- School of Environment and Natural Resources, The Ohio State University, Wooster, OH, United States
Ecological nutrient management is a strategy that can help create resilient cropping systems and reduce the negative impact that agricultural systems have on the environment. Ecological nutrient management enhances plant-soil-microbial interactions and optimizes crop production while providing key ecosystem services. Incorporating perennial legumes into crop rotations and implementing no-till to enhance organic nitrogen (N) soil pools could reduce the need for inorganic N fertilizer inputs and lead to improved soil health. Plant and soil N pools need to be further quantified to understand how to enhance soil health across a range of agroecosystems. This paper aims to quantify plant and soil N pools in systems contrasting in crop perenniality (corn–corn, corn–soy, and corn–forage–forage) and tillage intensity (chisel till vs. no-till). Key plant, soil, and organismal metrics of N cycling were measured including fine root production, N-Acetyl-B-Gulcosaminidase (NAG) enzyme activity, and soil protein, nematode enrichment opportunist (fungal and bacterial feeding nematodes) and the nematode Enrichment Index. Fine root production was determined using in-growth mesh cores. Findings reveal that monoculture cropping systems with reduced tillage intensity and rotations with perennial legumes had significantly greater fine root N (FRN), soil protein and NAG enzyme activity (p < 0.05) relative to corn-soy. Additionally, nematode bacterivore enrichment opportunists (b1) were significantly reduced in corn-corn systems when compared to all other crop rotation systems. Correlation analyses indicated positive and significant relationships between FRN and soil protein (p < 0.05). These results demonstrate that lengthening crop rotations with perennial legumes and incorporating no-till management can increase organic N inputs, N mineralization rates, and organic N storage. Such ecological approaches to management have the potential to reduce the need for inorganic N inputs, while increasing long-term soil health and crop productivity.
Introduction
The conventional approach to nutrient management is to apply inorganic sources of nitrogen fertilizer to ensure rapid crop nutrient uptake that maximizes crop yields. Typically, crops can only take up between one-third to one-half of the fertilizer that is applied (Robertson and Vitousek, 2009; Conant et al., 2017). The remaining fertilizer is generally lost to the environment contributing to eutrophication and global climate change (Robertson and Vitousek, 2009; Billen et al., 2013). In contrast, ecological nutrient management practices aim to optimize crop productivity while simultaneously enhancing soil fertility and long-term ecosystem sustainability (Drinkwater and Snapp, 2007a).
While there are numerous definitions and approaches to ecological nutrient management, here we define it as a form of management that seeks to foster plant-soil-microbial interactions that optimize crop production and simultaneously provide ecosystem services such as improved soil health and nutrient retention. In contrast, we classify conventional systems as those that depend on the use of inorganic nutrient additions and disruptive tillage practices to maintain yields. Ecological nutrient management provides a framework toward enhancing food production and increasing resilience, which is sorely needed due to accelerated population growth and climate change. The United Nations sustainability goals remind us that 8.2% of the world lives in extreme poverty and that 26.4% suffer from food insecurity (UN Sustainable Development Goals, 2020). Additionally, the effects of climate change continue to impact agricultural production around the world (Agovino et al., 2019). Thus, while access to safe and nutritious food must increase, it is no longer feasible to approach agriculture through the single lens of maximizing crop production. Ecological nutrient management could serve as a sustainable solution to food production around the world and serve as an effective way to reach the United Nations Sustainability Goal 2.4, which seeks to ensure sustainable food production and resilient agricultural practices that not only enhance crop production but also help to maintain ecosystems that strengthen the capacity for climate adaptation and improved soil quality (UN Sustainable Development Goals, 2020).
However, questions remain on the best ways to quantify nutrient pools, especially under the umbrella of Ecological Nutrient Management, which is essential for understanding which agroecosystems are most effective at boosting yields while enhancing soil health. Furthermore, the improvement of nutrient pools that contribute to soil health and long-term sustainability is essential for building resilient agroecosystems. The conventional model for managing nutrients within agricultural systems focuses solely on inorganic nutrient pools, inhibiting nitrification and dentification, and fertilizing to match crop N uptake (Drinkwater and Snapp, 2007a). However, these management strategies have been shown to produce unsustainable yields, develop systems that cannot withstand stressful conditions, and promote nutrient leaching and run-off (Syswerda et al., 2012; Jungers et al., 2019). Management strategies that encompass an ecological approach may provide a solution through shifting the focus to enhancing rhizosphere interactions. Specifically, management strategies than can maintain nutrient reservoirs for plant access, promote both organic and inorganic nutrient pools, enhance microbial mineralization, and reduce the size of inorganic nutrient pools have the potential to increase ecosystem resilience (Drinkwater and Snapp, 2007a). Crop diversification is one agricultural management practice that fits well within the ecological nutrient framework because it has been shown to maximize nutrient assimilation and produce increased yields under climatic stress (Bowles et al., 2020). However, the specific interactions between plant and soil nutrient pools within systems of varying crop diversity have yet to be quantified. In addition, reduced tillage intensity is another management practice that fosters and ecological approach. Specifically, no-till systems can enhance nutrient reservoirs for plant-uptake through greater aggregation caused by reduced mechanical disturbance (Jiao et al., 2006).
There have long been attempts to assess ecological nutrient management within the rhizosphere to gain a deeper understanding of how belowground processes can support crop productivity (Drinkwater and Snapp, 2007b; Zhang et al., 2017a). That said, these reviews have approached rhizosphere dynamics largely from the standpoint of which management practices can best increase rhizosphere processes and have been vague on the best ways in which to accurately quantify organic nutrient pools, such as the organic nitrogen (N) pool. In truth, advancements in soil health methodologies in recent years have helped bolster our understanding of nitrogen cycling within the rhizosphere (Moebius-Clune et al., 2008; Hurisso et al., 2018b).
For instance, fine root production plays a vital role in rhizosphere dynamics through maintaining organic N pools. Specifically, fine roots serve as a large source of N within rhizosphere systems and the fast turnover of fine roots can allow for N retention (Gordon and Jackson, 2000; Sprunger et al., 2018). Furthermore, the N supply provided by fine roots is essential for plant biogeochemical functions and can stimulate microbial growth and enhance N mineralization and the release of soluble N that can be used for plant growth within the rhizosphere (Gordon and Jackson, 2000; Jackson, 2000). Ecological nutrient management practices such as perenniality, have also been shown to produce a greater pool of fine root N relative to annual root systems (Dietzel et al., 2015; Sprunger et al., 2018). Moreover, the extensive root systems of perennial crops also allows for a greater N use efficiency (Sprunger et al., 2018). Therefore, diversifying cropping systems using perennial crops may enhance N sinks that can contribute to plant–microbe–soil N cycling and reduce the need for N fertilizer additions (Crews and Peoples, 2005; Dawson et al., 2008; Sprunger et al., 2018).
Quantifying soil N in agricultural systems is challenging as these pools constantly change over the course of a growing season. Moreover, when soil N is tested, most metrics only measure the inorganic forms of N. This leaves several key N pools unquantified, including the organic pool of N a key component of soil organic matter (Hurisso et al., 2018a). While other tests have been developed to measure important fluxes on soil N, including mineralizable N and potentially mineralizable N incubations, these tests still do a poor job of reflecting biologically available pools of N (Hurisso et al., 2018b). Recent efforts have led to the development of soil protein, which is a rapid soil health indicator that can effectively quantify the primary pool of organically bound N in soil (Hurisso et al., 2018b). Soil protein is a sensitive soil health indicator that can detect differences across a wide range of agroecosystems and has been shown to be strongly correlated with maize yields (Roper et al., 2017; Sprunger et al., 2018, 2020). In addition to soil protein, the rate of N mineralization in soil systems can function as an indicator of the quality of organic matter deposits and the rate of nutrient availability for plant use in soil systems. Enzyme activity of N-Acetyl-B-Glucosaminidase (NAG) can also serve as an important measure N mineralization within a system, as well as represent the functional capacity of soil microbial communities.
A major principle of ecological nutrient management is to assess dynamic interactions between organisms and their environment. Quantifying certain microfauna, such as nematode communities, presents an opportunity to not only understand community structure but also ecological function (Laakso et al., 2000; Ferris et al., 2001; Yeates, 2003). Since nematodes range in trophic complexity, they can provide insight into nitrogen cycling (Ferris, 2010). Specifically, bacterial feeders and fungal feeders that are in entry level decomposition channels (colonizer-persister groups 1 and 2), respond rapidly to influxes of resources because of their short life-cycles, and thus benefit more from resource subsidy (Bongers, 1990; Zhang et al., 2017b). Furthermore, bacterivore and fungivore nematodes prey on primary decomposers and can alter the mineralization rates of nutrients needed for plant growth. N mineralization rates can be significantly affected by bacterivore and fungivore grazing as they excrete and spread bacteria and fungi through the soil (Ingham et al., 1985; Chen and Ferris, 1999; Okada and Ferris, 2001). Thus, quantifying the abundances of enrichment opportunist bacterivores (b1) and fungivores (f 2) can indicate N mineralization throughout the soil food web (DuPont et al., 2009).
Here we seek to assess crop productivity and soil health by quantifying key above and belowground N pools in systems that have been managed conventionally vs. ecologically for almost six decades. The trial consists of conventionally managed systems that use chisel tillage and monoculture crops such as continuous corn (Zea mays) and ecological nutrient based systems that include corn rotated with perennial legumes under no-till. The specific objectives are to (1) evaluate N cycling in agroecosystems contrasting in crop rotational diversity and tillage intensity by quantifying plant and soil organic pools of N. (2) Investigate the relationship between nitrogen pools and crop productivity across all systems.
