- 1Department of Food and Human Nutritional Sciences, University of Manitoba, Winnipeg, MB, Canada
- 2Department of Food Science and Technology, Federal University of Technology, Minna, Nigeria
- 3Department of Home Economics, College of Education, Katsina-Ala, Nigeria
- 4Department of Food Science and Technology, University of Agriculture Makurdi, Makurdi, Nigeria
Defatted peanut meal is a low value agro-industrial residue from peanut oil production with potential use as a value addition food ingredient. In this study, peanuts were roasted at 100°C for 5 min, de-skinned and milled into whole peanut flour (WPF) from which the defatted meal (DPM) was prepared by acetone extraction and the peanut protein concentrate (PPC) obtained from the DPM using isoelectric pH precipitation. The protein content, amino acid profile, total phenolic content (TPC), total flavonoid content (TFC) and in vitro antioxidant properties of the peanut samples were then determined. Results showed that DPM had a TPC of 0.12 ± 0.02 mg gallic acid equivalent (GAE)/g, which was significantly (p < 0.05) higher than and twice the levels in WPF and PPC (0.06 ± 0.03 mg GAE/g). However, WPF had TFC of 0.21 ± 0.01 μg quercetin equivalent (QE)/g, which was significantly (p < 0.05) higher than DPM (0.16 ± 0.03 μg QE/g) and PPC (0.11 ± 0.05 μg QE/g). However, PPC had superior amino acid profile in addition to stronger radical scavenging and metal chelation activities than WPF and DPM. The results suggest that PPC is a protein rich product that could be utilized as an ingredient in food product fortification to enhance nutritional quality and in the formulation of functional foods with antioxidant benefits.
Introduction
Peanuts (Arachis hypogaea), also known as groundnuts are protein-rich edible oilseed legumes grown in the tropical and subtropical regions. Peanut is widely consumed globally in many forms and its demand or acceptance as food comes mainly from the flavor attributes and nutritional content. Nuts are rich in both macro and micronutrients and the daily consumption leads to long-term health benefits (Ros, 2010; Stevens-Barrón et al., 2019). Peanuts could be a good replacement for tree nuts especially for low-income communities and underdeveloped economies since both have similar morphology and nutritional profile. Comparatively, the market price of peanut is lowest among nuts but like tree nuts, peanuts have a complete essential amino acid profile almost in equivalent quality to animal proteins but with high level of unsaturated fatty acids (Arya et al., 2016; Wang et al., 2016). The entirety of the peanut plant is a rich conglomerate of phenolic compounds and the kernel, testa, hull and by-products are used in various food formulations (Arya et al., 2016; Toomer, 2020). For example, peanuts serve as a commercial source of raw material for the production of vegetable oil, roasted snacks, pasta, flour, beverages and many more other products (Toomer, 2018). Recent studies have suggested that peanuts could be used in composite flour for the production of flatbread (Salve et al., 2020), animal feeds (Duodu et al., 2018), food packaging (Riveros et al., 2018), and probiotics supplements (Klu et al., 2014). The interest in phenolic compounds derived from vegetables and their biological roles in nutrition and disease reduction is increasing (Giada, 2013; Gutiérrez-Grijalva, 2016).
Plants are potential sources of natural antioxidants and several studies have focused on the bioactive activities of phenolics, which involve their ability to act as potent antioxidants and free radical scavengers (Alov et al., 2015; Tungmunnithum et al., 2018; Kaurinovic and Vastag, 2019). Peanuts are rich in several vitamins and minerals, unsaturated fatty acids and fiber and these together with the phytochemicals contribute to promote health and reduce the risk of chronic diseases by functioning as antioxidants, antimicrobials, anti-inflammatory, anti-hypertension and anti-cancer agents (Chen and Blumberg, 2008; Prabasheela et al., 2015). Furthermore, current knowledge on the effects of nut consumption on human health has greatly increased in recent years and suggests that they may play a role in the prevention and management of chronic age-related diseases. Hence, frequent consumption of nuts is advised for better metabolic status, lower risk of coronary disease, and reduced risk of obesity (Ros, 2010; Rusu et al., 2019). This is because bioactive compounds in them have the potent capacity to prevent, ameliorate and manage diseases, thus promoting human longevity (De Camargo et al., 2017).
The defatted peanut meal is a low value agro-industrial residue derived from oil production and has some potential for valorization (Uddin et al., 2018). The meal contains ~50% protein, minerals, vitamins, fiber and phenolic acids, resveratrol, flavonoids and phytosterols (Bhat et al., 2019). High phenolic content remains in the defatted residues and these by-products could be useful in food fortification (De Camargo et al., 2014) and could be novel ingredients for the development of functional foods and nutraceuticals (Arya et al., 2016). The main phenolic compounds found in nuts are flavonoids (proanthocyanidin, isoflavones, catechin and epicatechin) and phenolic acids such as sinapic, vanillic, caffeic, m-coumaric, o-coumaric and ferulic acids (Attree et al., 2015; Ma et al., 2019). Peanuts have a good quantity of lipophilic phenolics than most nuts including tree nuts (De Camargo et al., 2017; Stevens-Barrón et al., 2019). Most phenolic compounds extracted from nuts are the hydrophilic type, however the lipophilic compounds possess several biological activities (Liu et al., 2014). Peanut meals are by-products of peanut oil processing, which removes tocopherols and some phospholipids from the product (Zhao et al., 2012). A strong positive correlation (R2 > 0.93) has been observed between the antioxidant of activity and total phenolic content of plant materials (Kchaou et al., 2014). Phenolic compounds exhibit radical scavenging activities and also mitigate lipid oxidation in foods as a result of their antioxidant properties, which are wholly related to their chemical structure. The antioxidant and free radical activities of phenolics are mainly due to their redox properties, which allows them to act as reducing agents, hydrogen/electron donors and singlet oxygen quenchers (Kasote et al., 2015; Kumari, 2017). Consumption of foods containing appreciable amounts of phenolic compounds could therefore, improve the antioxidant status of an individual by facilitating donation of electrons and scavenging of free radicals, especially the highly toxic reactive oxygen species (Moukette et al., 2015). Phuyal et al. (2020) reported that natural antioxidants present in several medicinal plants are responsible for inhibiting the destructive effects of oxidative stress. These plants contain phenols and flavonoids that act as free radical scavengers and reduce oxidative stress and may be an alternative remedy to curing various harmful human diseases. In addition, acting as antioxidants, TPC and TFC have been shown to produce other biological effects such as anti-inflammatory, antibacterial, antifungal, antimalarial, cytotoxicity and antiprotozoal activities (Larit et al., 2019).
Peanuts have been reported to contain antinutrients including trypsin inhibitor, α-amylase inhibitor, phytic acid, lectin and tannins (Lozano et al., 2019). Embaby (2011) investigated the effect of four heat processing methods (boiling, autoclaving, microwave and roasting) on six antinutrtional factors (trypsin inhibitor, α-amylase inhibitor, phytic acid, lectin and tannins) found in peanuts. Results showed that all the heat treatments significantly reduced the levels of the investigated antinutrients and improved in vitro protein digestibility of peanut seeds. Of all the heat treatments, roasting was the most effective in reducing the levels of antinutrients and improving the in vitro protein digestibility of peanut (Embaby, 2011).
Since antioxidants play an enormous physiological role in human and animals health, literature is replete with studies on the phytochemical content and bioactive role of nuts (Thakur et al., 2020). Some recent studies on peanut and by-products focused on few phytochemical components of agricultural residues like husk and hull. The use of peanut by-products in animal feed and human food, impact of different processes on peanut phytochemical content, peanut protein isolation and functional properties and nutritional components of the defatted meal have been evaluated with the aim of improving the final product nutrient quality (Jain et al., 2015; Vijayalaxmi et al., 2015; Arya et al., 2016; Duodu et al., 2018; Guo et al., 2020). Although much work has been carried out to assess the phytochemical content and techno-functional properties of peanut and their by-products especially those of defatted protein meal and protein concentrate (Yu et al., 2007; Kain and Chen, 2010), only few of the studies carried out a comparative study of the total phytochemical content and antioxidant properties of peanut by-products. Hence, the objective of this study was to compare the nutritional quality (amino acid composition), total phytochemical content and in vitro antioxidant properties of whole peanut protein flour (WPF), defatted peanut protein meal (DPM) and peanut protein concentrate (PPC).
