strong conditions are necessary to break the hydrogen bonds between chitin and protein, but prolonged exposure to such a harsh environment also poses a risk of degrading the chitin (Tan et al., 2021). In addition, insect exuviae are diverse, varying in thickness, strength, and elasticity (Andersen, 2010), and so far, there is no standardized extraction method for all types of exuviae. Therefore, the chitin extraction procedure should be tuned accordingly for different insect materials. Depending on insect species, life stage and body parts, deproteinization is typically carried out by treating the specimens with 0.5 M−2 M NaOH, at a temperature of 80–100°C, and incubation time of 16–20 h (Hahn et al., 2020b; Mohan et al., 2020).
In the present study, deproteinization of different exuviae was tuned by adjusting the number of repetitions needed to obtain a “clean” chitin precipitate. As described in section Deproteinization, deproteinization was performed by treating the samples with 1 M NaOH at 80°C for 1 h, and this step was repeated multiple times until the alkali solutions were clear to ensure that all proteins were eliminated. During the study, it was observed that fewer deproteinization cycles were needed to obtain clean chitin precipitate from BSF puparium (nine cycles), whereas more cycles were required for mealworm and cricket exuviae (13 cycles). This observation was comparable to previous studies employing a similar extraction method, in which 12–13 cycles of deproteinization were required to purify chitin from BSF source (D'Hondt et al., 2020; Soetemans et al., 2020).
The chitin purity of each biological sample, as based on glucosamine and acetic acid concentrations, was taken into account to correct for the amount of chitin obtained following the gravimetric extraction procedure, thus avoiding overestimation of chitin content. The purity of extracted chitin is presented in Table 5. All insect chitin contained a certain degree of impurities, even though the samples had been meticulously cleaned via successive purification steps. The highest purity was observed for chitin derived from BSF puparium (80–81%). Lower purity was observed for the chitin obtained from mealworm exuviae (54–56%) and cricket exuviae (66–68%), suggesting that chitin purification from these samples were challenging. A similar result was also observed by D'Hondt et al. (2020), where purified BSF chitin was shown to contain 64–71% chitin based on a liquid chromatography evaluation.
We assume that the less successful chitin purification from mealworm and cricket exuviae was due to their high lipid content since hydrophobic repulsion was observed throughout the study, thus reducing the accessibility of the acid and alkali solutions to solubilize minerals and proteins (Tan et al., 2021). The outer region of insect exuviae, especially that of mealworm larvae, contains a considerable amount of lipid (Pasquier et al., 2021), which serves as a barrier to protect the insect from abrasion and infection (Roer et al., 2015). Removal of the lipid fraction was attempted by pre-treating the exuviae with n-hexane according to a previously described method (Choi et al., 2017), but this additional step did not yield sufficient improvement in reducing the hydrophobic repulsion. Higher purity of extracted chitin can be achieved, for example, by applying the design of experiments that optimize all of the factors influencing extraction efficiency (e.g., different temperature, reagent, incubation time) as demonstrated by Hahn et al. (2020a), since an optimized deproteinization can also simultaneously eliminate soluble lipids (Hahn et al., 2020b). However, this complex design was not feasible due to the large assortment of tested insect exuviae in our study.
Based on the estimated chitin content in insect samples (Table 5), BSF puparium had a higher chitin content (18.5%) than the exuviae shed during the larval stage (10.9–11.1%) and the intact BSF at the adult stage (9.2–9.6%). This finding agrees with previous studies (Brigode et al., 2020; Wang et al., 2020), where the chitin content in the BSF puparium was found to be the highest compared to other development stages. During puparium formation, it is likely that the insect cuticle is sclerotized, and higher production of chitin occurs to increase protection before the pupal stage begins (Andersen, 2010; Soetemans et al., 2020). The lowest chitin content in tested BSF samples was observed in BSF adult flies, most likely due to the presence of other components that make up the intact biomass, such as protein and lipids (Brigode et al., 2020). Chitin in the exuviae of house cricket at various instar stages was 9.6–9.9%, slightly lower than the reported chitin content (10.9%) in the adult exoskeleton of different cricket species (Gryllus bimaculatus) (Kim et al., 2017). The estimated chitin content in mealworm exuviae was 7.9–8.6%, lower than the value of 11% and 18% obtained in previous studies (Song et al., 2018; Pasquier et al., 2021). Discrepancies in chitin yield between studies could be attributed to differences in species, life stages, body parts, and the extraction procedure itself. Furthermore, in this study, the purification degree (%) was used as a factor to correct for the presence of impurities in the extracted chitin. Consequently, this may contribute to lower chitin amount compared to other studies where the purity of extracted chitin was not evaluated.