Materials and Methods
Site Description
This study took place at The Northwest Crop Rotation Trial of The Ohio State University (established 1963). The Northwest Crop Rotation hereafter referred to as Hoytville, is located at Custar Wood County, OH at 41°13′N, 83°45′W. The Northwest soil series is a Hoytville Clay Loam and the taxonomic class is a fine, illitic mesic Mollic Epiaqualf. The site is a deep poorly drained soil with a slope range of 0–1% with high shrink swell potential.
Experimental Design
The site is arranged as a full factorial, randomized complete block design, with three blocks and plots being 30.5 × 6.4 m. The tillage treatment consisted of two different tillage intensities: no-till (NT) and chisel till (CT). No-till is zero tillage with the residue of the previous year left on the field. In contrast, chisel till is performed in the spring with 30% of the residue from the previous crop, where the soil is disturbed down to 12 cm. The crop rotation treatment consisted of three levels: corn–corn (Zea mays); corn–soybean (Glycine max) (CS); and a corn–forage–forage (CFF) rotation. The corn was planted in first half of June 2020. For the first 55 years of the experiment, the forage crop in the CFF system consisted of alfalfa (Medicago sativa). However, in 2019, the cropping system changed to include a mix of oats (Avena sativa) and red clover (Trifolium pretense), where the forage system was harvested once in October previous to planting with corn the following spring. This entire study was conducted during the corn phase of each rotation. All corn plots regardless of tillage and crop rotation were fertilized with urea (34–45 kg ha−1) during corn pre-planting in the Spring. Additionally, N fertilizer was added during the V5 corn stage (202 kg ha−1). Phosphorus and potassium was applied depending on soil test results. In 2020, the seeding rate for corn was ~84,014 seeds ha−1.
Aboveground Biomass
Three whole corn plants were randomly collected from each corn plot 2 weeks before grain was removed in October, 2020. The stalk and grain were dried and weighed. Next, the stalk was chipped, and subsamples for each plot were then collected and analyzed for N content using a Costech ECS 2010 CHNSO elemental analyzer. Aboveground biomass (kg/ha−1) (ABB) was calculated by multiplying the weight of one plant by the number of plants per hectare (Equation 1). Aboveground N (kg ha−1) (ABN) and was calculated by multiplying the ABB with the concentration of N (g kg−1).
Fine Root Biomass
Three ingrowth mesh cores were placed into each corn plot 2 weeks post corn emergence to measure the fine root biomass produced by the corn plant during the current growing season (Ontl et al., 2013; Sprunger et al., 2017). In certain cropping systems, ingrowth mesh cores have been found to overestimate fine root biomass, that said, such ingrowth mesh cores are still an excellent method for quantifying fine roots and comparing system level differences (Ontl et al., 2013). Ingrowth cores were made from plastic mesh with a hole size of 2 mm. The plastic mesh was stapled to form a cylinder of 5 cm in diameter × 13 cm long and the bottom was closed with a plastic cap. Soil cores were taken with hammer probes that had the same cylinder length as the mesh plastic cores. Soil was sieved to 4 mm and sand was then added in a 3:1 volume based soil to sand ratio. Ingrowth mesh cores were then filled and placed upright into the 5 cm dimeter × 13 cm depth holes. Stratified random sampling was used in which two ingrowth mesh cores were placed 1.9 cm from the harvest rows and one ingrowth mesh core was placed within one of the harvest rows on each plot in the given site. The ingrowth mesh cores were extracted at the same time as the aboveground biomass. Ingrowth mesh cores were extracted next to the whole corn plants that were collected for aboveground biomass 2 weeks before grain was removed in October 2020. After extraction cores were refrigerated (4°C) until processing. Corn fine roots were processed by a hydropneumatic elutriation and were washed free of soil over a 1 mm sieve. Fine root biomass (FRB) was dried at 60°C for 48 h, weighed, ground to a powder with a mortar and pestle for determination of N concentrations using a CN Elemental Analyzer (Elementar Americas; Mt. Laurel, NJ). Fine root biomass (kg ha−1) was calculated as the fine root core biomass (B) divided by the core area (CA) multiplied by 10,000 cm2/m2 (Equation 2). Fine root N (kg ha−1) (FRN) was calculated by multiplying the FRB (kg ha−1) with the concentration of N (g kg−1).
Soil Sampling
Soil samples were collected twice over the course of the growing season: 2 weeks post planting and just prior to corn harvest. Fifteen soil cores (1.9 cm diameter) were collected down to 10 cm in each plot to make one composite sample. Stratified random sampling was used to collect the soil, in which random soil samples were taken that encompassed the entire plot (Parsons, 2017). Soils were subsampled for elemental and nutrient analysis (5 g), soil moisture (45 g), soil texture (50 g), soil protein (40 g), and enzyme activity analysis (10 g).
Soil Property Analysis
Soils were sent to Spectrum Analytics (Washington Court House, OH) for elemental and nutrient analyses, which included organic matter and pH. Soil organic matter was determined via loss on ignition (Combs and Nathan, 1998). Soil moisture was determined using fresh soil (45 g), that was then dried at 105°C for 24 h, and finally weighed (Supplementary Table 1). Soil texture was assessed using the protocol adapted from (Gee and Bauder, 1986). In summary, the soil was separated into particle size of sand (0.05–2.00 mm), silt (0.002–0.05 mm), and clay (<0.002 mm) through determining the differences on settling rates by Stoke's Law. Soils were pretreated with sodium hexametaphosphate to enhance the separation and dispersion of soil aggregates and a hydrometer was used to measure the density of soil particles in suspension at specific periods of time.
Soil Protein Analysis
Soil protein, which measures the labile pool of N within the soil was determined using methods adapted from Hurisso et al. (2018b). Prior to analysis soils were air dried for 2 days immediately after sampling and on the third day samples were dried in a desiccator at 40°C for 24 h. After drying, samples were sieved and ground to 2 mm. Then, sodium citrate solution (24 ml) was added to the soil (3 g), shaken for 5 min, autoclaved for 30 min at 121°C, cooled for 40 mins, shaken for 30 mins, then the solution (1.5 ml) was transferred to a clean centrifuge tube and centrifuged for 3 mins. Soil protein was quantified using the colorimetric bicinchoninic-acid (BCA) assay (Thermo Scientific, Pierce, Rockford, IL) in a 96-well spectrophotometric plate reader at 562 nm.
Enzyme Sample Processing
N-Acetyl-β-Glucosaminidase (NAG) was measured using the protocol adapted from Tabatabai (1994) and Deng and Popova (2011). Prior to the analysis soils were sieved to 4 mm and refrigerated at 4°C until analyses was performed. Briefly, duplicates of soil (1 g) were placed within an Erlenmeyer flask and NAG Acetate buffer (4 ml) was added. Then 1 ml of p-nitrophenyl-N-Acetyl-β-D glucopyranoside substrate was added to each flask. The sample control was prepared through the same procedure, except no substrate was added to the flask. All samples were then incubated at 37°C for 1 h. After incubation 1 ml of 0.5 M CaCl2 and 4 ml [Tris (hydroxymethyl) aminomethane] THAM (pH 12) were added to terminate the reaction. Then, p-nitrophenyl-N-Acetyl-β-D glucopyranoside substrate (1 mL) was added to all control samples. The soil suspension was filtered, and the absorbance was measured at 415 nm. Enzyme activity was calculated using a calibration curve of standards containing 0, 100, 200, 300, 400, 500, 600, 700, 800, 900, or 1,000 nmol of p-nitrophenol.
Nematode Identification and Calculations
Free-living nematodes are soil microfauna which can detect changes in nutrient enrichment and thus indicate N cycling within varying systems. Free-living nematodes were extracted using the elutriation method (Oostenbrink, 1960). In summary, 100 cc of soil sample was soaked for 24 h, thoroughly stirred, passed through the elutriator, and the solution was collected in 50 ml centrifuge tubes. Next, the solution was processed using the centrifugal sugar flotation method (Hooper et al., 2005). The total number of nematodes were counted in each sample under a microscope at 50× magnification and 100 individuals were identified to family at 100–400×. If the sample contained <100 nematodes all individuals were identified. Nematode counts were expressed as the number of nematodes in each soil fraction (100 g of dry soil fraction). Each nematode was classified as an adult or juvenile to allow the determination of the population stage structure. Nematode taxa was assigned to trophic groups (Yeates, 2003) and functional guilds (Bongers and Bongers, 1998). The Enrichment Index (EI) was calculated using NINJA (Sieriebriennikov et al., 2014). The bacterial feeders cp-1 (b1) and fungal feeders cp-2 (f 2) nematode groups were calculated through the sum of the abundance of all b1 or f 2 of nematode feeding groups within each sample (DuPont et al., 2009).
Statistical Analysis
Normality of data was assessed through the use of studentized residuals plots with MASS in R (Venables and Ripley, 2002). For FRB, FRN, ABB, and ABN, a randomized complete block design Analysis of Variance (ANOVA) was conducted using the PROC MIXED procedure SAS v.9. Means separation was conducted using lsmeans and significant difference was determined at a = 0.05. Treatment was treated as a fixed factors and block was treated as a random factor. For soil protein, NAG, EI, b1, and f2 a repeated measure randomized complete block design ANOVA was conducted using PROC MIXED procedure SAS v.9., with time being the repeated measure variable. Repeated measures was used to account for the variance within each sampling time point. Graphing was performed with ggplot2 in R (Wickham, 2016). Correlations were conducted using the Pearson's method cor.test function R 3.1.1 (R Development Core Team, 2020).