Materials and Methods
Materials
Peanut seeds were purchased from the Minna main market, Niger State, Nigeria. All analytical grade reagents were purchased from Fisher Scientific (Oakville, ON, Canada) and Sigma Chemicals (St. Louis, MO, USA).
Sample Preparation
Preparation of Whole Peanut Flour
Whole peanut protein meal is the product obtained from roasted peanut seeds ground into flour prior to defatting. To produce the WPF, the peanut pods were separately cleaned of extraneous materials and the extracted seeds roasted at 100°C for 15 min with stirring to ensure uniform heat distribution. The roasted seeds were cooled to room temperature (RT), manually de-skinned and winnowed to obtain the naked seeds (seeds without the testa). The toasted, de-skinned and winnowed seeds were then milled using a manual grinder and sieved into a fine flour of 1 mm particle pore size. This flour was referred to as the WPF.
Preparation of Defatted Peanut Meal
The DPM was prepared using a previously described protocol (Girgih et al., 2016). The milled peanut protein flour was defatted by mixing 1 g of WPF with 10 mL of acetone. The mixture was then stirred in the fume hood for 3 h and decanted followed by second and third consecutive extractions of the residues. The resulting DPM from the third extraction was air-dried overnight in a fume hood and stored at −20°C.
Preparation of Peanut Protein Concentrate
The method of Yu et al. (2007) was adopted with some minor modifications to produce PPC as follows. DPM was dispersed in distilled water using the ratio of 1:10 (w:v, solid:liquid) and adjusted to pH 10 with 1 M NaOH solution. The alkaline mixture was continuously stirred for 1 h at RT followed by centrifugation (5000g for 20 min). The supernatant was collected and adjusted to pH 4.5 by adding 1 M HCl solution to precipitate the proteins. The suspension was centrifuged (5000g; 20 min; RT) after which the supernatant was discarded while the precipitate was collected and re-suspended in distilled water (1:10, solid:liquid). The mixture was stirred at RT for 1 h to remove salts and other soluble contaminants and then centrifuged again (5000g; 20 min; RT). The washed precipitate was freeze-dried, milled into powder to give the peanut protein concentrate (PPC) and then stored at −20°C. Protein contents of WPF, DPM and PPC were determined using the Lowry method (Markwell et al., 1978).
Determination of Phytochemical Content
Total Phenolic Content
The TPC was determined using Folin-Ciocalteu reagent following a previously described procedure (Adhikari et al., 2018) with some modifications. Briefly, 50 μL of sample extracts were mixed with 1 mL of Na2CO3 (2%, w/v) using a vortex. A 50 μL aliquot of 1 M Folin-Ciocalteu reagent was then added to the mixture and allowed to stand for 30 min at RT in the dark. The absorbance was taken at 750 nm using a spectrophotometer (Multiskan GO, Thermo Fischer Scientific). TPC was determined using gallic acid as a standard and the results were expressed as microgram gallic acid equivalents (GAE) per gram dry weight sample (μg GAE/g dry weight sample).
Total Flavonoid Content
The TFC was measured following the method described by Adhikari et al. (2018) with some modifications. A 300 μL aliquot of sample solution was mixed with 300 μL of double distilled water and 30 μL of NaNO2 (5%, w/v) added. The mixture was left at room temperature for 5 min and then 60 μL of AlCl3 (10%, w/v) was added to the mixture. After 5 min, 200 μL of 1 M NaOH was added to the mixture and vortexed. The absorbance reading was then taken at 500 nm using a spectrophotometer (Multiskan GO, Thermo Fischer Scientific). The TFC was calculated from the calibration curve plotted using quercetin as a standard and expressed in microgram quercetin equivalents (QE) per gram dry weight sample (μg QE/g dry weight sample).
Determination of Amino Acid Composition
The amino acid profiles of the samples were determined using an HPLC system after samples were hydrolyzed with 6 M HCl (Bidlingmeyer et al., 1984). Amino acid quantification was then performed using a 4.6 × 150 mm ion-exchange chromatography column on a Sykam Amino Acid Analyzer, (Model S2100/S4300) following the procedures outlined by the manufacturer (Skyam GmbH, Eresing, Germany). The amino acids were separated using an elution gradient consisting of sodium citrate buffers (pH 3.45 and pH 10.85) at 0.45 mL/min flow rate. The cysteine and methionine contents were determined after performic acid oxidation (Gehrke et al., 1985) while the tryptophan content was determined after alkaline hydrolysis (Landry and Delhaye, 1992).
Determination of in vitro Antioxidant Properties
DPPH Radical Scavenging Activity
The DRSA of WPF, DPM and PPC was determined using a previous method (Adefegha et al., 2015) with slight modifications for 96-well clear flat bottom plate. Samples were dissolved in 0.1 M sodium phosphate buffer, pH 7.0 containing 1% (w/v) Triton X-100. DPPH was dissolved in methanol to a final concentration of 100 μM. Samples (100 μL) were mixed with 100 μL of the DPPH solution in the 96-well plate to a final assay concentration of 1 mg/mL and incubated at RT in the dark for 30 min. The absorbance values of the blank (Ab) and samples (As) were measured at 517 nm. The control consisted of sodium phosphate buffer in place of the sample while reduced glutathione (GSH) was used as the positive control. The percent DRSA of the samples was determined using the following equation:
Superoxide Radical Scavenging Activity
The SRSA of samples was measured according to previously described method (Xie et al., 2008). An aliquot of 1 mg/mL peanut samples or GSH (80 μL in 50 mM Tris-HCl buffer containing 1 mM EDTA, pH 8.3) was mixed with 80 μL of the buffer in a clear bottom 96-well plate in the dark. Then, 40 μL of 1.5 mM pyrogallol dissolved in 10 mM HCl was added to each well. A blank reaction was conducted without the sample. The reaction rate (ΔA/min) for the blank (b) and sample (s) was measured immediately at 420 nm for 4 min at room temperature using the buffer as a control. The SRSA was calculated using the following equation:
Hydroxyl Radical Scavenging Activity
The HRSA was determined based on a previously reported method (Kumari and Ra, 2017). WPF, DPM and PPC samples and 3 mM of 1,10-phenanthroline were separately dissolved in 0.1 M sodium phosphate buffer (pH 7.4). FeSO4 (3 mM) and 0.01% (v/v) hydrogen peroxide were both separately dissolved in distilled water. An aliquot (50 μL) of peanut samples (equivalent to a final assay concentration of 1 mg/mL) or buffer (blank) was first added to a clear, flat bottom 96-well plate followed by 50 μL of 1,10-phenanthroline and then 50 μL of FeSO4. To initiate the Fenton reaction, 50 μL of hydrogen peroxide was added to the mixture, covered and incubated at 37°C for 1 h with shaking. The absorbance was measured using a spectrophotometer at 536 nm at 10 min intervals for 1 h. The HRSA was then calculated using the reaction rate (ΔA/min) as follows:
where b and s represent blank and sample, respectively.
