- Ryan Institute and School of Natural Sciences, National University of Ireland Galway, Galway, Ireland
Like many sectors, the expansion of aquaculture has issues related to sustainable resource use and environmental change. These challenges are widely recognised and are addressed with sectoral strategies. Even when culturing a single species, the specifics of impacts, constraints, and pressures are likely to vary in effects for different farm types. On the other hand, production efficiencies can drive farms towards homogeneity. A simple model is used in this study to demonstrate farm-scale budgets and the pressure to intensify production towards an optimum. A range of interventions can provide incentives for less intensive production: these include price premiums and altered cost bases. Integrated multitrophic aquaculture (IMTA) does not offer a route to less intensive production systems if the productivity of the extractive species (e.g., algae) is linked to the intensity of the fish farm, although alternative incentives for IMTA are possible. Increases in the intensity of production (as stocking density) can be mitigated by increasing farm capacity. An expanded production model suggests that this will lead to larger farms at relatively high stocking densities. Where farms are subject to variable economic and biological processes, this can lead to some combinations of intensity and capacity to have less variable earnings than others. The promotion of diverse aquaculture sectors may allow some of the ecological and social synergies available to smaller farms to be combined at a regional scale with the greater production of large farms. Cost, price and/or regulatory incentives are needed to create diverse production systems.
Introduction
Aquaculture is considered a key sector for future global food production (Costello et al., 2020). Growth rates in aquaculture are, however, heterogenous (Gentry et al., 2019), with the global rate of growth declining since the beginning of the century (FAO, 2020). The reasons for declining growth rates in aquaculture are varied and include restricted space for farms, public opposition, market issues, diseases, and licencing backlogs. Large-scale strategies are one response to the issues affecting aquaculture. The European Union guidelines for sustainable aquaculture made recommendations for licencing, spatial planning, business competitiveness, and capturing the benefits of shared environmental, welfare, and consumer protection (EC, 2013). China is also emphasising spatial planning and more environmentally sustainable aquaculture (Yu et al., 2020), while the United States focusses on improved regulation, sustainable management, technology, and public understanding (NOAA, 2011). While the current policies focus on improving the number, profitability, and sustainability of farms, they do not explicitly consider that social, resource use and economic optima may occur at different farm capacities or production intensities.
National and regional strategies attempt to influence the evolution of the focal sector. In aquaculture this evolution has often been towards production and economic efficiencies related to increases in scale. Production efficiencies are generally important for expanding the total size of a sector (Nielsen et al., 2016). Salmon aquaculture provides examples of growth trajectories involving increases in net pen capacity and farm production (Asche et al., 2013; Ellis et al., 2016). For example, the numbers of Scottish sites producing 1–50, 50–100, 101–200, and 201–500 t of salmon were approximately equal in the early 1990s. By 2014, the diversity of production had declined, with around half the sites in the >1,000 t category (Ellis et al., 2016). Increases in production scale occur widely, with the majority of European countries showing recent increases in FTE per aquaculture enterprise (Scientific, Technical and Economic Committee for Fisheries (STECF), 2018). The economic incentive to increase production of individual farms can be viewed in terms of profit optimisation. Once a farm is set up, further intensification can increase the profit margin: each additional fish stocked represents additional profit. Stocking increases eventually become unsustainable without additional investment, as biological production starts to become less efficient when crowding-related processes become limiting.
A simple model for the costs, sales and net earnings for a fish farm can be used to demonstrate the processes that tend to scale up and homogenise farm production. Changes in farm earnings may also occur in more complicated systems, such as those involving more than one species in an integrated multitrophic aquaculture (IMTA) system. IMTA involves growing different species together such that the wastes of one species (e.g., fish) supports the growth of one or more separate trophic levels (i.e., “extractive” species such as filter feeders or seaweed). A simple (fish-macroalgae) IMTA system is used to investigate how this type of aquaculture might change the optimum farm scale. Finally, the model can be used to demonstrate how farms of different intensity and capacity might perform in the face of temporal variation in key parameters.
A Farm-Scale Model
The implications of variations in farm intensity and capacity are likely to be applicable with a range of different species and contexts. The example used here to illustrate farm-scale economics is based on salmon, currently the most valuable aquaculture species in terms of international trade (FAO, 2020). The model could be generalised to describe aquaculture of any fed species and covers a production cycle with starting with juveniles and ending with the harvest of adults. For salmon this is approximately a 2-year process. Salmon farms are generally based on rearing juveniles in sea cages or pens, fed on a pellet diet. The parameters (Supplementary Data Sheet 1) of the modelled farm are mostly based on industry figures for salmon (MOWI, 2020).