Results
Organic Matter, Soil Texture, and pH
Rotation and tillage had a significant effect on organic matter (p < 0.05). The CC NT and CFF NT systems had significantly greater organic matter than all other systems (Table 1). Soil texture was similar between systems, however, NT systems and CC CT systems appeared to have greater clay content (%) than CS CT and CFF CT systems (Table 1). Lastly, pH was not significantly different between systems (Table 1).
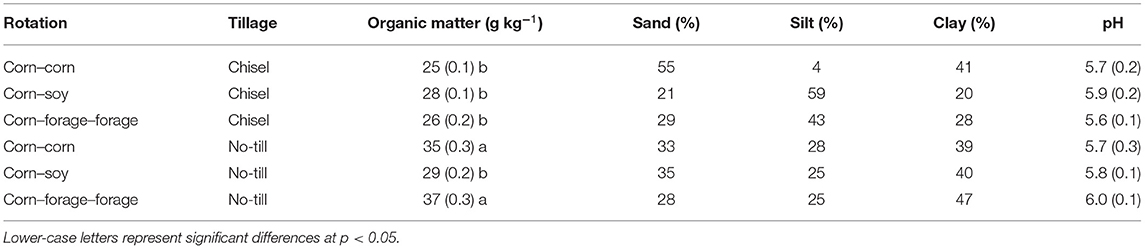
Table 1. Organic matter mean and (SE) (n = 3), sand, silt, clay, and pH mean (SE) (n = 3) sampled in October 2020 from systems comparing three different crop rotations and two tillage intensities.
Quantification of Plant and Soil Nitrogen Pools
Belowground N cycling varied between systems of contrasting tillage intensity and crop rotational diversity. For instance, tillage and crop rotation both had a significant main effect and there was also a significant tillage by crop rotation effect on FRN (p < 0.05). However, tillage was found to have the largest impact on FRN (F = 11.85; Supplementary Table 2). For instance, the CC NT and CFF NT systems had significantly greater FRN compared to all other systems (Table 2). Differences in tillage intensity and crop rotational diversity did not significantly impact FRB (Table 2). However, all NT systems and the CFF CT system FRB values trended higher. Aboveground plant compartments, including ABN and ABB were greatest in the CC NT systems, however, there were no significant effects for aboveground plant material (Table 2).
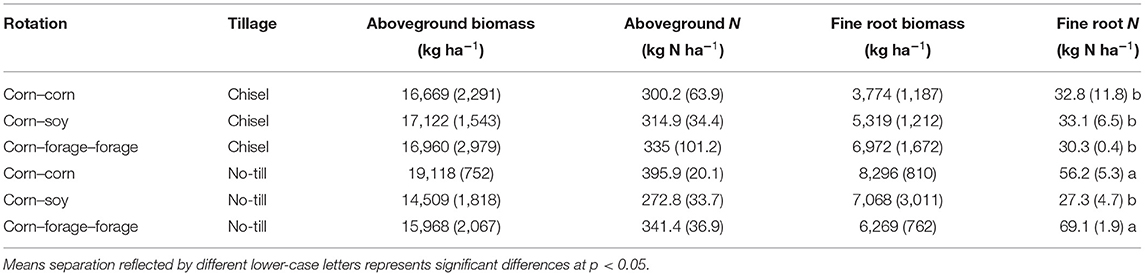
Table 2. Mean and (SE) (n = 3) of biomass and nitrogen content for total aboveground production and fine root production sampled at harvest (October 2020) from a long-term trial comparing tillage and crop rotational diversity in Northwest Ohio.
Soil N cycling was also significantly influenced by tillage intensity and crop rotational diversity. Tillage, rotation, and time were found to have significant main effects on soil protein (p < 0.05). In addition, tillage and rotation had a significant interaction effect on soil protein, however, tillage (F = 21.1) and rotation (F = 21.09) main effects were found to have the greatest impact on soil protein (p < 0.05), relative to the interaction effect between rotation and tillage (Supplementary Table 3). When averaged across all rotations, soil protein was 61% greater in the NT systems relative to the CT systems (Figure 1). The CC NT and CFF NT systems had significantly greater soil protein at harvest relative to all other systems and sampling time points (Figure 1). During planting all CS systems and CC CT systems were found to have reduced soil protein. In harvest, all CT systems and CS NT systems had reduced soil protein when compared to all other systems. Between planting and harvest soil protein increased in CC NT and CFF NT systems.
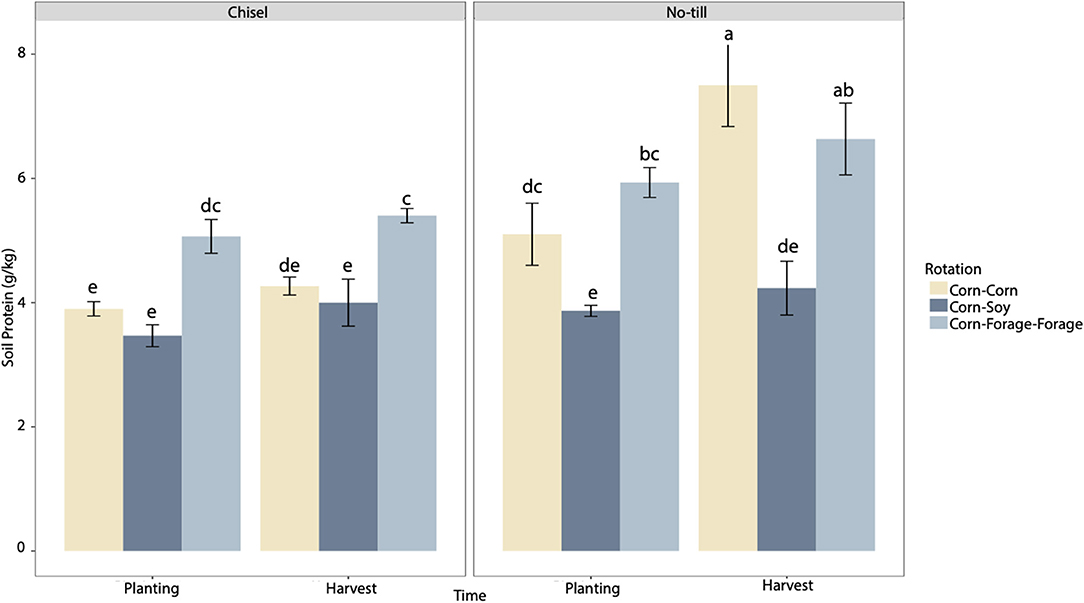
Figure 1. Soil protein (g kg−1) of three different crop rotations (corn–corn, corn–soy, corn–forage–forage) under no-till and chisel till treatments during two sample time points: planting (May 2020) and harvest (October 2020) (n = 3). Error bars represent one standard error of the mean. Means separation reflected by lower-case letters represent significant differences at p < 0.05 across all systems and time points.
Rotation and time both had significant main effects on NAG activity (p < 0.05). In addition, tillage and time had a significant interaction effect on NAG activity (p < 0.05). However, rotation was found to have the greatest impact on NAG activity, when compared to all other factors (F = 8.09) (Supplementary Table 2). NAG enzyme activity was greatest in CFF CT systems during planting relative to all other systems in both planting and harvest (Figure 2). During planting CC NT systems had significantly reduced NAG activity. In addition, at harvest, CS crop rotations appeared to be significantly reduced in NT and CT systems. When comparing dynamics over the course of the growing season, NAG activity was found to decrease over time in all systems except that of CC NT, where NAG activity had elevated values at harvest.
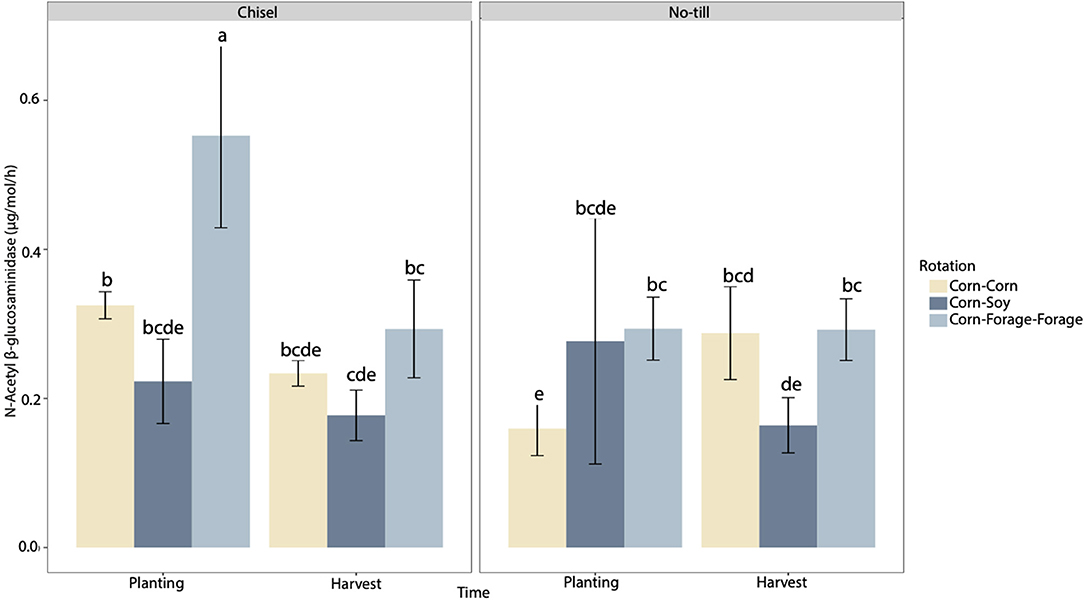
Figure 2. N-Acetyl-B-Glucosaminidase (ug/mol/h) of three different crop rotations (corn–corn, corn–soy, corn–forage–forage) under no-till and chisel till treatments during two time points: planting (May 2020) and harvest (October 2020) (n = 3). Error bars represent one standard error of the mean. Means separation reflected by lower-case letters represent significant differences at p < 0.05 across all systems and time points.
