- 1Institute of Food Science and Technology, Chinese Academy of Agricultural Sciences, Beijing, China
- 2China Oil and Foodstuffs Corporation Nutrition & Health Research Institute, Beijing, China
- 3Department of Food Science, University of Otago, Dunedin, New Zealand
- 4Faculty of Health Science, School of Nutrition Sciences, University of Ottawa, Ottawa, ON, Canada
- 5School of Science, Royal Melbourne Institute of Technology University, Melbourne, VIC, Australia
- 6School of Behavioural and Health Sciences, Australian Catholic University, North Sydney, NSW, Australia
Due to the rapid growth in the global population, the consumption of animal-based food products/food compounds has been associated with negative implications for food sustainability/security. As a result, there is an increasing demand for the development of plant-based food and compounds as alternatives. Meanwhile, a growing number of studies report the health benefits of food protein-based peptides prepared via enzymatic hydrolysis and exhibiting biological properties such as antioxidant, antihypertensive, anti-thrombotic, and antidiabetic activities. However, the inherent bitterness of some peptides hinders their application in food products as ingredients. This article aims to provide the latest findings on plant-based bioactive peptides, particularly their health benefits, manufacturing methods, detection and qualification of their bitterness properties, as well as debittering methods to reduce or eliminate this negative sensory characteristic. However, there is still a paucity of research on the biological property of debittered peptides. Therefore, the role of plant protein-derived bioactive peptides to meet the health targets of the Sustainable Development Goals can only be realised if advances are made in the industrial-scale bioprocessing and debittering of these peptides.
Introduction
According to the data from World Population Prospects (2019 revision), United Nations (2019) estimates a dramatic global population growth from 7.7 to 9.7 billion from 2019 to 2050, with the population over the age of 65 increasing from 9 to 16%. Not only this population growth prospects is raising questions on demand for food quantity, but it is also initiating discussions and debates on the sustainability of systems used in the current agri-food sector. In this context, some negative impacts on the environment through the manufacturing of animal-based food materials by the agricultural sector have been reported. Some of these impacts include the formation of a large number of greenhouse gases, farm land conversion (i.e., from forests, wetlands, and grasslands) and poor biodiversity, etc.
Unfortunately, at the same time, the contemporary “downstream” food industry is also generating a huge number of by-products and wastes. The disposal of these by-products could cause a huge negative impact on the environment. However, some of these by-products can be potentially used to develop novel food ingredients with health benefits. For instance, defatted seeds, which are considered “by-products” by the oil industry, are rich in proteins. A Food and Agriculture Organization of the United Nations (2012) report suggested that the annual volume of defatted meals of major oilseeds (i.e., soybean, rapeseed, cottonseed, sunflower seed, flaxseed, and peanut) was approximately 200 million tonnes, and these defatted meals contain up to 50% (w/w) protein. On the other hand, defatted cereal (e.g., rice bran, corn and wheat germ etc.) and vegetables (e.g., olive, shea butter cake and palm kernel cake etc.), which are also produced in large quantities annually, are promising sources of proteins (Zarei et al., 2012; Abdul-Mumeen et al., 2013; Rahman et al., 2013; Meshginfar et al., 2019; Görgüç et al., 2020). Therefore, there is an increasing demand in developing new food products and ingredients from these plant-based materials to improve the sustainability of food production processes and reduce the environmental footprint of food waste disposal.
Biopeptides and Fabrication Process
Bioactive peptides (or biopeptides) are part of proteins, with the structure of 2–20 amino acid residues linked by peptide bonds and a molecular weight below 6 kDa (Sarmadi and Ismail, 2010; Chalamaiah et al., 2018, 2019). Recently, the biological activities of food protein-derived biopeptides have been drawing interest from both research and industrial sectors. Compared with physical mixtures of amino acids at an equivalent amount, peptides with short chains, particularly di- and tripeptides, are more readily absorbed by the human body due to (1) the presence of specific transport systems for peptides; (2) the less hypertonic nature of peptides, eliminating problems with osmosis, and (3) improved stability and or solubility of peptides (Parrado et al., 1991; Siemensm et al., 1993; Clemente, 2001; Kang et al., 2012). To date, the most commonly used methods to produce biopeptides from foods are microbial fermentation of food products, and enzymatic hydrolysis of food proteins (Lee and Hur, 2017).
Microbial Fermentation to Produce Biopeptides
Bacteria or yeast are involved in microbial fermentation of the protein-containing food to produce biopeptides. Briefly, these microorganisms secret proteolytic enzymes during their growth so that food proteins are hydrolysed into biopeptides. The production of biopeptide via microbial fermentation is straightforward. Firstly, the selected microorganism is grown into its exponential phase in the growing media at the optimum conditions. Subsequently, these microorganisms are harvested, washed, suspended in sterile media and added into the target food material as a starter to induce the fermentation and the production of biopeptides (Daliri et al., 2016; Aguilar-Toalá et al., 2017). During the fermentation process, the type of microorganism, nature of the food matrix, and fermentation conditions significantly affect the outcomes (i.e., the types and quantities of peptides released).
To date, although dairy products are still the main source of proteins for generating biopeptides using the fermentation method (Wang et al., 2015; Najafian and Babji, 2018; Worsztynowicz et al., 2020; Yu et al., 2020), the preparation and characterisation of plant-based biopeptides are drawing significant research interests (see Table 1). For instance, Xiao et al. (2018) fermented red bean [Phaseolus angularis (Willd.) W. F. Wight.] using Cordyceps militaris (L.) Fr to release small peptides. The authors observed significant inhibitory effects of the fermented bean on angiotensin I converting enzyme (ACE), with an IC50 value of 0.63 mg/mL. As reported by Wu et al. (2018), whole-grain oats were fermented by Lactobacillus plantarum B1-6, Rhizopus oryzae, or their combination for 72 h, resulting in an increased degree of hydrolysis when both microorganisms were applied. In addition, the fermented oats had a higher ACE inhibitory activity, with an IC50 value of 0.42 mg/mL. Enhancement of bioactivity during fermentation has been associated with degradation of the rigid cell wall of plants by microbial enzymes, resulting in enhanced protein digestibility and biopeptide release (Di Stefano et al., 2019).
Enzymatic Hydrolysis to Produce Biopeptides
Compared with the fermentation method, the hydrolysis of food proteins using enzymes to produce biopeptides has the advantages of time effectiveness, ease in scaling up, and better predictability. The general flow diagram of biopeptide preparation using this approach is shown in Figure 1. Essentially, the first step involves extraction of food proteins from the food to avoid interference of other non-protein components (Görgüç et al., 2020). For instance, enzyme activity can be significantly inhibited by phenolic compounds (Cirkovic Velickovic and Stanic-Vucinic, 2018). Subsequently, these extracted proteins are hydrolysed using the selected enzyme(s) at controlled temperature and pH for a certain period (He et al., 2016; Ou and Peng, 2016). Similar to the release of biopeptide using the fermentation method, in this enzymatic hydrolysis process, the selected enzyme(s), nature of food proteins, and environmental conditions during the hydrolysis significantly affect the outcomes. In a study by Zhang et al. (2012), rice bran protein was hydrolysed using peptidases, Alcalase, Neutrase, papaya latex papain or porcine pancreas trypsin at 25–50°C and pH 7.0–8.5 depending on the nature of the enzyme. The result showed the Alcalase hydrolysis process produced hydrolysates with the highest degree of hydrolysis. The subsequent in vitro test result confirmed a high micellar cholesterol inhibition ability of the released biopeptides. To date, although the association between specific proteolytic enzymes and the release of specific peptides in food proteins has not been completely understood, it is well-accepted that the biopeptides with low molecular weight are more bioactive than ones with high molecular weight (Ruiz-Ruiz et al., 2013; García-Tejedor et al., 2014). The third step is usually the termination of protein hydrolysis by inactivating the enzyme(s), followed by fractionation to separate hydrolysates or peptides from the reaction mixture (containing residual unhydrolysed proteins and buffer components). This separation process is usually performed using desalting and membrane-filtration technologies. Finally, the peptides can be recovered using freeze-drying technique, followed by characterisation of their physicochemical and biological properties.
In their study, Ferri et al. (2017) digested rice byproducts with five commercial proteolytic enzymes. The resulting digesta was then fractionated into four molecular weights using cross-flow membrane filtration techniques. This allowed bioactivity characterisation such as anti-tyrosinase, anti-inflammatory, cytotoxicity, irritation capacity, antioxidant, and anti-hypertensive activities to be performed. Similarly, in their studies, Nimalaratne et al. (2015) and Zhang et al. (2016) used various proteases to hydrolyse chicken egg white protein and Pseudosciaena crocea muscle, respectively. The hydrolysates were separated using desalting and ultrafiltration methods before the permeate was freeze-dried and characterised. It is worth noting that in both studies, reverse-phase high-performance liquid chromatography (RP-HPLC) was used to purify the peptide fraction further, but this was more for analytical purposes.
Bioactivities and/or Health Benefits of Biopeptides
Generally, a wide range of bioactivities or health benefits has been reported for biopeptides, such as antioxidant, antimicrobial, anticancer, hypocholesterolemic, antihypertensive, immunomodulatory and opioid-like activities, etc. These bioactivities are significantly affected by the nature of the peptide, i.e., the type of amino acid, their sequence, and molecular weight, etc.
Antioxidant Activity
To date, many clinical trials have reported the correlation between the oxidative stress caused by reactive oxygen species (ROS) and the development of various chronic diseases including rheumatoid arthritis, diabetes, inflammation and even cancer (Sayin et al., 2014; Ibrahim et al., 2018). Therefore, the intake of a diet rich in antioxidants is well-recommended by clinicians, dietitians and health organisations. Meanwhile, food ingredients with antioxidant activity are also desired by the food industry, because these foods can delay and/or prevent oxidative deterioration of macromolecules in food product matrices, such as proteins and lipids, to improve the quality and shelf life of the food (Nwachukwu and Aluko, 2019).
Depending on the composition, structure, and hydrophobicity, some biopeptides show antioxidant properties to varying extents. For example, among 20 amino acids, Xu et al. (2017) observed stronger antioxidant activity in tryptophan, methionine, histidine, lysine, cysteine, arginine and tyrosine, than in others. Generally, biopeptides with antioxidant activities usually have short chains made from about 4 to 16 amino acids and low molecular weight in the range of 0.4–2 kDa.
The exact mechanism of antioxidant activities of these biopeptides is a continuing research endeavour. These peptides have been reported and used in different settings, such as inhibiting lipid peroxidation, scavenging free radicals, and chelating transition metal ions via various mechanisms (Wu et al., 2003; Rajapakse et al., 2005; Moure et al., 2006). For instance, in their studies, Ajibola et al. (2011) and Esfandi et al. (2019a,b) suggested that the scavenging of free radicals happens via hydrogen atom and single electron transfer by the tyrosine- and cysteine-containing biopeptides, respectively. In other studies, the histidine-containing biopeptides acted as antioxidants via the donation of hydrogen atom, entrapment of lipid peroxyl radical, and the chelating of metal ions by the imidazole group (Khan et al., 2014; Walters et al., 2018). Sarmadi and Ismail (2010) reported the antioxidant activity of cysteine due to sulfhydryl (-SH) groups' reacting with free radicals to form the disulfide bond (-SS-).
Some plant-based biopeptides with antioxidant properties are shown in Table 2. Other examples are given below. For instance, Megías et al. (2008) hydrolysed sunflower protein using pepsin and pancreatin and the hydrolysate exhibited significant copper-chelating properties due to the presence of histidine and arginine in the peptide sequence. Similarly, in a study by Girgih et al. (2014), hemp protein isolate was hydrolysed using pepsin, followed by pancreatin to generate biopeptides. Subsequently, the sequences of 23 peptides were identified in this protein hydrolysate and the subsequent in vitro and in vivo tests indicated the superior antioxidant properties of WVYY and PSLPA biopeptides. This indicates that gastrointestinal proteases can potentially generate antioxidant biopeptides during digestion of dietary proteins. Furthermore, Supawong et al. (2018) used Protease G6 to hydrolyse rice bran protein and the resultant biopeptides exhibited significant antioxidant activity. Subsequently, these peptides were incorporated into the fried fish cake as an example application. The authors reported that 2% (w/w) rice bran hydrolysate addition was able to reduce lipid oxidation by 79.8%, which was as equally effective as adding 0.02% (w/w) butylated hydroxyanisole/butylated hydroxytoluene (BHA/BHT). This demonstrates the potential of using biopeptides to preserve the quality of food products.
Antimicrobial Activity
The incorporation of food ingredients with antimicrobial activity can help prolong the shelf life of the food product, as well as retain food quality during storage (Lucera et al., 2012). For this purpose, the mechanism and application of various natural antimicrobial compounds, such as essential oils from plants (e.g., basil, clove, and rosemary), enzymes from animals (e.g., lysozyme and lactoferrin), short-chain organic acids (e.g., acetic and citric acid), and biopolymers (e.g., chitosan) have been investigated and explored.
Some peptides exhibit antimicrobial activity due to their unique structural features such as having unusually long chains (with 20–46 amino acid subunits), the presence of basic groups such as lysine or arginine, and an amphipathic nature (Toldrá et al., 2018; Ahmed and Hammami, 2019). Generally, many antimicrobial peptides contain cationic amino acids and they usually have a high content of some particular hydrophobic residues such as leucine, isoleucine, valine, phenylalanine and tryptophan (Fjell et al., 2012). These biopeptides can form channels and/or pores on the surface of microbial membranes, leading to membrane disruption and even cell division (Yadavalli et al., 2016; Toldrá et al., 2018). The mechanism of antimicrobial activity of biopeptides was reported by Fjell et al. (2012); Barreto-Santamaría et al. (2020), and Li et al. (2021). Firstly, these biopeptides interact with oppositely charged groups on microbes' membrane surfaces. Subsequently, the biopeptides attach onto this surface (aided by electrostatic/hydrophobic interactions). Finally, the lipids of the microbe membrane are displaced, leading to the disruption of cell membranes.