The total costs for the modelled farm reflect juvenile supply costs, feed costs, harvest costs, and costs of farm infrastructure and labour. The modelled farm buys juveniles (smolts) conditioned to sea water from a hatchery at a fixed cost.
Where Jn is the number of juveniles purchased and jp is the price per juvenile. The stocking rate is used in this paper as a measure of farm intensity.
Feed costs [the main component of farm budgets, MOWI (2020)] are made up of the feed consumed by harvested adults, and the feed consumed by fish that die before harvest.
Where An is the number of adults, h is the harvest weight, jw is the weight of juveniles purchased, the feed conversion ratio, fcr, is the weight of feed used divided by the weight gain by fed fish, and fc is the cost of the feed. The feed conversion ratio is probably density dependent (Liu et al., 2015; Wang et al., 2019), with more feed needed per kilogram of fish as densities increase. Costs rise with higher values of the fcr, while a density dependent fcr increases costs with stocking intensity (Supplementary Material). A density dependent fcr is not, however, included in the model for reasons of parsimony: fcr is modelled as a constant, with the impact of density included solely through a density dependent mortality rate.
To estimate the food “lost” to individuals that do not reach harvest, density independent (m1) and density dependent (m2) mortality rates are used. The capacity of the farm (C) reflects the volume that fish are reared in, so that density dependent mortality is reduced when crowding in mitigated by more (or larger) fish cages. The fish that die early are assumed, on average, to have half the mean weight gain that fish have at harvest. Density dependent mortality reflects observations of reduced welfare at higher stocking rates (Santurtun et al., 2018). Mortality is not well-characterised. Not only is mortality commercially sensitive, repeated experiments at stressful densities would be both expensive and ethically hard to justify. Disease is likely to have higher impacts at greater densities if transmission is facilitated by crowding.
The harvest cost is based on the fraction of the fish remaining following gutting (gwt), the fish weight at harvest (h) and a cost per kilogramme of fish harvested (hc):
Buying and maintaining infrastructure, salaries, and repaying interest on loans are assumed to generate a farm cost per fish generation. A reasonable capacity for the target farm initially simulated is six fish cages, each of 35,000 m3 volume. With the parameter values used in this study, this gives an adult stocking rate just below the threshold for welfare effects (Turnbull et al., 2005). Actual costs will vary by country and with management and investment decisions. What was felt to be a reasonable figure for the initial model farm was chosen, given an estimated initial equipment cost (in Norway) of 3.5–4.5 million Euro (MOWI, 2020). If the capacity of the farm is fixed, costs only vary with the stocking rate of smolts. Greater investment allows more, or larger, cages, such that:
Where C is the volumetric capacity of the pens in the farm (m3), I is the investment cost required per harvest cycle and vc is the rate at which investment is converted into farm volume.
The value of fish farm sales is a function of number of fish surviving to harvest, the gutted weight and the sale price per kilogramme (sp).
Where An is related to the initial stocking density by:
Net farm earnings are sales minus total costs. The model's equations can be added to a spreadsheet (Supplementary Material) to investigate farm budgets.
Seaweed is used to illustrate an extractive species growth alongside a salmon farm (an IMTA implementation). A seaweed farm does not require additional costs beyond set up and maintenance/harvest costs. Costs for a 1000 t, annual harvest farm were based on an extrapolation of the figures for a Laminaria digitata farm (Watson and Dring, 2011). It was assumed that efficiencies could be found so that production of seeded ropes and maintenance of a seaweed farm could be achieved for €200,000 per annum. Wet seaweed was assumed to be sold (ep) for €2 kg−1 (Watson and Dring, 2011).
While there is evidence for promotion of kelp growth in the vicinity of fish farms (Kerrigan and Suckling, 2018), the size of this effect is likely to depend on many factors, such as farm layout and current speeds. In the absence of a well-defined response in the literature, the influence of additional nitrogen available to seaweed configured in an IMTA with a salmon farm was assumed to follow a Michealis-Menten type relationship.
Where sb is the baseline seaweed harvest in the absence of nutrients from the fish farm, smax is the maximum harvest possible, Tf is the total food used by the farm, an index of potential nutrient supply to the environment, and ks is the half saturation constant (gives a measure of how quickly the nutrients supplied by a fish farm saturate the seaweed's capacity to respond).