Rotation had a significant effect on b1 enrichment opportunists (F = 4.65, p < 0.05; Supplementary Table 2). Nematode enrichment opportunists (b1) were found to be significantly greater in CS CT systems during planting when compared to CC planting, CS NT planting, CC CT harvest, and CFF CT harvest systems (Table 3). Although time did not have a significant effect, the abundance of b1 enrichment opportunists were found to decrease over time in CT treatments, and increase over time in NT systems. Fungal nematode enrichment opportunists (f 2) and the EI were not significantly impacted by tillage or rotation; however, CFF CT systems had the greatest f 2 abundances and EI (Table 3). Additionally, f2 abundances and EI were found to remain consistent over time.
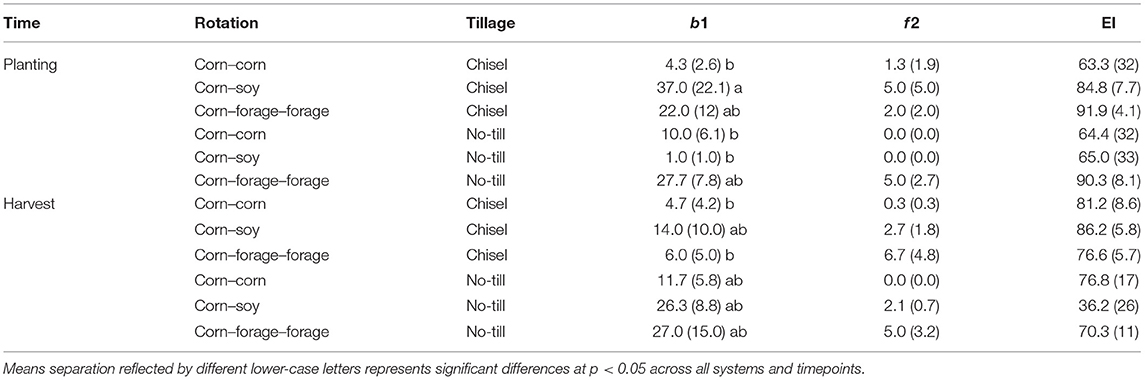
Table 3. Mean and (SE) (n = 3) of the abundance of enrichment opportunist bacterial feeders (b1), abundance of enrichment opportunist fungal feeders (f2), and the Enrichment Index (EI) from planting (May 2020) and harvest (October 2020) from a long-term trial comparing tillage and crop rotational diversity in Northwest Ohio.
Correlations Between Nitrogen Pools and Crop Productivity Across All Systems
Correlations were conducted between all plant and soil N pool indicators at harvest (Table 4). Broadly, positive relationships were found between most plant and soil N pools. Aboveground N was found to be positively and significantly correlated to ABB (Corr = 0.92, p < 0.05). Additionally, ABN had moderate and positive correlations with soil protein (Corr = 0.37, p > 0.05) and FRN (Corr = 0.31, p > 0.05). Aboveground biomass was weakly correlated with all indicators but had more moderate relationships with FRB (Corr = 0.31, p > 0.05), and FRN (Corr = 0.2, p > 0.05). Fine root nitrogen was positively and significantly correlated to soil protein (Corr = 0.60, p < 0.05; Figure 3A). In addition, FRN had moderate correlations with NAG (Corr = 0.34, p > 0.05; Figure 3B), FRB (Corr = 0.24, p > 0.05), and b1 (Corr = 0.50, p > 0.05; Figure 3D). Fine root biomass had weak relationships with all soil N pools, except that of a moderate relationship with NAG (Corr = 0.32) and was marginally significant at p < 0.1 (Figure 3C). NAG activity was found to have a stronger relationship with soil protein but it was not statistically significant (Corr = 0.63, p > 0.05) and NAG had a moderate relationship with nematode EI (Corr = 0.33, p > 0.05). Bacterial and fungal nematode enrichment opportunists had weak relationships to all soil N pools.
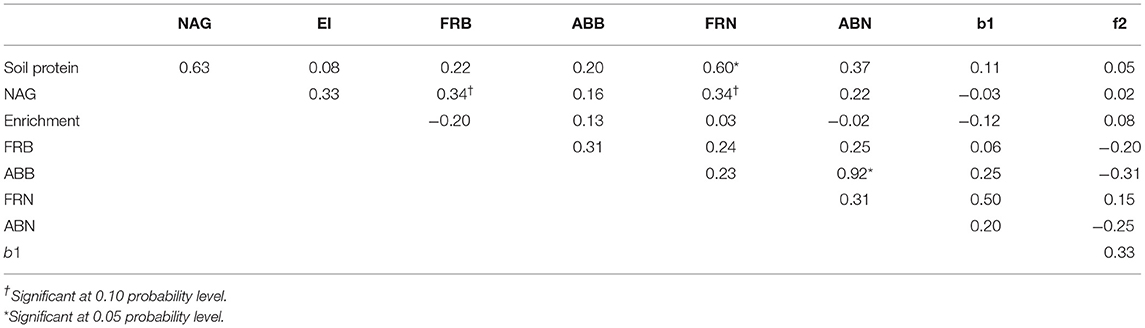
Table 4. Pearson's correlation coefficients (r) between plant and soil nitrogen pool indicators at harvest 2020.
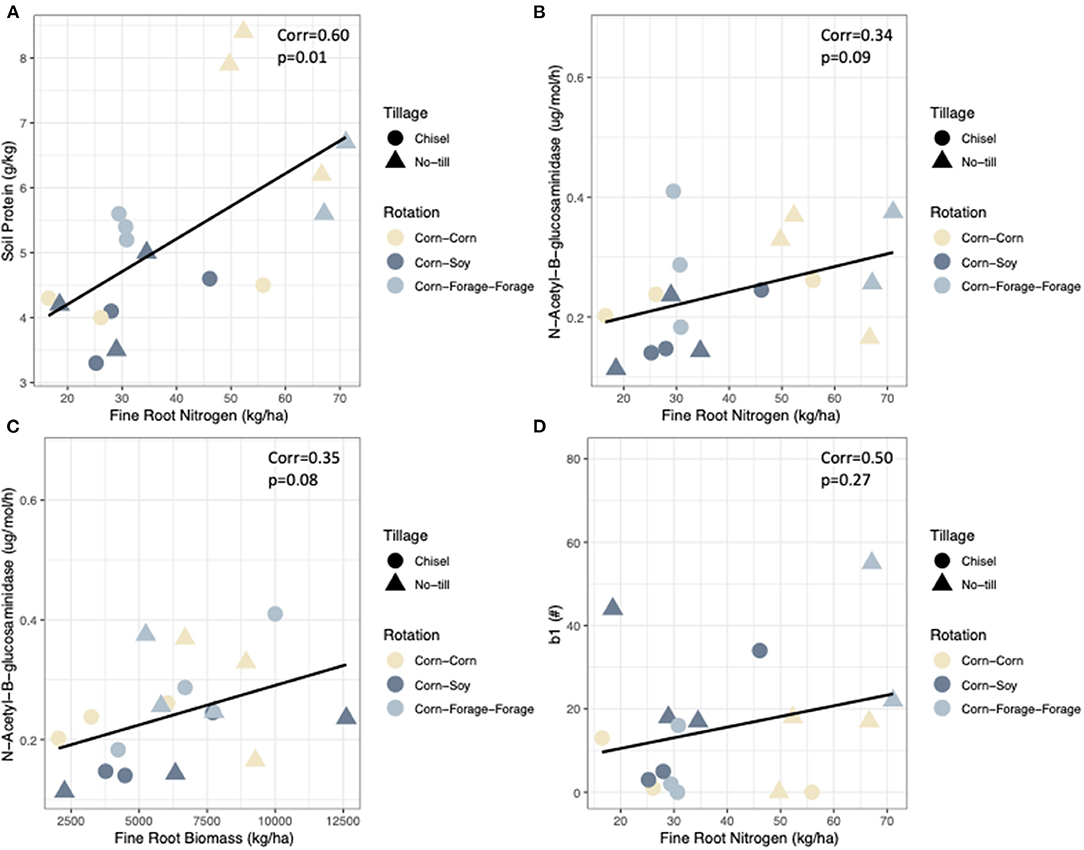
Figure 3. Pearson's correlations between key nitrogen pools across contrasting agroecosystems (A) NAG (ug/mol/h) and FRB (kg ha−1). (B) NAG (ug/mol/h) and FRN (kg ha−1). (C) Soil protein (g kg−1) and FRN (kg ha−1). (D) Enrichment opportunists (b1) and FRN (kg ha−1). The different systems are differentiated by shapes and colors, whereby shapes reflect tillage intensity and color represents crop rotational diversity.
