- 1Nanobioscience Group, Autonomous University of Coahuila, Saltillo, Mexico
- 2Group of Bioprocesses and Bioproducts, Food Research Department, Autonomous University of Coahuila, Saltillo, Mexico
- 3Instituto Cubano de Investigaciones de Los Derivados de la Caña de Azúcar (ICIDCA), Ciudad de la Habana, Cuba
- 4Center for Research and Advanced Studies of the National Polytechnic Institute (CINVESTAV-IPN), Unidad Saltillo, Ramos Arizpe, Mexico
- 5Chemistry and Biochemistry, National Technology of Mexico/Instituto Tecnológico de Durango, Durango, Mexico
The interaction between nanostructures and yeast cells, as well as the description of the effect of nanoparticles in ethanol production are open questions in the development of this nanobiotechnological process. The objective of the present study was to evaluate the ethanol production by Saccharomyces cerevisiae in the free and immobilized state on chitosan-coated manganese ferrite, using cane molasses as a carbon source. To obtain the chitosan-coated manganese ferrite, the one-step coprecipitation method was used. The nanoparticles were characterized by X-ray diffraction obtaining the typical diffraction pattern. The crystal size was calculated by the Scherrer equation as 15.2 nm. The kinetics of sugar consumption and ethanol production were evaluated by HPLC. With the immobilized system, it was possible to obtain an ethanol concentration of 56.15 g/L, as well as the total sugar consumption at 24 h of fermentation. Productivity and yield in this case were 2.3 ± 0.2 g/(L * h) and 0.28 ± 0.03, respectively. However, at the same time in the fermentation with free yeast, 39.1 g/L were obtained. The total consumption of fermentable sugar was observed only after 42 h, reaching an ethanol titer of 50.7 ± 3.1, productivity and yield of 1.4 ± 0.3 g/(L * h) and 0.25 ± 0.4, respectively. Therefore, a reduction in fermentation time, higher ethanol titer and productivity were demonstrated in the presence of nanoparticles. The application of manganese ferrite nanoparticles shows a beneficial effect on ethanol production. Research focused on the task of defining the mechanism of their action and evaluation of the reuse of biomass immobilized on manganese ferrite in the ethanol production process should be carried out in the future.
Introduction
The possibility of obtaining a renewable source of energy that is easy to access, safe and effective is one of the goals that humanity must achieve. Ethanol obtained by biotechnological methods is an important alternative to fossil fuels. The ethanol production from sugarcane shows the lowest production costs. Mainly, three types of raw materials are being used for this purpose, such as: sugar juice, starchy crops, and lignocellulosic materials (Mohd et al., 2017). The most common microorganisms used in fermentation from are the yeasts, especially, S. cerevisiae, though the bacterial species Zymomonas mobilis is also potentially used nowadays for this purpose (Arshad et al., 2018).
The biocatalysts immobilization has been the subject of research in recent decades, demonstrating that the immobilization of cells presents certain technical and economic advantages compared to a free-cell system (Elakkiya et al., 2016). In an attempt to improve ethanol fermentation, cell immobilization techniques have been developed to increase the rate of ethanol production, keep cells in a viable state, and achieve their normal function (Zapata and Peláez, 2010). Currently, in the immobilization of fungal spores and yeast cells, magnetic nanoparticles were used (Palacios et al., 2017). Nanoparticles have the ability to bind to the cell surface helping in the biomass separation and the biocatalysts reuse, since under the application of an external magnetic field, the particles are attracted and precipitated keeping the immobilized cells (Palacios et al., 2017).
Recently, many studies have focused on the synthesis of nanomaterials due to their excellent properties: a large surface-volume ratio, high specific surface area, easy separation under external magnetic fields and strong adsorption capacity (Vlazan et al., 2015). Manganese ferrite is an important member of ferrite family. Superparamagnetic iron oxide nanoparticles show a variety of applications in the modern era of science and engineering, for example, in medicine, mainly for magnetic hyperthermia (Mazarío et al., 2016), drug administration (Wahajuddin and Arora, 2012), as contrast agents in diagnosis by magnetic resonance imaging (MRI) (Mazarío et al., 2016), biosensors (Haun et al., 2010), ferrofluids (Moussaoui et al., 2016), they also can be used successfully in water at low temperature in thermochemical cycles (Prieto et al., 2007).
Various fabrication methods have been reported to prepare spinel manganese ferrite nanocrystals, including the sol-gel method (Banalata et al., 2014), coprecipitation (Irfán et al., 2012), synthesis in reverse micelles (Das et al., 2021) and the hydrothermal method (Mebdir and Sadiyha, 2019). Each method of ferrite obtaining has its advantages and disadvantages. The coprecipitation method offers many advantages over other methods, such as absence of contamination, more homogeneous mixing of the components and control of the particle size of the powders (Amighian et al., 2006), simplicity without the need for postcalcination. In the coprecipitation technique, several parameters, such as the relationship between ions, ionic strength, pH and precipitation temperature, can affect the structure and magnetic properties of ferrites (Goodarz et al., 2011). Coprecipitation from aqueous solutions is a relatively simple method and is therefore suitable for mass production. However, it provides limited control over stoichiometry, particle size, and size distribution (Amighian et al., 2006).
Although the methods for manganese ferrite obtaining are well-known, its functionalization with chitosan in one step was not previously described. However, Gregorio et al. (2012) and Osuna et al. (2012) described the method of magnetite nanoparticles synthesis coated with chitosan in one step coprecipitation, showing that the increase of chitosan concentration in the system does not lead to an increase in active amino groups. The optimal chitosan concentration was 0.125% (W/V).
