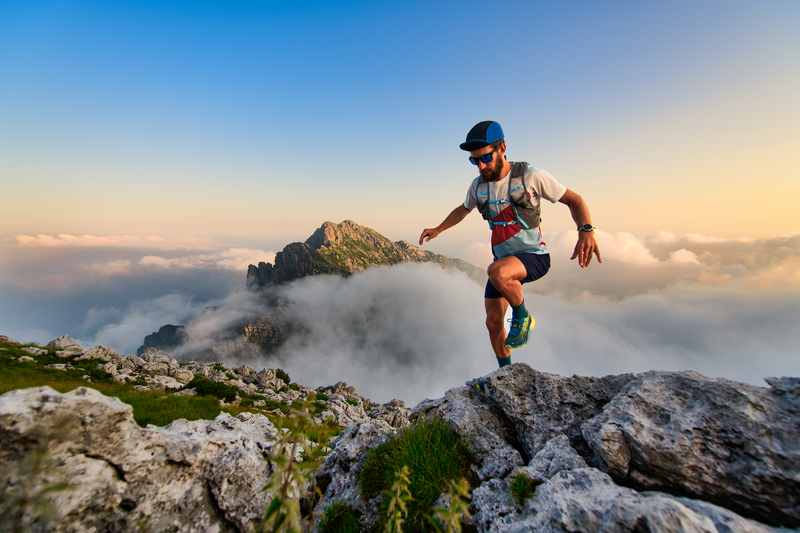
94% of researchers rate our articles as excellent or good
Learn more about the work of our research integrity team to safeguard the quality of each article we publish.
Find out more
ORIGINAL RESEARCH article
Front. Sustain. Food Syst. , 11 March 2021
Sec. Crop Biology and Sustainability
Volume 5 - 2021 | https://doi.org/10.3389/fsufs.2021.630628
This article is part of the Research Topic Plant Growth-Promoting Microorganisms for Sustainable Agricultural Production View all 50 articles
Bacillus thuringiensis strain NEB17, produces a bacteriocin, thuricin17 (Th17) and is known to promote the growth more effectively under salt stress conditions. In this study, bacterial salt stress tolerance screening and the possible changes in its secretome under two levels of NaCl stress was evaluated. The salt tolerance screening suggested that the bacterium is able to grow and survive in up to 900 mM NaCl. Thuricin17 production at salt levels from 100 to 500 mM NaCl was quantified using High Performance Liquid Chromatography (HPLC). Salt stress adversely affected the production of Th17 at levels as low as 100 mM NaCl; and the production stopped at 500 mM NaCl, despite the bacterium thriving at these salt levels. Hence, a comparative proteomic study was conducted on the supernatant of the bacterium after 42 h of growth, when Th17 production peaked in the control culture, as determined by Liquid Chromatography - Tandem Mass Spectrometry (LC-MS/MS). Optimal (salt free) bacterial culture served as a control and 200 and 500 mM NaCl as stress conditions. As salt levels increased, the major enzyme classes, transferases, hydrolases, lyases, and ligases showed increased abundance as compared to the control, mostly related to molecular function mechanisms. Some of the notable up-regulated proteins in 500 mM NaCl stress conditions included an S-layer protein, chitin binding domain 3 protein, enterotoxins, phosphopentomutase, glucose 6-phosphate isomerase and bacterial translation initiation factor; while notable down-regulated proteins included hemolytic enterotoxin, phospholipase, sphingomyelinase C, cold shock DNA-binding protein family and alcohol dehydrogenase. These results indicate that, as the salt stress levels increase, the bacterium probably shuts down the production of Th17 and regulates its molecular functional mechanisms to overcome stress. This study indicates that end users have the option of using Th17 as a biostimulant or the live bacterial inoculum depending on the soil salt characteristics, for crop production. The mass spectrometry proteomics data have been deposited to Mass Spectrometry Interactive Virtual Environment (MassIVE) with the dataset identifier PXD024069, and doi: 10.25345/C5RB8T.
Terrestrial plants have been co-evolving in symbiotic association with microbes for more than half a billion years (Knack et al., 2015). This association has been extensively studied with respect to the rhizosphere, which is a signal exchange center for both plants and microbial establishment (Desbrosses and Stougaard, 2011). Global agriculture is profoundly affected by overuse of fertilizers and harmful pesticides, and the growing adverse effects of climate change. In order to develop climate-resilient agriculture systems, appropriate microbes have been a sustainable resource as biopesticides and biofertilizers. Bacterial inoculum or isolated signal compounds from plant-associated microbes have been used in this context and newer compounds with new modes of action are constantly being recognized (Lyu et al., 2020).
Bacteriocins are small peptides secreted by most families of bacteria, either of plasmid or chromosomal origin synthesized at various stages of bacterial growth and under various environmental conditions (Daw and Falkiner, 1996). Bacillus species were first reported to produce bacteriocins in 1976 and the low-molecular-weight bacteriocins of the Gram-positive bacteria were reported to have bactericidal activity against certain closely related Gram-positive bacteria (Tagg et al., 1976). Based on their peptide characteristics, they are grouped into distinct classes and show substantial diversity in structure and function (Abriouel et al., 2011; Subramanian and Smith, 2015).
Bacillus thuringiensis NEB17, isolated from the rhizosphere of soybean plants at the Emile Lods Agronomy Research center of the Macdonald Campus, McGill University, produces a bacteriocin, thuricin17 (Th17), that has been purified and partially sequenced. Thuricin17 (Th17) was isolated from Bacillus thuringiensis NEB17 (Bt NEB17), a putative endophytic bacteria of soybean root nodules. It is a low molecular weight peptide of 3.162 kDa, and belongs to class IId of bacteriocins (Gray et al., 2006a,b; Lee et al., 2009). It has been reported to show functional similarities and anti-microbial activities with bacthuricin F4 (Jung et al., 2008a). The protein also has plant growth promoting activities and the bacteria when co-inoculated with Bradyrhizobium japonicum under nitrogen free conditions, improved soybean growth, nodulation and grain yield (Bai et al., 2002, 2003). Application of Th17 as a leaf spray and root drench, has positive effects on soybean and corn and stimulated plant growth. The leaves of 2-week-old soybean sprayed with Th17 showed increased activities of lignification-related and antioxidative enzymes and their isoforms suggesting that Th17 acts as an inducer of plant defense mechanisms (Jung et al., 2008a, 2011; Lee et al., 2009).