Discussion
Here we used a long-term trial to examine how important organic N pools differ in systems that have been historically managed through the lens of conventional vs. ecological nutrient management. Specifically, we quantified plant and soil N pools in agroecosystems contrasting in crop rotational diversity and tillage intensity. In addition, this study aimed to investigate the relationship between N pools and crop productivity. Systems that incorporated perennial legumes and reduced tillage intensity enhanced belowground fine root N, N mineralization, and organic N pools. Crop productivity was also positively correlated with belowground N cycling, with fine root N being positively correlated to aboveground biomass.
Ecological Nutrient Management Enhances Belowground Plant Nitrogen Pools
We found that belowground N pools of FRN were greater under the no-till systems, where perennial legumes also played a key role, identifying elevated levels under CFF relative to CS rotations. Leguminous perennials have greater fine root quality, and these nutrient deposits may have had an apparent legacy effect, through stimulating the quality of fine roots during the corn phase of the rotation (Frankenberger and Abdelmagid, 1985; Johnson et al., 2007; Tiemann et al., 2015). Generally, annual legume rotations in both intensities were found to have reduced FRN, which may have been due to the previous soybean crop depositing limited belowground biomass, thus reducing the amount of organic N deposited into the labile N pool and decreasing the N available for uptake in the corn crop rotation (Johnson et al., 2007; King et al., 2020). The elevated levels of FRN found within the NT systems, was most likely caused by decreased disturbance enhancing aggregate stability, which can result in greater organic N storage (McDaniel et al., 2014; King et al., 2020). Greater FRN indicates a faster turnover of fine roots, which primes the microbial pool and results in the rapid release of N into the soil system, thus alleviating N limitations (Gardner and Drinkwater, 2009; Dietzel et al., 2015; Tiemann et al., 2015; White et al., 2017; Jilling et al., 2018). Furthermore, the close proximity of fine roots to the soil can allow for greater N accrual through N deposits becoming enmeshed and protected in microaggregates (Rasse et al., 2005; Cates et al., 2016; Cates and Ruark, 2017). Although this study did not find differences among systems in aboveground plant N pools, belowground plant N pools are immensely important for long-term sustainability. For instance, Pugliese et al. (2019) found that belowground N deposited by perennial legume crops can be used toward plant re-growth. Specifically, greater FRN inputs can boost N organic pools and provide a greater amount of plant-available N during critical periods of corn growth, thus allowing for more efficient crop N uptake and yield (Culman et al., 2013; Osterholz et al., 2018; Pugliese et al., 2019). Our results therefore indicate that the use of a perennial legume in a crop rotation, rather than an annual legume, with reduced tillage intensity can enhance soil health through providing greater belowground organic N inputs.
Soil Nitrogen Pools Are Consistently Greater in Systems With Perennial Legumes
Soil N pools, which were measured through soil protein, NAG activity, and nematode enrichment opportunists were persistently greater in systems with perennial legumes, thus indicating improved soil health through organic N storage, and N mineralization. Labile pools of organic N within each system were measured using soil protein and were found to be greater in CC and CFF NT systems. This study also indicates that over the course of the growing season labile N pools increased in systems with the incorporation of a perennial legume. Similar to our findings other studies have also reported that the addition of a perennial legume in crop rotation systems may have also increased bulk N pools (Drinkwater et al., 1998; Jarecki and Lal, 2003; Grandy and Robertson, 2007; Carranca et al., 2015). The increases of soil protein within these systems may be caused by greater organic N inputs from perennial roots within the crop rotations (Cates et al., 2016; Sprunger et al., 2018). Enhanced labile N pools within systems with perennial legumes and less disturbance can increase soil quality, through increasing organic N storage and reducing N losses (Blesh, 2018, 2019). Specifically, perennial legumes have been shown to increase the amount of N in microaggregates (Tiemann et al., 2015). Moreover, labile N represents a pool that can also be easily accessed by soil organisms and made readily available to plants (Hurisso et al., 2018a). Thus, increasing the labile N pool also indicates a greater amount of organic N that can be utilized by subsequent crops (Blesh and Drinkwater, 2013). The increased organic N storage in systems of greater perenniality can also lead to reduced inorganic N additions (Bowles et al., 2020).
Systems where perennial legumes were incorporated also had greater N mineralization, which demonstrates the ability of these systems to provide greater N for crop uptake thus increasing crop productivity and soil health. Specifically, CFF rotations were found to have greater NAG enzyme activity, which represents the rate of microbial nutrient uptake and the chemical transformation of N (Tabatabai, 1994). In this study, the increase in NAG activity may be caused by perennial legumes within the crop rotation increasing substrate availability through enhanced N inputs (Geyer et al., 2016; Jilling et al., 2018). Additionally, our study indicated that CS rotations had reduced NAG enzyme activity, which may be caused by reduced biomass inputs from the previous soy crop, which decreased substrate availability for enzymes (McDaniel et al., 2014; Cates and Ruark, 2017). Our results indicated that the use of a perennial legume in annual crop rotations can augment N mineralization, which leads to improved soil health. That said, numerous other studies have found that lengthening rotations with annual legumes can also successfully enhance N pools and soil health (McDaniel et al., 2014; Tiemann et al., 2015; Perrone et al., 2020). The incorporation of legume cover crops has been shown to increase labile N pools relative to monocultures. For example, Liebman et al. (2018) found that hairy vetch (Vicia villosa var. AU Early Cover, HV) was successful at contributing the greatest levels of N into a corn based system relative to other cover crops, resulting in greater levels of inorganic N. Greater N pools in more diversified systems is likely due to the response of microbial communities leading to larger nutrient pools and enhanced soil quality (Bach and Hofmockel, 2015; Kallenbach et al., 2015). Enhanced enzyme activity in diverse systems has also been shown to positively affect aggregate formation and therefore lead to greater N retention (Tiemann et al., 2015; Austin et al., 2017).
In general, the nutrient enrichment opportunist values were highly variable and it was diffecult to detect an overall trend. That said, our results indicate that the CS system had a greater supply of N and enhanced nutrient enrichment than compared to CC at planting. Similarly, DuPont et al. (2009) also found greater b1 abundances in legume cover cropped systems compared to grain cover cropped systems. Monoculture systems lack diverse nutrient inputs, which may cause a decline in nutrient enrichment over time when compared to perennial and polyculture systems (DuPont et al., 2009, 2010; Tiemann et al., 2015; Song et al., 2016; Zhang et al., 2017b; Sprunger et al., 2019a; Wattenburger et al., 2019). More interesting is that both NAG and soil protein were found to be reduced in systems where b1 abundances were greater. This finding is parallel to Ugarte et al. (2013), which concluded that biologically based indicators may not be as effective as overall soil quality indicators. The incongruities between soil biological health indicators and overall soil quality indicators may be caused by the fact that soil biological indicators are more sensitive to changes in management and therefore reflect a more current state of soil function (Culman et al., 2013). These findings have important implications for plant growth, as bacterivore enrichment opportunists can accelerate the N mineralization rates and thus N availability for crop uptake (Yeates, 1999; Zhang et al., 2017b). Additionally, these results indicate that rotational systems that use a perennial or annual legume may result in greater N mineralization from soil fauna.
While enzymatic activity and soil protein were consistently greater in the CFF system, FRN and the enrichment of bacterial feeding nematodes were equivalent between CFF and CC systems. Despite these similarities, CFF systems are still more advantageous from an ecological nutrient management perspective relative to the CC systems. The perennial legumes are able to provide additional sources of N due to biological N fixation, and ultimately enhance N use efficiency within the crop rotation (Fujita et al., 1992). Roots of perennial legumes can allocate large amounts of organic N belowground, which can then be used for subsequent crop uptake from annual systems (Kavdir et al., 2005; Jungers et al., 2019). This additional source of N from perennial legumes can then reduce the amount of external inorganic N fertilizers needed to sustain subsequent corn crop yields (Yost et al., 2012; Blesh and Drinkwater, 2013).
Interactions Between Plant and Soil Nitrogen Pools Are Positively Related to Crop Productivity
This study found that plant–soil N pools, which were elevated in systems under ecological nutrient management, may result in enhanced crop productivity and soil health. Our correlation analysis revealed that ABN had positive relationships with soil protein, which indicates that enhanced soil quality is essential for greater crop productivity. These findings are supported by Glover et al. (2010) reporting that yields should be increased through enhanced soil quality rather than increased N fertilization. Moreover, this study indicates that ecologically managed systems can improve soil protein, thus indicating that these management strategies can also enhance crop productivity. We also found a positive relationships between FRN and soil protein, which indicates that FRN and soil protein may have a cyclical relationship in which the enhancement of both belowground plant and soil N pools can increase crop productivity. Furthermore, FRN was positively correlated with b1 abundances and NAG, representing a positive relationship between N mineralization and FRN. These positive relationships further reaffirm that the enhanced functioning of soil microbiomes is necessary for the cycling of nutrients and crop productivity (Griffiths, 1994; DuPont et al., 2009; Jansson and Hofmockel, 2020). Furthermore, these findings indicate that improving N cycling between plant and soil pools is essential for creating agricultural systems that can retain N and sustain yields (Sprunger et al., 2019b).