The antimicrobial activity of various plant-based biopeptides have been reported, including peptide hydrolysate of chia flour (Salvia hispanica L.) (Segura-Campos et al., 2013), black pepper peptides with GTCVLVL, SSVVGRL, and ALGTLLL residuals (Umadevi et al., 2018), and soybean peptides containing PGTAVFL and ILAFLEATLVDLVVVLWTA residuals (Dhayakaran et al., 2016). In their study, Pu and Tang (2017) purified an antilisterial peptide (Alpep7) from the bromelain hydrolysate of rice bran proteins and identified LVDHFPL residual as being responsible for this antimicrobial activity. Furthermore, a liposome system was successfully developed to deliver this biopeptide to the listerial biofilm to confirm its listericidal activity. Similarly, Xiao and Zhang (2012) digested Jatropha curcas meal using multiple enzymes (including pepsin, trypsin, Protamex, Neutrase, Flavourzyme, papain, Alcalase, or acid protease) into hydrolysates with different degrees of hydrolysis and the authors evaluated the antibacterial activity of the hydrolysates. Based on the peptide sequence analysis, the CAILTHLR peptide was found to be responsible for the inhibitory effect against various microbes, including Escherichia coli ATCC 25922, Shigella dysenteriae ATCC 51302, Pseudomonas aeruginosa ATCC 27553, Staphylococcus aureus ATCC 25923, Bacillus subtilis ATCC 23631, and Streptococcus pneumoniae ATCC 49619. The minimum inhibitory concentrations were in the range of 29–68 μg/mL.
Anticancer Activity
To date, many synthetic anticancer drugs show a wide range of side effects, such as nephrotoxic, neurotoxic, cardiotoxic and gonadotoxic effects (Oun et al., 2013; Ahar et al., 2014; Kamisli et al., 2015; Gutierrez et al., 2016; Van Acker et al., 2016). Therefore, biopeptides with anticancer activity are promising alternatives to prevent and/or decelerate cancer (Daliri et al., 2017). Some examples of plant-based biopeptides with anticancer activity are shown in Table 3. In a review by Chalamaiah et al. (2018), different mechanisms of anticancer activity of these peptides were reported. Some of the mechanisms included inducing cancer cell membrane damage, adhering to cell and inhibiting topoisomerases, modulating immune response, and/or inhibiting intracellular signalling of cancer cells.
Xue et al. (2015) reported that chickpea protein-derived peptide RQSHFANAQP dramatically increased p53 protein level, thereby inhibiting the proliferation of the MCF-7 and MDA-MB-231 breast cancer cells at the EC50 values of 2.38 and 1.50 μmol/mL, respectively. In another study, based on a 2-day fermentation of rapeseed meal using Bacillus subtilis and Actinomucor elegans, the released biopeptides significantly inhibited the proliferation of human HepG2 liver cancer and MCF-7 breast cancer cells (Xie et al., 2015).
Among the plant-based biopeptides with anticancer benefits, those obtained from soybean proteins have been attracting particular research interests (Badger et al., 2005; Hwang et al., 2011). In a study by Rayaprolu et al. (2013), high oleic acid soybean protein from N98-4445A and S03-543CR lines was hydrolysed using Alcalase and the released biopeptides inhibited the proliferation of colon, liver and lung cancer cells. Moreover, anticancer activity has been observed in several other biopeptides, such as lunasin (a peptide found in soy), RLQLQGVN, GLTSL, LSGNL, GEGSGA, MPACGSS, and MTEEY peptides (Vital et al., 2014; Fernández-Tomé et al., 2017). Lunasin is a peptide with 43 amino acid residues and a molecular weight of 5 kDa (Udenigwe and Aluko, 2012; Rizzello et al., 2016). Within this peptide, the RGD residue and polyaspartic acid chain with nine aspartic acid residues have been reported as being responsible for its anticarcinogenic activities (Dia and de Mejia, 2011). In an in vitro study by Lumen (2005), lunasin showed the ability to enter mammalian cells within a few minutes when orally ingested, followed by localisation in the nucleus to inhibit histone acetylation and prevent skin cancers. Similarly, in vitro and in vivo studies have confirmed the inhibitory effect of lunasin on murine Lewis lung carcinoma (LLC) and B16-F0 melanoma cells, but do not affect the growth rate of normal cell lines.
Hypocholesterolemic Activity
Cholesterol levels of around 50 mg/dL in the serum are required by the human body to maintain normal functions (Steinberg and Witztum, 2009). However, excessive amounts of cholesterol in the blood may result in the formation of plaques in the arteries, resulting in cardiovascular diseases (Daliri et al., 2017). Although many synthetic drugs have been developed to lower blood cholesterol, side effects including liver injury or failure, myopathy and diabetes have been reported from their consumption (Carter et al., 2013; Mancini et al., 2016). Therefore, there is an increasing demand in sourcing natural and food-based alternatives of these drugs.
Hypocholesterolemic activity has been observed in some plant-based biopeptides, including ones from rice bran (Zhang et al., 2012), cowpea (Marques et al., 2015; Hernandez and de Mejia, 2017), cumin seed (Siow et al., 2016), and soy (Duranti et al., 2004; Lammi et al., 2015). Recently, Coelho et al. (2018) hydrolysed chia seed protein into biopeptides using Alcalase and Flavourzyme. After purification via ultrafiltration, the separated biopeptides with low molecular weight (<3 kDa) showed a significant inhibitory effect on cholesterol synthesis in vitro, by reducing the enzymatic reaction velocity of 3-hydroxy-3-methylglutaryl coenzyme A (HMG-CoA) reductase by up to 80.7%. Similarly, Lammi et al. (2016) reported that lupin biopeptides produced by enzymatic hydrolysis significantly inhibited the ability of HepG2 cells to secrete mature proprotein convertase (PC) subtilisin/kexintype 9 (PCSK9) in patients with moderate hypercholesterolaemia.
Some soy protein hydrolysate or biopeptides also exhibit hypocholesterolemic activities. For example, Lammi et al. (2015) treated HepG2 cells with three peptides separated from hydrolysed soy glycinin. The peptides were IAVPGEVA, IAVPTGVA and LPYP. The result showed that these three biopeptides significantly affected the catalytic activities of HMG-CoA reductase. Moreover, the cholesterol metabolism was modulated by the three peptides based on the activation of low-density lipoprotein receptor-sterol regulatory element-binding protein 2 (LDLR-SREBP2) pathway, finally promoting cellular uptake of low-density lipoprotein by the cultured hepatocytes. In an in vitro study by Pak et al. (2005), the hypocholesterolaemic activity of soy 11S globulin peptide was observed based on the binding of Bile acid. This biopeptide has the amino acid sequence of IAPGEVA, and a molecular weight of 755.2 Da. After bile acid was bound with peptide, it could not be reabsorbed through the enterohepatic circulation, which further stimulates the transformation of cholesterol into bile acids in the liver.
Antihypertensive Activity
The overall prevalence of hypertension (i.e., high blood pressure) in the global population is estimated to be 1.13 billion people in 2015 and it is expected to be approximately 1.56 billion by 2025 (Kearney et al., 2005; Mills et al., 2016; World Health Organisation, 2021). The correlations between hypertension and many serious non-communicable diseases have been well-established (Lee and Hur, 2017). Therefore, the demand for functional foods or food ingredients with antihypertensive activity is increasing (Bhat et al., 2017). Unfortunately, many synthetic antihypertensive drugs have multiple side effects, including dizziness, dysgeusia, headache, angioedema, and cough (Daliri et al., 2017).
In the renin-angiotensin-aldosterone system of the human body, angiotensin I-converting enzyme (ACE) catalyses the conversion of angiotensin I to angiotensin II, finally increasing blood pressure. Therefore, compounds that influence this system have been drawing much research attention. Interestingly, some peptides have shown ability to lower blood pressure by inhibiting the activity of ACE. Piovesana et al. (2018) reported that ACE-inhibiting biopeptides usually have 2–12 amino acid subunits in the chain. Particularly, they often contain acidic (Aspartic and Glutamic acid), positively charged (in particular, an alkyl group at the C-terminus) and hydrophobic amino acid subunits. This is also confirmed in the study by Wu et al. (2006), where the correlation of ACE-inhibiting activity and structure of 168 di- and 140 tripeptides were investigated.
In a study by Marambe et al. (2008), flaxseed proteins hydrolysed using Flavourzyme (0.67 mg/mL) and the hydrolysate showed strong ACE inhibitory activity at a low IC50 values of 0.07 mg/mL. Similarly, Wang et al. (2017) hydrolysed protein derived from rice bran using trypsin to obtain YSL peptide with a molecular weight of 395 Da, which exhibited strong ACE inhibitory activity at an IC50 value of 76 μM. Li and Aluko (2010) hydrolysed pea protein using Alcalase and the separated IR, LF and EF peptides showed significant inhibition of the activities of ACE and renin. These peptides had IC50 values below 25 mM. ACE inhibitory effects have been observed in biopeptides from soy (Wu and Ding, 2002), bamboo shoots (Liu et al., 2013) and cocoa beans (Sarmadi et al., 2011). Interestingly, Roy et al. (2010) suggested that the pea-based ACE inhibitory peptides were more resistant to digestion than the ones derived from milk.
Immunomodulatory Activity
The immune system of the human body is comprised of many biological structures and a healthy immune system that can identify and kill invading microorganisms (Yang et al., 2018). However, this vital system can be negatively affected by a wide range of factors including stress, unhealthy diet and lifestyle, and overwhelming presence of pathogens and/or antigens (Segerstrom and Miller, 2004). Although some drugs have been developed to modulate the human immune responses, they also have disadvantages like toxicity and high cost (Gertsch et al., 2011). This makes the modulation of the immune system via the intake of food-based compounds promising.
Immunomodulatory activities have been observed in some plant-based biopeptides, including the ones from soybean, wheat, yellow pea seed and rice (Morris et al., 2007; Egusa and Otani, 2009; Ndiaye et al., 2012; Hartati et al., 2017; Wu et al., 2017). Some examples of these biopeptides are shown in Table 4. Although the exact mechanism(s) of how biopeptide affects the immune system has not been fully understood, Chalamaiah et al. (2018) suggested that some peptides exhibit this bioactivity via activating macrophages, stimulating phagocytosis, increasing the amount of leukocytes, improving immune modulators (e.g., cytokines, nitric oxide, and immunoglobulins), stimulating natural killer cells, and enhancing the stimulation of splenocytes, CD4+, CD8+, CD11b +, and CD56 + cells.
Udenigwe et al. (2009) used pepsin, ficin and papain to hydrolyse flaxseed proteins, followed by releasing and separating biopeptides with a molecular weight below 1 kDa. These peptides showed significant inhibitory effects on the production of nitric oxide induced by lipopolysaccharide in RAW 264.7 macrophages. No cytotoxicity was observed. On the other hand, Kong et al. (2008) hydrolysed soy protein using various enzymes to produce low-molecular weight peptides (<1,000 Da), which improved lymphocyte proliferation and phagocytosis of peritoneal macrophages in mice. Interestingly, the authors observed the positive correlations between the immunomodulating activity and content of positively charged peptides.
Opioid-Like Activity
Opioids such as morphine have been used as drugs due to their pain modulation functions (Bagley and Ingram, 2020). However, their long-term use leads to the development of tolerance and opioid use disorder. Therefore, biopeptides with opioid-like activity can be good alternatives to these drugs. Toldrá et al. (2018) described that YGGF and YP residuals as the common motifs present in most opioid peptides. Based on the study in the fundamental signalling mechanisms of opioid receptors, it is generally accepted that the three types of opioid receptors (μ, δ, κ) are activated by endogenous peptides derived from three different precursors, namely proopiomelanocortin, proenkephalin, and prodynorphin (Liu and Udenigwe, 2019). Unlike the animal-based opioid peptides which bind to μ receptors, the plant-based ones usually interact with δ receptors, except for soymorphins (Yoshikawa et al., 2003).
To date, researchers have identified opioid peptides from various plant proteins, including wheat (gluten, gliadin, and glutenin), barley (hordein), maize (zein), oats (avenin), rye (secalin), soybean (soya α-protein and cytochrome b), and spinach (rubiscolin) (Kaur et al., 2020). Leo Pruimboom and de Punder (2015) overviewed the degradation of gluten in the gastrointestinal tract and suggested that gluten exorphins, a morphine-like substance can be released. Following this, in Garg's et al. (2018) study, high opioid activity was observed in the gluten peptides containing YPG, YYPG, and YIPP motifs. Similarly, Yang et al. (2001) demonstrated that YPLDL and YPLDLF peptides identified in pepsin-digested spinach D-ribulose-1,5-bisphosphate carboxylase/oxygenase (RuBisCo) showed opioid activity in mouse vas deferens (MVD) assay.
The Bitterness Property of Biopeptides
As discussed in the previous sections, food proteins can be transformed into peptides with desired functional properties and biological activities. However, the sensory characteristics of the biopeptides are also changed dramatically. From application perspective, it is still quite challenging to directly use biopeptides as novel functional food ingredients due to their bitterness property.
Formation of Bitterness in the Biopeptides
Generally, most food proteins are not bitter. However, after proteolysis using some enzymes, the resultant hydrolysates or peptides may have bitterness. This bitter taste is due to the release of hydrophobic amino residues, which are usually buried within the native protein structure (Acquah et al., 2018) but get exposed after hydrolysis of the protein. For example, phenylalanine, tyrosine, tryptophan, and leucine, which are usually buried within the molecule, have bitter tastes in nature. During the hydrolysis of the proteins, these hydrophobic amino acid residues are gradually exposed and their bitterness can be detected by human bitter taste receptors (Maehashi and Huang, 2009). Generally, the bitterness of biopeptides intensifies with increase in degree of hydrolysis, since more hydrophobic amino acids are released. However, bitterness decreases when the proteins are intensively hydrolysed into peptides with low molecular weight or into free amino acids (Adler-Nissen, 1986; Fu et al., 2018). When native food protein is hydrolysed into peptides, it has been reported that the peptide bitterness intensity usually increases with the increase of hydrolysis degree and decrease of molecular weight, until the hydrolysis gives peptides with seven or fewer residues (Kim and Li-Chan, 2006; Maehashi et al., 2008; Kohl et al., 2013). Cho et al. (2004) investigated the correlation between the bitterness of soy protein hydrolysate and peptide molecular weights and showed that the hydrolysate with the strongest bitterness had a molecular weight of 4 kDa, while the least bitter hydrolysate was the one with a molecular weight of below 1 kDa. Contradictory results have also been reported. Humiski and Aluko (2007) found that the bitterness in pea hydrolysates was not related in any way to molecular weight.
Quantification and Prediction of Bitterness in Biopeptides
In order to quantify the bitterness of biopeptides using their structures, Ney (1979) developed the Q-rule to illustrate and predict the bitterness of a peptide. Briefly, the average hydrophobicity, Q, is calculated by summing the amino acid side chain hydrophobicity of a peptide and dividing this value by the number of amino acid residues in the peptide. A mathematical representation of the Q value is as follows:
where Q is the average hydrophobicity of a peptide (cal/mol), Δf is the free energy of transfer of the side chains in the amino acid residues (hydrophobicity, cal/mol) and n is the number of amino acid residues (dimensionless).