The goals the present study were: (1) to obtain and characterize chitosan-coated manganese ferrite nanoparticles by one step coprecipitation method; (2) to evaluate the ethanol production from blackstrap molasses by free S. cerevisiae and yeast immobilized on chitosan-coated manganese ferrite nanoparticles.
Materials and Methods
Materials
The salts hydrated manganous sulfate (Jalmek), hexa-hydrated ferric chloride (Jalmek) and sodium hydroxide (Jalmek) were of analytical grade and were used as reagents. Chitosan with low molecular weight and 75% deacetylation degree from Aldrich was used.
Preparation of Chitosan-Coated MnFe2O4 Nanoparticles
A nano-sized manganese magnetic ferrite powder was prepared by the one-step coprecipitation method (Osuna et al., 2012) using hydrated manganese sulfate, ferric chloride hexahydrate, sodium hydroxide, and chitosan as starting materials. Ferric chloride hexahydrate was dissolved together with the required amount of hydrated manganese sulfate in a distilled water at 70°C. The ratio between the Fe and Mn cations was kept at 2: 1, respectively. Upon reaching this temperature, chitosan was added at 0.125% (w/v). The mixture was stirred until chitosan was solubilized. To 100 mL of the mixture, 20 mL of the sodium hydroxide solution (8 M) were added dropwise, the pH was set at 14. Unlike the methodology proposed by Osuna et al. (2012), the mixture was subjected to hydrothermal treatment in an autoclave for 5 h.
After the reaction, a dark brown precipitate was formed which was separated using the magnet. It was washed with water several times to remove impurities until the pH of the residual solution reached 7. This process took about 4 h. Next, the sample was dried in an oven keeping the temperature at 40°C for 3 h. The powder was treated in the mortar. Manganese ferrite was prepared in a similar manner without the addition of chitosan.
The chitosan-coated ferrite was kept at room temperature in a desiccator before being used in the fermentation.
Characterization of Manganese Ferrite Coated With Chitosan
X-ray Diffraction and Particle Size
X-ray powder patterns were recorded using a Siemens D-5000 diffractometer operating with Cu-Kα radiation (λ = 1.54056 Å) at 35 kV and 25 mA in the range of 2 to 80°C with a scanning speed of 0.02°C/s. The average crystallite size of the samples was determined from the total width to half the maximum using the Scherrer's formula:
where D is the average size of the crystallite, λ is the wavelength of the X rays, θ is the corresponding Bragg diffraction angle (in radians), and β is the total width in the maximum half (in radians) after the instrumental error correction. The X-ray diffraction patterns were also subjected to a structural analysis by the Rietveld method by means of the material analysis using diffraction (MAUD) program version 2.80.
Vibrating Sample Magnetometer
The magnetic properties of the synthesized chitosan-coated nanoparticles were measured in an alternating gradient magnetometer (AGM) Micromag 2900 manufactured by Princeton Measurements. The powders were measured in triplicate at room temperature. The magnetic field was applied from 12 to −12 kOe.
Fourier Transform Infrared Spectroscopy
Nanoparticles were characterized by Fourier transform infrared spectroscopy (FTIR). The FTIR spectra of both samples were analyzed through a Thermo Nicolet Nexus Fourier FT-IR spectrometer in the frequency range of 4,000–900cm−1 using a KBr disc method.
Scanning Electron Microscopy
Chitosan-coated magnetic nanoparticles (C-MNP), yeast cells, and their complexes with C-MNP were lyophilized and subjected to analysis on environmental scanning electron microscope (SEM) Brand Philips model XL-30 conditioned with a spectrometer Apollo Brand EDAX (USA). Each sample dispersed in ethanol was fixed on a polished bronze mirror holder and then sputtered with copper (99.97% purity). Sample drying was performed in a high vacuum evaporator JEOL model JEE400 at 0.00002 mbar, 18 mA for 15 s. Micrographs were taken under the following conditions: working distance at 39 mm, spot size at 4.5, and energy at 20 or 40 keV.
Strain Preservation and Inoculum Preparation
A yeast strain S. cerevisiae-150 donated by the Technological Institute of Durango (Durango, Mexico), was used. The strain was stored at 4°C in solid medium containing 20 g/L of glucose, 10 g/L of peptone, 10 g/L of yeast extract, and 15 g/L of agar.
To obtain the inoculum, the sample taken with a microbiological loop was transferred to 30 mL of synthetic medium (glucose 20 g/L, peptone 10 g/L, yeast extract 10 g/L). Fermentation was carried out for 18 h, at 150 rpm and 32°C until obtaining a concentration of 107 cells/mL.
Alcoholic Fermentation
In assay molasses from the Tamaulipas sugar industry was used. This molasses is characterized by pH 5.23 ± 0.01, 82.16 ± 1.16° Brix, ashes at 9.17 ± 0.09%, percentage of reducing sugars and nitrogen 66.34 ± 1.14% and 0.21 ± 0.06%, respectively. Anaerobic fermentation was carried out in 500 mL Erlenmeyer flasks using 300 mL of medium with cane molasses adjusted to 20° Brix using the solution with 1.0 g/L of KH2PO4, 1.59 g/L of (NH4)2SO4, and 0.5 g/L of MgSO4 × 7H2O. The medium was autoclaved in reactors prior to fermentation. The reactors were equipped with rubber caps, glass capillaries, and a CO2 trap. The inoculum was applied at 10% (v/v) achieving a concentration of 106 cells/mL. Nanoparticles (C-MNP) were added to the inoculum 30 min before their addition to the fermentation medium, achieving the final concentration at 460 mg/mL. The yeast immobilization was confirmed by quantification of cells in the supernatant, using a Neubauer chamber and an optical microscope (Olympus), after the application of the magnetic field to the reactor. Fermentation was carried out for 42 h, at 150 rpm and 32°C. Sampling was done every 6 h.
