- 1Department of Food Science and Technology, University of California-Davis, Davis, CA, United States
- 2Department of Health Sciences and Technology, ETH Zurich, Zurich, Switzerland
- 3Department of Biological and Agricultural Engineering, University of California-Davis, Davis, CA, United States
- 4Department of Bioengineering, University of Massachusetts Dartmouth, Dartmouth, MA, United States
Insect farming has the potential to transform abundant residual biomass into feed that is compatible with non-ruminant animal production systems. However, insect cultivation generates its own by-products. There is a need to find valuable and sustainable applications for this material to enable commercial-scale insect farming. Soil application of by-products, which may be either basic broadcasting incorporation or part of a sustainable soil borne pest management practice, such as biosolarization, could offer an agricultural outlet. The objective of this study was to assess the potential of applying black soldier fly larvae (BSFL)-digested substrate as soil amendment for soil biosolarization and evaluate its impact on soil health. Sandy loam (SL) and sandy clay loam (CL) soils amended with BSFL-digested almond processing residues, i.e., spent pollinator hulls (SPH), at 2% dry weight (dw) were incubated under aerobic and anaerobic conditions for 15 days under a daily fluctuating temperature-interval (30–50°C). The microbial respiration, pH, electrical conductivity, volatile fatty acids, macronutrients, and germination index using radish seeds (Raphanus sativus L.) were quantified to assess the soil health after amendment application. Incubation showed a statistically significant (p < 0.05) increase in electrical conductivity related to amendment addition and a decrease potentially linked to microbiological activity, i.e., sequestering of ions. Under aerobic conditions, SPH addition increased the CO2-accumulation by a factor of 5–6 compared to the non-amended soils in SL and CL, respectively. This increase further suggests a higher microbiological activity and that SPH behaves like a partially stabilized organic material. Under anaerobic conditions, CO2-development remained unchanged. BSFL-digested residues significantly increased the carbon, nitrogen, C/N, phosphate, ammonium, and potassium in the two soil types, replenishing soils with essential macronutrients. However, greenhouse trials with lettuce seeds (Lactuca sativa) lasting 14 days resulted in a decrease of the biomass by 44.6 ± 35.4 and 35.2 ± 25.3% for SL and CL, respectively, compared to their respective non-amended soil samples. This reduction of the biomass resulted from residual phytotoxic compounds, indicating that BSFL-digested SPH have the potential to be used for biosolarization and as soil amendments, depending on the concentration and mitigation strategies. Application and environmental conditions must be carefully selected to minimize the persistence of soil phytotoxicity.
Introduction
In one decade, the production of almond kernels in the U.S. has increased from 640,000 t to 1.2 million t (USDA, 2020)1. This growth may partially be attributed to more health (Willett et al., 1995) and environmental (Pimentel and Pimentel, 2003) awareness in consumers, making them transition from animal to plant-based food and cosmetic products. Furthermore, targeted global marketing programs have effectively been implemented, resulting in a higher demand for almonds (ABC, 2019)2. Along with the increased production of almond kernels, the amount of hulls and shells, which by weight make up roughly 50 and 25%, respectively, of the almond fruit (Yousef et al., 2017), has surged as well. In the crop year 2018/2019 roughly 2.8 million t (ABC, 2019) of hulls and shells were generated. While hulls are mainly used for feedstock, shells are used for animal bedding due to the high lignin and cellulose content, making them unsuitable for animal feed (Velasco and Schoner, 1965). In contrast to the rising appetite for almonds, livestock production has been stable during the past 10 years, lowering the demand and thus the price for almond biomass side-streams (CDFA, 2019)3. Consequently, alternative uses for hulls and shells have been investigated during the last years. Many promising applications include insect farming (Palma et al., 2018), orchard soil amending before and after torrefaction (Pedrefio et al., 1996; Chiou et al., 2016), ethanol production (Offeman et al., 2014), and as plastic additives (Essabir et al., 2013).
Insect farming, using low-value organic residues, has the great advantage of generating a high-quality protein source that could be used for fish and poultry feeding or as a substitute for other commercially available protein sources (Leiber et al., 2015; Al-qazzaz et al., 2016; Gold et al., 2018). This additional and sustainable protein source could help address the major challenge of filling the predicted gap between available nourishment and effective population size by 2050 (Godfray et al., 2010; Smetana et al., 2016). Many different studies revealed that black soldier fly (Hermetia illucens) larvae (BSFL) grow on various waste and side streams, such as manure (Sheppard et al., 1995), food waste (Nguyen et al., 2015), or municipal organic waste (Diener and Studt, 2011). Palma et al. showed that BSF larvae could be successfully cultivated on almond hulls, whereby aeration and moisture content had significant effects on larvae growth (Palma et al., 2018). The usage of pollinator hulls showed the greatest increase in specific larvae growth compared to non-pareil hulls, monterey hulls, or mixed shells (Palma et al., 2019, 2020). Insect cultivation however leads to side-streams of leftover and non-consumed hulls, termed spent hulls, which are possibly not stable enough for direct soil applications, due to the short BSF larvae cultivation process (<20 days) relative to other organic waste stabilization systems. It has been reported that the direct soil application of amendments may have a negative impact on crop growth in the initial months or even years due to residual phytotoxicity from the amendments or limited nitrogen availability (Stamatiadis et al., 1999; Griffin and Hutchinson, 2007; Thomsen et al., 2018). In order to stabilize the organic matter in a short time-frame, achieve zero-waste goals2 and close life cycles in a sustainable way, spent hull residues must be carefully managed to minimize negative impacts on the environment.
Soil biosolarization (SBS) is a chemical-free alternative to soil fumigation that relies on solar heating and decomposition of organic material amended into soil to achieve pathogen and weed seed inactivation (Blok et al., 2000; Ros et al., 2008). Soil amendments help overcome the limitations that arise from only solarization methods, such as long treatment times, survival of pathogens in deeper soil layers, and weather dependency regarding heating, by creating a reductive environment and producing biocidals through microbial activity under low oxygen conditions (Blok et al., 2000; Ros et al., 2008; Patel et al., 2014). This biocidal activity can be traced back to the degradation of organic material and the formation of ammonium, nitrate, nitrite, organic acids, hydrogen sulfide, aldehydes, and ketones (Angus et al., 1994; Sarwar et al., 1998; Domínguez et al., 2014; Shea et al., 2021). Several studies positively correlated the potential efficacy of SBS with the stability degree of the amendments originating from wheat bran, green waste compost, or tomato processing residues (Simmons et al., 2013; Achmon et al., 2017; Fernández-Bayo et al., 2017, 2018). In addition to a decreased mortality in crops, such as strawberry (Domínguez et al., 2014), SBS was shown to positively impact crop yields and soil health. Particularly, SBS with manure, olive pomace, or beet vinasse as organic amendment showed a significant increase of strawberry and tomato yields (Domínguez et al., 2014; Díaz-Hernández et al., 2017). Furthermore, SBS with energy crops and digestates increased phosphate, potassium, and inorganic nitrogen amounts (Pognani et al., 2009; Fernández-Bayo et al., 2017).
The main objective was to assess the potential of using spent hulls from BSFL cultivation as an amendment for SBS in two different soil types and to investigate its implications on soil health. Its feasibility was characterized by monitoring metrics related to SBS efficacy (pH, volatile fatty acids, and microbial respiration) (Achmon et al., 2017), and parameters related to soil quality (conductivity, phytotoxicity, macronutrients, and crop yield). Experiments were conducted in the lab with fluctuating temperature under anaerobic (mimicking SBS) and aerobic (simulating broadcast soil application) conditions. To the best of our knowledge, there is only one study investigating the potential effects of soil application of spent hulls from BSFL (Palma et al., 2020).