According to this method, peptides with Q value over 1,400 cal/mol and molecular weights below 6 kDa are likely to be bitter, while those with Q-value below 1,300 cal/mol and molecular weights below 10 kDa should not be bitter. This principle was successful to a certain extent in interpreting the bitterness property in some peptides or hydrolysate from casein and soy (Murray et al., 2018; Iwaniak et al., 2020). However, there is an increasing number of studies confirming the correlation of amino acid position and their sequence and the bitterness property of peptides. For example, Kim et al. (2008) used computer simulation to study the structures of NALPE peptide and its 6 analogues. The results showed that the intensity of peptide bitterness could also be affected significantly by spatial orientation of hydrophobic regions in the structure, as well as proximity between polar groups and hydrophobic regions within the same plane space.
Mathematical models can be used to predict the bitterness of peptides. For instance, quantitative structure-activity relationship (QSAR) models were developed for this purpose, based on physicochemical properties of peptides, such as electronic charge, hydrophobicity and steric properties (Iwaniak et al., 2015; Agyei et al., 2016). Furthermore, Yin et al. (2010) introduced a descriptor, E, to the QSAR model, based on the multidimensional scaling of 237 physicochemical properties of the natural amino acid side chains. This improved the model's success in predicting the bitterness of 48 dipeptides (R2 = 0.97). Kim and Li-Chan (2006) used a database of 224 di- to tetradecapeptides and five amino acids to investigate the correlation between peptide structure and bitterness. The results showed that bulky hydrophobic amino acids at the C-terminus and bulky basic amino acids at the N-terminus of peptides significantly impacts peptide bitterness. Later on, Soltani et al. (2013) developed several models, including multiple linear regression, support vector machine, and artificial neural network models, to quantify the correlation between peptide structures and bitterness.
Detection and Quantification of Bitterness in Biopeptides
Because biopeptides are promising food ingredients, the importance of detecting and quantifying the perception of bitterness in food biopeptides cannot be underestimated. So far, methods for the detection and quantification of bitterness in biopeptides involve sensory evaluation, use of databases, electronic tongue, and/or calcium imaging technique.
Sensory Evaluation
The sensory evaluation of peptides and/or food products incorporated with biopeptides is the most straightforward method to detect and quantify bitterness. Most of the time, trained experts or a consumer panel is used for this purpose. In a study by Seo et al. (2008), soy protein was hydrolysed using various proteases and the bitterness of the diluted hydrolysate solutions was quantified by a sensory panel using the taste dilution analysis method. The results showed that bitterness of the hydrolysate increased with increase in degree of hydrolysis, and that the developed taste dilution analysis method can be applied as an alternative to the conventional hedonic scale sensory evaluation method. Moreover, Yu et al. (2013) studied the impact of adding soybean peptides on the sensory profile of a beverage product. The panellists reported that the bitter taste is the major sensory attributes affecting the overall acceptability of the beverage. In addition, the optimum peptide content in the formulation was 5%.
However, despite the fact that sensory evaluation methods have been widely used, they have some disadvantages. (1) The inherent bitterness from other food compounds such as polyphenols may interfere with the result. (2) Human panel method is well-associated with low objectivity and reproducibility. (3) Sensory evaluation can be time-consuming and not appropriate for screening purpose. And (4), the possible toxicity and allergenicity of some peptides need to be investigated before the sensory evaluation can be conducted.
Use of Bitterness Databases
Generally, the preparation and characterisation of biopeptides can be time-consuming. Therefore, some databases have been established to allow researchers to source the information (Table 5). For example, BitterDB database has more than 550 reported compounds with bitter taste, together with their molecular structures and bitterness intensities (Wiener et al., 2012). EROP-Moscow database allows researchers to search for many key features of oligopeptides as well as perform statistical analysis of the data (Zamyatnin et al., 2006). This database has 84 peptides with characteristic sensory attributes and most of them exhibit bitterness. BIOPEP-UWM database (formerly BIOPEP) can also be used as an effective tool. Iwaniak et al. (2016) incorporated 347 peptides and 10 amino acids with experimentally confirmed sequence, taste, and molecular and monoisotopic masses into this database. Researchers can even use this database to simulate the peptide release using selected proteolytic enzymes and predict the bitterness property of the peptides. For example, Pooja et al. (2017) predicted the dipeptidyl peptidase 4 inhibiting property, physicochemical characteristics and sensory profile of rice bran peptide produced using ficin using this database; however, they did not validate the bitterness property using human sensory panel or other methods.
Electronic Tongue
Electronic tongue is a multisensory system that consists of a number of low-selective sensors. It uses advanced mathematical procedures to process the collected signals and/or analyse multivariate data (Vlasov et al., 2005). During the last decade, detection, quantification and prediction of the peptide bitterness using electronic tongue has been reported (Ding et al., 2017; Zhang et al., 2018; Xu et al., 2019). For example, Newman et al. (2014a) used an electronic tongue to detect the bitterness of caffeine and dairy protein hydrolysates as well as trained panellists. Furthermore, the electronic tongue successfully detected weak bitterness in the sample and differentiated the bitterness between whey- and casein-based hydrolysates. This suggests that the electronic tongue was more sensitive and reliable than human panellists. Later on, the authors evaluated the bitterness of 19 dairy protein hydrolysates using an electronic tongue and correlated this data with the one generated by a trained sensory panel (Newman et al., 2014b). In this study, partial least square regression models were developed using the data from electronic tongue, molecular weight and relative hydrophobicity. These models can be potentially used to predict the bitterness of dairy protein hydrolysates.
Calcium Imaging Method
Proteins/genes named receptor type 2/taste receptor family B (T2R/TRB) were discovered for their putative functional activity as bitter taste receptors (Adler et al., 2000; Matsunami et al., 2000). So far, around 30 T2R candidate bitter taste receptors have been identified. In some taste receptor cells containing α-gustducin, a G-protein complex that is involved in sweet, bitter, and umami taste transduction, T2R proteins/genes can be invariably expressed (Wong et al., 1996). The latter elicits Ca2+ release from internal stores and/or promote Ca2+ entry into the cells (Ogura et al., 2002). Therefore, the amount of intracellular Ca2+ is correlated to bitterness and the released Ca2+ can be quantified using fluorescence calcium indicators. However, this new technology is still at the early stage and it has only been used to detect the cell response to pharmaceutical compounds. To date, there are no studies available using the calcium signalling method to detect or measure the bitterness in food-derived biopeptide products.
Debittering of Biopeptides
Regardless of the source of peptides, their bitterness dramatically limits their application in food and/or nutraceutical products. As a result, a variety of approaches such as separation of bitter peptides, enzymatic treatment, and encapsulation have been trialled to reduce, mask and/or eliminate this bitterness. However, the debittering of biopeptides is at the development stage and most of the reported debittering methods used animal-based peptides as examples. Therefore, some of these techniques are covered in this section to provide insights for debittering plant-based peptides.
Separation of Bitter Peptides
Since the exposed hydrophobic amino acid residues during food proteins hydrolysis result in bitterness in peptides, the separation of those bitter peptides from the hydrolysate seems like a reasonable approach.
Based on the π-π stacking interactions with aromatic side chains of peptides, activated carbon or macroporous resin can be used for debittering peptides (Clark et al., 2012). The separation efficiency depends on the affinity of the peptides to the adsorptive material. For example, 98.4 and 64.5% of phenylalanine was removed from whey protein hydrolysate using macroporous resin and activated carbon columns, respectively (Bu et al., 2020). Also, some bitter peptides can be removed by extraction using selected alcohols. Sinthusamran et al. (2020) studied the efficacy of different alcohols on decreasing the bitterness of salmon frame protein hydrolysates. The authors reported that 2-butanol worked more efficiently than iso-propanol in lowering the hydrolysates hydrophobicity and their bitterness intensity. Later on, 2-butanol and β-cyclodextrin were further used in combination to successfully debitter salmon frame protein hydrolysates (Singh et al., 2020).
However, it is worth noting the disadvantage of debittering peptides via the separation of hydrophobic amino acid residues. This process has been associated with the loss of essential hydrophobic amino acids and low processing efficiency. Moreover, the reported studies only focused on the development of debittering process and the bioactivity of debittered peptides still needs to be investigated.
Enzymatic Treatment of Biopeptides
The activity of a particular protease significantly affects the degree of hydrolysis of a protein, which is associated with the bitterness of peptides. For example, compared with the pea protein hydrolysed by papain, trypsin, bromelain and chymotrypsin, the hydrolysate prepared using Alcalase and Esperase had higher degree of hydrolysis and bitterness intensities (Arteaga et al., 2020). Similarly, soy protein hydrolysed by Alcalase was more bitter than the ones treated by Neutrase, papain, Corolase, and Flavourzyme (Meinlschmidt et al., 2016a). Generally, based on the mechanism of action and catalytic sites, proteases can be categorised into two groups: exopeptidases (E.C.3.4.11–3.4.19) and endopeptidases (E.C.3.4.21–3.4.99). Exopeptidases cleave free amino acids and/or low molecular weight peptides from the end of a polypeptide chain while endopeptidases act within a polypeptide chain (Stressler et al., 2015). Exopeptidases exhibit lower activity against intact protein molecules than endopeptidase and some exopeptidases show specificity toward hydrophobic amino acid residue at the N-terminal (Stressler et al., 2019). Therefore, exopeptidases can be used to cleave the bitter hydrophobic amino acid residue exposed during the protein hydrolysis by endopeptidases. For this purpose, aminopeptidases have been commonly used (Raksakulthai and Haard, 2003).
Microorganisms are a promising source of aminopeptidases and, to date, over 100 aminopeptidases have been identified from the genus Lactobacillus. Generally, aminopeptidase N (PepN, EC 3.4.11.2) and the proline-specific X-prolyl dipeptidyl aminopeptidase (PepX, EC 3.4.14.11) are two aminopeptidases that have been used for debittering purposes (Gonzales and Robert-Baudouy, 1996). Briefly, PepN hydrolyses almost all the amino acids from the amino (N-) terminus of a polypeptide chain, unless proline is present at the second position (which stops the hydrolysis) (Stressler et al., 2013). PepX is a proline-specific dipeptidyl peptidase that releases X-Proline dipeptides from the amino (N-) terminus of a polypeptide chain, as long as there are no proline or hydroxyproline at the second position (Stressler et al., 2014). Recently, Ewert et al. (2018) observed the superiority of PepN in debittering sodium caseinate hydrolysates, compared with PepX or aminopeptidase A (PepA). This might be due to the high specificity of PepX and PepA. PepX releases X-Proline dipeptides from the N-terminal of peptides, as a proline-specific aminopeptidase, while PepA is specific for the hydrophilic amino acids, such as Gtamic acid, Aspartic acid, and Serine. These amino acids are not associated with bitterness. During the enzymatic treatment, PepN was capable of cleaving bitter amino acids such as Leucine, Phenylalanine, Isoleucine, or Valine from N-terminus of most peptides. The debittering potential of PepN has been reported previously in a combination with PepX (Barry et al., 2000). Moreover, since aminopeptidases are released by microbes during microbial growth (Stressler et al., 2014), some particular strains have been used directly as debittering starters to produce protein hydrolysates. For example, the addition of Lactobacillus perolens, Rhizopus oryzae, and Actinomucor elegans significantly reduced the bitterness intensity of soy protein hydrolysates (Meinlschmidt et al., 2016b).
Alternatively, cross-linking of the peptides using transglutaminase (TG) has been used to decrease their bitterness. TG catalyses intra- and intermolecular cross-linking between glutamine and lysine residues in the peptide chain (Báez et al., 2011). This increases the molecular mass of peptides so that hydrophobic groups, which cause bitterness, can be buried again in the cross-linked polypeptide chain. As reported by Song et al. (2013), TG increased the content of 1,000–5,000 Da peptides in soybean protein hydrolysates via enzymatic cross-linking. The authors observed a significant decrease in the amount of bitter amino acids and improvement in sensory profile of the crosslinked peptide. However, it should be noted that the use of transglutaminase may compromise the functionality, biological activity and/or solubility of protein hydrolysates. Therefore, this approach may not be appropriate for certain food applications (Meng et al., 2020).
Encapsulation of Biopeptides
Encapsulation provides an opportunity to partially or completely mask the bitterness in peptides in addition to improving their other properties such as hygroscopicity and bioavailability. To date, several techniques such as spray drying, freeze drying, spray chilling, coacervation and double emulsion have been applied to encapsulate protein hydrolysates or biopeptides. The molecular and physicochemical properties of biopeptides, such as molecular weight, conformation, electrical characteristic, polarity, and stability, are the first factors to consider when choosing an appropriate carrier, as these properties will impact loading, retention, stability, and release from the encapsulated systems (McClements, 2018). Generally, for food applications, the selected carrier should meet some criteria such as edibility, biodegradability, non-toxicity, and inexpensiveness (Mohan et al., 2015). As a result, proteins, polysaccharides and lipids have been used as protective carriers for the encapsulation of protein hydrolysates, as shown in Table 6.
Spray Drying
During the spray drying of peptides, the solution/slurry/emulsion containing peptides is atomised into droplets and these droplets are dried into solid powder using hot air at a certain temperature and pressure (Kurozawa et al., 2009). To date, it is still the most economic and industrialised method used for encapsulation and this technique has been trialled to reduce the bitterness of a wide range of peptide products, including whey protein hydrolysates (Ma et al., 2014), casein hydrolysates (Molina Ortiz et al., 2009; Favaro-Trindade et al., 2010; Sarabandi et al., 2018), and mussel protein hydrolysates (Breternitz et al., 2017). In a study by Yang et al. (2012), whey protein hydrolysate with 21.42% degree of hydrolysis was mixed with maltodextrin (dextrose equivalence of 10) and maltodextrin/β-cyclodextrin, respectively, and the mixture was spray dried to produce whey protein hydrolysates powder. The sensory evaluation result indicated that both encapsulated whey protein hydrolysates exhibited one-eighth the bitterness intensity of non-encapsulated hydrolysates. The only issue with spray drying is the possibility of losing the native structure of biopeptides at high temperatures due to their high chemical reactivity with other peptides or food matrix components.
Freeze Drying
Contrary to spary drying, freeze drying (also known as lyophilisation) dehydrates the sample at low temperature and pressure to preserve the quality of bioactive compounds. However, compared with the compact or microsphere structure of spray dried powder, the freeze dried one exhibited a more porous or irregular structure so the masking of the bitterness of peptides might be compromised (Chranioti et al., 2016). For instance, Ma et al. (2014) encapsulated whey protein concentrate hydrolysate in whey protein concentrate-sodium alginate matrix, followed by spray- or freeze-drying. Although the sensory analysis suggested that both protein- and protein/polysaccharide-encapsulated hydrolysate exhibited lower bitterness intensity, the freeze-dried powder was more bitter than the spray-dried one due to the broken lamellar structure of the former. Meanwhile, the time consuming, high energy consumption and high cost nature of freeze drying also hinder its application (Sarabandi et al., 2020).