Sugars and Ethanol Quantification
Sugars (glucose and fructose) and ethanol were quantified by HPLC (Waters) containing a quaternary pump (Waters, model 600E), a refractive index detector (Waters, model 410), an automatic injector (Waters, model 717), aminex software HPX column 87-P (Bio-Rad, Cambridge, MA) and Empower 3 software. The 5 mM H2SO4 solution was used as the mobile phase. The injection volume was 20 μL, the column temperature was 60°C, the flow rate was 0.65 mL/min, and the run time was in the range of 30 to 40 min. To perform the quantification, an 8-point calibration curve was constructed for the glucose, fructose and ethanol standards (Sigma, St. Louis, MO), injected in triplicate. All reagents were HPLC grade. The chromatographic peaks of the samples were identified by comparison with the retention times of the standards. The total sugar concentration was calculated by summing the individual sugar concentrations.
Statistical Analysis
ANOVA tests with a significance level at p < 0.01 were performed using software Statistica 7.0 (Stat Soft, Tulsa, OK, USA). Kinetic data were analyzed by ANOVA test and plotted in Excel with corresponding standard deviations. Subsequently, the nanoparticles coated with chitosan were applied in the alcoholic fermentation of molasses.
Results and Discussion
Applying the one-step coprecipitation procedure proposed by Osuna et al. (2012) with modification by hydrothermal treatment in an autoclave, the dark brown materials susceptible to the effect of external magnetic field were obtained, which were characterized by different methods to verify that it is magnetic manganese ferrite nanoparticles with and without chitosan.
X-ray Diffraction and Particle Size
The XRD diffraction patterns of the manganese ferrite nanoparticles (Crystallography Open Database, Waerenborgh) are shown in Figure 1. The patterns show the reflection planes (111), (220), (311), (400), (511), (440) which confirm the presence of single-phase MnFe2O4 with a face-centered cubic structure in nanoparticles with and without chitosan at 2⊖ (Goodarz et al., 2011; Moussaoui et al., 2016).
The average particle size was determined from Scherrer's equation. Table 1 shows that the presence of chitosan during the synthesis of the nanoparticles favored the formation smaller particles in comparison with the ferrite synthesized in the absence of chitosan.
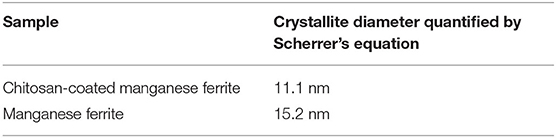
Table 1. Comparison of crystallite diameters quantified by Scherrer's equation for manganese ferrite obtained with and without chitosan.
Goodarz et al. (2011) synthesized manganese ferrite nanoparticles using a heat treatment method followed by calcination. The crystallite diameter of the nanoparticles obtained was calculated as 12 nm. This value is less than quantified for manganese ferrite obtained in the present study (15.2 nm) and greater than that detected for nanoparticles coated with chitosan (11 nm). According to different authors (Fontijn et al., 1999; Colombo et al., 2012; He et al., 2013), the size and properties of the nanoparticles strongly depend on the control of the operational parameters of the synthesis, such as the molar concentration of the solutions, the reaction temperature, the reaction rate, and the pH of the solution.
According to our knowledge, the method applied in the present study for the synthesis of chitosan-coated manganese ferrite nanoparticles was not previously reported. However, it was verified that the obtained material contains the nanoparticles of the compound of interest, as well as their crystallite size are comparable with the values reported in literature for other methods of their synthesis.
Magnetic Properties of Chitosan-Coated Manganese Ferrite
Figure 2 shows the hysteresis curves for chitosan-coated manganese ferrite. These curves were prepared using the averages of the magnetization values for the analyzed material and their replicas. A ferromagnetic behavior is seen, with a coercivity field at 185.52 ± 1.74 Oe, a saturation magnetization at 12.78 ± 0.43 emu/g, and a remanence at 5.6 emu/g. Saturation is a characteristic of magnetic materials. Magnetic saturation is the state reached in a sample when increases in the applied external magnetic field cannot further increase the magnetization of the material. At saturation, the total magnetic flux density does not increase with increases in applied external field. The field of coercivity refers to the intensity of the magnetic field necessary to bring the magnetization of the sample to zero once saturation is reached. The detected value of the coercivity field is lower than that reported in other publications (Goodarz et al., 2011, Irfán et al., 2012, Yang et al., 2010).
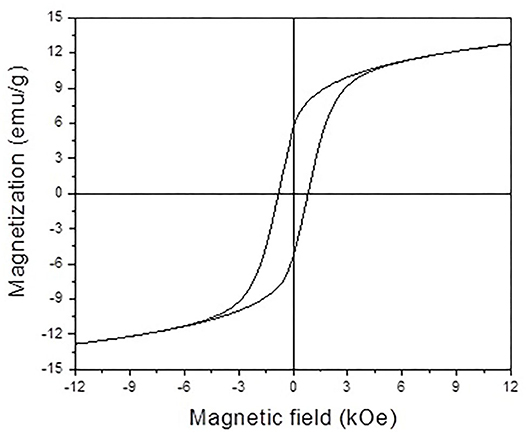
Figure 2. Magnetization curves determined at room temperature for chitosan-coated manganese ferrite nanoparticles.