Recent research on Th17 has highlighted its plant growth promotion and abiotic stress alleviation properties. A. thaliana responded positively to treatment with Th17 in the presence of salt stress (up to 250 mM NaCl). At this level of salt, chloroplast proteins and proteins of photosystems I and II that are generally strongly and negatively affected by salt stress and PEP carboxylase, Rubisco-oxygenase large subunit, and pyruvate kinase, were some of the noteworthy proteins enhanced by Th17 application, along with other stress related proteins (Subramanian et al., 2016b). Canola cultivar 04C111 however did not respond favorably to Th17. Seeds treated with 10−11 M Th17 were negatively affected under high temperature stress under greenhouse conditions and the plants from this treatment produced fewer seeds than the untreated controls (Schwinghamer et al., 2016). Application of Th17, under water stress conditions, to 1-month-old soybean plants increased plant biomass by 17%, root biomass by 37% and root nodule biomass by 55%, and also the amount of abscisic acid in soybean roots by 30% (Prudent et al., 2014). Application of Th17 to soybean seeds (variety Absolute RR) caused accelerated seed germination under salt stress of up to 150 mM NaCl, with the best response seen at 100 mM NaCl. The up-regulation of PEP carboxylase and a marked down-regulation of α- and β-subunits of conglycinin, glycinin, as compared to the control treatment, is indicative of efficient storage protein utilization in conjunction with thioredoxin; and in organ maturation and transition from one stage to another in a plant's lifecycle (Subramanian et al., 2016a). While Th17 increased soybean seed germination at both 15 and 22°C, under field conditions, the lower concentration of 10−11 M showed increased plant height, number of trifoliate and nodules (Gautam et al., 2016). In the biofuel crop switchgrass, Th17 contributed to increase in plant height especially in the post-establishment years in the field (Arunachalam et al., 2018).
Our studies using Th17 in plant growth promotion, especially under salt stressed conditions, suggests that the bacteriocin is capable of alleviating NaCl stress in both Arabidopsis and soybean. Hence our interest in this part of the research was to study the response of the bacteria to NaCl stress and how that might affect the production of Th17 and other proteins in the cell-free supernatant. This attempt to understand the bacterial response to salt stress was also to allow one to decide between using live bacterial inoculum or Th17 in specific formulations for crop production.
Stock cultures of Bacillus thuringiensis NEB17 isolated from soybean rhizosphere, have been maintained in King's B medium as previously described (Gray et al., 2006a).
Bacillus thuringiensis NEB17 was grown overnight in King's B medium in an orbital shaker set to 28°C and 120 rpm (Forma Scientific Inc., Model 4580) and diluted to an OD600 nm of 0.01 corresponding to approximately 107 cfu mL−1. King's B medium supplemented with NaCl at 100 mM increments up to 1,000 mM was used to grow the bacteria in 96-well plates (Fisher, Canada) for 48 h. The bacterial growth was recorded every 2 h in a Cytation Gen 5 imaging reader (BioTek Instruments, Inc., USA) and a growth graph plotted as indicated by the OD600nm. The experiment was repeated three times to confirm the NaCl stress effects on bacterial growth kinetics.
Once the bacteria were assessed for their capacity to tolerate NaCl stress, they were grown in 100 mL King's B medium in 250 mL conical flasks under optimal and 100 mM NaCl stress increments up to 500 mM NaCl, in an orbital shaker set to 28°C and 120 rpm. The experiment was conducted three times with every treatment having two replicates per experiment. Samples of 1 mL were taken from the flasks, centrifuged at 10,000 rpm for 10 min to pellet the cells. The clear supernatant was centrifuged again and the top clear liquid was collected to quantify Th17 using HPLC (following Gray et al., 2006a) every 6 for 48 h. In brief, the analyses of Th17 were performed using a Waters HPLC system equipped with a Waters 2487 Dual λ Absorbance Detector, Waters 1525 Binary HPLC pump and Waters717plus Autosampler. The conditions of the chromatography were as follows: column—Vydac C18 reversed-phase column (0.46 × 25 cm; 5μ) (catalog no.218TP54; Vydac, Hesperia, CA, USA), temperature −25°C, flow rate −1 mL/min, detector wavelength-−214 nm, gradient from 18 to 95% acetonitrile during the 18 min run. The peak corresponding to Th17 was identified by comparing with the retention time of a purified Th17 standard.
Bacterial cultures at 42 h of growth were centrifuged at 10,000 rpm for 10 min. at room temperature to pellet the cells and collect the supernatant. The supernatant was further purified by removing any remaining cells with a 0.22 μm filter under vacuum and then subjecting it to TCA precipitation (25% v/v) at 4°C overnight in a shaker set to 100 rpm. These samples were centrifuged at 10,000 rpm for 10 min. at 4°C to obtain total protein precipitates as pellets. The TCA precipitation was carried out for the three independent experimental setups. Each experiment was comprised of three replicates per treatment and the TCA precipitated proteins were pooled to have enough protein for further steps of purification. The pellets were further treated with 1 mL ice cold methanol (Cat no. 15468–7, Sigma-Aldrich Co., St. Louis, MO, USA), vortexed, incubated in −20°C for 20 min. and centrifuged (Micro12, Fisher Scientific, Denver Instrument Co., USA) at 12,000 rpm for 7 min. at 4°C. The supernatant was discarded and the procedure was repeated twice, followed by a similar incubation in acetone (Cat. no. 179124, Sigma-Aldrich, Co., St. Louis, MO, USA); both steps were conducted in order to remove phenolics and secondary metabolites that might otherwise interfere with LC-MS/MS analysis. The total proteins were then dissolved in 2 M urea. The proteins were diluted and quantified using the Lowry method, and samples of 10 μg in 20 μL of 1M urea were submitted to the Institut de recherches cliniques de Montréal (IRCM) for label free proteomic analysis using LC-MS/MS.