Ferric Reducing Antioxidant Power
The FRAP of samples was measured according to a previously reported method (Zhang et al., 2008), which was modified as follows. Peanut sample solution (250 μL) dissolved in 0.2 M sodium phosphate buffer at pH 6.6 or blank (250 μL of buffer) was mixed with 250 μL of same buffer and 250 μL of 1% (w/v) potassium ferricyanide solution. The peanut sample concentration in the assay mixture was 1 mg/mL, which was then heated at 50°C for 20 min. After incubation, 250 μL of 10% (w/v) aqueous trichloroacetic acid (TCA) was added. Thereafter, 250 μL was combined with 50 μL of 0.1% (w/v) FeCl3 solution and 200 μL of double distilled water and allowed to stand at RT for 10 min. The solution was then centrifuged at 1000 x g and 200 μL of the clear supernatant transferred to a 96-well plate for absorbance determination at 700 nm.
Metal Chelation Activity
The MCA of WPF, DPM and PPC samples was measured using a previously described method (Xie et al., 2008), which was modified as follows. An aliquot (1 mL) of a 1 mg/mL peanut sample solution or GSH was combined with 0.05 mL of 2 mM FeCl2 and 1.85 mL double distilled water in a reaction tube. A 0.1 mL aliquot of 5 mM ferrozine solution was added and mixed thoroughly. The mixture was left at room temperature for 10 min from which 200 μL was transferred into a 96-well plate. The blank reaction was performed by replacing sample with 1 mL of double distilled water. The absorbance values of both the blank (Ab) and samples (As) were measured at 562 nm using a spectrophotometer. The percentage MCA was calculated as follows:
Statistical Analysis
Phytochemical and antioxidant assays were conducted in triplicate and analyzed by one-way analysis of variance (ANOVA). The means were compared by Duncan's multiple range test and significant differences accepted at p < 0.05.
Results and Discussion
Total Phenolic and Flavonoid Contents
Phenolic compounds are known to play an important role in stabilizing lipids against peroxidation and inhibiting various types of oxidizing enzymes that promote oxidative stress and related morbidities (Forni et al., 2019; Liu J. et al., 2019). Results of the TPC and TFC of peanut samples indicate an effect of processing as shown in Table 1. The TPC value of DPM (0.12 mg GAE/g dry wt) was twice the values observed for WPF and PPC (0.06 mg GAE/g dry wt), which may have been caused by lipid removal coupled with the adopted processing method. These results are lower than TPC values reported for peanut testa that ranged between 2.47 and 84.53 mg GAE/g dry wt but similar to that of testa removed peanuts, which ranged from 0.07 to 0.12 mg GAE/g dry wt (Khaopha et al., 2012). Sebei et al. (2013) reported the TPC of peanut kernel with intact testa of four varieties in a range between 1.0 and 2.1 mg GAE/g dry wt. In another study, De Camargo et al. (2017) investigated the antioxidant capacity and antimicrobial effects of phenolics and flavonoids in peanut products and reported the TPC to be 150 and 3.61 mg CE/g dry wt in the peanut skin and blanched dry peanut meal. The TPC variations of peanut products could be attributed to the differences in cultivar, growth conditions, type of extracting solvents, ratio of solvent to sample and the duration of the extraction process (Sarkis et al., 2014; Coulibaly et al., 2018) as well as solubility of the sample material. Lower values of TFC were however observed in this study where WPF had TFC of 0.21 μg QE/g, which is significantly (p < 0.05) higher than DPM (0.16 μg QE/g) and PPC (0.11 μg QE/g). The low values of TFC could also be attributable to the processing and method of extraction and solvent used. Vijayalaxmi et al. (2015) extracted phenolic compounds from agricultural residues using two different solvent extraction methods and discovered variations arising from probably the solvents used and solubility of materials. Plant proteins generally exhibit low solubility and the presence of lipids in a flour could also alter the solubility by sequestering hydrophobic polyphenols, which could have contributed to the higher TPC of WPF compared to the DPM and PPC in which lipids were removed. The interactions between protein and phenolic compounds are made possible by the presence of several benzene rings and cyclic groups, which could result in complex formation that may cause unavailability of these species in food systems (Liu K. et al., 2019). Their interactions could affect the functional properties of proteins and phenolics. These phenomena could engineer the organoleptic properties of phenolic compounds and cause the foaming and gelling properties of proteins to be increased. In addition, during protein-phenolic interactions, both covalent and non-covalent bonds are involved but their formation depends on the type of phenolic compounds and proteins in the food system as well as conditions such as pH, temperature, ionic strengths and solubility (Altin et al., 2019). It is possible therefore that the results obtained in this study may have been influenced in part by the effects of protein-phenolic interactions.

Table 1. Phenolic, flavonoid, and protein contents of whole peanut protein flour, defatted peanut protein meal, and peanut protein concentrate*.
The major phenolic compounds in nuts are flavonoids, which are polyphenols and information from their chemical structure suggests they have the ability to scavenge free radicals and chelate redox active metals (Panche et al., 2016; Tungmunnithum et al., 2018). Table 1 shows that the TPC and TFC contents were directly related to degree of processing as defatting produced a decrease in their contents for DPM and PPC. Protein concentration resulted in further depletion of these polyphenolic compounds. These results suggest that TPC and TFC were lipid soluble, hence significant (p < 0.05) amounts were lost in DPM and PPC (during solvent pre-treatment) when compared to the intact WPF.
Protein Content
Peanuts have more protein than any other nut with levels comparable to or better than a serving of beans (Arya et al., 2016). Table 1 shows the protein content increased with processing, which was expected since each step involved the removal of mostly non-protein materials. The results correspond to literature values of reported increase in the protein content by at least 50% after defatting of peanut (Zhao et al., 2011). A similar report by Uddin et al. (2018) showed that the removal of lipids from raw peanuts increased the protein content from 25 to 53%, while Wu et al. (2009) reported 57% for defatted peanut meal. Furthermore, Yu et al. (2007) reported that protein content of defatted peanut meal was 53% while that of PPC derived from the defatted meal was 86%. However, higher values of protein content ranging from 80 to 90% (Sibt-e-Abbas et al., 2015) and up to 97% (Wu et al., 2009) have been reported for isolated peanut proteins.
Amino Acid Composition
The amino acid composition of a protein determines its functionality and bioactivity in biological systems (Sánchez and Vázquez, 2017). The general trend observed in this study was increases in the content of branched chain (BCAA), hydrophobic (HAA), negatively (NCAA) and sulfur containing amino acids (SCAA), while the aromatic (AAA) and positively charged (PCAA) amino acids showed decreases in their content with the removal of non-protein components as shown in Table 2. The BCAA content increased by 24% in the DPM and by 39.5% in the PPC in comparison to the contents in WPF. The HAA content of the DPM and PPC increased by 9.2% and 12.3%, respectively when compared to WPF. Similarly, the NCAA and SCAA levels were higher in the DPM and PPC than the WPF recording 12.8 and 24.3% content of NCAA and 15.7 and 30.1%, respectively. Histidine (His) is an essential amino acid with unique roles in proton buffering, metal ion chelation, scavenging of reactive oxygen and nitrogen species, erythropoiesis and the histaminergic system (Holeček, 2020). Considering all the amino acid side chains in proteins, only the imidazole ring of His is suitable to function as a pH buffer and either of the two nitrogen of the imidazole ring can bind or release a proton to form the acid or the base form, thus acting as buffering agent (Derave et al., 2010). There was an 8.6% increase in the His content of PPC when compared to WPF. Peanut proteins represent one of the best sources for high intake of arginine, a precursor for endogenous production of nitric oxide, which contributes to vasodilation and reduced blood pressure. Arginine level was 4.5 and 17% higher in DPM and PPC, respectively than in WPF. However, processing of DPM into PPC led to 14.4 and 16.5% decreases, in the levels of AAA and PCAA respectively, suggesting that purification depleted the level of protein fractions with higher levels of AAA and PCAA. PPC had the highest amount of NCAA (32.4%) and HAA (50.7%), which implies that the product has the most of available excess electrons that can be donated to neutralize free radicals and curb their destructive activities. All the nine essential amino acids were present in different concentrations similar to that in animal, and poultry proteins (Nasr et al., 2017a,b) in contrast to that of defatted hazel nuts meal, which was reported to have only seven essential amino acids present (Xu and Hanna, 2011). The enhanced amino acid profile of PPC shows that it can be used as a suitable ingredient for protein fortification in carbohydrate-based foods, which could reduce protein-energy malnutrition challenges.