The value of magnetic saturation is 15.78 emu/g reported by Goodarz et al. (2011), 16.3 emu/g reported by Irfán et al. (2012), 21.2 emu/g reported by Yang et al. (2010). It is worth mentioning that the values reported in the literature correspond to nanoparticles without chitosan. The presence of chitosan increases the percentage of non-magnetic matter in the system obtained. This is the cause of lower magnetic saturation value. Considering that the magnetization in magnetic nanoparticles depends directly on the crystallinity (Osuna et al., 2012), the higher magnetization values could be improved with an improvement in the crystallinity of the magnetic nanoparticles.
Then, the obtained results demonstrate the ferromagnetic behavior, which means that after removing the external magnetic field, the system maintains a certain amount of magnification. Furthermore, the magnetic properties of chitosan-coated manganese ferrite are similar to those reported in literature, although a different method was applied for its synthesis.
C-MNP Characterization by Fourier Transform Infrared Spectroscopy
FTIR analysis was performed to identify the presence of chitosan functional groups. In Figure 3, the comparison of spectra of chitosan and chitosan-coated nanoparticles is presented. The broad band around 3,365 cm−1 (A) is assigned to the stretching of the O-H group of the macromolecular association (Osuna et al., 2012). The band at 2,925 cm−1 (B) is assigned to the CH2- bond portion of the methylene groups, and the weak band at 2,856 cm−1 (C) is assigned to the -CH- bond of the methylene group that can be considered to be the characteristic peak of the chitosan structure. The characteristic bands at 1,709 cm−1 (D) and 1,633 cm−1 (E) can be assigned to the C = O groups of chitosan. The characteristic bands appearing at 1,570 cm−1 (F) correspond to the N-H bending vibration. The band at 1,321 cm−1 (G) is related to the stretching vibration C-N. Furthermore, the stretching vibration of C-O can also be found at 1,065 cm−1 (H) (Pavia et al., 1996). Thus, the FTIR results verify the presence of chitosan in the nanostructured material.
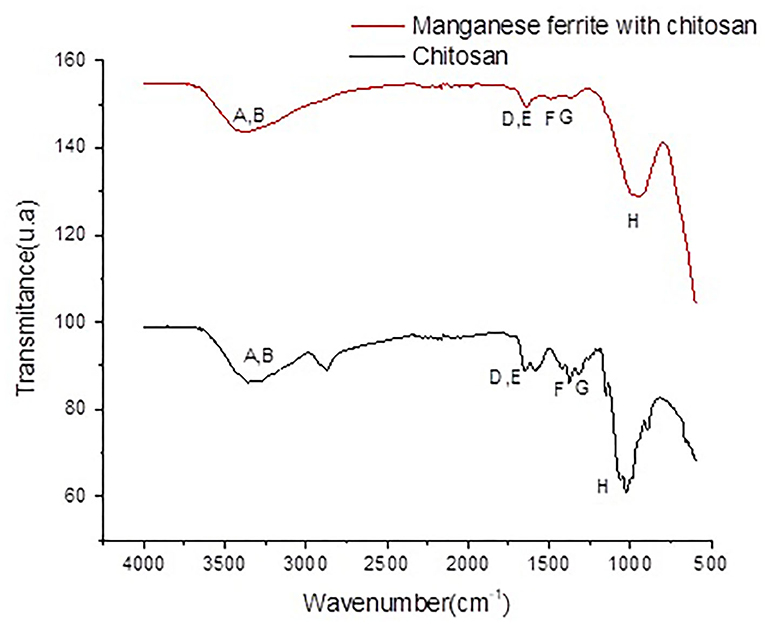
Figure 3. FT-IR spectra of chitosan and chitosan-coated magnetic nanoparticles in the region between 900 and 4,000 cm−1.
Scanning Electron Microscopy Characterization (SEM)
SEM micrographs of manganese ferrite (Figure 4A). Figure 4B shows S. cerevisiae-150 after 24 h of culture in cane molasses. The micrograph shows an approximate cell diameter of 2 to 4 μm that coincides with the literature data (López et al., 2016). Figure 4C shows the S. cerevisiae cells immobilized on the chitosan-coated manganese ferrite obtained after 24 h of culture in molasses.