The total proteins extracted were then digested with trypsin and subjected to LC-MS/MS using a Velos Orbitrap instrument (Thermo Fisher, MA, USA). Tandem mass spectra were extracted, and all MS/MS samples were analyzed using Mascot (Matrix Science, London, UK; version 2.3.02). Mascot was set up to search the Bacillus thuringiensis database assuming the digestion enzyme trypsin. Mascot searched with a fragment ion mass tolerance of 0.60 Da and a parent ion tolerance of 15 PPM. Carbamidomethyl of cysteine was specified in Mascot as a fixed modification. Oxidation of methionine was specified in Mascot as a variable modification.
Scaffold (version Scaffold 4.8.3), Proteome Software Inc., Portland, OR), was used to validate MS/MS-based peptide and protein identifications. Peptide identifications were accepted if they could be established at greater than 95.0% probability, as specified by the Peptide Prophet algorithm (Keller et al., 2002). Protein identifications were accepted if they could be established at greater than 95.0% probability and contained a minimum of two identified peptides. Protein probabilities were assigned by the Protein Prophet algorithm (Nesvizhskii et al., 2003).
Experiments were structured following a completely randomized design. The SAS statistical package 9.4 (SAS Institute Inc., Cary, NC, USA.) was utilized for data analysis. The Proc Mixed procedure and Tukey's multiple means comparison were used to determine differences among means at the 95% confidence level for bacterial growth and Th17 quantifications, at every time point.
Scaffold 4 was used to analyze the proteomics data for fold change and Fisher's exact test of the identified proteins, after subjecting the quantitative value of the spectra to the embedded normalization. The FASTA file generated was analyzed using Blast2GO-Pro 3.1.3 (Conesa et al., 2005; Götz et al., 2008, 2011), for the functional annotation and analysis of the protein sequences. Apart from these, Enzyme code (EC), KEGG maps and InterPro motifs were queried directly using the InterProScan web service. The mass spectrometry proteomics data have been deposited to Mass Spectrometry Interactive Virtual Environment (MassIVE), with the dataset identifier PXD024069 and doi: 10.25345/C5RB8T.
The bacteriocin thuricin17 has the ability to help plants withstand abiotic stressors such as salt (NaCl) and drought for both Arabidopsis (Subramanian et al., 2016b) and soybean (Prudent et al., 2014; Subramanian et al., 2016a). While the isolation of Th17 is rather straightforward, the use of the bacteria Bacillus thuringiensis NEB17 as the inoculum could also be a very useful method in agriculture systems. Hence the bacteria were subjected to salinity stress and the amount of thuricin17 quantified to formulate an application method.
Salt stress screening suggested that the bacteria were capable of growing very well under salt stress until 600 mM; the growth started to be affected from 700 mM declining drastically at 900 mM. The bacteria ceased to grow in 1,000 mM NaCl (Figure 1; Supplementary Table 1). This suggests that the Bacillus thuringiensis NEB17 is a salt tolerant strain.
Figure 1. Bacillus thuringiensis NEB17 growth under salt stress. Data represents the means and standard errors of bacterial growth in 96-well plates (n = 3, where every n = one 96-well plate with eight technical replicates per treatment).
Using a standard graph for Th17, the samples from optimal, 100–500 mM NaCl-stressed cultures were quantified for the capability of the bacteria to produce Th17 under salt stress. As the level of salt stress increased, the amount of Th17 decreased significantly (Figure 2). It was observed that in the 42 h culture, the amount of Th17 produced showed a steady decline in each of the NaCl stress levels and was statistically significant between every salt treatment (Supplementary Table 2). Hence, the 42 h cultures were further studied for the protein profiling to suggest differences in bacterial supernatant protein when the bacteria were subjected to 200 and 500 mM NaCl stress keeping the optimal conditions as control. Since Th17 sequence can only be partially characterized using trypsin, the LC/MS trypsin-based digestion was performed to understand the general bacterial physiology related to salt stress tolerance and the changes that reflect in the cell-free supernatant proteome.
Figure 2. HPLC quantification of thuricin17 under salt stress using pure thuricin17 as standard. The graph represents means and standard error of bacterial culture in 250 mL conical flask (n = 3, where every n = 2 technical replicates per treatment in every experiment).
The results from LC-MS was analyzed using Scaffold and Blast2GoPro (Supplementary Material 1). The total proteins identified and the associated spectra are mentioned in Table 1; and the differences in the protein profile, total unique peptides and spectra are represented as Venn diagrams (Figure 3). Overall, in comparison with bacteria grown under optimal conditions, cultures in 200 mM NaCl showed an increase in response to stress, regulation of cellular, catabolic, metabolic and biosynthetic processes (Figure 4A). In 500 mM NaCl-stressed culture, response to stress, intracellular organelle parts and organelles, ribonucleoprotein complexes and non-membrane bound organelles were negatively affected. At both the salt levels, increased enzyme activities of ligases, hydrolases, lyases, and transferases were observed that profoundly affected the molecular function responses (Figure 4B; Table 2).
Table 1. Total number of proteins identified at 95% protein probability and total spectra at 95% peptide probability, with minimum of two peptides.
Figure 3. Venn diagram for total proteins, total unique peptides and total unique spectra of Bacillus thuringiensis NEB17 cell-free supernatant proteomics (n = 3, where every n = a pool of three technical replicate of 100 mL culture per treatment).
Figure 4. (A) Representation of functional classification of proteins based on GO function distribution for biological process and molecular function in control and NaCl stressed bacterial culture. (B) Representation of functional classification of proteins based on GO function distribution for cellular component and enzyme code distribution.
Table 2. Enzyme code distribution differences as compared to control in the cell-free supernatant of Bacillus thuringiensis NEB17 under salt stress.