Spray Chilling
Spray chilling, also named spray cooling, congealing, or prilling, is another atomization-based encapsulation technique. It is a process of solidifying the atomised liquid spray into particles. Therefore, the carriers used in this technique are usually fats, vegetable oil or their derivatives with a high melting point (Oriani et al., 2016). As a result, the microcapsules are also called “solid lipid microparticles.” Briefly, the biopeptide solution is emulsified in a lipid at an elevated temperature above the lipid's melting point. Then the emulsion is cooled down to induce the crystallisation of the lipid phase and formation of the solid particles (shown as Figure 2). To date, spray chilling technique has been used in various applications, such as masking undesired odour or flavour, improving the appearance, increasing the stability, and achieving control-release property of various bioactive compounds (Tulini et al., 2016; Gottschalk et al., 2018; Kim et al., 2019) while the encapsulation of biopeptides using the spray chilling technique is still at the early stage. Salvim et al. (2015) reported that soy protein hydrolysate solution was emulsified in partially hydrogenated cotton seed oil, stabilised by polyglycerol polyricinoleate (PGPR) at a temperature beyond 50°C and, subsequently, the water-in-oil emulsion was pumped into a cold chamber (15°C) to produce solid lipid particles containing soybean protein hydrolysate. Although the encapsulation efficiency of the peptide was 96% and no chemical reactions were observed among the materials, the effect of encapsulation on the bitterness masking of the biopeptides was not investigated.
Complex Coacervation
Complex coacervation is another promising microencapsulation technique that has been extensively employed in the pharmaceutical, food, agricultural and textile industries. Complex coacervation in food ingredient encapsulation involves the interaction of oppositely charged polyelectrolytes, such as proteins and polysaccharides, in an aqueous form over a narrow pH range to form a surface-active agent which can be used for microencapsulation purpose (Timilsena et al., 2019). Mendanha et al. (2009) used complex coacervation between soybean protein isolate and pectin and the coacervates to encapsulate casein hydrolysate. The encapsulated peptides exhibited lower hygroscopicity and higher surface tension than the free hydrolysate and the encapsulation efficiency varied in the range of 78.8–91.62% in the microcapsule with different wall material-to-core ratios. Moreover, sensory panellists observed that the encapsulated hydrolysate with high encapsulation efficiency (78.8–91.62%) was less bitter than the free hydrolysate, indicating that encapsulation can serve as an efficient method for attenuation of the bitter taste of biopeptides.
Double Emulsions
Double emulsions are liquid dispersions where one emulsion is further dispersed in another liquid to produce double liquid droplets with multiple phases. Recently, this encapsulation system has been trailed for the stabilisation of peptides (Giroux et al., 2016; Jamshidi et al., 2018). Ying et al. (2021) illustrated the process of preparation of a water-in-oil-in-water (W1/O/W2) to encapsulate soy peptides. Firstly, concentrated bioactive peptide solution (40%, w/w) with molecular weight below 3,000 Da was prepared and homogenised in the medium chain triglycerides (MCT) oil, using polyglycerol polyricinoleate (PGPR) as the hydrophobic emulsifier, to stabilise the W/O interface (Figures 3A,B). Based on the optimisation of W1:O ratio and PGPR concentration, optimum W1/O emulsion was prepared, with the droplet size of 170 nm (Figure 3B). Subsequently, the W1/O emulsion was further emulsified in the outer aqueous phase to produce the final W1/O/W2 emulsion using octenyl succininc anhydride (OSA) starch and maltodextrin as the shell material with a high peptide encapsulation efficiency (>80%). Finally, in order to facilitate the potential application, this W1/O/ emulsion was further dehydrated into the peptide powder and high peptide encapsulation efficiency (>70%) was observed in the freeze dried powder (Figures 3C,D). The encapsulation of peptides in the double emulsion system is also still relatively new, and the effect of double encapsulation on bitterness masking has not been investigated.
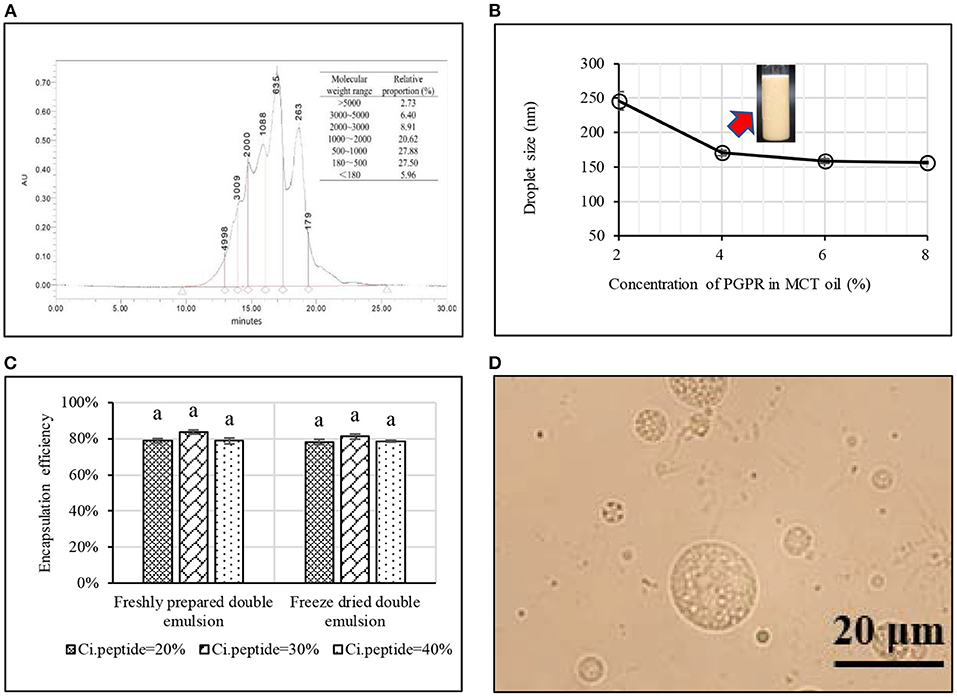
Figure 3. Encapsulation of biopeptides using water-in-oil-in-water (W1/O/W2) emulsion (Ying et al., 2021): (A) Molecular weight distribution of soy peptides; (B) Effect of polyglycerol polyricinoleate concentration on droplet size of W1/O emulsion; (C) Effect of dehydration technique and peptide content in W1 phase on peptide encapsulation efficiency in double emulsions; and (D) Morphology of reconstituted freeze dried peptide powder.
Conclusion
Due to the increasing global population and sustainability concerns about food security and the environment, plant-based proteins are good alternatives to animal-based ones to produce bioactive peptides. To date, a wide range of functional properties and biological activities including antioxidant, antimicrobial, anticancer, hypocholesterolaemic, antihypertensive, immunomodulatory, and opioid-like activities have been reported for plant-based biopeptides prepared via enzymatic hydrolysis of proteins. Although the biopeptides can be used to develop novel functional food products with desired functional properties and health benefits, many are associated with undesired bitter taste due to the presence of exposed hydrophobic residues. As a result, various bitterness detection and quantification methods have been used to better understand the nature and intensity of this bitterness property. Additionally, multiple physicochemical techniques have been developed to debitter these biopeptides with differing extent of success. Future research is required to investigate the correlation between functional and/or biological properties of peptides with their structures, as well as the impact of debittering processes on the bioactivity and bioavailability of peptides. The effect of the debittering process on the techno-functional properties of the peptides also needs to be studied.
Author Contributions
XY: data curation, writing—original draft, and writing—review and editing. BW: conceptualisation, data curation, writing—original draft, and writing—review and editing. DA, CU, and BA: writing—review and editing. All authors contributed to the article and approved the submitted version.
Conflict of Interest
XY is employed by China Oil and Foodstuffs Corporation Nutrition & Health Research Institute.
The remaining authors declare that the research was conducted in the absence of any commercial or financial relationships that could be construed as a potential conflict of interest.
Publisher's Note
All claims expressed in this article are solely those of the authors and do not necessarily represent those of their affiliated organizations, or those of the publisher, the editors and the reviewers. Any product that may be evaluated in this article, or claim that may be made by its manufacturer, is not guaranteed or endorsed by the publisher.
References
Abdul-Mumeen, I., Zakpaa, H. D., and Mills_Robertson, F. C. (2013). Biochemical and microbiological analysis of shea nut cake: a waste product from shea butter processing. J. Agric. Biotechnol. Sustain. Dev. 5, 61–68. doi: 10.5897/JABSD12.031
Acquah, C., Stefano, E. D., and Udenigwe, C. C. (2018). Role of hydrophobicity in food peptide functionality and bioactivity. J. Food Bioact. 4, 88–98. doi: 10.31665/JFB.2018.4164
Adler, E., Hoon, M. A., Mueller, K. L., Chandrashekar, J., Ryba, N. J., and Zuker, C. S. (2000). A novel family of mammalian taste receptors. Cell 100, 693–702. doi: 10.1016/S0092-8674(00)80705-9
Adler-Nissen, J. (1986). Enzymic Hydrolysis of Food Proteins. New York, NY: Elsevier Applied Science Publishers.
Aguilar-Toalá, J., Santiago-López, L., Peres, C., Peres, C., Garcia, H., Vallejo-Cordoba, B., et al. (2017). Assessment of multifunctional activity of bioactive peptides derived from fermented milk by specific Lactobacillus plantarum strains. J. Dairy Sci. 100, 65–75. doi: 10.3168/jds.2016-11846
Agyei, D., Ongkudon, C. M., Wei, C. Y., Chan, A. S., and Danquah, M. K. (2016). Bioprocess challenges to the isolation and purification of bioactive peptides. Food Bioprod. Process. 98, 244–256. doi: 10.1016/j.fbp.2016.02.003
Ahar, N. H., Khaki, A., Akbari, G., and Novin, M. G. (2014). The effect of busulfan on body weight, testis weight and mda enzymes in male rats. Int. J. Womens Health Reproduct. Sci. 2, 316–319. doi: 10.15296/ijwhr.2014.52
Ahmed, J., Preissner, S., Dunkel, M., Worth, C. L., Eckert, A., and Preissner, R. (2011). SuperSweet–a resource on natural and artificial sweetening agents. Nucleic Acids Res. 39, D377–D382. doi: 10.1093/nar/gkq917
Ahmed, T. A. E., and Hammami, R. (2019). Recent insights into structure–function relationships of antimicrobial peptides. J. Food Biochem. 43:e12546. doi: 10.1111/jfbc.12546
Ajibola, C. F., Fashakin, J. B., Fagbemi, T. N., and Aluko, R. E. (2011). Effect of peptide size on antioxidant properties of African yam bean seed (Sphenostylis stenocarpa) protein hydrolysate fractions. Int. J. Mol. Sci. 12, 6685–6702. doi: 10.3390/ijms12106685
Arteaga, V. G., Guardia, M. A., Muranyi, I., Eisner, P., and Schweiggert-Weisz, U. (2020). Effect of enzymatic hydrolysis on molecular weight distribution, techno-functional properties and sensory perception of pea protein isolates. Innovat. Food Sci. Emerg. Technol. 65:102449. doi: 10.1016/j.ifset.2020.102449
Ayyash, M., Liu, S. Q., Al Mheiri, A., Aldhaheri, M., Raeisi, B., Al-Nabulsi, A., et al. (2019). In vitro investigation of health-promoting benefits of fermented camel sausage by novel probiotic Lactobacillus plantarum: a comparative study with beef sausages. LWT Food Sci. Technol. 99, 346–354. doi: 10.1016/j.lwt.2018.09.084
Badger, T. M., Ronis, M. J. J., Simmen, R. C. M., and Simmen, F. A. (2005). Soy protein isolate and protection against cancer. J. Am. Coll. Nutr. 24, 146S−149S. doi: 10.1080/07315724.2005.10719456
Báez, G. D., Moro, A., Ballerini, G. A., Busti, P. A., and Delorenzi, N. J. (2011). Comparison between structural changes of heat-treated and transglutaminase cross-linked beta-lactoglobulin and their effects on foaming properties. Food Hydrocoll. 25, 1758–1765. doi: 10.1016/j.foodhyd.2011.02.033
Bagley, E. E., and Ingram, S. L. (2020). Endogenous opioid peptides in the descending pain modulatory circuit. Neuropharmacology 173, 108131. doi: 10.1016/j.neuropharm.2020.108131
Barreto-Santamaría, A., Rivera, Z. J., García, J. E., Curtidor, H., Patarroyo, M. E., Patarroyo, M. A., et al. (2020). Shorter antibacterial peptide having high selectivity for E. coli membranes and low potential for inducing resistance. Microorganisms 8:867. doi: 10.3390/microorganisms8060867
Barry, C. M., O'Cuinn, G., Harrington, D., O'Callaghan, D. M., and Fitzgerald, R. J. (2000). Debittering of a tryptic digest of bovine p-casein using porcine kidney general aminopeptidase and X-Prolydipeptidyl aminopeptidase from Lactococcus lactis subsp. cremoris AM2. J. Food Sci. 65, 1145–1150. doi: 10.1111/j.1365-2621.2000.tb10255.x
Bento, A. P., Gaulton, A., Hersey, A., Bellis, L. J., Chambers, J., Davies, M., et al. (2014). The ChEMBL bioactivity database: an update. Nucleic Acids Res. 42, 1083–1090. doi: 10.1093/nar/gkt1031
Bhat, Z. F., Kumar, S., and Bhat, H. F. (2017). Antihypertensive peptides of animal origin: a review. Crit. Rev. Food Sci. Nutr. 57, 566–578. doi: 10.1080/10408398.2014.898241
Breternitz, N. R., Bolini, H. M. A., and Hubinger, M. D. (2017). Sensory acceptance evaluation of a new food flavoring produced by microencapsulation of a mussel (Perna perna) protein hydrolysate. LWT Food Sci. Technol. 83, 141–149. doi: 10.1016/j.lwt.2017.05.016
Bu, T., Zhou, M., Zheng, J., Yang, P., Song, H., Li, S., et al. (2020). Preparation and characterization of a low-phenylalanine whey hydrolysate using two-step enzymatic hydrolysis and macroporous resin adsorption. LWT 132:109753. doi: 10.1016/j.lwt.2020.109753