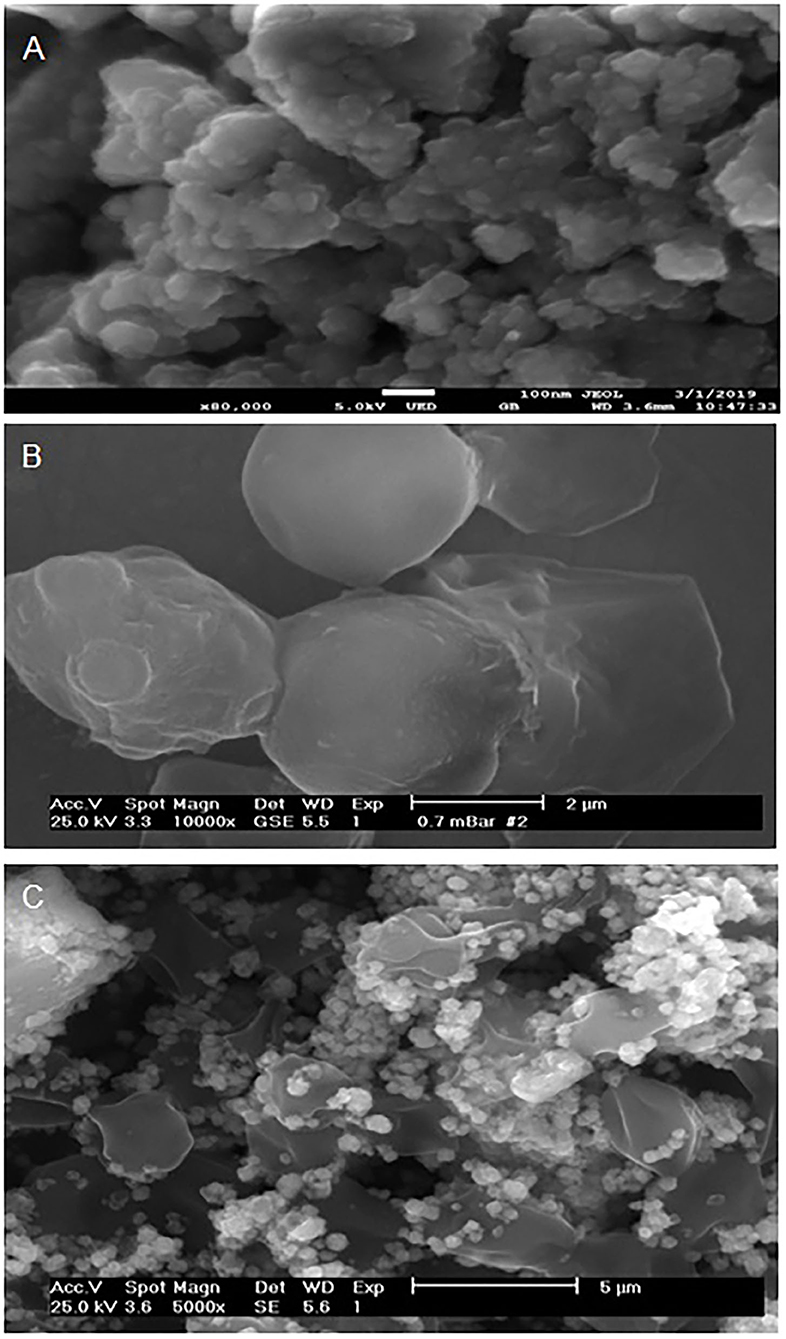
Figure 4. SEM micrographs of manganese ferrite (A), S. cerevisiae 150 (B), S. cerevisiae 150 immobilized on C-MNP (C).
The obtained micrographs are the evidence of the microorganism interaction with the nanoparticles. SEM analysis shows that the adsorption mechanism was not affected by the fermentation process. The micrographs are similar to those reported by Palacios et al. (2017), who immobilized Kluyveromyces marxianus in chitosan-coated magnetite. In both cases the nanoparticles are placed on the cell surface. However, unlike the SEM micrographs reported by Palacios et al. (2017) where the cells were completely covered with nanoparticles, in this study only a part of the cell surface interacted with the magnetic carrier, probably due to a lower C-MNP: cells ratio, due to increased cell concentrations during fermentation.
The nanostructured system can be described as an agglomerate of chitosan-coated nanoparticles, probably due to magnetic remanence (Figure 2). According to Figure 4C, this system covers the surface of the cells. Yeast cells with their almost spherical shape (Figure 4B) are partially covered with magnetic nanosystem (Figure 4C). Yeast interaction with C-MNP is evidenced by SEM micrographs.
Peng et al. (2010) reported SEM micrographs for Saccharomyces cerevisiae chemically immobilized on magnetite nanoparticles, which were functionalized with chitosan in the presence of glutaraldehyde in two-step methodology before interaction with yeast cells. Similarity is observed between the SEM micrographs presented in the present study and in the case of the report by Peng et al. (2010). The nanostructured system appears as agglomerates of many nanoparticles embedded in the chitosan matrix that were found around the cells. The surface of the adsorbent had many minute structures between spaces. The author attributed them to reactions that occur on the surface of the particle. It is worth mentioning that in the present study the immobilization was carried out without glutaraldehyde, which leads to define the interaction between support and yeast, as adsorption without chemical bond only due to weak bonds between the support and the microorganism.
Alcoholic Fermentation
A concentration of C-MNP (460 mg/mL) was applied to carry out the alcoholic fermentation of cane molasses by immobilized S. cerevisiae, giving the ration 2 × 103 cells/mg. This concentration was selected because the count of not immobilized cells in supernatant after 30 min of incubation and mixing with culture medium, using the Neubauer chamber, showed total adsorption of cells on magnetic support, after it removal by the external magnetic field.
In fermentation, the support without chitosan was not applied because it did not show interaction with the cells, monitoring by cell count. The incubation time was selected based on results reported by Palacios et al. (2017), who show the pseudo-steady state after this period of time.
Figure 5 shows kinetics of sugar consumption in the presence of free and immobilized yeast. In the presence of C-MNP, total sugar consumption was observed at 24 h, while in the presence of free yeast at 42 h. This result agrees with those observed in the kinetics of ethanol production (Figure 6). This result agrees with those observed in the ethanol production kinetics (Figure 6). With the immobilized system, it was possible to obtain an ethanol concentration of 56.15 g/L. Productivity and yield in this case were 2.3 ± 0.2 g/(L * h) and 0.28 ± 0.03, respectively. However, at the same time in the fermentation with free yeast 39.1 g/L of ethanol were obtained. After 42 h, an ethanol titer was 50.7 ± 3.1, productivity and yield of 1.4 ± 0.3 g/(L * h) and 0.25 ± 0.4, respectively. Therefore, a reduction in fermentation time, higher ethanol titer and productivity were demonstrated in the presence of nanoparticles. It is likely, that in the system with the agglomerated yeast cells coated with nanoparticles, an ethanol concentration gradient is achieved, preventing the inhibition of fermentation.
