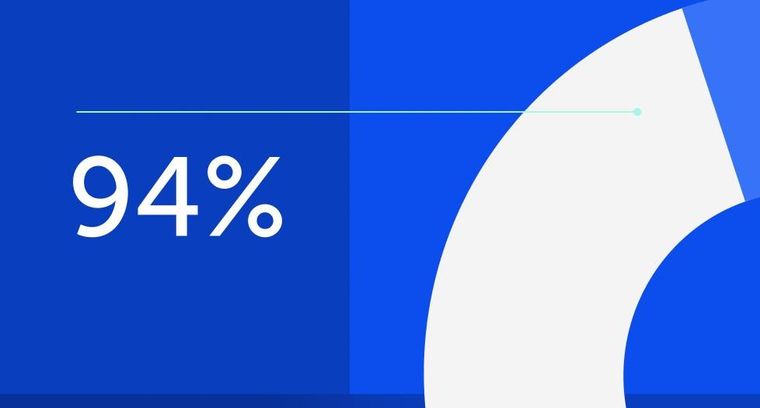
94% of researchers rate our articles as excellent or good
Learn more about the work of our research integrity team to safeguard the quality of each article we publish.
Find out more
REVIEW article
Front. Sustain. Food Syst., 29 April 2021
Sec. Sustainable Food Processing
Volume 5 - 2021 | https://doi.org/10.3389/fsufs.2021.630393
This article is part of the Research TopicSustainability and Efficiency of Food Packaging: from Food Preservation to SaleView all 7 articles
Food packaging has a crucial function in the modern food industry. New food packaging technologies seek to meet consumers and industrial's demands. Changes related to food production, sale practices and consumers' lifestyles, along with environmental awareness and the advance in new areas of knowledge (such as nanotechnology or biotechnology), act as driving forces to develop smart packages that can extend food shelf-life, keeping and supervising their innocuousness and quality and also taking care of the environment. This review describes the main concepts and types of active and intelligent food packaging, focusing on recent progress and new trends using biodegradable and biobased polymers. Numerous studies show the great possibilities of these materials. Future research needs to focus on some important aspects such as possibilities to scale-up, costs, regulatory aspects, and consumers' acceptance, to make these systems commercially viable.
Food packaging has a crucial function in modern food industry, since it contributes to preserve food products quality and guarantee food safety during its shelf-life (Ghaani et al., 2016). Traditional food packaging has four basic functions: protection and preservation, containment, communication and marketing, and convenience (Biji et al., 2015). Packages are used to protect the product from spoilage and damage brought about by environmental factors, such as microbes, insects, light, heat, oxygen, water vapor, smells, dirt, dust, etc. (Fuertes et al., 2016a). They can take different shapes and sizes to hold food products, with the aim of improving logistical efficiency (Yam and Lee, 2012). They communicate with the consumer by means of written texts (the ingredients list, nutritional facts, preparation instructions, etc.) and the brand logo. They adapt to consumer's lifestyle, for example, saving time (suitable for ready-to-eat meals) or facilitating their handling (easy to open, reclosable or suitable for microwave) (Vanderroost et al., 2014). Figure 1 resumes these functions, that traditionally, these have been fulfilled minimizing the interaction between food and package (Lee et al., 2015).