Materials and Methods
Spent Hulls and Soil Samples
Spent pollinator hulls were made available downstream of BSFL cultivation (Palma et al., 2018), originating from the VanderGheynst Lab, Department of Biological and Agricultural Engineering, UC Davis, CA, USA. As is described in Palma et al. (2018), pollinator hulls were obtained from a processor in 2017 from Chico, CA, USA from the prior years' harvest (2016). The hulls were reported to have a pH 4.74, 6 g kg−1 total nitrogen and 434 g kg−1 total carbon and particle size (d = 6.35 mm). The larvae cultivation conditions are described in Palma et al. (2018). Briefly, the initial C/N was set to 25 by adding urea. The hulls were inoculated with 1.3 larvae g−1 hulls BSFL (wet basis). Prior to inoculation onto hulls, larvae were reared for 5–10 days on chicken feed (Purina Premium Poultry Feed Layena Crumbles, Purina Animal Nutrition LLC, Shoreview, MN, USA). During the larvae cultivation studies on hulls, the aeration rate, temperature and moisture content during growing conditions were 160 mL min−1, 26–28°C, and 82% of fiber saturation point (wet basis), respectively. The feeding rate was 300 g wet weight (ww) on day 0 and 610 g ww added after 5 days of incubation. After 13 days of incubation, larvae were recovered from substrate and spent pollinator hulls (SPH) were stored at 25°C. Prior to the current study, the SPH were oven-dried for 24 h at 55°C.
Almond trees are most productive in loam-textured soils due to their permeability, water retention, and root zone aeration (Micke, 1996). Soil texture is known to affect soil aeration, which may impact anaerobic conditions during biosolarization and the capacity of the soil to remediate phytotoxicity after treatment. Therefore, two relevant soil types for almond production with different textures were selected, a sandy loam (SL) and a sandy clay loam (CL). SL (41, 37, and 22% sand, silt and clay, respectively) soil was collected from the 0–15 cm depth range at the University of California Kearney Agricultural Research and Extension Center (KARE) in Parlier, CA, USA. Similarly, CL (47, 27, and 26% sand, silt and clay, respectively) soil was obtained from the 0–15 cm depth range at the UC Davis Plant Pathology Research Fields. Both soil types were air-dried, sieved through a 2 mm screen, and stored at room temperature.
Biosolarization Simulation Assay on a Lab-Scale
Four different treatments were prepared: Non-amended sandy loam (SPH: 0% dw, SL), sandy loam mixed with spent pollinator hulls (SPH: 2% dw, SL + SPH), non-amended sandy clay loam (SPH: 0% dw, CL), and sandy clay loam mixed with spent pollinator hulls (SPH: 2% dw, CL + SPH). The selected amendment rate of 2% dw is equivalent to 33 t ha−1 (assuming the bulk density of SL and CL is around 1.65 g cm−3 and that the amendment is incorporated down to 10 cm), which is within the recommended application rates for compost (Donn et al., 2014). Prior to the experiments, distilled water was added to each sample to reach 80% of the field capacity (FC) and they were left to equilibrate overnight at 4°C.
For each treatment (SL, SL + SPH, CL, CL + SPH), six replicate 250 mL flasks were filled with 100 g of sample. The flasks were placed in an incubator with a fluctuating temperature range from 30 to 50°C in 12 h intervals (Thermo Fischer Scientific, Waltham). Tubes from each reactor were connected to a Micro-Oxymax Respirometer (Columbus instruments, OH, USA). For each treatment, three flasks experienced anaerobic conditions by closing the flask with a sealed lid connected to a gas sampling tube with a check valve to prevent air going in. Three samples were placed in an aerobic environment by having a lid connected to the gas sampling tube and an air supplying tube at an aeration rate of 20 mL min−1 (Achmon et al., 2016). Samples were incubated for 15 days where the CO2 that evolved was measured as an indicator for microbial respiration. In parallel, to monitor pH, electrical conductivity (EC, 25°C), volatile fatty acids (VFAs), and phytotoxicity changes during anaerobic experiments, 10 g of each sample (n = 3) was added to polypropylene tubes (15 mL, Fisherbrand Pittsburgh, PA, USA). These tubes were closed to promote anaerobic conditions and incubated in the same incubators for different incubation times. After 0, 1, 5, 10, and 15 days (t0, t1, t5, t10, t15), three replicates of each treatment were taken for analysis.
Soil Analysis of Incubated Samples
At each incubation point, samples incubated in 15 mL tubes were diluted with distilled water at a dilution ratio 1:1 (mass of sample dw/mass water) and shaken for 30 min. For each extract, the pH, EC, phytotoxicity and volatile fatty acid (VFA) content were monitored using the method described in the following. The pH (SevenCompact S220, Mettler Toledo, OH, USA) and the conductivity (SevenCompact S230, Mettler Toledo, OH, USA) were measured following vendor guidelines. Then, the samples were centrifuged for 20 min at 4,000 g. An aliquot of the supernatant was filtered through a 0.2 μm filter (Titan3, 17 mm, PTFE membrane, Thermo Fisher Scientific, MA, USA) to measure the volatile fatty acid (VFA) content, i.e., lactic acid, formic acid, acetic acid, propionic acid, isobutyric acid, butyric acid, using HPLC-UFLC-10Ai (Shimadzu, Columbia, MD, USA) equipped with an Aminex® HPX-87H (300 × 7.8 mm) column (Life Science Research, Education, Process Separations, Food Science, Hercules, CA, USA) and a SPD-M20A diode array detector set at 210 nm. The HPLC conditions are described elsewhere (Simmons et al., 2016).
To test the phytotoxicity on radish seeds (Raphanus sativus L., Ferry-Morse, KY, USA), a filter paper (Dia.: 9.0 cm, P5, Cat. No.: 09-801B, Thermo Fisher Scientific, MA, USA) was sterilized with UV light on both sides for 20 min each (Biosafety cabinet, 1300 Series A2, Thermo Fisher Scientific, MA, USA) and placed in a petri dish (Falcon, NY, USA), on which 10 seeds were placed and soaked with 5 mL of the previously mentioned supernatant. After 3 days of incubation in the dark at 25°C, the root length and number of germinated seeds were determined. As a control, 10 seeds (n = 3) were soaked with 5 mL of distilled water. The phytotoxicity was quantified by the germination index Gi (Equation 1) (Tiquia et al., 1996; Ko et al., 2008; Mitelut et al., 2011). Gi is defined as the product of the germination ratio and the length ratio:
where G represents the number of seed germinated per plate, L the root lengths (mm) of germinated seed, G0 the number of germinations of the control, and L0 (mm) the root lengths of the control. Only seeds with root lengths >0.5 mm were considered germinated.
Analysis of Macronutrients in Soils
Samples at time t0 and time t15 under anaerobic and aerobic conditions were oven-dried for 48 h at 55°C and sent to the analytical lab at the University of California Davis (Davis, CA, USA) to measure total carbon and total nitrogen (combustion method, AOAC Official Method 972.43, 1997), NO3-N and ammonium-nitrogen content (flow injection analyzer method, Rhoades, 1982), extractable phosphorus (Olsen method, Olsen and Sommers, 1982), and exchangeable potassium (cation exchange capacity, ICP-AES, Thomas, 1982).
Lettuce Growth in Greenhouse
To measure potential fertility or residual phytotoxicity of the spent hulls, the growth of lettuce (Lactuca sativa) was monitored in a greenhouse at 22°C and 80% relative humidity. Prior to transplanting the lettuce, seeds were germinated in petri dishes during 3 days. Germinated seeds of similar sizes were placed into pots (180 cm3) filled with sandy loam, SL, or sandy clay loam, CL, with fresh (2% dw) or without spent pollinator hull (SPH) amendments (n = 10). Coir-Lite Mix (0.75 yd3 Coir, 0.25 yd3 Perlite, UC Davis) was used as the control soil. After 14 days in the greenhouse, the seedlings were harvested, oven-dried (24 h, 55°C), and weighed to measure the lettuce biomass.
Data Analysis
For the statistical analysis IBM SPSS Statistics® (Version 23.0. IBM Corp., Armonk NY, USA) was used. A multiway ANOVA, followed by a one-way ANOVA with a Tukey's HSD post-hoc test or a paired-sample T-test was conducted with a confidence level of 95%. To correlate the pH, conductivity, and volatile fatty acids with the phytotoxicity and to correlate macronutrients with the lettuce biomass, Pearson's r was calculated in a two-tailed bivariate correlation test. Acetic acid concentrations were fitted with a 1st order decay model using the damped least-squares method (OriginPro 2019, OriginLab Corporation, Northampton, MA, USA) (Equation 2):
where C represents the initial acetic acid concentration, k the decay constant, t the time constant, y0 the offset.