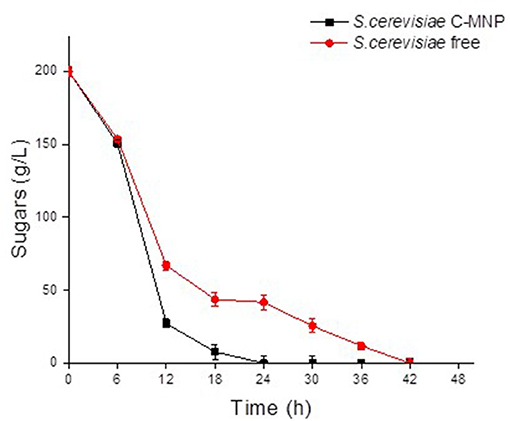
Figure 5. Kinetics of sugar consumption during cane molasses fermentation by free and immobilized on C-MNP S. cerevisiae-150 (molasses at 20° Brix, at 150 rpm and 32°C).
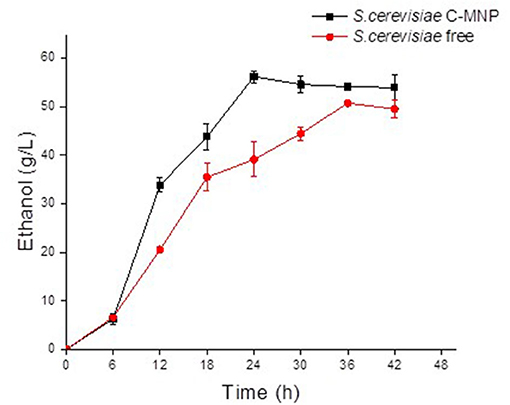
Figure 6. Kinetics of ethanol production during cane molasses fermentation by free and immobilized on C-MNP S. cerevisiae-150 (molasses at 20° Brix, at 150 rpm and 32°C).
Nuanpeng et al. (2018) studied the influence of immobilization of Saccharomyces by entrapment in 2% sodium alginate gel on ethanol production, observing that immobilization favored ethanol production. Zheng et al. (2012) using S. cerevisiae immobilized in an alginate-based MCM-41 mesoporous zeolite to ferment sugar molasses, obtained 78.6 g/L as the highest ethanol concentration. The method commonly used for yeast immobilization is gel trapping because the cells are protected from inhibition by alcohol, the yeast can be separated from the fermentation medium and reused in several cycles (Bai et al., 2008). In this case, the gel pore size must be sufficient so as not to limit the diffusion of enzymes responsible for the hydrolysis of oligo- and polysaccharides.
Rattanapan et al. (2011) used immobilized yeast cells on thin-layer silk cocoons for fermentations of blackstrap molasses by the adsorption method. The initial concentration of sugars was 240 g/L at the beginning of the fermentation process, 80.6 g/L of ethanol were obtained at 33°C after 48 h of fermentation. Vučurović and Razmovski (2012) used sugar beet pulp as a support for the S. cerevisiae immobilization in the bioethanol production from 120 g/L of sugars at the beginning; 52.3 g/L of alcohol was obtained after 48 h of fermentation. The immobilized cells demonstrate several advantages in ethanol production, such as high cell density, easy separation from the medium, high substrate conversion, lower inhibition, short reaction time, and cell recycling. The immobilized system shows faster substrate consumption compared to the free system.
In the present study, the highest ethanol concentration reached was 57 g/L when yeast immobilized in C-MNP was used. The observed differences can be related to the characteristics of the strain applied in the fermentation, with a higher concentration of fermentable sugars. The characteristics of the immobilized systems, for example, a greater protection of the strain against inhibitory effects, for example, ethanol, may be another factor that influences the production of ethanol.
It is worth mentioning that the optimization of the ethanol production process with S. cerevisiae immobilized in C-MNP can be optimized in future studies by selecting the support concentration, as well as the operational conditions of the process.
Conclusions
By applying the one-step coprecipitation procedure with the use of hydrothermal treatment in an autoclave, the dark brown chitosan-coated manganese ferrite nanoparticles were obtained. The nature of the material was verified by XRD, showing a particle size of around 15.2 nm. The magnetic properties were confirmed on a vibrating sample magnetometer. The presence of chitosan was verified by the FT-IR assay. The obtained the manganese ferrites nanoparticles were applied in the fermentation of cane molasses. Immobilization was confirmed by SEM micrographs and cell free counting. In the presence of immobilized yeast, a reduction in fermentation time, higher ethanol titer, and productivity were demonstrated when compared with free yeast. The application of manganese ferrite nanoparticles shows a beneficial effect on ethanol production. In the future, research should be carried out focused on the task of defining the mechanism of C-MNP action, optimization of their use, and evaluating the reuse of immobilized biomass on manganese ferrite in the ethanol production process.
Data Availability Statement
The raw data supporting the conclusions of this article will be made available by the authors, without undue reservation.