Carter, A. A., Gomes, T., Camacho, X., Juurlink, D. N., Shah, B. R., and Mamdani, M. M. (2013). Risk of incident diabetes among patients treated with statins: population based study. Br. Med. J. 346:f2610. doi: 10.1136/bmj.f2610
Chalamaiah, M., Keskin Ulug, S., Hong, H., and Wu, J. (2019). Regulatory requirements of bioactive peptides (protein hydrolysates) from food proteins. Journal of Functional Foods. 58, 123–129. doi: 10.1016/j.jff.2019.04.050
Chalamaiah, M., Yu, W., and Wu, J. (2018). Immunomodulatory and anticancer protein hydrolysates (peptides) from food proteins: a review. Food Chem. 245, 205–222. doi: 10.1016/j.foodchem.2017.10.087
Chaves-López, C., Serio, A., Paparella, A., Martuscelli, M., Corsetti, A., Tofalo, R., et al. (2014). Impact of microbial cultures on proteolysis and release of bioactive peptides in fermented milk. Food Microbiol. 42, 117–121. doi: 10.1016/j.fm.2014.03.005
Cho, M. J., Unklesbay, N., Hsieh, F. H., and Clarke, A. D. (2004). Hydrophobicity of bitter peptides from soy protein hydrolysates. J. Agric. Food Chem. 52, 5895–5901. doi: 10.1021/jf0495035
Chranioti, C., Chanioti, S., and Tzia, C. (2016). Comparison of spray, freeze and oven drying as a means of reducing bitter aftertaste of steviol glycosides (derived from Stevia rebaudiana Bertoni plant) - Evaluation of the final products. Food Chem. 190, 1151–1158. doi: 10.1016/j.foodchem.2015.06.083
Cirkovic Velickovic, T. D., and Stanic-Vucinic, D. J. (2018). The role of dietary phenolic compounds in protein digestion and processing technologies to improve their antinutritive properties. Compr. Rev. Food Sci. Food Saf. 17, 82–103. doi: 10.1111/1541-4337.12320
Clark, H. M., Alves, C. C., Franca, A. S., and Oliveira, L. S. J. L. (2012). Evaluation of the performance of an agricultural residue-based activated carbon aiming at removal of phenylalanine from aqueous solutions. LWT 49, 155–161. doi: 10.1016/j.lwt.2012.04.026
Clemente, A. (2001). Enzymatic protein hydrolysates in human nutrition. Trends Food Sci. Technol. 11, 254–262. doi: 10.1016/S0924-2244(01)00007-3
Coelho, M. S., Soares-Freitas, R. A. M., Arêas, J. A. G., Gandra, E. A., and Salas-Mellado, M. M. (2018). Peptides from chia present antibacterial activity and inhibit cholesterol synthesis. Plant Foods Hum. Nutr. 73, 101–107. doi: 10.1007/s11130-018-0668-z
Dagan-Wiener, A., Di Pizio, A., Nissim, I., Bahia, M. S., Dubovski, N., Margulis, E., et al. (2019). BitterDB: taste ligands and receptors database in 2019. Nucleic Acids Res. 47, D1179–D1185. doi: 10.1093/nar/gky974
Daliri, E. B.-M., Lee, B. H., and Oh, D. H. (2016). Current perspectives on antihypertensive probiotics. Probiot. Antimicrob. Prot. 9, 91–101. doi: 10.1007/s12602-016-9241-y
Daliri, E. B. M., Oh, D., and Lee, B. (2017). Bioactive peptides. Foods 6:32. doi: 10.3390/foods6050032
Dhayakaran, R., Neethirajan, S., and Weng, X. (2016). Investigation of the antimicrobial activity of soy peptides by developing a high throughput drug screening assay. Biochem. Biophys. Rep. 6, 149–157. doi: 10.1016/j.bbrep.2016.04.001
Di Stefano, E., Tsopmo, A., Oliviero, T., Fogliano, V., and Udenigwe, C. C. (2019). Bioprocessing of common pulses changed seed microstructures, and improved dipeptidyl peptidase-IV and α-glucosidase inhibitory activities. Sci. Rep. 9:15308. doi: 10.1038/s41598-019-51547-5
Dia, V. P., and de Mejia, E. G. (2011). Lunasin induces apoptosis and modifies the expression of genes associated with extracellular matrix and cell adhesion in human metastatic colon cancer cells. Mol. Nutr. Food Res. 55, 623–634. doi: 10.1002/mnfr.201000419
Ding, Y., Li, X., and Kan, J. (2017). Isolation and identification of flavor peptides from douchi (traditional Chinese soybean food). Int. J. Food Propert. 20, 1982–1994. doi: 10.1080/10942912.2017.1360906
Duranti, M., Lovati, M. R., Dani, V., Barbiroli, A., Scarafoni, A., Castiglioni, S., et al. (2004). The α′ subunit from soybean 7S globulin lowers plasma lipids and upregulates liver β-VLDL receptors in rats fed a hypercholesterolemic diet. J. Nutr. 134, 1334–1339. doi: 10.1093/jn/134.6.1334
Egusa, S., and Otani, H. (2009). Soybean protein fraction digested with neutral protease preparation, “Peptidase R”, produced by Rhizopus oryzae, stimulates innate cellular immune system in mouse. Int. Immunopharmacol. 9, 931–936. doi: 10.1016/j.intimp.2009.03.020
Esfandi, R., Walters, M. E., and Tsopmo, A. (2019a). Antioxidant properties and potential mechanisms of hydrolyzed proteins and peptides from cereals. Heliyon 5:e01538. doi: 10.1016/j.heliyon.2019.e01538
Esfandi, R., Willmore, W. G., and Tsopmo, A. (2019b). Peptidomic analysis of hydrolyzed oat bran proteins, and their in vitro antioxidant and metal chelating properties. Food Chem. 279, 49–57. doi: 10.1016/j.foodchem.2018.11.110
Ewert, J., Schlierenkamp, F., Nesensohn, L., Fischer, L., and Stressler, T. (2018). Improving the colloidal and sensory properties of a caseinate hydrolysate using particular exopeptidases. Food Funct. 9, 5989–5998. doi: 10.1039/C8FO01749B
Favaro-Trindade, C. S., Santana, A. S., Monterrey-Quintero, E. S., Trindade, M. A., and Netto, F. M. (2010). The use of spray drying technology to reduce bitter taste of casein hydrolysate. Food Hydrocoll. 24, 336–340. doi: 10.1016/j.foodhyd.2009.10.012
Fernández-Tomé, S., Sanchón, J., Recio, I., and Hernández-Ledesma, B. (2017). Transepithelial transport of lunasin and derived peptides: inhibitory effects on the gastrointestinal cancer cells viability. J. Food Composit. Anal. 68, 101–110. doi: 10.1016/j.jfca.2017.01.011
Ferri, M., Graen-Heedfeld, J., Bretz, K., Guillon, F., Michelini, E., Calabretta, M. M., et al. (2017). Peptide fractions obtained from rice by-products by means of an environment-friendly process show in vitro health-related bioactivities. PLoS ONE 12:e0170954. doi: 10.1371/journal.pone.0170954
Fjell, C. D., Hiss, J. A., Hancock, R. E. W., and Schneider, G. (2012). Designing antimicrobial peptides: form follows function. Nat. Rev. Drug Discov. 11, 37–51. doi: 10.1038/nrd3591
Food Agriculture Organization of the United Nations. (2012). FAOSTAT Database. Rome: FAO. Available online at: http://www.fao.org/faostat/en/#home
Fu, Y., Liu, J., Hansen, E. T., Bredie, W. L. P., and Lametsch, R. (2018). Structural characteristics of low bitter and high umami protein hydrolysates prepared from bovine muscle and porcine plasma. Food Chem. 257, 163–171. doi: 10.1016/j.foodchem.2018.02.159
García-Tejedor, A., Sánchez-Rivera, L., Castelló-Ruiz, M., Recio, I., Salom, J. B., and Manzanares, P. (2014). Novel antihypertensive lactoferrin-derived peptides produced by Kluyveromyces marxianus: gastrointestinal stability profile and in vivo angiotensin I-converting enzyme (ACE) inhibition. J. Agric. Food Chem. 62, 1609–1616. doi: 10.1021/jf4053868
Garg, S., Apostolopoulos, V., Nurgali, K., and Mishra, V. K. (2018). Evaluation of in silico approach for prediction of presence of opioid peptides in wheat. J. Funct. Foods 41, 34–40. doi: 10.1016/j.jff.2017.12.022
Gertsch, J., Viveros-paredes, J. M., and Taylor, P. (2011). Plant immunostimulants-Scientific paradigm or myth? J. Ethnopharmacol. 136, 385–391. doi: 10.1016/j.jep.2010.06.044
Gilson, M., Liu, T., Baitaluk, M., Nicola, G., Hwang, L., and Chong, J. (2016). BindingDB in 2015: a public database for medicinal chemistry, computational chemistry and systems pharmacology. Nucleic Acids Res. 44, D1045–D1053. doi: 10.1093/nar/gkv1072
Girgih, A. T., He, R., Malomo, S., Offengenden, M., Wu, J., and Aluko, R. E. (2014). Structural and functional characterization of hemp seed (Cannabis sativa L.) protein-derived antioxidant and antihypertensive peptides. J. Func. Foods 6, 384–394. doi: 10.1016/j.jff.2013.11.005
Giroux, H. J., Robitaille, G., and Britten, M. (2016). Controlled release of casein-derived peptides in the gastrointestinal environment by encapsulation in water-in-oil-in-water double emulsions. LWT Food Sci. Technol. 69, 225–232. doi: 10.1016/j.lwt.2016.01.050
Gonzales, T., and Robert-Baudouy, J. (1996). Bacterial aminopeptidases: properties and functions. FEMS Microbiol. Rev. 18, 319–344. doi: 10.1111/j.1574-6976.1996.tb00247.x
González-García, E., Puchalska, P., Marina, M. L., and García, M. C. (2015). Fractionation and identification of antioxidant and angiotensin-converting enzyme-inhibitory peptides obtained from plum (Prunus domestica L.) stones. J. Funct. Foods 19, 376–384. doi: 10.1016/j.jff.2015.08.033
Görgüç, A., Özer, P., and Yilmaz, F. M. (2020). Simultaneous effect of vacuum and ultrasound assisted enzymatic extraction on the recovery of plant protein and bioactive compounds from sesame bran. J. Food Composit Anal. 87:103424. doi: 10.1016/j.jfca.2020.103424
Gottschalk, P., Brodesser, B., Poncelet, D., Jaeger, H., Rennhofer, H., and Cole, S. (2018). Formation of essential oil containing microparticles comprising a hydrogenated vegetable oil matrix and characterisation thereof. J. Microencapsul. 35, 513–521. doi: 10.1080/02652048.2018.1515998
Gutierrez, K., Glanzner, W. G., Chemeris, R. O., Rigo, M. L., Comim, F. V., Bordignon, V., et al. (2016). Gonadotoxic effects of busulfan in two strains of mice. Reprod. Toxicol. 59, 31–39. doi: 10.1016/j.reprotox.2015.09.002
Hartati, F. K., Widjanarko, S. B., Widyaningsih, T. D., and Rifa'i, M. (2017). Anti-Inflammatory evaluation of black rice extract inhibits TNF-α, IFN-γ and IL-6 cytokines produced by immunocompetent cells. Food Agric. Immunol. 28, 1116–1125. doi: 10.1080/09540105.2017.1332006
He, R., Girgih, A. T., Rozoy, E., Bazinet, L., Ju, X.-R., and Aluko, R. E. (2016). Selective separation and concentration of antihypertensive peptides from rapeseed protein hydrolysate by electrodialysis with ultrafiltration membranes. Food Chem. 197, 1008–1014. doi: 10.1016/j.foodchem.2015.11.081
Hernandez, L. M. R., and de Mejia, E. G. (2017). Bean peptides have higher in silico binding affinities than Ezetimibe for the N-terminal domain of cholesterol receptor Niemann-Pick C1 Like-1. Peptides 17, 30052–30059. doi: 10.1016/j.peptides.2017.02.011
Humiski, L. M., and Aluko, R. E. (2007). Physicochemical and bitterness properties of enzymatic pea protein hydrolysates. J. Food Sci. 72, S605–S611. doi: 10.1111/j.1750-3841.2007.00475.x
Hwang, J. S., Yoo, H. J., Song, H. J., Kim, K. K., Chun, Y. J., Matsui, T., et al. (2011). Inflammation-related signaling pathways implicating TGFβ are revealed in the expression profiling of MCF7 cell treated with fermented soybean, Chungkookjang. Nutr. Cancer 63, 645–652. doi: 10.1080/01635581.2011.551987
Ibrahim, H. R., Isono, H., and Miyata, T. (2018). Potential antioxidant bioactive peptides from camel milk proteins. Anim. Nutr. 4, 273–280. doi: 10.1016/j.aninu.2018.05.004
Iwaniak, A., Hrynkiewicz, M., Minkiewicz, P., Bucholska, J., and Darewicz, M. (2020). Soybean (glycine max) protein hydrolysates as sources of peptide bitter-tasting indicators: an analysis based on hybrid and fragmentomic approaches. Appl. Sci. 10:2514. doi: 10.3390/app10072514
Iwaniak, A., Minkiewicz, P., Darewicz, M., and Hrynkiewicz, M. (2016). Food protein-originating peptides as tastants-physiological, technological, sensory, and bioinformatic approaches. Food Res. Int. 89, 27–38. doi: 10.1016/j.foodres.2016.08.010
Iwaniak, A., Minkiewicz, P., Darewicz, M., Protasiewicz, M., and Mogut, D. (2015). Chemometrics and cheminformatics in the analysis of biologically active peptides from food sources. J. Funct. Foods 16, 334–351. doi: 10.1016/j.jff.2015.04.038
Jakubczyk, A., Karaś, M., Baraniak, B., and Pietrzak, M. (2013). The impact of fermentation and in vitro digestion on formation angiotensin converting enzyme (ACE) inhibitory peptides from pea proteins. Food Chem. 141, 3774–3780. doi: 10.1016/j.foodchem.2013.06.095
Jamshidi, A., Shabanpour, B., Pourashouri, P., and Raeisi, M. (2018). Using WPC-inulin-fucoidan complexes for encapsulation of fish protein hydrolysate and fish oil in W1/O/W2 emulsion: characterization and nutritional quality. Food Res. Int. 114, 240–250. doi: 10.1016/j.foodres.2018.07.066