The insect chitin extracts retained a light brown or grayish appearance (Supplementary Figure 1). This indicates the presence of pigment (e.g., melanin), which may comprise up to 2.5 mg in a BSF individual at the pupal stage (Ushakova et al., 2017). When a non-pigmented product is preferred, an additional decolorization step can be introduced, namely by treating the chitin with potassium permanganate (Liu et al., 2012; Waśko et al., 2016), sodium hypochlorite (Poerio et al., 2020), glacial acetone (Soon et al., 2018) and water-methanol-chloroform solution (Mol et al., 2018).
In highly automated rearing systems, frass and exuviae can be easily separated using a vacuum separator, taking advantage of their difference in settling velocity and density (Kröncke et al., 2020). Separation of exuviae and frass can also be done by sieving based on particle size (Wynants et al., 2018). In rearing facilities, casted-off exuviae are continuously exposed to frass. Thus, in systems where separation is not optimal, there is a risk of measuring chitin from fungi, which grow well on highly decomposable material like frass (Poveda et al., 2019). Exuviae may also contain fungal spores, but due to the hydrophobic and antifungal properties of cuticular lipid (Gołebiowski et al., 2014), the expected abundance is low. An additional measurement could be conducted to quantify fungal biomass in frass (e.g., qPCR or ergosterol analysis) to evaluate whether the presence of frass-derived fungi in collected exuviae significantly influences the determination of chitin content.
The extraction method used in this study used concentrated acid and base solutions and may lead to a considerable amount of waste when applied on a larger scale. Recently, several studies have initiated the development of environmentally friendly extraction methods where less or no conventional organic solvents were used. For example, the use of alcalase, an endoproteinase enzyme from Bacillus licheniformis, was considered a promising option to solubilize proteins from mealworm exuviae (Jantzen da Silva Lucas et al., 2021). Liquid fermentation by lactic acid-producing Lactobacillus plantarum, protease-producing B. subtilis, and lipase-producing Pseudomonas fluorescens was used to obtain chitin from BSF exuviae (Tan et al., 2021). The possibility of using deep eutectic solvents was also evaluated to remove minerals and proteins from BSF prepupae (Zhou et al., 2019). Another alternative chitin extraction method was superheated water hydrolysis (treating exuviae with 150°C water for 20 h), which reportedly could weaken and disrupt hydrogen bonds, leading to the liberation of chitin molecules from the chitin-protein matrix (Bhavsar et al., 2021). Further research should focus on optimizing these green extraction methods to minimize the environmental impacts of harsh chemicals, especially for industrial purposes where maximum chitin yield is desired.
Conclusion
Emerging studies have shown great interest in the potential application of the chitin component in the residual streams of insect farming. Thus, knowledge of the chitin content in this novel source is becoming increasingly important. For the first time, liquid chromatography with electrochemical detection (ECD) was successfully applied for quantitative analysis of glucosamine to evaluate the purity and estimate chitin content in insect exuviae. The determination results were accurate, precise, and in good agreement with those obtained by an established tandem mass spectrometry (MS/MS) method. Even though ECD is generally less sensitive than MS/MS, namely in detecting trace amounts of N-acetylglucosamine, the method also presented practical advantages, such as less preparation time and lower instrument cost than MS/MS. The HPLC analysis revealed the presence of impurities in the extracted chitin, but this factor was weighed in the calculation to avoid chitin overestimation. Further research is warranted to optimize the acid hydrolysis conditions of the chitin, as this could significantly influence the final results. With the high chitin content (7.9–18.5%) and the forecasted growth of the insect farming industry, insect exuviae serve good prospects as a valuable alternative source of chitin.
Data Availability Statement
The original contributions presented in the study are included in the article/Supplementary Material, further inquiries can be directed to the corresponding author.