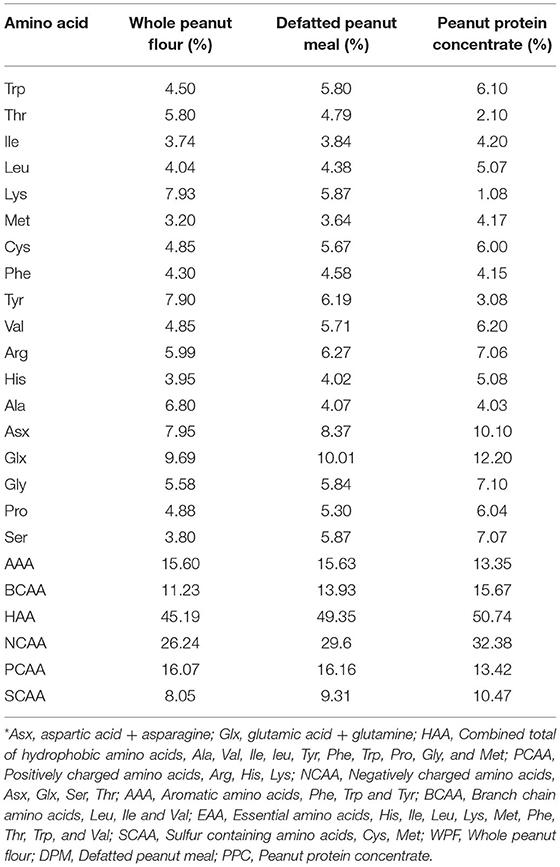
Table 2. Amino acid profile of whole peanut flour, defatted peanut protein meal, and peanut protein concentrate*.
Antioxidant Properties
GSH is a non-protein thiol and tripeptide made up of glycine, glutamate and cysteine and widely distributed in nature. It is an endogenous antioxidant that sequesters metal ions and scavenges free radicals to break the chain reactions that cause oxidative damage (Al-Anazi et al., 2015; Solovyova and Kuznetsova, 2015). The thiol group in GSH is the most chemically reactive group responsible for maintaining oxidation homeostasis in the cellular environment. GSH is the main regulator of redox homeostasis of the cellular environment, hence we have used this molecule to estimate the comparative antioxidant potency of the peanut products. The free radical scavenging activity of peanut products and GSH were determined using electron donation (DRSA and SRSA), hydrogen atom transfer (FRAP) and non-covalent sequestration of cations (MCA) assays. Vitamin C is a non-enzymatic endogenous antioxidant characterized by the ability to rapidly inactivate radicals and oxidants and could sometimes be used as a standard in comparison to experimental samples. Epidemiological studies have shown that people who consume a diet rich in vitamin C, presents a low risk of oxidative stress or other related diseases associated with free radicals, when compared with those who have a diet deficient in this vitamin (Grigorescu et al., 2015). Therefore, vitamin C was also used as a standard in one of the radical scavenging assays.
DPPH Radical Scavenging Activity
The DRSA determines antioxidant capacity of biological compounds by estimating the neutralization of the free radicals formed in the oxidant system (Chen et al., 2013). DPPH is an indirect method that measures the decrease in absorbance of the radical ion at 517 nm (Shahidi and Zhong, 2015). The DRSA of vitamin C (positive control) was the strongest when compared to WPF, DPM and PPC but all the samples exhibited dose-dependent effect to varying degrees with an inverse relationship between absorbance and dose (Figure 1). Vitamin C showed the most DRSA with larger decreases in absorbance than the peanut samples. The WPF and DPM had similar decreases in absorbance, except at 200 μg/mL where WPF produced a stronger effect. However, the PPC had weak DRSA as shown by the minimal decreases in absorbance, when compared to WPF and DPM. The DRSA of moringa seed kernels was also shown to increase proportionately with increase in antioxidant concentration (Jahan et al., 2018). Another study on different pine nut varieties and co-products also showed dose response effects for the DRSA (Zhang et al., 2020) and maya nuts exhibited similar antiradical behavior (Ozer, 2017). Similarly, a study with Tunisian dates also showed a positive correlation of DRSA with sample concentration (Kchaou et al., 2014). The weak activity of PPC indicate a negative effect of protein isolation, which indicates that the DRSA of the peanut is due mainly to the non-protein components.
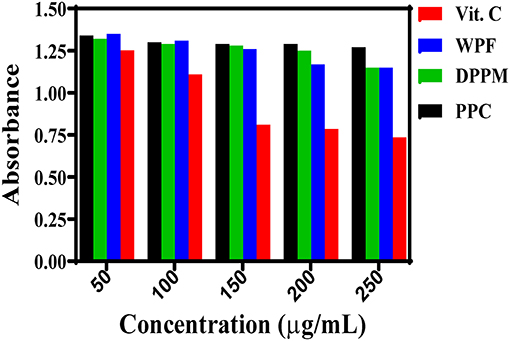
Figure 1. Dose-response DPPH radical scavenging effects of different peanut protein preparations (WPF, whole peanut flour; DPPM, defatted peanut protein meal; PPC, peanut protein concentrate) in comparison to a natural antioxidant (Vit. C).
Superoxide Radical Scavenging Activity
Biological reactions within the body are responsible for generating the superoxide radicals, which are highly toxic species that could damage human health (Collin, 2019). This is because the superoxide radical promotes oxidation of fatty acids to form toxic peroxides that destroy vital cellular components (He et al., 2013). In addition, the superoxide radical is involved in cellular reactions that lead to the production of other highly reactive compounds, such as the hydroxyl radical and hydrogen peroxide. Therefore, compounds that can effectively scavenge the superoxide radical could provide great protection to vital cellular components and reduce the risk of chronic health diseases. To determine the SRSA, pyrogallol was used for spontaneous oxidation producing the superoxide radical that can then be scavenged by antioxidant compounds. During the assay, the decrease in absorbance at 560 nm in the presence of antioxidants indicates neutralization of superoxide anions generated within the reaction mixture. The SRSA data as shown suggest significantly (p < 0.05) stronger effect of GSH (Figure 2). For the peanut products, WPF was the weakest while PPC had significantly (p < 0.05) higher value. Zhang et al. (2014) reported the SRSA of peanut meal to be 39.3%, which is similar to the 37% obtained for DPM in this study. Therefore, the strong SRSA of the PPC indicate great contributions from the protein constituents or it could also be a reflection of better synergistic interactions between protein and non-protein constituents when compared to similar interactions in WPF and DPM. In addition, the PPC has a significantly (p < 0.05) higher level of NCAA, which provides surplus electrons that can be readily donated to quench the superoxide radical.
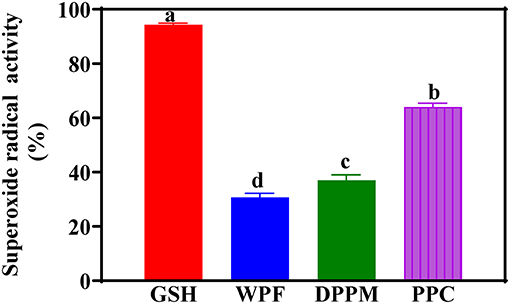
Figure 2. Superoxide radical scavenging activities of different peanut protein preparations (WPF, whole peanut flour; DPPM, defatted peanut protein meal; PPC, peanut protein concentrate) in comparison to glutathione (GSH) a natural antioxidant. Values are means ± std and bars with different letters are significantly different at p < 0.05.