Different scenarios were used to illustrate how farm economics may vary under scale related assumptions. Earnings examples are first developed using a fixed farm capacity (so that farms vary only in stocking density), before allowing both stocking density and farm investment in capacity to vary. The effect of an organic salmon price premium was illustrated using a sales price increase from €5.9 to €8.9 kg−1 for fish grown at adult densities below 10 kg m−3 (using the 6 × 35,000 m3 pen volume as a reference). The effect of finding cheaper farm set ups at low smolt density (e.g., using pre-existing ponds) can be illustrated by halving the fixed costs below a density threshold. Finally, the influence of cost and price variability can be simulated by a Monte Carlo process: selecting parameter values from a range around the mean.
Optimal Farm Intensity
The overall pattern for the simulated farm with fixed capacity is for costs to rise as inputs (juveniles stocked) increase (Figure 1A). The smallest farms do not make a profit, as costs exceed sales. As the number of juveniles stocked increases, earnings rise before eventually starting to decrease [a similar result has been reported for a cod model (Björnsson et al., 2012)]. The decreases in sales and earnings occur as a result of declines in the adult population due to progressively stronger mortality as the initial stocking density rises. The scenario of an earnings collapse with very high stocking rates represents a realistic outcome. A very high stocking density would probably result in complete mortality, with no juveniles successfully growing to adulthood.
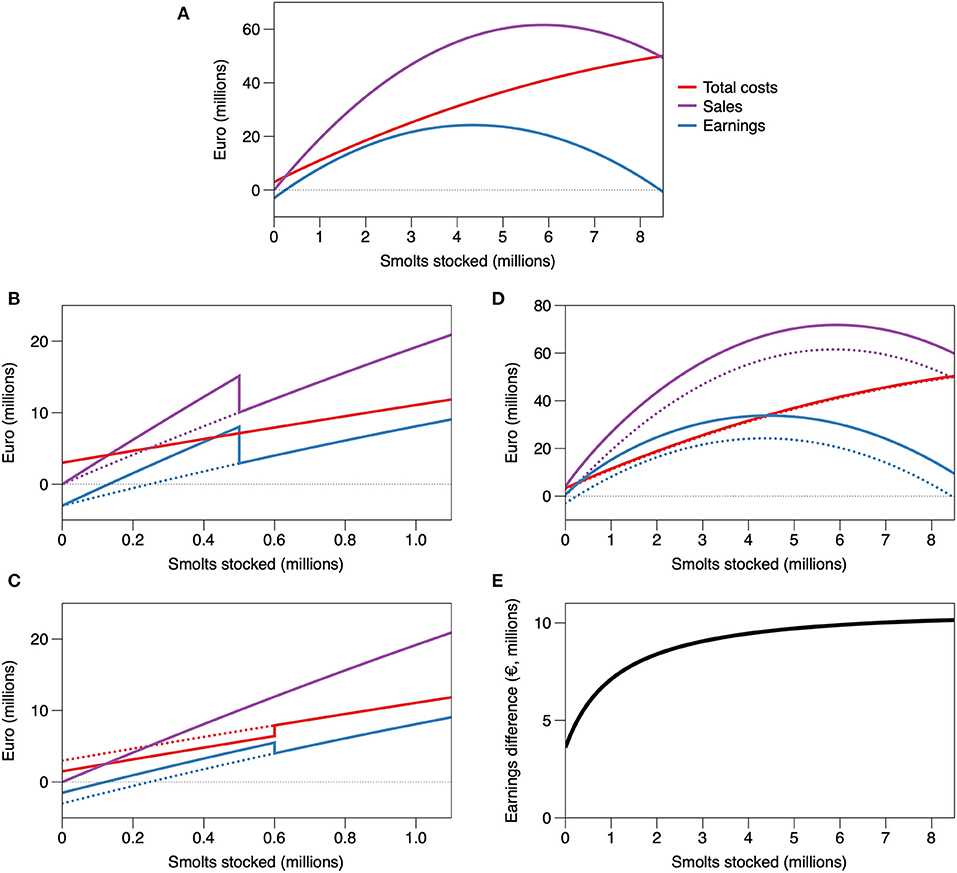
Figure 1. Modelled costs, sales value, and net earnings for a fish farm at a fixed pen capacity (6 × 35,000 m3). (A) Showing earnings across a range of juvenile (smolt) stocking densities, (B) Impact of a price premium (sales at €8.9 kg−1) below a stocking threshold, as occurs for organic salmon, (C) Impact of reduced fixed costs below a certain stocking level (costs reduced by 50% below 0.6 million smolts as an example), (D) Integrated multitrophic aquaculture (IMTA) at different smolt stocking levels, comparing IMTA and fish-only farms, (E) Difference between the earnings of IMTA and fish-only systems. Dotted lines in (B–D) indicate the original values in panel (A). Some graphs are truncated at 1.1 million smolts as this is the figure used in the industry handbook (MOWI, 2020).