Ecological Nutrient Management Provides a Framework for Resilient Food Systems
Our study found that agricultural practices that fall within the ecological nutrient management framework such as increased crop diversity through the use of perennial legumes appear to play an important role in enhancing nutrient pools that bolster soil quality. This is especially important as improved soil quality is a key element within the United Nations Sustainability Goal 2.4, which seeks to implement agricultural practices that progressively improve land and soil quality (UN Sustainable Development Goals, 2020). While ecological nutrient management practices did not have a clear advantage in terms of crop production in this study, the increased organic nutrient pools found in the CFF systems could be important for prolonged food production and climate resiliency. In recent years, the Midwestern United States has experienced enhanced flooding due to extreme rainfall events, which has been shown to reduce the availability of inorganic N and substantially reduce crop yields (Alaoui-Sosse et al., 2005; Yin et al., 2020). Furthermore, increased perenniality within agroecosystems could provide more flexibility for farmers, where rotations could remain under forage crops. This could be especially important in regions where early summer flooding events prevent the planting of annual row-crops, as was evident across the Midwestern Corn Belt in 2019 (Lawal et al., 2021). That said, there are certainly food security trade-offs associated with leaving rotations under perennial forage, as increased acreage under forages reduces overall food production for human consumption (Reckling et al., 2016). For this reason, it will also be important to consider other types of perennial crops that could be produced for both grain and forage, including perennial intermediate wheat grass and perennial cereal rye (Ryan et al., 2018; Pugliese et al., 2019). Thus, perennializing agriculture could serve as an effective way toward meeting sustainable food production goals in the face of climate change. However, such alterations will take time as there are currently barriers to breeding, weed management, and market opportunities in perennial grain cropping systems (Ryan et al., 2018).
Conclusion
Ecological nutrient management strategies including the incorporation of perennial legumes into crop rotations and reduced tillage intensity present a solution for creating resilient agroecosystems because of their ability to enhance key rhizosphere processes. Our findings demonstrate that crop rotations that are lengthened with perennial legumes are capable of increasing belowground organic N inputs, N cycling through microbial communities and microfauna, and soil organic N storage. Moreover, perennial legumes in conjunction with no-till management have the capacity to increase soil health and sustain crop productivity. That said, continuous corn systems were also able to sustain certain soil N dynamics and fine root N levels when compared to corn rotated with perennial legumes. Despite these similarities, rotations that include perennial legumes are still more adventagous from an ecological nutrient management perspective as such rotations have more efficient N cycling and are less dependant on external fertilizer inputs. The positive relationships found between belowground plant N pools, and soil N pools demonstrates the need for agroecosystems that maximize belowground production, as rhizosphere processes are essential for improved soil health and building resilient agroecosystems. Perennial crops and legumes in conjunction with reduced tillage intensity should be considered when working to design agroecosystems that seek to meet United Nations Sustainability Goals related to food production and climate adaptation.
Data Availability Statement
The original contributions presented in the study are included in the article/Supplementary Material, further inquiries can be directed to the corresponding author.
Author Contributions
All authors listed have made a substantial, direct and intellectual contribution to the work, and approved it for publication.
Funding
This work was funded by the Ohio State University. TM was supported by the Ohio State University Inclusive Excellence Fellowship. This work was also supported by a USDA-NIFA Sustainable Agriculture Research and Education (SARE) program Graduate Student Grant (Project no. GNC20-308).
Conflict of Interest
The authors declare that the research was conducted in the absence of any commercial or financial relationships that could be construed as a potential conflict of interest.
Publisher's Note
All claims expressed in this article are solely those of the authors and do not necessarily represent those of their affiliated organizations, or those of the publisher, the editors and the reviewers. Any product that may be evaluated in this article, or claim that may be made by its manufacturer, is not guaranteed or endorsed by the publisher.
Acknowledgments
We would like to thank Meredith Mann for providing feedback and conducting essential analyses for this article. We would also like to thank the field technicians that maintain the Northwest Crop Rotation Trial at the Ohio State University.
Supplementary Material
The Supplementary Material for this article can be found online at: https://www.frontiersin.org/articles/10.3389/fsufs.2021.705577/full#supplementary-material
References
Agovino, M., Casaccia, M., Ciommi, M., Ferrara, M., and Marchesano, K. (2019). Agriculture, climate change and sustainability: the case of EU-28. Ecol. Indic. 105, 525–543. doi: 10.1016/j.ecolind.2018.04.064
Alaoui-Sosse, B., Gerard, B., Binet, P., Toussant, M. L., and Badot, P. (2005). Influence of flooding on growth, nitrogen availability in soil, and nitrate reduction of young oak seedlings (Quercus robur L.). Ann. For. Sci. 62, 593–600. doi: 10.1051/forest
Austin, E. E., Wickings, K., McDaniel, M. D., Robertson, G. P., and Grandy, A. S. (2017). Cover crop root contributions to soil carbon in a no-till corn bioenergy cropping system. GCB Bioenergy 9, 1252–1263. doi: 10.1111/gcbb.12428
Bach, E. M., and Hofmockel, K. S. (2015). Coupled carbon and nitrogen inputs increase microbial biomass and activity in prairie bioenergy systems. Ecosystems 18, 417–427. doi: 10.1007/s10021-014-9835-8
Billen, G., Garnier, J., and Lassaletta, L. (2013). The nitrogen cascade from agricultural soils to the sea: modelling nitrogen transfers at regional watershed and global scales. Philos. Trans. R. Soc. B Biol. Sci. 368:20130123. doi: 10.1098/rstb.2013.0123
Blesh, J. (2018). Functional traits in cover crop mixtures: biological nitrogen fixation and multifunctionality. J. Appl. Ecol. 55, 38–48. doi: 10.1111/1365-2664.13011
Blesh, J. (2019). Feedbacks between nitrogen fixation and soil organic matter increase ecosystem functions in diversified agroecosystems. Ecol. Appl. 29:e01986. doi: 10.1002/eap.1986
Blesh, J., and Drinkwater, L. E. (2013). The impact of nitrogen source and crop rotation on nitrogen mass balances in the Mississippi River Basin. Ecol. Appl. 23, 1017–1035. doi: 10.1890/12-0132.1
Bongers, T. (1990). The maturity index: an ecological measure of environmental disturbance based on nematode species composition. Oecologia 83, 14–19. doi: 10.1007/BF00324627
Bongers, T., and Bongers, M. (1998). Functional diversity of nematodes. Appl. Soil Ecol. 10, 239–251. doi: 10.1016/S0929-1393(98)00123-1
Bowles, T. M., Mooshammer, M., Socolar, Y., Calderón, F., Cavigelli, M. A., Culman, S. W., et al. (2020). Long-term evidence shows that crop-rotation diversification increases agricultural resilience to adverse growing conditions in North America. One Earth 2, 284–293. doi: 10.1016/j.oneear.2020.02.007
Carranca, C., Torres, M. O., and Madeira, M. (2015). Underestimated role of legume roots for soil N fertility. Agron. Sustain. Dev. 35, 1095–1102. doi: 10.1007/s13593-015-0297-y
Cates, A. M., and Ruark, M. D. (2017). Soil aggregate and particulate C and N under corn rotations: responses to management and correlations with yield. Plant Soil 415, 521–533. doi: 10.1007/s11104-016-3121-9
Cates, A. M., Ruark, M. D., Hedtcke, J. L., and Posner, J. L. (2016). Long-term tillage, rotation and perennialization effects on particulate and aggregate soil organic matter. Soil Tillage Res. 155, 371–380. doi: 10.1016/j.still.2015.09.008
Chen, J., and Ferris, H. (1999). The effects of nematode grazing on nitrogen mineralization during fungal decomposition of organic matter. Soil Biol. Biochem. 31, 1265–1279. doi: 10.1016/S0038-0717(99)00042-5
Combs, M., and Nathan, M. V. (1998). “Soil organic matter,” in Recommended Chemical Soil Test Procedures for the North Central Region (Revised), ed. J. R. Brown. North Central Regional Research Pub. 221 (Missouri: Missouri Agricultural).