Results and Discussion
Assessment of the Potential of Spent Hulls for Soil Biosolarization
Microbial Respiration of Amended Samples
CO2, CH4, and H2 were monitored, however, no quantifiable amounts of CH4 and H2 were measured, confirming that these gases were not produced or below the detection limit of the measurement system. To determine the degree of stability of the spent pollinator hulls (SPH) in the soils, the microbial activity was assessed by monitoring the CO2 evolution during incubation under anaerobic (AN) and aerobic (AE) conditions. After 15 days under AE conditions, SPH addition increased the CO2 accumulation significantly (Supplementary Table 1, p < 0.05) by a factor of 5 and 6 compared to the non-amended soils in SL and CL, respectively. Compared to AN conditions, AE non-amended soil samples (SL, AE and CL, AE) released 26 and nine times more CO2, respectively. When spent hulls were present, 150 and 68 times more CO2 was released for SL + SPH and CL + SPH, respectively. In the AN environment, added hulls and soil type were irrelevant (Figure 1). When comparing these respiration rates to other samples with slightly higher amendment rates of non-treated organic wastes, such as white wine grape pomace (5%), red wine grape pomace (5%), or tomato pomace (5%), a lower amount of CO2 was released by SPH (Achmon et al., 2016). Compared to respiration rates of partially composted amended soils at 2.5% similar amounts of CO2 were observed (i.e., around 200 mL/100 g dw), suggesting that digested SPH were only partially stabilized by BSFL digestion (Fernandez-Bayo et al., 2018). It has been shown that Actinomyces spp., Dysgonomonas spp., and Enterococcus spp. play an important role in the degradation process of organic matter in the BSFL gut, resulting in a reduced respiration rate in the soil compared with non-treated organic waste (Klammsteiner et al., 2020).
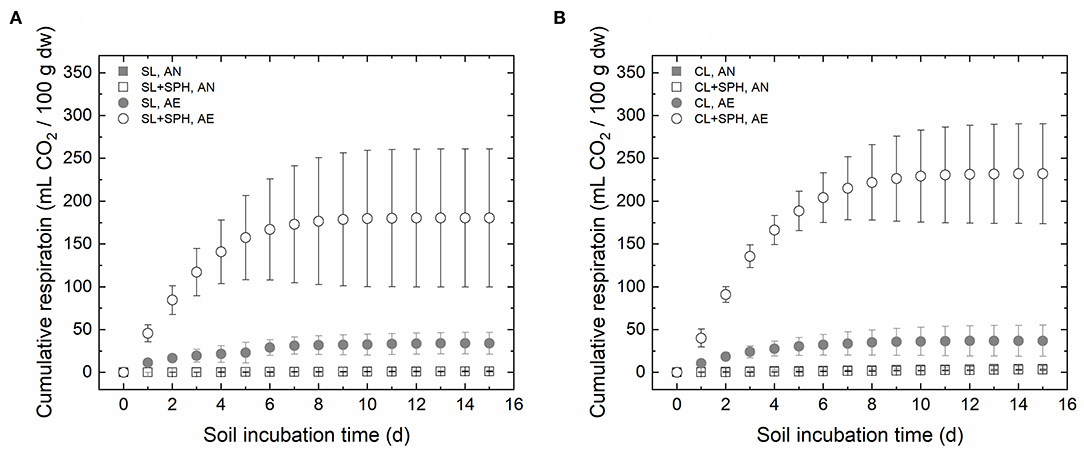
Figure 1. CO2 accumulation during 15 days in (A) sandy loam (SL) and (B) sandy clay loam (CL), with (2% dw) and without spent pollinator hulls (SPH) under anaerobic and aerobic conditions. AN, anaerobic; AE, aerobic. Bars represent standard deviations (n = 3).
Analyses of pH, Electrical Conductivity, and VFAs Related to Biosolarization
After 15 days of incubation under AN conditions, the pH of SL and SL + SPH was at 7.29 ± 0.08 and 7.40 ± 0.14, respectively (Figure 2A). In the case of CL and CL + SPH, the pH reached values of 7.56 ± 0.12 and 8.02 ± 0.03, respectively (Figure 2B). Soil pH for all anaerobic samples was significantly affected by the soil type, the presence of spent hulls, time, interactions of soil and spent hulls, soil and time, spent hulls and time (Supplementary Table 2, p < 0.05). When comparing initial (t0) and final (t15) conditions of the same soil sample, only CL + SPH had a significantly higher pH than after incubation (p < 0.05). This suggests that the type of soil affected the evolution of the pH when spent hulls were incorporated. Moreover, by adjusting the water content to 80% of the field capacity, CL resulted in a higher volumetric soil moisture content. The pH of the soil tends to increase more as the ratio of water to soil increases (Thomas, 1996). Various mechanisms, such as decrease in exchangeable and soluble aluminum, consumption of protons by carboxylic, phenolic, and enolic groups, and liming effect of residues, have been proposed to explain an increasing pH after amendment addition (Wong et al., 1998; Haynes and Mokolobate, 2001; Naramabuye and Haynes, 2006). It appears that in CL, a sufficient amount of moisture was present, and enough time was given for soil chemical reactions to take place, thus resulting in a significantly higher pH compared with the initial state of CL + SPH.
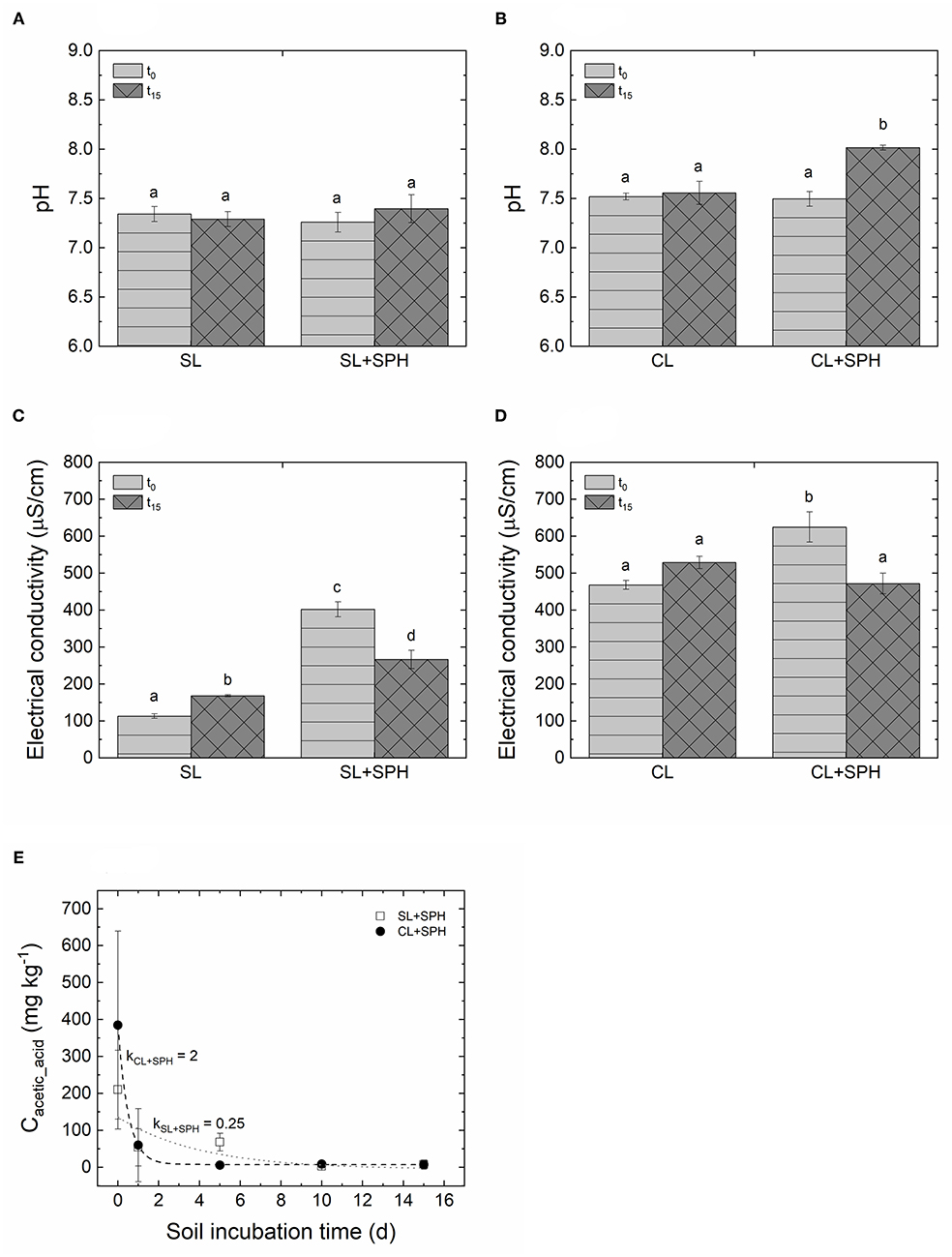
Figure 2. (A) pH for sandy loam (SL), (B) pH for sandy clay loam (CL), (C) electrical conductivity (25°C) for SL, (D) electrical conductivity (25°C) for CL with (2% dw) and without amended spent pollinator hulls (SPH) under anaerobic conditions for initial (0 days, t0) and final time (15 days, t15). Different letters indicate significant differences (p < 0.05) in a one-way ANOVA followed by a Tukey's HSD post-hoc test. (E) Acetic acid concentration after anaerobic incubation measured for the time-points t0, t1, t5, t10, and t15 and fitted with a 1st order decay model (dotted line: SL + SPH; dashed line: CL + SPH). Parameter k represents the decay constant. Bars represent standard deviations (n = 3).