Further analysis, such as the Fisher test and fold change between contrasts 1 control and 200 mM, 2 control and 500 mM, and 3 200 and 500 mM NaCl were performed to compare the protein responses affected in the bacteria. Based on Fishers test (Supplementary Material 2), the contrast of bacterial control with 200 mM NaCl saw an increase in proteins such as two isoforms of cold-shock DNA-binding protein family, Hbl L2 protein, collagenase and pyruvate dehydrogenase complex E2 component. In contrast control vs. 500 mM NaCl, a 3D domain protein, elongation factor G, 2-oxoisovalerate dehydrogenase beta subunit, phosphopentomutase, dihydrolipoyl dehydrogenase, S-layer protein, ornithine carbamoyltransferase, collagenase isoforms and a conserved hypothetical protein were up-regulated and statistically significant. The hemolytic enterotoxin, an cold-shock DNA-binding protein isoform, glycerol phosphate lipotechoic acid synthase, sphingomyelinase C, alveolysin, extra-cellular solute binding protein family 5, sulfatase and a universal stress protein were down-regulated. In contrast, 200 vs. 500 mM NaCl salt stress, except for a 3D domain protein, isoforms of cold-shock DNA-binding, hemolytic enterotoxin, a 50S ribosomal protein L15, phospholipase C, sphingomyelinase C, alveolysin and LSU ribosomal protein L16P were all down-regulated in 500 mM NaCl-stressed supernatant. This indicates that as the salt stress level increases, some of the bacterial pathogenicity factors are being affected.
Protein data (quantitative spectra) of two or more-fold change was considered for biological interpretation (Supplementary Material 3) along with those identified with Fishers test. In contrast 1. control vs. 200 mM and 2. control vs. 500 mM, 59 proteins each and in contrast 3. 200 vs. 500 mM, 26 proteins that were above two-fold change were identified. In contrast 1. the two isoforms of cold-shock DNA-binding protein family had fold changes of 12.8 and 14.7, as compared to control. In contrast 2, dihydrolipoyl dehydrogenase, phosphopentomutase, two isoforms of 3D domain protein, 2-oxoisovalerate dehydrogenase beta subunit, elongation factor G and ornithine carbamoyltransferase were detected with more than a two-fold increase. Contrast 3 however had only the 3D domain protein with 2.3-fold change as compared to 200 mM NaCl, in keeping with the Fishers test data (Table 3).
Since thuricin17 was first isolated and characterized, its potential use in agriculture has been tested on a range of plants as a plant growth promoter. In our studies, spanning over a decade, Th17 as a leaf spray on soybean, or as a root drench in soybean and corn (Jung et al., 2008a; Lee et al., 2009), in association with abiotic stress for Arabidopsis (Subramanian et al., 2016b), soybean (Prudent et al., 2014; Subramanian et al., 2016a) and under field conditions for soybean (Gautam et al., 2016) and switchgrass (Arunachalam et al., 2018) have demonstrated positive plant growth promotion and yield. Bt NEB17 when co-inoculated with Bradyrhizobium japonicum 532C under nitrogen free conditions, promoted soybean plant growth, nodulation, and yield (Bai et al., 2002). But, so far Bt NEB17 has not been evaluated for its capability to tolerate any stress or to determine if Th17 production is affected if the bacterial culture is subjected to any stress. Hence, in this study, Bt NEB17 was subjected to NaCl stress and the cell-free supernatant was evaluated to study the commonness or differences the bacteria undergo during their first 48 h of growth. Also, Th17 was quantified to determine if a stress environment caused an increase or decrease in its production. While the bacteria grew well up to 600 mM NaCl and the effect of salt stress started to be manifested from 700 mM NaCl, the production of Th17 started to decline from 100 mM NaCl and was half the amount at 200 mM NaCl. The amount declined further and by 500 mM NaCl, the production almost stopped. In all probability, the bacteria were utilizing their resources to deal with and thrive under the salt stress rather than invest energy in Th17 production. Also, because in a closed environment like a conical flask where they do not need to ward off closely related Bacilli for rhizhospheric competition (Jung et al., 2008b), their resources could be allocated more for their survival. Bacterial populations display growth and colony size heterogeneity as a mechanism to adapt to stress. In this study, as the level of salt increased, the cell density of the cultures decreased, particularly at very high NaCl concentrations. In previous studies, Bacillus cereus ATCC 14579 displayed a low population variability under mild salt stress (2.5% NaCl stress) and the colony sizes were highly heterogenous under severe salt stress (5% NaCl stress) which affected the cell density in liquid cultures (Den Besten et al., 2007). Whole genome expression analyses suggest an overlap of salt stress gene expression, in both these conditions, that involved genes that are part of general stress responses (Den Besten et al., 2009).
Proteins and secondary metabolites play key roles in metabolic processes of an organism. They also play key roles in the rhizosphere, enriching and establishing beneficial partners. From a bacterial point of view, nutrient cycling, degradation of root exudates for enriching and attracting beneficial partners to their niche, etc., all have intricate evolutionary patterns. The label free proteomics approach to determine what the bacteria changed to accommodate to NaCl stress is interesting to consider.
Among the Bacillus cereus sensu lato group, survival in salt stress is particularly relevant to the food industry since salt is added as an additive and/or preservative. Cross protection between salt, heat, hydrogen peroxide, acid, and ethanol is established in Bacillus sps, in the context of food processing, where some of these are regular food contaminants. For example, Bacillus cereus NCIMB 11796 produced both general and stress specific (salt, heat) proteins that were accumulated differentially between stressed and unstressed cells (Browne and Dowds, 2001). The Bacillus group manifests a group of proteins that have unspecific but protective function regardless of stress exposure, and these are referred to as general stress proteins (GSPs). Many of these general stress proteins are seen at the onset and during the stationary phase. A significant amount of work has been done with B. cereus and model bacterium B. subtilis with respect to the cellular responses to stress. For example, Bacillus subtilis strains IS58 and 168 (trpC2) were reported to have about 50 such GSPs and about 40% of the cells translational capacity was devoted to producing them. Also, the σB-dependent stress response varied in intensity, between the strains and the mutants in this study (Bernhardt et al., 1997). A comprehensive microarray and proteomics of Bacillus subtilis 168 (trpC2) wild-type strain was studied for very early (10–120 min. after exposure to NaCl stress) transcriptome and proteome changes in response to salt stress (6% NaCl). A sudden change in osmolarity caused the bacterial membrane proteins to increase by 20% in the first 10 min. of exposure. The immediate response was the activation of σB regulon that confers broad stress resistance to the cells (Hahne et al., 2010).