For a salmon farm based on the model and parameters of Table 1 (with a six pen capacity costing €3 million), the weight of adults to be harvested from 1.1 million smolts would be 4,217 t, mortality 0.15, gutted weight yield per smolt of 3.22 kg, and final stocking density of 20.1 kg m−3. These values are consistent with industry norms (MOWI, 2020). The model implies that farms more intensive than 4,200 t may be more profitable. Environmental constraints, regulation, and/or increased risks (e.g., all investment in a single location) are probably reflected in a lower farming intensity than the modelled peak being considered relevant in the salmon farming yearbook (MOWI, 2020). The possibilities of increased profit from larger and potentially more intensive farms are, however, illustrated by proposals within the industry for production volumes over 10,000 t at offshore and onshore farms.
Promoting Diverse Farm Production Intensities
If most species grown in aquaculture have a production scale where profitability is maximised, there a number of ways in which a diversity of scales can be achieved. Taking the simple model presented here, steps in the sales price, such as thresholds below which the product is more valuable, can be a means for lower and higher intensity production to coexist (Figure 1B). The production and marketing of organic salmon grown at densities of <10 kg m−3 is an example of how price variability can create economic viability at different farm intensities. As an alternative, or alongside changes in the price structure, the cost base can be altered. For example, it may be cheaper to use pre-existing ponds like the esteros (Yúfera and Arias, 2010) of southern Europe rather than to use more costly new infrastructure (Figure 1C). While lowering feed costs is a priority for carnivorous fish, a focus on routes to profitability for small farms may further incentivize diversifying production with omnivorous or herbivorous species. Changes to the way personnel and social capital are invested in production [community-based aquaculture (Bradford et al., 2020)] could also offer opportunities to change the cost base, including varying the cost of licences, to target profitability of less intensive production. In a full life cycle assessment (Samuel-Fitwi et al., 2013; Liu et al., 2016), there are likely to be a number of points where specific benefits could be subsidised or linked to the market to support a range of farm intensities and capacities.
A potential innovation in aquaculture is to expand the range and prevalence of integrated multitrophic aquaculture (IMTA). Looking at the relative prevalence of IMTA in Asia and Europe, it is tempting to conclude that IMTA suits small scale operations, while established large-scale fish farmers in Europe are not incentivised to complicate their businesses by adding less profitable extractive species (Hughes and Black, 2016). The simple economic model of a fish farm suggests that a small-scale optimum for IMTA profitability is not inevitable (Figure 1D). If growth of the extractive species is stimulated by the waste production of fish, the difference between farm earnings with and without IMTA is likely to mimic this growth response (Figure 1E). This means that two unintended consequences are possible: (a) the optimum IMTA profitability is at a higher farm intensity than the original fish monoculture, and (b) the rate of return for marginal increases of juvenile stocking is greater in IMTA than in monoculture. Both consequences would make increasing juvenile stocking numbers attractive for IMTA farmers and would disincentivize less intensive production.
A policy intervention that could be used to incentivize IMTA is a credit that directly rewards the removal of carbon or nitrogen (Chopin et al., 2012) by seaweed. Using a tax that increases the costs of intensive production (e.g., a nitrogen tax) can produce a lower optimum production density (i.e., a steeper total costs curve in Figure 1A). On its own, however, a nitrogen tax does not tend to incentivise the least intensive farms. As costs are added to all farms, the least intensive farms also become less profitable. This reflects the finding that such Pigouvian taxes can reduce production intensity, but at the expense of the viability of less intense farms (León-Santana and Hernández, 2008). Adding tax credits for nitrogen removal in IMTA produces the same response as Figure 1E: the gross value of the credit is likely to match the stimulation of extractive species growth when increasing fish densities. Encouraging a range of farm intensities would need a carefully set up, and potentially complex, tax and credit system. Low intensity IMTA may therefore need the type of price incentive that exists for organic aquaculture (van Osch et al., 2017), or an explicit subsidy for the ecosystem benefits, and/or synergies between culture species that can only be realised at lower farm intensity or capacity.
Benefits at Different Farm Capacity and Intensity
While increasing the intensity of production eventually reduces earnings, investments to scale up farm capacity can mitigate this. The farms with the highest earnings are likely to have a large capacity and to stock high numbers of smolts (Figure 2A). Low capacity-high intensity or low intensity-high capacity farms are not financially viable. The basic economic incentives therefore promote both larger and more intensive farming.