For about five decades, plastic packages have been widely used by the food industry due to their advantageous characteristics. They are economical, functional, light and very versatile, since they can be rigid (bottles, jars, boxes, cases), thermoformed (food trays) or flexible (woven mesh, multi-layer, films). Therefore, they have replaced other traditional materials such as glass, metals (aluminum, laminated, tinplate and steel), paper and cardboard, until representing recently 37% of food packaging materials (Food Packaging Forum, 2015). This massive use has caused serious environmental problems worldwide, because most of these materials derive from petroleum, are non-degradable and during their manufacturing and disposal they pollute the environment. The development of new eco-friendly packages along with innovative packaging concepts is changing the market. The use of biodegradable and renewable materials represents a great alternative to protect the environment and give economic value to underutilized products or industrial waste materials (Cazón et al., 2017). In this sense, bioplastics have begun to gain prominence. According to the European Bioplastics Organization, they are defined as plastic materials that are either biobased (partly or entirely) or biodegradable, or feature both properties. “Biobased” means that the material or product is derived from biomass and “biodegradable” means that it can be biological degraded down to base substances such as water, carbon dioxide, methane, basic elements, and biomass by living organisms that are available in the environment (Goswami and O'Haire, 2016). Thus, bioplastics can be classified into three main groups (Figure 2): (i) biobased but non-biodegradable plastics such as bio-polyethylene (Bio-PE), bio-polyamide (Bio-PA), bio-polyethylene terephthalate (Bio-PET), bio-polytrimethylene terephthalate (Bio-PTT), bio-polyurethanes (Bio-PU), biopolypropylene (Bio-PP); (ii) plastics that are biodegradable and based on fossil resources, such as poly(butylene adipate-co-terephthalate) (PBAT), poly(butylene succinate-co-butylene adipate) (PBSA), polyvinyl alcohol (PVA), polyglycolic acid (PGA), polycaprolactone (PCL); and (iii) plastics that are both biobased and biodegradable. This last group include: polymers directly extracted from biomass such as polysaccharides (e.g., starch, cellulose, chitin, etc.) and proteins (e.g., collagen, gelatin, casein, whey, soy protein, zein, wheat gluten, etc.); whose further modification can produce additional valuable biobased materials such as cellulose acetate, cellulose acetate butyrate, cellulose acetate propionate, cellulose nitrate, regenerated cellulose, carboxymethyl cellulose, lignocellulosic products, chitosan, etc.); polymers produced by chemical synthesis using renewable biobased monomers, such as polylactic acid (PLA), a bio-polyester polymerized from lactic acid monomers produced by fermentation of carbohydrate feedstock; and polymers produced by microorganisms or genetically modified bacteria such as polyhydroxyalkonoates (PHA) like polyhydroxybutyrate (PHB) and polyhydroxyvalerate (PHV) and bacterial cellulose (Petersen et al., 1999). Biobased and biodegradable plastics heads for a circular economy and sustainability (Bio-based News—www.news.bio-based.eu). Figure 3 shows the life cycle assessment (LCA) of the three types of bioplastics mentioned before. Biobased plastics, which are generally drop-in products for their petroleum-based counterparts, are adequate for material recycling and/or energy recovery, whereas biodegradable plastics are intended for organic recycling. These last ones are specially promising when plastic products withhold food, as in those cases material recycling would be unreasonably expensive. The proper handling, separation and collection of bio-waste could increase the yield of valuable compost that could be used later as fertilizer for those crops that are at the beginning of the cycle. In this way bioplastics reduce the environmental impact (European Bioplastics Organization). Figure 4 shows the evolution of the number of publications during the last 10 years concerning these materials as food packaging. The increment observed shows the interest in the area and the preference of biodegradable ones for this application, according to was noted above.
Figure 2. Classification of bioplastics into three main groups: () biobased but non-biodegradable polymers; () plastics that are biodegradable and based on fossil resources, and () polymers that are both biobased and biodegradable.
Figure 3. Life cycle assessment (LCA) of the three types of bioplastics: () biobased but non-biodegradable polymers; () plastics that are biodegradable and based on fossil resources, and () polymers that are both biobased and biodegradable.
Figure 4. Evolution of the number of publications during the last 10 years (2010–2020) with the terms “renewable + biobased + biodegradable food packaging” (), “renewable” (), “biobased” (), or “biodegradable” () as title and/or topic (Scholar Google, March 2021, http://scholar.google.com).
Currently novel food packaging technologies seek to meet new consumers' and industrial demands (Figure 5). Changes in production practices and food sale (for example, market globalization that gives a longer food distribution as a result), population increase, consumers' lifestyles, who reduce time devoted to buying fresh food and cooking but ask for safer and healthier foods and try to take care of environment, along with the advance in new areas of knowledge, such as nanotechnology and biotechnology, act as driving forces to develop new and better packages that can extend food shelf-life, keeping and supervising their innocuousness and quality. In this context, new concepts of smart packaging arise, including active and intelligent packaging, which besides interacting with food establish a communication with the consumer (Biji et al., 2015).