Conant, R. T., Cerri, C. E. P., Osborne, B. B., and Paustian, K. (2017). Grassland management impacts on soil carbon stocks: a new synthesis. Ecol. Appl. 27, 662–668. doi: 10.1002/eap.1473
Crews, T. E., and Peoples, M. B. (2005). Can the synchrony of nitrogen supply and crop demand be improved in legume and fertilizer-based agroecosystems? A Review. Nutr. Cycl. Agroecosyst. 72, 101–120. doi: 10.1007/s10705-004-6480-1
Culman, S. W., Snapp, S. S., Green, J. M., and Gentry, L. E. (2013). Short- and long-term labile soil carbon and nitrogen dynamics reflect management and predict corn agronomic performance. Agron. J. 105:493. doi: 10.2134/agronj2012.0382
Dawson, J. C., Huggins, D. R., and Jones, S. S. (2008). Characterizing nitrogen use efficiency in natural and agricultural ecosystems to improve the performance of cereal crops in low-input and organic agricultural systems. Field Crops Res. 107, 89–101. doi: 10.1016/j.fcr.2008.01.001
Deng, S., and Popova, I. (2011). “Carbohydrate Hydrolases,” in Methods of Soil Enzymology (New York, NY: Wiley) 185–209. doi: 10.2136/sssabookser9.c9
Dietzel, R., Jarchow, M. E., and Liebman, M. (2015). Above- and belowground growth, biomass, and nitrogen use in maize and reconstructed prairie cropping systems. Crop Sci. 55:910. doi: 10.2135/cropsci2014.08.0572
Drinkwater, L., Wagoner, P., and Sarrantonio, M. (1998). Legume-based cropping systems have reduced carbon and nitrogen losses. Nature 396, 262–265. doi: 10.1038/24376
Drinkwater, L. E., and Snapp, S. S. (2007a). Nutrients in agroecosystems: rethinking the management paradigm. Adv. Agron. 92, 163–186. doi: 10.1016/S0065-2113(04)92003-2
Drinkwater, L. E., and Snapp, S. S. (2007b). “Understanding and managing the rhizosphere in agroecosystems” in The Rhizosphere (Amsterdam: Elseiver), 127–153. doi: 10.1016/B978-012088775-0/50008-2
DuPont, S. T., Culman, S. W., Ferris, H., Buckley, D. H., and Glover, J. D. (2010). No-tillage conversion of harvested perennial grassland to annual cropland reduces root biomass, decreases active carbon stocks, and impacts soil biota. J. Agric. Ecol. Environ. 137, 25–32. doi: 10.1016/j.agee.2009.12.021
DuPont, S. T., Ferris, H., and Van Horn, M. (2009). Effects of cover crop quality and quantity on nematode-based soil food webs and nutrient cycling. Appl. Soil Ecol. 41, 157–167. doi: 10.1016/j.apsoil.2008.10.004
Ferris, H. (2010). Contribution of nematodes to the structure and function of the soil food web. J. Nematol. 42, 63–67. doi: 10.1016/0929-1393(95)00071-2
Ferris, H., Bongers, T., and de Goede, R. G. M. (2001). A framework for soil food web diagnostics: extension of the nematode faunal analysis concept. Appl. Soil Ecol. 18, 13–29. doi: 10.1016/S0929-1393(01)00152-4
Frankenberger, W. T., and Abdelmagid, H. M. (1985). Kinetic parameters of nitrogen mineralization rates of leguminous crops incorporated into soil. Plant Soil 87, 257–271. doi: 10.1007/BF02181865
Fujita, K., Ofosu-Budu, K. G., and Ogata, S. (1992). Biological nitrogen fixation in mixed legume-cereal cropping systems. Plant Soil 141, 155–175.
Gardner, J. B., and Drinkwater, L. E. (2009). The fate of nitrogen in grain cropping systems: a meta-analysis of 15N field experiments. Ecol. Appl. 19, 2167–2184. doi: 10.1890/08-1122.1
Gee, G. W., and Bauder, J. W. (1986). “Particle-size analysis,” in Methods of Soil Analysis. Physical and Mineralogical Methods. Agronomy Monograph 9, 2th Edn, ed A. Klute (Madison, WI: American Society of Agronomy), 383–411.
Geyer, K. M., Kyker-Snowman, E., Grandy, A. S., and Frey, S. D. (2016). Microbial carbon use efficiency: accounting for population, community, and ecosystem-scale controls over the fate of metabolized organic matter. Biogeochemistry 127, 173–188. doi: 10.1007/s10533-016-0191-y
Glover, J. D., Culman, S. W., DuPont, S. T., Broussard, W., Young, L., Mangan, M. E., et al. (2010). Harvested perennial grasslands provide ecological benchmarks for agricultural sustainability. Agric. Ecosyst. Environ. 137, 3–12. doi: 10.1016/j.agee.2009.11.001
Gordon, W. S., and Jackson, R. B. (2000). Nutrient concentrations in fine roots. Ecology 81, 275–280. doi: 10.1890/0012-9658(2000)081[0275:NCIFR]2.0.CO;2
Grandy, A. S., and Robertson, G. P. (2007). Land-use intensity effects on soil organic carbon accumulation rates and mechanisms. Ecosystems 10, 59–74. doi: 10.1007/s10021-006-9010-y
Griffiths, B. S. (1994). Microbial-feeding nematodes and protozoa in soil: their effectson microbial activity and nitrogen mineralization in decomposition hotspots and the rhizosphere. Plant Soil 164, 25–33. doi: 10.1007/BF00010107
Hooper, H. J., Hallmann, J., and Subbotin, S. A. (2005). “Methods for extraction and detection of plant and soil nematodes,” in Plant Parasitic Nematodes in Subtropical and Tropical Agriculture (Egham: CABI), 2.
Hurisso, T. T., Culman, S. W., and Zhao, K. (2018a). Repeatability and spatiotemporal variability of emerging soil health indicators relative to routine soil nutrient tests. Soil Sci. Soc. Am. J. 82, 939–948. doi: 10.2136/sssaj2018.03.0098
Hurisso, T. T., Moebius-Clune, D. J., Culman, S. W., Moebius-Clune, B. N., Thies, J. E., and van Es, H. M. (2018b). Soil protein as a rapid soil health indicator of potentially available organic nitrogen. Agric. Environ. Lett. 3:180006er. doi: 10.2134/ael2018.02.0006er
Ingham, R. E., Trofymow, J. A., Ingham, E. R., and Coleman, D. C. (1985). Interactions of bacteria, fungi, and their nematode grazers: effects on nutrient cycling and plant growth. Ecol. Monogr. 55, 119–140. doi: 10.2307/1942528
Jackson, L. E. (2000). Fates and losses of nitrogen from a nitrogen-15-labeled cover crop in an intensively managed vegetable system. Soil Sci. Soc. Am. J. 64, 1404–1412. doi: 10.2136/sssaj2000.6441404x
Jansson, J. K., and Hofmockel, K. S. (2020). Soil microbiomes and climate change. Nat. Rev. Microbiol. 18, 35–46. doi: 10.1038/s41579-019-0265-7
Jarecki, M. K., and Lal, R. (2003). Crop management for soil carbon sequestration. Crit. Rev. Plant Sci. 22, 471–502. doi: 10.1080/713608318
Jiao, Y., Whalen, J. K., and Hendershot, W. H. (2006). No-tillage and manure applications increase aggregation and improve nutrient retention in a sandy-loam soil. Geoderma 134, 24–33. doi: 10.1016/j.geoderma.2005.08.012
Jilling, A., Keiluweit, M., Contosta, A. R., Frey, S., Schimel, J., Schnecker, J., et al. (2018). Minerals in the rhizosphere: overlooked mediators of soil nitrogen availability to plants and microbes. Biogeochemistry 139, 103–122. doi: 10.1007/s10533-018-0459-5
Johnson, J. M.-F., Barbour, N. W., and Weyers, S. L. (2007). Chemical composition of crop biomass impacts its decomposition. Soil Sci. Soc. Am. J. 71, 155–162. doi: 10.2136/sssaj2005.0419
Jungers, J. M., DeHaan, L. H., Mulla, D. J., Sheaffer, C. C., and Wyse, D. L. (2019). Reduced nitrate leaching in a perennial grain crop compared to maize in the Upper Midwest, USA. Agric. Ecosyst. Environ. 272, 63–73. doi: 10.1016/j.agee.2018.11.007
Kallenbach, C. M., Grandy, A. S., Frey, S. D., and Diefendorf, A. F. (2015). Microbial physiology and necromass regulate agricultural soil carbon accumulation. Soil Biol. Biochem. 91, 279–290. doi: 10.1016/j.soilbio.2015.09.005
Kavdir, Y., Rasse, D. P., and Smucker, A. J. M. (2005). Specific contributions of decaying alfalfa roots to nitrate leaching in a Kalamazoo loam soil. Agric. Ecosyst. Environ. 109, 97–106. doi: 10.1016/j.agee.2005.02.020
King, A. E., Congreves, K. A., Deen, B., Dunfield, K. E., Simpson, M. J., Voroney, R. P., et al. (2020). Crop rotations differ in soil carbon stabilization efficiency, but the response to quality of structural plant inputs is ambiguous. Plant Soil 457, 207–224. doi: 10.1007/s11104-020-04728-5
Laakso, J., Setäl,ä, H., and Palojärvi, A. (2000). Influence of decomposer food web structure and nitrogen availability on plant growth. Plant Soil 225, 153–165. doi: 10.1023/A:1026534812422
Lawal, A., Kerner, H., Becker-Reshef, I., and Meyer, S. (2021). Mapping the location and extent of 2019 prevent planting acres in south dakota using remote sensing techniques. Remote Sens. 13:2430. doi: 10.3390/rs13132430
Liebman, A. M., Grossman, J., Brown, M., Wells, M. S., Reberg-Horton, S. C., and Shi, W. (2018). Legume cover crops and tillage impact nitrogen dynamics in organic corn production. Agron. J. 110, 1046–1057. doi: 10.2134/agronj2017.08.0474
McDaniel, M. D., Tiemann, L. K., and Grandy, A. S. (2014). Does agricultural crop diversity enhance soil microbial biomass and organic matter dynamics? A meta-analysis. Ecol. Appl. 24, 560–570. doi: 10.1890/13-0616.1
Moebius-Clune, B. N., van Es, H. M., Idowu, O. J., Schindelbeck, R. R., Moebius-Clune, D. J., Wolfe, D. W., et al. (2008). Long-term effects of harvesting maize stover and tillage on soil quality. Soil Sci. Soc. Am. J. 72, 960–969. doi: 10.2136/sssaj2007.0248
Okada, H., and Ferris, H. (2001). Effect of temperature on growth and nitrogen mineralization of fungi and fungal-feeding nematodes. Plant Soil 234, 253–262. doi: 10.1023/A:1017957929476
Ontl, T. A., Hofmockel, K. S., Cambardella, C. A., Schulte, L. A., and Kolka, R. K. (2013). Topographic and soil influences on root productivity of three bioenergy cropping systems. New Phytol. 199, 727–737. doi: 10.1111/nph.12302
Oostenbrink, M. (1960). “The family Criconematidae,” in Nematology. Fundamentals and Recent Advances with Emphasis on Plant Parasitic and Soil Forms (Chapel Hill: The University of North Carolina Press), 196–205.