Author Contributions
AI, RR, CA, and JM-H: conceptualization and supervision. AN and RR: methodology. AN, AI, RR, and JM-H: software. AI and RR: validation and data curation. AI, RR, and JM-H: formal analysis. AN and JG: investigation. AI, RR, MA-G, NS-C, and JM-H: resources. AN: writing—original draft preparation. AI, RR, CA, AF, JS-C, and JM-H: writing-review and editing. JS-C: visualization. JM-H: project administration. AI and JM-H: funding acquisition.
Funding
Authors thank to National Council of Science and Technology (CONACyT-Mexico) for the financial support given to A.N.C. scholarship.
Conflict of Interest
The authors declare that the research was conducted in the absence of any commercial or financial relationships that could be construed as a potential conflict of interest.
References
Amighian, J., Mozaffari, M., and Nasr, B. (2006). Preparation of nano-sized manganese ferrite (MnFe2O4) via coprecipitation method. Phys. Status Solid. 3, 3188–3192. doi: 10.1002/pssc.200567054
Arshad, M., Hussain, T., Chaudhry, N., Sadia, H., Aslam, B., and Tahir, U. (2018). Enhancing profitability of ethanol fermentation through gamma ray mutagenesis of Saccharomyces cerevisiae. Pol. J. Environ. Stud. 28, 35–41. doi: 10.15244/pjoes/78708
Bai, W., Anderson, A., and Moo-Young, M. (2008). Ethanol fermentation technologies from sugar and starch feedstocks. Biotechnol. Adv. 26, 89–105. doi: 10.1016/j.biotechadv.2007.09.002
Banalata, K., Sanjana, D., Sujan, D., Tapas, K., Panchanan, P., and Dibakar, D. (2014). Biocompatible mesoporous silica-coated superparamagnetic manganese ferrite nanoparticles for targeted drug delivery and MR imaging applications. J. Colloid Interf. Sci. 431, 31–41. doi: 10.1016/j.jcis.2014.06.003
Colombo, M., Carregal, S., Casula, F., Gutiérrez, L., Morales, M., Bohm, I., et al. (2012). Biological applications of magnetic nanoparticles. Chem. Soc. Rev. 41, 4306–4334. doi: 10.1039/c2cs15337h
Das, A., Yadav, N., Manchala, S., Bungla, M., and Ganguli, A. (2021). Mechanistic investigations of growth of anisotropic nanostructures in reverse micelles. ACS Omega 6, 1007–1029. doi: 10.1021/acsomega.0c04033
Elakkiya, D., Prabhakaran, M., and Thirumarimurugan, T. (2016). Methods of cell immobilization and its applications. Int. J. Innovat. Res. Sci. Eng. Technol. 5, 5429–5433. doi: 10.15680/IJIRSET.2016.0504175
Fontijn, W., Van der Zaag, P., and Metselaar, R. (1999). A consistent interpretation of the magneto-optical spectra of spinel type ferrites. J. Appl. Phys. 85, 5100–5105. doi: 10.1063/1.369091
Goodarz, M., Saion, E., Bin, H., Hashim, M., and Shaari, H. (2011). Synthesis and characterization of manganese ferrite nanoparticles by thermal treatment method. J. Magnet. Magnet. Mater. 323, 1745–1749. doi: 10.1016/j.jmmm.2011.01.016
Gregorio, M., Pineda, G., Rivera, E., Hurtado, G., Saade, H., Martínez, J. L., et al. (2012). One step method for preparation of magnetic nanoparticles coated with chitosan. J. Nanomater. 2012, 1–8. doi: 10.1155/2012/813958
Haun, J., Yoon, J., Lee, H., and Weissleder, R. (2010). Magnetic nanoparticle biosensors. Nanomed. Nanobiotechnol. 2, 291–304. doi: 10.1002/wnan.84
He, X., Zhong, W., and Au, C. T. (2013). Size dependence of the magnetic properties of Ni nanoparticles prepared by thermal decomposition method. Nanoscale Res. Lett. 8:446. doi: 10.1186/1556-276X-8-446
Irfán, E., Rabab, Z., Kiran, M., Arifa, J., and Nasir, A. (2012). Co-precipitation synthesis, physical and magnetic properties of manganese ferrite powder. Afr. J. Pure Appl. Chem. 6, 1–5. doi: 10.5897/AJPAC11.054
López, C., Figueroa, V., Lugo, M., Pereira, C., Garay, H., Barbosa, J., et al. (2016). The intrinsic antimicrobial activity of citric acid-coated manganese ferrite nanoparticles is enhanced after conjugation with the antifungal peptide Cm-p5. Int. J. Nanomed. 11:3849. doi: 10.2147/IJN.S107561
Mazarío, E., Mayoral, A., Salas, E., Menéndez, N., Herrasti, P., and Sánchez, J. (2016). Synthesis and characterization of manganese ferrite nanoparticles obtained by electrochemical/chemical method. Mater. Design 111, 646–650. doi: 10.1016/j.matdes.2016.09.031
Mebdir, A., and Sadiyha, Y. (2019). Zinc oxide nanoparticles and nanorods as antimicrobial agents: particle size influence. Nano Biomed. Eng. 11, 375–380. doi: 10.5101/nbe.v11i4.p375-380
Mohd, H., Abdulla, R., Jambo, S., Marbawi, H., Gansau, J., Mohd, A., et al. (2017). Yeasts in sustainable bioethanol production: a review. Biochem. Biophys. Rep. 10, 53–61. doi: 10.1016/j.bbrep.2017.03.003
Moussaoui, H., Mahfoud, T., Habouti, S., ElMaalam, K., BenAli, M., Hamedoun, M., et al. (2016). Synthesis and magnetic properties of tin spinel ferrites doped manganese. J. Magnet. Magnet. Mater. 405, 181–186. doi: 10.1016/j.jmmm.2015.12.059
Nuanpeng, S., Thanonkeo, S., Klanritac, P., and Thanonkeo, P. (2018). Ethanol production from sweet sorghum by Saccharomyces cerevisiae DBKKUY-53 immobilized on alginate-loofah matrices. Braz. J. Microbiol. 49(Suppl 1), 140–150. doi: 10.1016/j.bjm.2017.12.011
Osuna, Y., Gregorio, K., Gaona, G., de la Garza, M., Ilyna, A., Díaz, E., et al. (2012). Chitosan-coated magnetic nanoparticles with low chitosan content prepared in one-step. J. Nanomater. 2012, 1–7. doi: 10.1155/2012/327562
Palacios, S., Ramos, R., Ruiz, H., Aguilar, M., Martínez, J., Segura, P., et al. (2017). Trichoderma sp. spores and Kluyveromyces marxianus cells magnetic separation: immobilization on chitosan-coated magnetic nanoparticles. Preparat. Biochem. Biotechnol. 47, 554–561. doi: 10.1080/10826068.2016.1275007
Pavia, D., Lampman, G., and Kriz, G. (1996). Introduction to Spectroscopy, 2nd Edn. New York, NY: Saunders Golden Sunburst Series.