Figure 6 shows the evolution of publications on smart food packaging during the last 10 years, differentiating them between active and intelligent. The increased number of publications was significantly higher for active packaging, which became more marked since 2015, although those of intelligent packaging also grew steadily during the analyzed period. These trends reflect the interest in these new packaging technologies that focus on a safer and more efficient food supply chain, reducing food loss and waste and preventing unnecessary transport and logistics from an early stage. Among these publications, excellent reviews on active and intelligent food packaging systems have been reported during last years (Vanderroost et al., 2014; Bastarrachea et al., 2015; Biji et al., 2015; Brockgreitens and Abbas, 2016; Fuertes et al., 2016a; Ghaani et al., 2016; Mellinas et al., 2016; Ahmed et al., 2017; Fang et al., 2017; Haghighi-Manesh and Azizi, 2017; Gaikwad et al., 2018, 2019, 2020; Han et al., 2018a; Majid et al., 2018; Poyatos-Racionero et al., 2018; Schumann and Schmid, 2018; Vilela et al., 2018; Yildirim et al., 2018). As far as we know, only that recently published by Halonen et al. (2020) deals with describing the development of biobased antimicrobial films and sensors to be used as indirect indicators of food spoilage. In this context, the present review attempts to describe recent progress and new trends in the production of smart, active and intelligent food packaging, with focus on biodegradable and biobased polymers, which will be referred as biopolymers throughout this review.
Figure 6. Evolution of the number of publications during the last 10 years (2010–2020) with the terms “smart food packaging” (), “active” (), and “intelligent” (), as title and/or topic (Scholar Google, March 2021, http://scholar.google.com).
Smart packaging is a broad term that describes new packaging concepts, most of which can be classified in one of two main categories: active or intelligent packaging (Kerry, 2012). Considering the features described by diverse authors, we can define:
– An active package as the one that modifies the condition of packaged foods to extend shelf-life or improve its safety or sensorial properties, keeping its quality. Sometimes this is achieved by the intrinsic properties of the polymer and others adding some specific additives in the packaging material or in the headspace in order to attain a better package yield (Kuorwel et al., 2015; Fuertes et al., 2016a). In this sense, the European Community regulation defines active materials and components as those aimed at extending shelf-life or keeping or improving the condition of packaged foods, designed to deliberately incorporate components that release or absorb substances toward or from the packaged foods and the environment surrounding the foods (Framework Regulation on Food Contact Materials 1935/2004 and 450/2009, 2020). Although bioactive packaging can also be considered in this category, they have another purpose that is to provoke a direct and positive impact on consumers' health through the generation of healthier packaged foods (Lopez-Rubio, 2011).
– An intelligent package as the packaging system capable of carrying out intelligent functions, such as detecting, registering, locating, communicating and applying scientific logics, in order to ease decision-making, extend shelf-life, improve safety and quality, provide information and warn of possible problems (Yam and Lee, 2012). The European Community Framework Regulation (Framework Regulation on Food Contact Materials 1935/2004 and 450/2009, 2020) acknowledges them as those materials and objects that control the state of packaged foods or the environment surrounding them. These systems, which are attached as labels or incorporated or printed on the food packaging material, offer better possibilities to check product quality, track critical items and provide more detailed information during all the food supply chain (storage, transport, distribution and sale) (Lee et al., 2015). They can also inform about product history such as storage conditions, composition of headspace, microbial growth, etc. (Realini and Marcos, 2014).
Intelligent packages do not act directly to extend food shelf-life and do not aim at releasing their components onto the food, as active packaging does. Instead, intelligent packages have the aim to convey information related with food quality to manufacturers, retailers, and/or consumers.
These definitions imply product-package-environment interactions (Vanderroost et al., 2014; Biji et al., 2015; Fang et al., 2017). Thus, active packaging would be considered as an extension of the protection and preservation function of traditional food packaging, while intelligent ones can be considered as an extension of their communication and marketing function (Fuertes et al., 2016a). So, smart packaging gives a total packaging solution: on the one hand, it monitors changes in the product or the environment (intelligent packaging), and on the other hand, it acts upon these changes (active packaging) (Vanderroost et al., 2014; Janjarasskul and Suppakul, 2018). Figure 7 shows a schematic diagram of active and intelligent packaging concepts and Figure 8 presents the classification of smart packaging analyzed in this manuscript.
Active packaging is one of the most dynamic technologies used to preserve foods. Its activity is based on the intrinsic properties of the polymer or the specific properties of additives included into the packaging systems (Fuertes et al., 2016a; Mellinas et al., 2016).
Among biopolymers, chitosan have antibacterial and antifungal activity per se, which is attributed to changes in cellular permeability of microorganisms caused by electrostatic interactions between the chitosan amine groups and the electronegative charges on cellular surface (Lunkov et al., 2018). The introduction of an active monomer into the polymer chain could be an alternative route that conduct to polymers with new functionalities (Dainelli et al., 2008).