Author Contributions
AN and WdB designed the research. AN carried out the experiment, analyzed the data, and wrote the manuscript in consultation with WdB. All authors contributed to the article and approved the submitted version.
Funding
This work was supported by the Dutch Research Council (NWO) [grant number ALWGK.2016.010]. This publication is publication number 7322 of the Netherlands Institute of Ecology (NIOO-KNAW).
Conflict of Interest
The authors declare that the research was conducted in the absence of any commercial or financial relationships that could be construed as a potential conflict of interest.
Publisher's Note
All claims expressed in this article are solely those of the authors and do not necessarily represent those of their affiliated organizations, or those of the publisher, the editors and the reviewers. Any product that may be evaluated in this article, or claim that may be made by its manufacturer, is not guaranteed or endorsed by the publisher.
Acknowledgments
The authors are thankful to Ciska Raaijmakers for technical assistance in HPLC analyses.
Supplementary Material
The Supplementary Material for this article can be found online at: https://www.frontiersin.org/articles/10.3389/fsufs.2021.795694/full#supplementary-material
Supplementary Figure 1. The final product of the chitin extraction procedure (left to right: pure commercial chitin, mealworm exuviae, BSF adult, BSF puparium, BSF larval exuviae, cricket exuviae).
Supplementary Figure 2. (A) LC-MS/MS chromatogram of glucosamine standard. (B) LC-MS/MS chromatogram of N-acetylglucosamine standard.
Supplementary Figure 3. LC-ECD chromatogram of glucosamine and N-acetylglucosamine standards.
References
Adaway, J. E., Keevil, B. G., and Owen, L. J. (2015). Liquid chromatography tandem mass spectrometry in the clinical laboratory. Ann. Clin. Biochem. 52, 18–38. doi: 10.1177/0004563214557678
Andersen, S. O. (2010). Insect cuticular sclerotization: a review. Insect Biochem. Mol. Biol. 40, 166–178. doi: 10.1016/j.ibmb.2009.10.007
Andreo-Jimenez, B., Schilder, M. T., Nijhuis, E. H., te Beest, D. E., Bloem, J., Visser, J. H. M., et al. (2021). Chitin- and keratin-rich soil amendments suppress Rhizoctonia solani disease via changes to the soil microbial community. Appl. Env. Microbiol. 87:e00318–21. doi: 10.1128/AEM.00318-21
Anon (2019). Edible Insects Market by Product Type (Whole Insect, Insect Powder, Insect Meal), Insect Type (Crickets, Black Soldier Fly, Mealworms), Application (Animal Feed, Protein Bar and Shakes, Bakery, Confectionery, Beverages)–Global Forecast to 2030. Available online at: https://www.researchandmarkets.com/reports/4757400/edible-insects-market-by-product-type-whole (accessed May 23, 2021).
Bhavsar, P. S., Dalla Fontana, G., and Zoccola, M. (2021). Sustainable superheated water hydrolysis of black soldier fly exuviae for chitin extraction and use of the obtained chitosan in the textile field. ACS Omega 6, 8884–8893. doi: 10.1021/acsomega.0c06040
Brandhorst, G., Oellerich, M., Maine, G., Taylor, P., Veen, G., and Wallemacq, P. (2012). Liquid chromatography–tandem mass spectrometry or automated immunoassays: what are the future trends in therapeutic drug monitoring? Clin. Chem. 58, 821–825. doi: 10.1373/clinchem.2011.167189
Brigode, C., Hobbi, P., Jafari, H., Verwilghen, F., Baeten, E., and Shavandi, A. (2020). Isolation and physicochemical properties of chitin polymer from insect farm side stream as a new source of renewable biopolymer. J. Clean. Prod. 275:122924. doi: 10.1016/j.jclepro.2020.122924
Choi, B. D., Wong, N. A. K., and Auh, J.-H. (2017). Defatting and sonication enhances protein extraction from edible insects. Korean J. Food Sci. Anim. Resour. 37, 955–961. doi: 10.5851/kosfa.2017.37.6.955
Cohen, E. (1993). Chitin synthesis and degradation as targets for pesticide action. Arch. Insect Biochem. Physiol. 22, 245–261. doi: 10.1002/arch.940220118
Crespo, M. O. P., Martínez, M. V., Hernández, J. L., and Lage Yusty, M. A. (2006). High-performance liquid chromatographic determination of chitin in the snow crab, Chionoecetes opilio. J. Chromatogr. A 1116, 189–192. doi: 10.1016/j.chroma.2006.03.068
D'Hondt, E., Soetemans, L., Bastiaens, L., Maesen, M., Jespers, V., van den Bosch, B., et al. (2020). Simplified determination of the content and average degree of acetylation of chitin in crude black soldier fly larvae samples. Carbohydr. Res. 488:107899. doi: 10.1016/j.carres.2019.107899
Dossey, A. T., Tatum, J. T., and McGill, W. L. (2016). “Chapter 5 - Modern insect-based food industry: current status, insect processing technology, and recommendations moving forward,” in Insects as Sustainable Food Ingredients, eds A. T. Dossey, J. A. Morales-Ramos, and M. G. Rojas (San Diego, CA: Academic Press), 113–152.