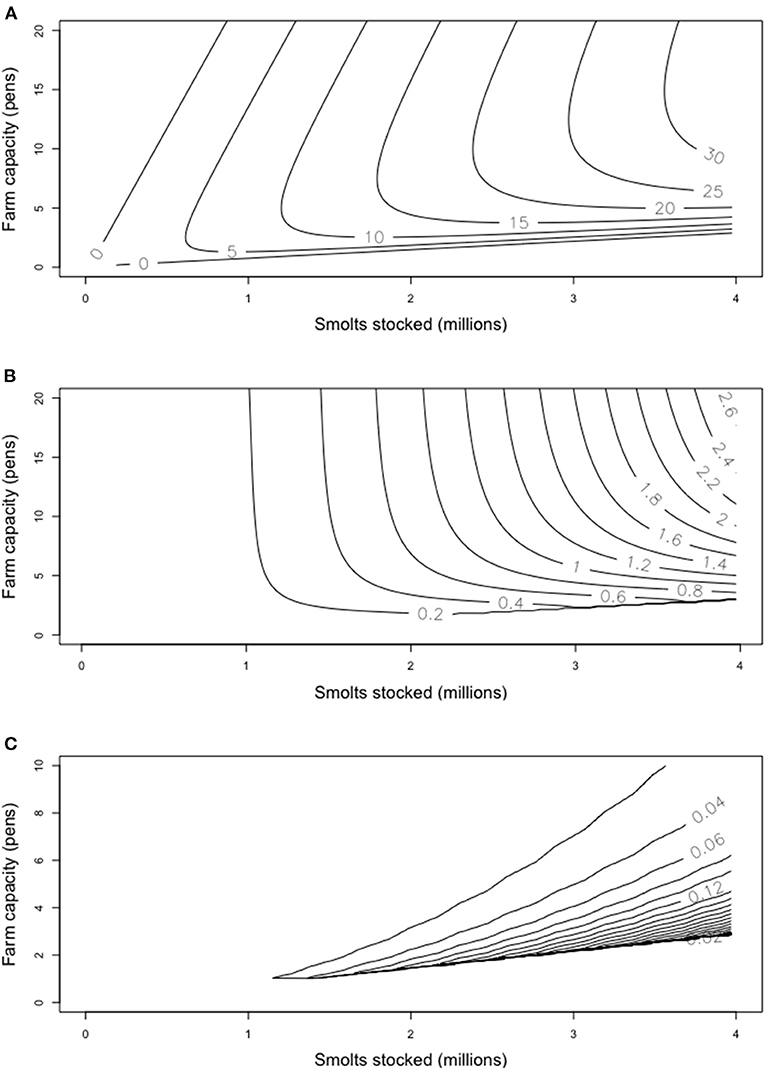
Figure 2. Results from 50 simulated production cycles for farms at different stocking densities and capacities (expressed as number of 35,000 m3 pens). (A) Average earnings (million Euro); (B) Variance in earnings for simulations where all parameters except harvest weight vary by 2.5%, chosen from a uniform distribution; (C) Variance in earnings when only the density dependent mortality rate varies by 2.5%.
Earnings vary in separate harvests in the Monte Carlo simulations. Broadly speaking, earnings variability increases with mean earnings. The contours in Figure 2B, however, do not quite match those of Figure 2A. This indicates that farms of similar earnings may have different temporal variance in their earnings over time.
In aquaculture, disease represents one of the sources of variability between years. Temporal prevalence of diseases may be linked to environmental cues like anomalously warm temperatures (Oldham et al., 2016), leading to outbreaks that track environmental variability. The impacts of disease are also likely to be density dependent, where higher densities lead to greater transmission and individuals being more stressed (Turnbull et al., 2005). If the Monte Carlo simulations are restricted to reflect dominance by density dependent processes, earnings variability is concentrated in the high intensity-lower capacity farms (Figure 2C).
The changes in variance with farm capacity and intensity imply that a diverse collection of farms may have more stable earnings than a homogenous sector. This type of benefit-of-diversity effect has been seen in agriculture for comparisons of yield in response to climate variability (Reidsma et al., 2010). A range of farm types may also dampen the tendency for the cycles in profitability in aquaculture. Such cycles are often associated with cash flow and trade issues, for example in the salmon farming industry (Asche and Bjørndal, 2011).