Hydroxyl Radical Scavenging Activity
The hydroxyl radical is the most reactive oxygen species produced during metabolism and known to be the cause of many severe physiological disorders including cancer, diabetes, atherosclerosis, Alzheimer's disease and aging. The hydroxyl radical and ferric ions are generated through the Fenton reaction in the presence of hydrogen peroxide and ferrous ions. Continuous redox reactions occur in which the ferrous ions are oxidized to ferric ions and then reduced again to ferrous ions. Figure 3 shows that among the peanut products, the PPC had the strongest HRSA, though lower than that of GSH. The PPC activity is similar to ~70% HRSA reported for a peanut skin extract (Wang et al., 2007). The results of the current study suggest that increased protein purity (removal of non-protein compounds) may be responsible for the enhanced HRSA. This could be due to reduced interference from non-protein materials, which enabled a more efficient electron donating capability of the amino acid constituents. The presence of higher levels of NCAAs could also have contributed to the stronger HRSA of the PPC when compared to WPF and DPM.
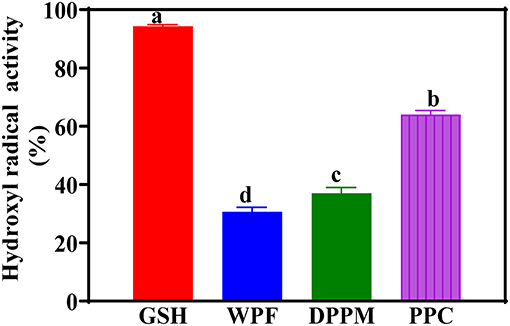
Figure 3. Hydroxyl radical scavenging activities of different peanut protein preparations (WPF, whole peanut flour; DPPM, defatted peanut protein meal; PPC, peanut protein concentrate) in comparison to glutathione (GSH) a natural antioxidant. Values are means ± std and bars with different letters are significantly different at p < 0.05.
Ferric Reducing Antioxidant Power
FRAP is a method based on the ability of an antioxidant compound to reduce ferric ion (Fe3+) into the ferrous (Fe2+) form. Figure 4 also shows that the PPC had the strongest reducing power among the peanut products, though significantly (p < 0.05) lower than that of GSH. The results concur with the stronger SRSA and HRSA of PPC when compared to WPF and DPM. Therefore, the electron donating ability of peanut protein amino acids was improved through removal of non-protein materials. A previous work reported a higher FRAP activity (1.07 absorbance) for a peanut protein isolate (88.5% protein content), though the assay was carried out at 2.5 mg/mL when compared to the 1 mg/mL used in the current work (Jamdar et al., 2010). The FRAP values of the WPF, DPM, and PPC in this study are also lower than another work, which reported 0.22 to 0.24 absorbance values (Karamać et al., 2016).
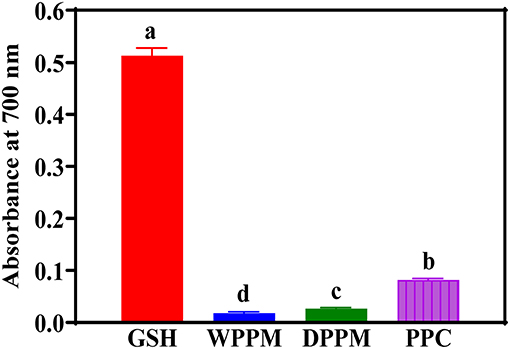
Figure 4. Ferric reducing antioxidant power activities of different peanut protein preparations (WPF, whole peanut flour; DPPM, defatted peanut protein meal; PPC, peanut protein concentrate) in comparison to glutathione (GSH) a natural antioxidant. Values are means ± std and bars with different letters are significantly at p < 0.05.
Metal Chelation Activity
The spontaneous degeneration of peroxides to form ROS species is very slow but the presence of metals with more than one redox state can catalyze the reaction and make it fast. Some examples of metals of interest are Fe and Cu and sequestering of these methods will block metal-catalyzed free radical formation. The MCA of the peanut products indicate significantly stronger ability of PPC when compared to DPM and WPF (Figure 5). Therefore, protein purity also enhanced complexation with the metal cation. Since a negative charge on the protein facilitates metal binding, it is possible that removal of non-protein materials that interact with negatively charged amino acids contributed to the improved MCA of PPC. Moreover, in comparison to WPF and DPM, the PPC has significantly (p < 0.05) higher levels of histidine (Table 2), which is a well-known metal-chelating amino acid. A previous work showed that flaxseed protein isolate had a 25.9% MCA at 1.54 mg/mL sample concentration, which makes it weaker than the 59.5% obtained in this work using 1 mg/mL (Karamać et al., 2016). Similarly, the MCA obtained for PPC in this work is higher than the 15% reported for another flaxseed protein isolate at 2.3 mg/mL sample concentration (Hwang et al., 2016).
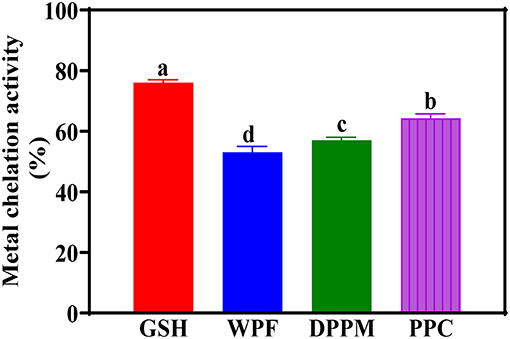
Figure 5. Metal chelation activities of different peanut protein preparations (WPF, whole peanut flour; DPPM, defatted peanut protein meal; PPC, peanut protein concentrate) in comparison to glutathione (GSH) a natural antioxidant. Values are means ± std and bars with different letters are significantly different at p < 0.05.
Conclusions
The processing conditions and extraction methods utilized in the preparation of the samples were observed to influence the phytochemical content and antioxidant activity of the peanut products. DPM had twice the amount of TPC than WPF and PPC, which indicates that a large fraction of the total polyphenols was not very soluble in the lipid phase. In contrast, defatting of WPF led to reductions in the TFC of DPM and PPC, which suggest a high proportion of the flavonoids were partitioned in the lipid phase. Processing of the flour into a meal and protein concentrate improved the nutritional composition, which was reflected in terms of higher protein and essential amino acid contents. With the exception of DRSA, the PPC displayed stronger antioxidant activity than WPF and DPM, which suggests the beneficial effects of removal of non-protein materials. However, the stronger antioxidant properties of PPC could also be attributed to higher levels of NCAAs and histidine, which enhanced electron donating and metal binding capacities, respectively. Therefore, PPC could be considered a suitable ingredient to formulate food products with ability to scavenge free radicals and bind metal cations as means of reducing or preventing the damaging health effects of reactive oxygen species. However, future research work is needed to investigate the role of non-protein components in peanut flour functionality.
Data Availability Statement
The original contributions presented in the study are included in the article/supplementary material, further inquiries can be directed to the corresponding authors.
Author Contributions
NA: conceptualization, methodology, formal analysis, data curation, and writing-review and editing. AB and JG: investigation, formal analysis, and data curation. RA: methodology, funding acquisition, data curation, visualization, and writing-review and editing. AG: conceptualization, project administration, visualization, methodology, data curation, writing-original draft, and writing-review and editing. All authors contributed to the article and approved the submitted version.
Funding
The authors acknowledge support from the Natural Sciences and Engineering Council of Canada (NSERC), funding reference number RGPIN 2018-06019.
Conflict of Interest
The authors declare that the research was conducted in the absence of any commercial or financial relationships that could be construed as a potential conflict of interest.
Publisher's Note
All claims expressed in this article are solely those of the authors and do not necessarily represent those of their affiliated organizations, or those of the publisher, the editors and the reviewers. Any product that may be evaluated in this article, or claim that may be made by its manufacturer, is not guaranteed or endorsed by the publisher.