Kamisli, S., Ciftci, O., Kaya, K., Cetin, A., Kamisli, O., and Ozcan, C. (2015). Hesperidin protects brain and sciatic nerve tissues against cisplatin-induced oxidative, histological and electromyographical side effects in rats. Toxicol. Ind. Health 31, 841–851. doi: 10.1177/0748233713483192
Kang, S., Mullen, J., Miranda, L. P., and Deshpande, R. (2012). Utilization of tyrosine- and histidine-containing dipeptides to enhance productivity and culture viability. Biotechnol. Bioeng. 109, 2286–2294. doi: 10.1002/bit.24507
Kannan, A., Hettiarachchy, N., Johnson, M. G., and Nannapaneni, R. (2008). Human colon and liver cancer cell proliferation inhibition by peptide hydrolysates derived from heat-stabilized defatted rice bran. J. Agric. Food Chem. 56, 11643–11647. doi: 10.1021/jf802558v
Kannan, A., Hettiarachchya, N., Lay, J. O., and Liyanage, R. (2010). Human cancer cell proliferation inhibition by a pentapeptide isolated and characterized from rice bran. Peptides 31, 1629–1634. doi: 10.1016/j.peptides.2010.05.018
Kaur, J., Kumar, V., Sharma, K., Kaur, S., Gat, Y., Goyal, A., et al. (2020). Opioid peptides: an overview of functional significance. Int. J. Pept. Res. Ther. 26, 33–41. doi: 10.1007/s10989-019-09813-7
Kearney, P. M., Whelton, M., Reynolds, K., Whelton, P. K., and He, J. (2005). Global burden of hypertension: analysis of worldwide data. Lancet 365, 217–223. doi: 10.1016/S0140-6736(05)17741-1
Khan, A., Nazar, H., Sabir, S. M., Irshad, M., Awan, S. I., Abbas, R., et al. (2014). Antioxidant activity and inhibitory effect of some commonly used medicinal plants against lipid per-oxidation in mice brain. Afr. J. Trad. Compl. Alternat. Med. 11, 83–90. doi: 10.4314/ajtcam.v11i5.14
Kim, G. Y., Lee, J., Lim, S., Kang, H., Ahn, S. I., Jhoo, J. W., et al. (2019). Microencapsulation of caramel flavor and properties of ready-to-drink milk beverages supplemented with coffee containing these microcapsules. Food Sci. Anim. Resourc. 39, 780–791. doi: 10.5851/kosfa.2019.e68
Kim, H. O., and Li-Chan, E. C. (2006). Quantitative structure-activity relationship study of bitter peptides. J. Agric. Food Chem. 54, 10102–10111. doi: 10.1021/jf062422j
Kim, M.-R., Yukio, K., Kim, K. M., and Lee, C.-H. (2008). Tastes and structures of bitter peptide, asparagine-alanine-leucine-proline-glutamate, and its synthetic analogues. J. Agric. Food Chem. 56, 5852–5858. doi: 10.1021/jf7036664
Kim, S., Thiessen, P. A., Bolton, E. E., Chen, J., Fu, G., Gindulyte, A., et al. (2016). PubChem substance and compound databases. Nucleic Acids Res. 44, D1202–D1213. doi: 10.1093/nar/gkv951
Kim, S. E., Kim, H. H., Kim, J. Y., Kang, Y. I., Woo, H. J., and Lee, H. J. (2000). Anticancer activity of hydrophobic peptides from soy proteins. BioFactors 12, 151–155. doi: 10.1002/biof.5520120124
Kohl, S., Behrens, M., Dunkel, A., Hofmann, T., and Meyerhof, W. (2013). Amino acids and peptides activate at least five members of the human bitter taste receptor family. J. Agric. Food Chem. 61, 53–60. doi: 10.1021/jf303146h
Kong, X., Guo, M., Hua, Y., Cao, D., and Zhang, C. (2008). Enzymatic preparation of immunomodulating hydrolysates from soy proteins. Bioresour. Technol. 99, 8873–8879. doi: 10.1016/j.biortech.2008.04.056
Kumar, R., Chaudhary, K., Sharma, M., Nagpal, G., Chauhan, J. S., Singh, S., et al. (2015). AHTPDB: a comprehensive platform for analysis and presentation of antihypertensive peptides. Nucleic Acids Res. 43, D956–D962 doi: 10.1093/nar/gku1141
Kurozawa, L. E., Park, K. J., and Hubinger, M. D. (2009). Effect of carrier agents on the physicochemical properties of a spray dried chicken meat protein hydrolysate. J. Food Eng. 94, 326–333. doi: 10.1016/j.jfoodeng.2009.03.025
Lammi, C., Zanoni, C., and Arnoldi, A. (2015). IAVPGEVA, IAVPTGVA, and LPYP, three peptides from soy glycinin, modulate cholesterol metabolism in HepG2 cells through the activation of the LDLR-SREBP2 pathway. J. Funct. Foods 14, 469–478. doi: 10.1016/j.jff.2015.02.021
Lammi, C., Zanoni, C., Calabresi, L., and Arnoldi, A. (2016). Lupin protein exerts cholesterol-lowering effects targeting PCSK9: from clinical evidences to elucidation of the in vitro molecular mechanism using HepG2 cells. J. Funct. Foods 23, 230–240. doi: 10.1016/j.jff.2016.02.042
Lee, S. Y., and Hur, S. J. (2017). Antihypertensive peptides from animal products, marine organisms, and plants. Food Chem. 228, 506–517. doi: 10.1016/j.foodchem.2017.02.039
Leo Pruimboom, L., and de Punder, K. (2015). The opioid effects of gluten exorphins: aymptomatic celiac disease. J. Health Popul. Nutr. 33:24. doi: 10.1186/s41043-015-0032-y
Li, F., Gao, Z., Wang, K., Zhao, Y., Wang, H., Zhao, M., et al. (2021). A novel defensin-like peptide contributing to antimicrobial and antioxidant capacity of the tick dermacentor silvarum (Acari: Ixodidae). Exp. Appl. Acarol. 83, 271–283. doi: 10.1007/s10493-020-00584-1
Li, H., and Aluko, R. E. (2010). Identification and inhibitory properties of multifunctional peptides from pea protein hydrolysate. J. Agric. Food Chem. 58, 11471–11476. doi: 10.1021/jf102538g
Li, J. T., Zhang, J. L., He, H., Ma, Z. L., Nie, Z. K., Wang, Z. Z., et al. (2013). Apoptosis in human hepatoma HepG2 cells induced by corn peptides and its anti-tumor efficacy in H22 tumor bearing mice. Food Chem. Toxicol. 5, 297–305. doi: 10.1016/j.fct.2012.09.038
Limón, R. I., Peñas, E., Torino, M. I., Martínez-Villaluenga, C., Dueñas, M., and Frias, J. (2015). Fermentation enhances the content of bioactive compounds in kidney bean extracts. Food Chem. 172, 343–352. doi: 10.1016/j.foodchem.2014.09.084
Liu, L., Liu, L., Lu, B., Chen, M., and Zhang, Y. (2013). Evaluation of bamboo shoot peptide preparation with angiotensin converting enzyme inhibitory and antioxidant abilities from byproducts of canned bamboo shoots. J. Agric. Food Chem. 61, 5526–5533. doi: 10.1021/jf305064h
Liu, Z., and Udenigwe, C. C. (2019). Role of food-derived opioid peptides in the central nervous and gastrointestinal systems. J. Food Biochem. 43, 1–7. doi: 10.1111/jfbc.12629
Lucera, A., Costa, C., Conte, A., and Del Nobile, M. A. (2012). Food applications of natural antimicrobial compounds. Front. Microbiol. 3:287. doi: 10.3389/fmicb.2012.00287
Lumen, B. O. D. (2005). Lunasin: a cancer-preventive soy peptide. Nutr. Rev. 63, 16–21. doi: 10.1111/j.1753-4887.2005.tb00106.x
Ma, J.-J., Mao, X.-Y., Wang, Q., Yang, S., Zhang, D., Chen, S.-W., et al. (2014). Effect of spray drying and freeze drying on the immunomodulatory activity, bitter taste and hygroscopicity of hydrolysate derived from whey protein concentrate. LWT Food Sci. Technol. 56, 296–302. doi: 10.1016/j.lwt.2013.12.019
Maehashi, K., and Huang, L. (2009). Bitter peptides and bitter taste receptors. Cell. Mol. Life Sci. 66, 1661–1671. doi: 10.1007/s00018-009-8755-9
Maehashi, K., Matano, M., Wang, H., Vo, L. A., Yamamoto, Y., and Huang, L. (2008). Bitter peptides activate hTAS2Rs, the human bitter receptors. Biochem. Biophys. Res. Commun. 365, 851–855. doi: 10.1016/j.bbrc.2007.11.070
Mahdi, C., Untari, H., Padaga, M. C., and Raharjo, S. J. (2018). The characterization of bioactive peptides of goat milk fermented to activities as anti-hypercholerolemia. Int. Food Res. J. 25, 17–23. doi: 10.1088/1757-899X/299/1/012014
Mancini, G. J., Baker, S., Bergeron, J., Fitchett, D., Frohlich, J., Genest, J., et al. (2016). Diagnosis, prevention, and management of statin adverse effects and intolerance: Canadian consensus working group update. Can. J. Cardiol. 32, S35–S65. doi: 10.1016/j.cjca.2016.01.003
Marambe, P. W., Shand, P. J., and Wanasundara, J. P. D. (2008). An in-vitro investigation of selected biological activities of hydrolysed flaxseed (Linum usitatissimum L.) proteins. J. Am. Oil Chem. Soc. 85, 1155–1164. doi: 10.1007/s11746-008-1293-z
Marques, M. R., Freitas, R. A. M. S., Carlos, A. C. C., Siguemoto, É. S., Fontanari, G. G., and Arêas, J. A. (2015). Peptides from cowpea present antioxidant activity, inhibit cholesterol synthesis and its solubilisation into micelles. Food Chem. 168, 288–293. doi: 10.1016/j.foodchem.2014.07.049
Matsunami, H., Montmayeur, J. P., and Buck, L. B. (2000). A family of candidate taste receptors in human and mouse. Nature 404, 601–604. doi: 10.1038/35007072
McClements, D. J. (2018). Encapsulation, protection, and delivery of bioactive proteins and peptides using nanoparticle and microparticle systems: a review. Adv. Colloid Interface Sci. 253, 1–22. doi: 10.1016/j.cis.2018.02.002
Megías, C., Pedroche, J., Yust, M. M., Girón-Calle, J., Alaiz, M., Millán, F., et al. (2008). Production of copper-chelating peptides after hydrolysis of sunflower proteins with pepsin and pancreatin. LWT Food Sci. Technol. 41, 1973–1977. doi: 10.1016/j.lwt.2007.11.010
Meinlschmidt, P., Schweiggert-Weisz, U., Brode, V., and Eisner, P. (2016a). Enzyme assisted degradation of potential soy protein allergens with special emphasis on the technofunctionality and the avoidance of a bitter taste formation. LWT Food Sci. Technol. 68, 707–716. doi: 10.1016/j.lwt.2016.01.023
Meinlschmidt, P., Schweiggert-Weisz, U., and Eisner, P. (2016b). Soy protein hydrolysates fermentation: effect of debittering and degradation of major soy allergens. LWT Food Sci. Technol. 71, 202–212. doi: 10.1016/j.lwt.2016.03.026
Mendanha, D. V., Molina Ortiz, S. E., Favaro-Trindade, C. S., Mauri, A., Monterrey-Quintero, E. S., and Thomazini, M. (2009). Microencapsulation of casein hydrolysate by complex coacervation with SPI/pectin. Food Res. Int. 42, 1099–1104. doi: 10.1016/j.foodres.2009.05.007
Meng, S., Tan, Y., Chang, S., Li, J., Maleki, S., and Puppala, N. J. F. C. (2020). Peanut allergen reduction and functional property improvement by means of enzymatic hydrolysis and transglutaminase crosslinking. Food Chem. 302:125186. doi: 10.1016/j.foodchem.2019.125186
Meshginfar, N., Mahoonak, A. S., Hosseinian, F., and Tsopmo, A. (2019). Physicochemical, antioxidant, calcium binding, and angiotensin converting enzyme inhibitory properties of hydrolyzed tomato seed proteins. J. Food Biochem. 43:e12721. doi: 10.1111/jfbc.12721
Mills, K. T., Bundy, J. D., Kelly, T. N., Reed, J. E., Kearney, P. M., Reynolds, K., et al. (2016). Global disparities of hypertension prevalence and control. Circulation 134, 441–450. doi: 10.1161/CIRCULATIONAHA.115.018912
Minkiewicz, P., Iwaniak, A., and Darewicz, M. (2019). BIOPEP-UWM database of bioactive peptides: current opportunities. Int. J. Mol. Sci. 20:5978. doi: 10.3390/ijms20235978
Mohan, A., Rajendran, S. R. C. K., He, Q. S., Bazinet, L., and Udenigwe, C. C. (2015). Encapsulation of food protein hydrolysates and peptides: a review. RSC Adv. 5, 79270–79278. doi: 10.1039/C5RA13419F
Molina Ortiz, S. E., Mauri, A., Monterrey-Quintero, E. S., Trindade, M. A., Santana, A. S., and Favaro-Trindade, C. S. (2009). Production and properties of casein hydrolysate microencapsulated by spray drying with soybean protein isolate. LWT Food Sci. Technol. 42, 919–923. doi: 10.1016/j.lwt.2008.12.004
Montoya-Rodriguez, A., Gonzalez de Mejía, E., Dia, V. P., Reyes-Moreno, C., and Milan-Carrillo, J. (2014). Extrusion improved the anti-inflammatory effect of amaranth (Amaranthus hypochondriacus) hydrolysates in LPS-induced humanTHP-1 macrophage-like and mouse RAW 264.7 macrophages by preventing activation of NF-κB signaling. Mol. Nutr. Food Res. 58, 1028–1041. doi: 10.1002/mnfr.201300764
Morris, H. J., Carrillo, O., Almarales, A., Bermudez, R. C., Lebeque, Y., Fontaine, R., et al. (2007). Immunostimulant activity of an enzymatic protein hydrolysate from green microalga Chlorella vulgaris on undernourished mice. Enzyme Microb. Technol. 40, 456–460. doi: 10.1016/j.enzmictec.2006.07.021
Moure, A., Domínguez, H., and Paraj,ó, J. C. (2006). Antioxidant properties of ultrafiltration-recovered soy protein fractions from industrial effluents and their hydrolysates. Process Biochem. 41, 447–456. doi: 10.1016/j.procbio.2005.07.014
Murray, N. M., O'Riordan, D., Jacquier, J. C., O'Sullivan, M., Holton, T. A., Wynne, K., et al. (2018). Peptidomic screening of bitter and nonbitter casein hydrolysate fractions for insulinogenic peptides. J. Dairy Sci. 101, 2826–2837. doi: 10.3168/jds.2017-13853