Growth of farm capacity and intensity over time may cause some positive synergies to be lost. In many areas of Europe, fish farms are based in peripheral communities, giving them particular socio-economic importance for the coastal areas where they are based. Smaller operators may need to differentiate their offer to persist in the market, competing on quality, supply to local markets or through innovation in processing and packaging (Llorente et al., 2020). Larger companies and farms be disconnected from the adjoining community. For example, fish from a large and intensive farm may not be available locally (Bresnihan, 2016). With no distinctive produce to offer tourists, additional benefits do not accrue to the community. Collaboration, by sharing resources and experience among smaller local businesses, may create wider social benefits in terms of shared community values and resilience. Both formal and informal cooperation among small entities in the aquaculture sector can help businesses persist (Cush and Varley, 2013).
A further synergy, perhaps more available to small capacity and low intensity aquaculture producers, is the opportunity to develop alongside conservation (and other sectors). This is particularly relevant in areas like coastal waters, where there is limited space available for aquaculture. Aquaculture can have positive local influences on ecosystems, including through the provision of habitat and through ecosystem services, for example if extractive organisms are being grown (Froehlich et al., 2017). Of course much needs to be done to find the appropriate scale and type of locally synergistic aquaculture (Le Gouvello et al., 2017). It seems likely that locally-based enterprises are best placed to have the networks, local knowledge and flexibility to find these synergies.
Conclusion
The benefit of stressing a diversity of farm types in aquaculture strategies is that this approach is inclusionary. Diversity as a goal avoids casting the debate as one of intensive or extensive aquaculture at a national or regional level (“sea sparing” or “sea sharing”). While the impacts of larger farms will vary with context and location, the challenges of maintaining and expanding aquaculture production will not be met without intensive, high volume production. Accepting and promoting diversity, however, increases the range of situations in which aquaculture can be developed. The concept of scale-dependent synergies with different aspects of economic, social or ecological sustainability allows a farm diversity-promoting framework to integrate with the ecosystem approach to aquaculture (FAO, 2008). Diverse aquaculture sectors are more likely to produce the heterogenous and flexible production systems identified as key to resilient global food production (Troell et al., 2014; Urruty et al., 2016). The challenges are to gather the appropriate data on farm production, including for other species and implementations of IMTA, and to develop policies that enable diversity without having unintended consequences at any particular scale.
Data Availability Statement
The original contributions generated for the study are included in the article/Supplementary Material, further inquiries can be directed to the corresponding author.
Author Contributions
The author confirms being the sole contributor of this work and has approved it for publication.
Conflict of Interest
The author declares that the research was conducted in the absence of any commercial or financial relationships that could be construed as a potential conflict of interest.
Supplementary Material
The Supplementary Material for this article can be found online at: https://www.frontiersin.org/articles/10.3389/fsufs.2021.655346/full#supplementary-material
References
Asche, F., and Bjørndal, T. (2011). The Economics of Salmon Aquaculture. Hoboken, NJ: Wiley-Blackwell.
Asche, F., Roll, K. H., Sandvold, H. N., Sorvig, A., and Zhang, D. (2013). Salmon aquaculture: larger companies and increased production. Aquac. Econ. Manag. 17, 322–339. doi: 10.1080/13657305.2013.812156
Björnsson, B., Steinarsson, A., Oddgeirsson, M., and Ólafsdóttir, S. R. (2012). Optimal stocking density of juvenile Atlantic cod (Gadus morhua L.) reared in a land-based farm. Aquaculture 356–357, 342–350. doi: 10.1016/j.aquaculture.2012.04.047
Bradford, J., Filgueira, R., and Bailey, M. (2020). Exploring community-based marine aquaculture as a coastal resource management opportunity in Nova Scotia, Canada. Facets 5, 26–48. doi: 10.1139/facets-2019-0010
Bresnihan, P. (2016). The Dynamics of Environmental Sustainability and Local Development: Aquaculture. No. 143. Dublin: National Economic and Social Council. Available online at: http://files.nesc.ie/nesc_reports/en/143_EnvSus_and_LocDev_Aquaculture.pdf (accessed January 15, 2021).
Chopin, T., Cooper, J. A., Reid, G., Cross, S., and Moore, C. (2012). Open-water integrated multi-trophic aquaculture: environmental biomitigation and economic diversification of fed aquaculture by extractive aquaculture. Rev. Aquac. 4, 209–220. doi: 10.1111/j.1753-5131.2012.01074.x
Costello, C., Cao, L., Gelcich, S., Cisneros-Mata, M. A., Free, C. M., Froehlich, H. E., et al. (2020). The future of food from the sea. Nature 588, 95–100. doi: 10.1038/s41586-020-2616-y
Cush, P., and Varley, T. (2013). Cooperation as a survival strategy among west of Ireland small-scale mussel farmers. Marit. Stud. 12:11. doi: 10.1186/2212-9790-12-11
EC (2013). Strategic Guidelines for the Sustainable Development of EU Aquaculture. COM/2013/0229. Available online at: https://eur-lex.europa.eu/legal-content/EN/TXT/?uri=CELEX:52013DC0229 (accessed January 15, 2021).