However, from an agriculture perspective, salt stress is detrimental to both crop production and to the microorganismal populations in soils. Studies at high salt stress levels was reported to favor the transfer of plasmid pAW63 from Bacillus thuringiensis subsp. kurstaki to other Bt strains. While the salt tolerance in Bt varies between strains, some strains alter their phenotype by modifying their mobility, reductions in cell number, cell clumping, and formation of filaments (Beuls et al., 2012). A study of Bt in rice fields suggests that the plasmids of various strains studied did not have any correlation with crystal morphology or salt tolerance of the strains (Das and Dangar, 2007). Since Bt NEB17 was isolated from Macdonald campus soil, Quebec, it is highly likely that the bacterium is a psychrotropic and a psychrotolerant strain. For cold adaptation, Bt strains use major cold-shock proteins (Csps) to survive and grow under low temperature (Francis et al., 1998). Natural cold adapted isolates of Bacillus thuringiensis have various combinations of nhe, hblA, and cytK genes that are toxigenic by nature and cspA that confer psychrotolerance to the bacteria (Bartoszewicz et al., 2009). While Csps are mostly associated with cold stress adaptation, they are also reported to be present during the early exponential phase of cell differentiation and proliferation under optimal conditions, and their levels are non-detectable in the late exponential phase. Culture dilutions and additional nutrient availability also play important roles in Csp induction (Brandi et al., 1999; Yamanaka and Inouye, 2001; Ermolenko and Makhatadze, 2002). Some members of the Csp family in Bacillus subtilis are reported to be involved in several cellular processes (Graumann et al., 1997), and general stress adaptation responses such as osmotic, oxidative, pH, starvation, ethanol, and host cell invasion (Keto-Timonen et al., 2016) and can hence be termed multi-functional (Lindquist and Mertens, 2018). In this study, two isoforms of cold-shock DNA-binding proteins (7 kDa) were seen to be up-regulated at 200 mM NaCl stress but down-regulated at 500 mM NaCl stress. As in cold temperature, these protein isofoms also help when the bacteria are affected by salt stress and probably function as general stress response proteins.
While insecticidal toxins are wide spread in Bt, most of these are seen in isolates from invertebrate samples as compared to water, soil, or plant samples (Espinasse et al., 2003). This suggests an evolutionary significance in the bacterial colonization dependent on the ecological niche they have evolved in. Repeated culturing of Bt in rich culture medium can alter some genetic, metabolic, pathogenic, and structural determinants over time, as compared to fresh isolates from plant tissues. Some of these changes include complete loss of δ-endotoxins and β-exotoxin production, changes in fatty acid profiles and mutation of cry genes, thereby altering the virulence determinants (Bizzarri et al., 2008). Of the several enterotoxins identified in Bt, two of the most important that contribute to food poisoning are hemolytic (Hbl) and non-haemolytic (Nhe) toxins. While these toxins were studied more in B. cereus, it is now evident that they are more prevalent in Bt populations (Gaviria Rivera et al., 2000) as are other associated toxins (Kim et al., 2015).
HBL is the best characterized three-component hemolysin enterotoxin. It is comprised of two lytic components L1 and L2 and a binding component B all of which are involved in hemolysis, vascular permeability and dermal damage (dermonecrotic). Many Bt strains do not contain L2 and B components but can still be cytotoxic when they cross react with Nhe (Prüß et al., 1999). The SinR regulon harbors genes coding for Hbl enterotoxin. This along with the main virulence regulator PlcR, controls Hbl expression (Fagerlund et al., 2014). In this study, the Hbl L2 protein component was not affected by 200 mM NaCl. But the hemolytic enterotoxin was significantly and negatively affected at 500 mM NaCl. Also, phospholipase C was down-regulated at 500 mM NaCl. PlcR regulator controls the transcription of genes encoding extracellular proteins including phospholipase C, proteases and enterotoxins (Hbl and Nhe). The plcR gene is activated during the onset of the stationary phase and acts as a quorum sensing effector. The disruption of this gene greatly reduces the haemolytic activity of Bt and thereby insecticidal virulence (Gominet et al., 2001).
Likewise, sphingomyelinase C and alveolysin were affected by 500 mM NaCl. Cereolysin B, or sphingomyelinase (cerB), is also controlled by plcR and is involved in pathogenesis (Kim et al., 2015). Alveolysin is a thiol-activated toxin first identified in Bacillus alvei that colonizes bee-hives. This protein shares homology with other toxins such as listeriolysin O, perfringolysin O, pneumolysin, and streptolysin O, all of which have a single cysteine in their conserved peptide sequence ECTGLAWEWWR (Geoffroy et al., 1990). It is an exoprotein that has a lethal membrane damaging and cardiotoxic nature when it binds to membrane cholesterol and withdraws its interaction with other membrane lipids (Thelestam et al., 1981). It is possible that Bt NEB17 being a rhizospheric bacteria uses this toxin as a defense to prevent other Bacilli from colonizing the same niche space and this capability is lost under salt stress.
In this study, the effect of NaCl on bacterial growth, production of the bacteriocin thuricin17 and characteristics of the bacterial culture cell-free supernatant were determined, to study the changes in bacterial physiology in response to salt stress. The comparison of two levels of salt stress is another step in understanding the mechanism of the bacterial tolerance to salt and how they manipulate their external environment in order to maintain a healthy population under adverse conditions. Salt stress adversely affects the bacterial defense mechanisms but does not affect growth until the NaCl levels are 700 mM or greater. This study is another step to understanding bacterial growth under salt stress that can help the end user use either the live inoculum or Th17 as a biostimulant, for crop production.