On the other hand, active agents can be incorporated into the packaging material, coated on its surface (Halonen et al., 2020), or also inside certain elements associated with the package, such as bags, labels, pads, bottle caps (Ortiz et al., 2013; Gómez-Estaca et al., 2014) instead of being applied directly on foods, in order to meet the desired objective with lower concentrations, thus limiting undesired flavors and smells to foods (Kapetanakou and Skandamis, 2016).
These active compounds are added to release or absorb substances to/from the packaged food or its surrounding environment, or to make changes in food composition or organoleptic features, as long as these changes adjust to current regulations (Ortiz et al., 2014). These active agents may migrate (partially or completely) through gradual diffusion into the food or headspace, or act only when the food is in direct contact with the packaging (Brockgreitens and Abbas, 2016; Halonen et al., 2020).
The nature of active agents can be diverse, including organic acids, enzymes, bacteriocins, fungicides, natural extracts, ions, ethanol, polyphenols, protein hydrolysates, etc. (Salgado et al., 2015). Currently there is a preference in the use of natural additives, instead of synthetic ones that sometimes are associated to certain health risks. Even some agro-industrial waste or byproducts, as those derived from fruit and vegetable processing, or from wine, beer, dairy and meat industries, provide practical and economical sources of potent active compounds (Sanches-Silva et al., 2014; Bhargava et al., 2020). Thermal resistance and action mechanism of these additives should be considered to avoid their damage or loss during material's processing and to ensure their activity in the package. The previous encapsulation of active compounds, could contribute to protect them during processing and to control their release, modulating their activity (Ezhilarasi et al., 2012; Salgado et al., 2019a). Different biopolymers have been studied to encapsulate bioactives such as whey protein isolate, soy protein isolate, zein protein, chitosan, maltodextrin, and modified starch by different techniques (Ezhilarasi et al., 2012; Ðordević et al., 2015; Brandelli et al., 2017; Di Giorgio et al., 2019).
Active package systems for the food industry, with different functionality, are described below with more detail.
Microbial growth increases food-borne diseases risk and accelerates smell, color and texture changes of foods, resulting in a shorter shelf-life. The development of antimicrobial packages presents a promising approach to inhibit, reduce or delay microorganism growth. They can be classified into two types: (i) those that contain antimicrobial agents that migrate to food surface and (ii) those that are effective against surface microbes without requiring the migration of the active agent to food (Han, 2014). Malhotra et al. (2015), Mousavi Khaneghah et al. (2018), Sofi et al. (2018), Huang et al. (2019), Said and Sarbon (2019), and Al-Tayyar et al. (2020) recently reviewed the advances on antimicrobial packaging. The growing trend is to apply natural antimicrobial compounds into the packaging (Kapetanakou and Skandamis, 2016). In this sense, the following substances have been recently incorporated into biopolymers food packaging to activate them with antimicrobial properties in food packages based on biopolymers:
– Organic acids and their salts. Sorbic, benzoic, acetic, propionic, ascorbic acids are strong antimicrobial agents as they can alter membrane transport and permeability, and reduce intracellular pH. Ascorbic, citric, lactic, tartaric, gallic, and vanillic acids have also been used as preservative agents (Coban, 2020). Sodium benzoate and potassium sorbate have a GRAS status and can inhibit the growth of bacterial and fungal cells, being the preservative effect of sorbic acid 5–10 times more resistant than that of benzoate while sodium citrate inhibits the growth of Listeria monocytogenes and Escherichia coli O157:H7 (Rawdkuen, 2018; Huang et al., 2019). They had been incorporated as antimicrobial agent in biopolymers and nanocomposite films such as chitosan (Remedio et al., 2019), pullulan and gelatin (Kowalczyk et al., 2020), and corn starch/chitosan/nanoclay (Na-MMT) (Jha, 2020).
– Plant extracts and essential oils. Aqueous or alcoholic extracts and essential oils from spices and vegetables such as cinnamon, allspice, clove, ginger, sage, eucalyptus, thyme, mustard, basil, rosemary, oregano, onion, garlic, lemon, horseradish, and others have been studied as antimicrobial agents (Çoban et al., 2016; Noshirvani et al., 2017; Ribeiro-Santos et al., 2017; Sirocchi et al., 2017; Mir et al., 2018; Bouarab Chibane et al., 2019), and were incorporated in chitosan-carboxymethyl cellulose, starch, gelatin, PLA, soy protein isolated, alginate, cellulose acetate films, and were proved to be effective as packaging of refrigerated beef meat, fresh rainbow, lard, chicken breast cuts, bologna and ham, etc.