References
Adefegha, S. A., Oyeleye, S. I., and Oboh, G. (2015). Distribution of phenolic contents, antidiabetic potentials, antihypertensive properties, and antioxidative effects of soursop fruit parts. Biochem. Res. Int. 2015:347673. doi: 10.1155/2015/347673
Adhikari, B., Dhungana, S. K., Ali, M. W., Adhikari, A., Kim, I.-D., and Shin, D.-H. (2018). Resveratrol, total phenolic and flavonoid contents, and antioxidant potential of seeds and sprouts of Korean peanuts. Food Sci. Biotechnol. 27, 1275–1284. doi: 10.1007/s10068-018-0364-7
Al-Anazi, M. S., Virk, P., Elobeid, M., and Siddiqui, M. I. (2015). Ameliorative effects of Rosmarinus officinalis leaf extract and Vitamin C on cadmium-induced oxidative stress in Nile tilapia Oreochromis niloticus. J. Environ. Biol. 36, 1401–1408.
Alov, P., Tsakovska, I., and Pajeva, I. (2015). Computational studies of free radical-scavenging properties of phenolic compounds. Curr. Top. Med. Chem. 15, 85–104. doi: 10.2174/1568026615666141209143702
Altin, G., Ayar, E., and Ozcelik, B. (2019). Phenolic-protein interaction: effects on functional properties of phenolics and advantages on phenolic delivery platform development. Glob. J. Med. Res. Nutr. Food Sci. 19, 1–11.
Arya, S. S., Salve, A. R., and Chauhan, S. (2016). Peanuts as functional food: a review. J. Food Sci. Technol. 53, 31–41. doi: 10.1007/s13197-015-2007-9
Attree, R., Du, B., and Xu, B. (2015). Distribution of phenolic compounds in seed coat and cotyledon, and their contribution to antioxidant capacities of red and black seed coat peanuts (Arachis hypogaea L.). Ind. Crops Prod. 67, 448–456. doi: 10.1016/j.indcrop.2015.01.080
Bhat, E., Sajjad, N., Manzoor, I., and Rasool, A. (2019). Bioactive compounds in peanuts and banana. Biochem. Anal, Biochem. 8:38. doi: 10.35248/2161-1009.19.8.382
Bidlingmeyer, B. A., Cohen, S. A., and Tarvin, T. L. (1984). Rapid analysis of amino acids using pre-column derivatization. J. Chromatogr. B Biomed. Sci. Appl. 336, 93–104. doi: 10.1016/S0378-4347(00)85133-6
Chen, C. Y. O., and Blumberg, J. B. (2008). Phytochemical composition of nuts. Asia Pac. J. Clin. Nutr. 17, 329–332.
Chen, Z., Bertin, R., and Froldi, G. (2013). EC50 estimation of antioxidant activity in DPPH assay using several statistical programs. Food Chem. 138, 414–420. doi: 10.1016/j.foodchem.2012.11.001
Collin, F. (2019). Chemical basis of reactive oxygen species reactivity and involvement in neurodegenerative diseases. Int. J. Mol. Sci. 20:2407. doi: 10.3390/ijms20102407
Coulibaly, M., N'dri, D. Y., Abouo, V., Kouamé, C. A., Kouassi, N. K., and Amani, G. N. G. (2018). The impact of post-harvest traditional technologies on nutritional value and antioxidant activity of seeds kernels “akpi” of Côte d'Ivoire (West Africa). Int. J. Food Sci. Nutr. Eng. 8, 15–26. doi: 10.5923/j.food.20180802.01
De Camargo, A. C., Regitano-D'arce, M. A. B., and Shahidi, F. (2017). Phenolic profile of peanut by-products: antioxidant potential and inhibition of alpha-glucosidase and lipase activities. J. Am. Oil Chem. Soc. 94, 959–971. doi: 10.1007/s11746-017-2996-9
De Camargo, A. C., Vidal, C. M. M., Canniatti-Brazaca, S. G., and Shahidi, F. (2014). Fortification of cookies with peanut skins: effects on the composition, polyphenols, antioxidant properties, and sensory quality. J. Agric. Food Chem. 62, 11228–11235. doi: 10.1021/jf503625p
Derave, W., Everaert, I., Beeckman, S., and Baguet, A. (2010). Muscle carnosine metabolism and β-alanine supplementation in relation to exercise and training. Sports Med. 40, 247–263. doi: 10.2165/11530310-000000000-00000
Duodu, C. P., Adjei-Boateng, D., Edziyie, R. E., Agbo, N. W., Owusu-Boateng, G., Larsen, B. K., et al. (2018). Processing techniques of selected oilseed by-products of potential use in animal feed: Effects on proximate nutrient composition, amino acid profile and antinutrients. Anim. Nutr. 4, 442–451. doi: 10.1016/j.aninu.2018.05.007
Embaby, H. E. S. (2011). Effect of heat treatments on certain antinutrients and in vitro protein digestibility of peanut and sesame seeds. Food Sci. Technol. Res. 17, 31–38. doi: 10.3136/fstr.17.31
Forni, C., Facchiano, F., Bartoli, M., Pieretti, S., Facchiano, A., D'arcangelo, D., et al. (2019). Beneficial role of phytochemicals on oxidative stress and age-related diseases. Biomed Res. Int. 2019, 8748253–8748253. doi: 10.1155/2019/8748253
Gehrke, C. W., Wall Sr, L. L., Absheer, J. S., Kaiser, F. E., and Zumwalt, R. W. (1985). Sample preparation for chromatography of amino acids: acid hydrolysis of proteins. J. Assoc. Off. Anal. Chem. 68, 811–821. doi: 10.1093/jaoac/68.5.811
Giada, M. (2013). Food Phenolic Compounds: Main Classes, Sources, and Their Antioxidant Power. Oxidative Stress and Chronic Degenerative Diseases—A Role for Antioxidants. London: InTech, 87–112.
Girgih, A. T., Nwachukwu, I. D., Iwar, M. I., Fagbemi, T. N., and Aluko, R. E. (2016). Effect of peptide size on the antioxidant properties of giant African land snail (Archachatina marginata) meat protein hydrolysates. Curr. Top. Nutraceutical Res. 14, 109–118.
Grigorescu, R., Gruia, M. I., Nacea, V., Nitu, C., Negoita, V., and Glavan, D. (2015). The evaluation of non-enzymatic antioxidants effects in limiting tumor- associated oxidative stress, in a tumor rat model. J. Med. Life 8, 513–516.
Guo, C., Xie, Y.-J., Zhu, M.-T., Xiong, Q., Chen, Y., Yu, Q., et al. (2020). Influence of different cooking methods on the nutritional and potentially harmful components of peanuts. Food Chem. 316:126269. doi: 10.1016/j.foodchem.2020.126269
Gutiérrez-Grijalva, E. P. (2016). 1. Review: dietary phenolic compounds, health benefits and bioaccessibility. Arch. Latin Am. Nutr. 66, 87–100.