The datasets presented in this study can be found in online repositories. The names of the repository/repositories and accession number(s) can be found below: CCMS MassIVE, Accession No: MSV000086823.
SS designed, performed experiments, data analysis, and wrote the manuscript. AS contributed to HPLC quantification of thuricin17. AS and DS contributed to procuring reagents/materials/analysis tools/funding. DS provided the conceptual context for the work. All authors contributed to the article and approved the submitted version.
The authors acknowledge funding to support this work supported through funds from the Natural Sciences and Engineering Research Council of Canada.
The authors declare that the research was conducted in the absence of any commercial or financial relationships that could be construed as a potential conflict of interest.
The authors would like to thank Natural Sciences and Engineering Research Council of Canada (NSERC), Green Crop Network for funding, Dr. Denis Fauberts' group from Institute de recherches cliniques de Montréal (IRCM) for label free LC-MS/MS proteomics service, and Jeremy Carver from The Center for Computational Mass Spectrometry (CCMS), University of California, San Diego (UCSD) for all help in data submission on Mass Spectrometry Interactive Virtual Environment (MassIVE).
The Supplementary Material for this article can be found online at: https://www.frontiersin.org/articles/10.3389/fsufs.2021.630628/full#supplementary-material
Supplementary Table 1. Bacterial growth under salt stress data with statistical analysis.
Supplementary Table 2. Thuricin17 quantification under salt stress data with statistical analysis.
Supplementary Material 1. Protein data Blast2GoPro for all the treatment replicates of Bt NEB17 and under salt stress.
Supplementary Material 2. Fishers test data between contrasts of Bt NEB17.
Supplementary Material 3. Fold change data between contrasts of Bt NEB17.
Abriouel, H., Franz, C. M., Omar, N. B., and Gálvez, A. (2011). Diversity and applications of Bacillus bacteriocins. FEMS Microbiol. Rev. 35, 201–232. doi: 10.1111/j.1574-6976.2010.00244.x
Arunachalam, S., Schwinghamer, T., Dutilleul, P., and Smith, D. L. (2018). Multi-year effects of biochar, lipo-chitooligosaccharide, thuricin 17, and experimental bio-fertilizer for switchgrass. Agron. J. 110, 77–84. doi: 10.2134/agronj2017.05.0278
Bai, Y., D'Aoust, F., Smith, D. L., and Driscoll, B. T. (2002). Isolation of plant-growth-promoting Bacillus strains from soybean root nodules. Can. J. Microbiol. 48, 230–238. doi: 10.1139/w02-014
Bai, Y., Zhou, X., and Smith, D. L. (2003). Enhanced soybean plant growth resulting from coinoculation of Bacillus strains with Bradyrhizobium japonicum. Crop Sci. 43, 1774–1781. doi: 10.2135/cropsci2003.1774
Bartoszewicz, M., Bideshi, D. K., Kraszewska, A., Modzelewska, E., and Swiecicka, I. (2009). Natural isolates of Bacillus thuringiensis display genetic and psychrotrophic properties characteristic of Bacillus weihenstephanensis. J. Appl. Microbiol. 106, 1967–1975. doi: 10.1111/j.1365-2672.2009.04166.x
Bernhardt, J., Vslker, U., Vslker, A., Antelmann, H., Schmid, R., Mach, H., et al. (1997). Specific and general stress proteins in Bacillus subtilis - a two-dimensional protein electrophoresis study. Microbiology 143, 999–1017.
Beuls, E., Modrie, P., Deserranno, C., and Mahillon, J. (2012). High-salt stress conditions increase the pAW63 transfer frequency in Bacillus thuringiensis. Appl. Environ. Microbiol. 78, 7128–7131. doi: 10.1128/AEM.01105-12
Bizzarri, M. F., Bishop, A. H., Dinsdale, A., and Logan, N. A. (2008). Changes in the properties of Bacillus thuringiensis after prolonged culture in a rich medium. J. Appl. Microbiol. 104, 60–69. doi: 10.1111/j.1365-2672.2007.03537.x
Brandi, A., Spurio, R., Gualerzi, C. O., and Pon, C. L. (1999). Massive presence of the Escherichia coli ‘major cold-shock protein’ CspA under non-stress conditions. EMBO J. 18, 1653–1659. doi: 10.1093/emboj/18.6.1653
Browne, N., and Dowds, B. C. A. (2001). Heat and salt stress in the food pathogen Bacillus cereus. J. Appl. Microbiol. 91, 1085–1094. doi: 10.1046/j.1365-2672.2001.01478.x
Conesa, A., Götz, S., Garcia-Gomez, M., Terol, J., Talon, M., and Robles, M. (2005). Blast2GO: a universal tool for annotation, visualization and analysis in functional genomics research. Bioinformatics 21, 3674–3676. doi: 10.1093/bioinformatics/bti610
Das, J., and Dangar, T. K. (2007). Diversity of Bacillus thuringiensis in the rice field soils of different ecologies in India. Indian J. Microbiol. 47, 364–368. doi: 10.1007/s12088-007-0065-z
Daw, M. A., and Falkiner, F. R. (1996). Bacteriocins: nature, function and structure. Micron 27, 467–479. doi: 10.1016/S0968-4328(96)00028-5
Den Besten, H. M. W., Ingham, C. J., Van Hylckama Vlieg, J. E. T., Beerthuyzen, M. M., Zwietering, M. H., and Abee, T. (2007). Quantitative analysis of population heterogeneity of the adaptive salt stress response and growth capacity of Bacillus cereus ATCC 14579. Appl. Environ. Microbiol. 73, 4797–4804. doi: 10.1128/AEM.00404-07