EFSA Panel on Nutrition N. F., Allergens, F., Turck, D., Castenmiller, J., De Henauw, S., Hirsch-Ernst, KI, et al. (2021). Safety of dried yellow mealworm (Tenebrio molitor larva) as a novel food pursuant to Regulation (EU) 2015/2283. EFSA J. 19:e06343. doi: 10.2903/j.efsa.2021.6343
Einbu, A., and Vårum, K. M. (2008). Characterization of chitin and its hydrolysis to GlcNAc and GlcN. Biomacromolecules 9, 1870–1875. doi: 10.1021/bm8001123
EU (2017). Commission regulation (EU) 2017/893 of 24 May 2017. Off. J. Eur. Union L 138/92. p. 2–25. Available online at: https://eur-lex.europa.eu/legal-content/EN/TXT/PDF/?uri=CELEX:32017R0893&from=EN (accessed August 3, 2021).
Gołebiowski, M., Urbanek, A., Oleszczak, A., Dawgul, M., Kamysz, W., Boguś, M. I., et al. (2014). The antifungal activity of fatty acids of all stages of Sarcophaga carnaria L. (Diptera: Sarcophagidae). Microbiol. Res. 169, 279–286. doi: 10.1016/j.micres.2013.07.011
Hackman, R. (1962). Studies on chitin V: the action of mineral acids on chitin. Aust. J. Biol. Sci. 15, 526–537. doi: 10.1071/BI9620526
Hahn, T., Roth, A., Ji, R., Schmitt, E., and Zibek, S. (2020a). Chitosan production with larval exoskeletons derived from the insect protein production. J. Biotechnol. 310, 62–67. doi: 10.1016/j.jbiotec.2019.12.015
Hahn, T., Tafi, E., Paul, A., Salvia, R., Falabella, P., and Zibek, S. (2020b). Current state of chitin purification and chitosan production from insects. J. Chem. Technol. 95, 2775–2795. doi: 10.1002/jctb.6533
Han, X., and Heinonen, M. (2021). Development of ultra-high performance liquid chromatographic and fluorescent method for the analysis of insect chitin. Food Chem. 334:127577. doi: 10.1016/j.foodchem.2020.127577
Harvey, D. J. (2011). Derivatization of carbohydrates for analysis by chromatography; electrophoresis and mass spectrometry. J. Chromatogr. B 879, 1196–1225. doi: 10.1016/j.jchromb.2010.11.010
Hubert Ribeiro, T. M., and dos Santos, M. C. (2019). “Chitin adsorbents to wastewater treatment,” in Nanomaterials For Eco-friendly Applications, ed A. Kopp Alves (Cham: Springer International Publishing), 131–140.