Najafian, L., and Babji, A. S. (2018). Fractionation and identification of novel antioxidant peptides from fermented fish (pekasam). J. Food Measure. Char. 12, 2174–2183. doi: 10.1007/s11694-018-9833-1
Ndiaye, F., Vuong, T., Duarte, J., Aluko, R. E., and Matar, C. (2012). Anti-oxidant, anti-inflammatory and immunomodulating properties of an enzymatic protein hydrolysate from yellow field pea seeds. Euro. J. Nutr. 51, 29–37. doi: 10.1007/s00394-011-0186-3
Newman, J., Egan, T., Harbourne, N., O'Riordan, D., Jacquier, J. C., and O'Sullivan, M. (2014a). Correlation of sensory bitterness in dairy protein hydrolysates: comparison of prediction models built using sensory, chromatographic and electronic tongue data. Talanta 126, 46–53. doi: 10.1016/j.talanta.2014.03.036
Newman, J., Harbourne, N., O'Riordan, D., Jacquier, J. C., and O'Sullivan, M. (2014b). Comparison of a trained sensory panel and an electronic tongue in the assessment of bitter dairy protein hydrolysates. J. Food Eng. 128, 127–131. doi: 10.1016/j.jfoodeng.2013.12.019
Ney, K. H. (1979). Bitterness of Peptides: Amino Acid Composition and Chain Length. ACS Publications. doi: 10.1021/bk-1979-0115.ch006
Nimalaratne, C., Bandara, N., and Wu, J. (2015). Purification and characterization of antioxidant peptides from enzymatically hydrolyzed chicken egg white. Food Chem. 188, 467–472. doi: 10.1016/j.foodchem.2015.05.014
Nwachukwu, I. D., and Aluko, R. E. (2019). Structural and functional properties of food protein-derived antioxidant peptides. J. Food Biochem. 43, 1–13. doi: 10.1111/jfbc.12761
Ogura, T., Margolskee, R. F., and Kinnamon, S. C. (2002). Taste receptor cell responses to the bitter stimulus denatonium involve Ca2+ influx via store-operated channels. J. Neurophysiol. 87, 3152–3155. doi: 10.1152/jn.2002.87.6.3152
Ohata, M., Uchida, S., Zhou, L., and Arihara, K. (2016). Antioxidant activity of fermented meat sauce and isolation of an associated antioxidant peptide. Food Chem. 194, 1034–1039. doi: 10.1016/j.foodchem.2015.08.089
Oriani, V. B., Alvim, I. D., Consoli, L., Molina, G., Pastore, G. M., and Hubinger, M. D. (2016). Solid lipid microparticles produced by spray chilling technique to deliver ginger oleoresin: structure and compound retention. Food Res. Int. 80, 41–19. doi: 10.1016/j.foodres.2015.12.015
Ou, S., and Peng, X. (2016). Angiotensin-I-converting enzyme inhibitory activities and in vivo antihypertensive effects of sardine protein hydrolysate. J. Food Sci. 81, H2831–H2284. doi: 10.1111/1750-3841.13508
Oun, R., Plumb, J., Rowan, E., and Wheate, N. (2013). Encapsulation of cisplatin by cucurbit[7] uril decreases the neurotoxic and cardiotoxic side effects of cisplatin. Toxicol. Lett. 221:S92. doi: 10.1016/j.toxlet.2013.05.121
Pak, V. V., Koo, M. S., Kasymova, T. D., and Kwon, D. Y. (2005). Isolation and identification of peptides from soy 11S-globulin with hypocholesterolemic activity. Chem. Nat. Compounds 41, 710–714. doi: 10.1007/s10600-006-0017-6
Parrado, J., Bautista, J., and Machado, A. (1991). Production of soluble enzymic protein hydrolyzate from industrially defatted nondehulled sunflower meal. J. Agric. Food Chem. 39, 447–450. doi: 10.1021/jf00003a004
Piovesana, S., Capriotti, A. L., Cavaliere, C., La Barbera, G., Montone, C. M., Zenezini Chiozzi, R., et al. (2018). Recent trends and analytical challenges in plant bioactive peptide separation, identification and validation. Anal. Bioanal. Chem. 410, 3425–3444. doi: 10.1007/s00216-018-0852-x
Pooja, K., Rani, S., Kanwate, B., and Pal, G. K. (2017). Physico-chemical, sensory and toxicity characteristics of dipeptidyl peptidase-IV inhibitory peptides from rice bran-derived globulin using computational approaches. Int. J. Pept. Res. Ther. 23, 519–529. doi: 10.1007/s10989-017-9586-4
Pu, C., and Tang, W. (2017). The antibacterial and antibiofilm efficacies of a liposomal peptide originating from rice bran protein against listeria monocytogenes. Food Funct. 11, 4159–4169. doi: 10.1039/C7FO00994A
Rahman, M. M., Abdullah, R. B., Wan Embong, W. K., Nakagawa, T., and Akashi, R. (2013). Effect of palm kernel cake as protein source in a concentrate diet on intake, digestibility and live weight gain of goats fed napier grass. Trop. Anim. Health Product. 45, 873–878. doi: 10.1007/s11250-012-0300-4
Rajapakse, N., Mendis, E., Jung, W. K., Je, J. Y., and Kim, S. K. (2005). Purification of a radical scavenging peptide from fermented mussel sauce and its antioxidant properties. Food Res. Int. 38, 175–182. doi: 10.1016/j.foodres.2004.10.002
Raksakulthai, R., and Haard, N. F. (2003). Exopeptidases and their application to reduce bitterness in food: a review. Crit. Rev. Food Sci. Nutr. 43, 401–445. doi: 10.1080/10408690390826572
Rawlings, N. D., Barrett, A. J., Thomas, P. D., Huang, X., Bateman, A., and Finn, R. D. (2018). The MEROPS database of proteolytic enzymes, their substrates and inhibitors in 2017 and a comparison with peptidases in the PANTHER database. Nucleic Acids Res. 46, D624–D632. doi: 10.1093/nar/gkx1134
Rayaprolu, S. J., Hettiarachchy, N. S., Chen, P., Kanna, A., and Mauromoskakos, A. (2013). Mauromostakos peptides derived from high oleic acid soybean meals inhibit colon, liver and lung cancer cell growth. Food Res. Int. 50, 282–288. doi: 10.1016/j.foodres.2012.10.021
Rizzello, C. G., Tagliazucchi, D., Babini, E., Sefora Rutella, G., Taneyo Saa, D. L., and Gianotti, A. (2016). Bioactive peptides from vegetable food matrices: research trends and novel biotechnologies for synthesis and recovery. J. Funct. Foods 27, 549–569. doi: 10.1016/j.jff.2016.09.023
Roy, F., Boye, J. I., and Simpson, B. K. (2010). Bioactive proteins and peptides in pulse crops: pea, chickpea and lentil. Food Res. Int. 43, 432–442. doi: 10.1016/j.foodres.2009.09.002
Ruiz-Ruiz, J., Dávila-Ortíz, G., Chel-Guerrero, L., and Betancur-Ancona, D. (2013). Angiotensin I-converting enzyme inhibitory and antioxidant peptide fractions from hard-to-cook bean enzymatic hydrolysates. J. Food Biochem. 37, 26–35. doi: 10.1111/j.1745-4514.2011.00594.x
Salvim, M. O., Thomazini, M., Pelaquim, F. P., Urbano, A., Moraes, I. C. F., and Favaro-Trindade, C. S. (2015). Production and structural characterization of solid lipid microparticles loaded with soybean protein hydrolysate. Food Res. Int. 76 (Pt 3), 689–696. doi: 10.1016/j.foodres.2015.08.003
Sarabandi, K., Gharehbeglou, P., and Jafari, S. M. (2020). Spray-drying encapsulation of protein hydrolysates and bioactive peptides: opportunities and challenges. Dry. Technol. 38, 577–595. doi: 10.1080/07373937.2019.1689399
Sarabandi, K., Mahoonak, A. S., Hamishekar, H., Ghorbani, M., and Jafari, S. M. (2018). Microencapsulation of casein hydrolysates: physicochemical, antioxidant and microstructure properties. J. Food Eng. 237, 86–95. doi: 10.1016/j.jfoodeng.2018.05.036
Sarmadi, B., Ismail, A., and Hamid, M. (2011). Antioxidant and angiotensin converting enzyme (ACE) inhibitory activities of cocoa (Theobroma cacao L.) autolysates. Food Res. Int. 44 290–296. doi: 10.1016/j.foodres.2010.10.017
Sarmadi, B. H., and Ismail, A. (2010). Antioxidative peptides from food proteins: a review. Peptides 31, 1949–1956. doi: 10.1016/j.peptides.2010.06.020
Sayin, V., Ibrahim, M. X., Larsson, E., Nilsson, J. A., Lindahl, P., and Bergo, M. O. (2014). Antioxidants accelerate lung cancer progression in mice. Sci. Transl. Med. 6:221. doi: 10.1126/scitranslmed.3007653
Schomburg, I., Jeske, L., Ulbrich, M., Placzek, S., Chang, A., and Schomburg, D. (2017). The BRENDA enzyme information system–from a database to an expert system. J. Biotechnol. 261, 194–206. doi: 10.1016/j.jbiotec.2017.04.020
Segerstrom, S. C., and Miller, G. E. (2004). Psycological stress and the human immune system: a meta analytic study of 30 years of inquiry. Psychol. Bull. 130, 601–630. doi: 10.1037/0033-2909.130.4.601
Segura-Campos, M. R., Salazar-Vega, I. M., Chel-Guerrero, L. A., and Betancur-Ancona, D. A. (2013). Biological potential of chia (Salvia hispanica L.) protein hydrolysates and their incorporation into functional foods. LWT Food Sci. Technol. 50, 723–731. doi: 10.1016/j.lwt.2012.07.017
Seo, W. H., Lee, H. G., and Baek, H. H. (2008). Evaluation of bitterness in enzymatic hydrolysates of soy protein isolate by taste dilution analysis. J. Food Sci. 73, S41–S46 doi: 10.1111/j.1750-3841.2007.00610.x
Sheih, I. C., Fang, T. J., Wu, T., and Lin, P. (2010). Anticancer and antioxidant activities of the peptide fraction from algae protein waste. J. Agric. Food Chem. 58, 1202–1207. doi: 10.1021/jf903089m
Shtatland, T., Guettler, D., Kossodo, M., Pivovarov, M., and Weissleder, R. (2007). PepBank–a database of peptides based on sequence text mining and public peptide data sources. BMC Bioinformatics 8:280. doi: 10.1186/1471-2105-8-280
Siemensm, A. D., Weijer, W. J., and Bak, H. J. (1993). The importance of peptide lengths in hypoallergenic infant formulae. Trends Food Sci. Technol. 4, 16–21. doi: 10.1016/S0924-2244(05)80006-8
Singh, A., Idowu, A. T., Benjakul, S., Kishimura, H., Aluko, R. E., and Kumagai, Y. (2020). Debittering of salmon (Salmo salar) frame protein hydrolysate using 2-butanol in combination with β-cyclodextrin: impact on some physicochemical characteristics and antioxidant activities. Food Chem. 321:126686. doi: 10.1016/j.foodchem.2020.126686
Sinthusamran, S., Idowu, A. T., Benjakul, S., Prodpran, T., Yesilsu, A. F., and Kishimura, H. (2020). Effect of proteases and alcohols used for debittering on characteristics and antioxidative activity of protein hydrolysate from salmon frames. J. Food Sic. Technol. 57, 473–483. doi: 10.1007/s13197-019-04075-z
Siow, H.-L., Choi, S.-B., and Gan, C.-Y. (2016). Structure-Activity studies of protease activating, lipase inhibiting, bile acid binding and cholesterol-lowering effects of pre-screened cumin seed bioactive peptides. J. Funct. Foods 27, 600–611. doi: 10.1016/j.jff.2016.10.013
Soltani, S., Haghaei, H., Shayanfar, A., Vallipour, J., Asadpour Zeynali, K., and Jouyban, A. (2013). QSBR study of bitter taste of peptides: application of GA-PLS in combination with MLR, SVM, and ANN approaches. Biomed. Res. Int. 2013:501310. doi: 10.1155/2013/501310
Song, N., Tan, C., Huang, M., Liu, P., Eric, K., Zhang, X., et al. (2013). Transglutaminase cross-linking effect on sensory characteristics and antioxidant activities of maillard reaction products from soybean protein hydrolysates. Food Chem. 136, 144–151. doi: 10.1016/j.foodchem.2012.07.100
Steinberg, D., and Witztum, J. L. (2009). Inhibition of PCSK9: a powerful weapon for achieving ideal LDL cholesterol levels. Proc. Natl. Acad. Sci. U.S.A. 106, 9546–9547. doi: 10.1073/pnas.0904560106
Stressler, T., Eisele, T., Baur, C., Wangler, J., Kuhn, A., and Fischer, L. (2015). Extracellular peptidases from insect-and compost-associated microorganisms: screening and usage for wheat gluten hydrolysis. Euro. Food Res. Technol. 241, 263–274. doi: 10.1007/s00217-015-2452-8
Stressler, T., Eisele, T., Ewert, J., Kranz, B., and Fischer, L. (2019). Proving the synergistic effect of Alcalase, PepX and PepN during casein hydrolysis by complete degradation of the released opioid precursor peptide VYPFPGPIPN. Euro. Food Res. Technol. 245, 61–71. doi: 10.1007/s00217-018-3140-2
Stressler, T., Eisele, T., Kranz, B., and Fischer, L. (2014). PepX from Lactobacillus helveticus: automated multi-step purification and determination of kinetic parameters with original tripeptide substrates. J. Mol. Catal. 108, 103–110. doi: 10.1016/j.molcatb.2014.07.006
Stressler, T., Eisele, T., Schlayer, M., Lutz-Wahl, S., and Fischer, L. (2013). Characterization of the recombinant exopeptidases PepX and PepN from Lactobacillus helveticus ATCC 12046 important for food protein hydrolysis. PLOS ONE 8:e70055. doi: 10.1371/journal.pone.0070055
Supawong, S., Thawornchinsombut, S., and Park, J. W. (2018). Controlling lipid oxidation and volatile compounds in frozen fried fish cake prepared with rice bran hydrolysate. J. Aquat. Food Product Technol. 27, 885–899. doi: 10.1080/10498850.2018.1508103
Timilsena, Y. P., Akanbi, T. O., Khalid, N., Adhikari, B., and Barrow, C. J. (2019). Complex coacervation: principles, mechanisms and applications in microencapsulation. Int. J. Biol. Macromol. 121, 1276–1286. doi: 10.1016/j.ijbiomac.2018.10.144