The electrical conductivity (EC) at 25°C in AN samples was significantly affected by the soil type, the presence of spent hulls, time, interactions of soil and spent hulls, spent hulls and time (Supplementary Table 3, p < 0.05). Significant differences of pH and EC between soil types are shown in Supplementary Figure 1. A one-way ANOVA for SL (Figure 2C) and CL (Figure 2D) followed by a Tukey's HSD post-hoc test showed that the EC increased (p < 0.05) in non-amended soil samples of SL, however, decreased after 15 days (p < 0.05) when spent hulls were added. Before incubation, both SL and CL showed significantly (p < 0.05) higher EC when spent hulls were added. After 15 days of incubation, SL + SPH still had a significantly higher EC than the non-amended soil (p < 0.05), while in CL + SPH it decreased back to the original EC as was found in CL. Similarly, to the significantly increased pH in CL + SPH, the higher soil moisture content resulted in a larger soil activity. Overall, the amendments seemed to introduce salts in form of nutrients to the soil, however, they thereby increased microbial activity and decreased the EC over time. This suggests that the ions were sequestered in the microbial biomass (Stamatiadis et al., 1999; Piotrowska et al., 2011). The increased microbial activity in the soil might be a result of microbial community shifts introduced by SPH addition, originating from the BSFL gut, i.e., Actinomyces spp., Dysgonomonas spp., and Enterococcus spp. (Klammsteiner et al., 2020). Identifying microorganisms before and after SPH addition to the soil in the near future would allow further insights into the reasons for this increased activity.
No volatile fatty acids (VFAs) were detected in non-amended soils. Of the tested VFAs, only acetic acid was observed in samples with SPH under anaerobic conditions on a lab-scale. Acetic acid concentrations dropped to around 5 mg kg−1 within 10 days for SL + SPH and CL + SPH (Figure 2E). Upon fitting a 1st order decay model to the 5 measured points (R2 = 0.97 for CL and R2 = 0.63 for SL), results suggested acetic acid may degrade slightly slower in SL soil (k = 0.25) compared to CL soil (k = 2).
Based on the VFA development during 15 days, BSFL-digested pollinator hulls seem to be an appropriate organic material to be implemented in SBS approaches. The presence of acetic acid at the beginning of the incubation suggested that BSFL digestion did not manage to break down all phytotoxic organic acids in pollinator hulls. Furthermore, an increased microbial activity was attributed to the high respiration rates, indicating available sources of organic carbon that can be converted in biopesticides under the anaerobic conditions of SBS, decreasing the soil treatment time compared to traditional solarization of 4–6 weeks (Stapleton and DeVay, 1986). The pH change and detected acetic acid concentrations in SPH amended soil correspond to values, for which successful pest inactivation levels were achieved (Fernández-Bayo et al., 2018).
Phytotoxicity of Spent Hulls-Amended Soil
Spent Hulls Preliminary Assessment
The germination index (Gi) was used to determine the impact of spent almond hulls and incubation on the growth of radish seeds as the indicator of phytotoxicity. The lower the index, the higher the phytotoxicity. Preliminary analyses on water extracts from spent pollinator hulls at a dilution rate of 1:10 (g dw/g water) and at 1:100 had a Gi of 13 ± 7 and 42 ± 4%, respectively. The extract from the same SPH mixed (2% dw) with SL and CL at a 1:10 (g dw/g water) dilution of non-incubated samples showed a Gi of 120 ± 7 and 104 ± 14%, respectively (Figure 3). Given the toxicity found in the extracted almond hulls at 1:100 dilution, the buffer effect in the phytotoxicity can be explained by the effect of mixing the spent hulls with the soils and the potential adsorption/degradation to the soil particles of the compounds responsible for phytotoxicity. The reduction in phytotoxicity over time was observed in biosolarization trials with compost (Simmons et al., 2013), tomato (Achmon et al., 2016), and wine processing solid wastes (Achmon et al., 2016).
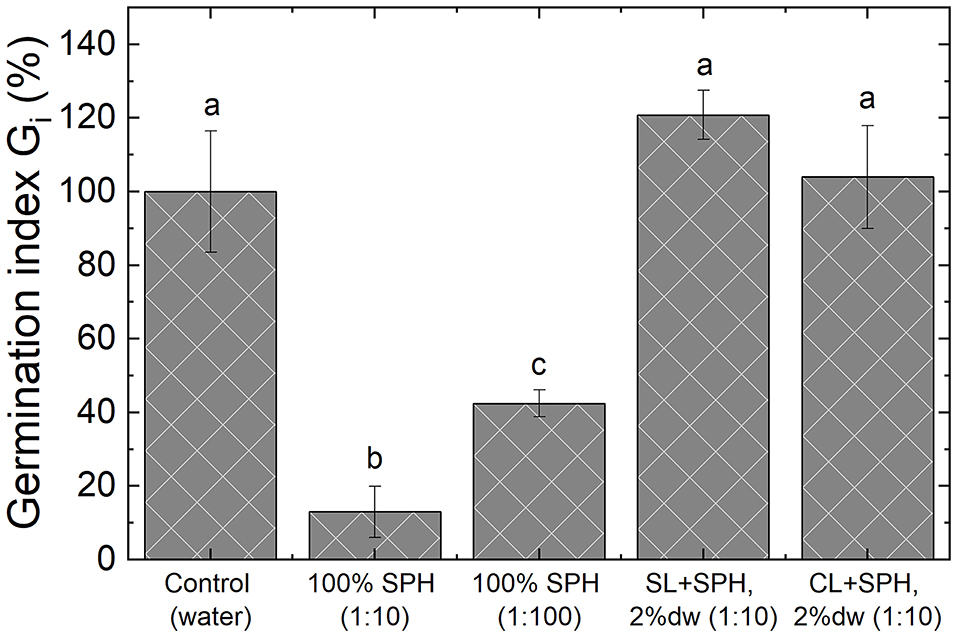
Figure 3. Phytotoxicity of water extracts of spent pollinator hulls (SPH) for different concentrations (1:10 and 1:100) and the control (water) compared to 2% dw spent pollinator hulls in sandy loam (SL + SPH) and sandy clay loam (CL + SPH) at same concentrations (1:10) for radish seeds. Different letters indicate significant differences in a one-way ANOVA followed by a Tukey's HSD post-hoc test. Bars represent standard deviations (10 seeds, n = 3).
Phytotoxicity Evolution in the Amended Soil
To assess if incubation under anaerobic (AN) or aerobic (AE) conditions of SPH in the soil can develop some phytotoxicity, a dilution ratio of 1:1 (g of sample dw/g water) was used in soils amended with SPH before and after incubation in AN and AE conditions for 15 days. The Gi of water extract from the incubated samples in AN conditions showed a significant effect (p < 0.05) of the interaction between SPH and soil type and between SPH and incubation type, i.e., non-incubated, incubated in AE, or incubated in AN conditions (Supplementary Table 4). Even though AE samples showed a slight improvement, when similar samples were compared under AN and AE conditions, there was no significant improvement of the germination index. Only SL + SPH under AN conditions showed a significantly higher Gi after 15 days compared to SL under AN conditions and CL + SPH before incubation (Figure 4). Furthermore, a paired-sample T-test (Supplementary Table 5) for same soil samples under the same conditions, i.e., AN and AE with and without adding SPH, differed significantly for AN SL and AN SL + SPH (t = −7.792, p = 0.016), and AE SL and AE SL + SPH (t = −4.794, p = 0.041).