Osterholz, W. R., Liebman, M., and Castellano, M. J. (2018). Can soil nitrogen dynamics explain the yield benefit of crop diversification? Field Crops Res. 219, 33–42. doi: 10.1016/j.fcr.2018.01.026
Parsons, V. L. (2017). “Stratified Sampling,” in Wiley Stats Ref: Statisitcs Reference Online (Hoboken, NJ: Wiley). doi: 10.1002/9781118445112.stat05999.pub2
Perrone, S., Grossman, J., Liebman, A., Sooksa-nguan, T., and Gutknecht, J. (2020). Nitrogen fixation and productivity of winter annual legume cover crops in Upper Midwest organic cropping systems. Nutr. Cycl. Agroecosyst. 117, 61–76. doi: 10.1007/s10705-020-10055-z
Pugliese, J. Y., Culman, S. W., and Sprunger, C. D. (2019). Harvesting forage of the perennial grain crop kernza (Thinopyrum intermedium) increases root biomass and soil nitrogen cycling. Plant Soil 437, 241–254. doi: 10.1007/s11104-019-03974-6
R Development Core Team (2020). R: A Language and Environment for Statistical Computing. Vienna: R Foundation for Statistical Computing.
Rasse, D. P., Rumpel, C., and Dignac, M.-F. (2005). Is soil carbon mostly root carbon? Mechanisms for a specific stabilisation. Plant Soil 269, 341–356.
Reckling, M., Bergkvist, G., Watson, C. A., Stoddard, F. L., Zander, P. M., Walker, R. L., et al. (2016). Trade-offs between economic and environmental impacts of introducing legumes into cropping systems. Front. Plant Sci. 7:669. doi: 10.3389/fpls.2016.00669
Robertson, G., and Vitousek, P. (2009). Nitrogen in agriculture: balancing the cost of an essential resource. Annu Rev. Environ. Resourc. 34, 97–125. doi: 10.1146/ANNUREV.ENVIRON.032108.105046
Roper, W. R., Osmond, D. L., Heitman, J. L., Wagger, M. G., and Reberg-Horton, S. C. (2017). Soil health indicators do not differentiate among agronomic management systems in North Carolina soils. Soil Sci. Soc. Am. J. 81, 828–843. doi: 10.2136/sssaj2016.12.0400
Ryan, M. R., Crews, T. E., Culman, S. W., DeHaan, L. R., Hayes, R. C., Jungers, J. M., et al. (2018). Managing for multifunctionality in perennial grain crops. Bioscience 68, 294–304. doi: 10.1093/biosci/biy014
Sieriebriennikov, B., Ferris, H., and de Goede, R. G. M. (2014). NINJA: an automated calculation system for nematode-based biological monitoring. Eur. J. Soil Biol. 61, 90–93. doi: 10.1016/j.ejsobi.2014.02.004
Song, M., Li, X., Jing, S., Lei, L., Wang, J., and Wan, S. (2016). Responses of soil nematodes to water and nitrogen additions in an old-field grassland. Appl. Soil Ecol. 102, 53–60. doi: 10.1016/j.apsoil.2016.02.011
Sprunger, C. D., Culman, S. W., Palm, C. A., Thuita, M., and Vanlauwe, B. (2019b). Long-term application of low C:N residues enhances maize yield and soil nutrient pools across Kenya. Nutr. Cycl. Agroecosyst. 114, 261–276. doi: 10.1007/s10705-019-10005-4
Sprunger, C. D., Culman, S. W., Peralta, A. L., DuPont, S. T., Lennon, J. T., and Snapp, S. S. (2019a). Perennial grain crop roots and nitrogen management shape soil food webs and soil carbon dynamics. Soil Biol. Biochem. 137, 107573. doi: 10.1016/j.soilbio.2019.107573
Sprunger, C. D., Culman, S. W., Robertson, G. P., and Snapp, S. S. (2018). How does nitrogen and perenniality influence belowground biomass and nitrogen use efficiency in small grain cereals? Crop Sci. 58:2110. doi: 10.2135/cropsci2018.02.0123
Sprunger, C. D., Martin, T., and Mann, M. (2020). Systems with greater perenniality and crop diversity enhance soil biological health. Agric. Environ. Lett. 5:e20030. doi: 10.1002/ael2.20030
Sprunger, C. D., Oates, L. G., Jackson, R. D., and Robertson, G. P. (2017). Plant community composition influences fine root production and biomass allocation in perennial bioenergy cropping systems of the upper Midwest, USA. Biomass Bioenergy 105, 248–258. doi: 10.1016/j.biombioe.2017.07.007
Syswerda, S. P., Basso, B., Hamilton, S. K., Tausig, J. B., and Robertson, G. P. (2012). Long-term nitrate loss along an agricultural intensity gradient in the Upper Midwest USA. Agric. Ecosyst. Environ. 149, 10–19. doi: 10.1016/j.agee.2011.12.007
Tabatabai, M. A. (1994). “Soil enzymes,” in Methods of Soil Analysis (New York, NY: Wiley), 775–833. doi: 10.2136/sssabookser5.2.c37
Tiemann, L. K., Grandy, A. S., Atkinson, E. E., Marin-Spiotta, E., and McDaniel, M. D. (2015). Crop rotational diversity enhances belowground communities and functions in an agroecosystem. Ecol. Lett. 18, 761–771. doi: 10.1111/ele.12453
Ugarte, C. M., Zaborski, E. R., and Wander, M. M. (2013). Nematode indicators as integrative measures of soil condition in organic cropping systems. Soil Biol. Biochem. 64, 103–113. doi: 10.1016/j.soilbio.2013.03.035
UN Sustainable Development Goals (2020). The Sustainable Development Goals Report. Available online at: https://unstats.un.org/sdgs/report/2020/The-Sustainable-Development-Goals-Report-2020.pdf (accessed April 12, 2021).
Venables, W. N., and Ripley, B. D. (2002). Random and mixed effects, in Modern Applied Statistics with S, eds W. N. Venables and B. D. Ripley (New York, NY: Springer), 271–300. doi: 10.1007/978-0-387-21706-2_10
Wattenburger, C. J., Halverson, L. J., and Hofmockel, K. S. (2019). Agricultural management affects root-associated microbiome recruitment over maize development. Phytobiomes J. 3, 260–272. doi: 10.1094/PBIOMES-03-19-0016-R
White, C. M., DuPont, S. T., Hautau, M., Hartman, D., Finney, D. M., Bradley, B., et al. (2017). Managing the trade off between nitrogen supply and retention with cover crop mixtures. Agric. Ecosyst. Environ. 237, 121–133. doi: 10.1016/j.agee.2016.12.016
Yeates, G. W. (1999). Effects of plants on nematode community structure. Annu. Rev. Phytopathol. Palo Alto 37:127. doi: 10.1016/B978-0-444-50019-9.50010-8
Yeates, G. W. (2003). Nematodes as soil indicators: functional and biodiversity aspects. Biol. Fertil. Soils 37, 199–210. doi: 10.1007/s00374-003-0586-5
Yin, Y., Byrne, B., Liu, J., Wennberg, P., Davis, K. J., Magney, T., et al. (2020). Cropland carbon uptake delayed and reduced by 2019 Midwest floods. AGU Adv. 1:e2019AV000140. doi: 10.1029/2019AV000140
Yost, M. A., Coulter, J. A., Russelle, M. P., Sheaffer, C. C., and Kaiser, D. E. (2012), Alfalfa nitrogen credit to first-year corn: potassium, regrowth, tillage timing effects. Agron J. 104, 953–962. doi: 10.2134/agronj2011.0384
Zhang, R., Vivanco, J. M., and Shen, Q. (2017a). The unseen rhizosphere root–soil–microbe interactions for crop production. Curr. Opin. Microbiol. 37, 8–14. doi: 10.1016/j.mib.2017.03.008
Keywords: roots, soil health, nematodes, ecological nutrient management, perennial agroecosystems
Citation: Martin T and Sprunger CD (2021) Belowground Dynamics Influence Nitrogen Cycling and Crop Productivity in Diversified Corn Systems. Front. Sustain. Food Syst. 5:705577. doi: 10.3389/fsufs.2021.705577
Received: 05 May 2021; Accepted: 15 October 2021;
Published: 11 November 2021.
Edited by:
Meagan Schipanski, Colorado State University, United StatesReviewed by:
Hanna J. Poffenbarger, University of Kentucky, United StatesCaitlin Peterson, University of California, Davis, United States
Copyright © 2021 Martin and Sprunger. This is an open-access article distributed under the terms of the Creative Commons Attribution License (CC BY). The use, distribution or reproduction in other forums is permitted, provided the original author(s) and the copyright owner(s) are credited and that the original publication in this journal is cited, in accordance with accepted academic practice. No use, distribution or reproduction is permitted which does not comply with these terms.
*Correspondence: Christine D. Sprunger, U3BydW5nZXIuMjlAb3N1LmVkdQ==