Toldrá, F., Reig, M., Aristoy, M. C., and Mora, L. (2018). Generation of bioactive peptides during food processing. Food Chem. 267, 395–404. doi: 10.1016/j.foodchem.2017.06.119
Tonolo, F., Moretto, L., Folda, A., Scalcon, V., Bindoli, A., Bellamio, M., et al. (2019). Antioxidant properties of fermented soy during shelf life. Plant Foods Hum. Nutr. 74, 287–292. doi: 10.1007/s11130-019-00738-6
Tulini, F. L., Souza, V. B., Echalar-Barrientos, M. A., Thomazini, M., Pallone, E. M. J. A., and Favaro-Trindade, C. S. (2016). Development of solid lipid microparticles loaded with a proanthocyanidinrich cinnamon extract (Cinnamomum zeylanicum): potential for increasing antioxidant content in functional foods for diabetic population. Food Res. Int. 85, 10–18. doi: 10.1016/j.foodres.2016.04.006
Udenigwe, C. C., and Aluko, R. E. (2012). Food protein-derived bioactive peptides: production, processing, and potential health benefits. J. Food Sci. 77, R11–R24. doi: 10.1111/j.1750-3841.2011.02455.x
Udenigwe, C. C., Lu, Y. L., Han, C. H., Hou, W. C., and Aluko, R. E. (2009). Flaxseed protein-derived peptide fractions: antioxidant properties and inhibition of lipopolysaccharide-induced nitric oxide production in murine macrophages. Food Chem. 116, 277–284. doi: 10.1016/j.foodchem.2009.02.046
Umadevi, P., Soumya, M., George, J. K., and Anandaraj, M. (2018). Proteomics assisted profiling of antimicrobial peptide signatures from black pepper (Piper nigrum L.). Physiol. Mol. Biol. Plants 24, 379–387. doi: 10.1007/s12298-018-0524-5
Van Acker, T., Van Malderen, S. J., Van Heerden, M., McDuffie, J. E., Cuyckens, F., and Vanhaecke, F. (2016). High-resolution laser ablation-inductively coupled plasma-mass spectrometry imaging of cisplatin-induced nephrotoxic side effects. Anal. Chim. Acta 945, 23–30. doi: 10.1016/j.aca.2016.10.014
Vital, D. A. L., de Mejía, E. G., Dia, V. P., and Loarca-Piña, G. (2014). Peptides in common bean fractions inhibit human colorectal cancer cells. Food Chem. 157, 347–355. doi: 10.1016/j.foodchem.2014.02.050
Vlasov, Y., Legin, A., Rudnitskaya, A., Di Natale, C., and D'Amico, A. (2005). Nonspecific sensor arrays (electronic tongue) for chemical analysis of liquids (IUPAC technical report). Pure Appl. Chem. 77, 1965–1983. doi: 10.1351/pac200577111965
Walters, M., Esfandi, R., and Tsopmo, A. (2018). Potential of food hydrolyzed proteins and peptides to chelate iron or calcium and enhance their absorption. Foods 7:172. doi: 10.3390/foods7100172
Wang, J., Li, C., Xue, J., Yang, J., Zhang, Q., Zhang, H., et al. (2015). Fermentation characteristics and angiotensin I-converting enzyme–inhibitory activity of Lactobacillus helveticus isolate H9 in cow milk, soy milk, and mare milk. J. Dairy Sci. 98, 3655–3664. doi: 10.3168/jds.2015-9336
Wang, L., Zhang, J., Yuan, Q., Xie, H., Shi, J., and Ju, X. (2016). Separation and purification of an anti-tumor peptide from rapeseed (Brassica campestris L.) and the effect on cell apoptosis. Food Funct. 7, 2239–2248. doi: 10.1039/C6FO00042H
Wang, X., Chen, H., Fu, X., Li, S., and Wei, J. (2017). A novel antioxidant and ACE inhibitory peptide from rice bran protein: Biochemical characterization and molecular docking study. LWT Food Sci. Technol. 75, 93–99. doi: 10.1016/j.lwt.2016.08.047
Wang, Z., and Zhang, X. (2017). Isolation and identification of anti-proliferative peptides from Spirulina platensis using three-step hydrolysis. J. Sci. Food Agric. 97, 918–922. doi: 10.1002/jsfa.7815
Wiener, A., Shudler, M., Levit, A., and Niv, M. Y. (2012). BitterDB: a database of bitter compounds. Nucleic Acids Res. 40, D413–D419. doi: 10.1093/nar/gkr755
Wong, G. T., Gannon, K. S., and Margolskee, R. F. (1996). Transduction of bitter and sweet taste by gustducin. Nature 381, 796–800. doi: 10.1038/381796a0
World Health Organisation (2021). Hypertesion. Available online at: https://www.who.int/news-room/fact-sheets/detail/hypertension
Worsztynowicz, P., Białas, W., and Grajek, W. (2020). Integrated approach for obtaining bioactive peptides from whey proteins hydrolysed using a new proteolytic lactic acid bacteria. Food Chem. 312:126035. doi: 10.1016/j.foodchem.2019.126035
Wu, H., Rui, X., Li, W., Xiao, Y., Zhou, J., and Dong, M. (2018). Whole-grain oats (Avena sativa L.) as a carrier of lactic acid bacteria and a supplement rich in angiotensin I-converting enzyme inhibitory peptides through solid-state fermentation. Food Funct. 9, 2270–2281. doi: 10.1039/C7FO01578J
Wu, H. C., Chen, H. M., and Shiau, C. Y. (2003). Free amino acids and peptides as related to antioxidant properties in protein hydrolysates of mackerel (Scomber austriasicus). Food Res. Int. 36, 949–957. doi: 10.1016/S0963-9969(03)00104-2
Wu, J., Aluko, R. E., and Nakai, S. (2006). Structural requirements of angiotensin I-converting enzyme inhibitory peptides: quantitative structure–activity relationship study of Di- and tripeptides. J. Agric. Food Chem. 54, 732–738. doi: 10.1021/jf051263l
Wu, J., and Ding, X. (2002). Characterization of inhibition and stability of soy-proteinderived angiotensin I-converting enzyme inhibitory peptides. Food Res. Int. 35, 367–375. doi: 10.1016/S0963-9969(01)00131-4
Wu, W., Zhang, M., Ren, Y., Cai, X., Yin, Z., Zhang, X., et al. (2017). Characterization and immunomodulatory activity of a novel peptide, ECFSTA, from wheat germ globulin. J. Agric. Food Chem. 65, 5561–5569. doi: 10.1021/acs.jafc.7b01360
Wu, W., Zhang, M., Sun, C., Brennan, M., Li, H., Wang, G., et al. (2016). Enzymatic preparation of immunomodulatory hydrolysates from defatted wheat germ (Triticum vulgare) globulin. Int. J. Food Sci. Technol. 51, 2556–2566. doi: 10.1111/ijfs.13238
Xiao, J., and Zhang, H. (2012). An escherichia coli cell membrane chromatography-offline LC-TOF-MS method for screening and identifying antimicrobial peptides from Jatropha curcas meal protein isolate hydrolysates. J. Biomol. Screen. 17, 752–760. doi: 10.1177/1087057112442744
Xiao, Y., Sun, M., Zhang, Q., Chen, Y., Miao, J., Rui, X., et al. (2018). Effects of Cordyceps militaris (L.) Fr. fermentation on the nutritional, physicochemical, functional properties and angiotensin I converting enzyme inhibitory activity of red bean [Phaseolus angularis (Willd.) W.F. Wight.] flour. J. Food Sci. Technol. 55, 1244–1255. doi: 10.1007/s13197-018-3035-z
Xie, H., Wang, Y., Zhang, J., Chen, J., Wu, D., and Wang, L. (2015). Study of the fermentation conditions and the antiproliferative activity of rapeseed peptides by bacterial and enzymatic cooperation. Int. J. Food Sci. 50, 619–625. doi: 10.1111/ijfs.12682
Xu, N., Chen, G., and Liu, H. (2017). Antioxidative categorization of twenty amino acids based on experimental evaluation. Molecules 22:2066. doi: 10.3390/molecules22122066
Xu, Q., Singh, N., Hong, H., Yan, X., Yu, W., Jiang, X., et al. (2019). Hen protein-derived peptides as the blockers of human bitter taste receptors T2R4, T2R7 and T2R14. Food Chem. 283, 621–627. doi: 10.1016/j.foodchem.2019.01.059
Xue, Z., Wen, H., Zhai, L., Yu, Y., Li, Y., Yu, W., et al. (2015). Antioxidant activity and anti-proliferative effect of a bioactive peptide from chickpea (Cicer arietinum L.). Food Res. Int. 77, 75–81. doi: 10.1016/j.foodres.2015.09.027
Xue, Z., Yu, W., Wu, M., and Wang, J. (2009). In vivo antitumor and antioxidative effects of a rapeseed meal protein hydrolysate on an s180 tumor-bearing murine model Biosci. Biotechnol. Biochem. 73, 2412–2415. doi: 10.1271/bbb.90374
Yadavalli, S. S., Carey, J. N., Leibman, R. S., Chen, A. I., Stern, A. M., Roggiani, M., et al. (2016). Antimicrobial peptides trigger a division block in Escherichia coli through stimulation of a signalling system. Nat. Commun. 7:12340. doi: 10.1038/ncomms12340
Yang, S., Mao, X.-Y., Li, F.-F., Zhang, D., Leng, X.-J., Ren, F.-Z., et al. (2012). The improving effect of spray-drying encapsulation process on the bitter taste and stability of whey protein hydrolysate. Euro. Food Res. Technol. 235, 91–97. doi: 10.1007/s00217-012-1735-6
Yang, S., Yunden, J., Sonoda, S., Doyama, N., Lipkowski, A. W., Kawamura, Y., et al. (2001). Rubiscolin, a δ selective opioid peptide derived from plant rubisco. FEBS Lett. 509, 213–217. doi: 10.1016/S0014-5793(01)03042-3
Yang, Y., Zhao, X., Li, J., Jiang, H., Shan, X., Wang, Y., et al. (2018). A β-glucan from durvillaea antarctica has immunomodulatory effects on RAW264.7 macrophages via toll-like receptor 4. Carbohyd. Polym. 191, 255–265. doi: 10.1016/j.carbpol.2018.03.019
Yin, J., Diao, Y., Wen, Z., Wang, Z., and Li, M. (2010). Studying peptides biological activities based on multidimensional descriptors (E) using support vector regression. Int. J. Peptide Res. Ther. 16, 111–121. doi: 10.1007/s10989-010-9210-3
Ying, X., Gao, J., Liu, J., Ma, C., Lv, C., Adhikari, B., et al. (2021). Preparation and drying of water-in-oil-in-water (W/O/W) double emulsion to encapsulate soy peptides. Food Res. Int. 141:110148. doi: 10.1016/j.foodres.2021.110148
Yoshikawa, M., Takahashi, M., and Yang, S. (2003). Delta opioid peptides derived from plant proteins. Curr. Pharm. Des. 9, 1325–1330. doi: 10.2174/1381612033454838
Yu, D., Feng, M. Q., Sun, J., Xu, X. L., and Zhou, G. H. (2020). Protein degradation and peptide formation with antioxidant activity in pork protein extracts inoculated with Lactobacillus plantarum and Staphylococcus simulans. Meat. Sci. 160, 1–8. doi: 10.1016/j.meatsci.2019.107958
Yu, L., Guo, J., Yi, G., and Yu, Q. (2013). Optimization of soybean-peptide beverages formulation by using fuzzy logical sensory evaluation. Adv. Mater. Res. 781–784, 1540–1545. doi: 10.4028/www.scientific.net/AMR.781-784.1540
Zamyatnin, A. A., Borchikov, A. S., Vladimirov, M. G., and Voronina, O. L. (2006). The EROP-moscow oligopeptide database. Nucleic Acids Res. 34 (Suppl. 1), D261–D266. doi: 10.1093/nar/gkj008
Zarei, M., Ebrahimpour, A., Abdul-Hamid, A., Anwar, F., and Saari, N. (2012). Production of defatted palm kernel cake protein hydrolysate as a valuable source of natural antioxidants. Int. J. Mol. Sci. 13, 8097–8111. doi: 10.3390/ijms13078097
Zhang, C., Alashi, A. M., Singh, N., Liu, K., Chelikani, P., and Aluko, R. E. (2018). Beef protein-derived peptides as bitter taste receptor t2r4 blockers. J. Agric. Food Chem. 66, 4902–4912. doi: 10.1021/acs.jafc.8b00830
Zhang, H., Yokoyama, W. H., and Zhang, H. (2012). Concentration-dependent displacement of cholesterol in micelles by hydrophobic rice bran protein hydrolysates. J. Sci. Food Agric. 92, 1395–1401. doi: 10.1002/jsfa.4713
Zhang, N., Zhang, C., Chen, Y., and Zheng, B. (2016). Purification and characterization of antioxidant peptides of pseudosciaena crocea protein hydrolysates. Molecules 22:57. doi: 10.3390/molecules22010057
Zhuang, H., Tang, N., and Yuan, Y. (2013). Purification and identification of antioxidant peptides from corn gluten meal. J. Funct. Foods 5, 1810–1821. doi: 10.1016/j.jff.2013.08.013
Keywords: peptides, flavour masking, encapsulation, protein, enzymatic hydrolysis, nutraceuticals
Citation: Ying X, Agyei D, Udenigwe C, Adhikari B and Wang B (2021) Manufacturing of Plant-Based Bioactive Peptides Using Enzymatic Methods to Meet Health and Sustainability Targets of the Sustainable Development Goals. Front. Sustain. Food Syst. 5:769028. doi: 10.3389/fsufs.2021.769028
Received: 01 September 2021; Accepted: 22 October 2021;
Published: 17 November 2021.
Edited by:
Osvaldo H. Campanella, Purdue University, United StatesReviewed by:
Michelle Lisa Colgrave, Commonwealth Scientific and Industrial Research Organisation (CSIRO), AustraliaAyodeji B. Oyenihi, Cape Peninsula University of Technology, South Africa
Copyright © 2021 Ying, Agyei, Udenigwe, Adhikari and Wang. This is an open-access article distributed under the terms of the Creative Commons Attribution License (CC BY). The use, distribution or reproduction in other forums is permitted, provided the original author(s) and the copyright owner(s) are credited and that the original publication in this journal is cited, in accordance with accepted academic practice. No use, distribution or reproduction is permitted which does not comply with these terms.
*Correspondence: Bo Wang, Ym8ud2FuZ0BhY3UuZWR1LmF1