Den Besten, H. M. W., Mols, M., Moezelaar, R., Zwietering, M. H., and Abee, T. (2009). Phenotypic and transcriptomic analyses of mildly and severely salt-stressed Bacillus cereus ATCC 14579 cells. Appl. Environ. Microbiol. 75, 4111–4119. doi: 10.1128/AEM.02891-08
Desbrosses, G. J., and Stougaard, J. (2011). Root nodulation: a paradigm for how plant-microbe symbiosis influences host developmental pathways. Cell. Host. Microbe 10, 348–358. doi: 10.1016/j.chom.2011.09.005
Ermolenko, D. N., and Makhatadze, G. I. (2002). Cellular and molecular life sciences bacterial cold-shock proteins. Structure 59, 1902–1913. doi: 10.1007/PL00012513
Espinasse, S., Chaufaux, J., Buisson, C., Perchat, S., Gohar, M., Bourguet, D., et al. (2003). Occurrence and linkage between secreted insecticidal toxins in natural isolates of Bacillus thuringiensis. Curr. Microbiol. 47, 501–507. doi: 10.1007/s00284-003-4097-2
Fagerlund, A., Dubois, T., Økstad, O. A., Verplaetse, E., Gilois, N., Bennaceur, I., et al. (2014). SinR controls enterotoxin expression in Bacillus thuringiensis biofilms. PLoS ONE 9:0087532. doi: 10.1371/journal.pone.0087532
Francis, K. P., Mayr, R., Von Stetten, F., Stewart, G. S. A. B., and Scherer, S. (1998). Discrimination of psychrotrophic and mesophilic strains of the Bacillus cereus group by PCR targeting of major cold shock protein genes. Appl. Environ. Microbiol. 64, 3525–3529. doi: 10.1128/AEM.64.9.3525-3529.1998
Gautam, K., Schwinghamer, T. D., and Smith, D. L. (2016). The response of soybean to nod factors and a bacteriocin. Plant Signal. Behav. 11:e1241934. doi: 10.1080/15592324.2016.1241934
Gaviria Rivera, A. M., Granum, P. E., and Priest, F. G. (2000). Common occurrence of enterotoxin genes and enterotoxicity in Bacillus thuringiensis. FEMS Microbiol. Lett. 190, 151–155. doi: 10.1111/j.1574-6968.2000.tb09278.x
Geoffroy, C., Mengaud, J., Alouf, J. E., and Cossart, P. (1990). Alveolysin, the thiol-activated toxin of Bacillus alvei, is homologous to listeriolysin O, perfringolysin O, pneumolysin, and streptolysin O and contains a single cysteine. J. Bacteriol. 172, 7301–7305. doi: 10.1128/JB.172.12.7301-7305.1990
Gominet, M., Slamti, L., Gilois, N., Rose, M., and Lereclus, D. (2001). Oligopeptide permease is required for expression of the Bacillus thuringiensis plcR regulon and for virulence. Mol. Microbiol. 40, 963–975. doi: 10.1046/j.1365-2958.2001.02440.x
Götz, S., Arnold, R., Sebastián-León, P., Martín-Rodríguez, S., Tischler, P., Jehl, M. A., et al. (2011). B2G-FAR, a species centered GO annotation repository. Bioinformatics 27, 919–924. doi: 10.1093/bioinformatics/btr059
Götz, S., García-Gómez, J. M., Terol, J., Williams, T. D., Nagaraj, S. H., Nueda, M. J., et al. (2008). High-throughput functional annotation and data mining with the Blast2GOsuite. Nucleic Acids Res. 36, 3420–3435. doi: 10.1093/nar/gkn176
Graumann, P., Wendrich, T. M., Weber, M. H. W., Schröder, K., and Marahiel, M. A. (1997). A family of cold shock proteins in Bacillus subtilis is essential for cellular growth and for efficient protein synthesis at optimal and low temperatures. Mol. Microbiol. 25, 741–756. doi: 10.1046/j.1365-2958.1997.5121878.x
Gray, E. J., Di Falco, M. R., Souleimanov, A., and Smith, D. L. (2006b). Proteomic analysis of the bacteriocin thuricin 17 produced by Bacillus thuringiensis NEB17. FEMS Microbiol. Lett. 255, 27–32. doi: 10.1111/j.1574-6968.2005.00054.x
Gray, E. J., Lee, K. D., Souleimanov, A. M., Di Falco, M. R., Zhou, X., Ly, A., et al. (2006a). A novel bacteriocin, thuricin 17, produced by plant growth promoting rhizobacteria strain Bacillus thuringiensis NEB17: isolation and classification. J. Appl. Microbiol. 100, 545–554. doi: 10.1111/j.1365-2672.2006.02822.x
Hahne, H., Mäder, U., Otto, A., Bonn, F., Steil, L., Bremer, E., et al. (2010). A comprehensive proteomics and transcriptomics analysis of Bacillus subtilis salt stress adaptation. J. Bacteriol. 192, 870–882. doi: 10.1128/JB.01106-09
Jung, W.-J., Mabood, F., Souleimanov, A., and Smith, D. L. (2008a). Effect of chitin hexamer and thuricin 17 on lignification-related and antioxidative enzymes on soybean plants. J. Plant Biol. 51, 145–149. doi: 10.1007/BF03030724
Jung, W. J., Mabood, F., Souleimanov, A., and Smith, D. L. (2011). Induction of defense-related enzymes in soybean leaves by class IId bacteriocins (thuricin 17 and bacthuricin F4) purified from Bacillus Strains. Microbiol. Res. 167, 14–19. doi: 10.1016/j.micres.2011.02.004
Jung, W. J., Mabood, M., Souleimanov, A., Zhou, X., Jaoua, S., Kamoun, F., et al. (2008b). Stability and antibacterial activity of bacteriocins produced by Bacillus thuringiensis and Bacillus thuringiensis ssp. kurstaki. J. Microbiol. Biotechnol. 18, 1836–1840. doi: 10.4014/jmb.0800.120
Keller, A., Nesvizhskii, A. I., Kolker, E., and Aebersold, R. (2002). Empirical statistical model to estimate the accuracy of peptide identifications made by MS/MS and data base search. Anal. Chem. 74, 5383–5392. doi: 10.1021/ac025747h