He, R., Girgih, A. T., Malomo, S. A., Ju, X., and Aluko, R. E. (2013). Antioxidant activities of enzymatic rapeseed protein hydrolysates and the membrane ultrafiltration fractions. J. Funct. Foods 5, 219–227. doi: 10.1016/j.jff.2012.10.008
Holeček, M. (2020). Histidine in health and disease: metabolism, physiological importance, and use as a supplement. Nutrients 12:848. doi: 10.3390/nu12030848
Hwang, C. F., Chen, Y. A., Luo, C., and Chiang, W. D. (2016). Antioxidant and antibacterial activities of peptide fractions from flaxseed protein hydrolysed by protease from Bacillus altitudinis HK02. Int. J. Food Sci. Technol. 51, 681–689. doi: 10.1111/ijfs.13030
Jahan, I. A., Hossain, M. H., Ahmed, K. S., Sultana, Z., Biswas, P. K., and Nada, K. (2018). Antioxidant activity of Moringa oleifera seed extracts. Orient. Pharm. Exp. Med. 18, 299–307. doi: 10.1007/s13596-018-0333-y
Jain, M., Gallo, M., Chengalrayan, K., Shaikh, N. P., Macdonald, G. E., and Davis, J. M. (2015). Phorate-induced host defence responses condition acquired resistance to tomato spotted wilt in cultivated peanut (Arachis hypogaea L.). J. Phytopathol. 163, 853–866. doi: 10.1111/jph.12385
Jamdar, S. N., Rajalakshmi, V., Pednekar, M. D., Juan, F., Yardi, V., and Sharma, A. (2010). Influence of degree of hydrolysis on functional properties, antioxidant activity and ACE inhibitory activity of peanut protein hydrolysate. Food Chem. 121, 178–184. doi: 10.1016/j.foodchem.2009.12.027
Kain, R. J., and Chen, Z. (2010). Physico-functional properties of peanut meal flour as affected by processing methods. J. Food Biochem. 34, 229–243. doi: 10.1111/j.1745-4514.2009.00252.x
Karamać, M., Kosińska-Cagnazzo, A., and Kulczyk, A. (2016). Use of different proteases to obtain flaxseed protein hydrolysates with antioxidant activity. Int. J. Mol. Sci. 17:1027. doi: 10.3390/ijms17071027
Kasote, D. M., Katyare, S. S., Hegde, M. V., and Bae, H. (2015). Significance of antioxidant potential of plants and its relevance to therapeutic applications. Int. J. Biol. Sci. 11, 982–991. doi: 10.7150/ijbs.12096
Kaurinovic, B., and Vastag, D. (2019). Flavonoids and Phenolic Acids as Potential Natural Antioxidants Antioxidants. London: IntechOpen.
Kchaou, W., Abbès, F., Attia, H., and Besbes, S. (2014). In vitro antioxidant activities of three selected dates from Tunisia (Phoenix dactylifera L.). J. Chem. 2014:367681. doi: 10.1155/2014/367681
Khaopha, S., Senawong, T., Jogloy, S., and Patanothai, A. (2012). Comparison of total phenolic content and composition of individual phenolic acids in testae and testa-removed kernels of 15 Valencia-type peanut (Arachis hypogaea L.) genotypes. Afr. J. Biotechnol. 11, 15923–15930. doi: 10.5897/AJB12.1389
Klu, Y. A. K., Phillips, R. D., and Chen, J. (2014). Survival of four commercial probiotic mixtures in full fat and reduced fat peanut butter. Food Microbiol. 44, 34–40. doi: 10.1016/j.fm.2014.04.018
Kumari, A. (2017). Evaluation of total phenolic, flavonoid content, and dpph free radical scavenging activity of methanolic extract of ailanthus excelsa roxb. Evaluation 10:188. doi: 10.22159/ajpcr.2017.v10i4.16507
Kumari, A., and Ra, S. (2017). Evaluation of total phenolic, flavonoid content and DPPH free radical scavenging activity of methanolic extract of Ailanthus excelsa roxb. Asian J. Pharm. Clin. Res. 10, 188–191.
Landry, J., and Delhaye, S. (1992). Simplified procedure for the determination of tryptophan of foods and feedstuffs from barytic hydrolysis. J. Agric. Food Chem. 40, 776–779. doi: 10.1021/jf00017a014
Larit, F., León, F., Benyahia, S., and Cutler, S. J. (2019). Total phenolic and flavonoid content and biological activities of extracts and isolated compounds of cytisus villosus pourr. Biomolecules 9:732. doi: 10.3390/biom9110732
Liu, J., Yong, H., Yao, X., Hu, H., Yun, D., and Xiao, L. (2019). Recent advances in phenolic–protein conjugates: synthesis, characterization, biological activities and potential applications. RSC Adv. 9, 35825–35840. doi: 10.1039/C9RA07808H
Liu, K., Luo, M., and Wei, S. (2019). The bioprotective effects of polyphenols on metabolic syndrome against oxidative stress: evidences and perspectives. Oxid. Med. Cell. Longev. 2019:6713194. doi: 10.1155/2019/6713194
Liu, L., Jin, C., and Zhang, Y. (2014). Lipophilic phenolic compounds (Lipo-PCs): emerging antioxidants applied in lipid systems. RSC Adv. 4, 2879–2891. doi: 10.1039/C3RA44792H
Lozano, M. G., De Oliveira Sartori, A. G., Markowicz Bastos, D. H., and Bismara Regitano-D'arce, M. A. (2019). Selected nutrients and antinutrients in peanut cultivars harvested in Brazil. J. Sci. Food Agric. 99, 5334–5340. doi: 10.1002/jsfa.9772
Ma, F.-F., Wang, H., Wei, C.-K., Thakur, K., Wei, Z.-J., and Jiang, L. (2019). Three novel ACE inhibitory peptides isolated from Ginkgo biloba seeds: purification, inhibitory kinetic and mechanism. Front. Pharmacol. 9:1579. doi: 10.3389/fphar.2018.01579
Markwell, M. A. K., Haas, S. M., Bieber, L. L., and Tolbert, N. (1978). A modification of the Lowry procedure to simplify protein determination in membrane and lipoprotein samples. Anal. Biochem. 87, 206–210. doi: 10.1016/0003-2697(78)90586-9
Moukette, B. M., Pieme, C. A., Njimou, J. R., Biapa, C. P. N., Marco, B., and Ngogang, J. Y. (2015). In vitro antioxidant properties, free radicals scavenging activities of extracts and polyphenol composition of a non-timber forest product used as spice: Monodora myristica. Biol. Res. 48:15. doi: 10.1186/s40659-015-0003-1
Nasr, M. A. F., Abd-Elhamid, T., and Hussein, M. A. (2017a). Growth performance, carcass characteristics, meat quality and muscle amino-acid profile of different rabbits breeds and their crosses. Meat Sci. 134, 150–157. doi: 10.1016/j.meatsci.2017.07.027
Nasr, M. A. F., Ali, E. M. R., and Hussein, M. A. (2017b). Performance, carcass traits, meat quality and amino acid profile of different Japanese quails strains. J. Food Sci. Technol. 54, 4189–4196. doi: 10.1007/s13197-017-2881-4
Ozer, H. K. (2017). Phenolic compositions and antioxidant activities of Maya nut (Brosimum alicastrum): Comparison with commercial nuts. Int. J. Food Prop. 20, 2772–2781. doi: 10.1080/10942912.2016.1252389
Panche, A., Diwan, A., and Chandra, S. (2016). Flavonoids: an overview. J. Nutr. Sci. 5:e47. doi: 10.1017/jns.2016.41
Phuyal, N., Jha, P. K., Raturi, P. P., and Rajbhandary, S. (2020). Total phenolic, flavonoid contents, and antioxidant activities of fruit, seed, and bark extracts of Zanthoxylum armatum DC. Sci. World J. 2020:8780704. doi: 10.1155/2020/8780704
Prabasheela, B., Venkateshwari, R., Nivetha, S., Mohanapriya, P., Jayashree, T., Vimala, R., et al. (2015). Phytochemical analysis and antioxidant activity of Arachishypogaea. J. Chem. Pharm. Res. 7, 116–121.