Peng, Q., Liu, Y., Zeng, G., Xu, W., Yang, C., and Zhang, J. (2010). Biosorption of copper (II) by Immobilizing Saccharomyces cerevisiae on the surface of chitosan-coated magnetic nanoparticles from aqueous solution. J. Hazard. Mater. 177, 676–682. doi: 10.1016/j.jhazmat.2009.12.084
Prieto, F., Sánchez, F., Méndez, M., García, G., and Gordillo, A. (2007). Obtención y caracterización de ferritas ternarias de manganeso por mecanosíntesis. Bol. Soc. Geol. Mex. 59, 125–132. doi: 10.18268/bsgm2007v59n1a10
Rattanapan, A., Limtong, S., and Phisalaphong, M. (2011). Ethanol production by repeated batch and continuous fermentations of blackstrap molasses using immobilized yeast cells on thin-shell silk cocoons. Appl. Energy 88, 4400–4404. doi: 10.1016/j.apenergy.2011.05.020
Vlazan, P., Miron, I., and Sfirloaga, P. (2015). Cobalt ferrite substituted with Mn: synthesis method, characterization and magnetic properties. Ceram. Int. 41, 3760–3765. doi: 10.1016/j.ceramint.2014.11.051
Vučurović, V., and Razmovski, N. (2012). Sugar beet pulp as support for Saccharomyces cerevisiae immobilization in bioethanol production. Indust. Crops Prod. 39, 128–134. doi: 10.1016/j.indcrop.2012.02.002
Wahajuddin, S., and Arora, S. (2012). Superparamagnetic iron oxide nanoparticles: magnetic nanoplatforms as drug carriers. Int. J. Nanomedicine 7, 3445–3471. doi: 10.2147/IJN.S30320
Yang, H., Zhang, C., Shi, X., Hu, H., Du, X., Fang, Y., et al. (2010). Water-soluble superparamagnetic manganese ferrite nanoparticles for magnetic resonance imaging. Biomaterials 31, 3667–3673. doi: 10.1016/j.biomaterials.2010.01.055
Zapata, A., and Peláez, C. (2010). Producción en continuo de etanol a partir de banano de rechazo (cáscara y pulpa) empleando células inmovilizadas. Tumbaga 5, 49–60.
Keywords: ethanol production, manganese ferrite, Saccharomyces, sugarcane molasses, immobilization
Citation: Núñez Caraballo A, Iliná A, Ramos González R, Aguilar CN, Michelena Álvarez G, Flores Gallegos AC, Sandoval-Cortés J, Aguilar-Gonzalez MA, Soto-Cruz NO, García García JD and Martínez-Hernández JL (2021) Sustainable Ethanol Production From Sugarcane Molasses by Saccharomyces cerevisiae Immobilized on Chitosan-Coated Manganese Ferrite. Front. Sustain. Food Syst. 5:683170. doi: 10.3389/fsufs.2021.683170
Received: 20 March 2021; Accepted: 30 April 2021;
Published: 07 June 2021.
Edited by:
Guillermo Raul Castro, Consejo Nacional de Investigaciones Científicas y Técnicas (CONICET), ArgentinaReviewed by:
Shi'An Wang, Qingdao Institute of Bioenergy and Bioprocess Technology (CAS), ChinaAchyut Adhikari, Louisiana State University, United States
Copyright © 2021 Núñez Caraballo, Iliná, Ramos González, Aguilar, Michelena Álvarez, Flores Gallegos, Sandoval-Cortés, Aguilar-Gonzalez, Soto-Cruz, García García and Martínez-Hernández. This is an open-access article distributed under the terms of the Creative Commons Attribution License (CC BY). The use, distribution or reproduction in other forums is permitted, provided the original author(s) and the copyright owner(s) are credited and that the original publication in this journal is cited, in accordance with accepted academic practice. No use, distribution or reproduction is permitted which does not comply with these terms.
*Correspondence: José L. Martínez-Hernández, am9zZS1tYXJ0aW5leiYjeDAwMDQwO3VhZGVjLmVkdS5teA==