Ellis, T., Turnbull, J. F., Knowles, T.G, Lines, J. A., and Auchterlonie, N. A. (2016). Trends during development of Scottish salmon farming: an example of sustainable intensification? Aquaculture 458, 82–99. doi: 10.1016/j.aquaculture.2016.02.012
FAO (2008). “Building an ecosystem approach to aquaculture,” in FAO/Universitat de les Illes Balears Expert Workshop, 7-11 May, 2007, eds D. Soto, J. Aguilar-Manjarrez, N. Hishamunda, and Food and Agriculture Organization of the United Nations, Rome (Palma de Mallorca).
FAO (2020). The State of World Fisheries and Aquaculture 2020. Rome: Food and Agriculture Organization of the United Nations. Available online at: http://www.fao.org/publications/sofia/2020/en/ (accessed June 25, 2020).
Froehlich, H. E., Gentry, R. R., and Halpern, B. S. (2017). Conservation aquaculture: shifting the narrative and paradigm of aquaculture's role in resource management. Biol. Conserv. 215, 162–168. doi: 10.1016/j.biocon.2017.09.012
Gentry, R. R., Ruff, E. O., and Lester, S. E. (2019). Temporal patterns of adoption of mariculture innovation globally. Nat. Sustain. 2, 949–956. doi: 10.1038/s41893-019-0395-y
Hughes, A., and Black, J. (2016). Going beyond the search for solutions: understanding trade-offs in European integrated multi-trophic aquaculture development. Aquac. Environ. Interact. 8, 191–199. doi: 10.3354/aei00174
Kerrigan, D., and Suckling, C. C. (2018). A meta-analysis of integrated multitrophic aquaculture: extractive species growth is most successful within close proximity to open-water fish farms. Rev. Aquac. 10, 560–572. doi: 10.1111/raq.12186
Le Gouvello, R., Hochart, L.-E., Laffoley, D., Simard, F., Andrade, C., Angel, D., et al. (2017). Aquaculture and marine protected areas: potential opportunities and synergies. Aquat. Conserv. Mar. Freshw. Ecosyst. 27, 138–150. doi: 10.1002/aqc.2821
León-Santana, M., and Hernández, J. M. (2008). Optimum management and environmental protection in the aquaculture industry. Ecol. Econ. 64, 849–857. doi: 10.1016/j.ecolecon.2007.05.006
Liu, B., Liu, Y., and Wang, X. (2015). The effect of stocking density on growth and seven physiological parameters with assessment of their potential as stress response indicators for the Atlantic salmon (Salmo salar). Mar. Freshw. Behav. Physiol. 48, 177–192. doi: 10.1080/10236244.2015.1034956
Liu, Y., Rosten, T. W., Henriksen, K., Skontorp Hognes, E., Summerfelt, S., and Vinci, B. (2016). Comparative economic performance and carbon footprint of two farming models for producing Atlantic salmon (Salmo salar): land-based closed containment system in freshwater and open net pen in seawater. Aquacult. Eng. 71, 1–12. doi: 10.1016/j.aquaeng.2016.01.001
Llorente, I., Fernández-Polanco, J., Baraibar-Diez, E., Odriozola, M. D., Bjørndal, T., Asche, F., et al. (2020). Assessment of the economic performance of the seabream and seabass aquaculture industry in the European Union. Mar. Policy 117:103876. doi: 10.1016/j.marpol.2020.103876
MOWI (2020). Salmon Farming Industry Handbook 2020. Available online at: https://mowi.com/it/wp-content/uploads/sites/16/2020/06/Mowi-Salmon-Farming-Industry-Handbook-2020.pdf (accessed March 11, 2020).
Nielsen, R., Asche, F., and Nielsen, M. (2016). Restructuring European freshwater aquaculture from family-owned to large-scale firms—lessons from Danish aquaculture. Aquac. Res. 47, 3852–3866. doi: 10.1111/are.12836
NOAA (2011). Marine Aquaculture Policy. National Oceanic and Atmospheric Administration. Available online at: https://media.fisheries.noaa.gov/dam-migration/2011_noaa_aquaculture_policy.pdf (accessed March 11, 2020).