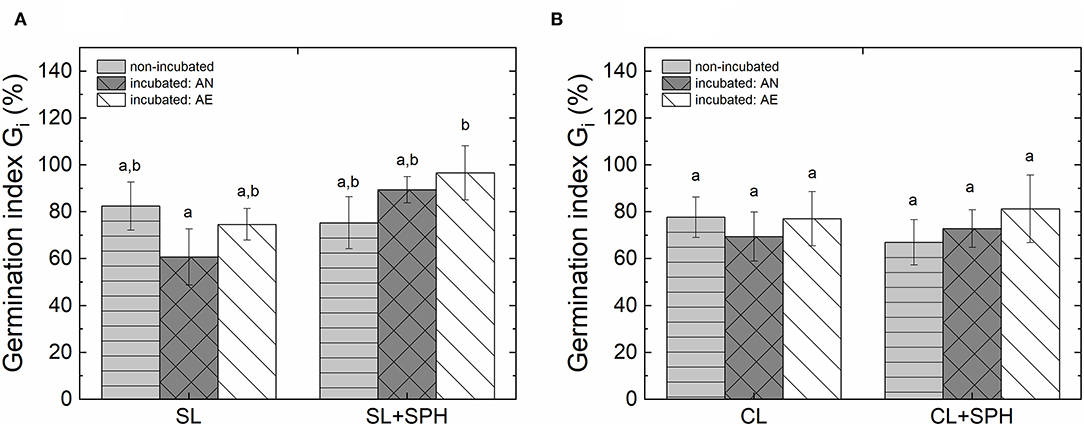
Figure 4. Phytotoxicity of water extracts (Dilution rate: 1:1 g dw/g water) for non-incubated and incubated (15 days) samples for two different soil types for radish seeds: (A) sandy loam, SL and (B) sandy clay loam, CL with (SPH, 2% dw) and without amended spent pollinator hulls under anaerobic (AN) and aerobic (AE) conditions. Different letters indicate significant differences in a one-way ANOVA followed by a Tukey's HSD post-hoc test. Bars represent standard deviations (10 seeds, n = 3).
Incubation of SL under AN conditions promoted some phytotoxicity in the soil. This may have been due to either changes in the soil or production of phytotoxic metabolites. This increase of the background phytotoxicity in the non-amended soil was not observed in the amended SL soil after incubation, suggesting that the amendments may have buffered the phytotoxicity or facilitated decomposition of phytotoxic metabolites. Furthermore, the drop in acetic acid (Figure 2E) observed in this study may have also contributed to the lower phytotoxicity observed in the SPH-amended soils undergoing incubation. It needs to be highlighted that both soils displayed a slight natural intrinsic phytotoxic effect on the model seed (Gi ~ 80%).
When correlating the pH, EC, VFA concentration to the phytotoxicity levels, no significant correlations were observed. Phytotoxic compounds seemed to be present, however, non-detected VFAs and organic matter might further contribute to the evaluated phytotoxicity (Shea et al., 2021).
Impact of Spent Hulls on Lettuce Growth
To further elucidate positive or negative effects of SPH on soil quality, the growth of fertigated lettuce was assessed in greenhouse trials. A multiway ANOVA showed a significant effect of the soil type and SPH addition on the soil phytotoxicity (Supplementary Table 6). For both soils, a significant reduction of the dried biomass (shoot and root system) of lettuce seedlings was observed in the samples amended with spent hulls after 14 days post-transplantation (p < 0.005, Figure 5 and Supplementary Figure 3) in comparison to the non-amended soils. Phytotoxic effects seemed to hinder the growth of the shoot system in particular, which was not quantified in the germination studies with radish seeds. Differing plant sensitivities to SPH phytotoxicity or differences in phytotoxin dilution in the varying growing formats could furthermore be attributed to the observation of an increased phytotoxic effect.
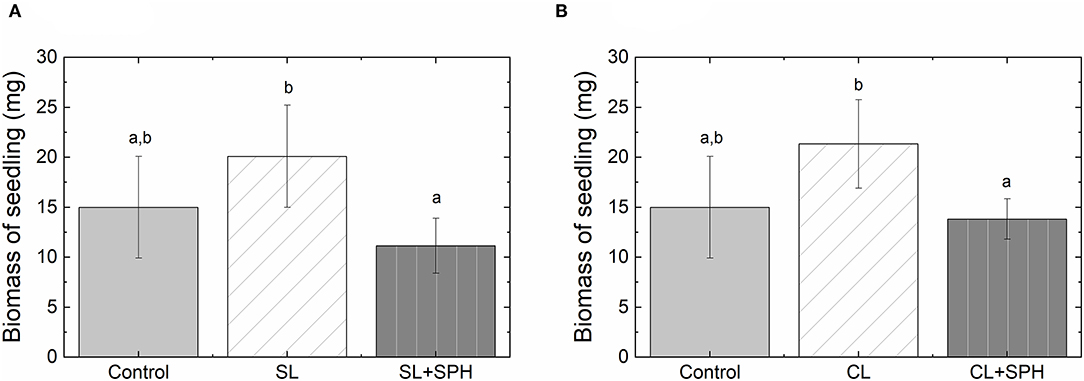
Figure 5. Comparison of dried lettuce (Lactuca sativa) biomass in different soil types: (A) sandy loam, SL and (B) sandy clay loam, CL with (2% dw) and without spent hulls after 14 days. The control soil: Coir-Lite Mix, 0.75 yd3 Coir, 0.25 yd3 Perlite, no SPH. Different letters indicate significant differences in a one-way ANOVA followed by a Tukey's HSD post-hoc test. Bars represent standard deviations (n = 10).
Impact of Spent Hulls on Soil Health
Significant effects of the incubation environment (AN, AE), soil type (SL, CL), SPH addition, and their interactions were observed (p < 0.05) on the macronutrient concentrations, i.e., carbon, nitrogen, nitrate, phosphate, ammonium, and potassium (Supplementary Tables 7–13). Spent almond hulls significantly (p < 0.05) increased the amount of carbon, nitrogen, C/N, phosphate, ammonium, and potassium content in SL + SPH, while nitrate did not significantly increase at the initial time t0 (Tables 1, 2, Supplementary Figure 4). Initial C/N values increased by about a factor of 2 with SPH amendment and remained significantly higher even after AN and AE incubation. A C/N ratio of stable and mature compost was defined by by Chefetz et al. (1996) between 10 and 15 and by Fang et al. (1999) between 17 and 19. A lower pH, a lower Gi index and a higher ammonium level furthermore indicate a lower stability (Palma et al., 2020). Alongside the rather low values of detected VFAs and rapid decrease (Figure 2E), this further suggests that SPH is a stable amendment. After 15 days under anaerobic conditions, nitrate in SL + SPH was reduced to 0.2 ± 0.0 mg kg−1, while ammonium increased significantly to 20.9 ± 1.5 mg kg−1. This effect must be considered for soil health and phytotoxicity in lower depths of the soil if amendments are distributed there. Ammonium levels were significantly higher in CL (+SPH) compared to SL (+SPH). This might potentially explain the slightly higher intrinsic phytotoxicity in CL, as ammonium can display a phytotoxic effect in sufficient quantities (Wong, 1985). Additionally, when comparing ammonium levels at t15 (with and without hulls), they decreased under aerobic conditions (Tables 1, 2, Supplementary Figure 4). Ammonium is expected to oxidize to nitrate under aerated conditions. Due to the texture of the soil, anaerobic conditions are better kept in CL compared to SL, favoring turning nitrate into ammonium. For biosolarization this is beneficial, as it can be one of the mechanisms associated with pathogen inactivation (Stapleton, 2000).