Jantzen da Silva Lucas, A., Quadro Oreste, E., Leão Gouveia Costa, H., Martín López, H., Dias Medeiros Saad, C., and Prentice, C. (2021). Extraction, physicochemical characterization, and morphological properties of chitin and chitosan from cuticles of edible insects. Food Chem. 343:128550. doi: 10.1016/j.foodchem.2020.128550
Jardine, A., and Sayed, S. (2018). Valorisation of chitinous biomass for antimicrobial applications. Pure Appl. Chem. 90, 293–304. doi: 10.1515/pac-2017-0707
Kaya, M., Baublys, V., Šatkauskiene, I., Akyuz, B., Bulut, E., and Tubelyte, V. (2015). First chitin extraction from Plumatella repens (Bryozoa) with comparison to chitins of insect and fungal origin. Int. J. Biol. Macromol. 79, 126–132. doi: 10.1016/j.ijbiomac.2015.04.066
Kim, M.-W., Song, Y.-S., Han, Y. S., Jo, Y. H., Choi, M. H., Park, Y.-K., et al. (2017). Production of chitin and chitosan from the exoskeleton of adult two-spotted field crickets (Gryllus bimaculatus). Entomol. Res. 47, 279–285. doi: 10.1111/1748-5967.12239
Kramer, K. J., Hopkins, T. L., and Schaefer, J. (1995). Applications of solids NMR to the analysis of insect sclerotized structures. Insect Biochem. Mol. Biol. 25, 1067–1080. doi: 10.1016/0965-1748(95)00053-4
Kröncke, N., Baur, A., Böschen, V., Demtröder, S., Benning, R., and Delgado, A. (2020). “Automation of insect mass rearing and processing technologies of mealworms (Tenebrio molitor),” in African Edible Insects as Alternative Source of Food, Oil, Protein, and Bioactive Components, ed A. Adam Mariod (Cham: Springer International Publishing), 123–139.
Liu, S., Sun, J., Yu, L., Zhang, C., Bi, J., Zhu, F., et al. (2012). Extraction and characterization of chitin from the beetle Holotrichia parallela Motschulsky. Molecules 17, 4604–4611. doi: 10.3390/molecules17044604
Marei, N. H., El-Samie, E. A., Salah, T., Saad, G. R., and Elwahy, A. H. M. (2016). Isolation and characterization of chitosan from different local insects in Egypt. Int. J. Biol. Macromol. 82, 871–877. doi: 10.1016/j.ijbiomac.2015.10.024
Masuda, T., Kitahara, K. I., Aikawa, Y., and Arai, S. (2002). Determination of carbohydrates by HPLC-ECD with a novel stationary phase prepared from polystyrene-based resin and tertiary amines. Anal. Sci. 17icas, i895–i898. doi: 10.14891/analscisp.17icas.0.i895.0
Mohan, K., Ganesan, A. R., Muralisankar, T., Jayakumar, R., Sathishkumar, P., Uthayakumar, V., et al. (2020). Recent insights into the extraction, characterization, and bioactivities of chitin and chitosan from insects. Trends Food Sci. Technol. 105, 17–42. doi: 10.1016/j.tifs.2020.08.016
Mol, A., Kaya, M., Mujtaba, M., and Akyuz, B. (2018). Extraction of high thermally stable and nanofibrous chitin from cicada (Cicadoidea). Entomol. Res. 48, 480–489. doi: 10.1111/1748-5967.12299
Muzzarelli, R. A. A. (2011). “Chitin nanostructures in living organisms,” in Chitin: Formation and Diagenesis, ed N. S. Gupta (Dordrecht: Springer Netherlands), 1–34.
Olofsson, M. A., and Bylund, D. (2016). Liquid chromatography with electrospray ionization and tandem mass spectrometry applied in the quantitative analysis of chitin-derived glucosamine for a rapid estimation of fungal biomass in soil. Int. J. Anal. Chem. 2016, 9269357–9269357. doi: 10.1155/2016/9269357
Pashkova, E., Pirogov, A., Bendryshev, A., Ivanaynen, E., and Shpigun, O. (2009). Determination of underivatized glucosamine in human plasma by high-performance liquid chromatography with electrochemical detection: application to pharmacokinetic study. J. Pharm. Biomed. 50, 671–674. doi: 10.1016/j.jpba.2009.01.031
Pasquier, E., Beaumont, M., Mattos, B. D., Otoni, C. G., Winter, A., Rosenau, T., et al. (2021). Upcycling byproducts from insect (fly larvae and mealworm) farming into chitin nanofibers and films. ACS Sustain. Chem. Eng. 9:13618. doi: 10.1021/acssuschemeng.1c05035
Poerio, A., Petit, C., Jehl, J.-P., Arab-Tehrany, E., Mano, J. F., and Cleymand, F. (2020). Extraction and physicochemical characterization of chitin from Cicada orni sloughs of the south-eastern French mediterranean basin. Molecules 25:2543. doi: 10.3390/molecules25112543
Poveda, J., Jiménez-Gómez, A., Saati-Santamaría, Z., Usategui-Martín, R., Rivas, R., and García-Fraile, P. (2019). Mealworm frass as a potential biofertilizer and abiotic stress tolerance-inductor in plants. Appl. Soil Ecol. 142, 110–122. doi: 10.1016/j.apsoil.2019.04.016
Purkayastha, D., and Sarkar, S. (2020). Physicochemical structure analysis of chitin extracted from pupa exuviae and dead imago of wild black soldier fly (Hermetia illucens). J. Polym. Environ. 28, 445–457. doi: 10.1007/s10924-019-01620-x
Roberts, G. A. F. (ed). (1992). “Structure of chitin and chitosan,” in Chitin Chemistry (London: Macmillan Education UK), 1–53.