Oldham, T., Rodger, H., and Nowak, B. F. (2016). Incidence and distribution of amoebic gill disease (AGD)—an epidemiological review. Aquaculture 457, 35–42. doi: 10.1016/j.aquaculture.2016.02.013
Reidsma, P., Ewert, F., Lansink, A. O., and Leemans, R. (2010). Adaptation to climate change and climate variability in European agriculture: the importance of farm level responses. Eur. J. Agron. 32, 91–102. doi: 10.1016/j.eja.2009.06.003
Samuel-Fitwi, B., Nagel, F., Meyer, S., Schroeder, J. P., and Schulz, C. (2013). Comparative life cycle assessment (LCA) of raising rainbow trout (Oncorhynchus mykiss) in different production systems. Aquac. Eng. 54, 85–92. doi: 10.1016/j.aquaeng.2012.12.002
Santurtun, E., Broom, D. M., and Phillips, C. J. C. (2018). A review of factors affecting the welfare of Atlantic salmon (Salmo salar). Anim. Welf. 27, 193–204. doi: 10.7120/09627286.27.3.193
Scientific Technical and Economic Committee for Fisheries (STECF). (2018). Economic Report of the EU Aquaculture sector (STECF-18-19). Luxembourg: Publications Office of the European Union.
Troell, M., Naylor, R. L., Metian, M., Beveridge, M., Tyedmers, P. H., Folke, C., et al. (2014). Does aquaculture add resilience to the global food system? Proc. Natl. Acad. Sci. U.S.A. 111, 13257–13263. doi: 10.1073/pnas.1404067111
Turnbull, J., Bell, A., Adams, C., Bron, J., and Huntingford, F. (2005). Stocking density and welfare of cage farmed Atlantic salmon: application of a multivariate analysis. Aquaculture 243, 121–132. doi: 10.1016/j.aquaculture.2004.09.022
Urruty, N., Tailliez-Lefebvre, D., and Huyghe, C. (2016). Stability, robustness, vulnerability, and resilience of agricultural systems. A review. Agron. Sustain. Dev. 36:15. doi: 10.1007/s13593-015-0347-5
van Osch, S., Hynes, S., O'Higgins, T., Hanley, N., Campbell, D., and Freeman, S. (2017). Estimating the Irish public's willingness to pay for more sustainable salmon produced by integrated multi-trophic aquaculture. Mar. Policy 84, 220–227. doi: 10.1016/j.marpol.2017.07.005
Wang, Y., Chi, L., Liu, Q., Xiao, Y., Ma, D., Xiao, Z., et al. (2019). Effects of stocking density on the growth and immunity of Atlantic salmon Salmo salar reared in recirculating aquaculture system (RAS). J. Oceanol. Limnol. 37, 350–360. doi: 10.1007/s00343-019-7350-7
Watson, L., and Dring, M. (2011). Business Plan for the Establishment of a Seaweed Hatchery and Grow-out Farm. Dublin: BIM. Available online at: http://www.bim.ie/media/bim/content/publications/Business,Plan,fot,the,Establishment,of,a,Seaweed,Hatchery,and,Grow-out,Farm.pdf (accessed July 10, 2020).
Yu, J., Yin, W., and Liu, D. (2020). Evolution of mariculture policies in China: experience and challenge. Mar. Policy 119:104062. doi: 10.1016/j.marpol.2020.104062
Yúfera, M., and Arias, A. M. (2010). Traditional polyculture in “Esteros” in the Bay of Cádiz (Spain). Hopes and perspectives for the prevalence of a unique activity in Europe. Aquac. Eur. 35, 22–25. http://hdl.handle.net/10261/50632
Keywords: extensive aquaculture, conservation aquaculture, integrated multitrophic aquaculture, heterogenous, resilience
Citation: Johnson MP (2021) Farm Production Diversity in Aquaculture Has Been Overlooked as a Contributor to Sustainability. Front. Sustain. Food Syst. 5:655346. doi: 10.3389/fsufs.2021.655346
Received: 18 January 2021; Accepted: 24 May 2021;
Published: 15 June 2021.
Edited by:
Åsa Strand, IVL–Swedish Environmental Research Institute, SwedenReviewed by:
Emma Louise Burns, Australian National University, AustraliaHalmar Halide, Hasanuddin University, Indonesia
Copyright © 2021 Johnson. This is an open-access article distributed under the terms of the Creative Commons Attribution License (CC BY). The use, distribution or reproduction in other forums is permitted, provided the original author(s) and the copyright owner(s) are credited and that the original publication in this journal is cited, in accordance with accepted academic practice. No use, distribution or reproduction is permitted which does not comply with these terms.
*Correspondence: Mark P. Johnson, bWFyay5qb2huc29uQG51aWdhbHdheS5pZQ==