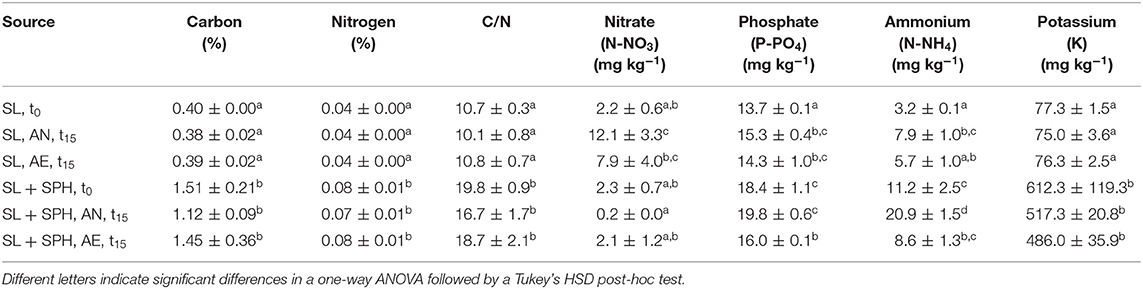
Table 1. Carbon, nitrogen, C/N ratio, nitrate, phosphate, ammonium, and potassium concentrations (n = 3) in sandy loam (SL) after 15 days (t15) under different conditions (AN, anaerobic; AE, aerobic), with and without spent pollinator hulls (SPH) and at initial time (t0).
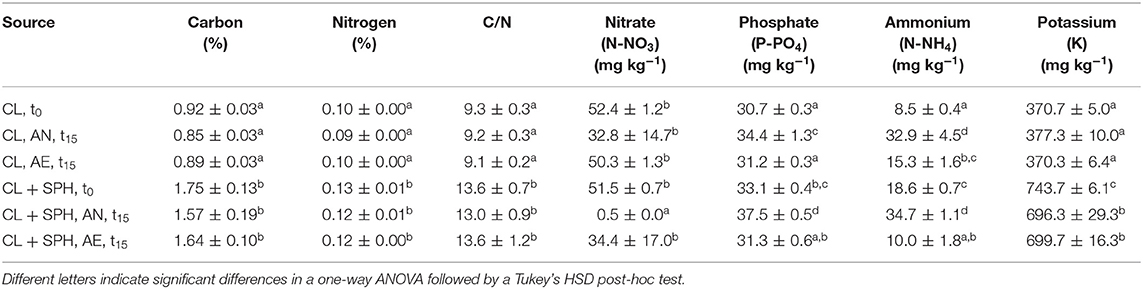
Table 2. Carbon, nitrogen, C/N ratio, nitrate, phosphate, ammonium, and potassium concentrations (n = 3) in sandy clay loam (CL) after 15 days (t15) under different conditions (AN, anaerobic; AE, aerobic), with and without spent pollinator hulls (SPH) and at initial time (t0).
The total amount of carbon that is retained in the soil is proportionally small to the additional CO2 that is released due to the amendments under AE conditions. For SL + SPH (AE) and CL + SPH (AE) the carbon content dropped by 0.064 and 0.235% dw, respectively (Supplementary Figure 4). However, in a paired-sample T-test, both SL + SPH (t = 0.212, p = 0.852) and CL + SPH (t = 0.953, p = 0.441) were not significantly different.
When correlating these soil nutrients to the phytotoxicity in SL, SL + SPH, CL, CL + SPH at t0 and t15, no macronutrient was significant at the 95% confidence interval. Ammonium had a Pearson's coefficient (r) of 0.208 (N = 10), for nitrate r = 0.299 (N = 10) and the soil had a coefficient equal to 0.309 (N = 10), further suggesting an intrinsic phytotoxicity, originating from undetected VFAs, ketones, aldehydes, or other phytotoxic molecules present in almond residue, such as cyanide (Chaouali et al., 2013). Cyanide was shown to reduce lettuce seed germination (Taylorson and Hendricks, 1973) and lettuce root length inhibition by more than half (Alström and Burns, 1989).
Conclusions
The addition of spent hulls from black soldier fly larvae cultivation in soils had a significant impact on the properties of the studied SL and CL soils. SPH addition resulted in a significant increase of carbon, nitrogen, C/N, phosphate, ammonium, and potassium in SL and CL. Respiration was significantly higher in both soils when SPH were incubated under AE conditions, mimicking broadcast soil application. The electrical conductivity after 15 days decreased significantly in the amended soil, suggesting that salts introduced by the amendments were sequestered in the microbial biomass following an increased microbial activity. The results indicated that phytotoxic compounds are present in SPH and SBS may be limited due to a higher stability of the SPH compared to another non-degraded biomass. The phytotoxic effect was further quantified in greenhouse trials where there was a significant reduction of the lettuce seedling biomass after 14 days when plants were grown in soil amended with SPH relative to non-amended soil. Further research is needed to determine the responsible compounds of the phytotoxicity and the role of microbial communities in the soil after SPH addition. Possible mitigation strategies could include implementing a buffer period between amendment and planting to allow phytotoxins to degrade or using transplanting methods where more mature plants can tolerate phytotoxins better, while profiting from the added nutrients. Although lab-scale experiments suggested that BSFL-digested almond hulls have the potential to be used as amendments in various types of soil, longer-term experiments and field studies with crop production will allow more conclusive statements on soil health, biomass yield, and the ability to control soilborne diseases.
Data Availability Statement
The raw data supporting the conclusions of this article will be made available by the authors, without undue reservation.
Author Contributions
CS, JF-B, and RA contributed toward the conception of the work, designed the experiments, drafted the work, and critically revised it for intellectual content. RA conducted the experiments. RA and JF-B acquired, analyzed, and interpreted the data. RA, CS, LP, JV, and JF-B contributed the final approval of the completed revision and ensured accountability for all aspects of the work in ensuring that questions related to accuracy and integrity of the work were appropriately managed. CS and JV provided the financial support. All authors contributed to the article and approved the submitted version.
Funding
This work was supported by the Almond Board of California (project 17-VandergheynstJ-COC-01), the National Institute of Food and Agriculture (project CA-D-BAE-2228-RR), and a gift from the Methionine Task Force.
Conflict of Interest
The authors declare that the research was conducted in the absence of any commercial or financial relationships that could be construed as a potential conflict of interest.
Acknowledgments
The authors were grateful to Andrew Hutchinson for his valuable assistance at the orchard park at UC Davis, Dr. Emily Shea for HPLC assistance and scientific input, Dr. Moritz Gold and Prof. Dr. Alexander Mathys from the Sustainable Food Processing group at ETH Zurich for fruitful discussions.
Supplementary Material
The Supplementary Material for this article can be found online at: https://www.frontiersin.org/articles/10.3389/fsufs.2021.664635/full#supplementary-material
Footnotes
1. ^USDA's National Agricultural Statistic's Service (Released July 7th, 2020): https://www.nass.usda.gov/Statistics_by_State/California.
2. ^Almond Board of California's annual reports (2019): https://www.almonds.com/about-us/annual-publications.
3. ^CDFA Agricultural Statistics Review: https://www.cdfa.ca.gov/Statistics/.
References
Achmon, Y., Fernández-Bayo, J. D., Hernandez, K., McCurry, D. G., Harrold, D. R., Su, J., et al. (2017). Weed seed inactivation in soil mesocosms via biosolarization with mature compost and tomato processing waste amendments. Pest Manag. Sci. 73, 862–873. doi: 10.1002/ps.4354
Achmon, Y., Harrold, D. R., Claypool, J. T., Stapleton, J. J., VanderGheynst, J. S., and Simmons, C. W. (2016). Assessment of tomato and wine processing solid wastes as soil amendments for biosolarization. Waste Manag. 48, 156–164. doi: 10.1016/j.wasman.2015.10.022
Al-qazzaz, M. F. A., Ismail, D., and Akit, H. (2016). Revista Brasileira de Zootecnia effect of using insect larvae meal as a complete protein source on quality and productivity characteristics of laying hens. Rev. Bras. Zootec. 45, 518–523. doi: 10.1590/s1806-92902016000900003
Alström, S., and Burns, R. G. (1989). Cyanide production by rhizobacteria as a possible mechanism of plant growth inhibition. Biol. Fertil. Soils 7, 232–238. doi: 10.1007/BF00709654
Angus, F., Gardner, A., Kirkegaard, J., and Desmarchelier, J. (1994). Biofumigation: isothiocyanates released from Brassica roots inhibit growth of the take-all fungus. Plant Soil 162, 107–112. doi: 10.1007/BF01416095
AOAC Official Method 972.43 (1997). Microchemical Determination of Carbon, Hydrogen, and Nitrogen, Automated Method, in Official Methods of Analysis of AOAC International, 16th Edn., Chapter 12. Arlington, VA: AOAC International.