Riveros, C. G., Martin, M. P., Aguirre, A., and Grosso, N. R. (2018). Film preparation with high protein defatted peanut flour: characterisation and potential use as food packaging. Int. J. Food Sci. Technol. 53, 969–975. doi: 10.1111/ijfs.13670
Rusu, M. E., Mocan, A., Ferreira, I. C., and Popa, D.-S. (2019). Health benefits of nut consumption in middle-aged and elderly population. Antioxidants 8:302. doi: 10.3390/antiox8080302
Salve, A. R., Leblanc, J. G., and Arya, S. S. (2020). Effect of processing on polyphenol profile, aflatoxin concentration and allergenicity of peanuts. J. Food Sci. Techn. 58, 2714–24. doi: 10.1007/s13197-020-04779-7
Sánchez, A., and Vázquez, A. (2017). Bioactive peptides: a review. Food Qual. Saf. 1, 29–46. doi: 10.1093/fqs/fyx006
Sarkis, J. R., Côrrea, A. P. F., Michel, I., Brandeli, A., Tessaro, I. C., and Marczak, L. D. (2014). Evaluation of the phenolic content and antioxidant activity of different seed and nut cakes from the edible oil industry. J. Am. Oil Chem. Soc. 91, 1773–1782. doi: 10.1007/S11746-014-2514-2
Sebei, K., Gnouma, A., Herchi, W., Sakouhi, F., and Boukhchina, S. (2013). Lipids, proteins, phenolic composition, antioxidant and antibacterial activities of seeds of peanuts (Arachis hypogaea l) cultivated in Tunisia. Biol. Res. 46, 257–263. doi: 10.4067/S0716-97602013000300006
Shahidi, F., and Zhong, Y. (2015). Measurement of antioxidant activity. J. Funct. Foods 18, 757–781. doi: 10.1016/j.jff.2015.01.047
Sibt-e-Abbas, M., Butt, M. S., Sultan, M. T., Sharif, M. K., Ahmad, A. N., and Batool, R. (2015). Nutritional and functional properties of protein isolates extracted from defatted peanut flour. Int. Food Res. J. 22, 1533–1537.
Solovyova, N. V., and Kuznetsova, T. Y. (2015). Quantum chemical modeling of antioxidant activity of glutathione interacting with hydroxyl-and superoxide anion radicals. Ukr. Biochem. J. 87, 156–162. doi: 10.15407/ubj87.02.156
Stevens-Barrón, J. C., De La Rosa, L. A., Wall-Medrano, A., Álvarez-Parrilla, E., Rodríguez-Ramirez, R., Robles-Zepeda, R. E., et al. (2019). Chemical composition and in vitro bioaccessibility of antioxidant phytochemicals from selected edible nuts. Nutrients 11, 2303. doi: 10.3390/nu11102303
Thakur, M., Singh, K., and Khedkar, R. (2020). “Phytochemicals: extraction process, safety assessment, toxicological evaluations, and regulatory issues,” in Functional and Preservative Properties of Phytochemicals, ed B. Prakash (Waltham, MA: Academic Press), 341–361.
Toomer, O. T. (2018). Nutritional chemistry of the peanut (Arachis hypogaea). Crit. Rev. Food Sci. Nutr. 58, 3042–3053. doi: 10.1080/10408398.2017.1339015
Toomer, O. T. (2020). A comprehensive review of the value-added uses of peanut (Arachis hypogaea) skins and by-products. Crit. Rev. Food Sci. Nutr. 60, 341–350. doi: 10.1080/10408398.2018.1538101
Tungmunnithum, D., Thongboonyou, A., Pholboon, A., and Yangsabai, A. (2018). Flavonoids and other phenolic compounds from medicinal plants for pharmaceutical and medical aspects: an overview. Medicines (Basel, Switzerland) 5:93. doi: 10.3390/medicines5030093
Uddin, M. S., Islam, A., Rahman, M. M., Uddin, M. B., and Mazumder, A. R. (2018). Isolation of protein from defatted peanut meal and characterize their nutritional profile. Chem. Res. J. 3, 187–196.
Vijayalaxmi, S., Jayalakshmi, S., and Sreeramulu, K. (2015). Polyphenols from different agricultural residues: extraction, identification and their antioxidant properties. J. Food Sci. Technol. 52, 2761–2769. doi: 10.1007/s13197-014-1295-9
Wang, J., Yuan, X., Jin, Z., Tian, Y., and Song, H. (2007). Free radical and reactive oxygen species scavenging activities of peanut skins extract. Food Chem. 104, 242–250. doi: 10.1016/j.foodchem.2006.11.035
Wang, Q., Shi, A., Liu, H., Liu, L., Zhang, Y., Li, N., et al. (2016). Peanut By-Products Utilization Technology Peanuts: Processing Technology and Product Development. Amsterdam: Elsevier, 211–325.
Wu, H., Wang, Q., Ma, T., and Ren, J. (2009). Comparative studies on the functional properties of various protein concentrate preparations of peanut protein. Food Res. Int. 42, 343–348. doi: 10.1016/j.foodres.2008.12.006
Xie, Z., Huang, J., Xu, X., and Jin, Z. (2008). Antioxidant activity of peptides isolated from alfalfa leaf protein hydrolysate. Food Chem. 111, 370–376. doi: 10.1016/j.foodchem.2008.03.078
Xu, Y., and Hanna, M. A. (2011). Nutritional and anti-nutritional compositions of defatted Nebraska hybrid hazelnut meal. Int. J. Food Sci. Technol. 46, 2022–2029. doi: 10.1111/j.1365-2621.2011.02712.x
Yu, J., Ahmedna, M., and Goktepe, I. (2007). Peanut protein concentrate: production and functional properties as affected by processing. Food Chem. 103, 121–129. doi: 10.1016/j.foodchem.2006.08.012
Zhang, S. B., Wang, Z., and Xu, S. Y. (2008). Antioxidant and antithrombotic activities of rapeseed peptides. J. Am. Oil Chem. Soc. 85, 521–527. doi: 10.1007/s11746-008-1217-y
Zhang, X., Lian, H., Shi, J., Meng, W., and Peng, Y. (2020). Plant extracts such as pine nut shell, peanut shell and jujube leaf improved the antioxidant ability and gas permeability of chitosan films. Int. J. Biol. Macromol. 148, 1242–1250. doi: 10.1016/j.ijbiomac.2019.11.108
Zhang, Y., Liu, J., Lu, X., Zhang, H., Wang, L., Guo, X., et al. (2014). Isolation and identification of an antioxidant peptide prepared from fermented peanut meal using Bacillus subtilis fermentation. Int. J. Food Prop. 17, 1237–1253. doi: 10.1080/10942912.2012.675605
Zhao, G., Liu, Y., Zhao, M., Ren, J., and Yang, B. (2011). Enzymatic hydrolysis and their effects on conformational and functional properties of peanut protein isolate. Food Chem. 127, 1438–1443. doi: 10.1016/j.foodchem.2011.01.046
Keywords: polyphenols, flavonoids, whole peanut flour, peanut protein meal, peanut protein concentrate, antioxidant properties, amino acid profile
Citation: Asen ND, Badamasi AT, Gborigo JT, Aluko RE and Girgih AT (2021) Comparative Evaluation of the Antioxidant Properties of Whole Peanut Flour, Defatted Peanut Protein Meal, and Peanut Protein Concentrate. Front. Sustain. Food Syst. 5:765364. doi: 10.3389/fsufs.2021.765364
Received: 26 August 2021; Accepted: 11 November 2021;
Published: 10 December 2021.
Edited by:
Dominic Agyei, University of Otago, New ZealandReviewed by:
Beatriz Mello, Federal University of São Carlos, BrazilVânia Regina Nicoletti, São Paulo State University, Brazil
Apollinaire Tsopmo, Carleton University, Canada
Copyright © 2021 Asen, Badamasi, Gborigo, Aluko and Girgih. This is an open-access article distributed under the terms of the Creative Commons Attribution License (CC BY). The use, distribution or reproduction in other forums is permitted, provided the original author(s) and the copyright owner(s) are credited and that the original publication in this journal is cited, in accordance with accepted academic practice. No use, distribution or reproduction is permitted which does not comply with these terms.
*Correspondence: Abraham T. Girgih, YWJyYWhhbS5naXJnaWgmI3gwMDA0MDt1YW0uZWR1Lm5n; Rotimi E. Aluko, cm90aW1pLmFsdWtvJiN4MDAwNDA7dW1hbml0b2JhLmNh