Keto-Timonen, R., Hietala, N., Palonen, E., Hakakorpi, A., Lindström, M., and Korkeala, H. (2016). Cold Shock proteins: a minireview with special emphasis on Csp-family of enteropathogenic Yersinia. Front. Microbiol. 7, 1–7. doi: 10.3389/fmicb.2016.01151
Kim, M. J., Han, J. K., Park, J. S., Lee, J. S., Lee, S. H., Cho, J., et al. (2015). Various enterotoxin and other virulence factor genes widespread among Bacillus cereus and Bacillus thuringiensis strains. J. Microbiol. Biotechnol. 25, 872–879. doi: 10.4014/jmb.1502.02003
Knack, J. J., Wilcox, L. W., Delaux, P.-M., Ané, J.-M., Piotrowski, M. J., Cook, M. E., et al. (2015). Microbiomes of streptophyte algae and bryophytes suggest that a functional suite of microbiota fostered plant colonization of land. Int. J. Plant Sci. 176, 405–420. doi: 10.1086/681161
Lee, K. D., Gray, E. J., Mabood, M., Jung, W. J., Charles, T., Clark, R. D. S., et al. (2009). The class IId bacteriocin thuricin-17 increases plant growth. Planta 229, 747–755. doi: 10.1007/s00425-008-0870-6
Lindquist, J. A., and Mertens, P. R. (2018). Cold shock proteins: from cellular mechanisms to pathophysiology and disease. Cell Commun. Signal. 16, 1–14. doi: 10.1186/s12964-018-0274-6
Lyu, D., Backer, R., Lyu, D., Backer, R., Subramanian, S., and Smith, D. L. (2020). Phytomicrobiome coordination signals hold potential for climate change-resilient agriculture. Front. Plant Sci. 1:634. doi: 10.3389/fpls.2020.00634
Nesvizhskii, A. I., Keller, A., Kolker, E., and Aebersold, R. (2003). A statistical model for identifying proteins by tandem mass spectrometry. Anal. Chem. 75, 4646–4658. doi: 10.1021/ac0341261
Prudent, M., Salon, C., Souleimanov, A., Emery, R. J. N., and Smith, D. L. (2014). Soybean is less impacted by water stress using Bradyrhizobium japonicum and thuricin-17 from Bacillus thuringiensis. Agron. Sustain. Dev. 35, 749–757. doi: 10.1007/s13593-014-0256-z
Prüß, B. M., Dietrich, R., Nibler, B., Märtlbauer, E., and Scherer, S. (1999). The hemolytic enterotoxin HBL is broadly distributed among species of the Bacillus cereus group. Appl. Environ. Microbiol. 65, 5436–5442. doi: 10.1128/AEM.65.12.5436-5442.1999
Schwinghamer, T., Souleimanov, A., Dutilleul, P., and Smith, D. L. (2016). The response of canola cultivars to lipo-chitooligosaccharide (Nod Bj V [C18:1, MeFuc]) and thuricin 17.” Plant Growth Regul. 78, 421–434. doi: 10.1007/s10725-015-0104-4
Subramanian, S., Ricci, E., Souleimanov, A., and Smith, D. L. (2016a). A proteomic approach to lipo-chitooligosaccharide and thuricin 17 effects on soybean germination unstressed and salt stress. PLoS ONE 11:160660. doi: 10.1371/journal.pone.0160660
Subramanian, S., and Smith, D. L. (2015). Bacteriocins from the rhizosphere microbiome - from an agriculture perspective. Front. Plant Sci. 6:909. doi: 10.3389/fpls.2015.00909
Subramanian, S., Souleimanov, A., and Smith, D. L. (2016b). Proteomic studies on the effects of lipo-chitooligosaccharide and thuricin 17 under unstressed and salt stressed conditions in Arabidopsis Thaliana. Front. Plant Sci. 7:1314. doi: 10.3389/fpls.2016.01314
Tagg, J., Daiani, A., and Wannamaker, L. (1976). Bacteriocins of gram-positive bacteria. Bacteriol. Rev. 40, 722–756.
Thelestam, M., Alouf, J. E., Geoffroy, C., and Mollby, R. (1981). Membrane-damaging action of alveolysin from Bacillus alvei. Infect. Immun. 32, 1187–1192. doi: 10.1128/IAI.32.3.1187-1192.1981
Keywords: Bacillus thuringiensis NEB17, Thuricin17, bacteriocin, NaCl stress, proteins
Citation: Subramanian S, Souleimanov A and Smith DL (2021) Thuricin17 Production and Proteome Differences in Bacillus thuringiensis NEB17 Cell-Free Supernatant Under NaCl Stress. Front. Sustain. Food Syst. 5:630628. doi: 10.3389/fsufs.2021.630628
Received: 18 November 2020; Accepted: 18 February 2021;
Published: 11 March 2021.
Edited by:
Saveetha Kandasamy, A&L Canada Laboratories, CanadaReviewed by:
Norma Margarita De La Fuente Salcido, Universidad Autónoma de Coahuila, MexicoCopyright © 2021 Subramanian, Souleimanov and Smith. This is an open-access article distributed under the terms of the Creative Commons Attribution License (CC BY). The use, distribution or reproduction in other forums is permitted, provided the original author(s) and the copyright owner(s) are credited and that the original publication in this journal is cited, in accordance with accepted academic practice. No use, distribution or reproduction is permitted which does not comply with these terms.
*Correspondence: Donald L. Smith, ZG9uYWxkLnNtaXRoQG1jZ2lsbC5jYQ==
Disclaimer: All claims expressed in this article are solely those of the authors and do not necessarily represent those of their affiliated organizations, or those of the publisher, the editors and the reviewers. Any product that may be evaluated in this article or claim that may be made by its manufacturer is not guaranteed or endorsed by the publisher.
Research integrity at Frontiers
Learn more about the work of our research integrity team to safeguard the quality of each article we publish.