Blok, W. J., Lamers, J. G., Termorshuizen, A. J., and Bollen, G. J. (2000). Control of soilborne plant pathogens by incorporating fresh organic amendments followed by tarping. Am. Phytopathol. Soc. 90, 253–259. doi: 10.1094/PHYTO.2000.90.3.253
Chaouali, N., Gana, I., Dorra, A., Khelifi, F., Nouioui, A., Masri, W., et al. (2013). Potential toxic levels of cyanide in almonds (Prunus amygdalus), apricot kernels (Prunus armeniaca), and almond syrup. ISRN Toxicol. 2013, 1–6. doi: 10.1155/2013/610648
Chefetz, B., Hatcher, P. G., Hadar, Y., and Chen, Y. (1996). Chemical and biological characterization of organic matter during composting of municipal solid waste. J. Environ. Qual. 25, 776. doi: 10.2134/jeq1996.00472425002500040018x
Chiou, B., Valenzuela-medina, D., Bilbao-sainz, C., Klamczynski, A. P., Avena-bustillos, R. J., Milczarek, R. R., et al. (2016). Torrefaction of almond shells: effects of torrefaction conditions on properties of solid and condensate products. Ind. Crop. Prod. 86, 40–48. doi: 10.1016/j.indcrop.2016.03.030
Díaz-Hernández, S., Gallo-Llobet, L., Domínguez-Correa, P., and Rodríguez, A. (2017). Effect of repeated cycles of soil solarization and biosolarization on corky root, weeds and fruit yield in screen-house tomatoes under subtropical climate conditions in the Canary Islands. Crop Prot. 94, 20–27. doi: 10.1016/j.cropro.2016.12.010
Diener, S., and Studt, N. M. (2011). Biological treatment of municipal organic waste using black soldier fly larvae. Waste Biomass Valoriz. 2, 357–363. doi: 10.1007/s12649-011-9079-1
Domínguez, P., Miranda, L., Soria, C., de los Santos, B., Chamorro, M., Romero, F., et al. (2014). Soil biosolarization for sustainable strawberry production. Agron. Sustain. Dev. 34, 821–829. doi: 10.1007/s13593-014-0211-z
Donn, S., Wheatley, R. E., McKenzie, B. M., Loades, K. W., and Hallett, P. D. (2014). Improved soil fertility from compost amendment increases root growth and reinforcement of surface soil on slopes. Ecol. Eng. 71, 458–465. doi: 10.1016/j.ecoleng.2014.07.066
Essabir, H., Nekhlaoui, S., Malha, M., Bensalah, M. O., Arrakhiz, F. Z., Qaiss, A., et al. (2013). Bio-composites based on polypropylene reinforced with almond shells particles: mechanical and thermal properties. Mater. Des. 51, 225–230. doi: 10.1016/j.matdes.2013.04.031
Fang, M., Wong, J. W. C., Ma, K. K., and Wong, M. H. (1999). Co-composting of sewage sludge and coal fly ash: nutrient transformations. Bioresour. Technol. 67, 19–24. doi: 10.1016/S0960-8524(99)00095-4
Fernández-Bayo, J. D., Achmon, Y., Harrold, D. R., Mccurry, D. G., Hernandez, K., Dahlquist-willard, R. M., et al. (2017). Assessment of two solid anaerobic digestate soil amendments for effects on soil quality and biosolarization efficacy. J. Agric. Food Chem. 65, 3434–3442. doi: 10.1021/acs.jafc.6b04816
Fernández-Bayo, J. D., Randall, T. E., Harrold, D. R., Achmon, Y., Hestmark, K. V., Su, J., et al. (2018). Effect of management of organic wastes on inactivation of Brassica nigra and Fusarium oxysporum f.sp. lactucae using soil biosolarization. Pest Manag. Sci. 74, 1892–1902. doi: 10.1002/ps.4891
Fernandez-Bayo, J. D., Yazdani, R., Simmons, C. W., and VanderGheynst, J. S. (2018). Comparison of thermophilic anaerobic and aerobic treatment processes for stabilization of green and food wastes and production of soil amendments. Waste Manag. 77, 555–564. doi: 10.1016/j.wasman.2018.05.006
Godfray, C. H. J., Beddingtion, J. R., Crute, I. R., Haddad, L., Lawrence, D., Muir, J. F., et al. (2010). Food security: the challenge of feeding 9 billion people. Science 327, 812–819. doi: 10.1126/science.1185383
Gold, M., Tomberlin, J. K., Diener, S., Zurbrügg, C., and Mathys, A. (2018). Decomposition of biowaste macronutrients, microbes, and chemicals in black soldier fly larval treatment: a review. Waste Manag. 82, 302–318. doi: 10.1016/j.wasman.2018.10.022
Griffin, T. S., and Hutchinson, M. (2007). Compost maturity effects on nitrogen and carbon mineralization and plant growth. Compost Sci. Util. 15, 228–236. doi: 10.1080/1065657X.2007.10702338
Haynes, R. J., and Mokolobate, M. S. (2001). Amelioration of Al toxicity and P deficiency in acid soils by additions of organic residues: a critical review of the phenomenon and the mechanisms involved. Nutr. Cycl. Agroecosyst. 59, 47–63. doi: 10.1023/A:1009823600950
Klammsteiner, T., Walter, A., Bogataj, T., Heussler, C. D., Stres, B., Steiner, F. M., et al. (2020). The core gut microbiome of black soldier fly (Hermetia illucens) larvae raised on low-bioburden diets. Front. Microbiol. 11:993. doi: 10.3389/fmicb.2020.00993
Ko, H. J., Kim, K. Y., Kim, H. T., Kim, C. N., and Umeda, M. (2008). Evaluation of maturity parameters and heavy metal contents in composts made from animal manure. Waste Manag. 28, 813–820. doi: 10.1016/j.wasman.2007.05.010
Leiber, F., Gelencsér, T., Stamer, A., Amsler, Z., Wohlfahrt, J., Früh, B., et al. (2015). Insect and legume-based protein sources to replace soybean cake in an organic broiler diet: effects on growth performance and physical meat quality. Renew. Agric. Food Syst. 32, 21–27. doi: 10.1017/S1742170515000496
Mitelut, A. C., Popa, M. E., Mitelut, A. C., and Popa, M. E. (2011). Seed germination bioassay for toxicity evaluation of different composting biodegradable materials. Rom. Biotechnol. Lett. 16, 121–129.
Naramabuye, F. X., and Haynes, R. J. (2006). Effect of organic amendments on soil pH and Al solubility and use of laboratory indices to predict their liming effect. Soil Sci. 171, 754–763. doi: 10.1097/01.ss.0000228366.17459.19
Nguyen, T. T. X., Tomberlin, J. K., and Vanlaerhoven, S. (2015). Ability of black soldier fly (Diptera: Stratiomyidae) larvae to recycle food waste. Environ. Entomol. 44, 406–410. doi: 10.1093/ee/nvv002
Offeman, R. D., Holtman, K. M., Covello, K. M., and Orts, W. J. (2014). Almond hulls as a biofuels feedstock: variations in carbohydrates by variety and location in California. Ind. Crop. Prod. 54, 109–114. doi: 10.1016/j.indcrop.2014.01.010
Olsen, S. R., and Sommers, L. E. (1982). “Phosphorus,” in Methods of Soil Analysis: Part 2. Chemical and Microbiological Properties, 2nd ed., ed A. L. Page (Madison, WI: ASA and SSSA) 403–30.
Palma, L., Ceballos, S. J., Johnson, P. C., Niemeier, D., Pitesky, M., and VanderGheynst, J. S. (2018). Cultivation of black soldier fly larvae on almond byproducts: impacts of aeration and moisture on larvae growth and composition. J. Sci. Food Agric. 98, 5893–5900. doi: 10.1002/jsfa.9252
Palma, L., Fernández-Bayo, J. D., Niemeier, D., Pitesky, M., and Vandergheynst, J. S. (2019). Managing high fiber food waste for the cultivation of black soldier fly larvae. NPJ Sci. Food 3:15. doi: 10.1038/s41538-019-0047-7
Palma, L., Fernández-Bayo, J. D., and Vandergheynst, J. S. (2020). Almond by-product composition impacts the rearing of black soldier fly larvae and quality of the spent substrate as a soil amendment. J. Sci. Food Agric. 100, 4618–4626. doi: 10.1002/jsfa.10522
Patel, N., Desai, P., Patel, N., Jha, A., and Gautam, H. K. (2014). Agronanotechnology for plant fungal disease management: a review. Int. J. Curr. Microbiol. Appl. Sci. 3, 71–84.