Roer, R., Abehsera, S., and Sagi, A. (2015). Exoskeletons across the pancrustacea: comparative morphology, physiology, biochemistry and genetics. Integr. Comp. Biol. 55, 771–791. doi: 10.1093/icb/icv080
Sagheer, F. A. A., Al-Sughayer, M. A., Muslim, S., and Elsabee, M. Z. (2009). Extraction and characterization of chitin and chitosan from marine sources in Arabian Gulf. Carbohydr. Polym. 77, 410–419. doi: 10.1016/j.carbpol.2009.01.032
Silva, S. S., Rodrigues, L. C., Fernandes, E. M., and Reis, R. L. (2020). “Chapter 1–Fundamentals on biopolymers and global demand,” in Biopolymer Membranes and Films, eds M. A. De Moraes, C. F. Da Silva, and R. S. Vieira (Amsterdam: Elsevier), 3–34.
Smets, R., and van der Borght, M. (2021). Enhancing the specificity of chitin determinations through glucosamine analysis via ultra-performance LC-MS. Anal. Bioanal. Chem. 413, 3119–3130. doi: 10.1007/s00216-021-03252-4
Soetemans, L., Uyttebroek, M., and Bastiaens, L. (2020). Characteristics of chitin extracted from black soldier fly in different life stages. Int. J. Biol. Macromol. 165, 3206–3214. doi: 10.1016/j.ijbiomac.2020.11.041
Song, Y.-S., Kim, M.-W., Moon, C., Seo, D.-J., Han, Y. S., Jo, Y. H., et al. (2018). Extraction of chitin and chitosan from larval exuvium and whole body of edible mealworm, Tenebrio molitor. Entomol. Res. 48, 227–233. doi: 10.1111/1748-5967.12304
Soon, C. Y., Tee, Y. B., Tan, C. H., Rosnita, A. T., and Khalina, A. (2018). Extraction and physicochemical characterization of chitin and chitosan from Zophobas morio larvae in varying sodium hydroxide concentration. Int. J. Biol. Macromol. 108, 135–142. doi: 10.1016/j.ijbiomac.2017.11.138
Suderman, R. J., Dittmer, N. T., Kanost, M. R., and Kramer, K. J. (2006). Model reactions for insect cuticle sclerotization: cross-linking of recombinant cuticular proteins upon their laccase-catalyzed oxidative conjugation with catechols. Insect Biochem. Mol. Biol. 36, 353–365. doi: 10.1016/j.ibmb.2006.01.012
Tan, Y. N., Chin, Y. L., and Chen, W. N. (2021). Comparison of sustainable lipid and protein removal methods for the isolation of insect chitin from black soldier fly exoskeleton. ACS Food Sci. Technol. 1, 698–706. doi: 10.1021/acsfoodscitech.0c00104
Tolaimate, A., Desbrieres, J., Rhazi, M., and Alagui, A. (2003). Contribution to the preparation of chitins and chitosans with controlled physico-chemical properties. Polymer 44, 7939–7952. doi: 10.1016/j.polymer.2003.10.025
Ushakova, N. A., Dontsov, A. E., Bastrakov, A. I., Garmash, N. Y., and Pavlov, D. S. (2017). Paramagnetics melanin and Mn2+ in black soldier fly Hermetia illucens. Dokl. Biochem. Biophys. 473, 102–105. doi: 10.1134/S1607672917020041
van Huis, A. (2021). Prospects of insects as food and feed. Org. Agric. 11, 301–308. doi: 10.1007/s13165-020-00290-7
Wang, H., Rehman, K., u. Feng, W., Yang, D., Rehman, R. u., Cai, M., et al. (2020). Physicochemical structure of chitin in the developing stages of black soldier fly. Int. J. Biol. Macromol. 149, 901–907. doi: 10.1016/j.ijbiomac.2020.01.293