Pedrefio, J. N., Gmez, I., and Moral, R. (1996). Improving the agricultural value of a semi-arid soil by addition of sewage sludge and almond residue. Agric. Ecosyst. Environ. 58, 115–119. doi: 10.1016/0167-8809(95)01005-X
Pimentel, D., and Pimentel, M. (2003). Sustainability of meat-based and plant-based diets and the environment. Am. Soc. Clin. Nutr. 78, 660–663. doi: 10.1093/ajcn/78.3.660S
Piotrowska, A., Rao, M. A., Scotti, R., and Gianfreda, L. (2011). Changes in soil chemical and biochemical properties following amendment with crude and dephenolized olive mill waste water (OMW). Geoderma 161, 8–17. doi: 10.1016/j.geoderma.2010.11.011
Pognani, M., Imporzano, G. D., Scaglia, B., Adani, F., and Celoria, V. (2009). Substituting energy crops with organic fraction of municipal solid waste for biogas production at farm level: a full-scale plant study. Process Biochem. 44, 817–821. doi: 10.1016/j.procbio.2009.03.014
Rhoades, J. D. (1982). “Soluble salts,” in Methods of Soil Analysis: Part 2: Chemical and Microbiological Properties. Monograph Number 9, 2nd Edn., ed A. L. Page (Madison, WI: ASA) 167–79.
Ros, M., Garcia, C., Hernandez, M. T., Lacasa, A., Fernandez, P., and Pascual, J. A. (2008). Effects of biosolarization as methyl bromide alternative for Meloidogyne incognita control on quality of soil under pepper. Biol. Fertil. Soils 45, 37–44. doi: 10.1007/s00374-008-0307-1
Sarwar, M., Kirkegaard, J. A., Wong, P. T. W., and Desmarchelier, J. M. (1998). Biofumigation potential of brassicas. Plant Soil 201, 103–112. doi: 10.1023/A:1004381129991
Shea, E., Fernandez-Bayo, J. D., Pastrana, A. M., and Simmons, C. W. (2021). Identification and evaluation of volatile organic compounds evolved during solarization with almond hull and shell amendments. J. Air Waste Manage. Assoc. 71, 400–12.
Sheppard, D. C., Larry, G., Thompson, S. A., and Savage, S. (1995). A value added manure management system using the black soldier fly. Bioresour. Technol. 50, 275–279. doi: 10.1016/0960-8524(94)90102-3
Simmons, C. W., Guo, H., Claypool, J. T., Marshall, M. N., Perano, K. M., Stapleton, J. J., et al. (2013). Managing compost stability and amendment to soil to enhance soil heating during soil solarization. Waste Manag. 33, 1090–1096. doi: 10.1016/j.wasman.2013.01.015
Simmons, C. W., Higgins, B., Staley, S., Joh, L. D., Simmons, B. A., Singer, S. W., et al. (2016). The role of organic matter amendment level on soil heating, organic acid accumulation, and development of bacterial communities in solarized soil. Appl. Soil Ecol. 106, 37–46. doi: 10.1016/j.apsoil.2016.04.018
Smetana, S., Palanisamy, M., Mathys, A., and Heinz, V. (2016). Sustainability of insect use for feed and food: Life Cycle Assessment perspective. J. Clean. Prod. 137, 741–751. doi: 10.1016/j.jclepro.2016.07.148
Stamatiadis, S., Werner, M., and Buchanan, M. (1999). Field assessment of soil quality as affected by compost and fertilizer application in a broccoli field (San Benito County, California). Appl. Soil Ecol. 12, 217–225. doi: 10.1016/S0929-1393(99)00013-X
Stapleton, J. J. (2000). Soil solarization in various agricultural production systems. Crop Prot. 19, 837–841. doi: 10.1016/S0261-2194(00)00111-3
Stapleton, J. J., and DeVay, J. E. (1986). Soil solarization: a non-chemical approach for management of plant pathogens and pests. Crop Prot. 5, 190–198. doi: 10.1016/0261-2194(86)90101-8
Taylorson, R. B., and Hendricks, S. B. (1973). Promotion of seed germination by cyanide. Plant Physiol. 52, 23–27. doi: 10.1104/pp.52.1.23
Thomas, G. W. (1982). “Exchangeable cations,” in Methods of Soil Analysis: Part 2. Chemical and Microbiological Properties. ASA Monograph Number 9, ed A. L. Page (Madison, WI: Soil Science Society of America) 159–66.
Thomas, G. W. (1996). “Soil pH and soil acidity,” in Methods of Soil Analysis: Part 3 Chemical Methods, ed D. L. Sparks (Madison, WI: Soil Science Society of America) 5, 475–490. doi: 10.2136/sssabookser5.3.c16
Thomsen, E. O., Culumber, C. M., Reeve, J. R., Cardon, G., Alston, D., Black, B. L., et al. (2018). Strategies for Managing Soil Fertility and Health in Organic Orchards–A Fact Sheet. Logan, UT: Horticulture Utah State University Extension, 1–9.
Tiquia, S. M., Tam, N. F. Y., and Hodgkiss, I. J. (1996). Effects of composting on phytotoxicity of spent pig-manure sawdust litter. Environ. Pollut. 93, 249–256. doi: 10.1016/S0269-7491(96)00052-8
Velasco, M., and Schoner, C. (1965). Composition and feeding value of almond hulls and hull-shell meal. Calif. Agric. 19, 12–14.
Willett, W., Sacks, F., Trichopoulou, A., Drescher, G., Ferro-Luzzi, A., Helsing, E., et al. (1995). Mediterranean diet pyramid: a cultural model for healthy eating. Am. J. Clin. Nutr. 61, 1402S−1406S. doi: 10.1093/ajcn/61.6.1402S
Wong, M. H. (1985). Phytotoxicity of refuse compost during the process of maturation. Environ. Pollut. A Ecol. Biol. 37, 159–174. doi: 10.1016/0143-1471(85)90006-6
Wong, M. T. F., Nortcliff, S., and Swift, R. S. (1998). Method for determining the acid ameliorating capacity of plant residue compost, urban waste compost, farmyard manure, and peat applied to tropical soils. Commun. Soil Sci. Plant Anal. 29, 2927–2937. doi: 10.1080/00103629809370166
Yousef, M., Hassan, E., Mohammad, K., Dindarlou, S., Odongo, N. E., and Salem, A. Z. M. (2017). The chemical composition and in vitro digestibility evaluation of almond tree (Prunus dulcis D. A. Webb syn. Prunus amygdalus; var. Shokoufeh) leaves versus hulls and green versus dry leaves as feed for ruminants. Agrofor. Syst. 91, 773–780. doi: 10.1007/s10457-016-9964-5
Keywords: black soldier fly, almond residue, biosolarization, phytotoxcitiy, circular economy, sustainability
Citation: Axelrod R, Palma Miner L, VanderGheynst JS, Simmons CW and Fernandez-Bayo JD (2021) Soil Application of Almond Residue Biomass Following Black Soldier Fly Larvae Cultivation. Front. Sustain. Food Syst. 5:664635. doi: 10.3389/fsufs.2021.664635
Received: 05 February 2021; Accepted: 11 May 2021;
Published: 03 June 2021.
Edited by:
Paula Alvarenga, University of Lisbon, PortugalReviewed by:
Javier Veloso, University of A Coruña, SpainBlaz Stres, University of Ljubljana, Slovenia
Pedro Guirao, Miguel Hernández University of Elche, Spain
Copyright © 2021 Axelrod, Palma Miner, VanderGheynst, Simmons and Fernandez-Bayo. This is an open-access article distributed under the terms of the Creative Commons Attribution License (CC BY). The use, distribution or reproduction in other forums is permitted, provided the original author(s) and the copyright owner(s) are credited and that the original publication in this journal is cited, in accordance with accepted academic practice. No use, distribution or reproduction is permitted which does not comply with these terms.
*Correspondence: Christopher W. Simmons, Y3dzaW1tb25zQHVjZGF2aXMuZWR1