Wang, X., Chen, X., Chen, L., Wang, B., Peng, C., He, C., et al. (2008). Optimizing high-performance liquid chromatography method for quantification of glucosamine using 6-aminoquinolyl-N-hydroxysuccinimidyl carbamate derivatization in rat plasma: application to a pharmacokinetic study. Biomed. Chromatogr. 22, 1265–1271. doi: 10.1002/bmc.1056
Waśko, A., Bulak, P., Polak-Berecka, M., Nowak, K., Polakowski, C., and Bieganowski, A. (2016). The first report of the physicochemical structure of chitin isolated from Hermetia illucens. Int. J. Biol. Macromol. 92, 316–320. doi: 10.1016/j.ijbiomac.2016.07.038
Wynants, E., Crauwels, S., Verreth, C., Gianotten, N., Lievens, B., Claes, J., et al. (2018). Microbial dynamics during production of lesser mealworms (Alphitobius diaperinus) for human consumption at industrial scale. Food Microbiol. 70, 181–191. doi: 10.1016/j.fm.2017.09.012
Yang, T.-L. (2011). Chitin-based materials in tissue engineering: applications in soft tissue and epithelial organ. Int. J. Mol. Sci. 12, 1936–1963. doi: 10.3390/ijms12031936
Zainol Abidin, N. A., Kormin, F., Zainol Abidin, N. A., Mohamed Anuar, N. A. F., and Abu Bakar, M. F. (2020). The potential of insects as alternative sources of chitin: an overview on the chemical method of extraction from various sources. Int. J. Mol. Sci. 21:4978. doi: 10.3390/ijms21144978
Zhou, J. Z., Waszkuc, T., and Mohammed, F. (2005). Determination of glucosamine in raw materials and dietary supplements containing glucosamine sulfate and/or glucosamine hydrochloride by high-performance liquid chromatography with FMOC-Su derivatization: collaborative study. J. AOAC Int. 88, 1048–1058. doi: 10.1093/jaoac/88.4.1048
Zhou, P., Li, J., Yan, T., Wang, X., Huang, J., Kuang, Z., et al. (2019). Selectivity of deproteinization and demineralization using natural deep eutectic solvents for production of insect chitin (Hermetia illucens). Carbohydr. Polym. 225:115255. doi: 10.1016/j.carbpol.2019.115255
Keywords: exuviae, insect farming, chitin extraction, glucosamine, LC-ECD, LC-MS/MS
Citation: Nurfikari A and de Boer W (2021) Chitin Determination in Residual Streams Derived From Insect Production by LC-ECD and LC-MS/MS Methods. Front. Sustain. Food Syst. 5:795694. doi: 10.3389/fsufs.2021.795694
Received: 15 October 2021; Accepted: 14 November 2021;
Published: 06 December 2021.
Edited by:
Florian Wichern, Rhine-Waal University of Applied Sciences, GermanyReviewed by:
Mik Van Der Borght, KU Leuven, BelgiumGuadalupe Virginia Nevárez-Moorillón, Autonomous University of Chihuahua, Mexico
Copyright © 2021 Nurfikari and de Boer. This is an open-access article distributed under the terms of the Creative Commons Attribution License (CC BY). The use, distribution or reproduction in other forums is permitted, provided the original author(s) and the copyright owner(s) are credited and that the original publication in this journal is cited, in accordance with accepted academic practice. No use, distribution or reproduction is permitted which does not comply with these terms.
*Correspondence: Azkia Nurfikari, YS5udXJmaUBuaW9vLmtuYXcubmw=