– Bacteriocins. They are antimicrobial peptides produced by microorganisms that can inhibit other strictly related microorganisms. They are not toxic, thermostable, commercially available and used for specific applications in a wide range of foods (Johnson et al., 2018; Santos et al., 2018). Nisin, produced by a Lactococcus lactis, is the most effective bacteriocin against Gram positive bacteria. It acts especially in acid conditions by incorporating into the cytoplasmic membrane of the target cells. Nisin had been incorporated in PLA (Holcapkova et al., 2018), chitosan (Remedio et al., 2019), and carboxymethyl/chitosan films (Zimet et al., 2019). Natamycin and pediocin were also used as antimicrobial agents in pectin and sodium caseinate and corn starch films (Meira et al., 2017; Eghbal et al., 2019).
– Enzymes. Lysozyme can inhibit bacterial infections, especially those caused by Gram-positive bacteria. Its activity is attributed to its ability to hydrolyze peptidoglycans (the main cell wall component), causing the loss of intracellular materials and bacterial death (Syngai and Ahmed, 2019). Lyzozyme had been immobilized on calcium alginate (Wang et al., 2018a), pullulan (Silva et al., 2018), and Jackfruit starch (Bonomo et al., 2018) films. Lactoperoxidase, a glycoprotein enzyme found naturally in milk, saliva, and tear, is another antimicrobial agent with broad spectrum that was included in alginate, chitosan, gelatin (Ehsani et al., 2019), and whey protein films (Shokri and Ehsani, 2017; Farshidi et al., 2018); while lactoferrin had been proposed as antimicrobial agent in pullulan films (Zhao et al., 2019).
– Inorganic and metal oxide-based nanoparticles. The use of metal oxide-based nanoparticles, such as ZnO, MgO, CuO, and TiO2, had been explored as antimicrobial agents to be applied in food packaging because their high stability and potent antimicrobial activities (Garcia et al., 2018; Hoseinnejad et al., 2018; Dobrucka and Ankiel, 2019). Particularly, silver salts work by direct contact, but they slowly migrate and react preferably with organic products. Silver ions inhibit a wide range of enzymes and have a strong broad-spectrum antimicrobial activity; they particularly reduce the growth of pathogens transmitted by foods such as Salmonella, Escherichia coli and Campylobacter in fresh meat (Realini and Marcos, 2014). The addition of silver nanoparticles to different polymers allowed an effective inhibition of microorganism growth. For example, Singh and Sahareen (2017) developed cellulosic packets impregnated with silver nanoparticles that enhance the shelf-life of vegetables, showing antimicrobial activity against Aeromonas hydrophila; and Musso et al. (2017a) developed gelatin films with important antimicrobial properties against E. coli by incorporating silver nanoparticles. Also, Arfat et al. (2017) developed a nanocomposite film based on fish skin gelatin and bimetallic silver-copper nanoparticles with higher antibacterial activity against L. monocytogenes and S. enterica. Recently, Kraśniewska et al. (2020) reviewed the biopolymers-based materials containing silver nanoparticles as food active packaging.
– Macromolecules. Some polymers such as chitosan that have inherent antimicrobial properties, had been used as films and coatings (Lunkov et al., 2018) or had been added as an additive in whey protein films (Vanden Braber et al., 2021). Their action method has been mentioned above.
– Ethanol. Ethanol (C2H5OH) is used as an antimicrobial agent, particularly effective against fungi although it can also inhibit yeast and bacteria growth (Larson, 1991). Several reports have shown that spraying with ethanol 95% delays the appearance of fungi and extends the shelf-life of bread, cake, and pizza (Pereira de Abreu et al., 2012; Mane, 2016). However, a more practical and safer method currently consists of using ethanol-emitter bags because C2H5OH absorbed or embedded in a carrier material allows its vapor-controlled release (Hempel et al., 2013). Commercial devices are produced by the direct adsorption of C2H5OH and H2O on adsorbents, such as silica, packaged as sachets based on paper and laminated ethyl-vinyl-acetate or low-density polyethylene. When food is packed with these bags, H2O is absorbed by the food and C2H5OH vapor is released and spread in the headspace (Dobrucka and Cierpiszewski, 2014). Nevertheless, ethanol can be absorbed by the food product generating nasty smells, which could be reduced heating up the food before its consumption. Therefore, controlled release technologies should overcome the disadvantages of conventional ethanol emitters. Mu et al. (2017) developed a controlled release ethanol emitter based on an ethanol-gel prepared by ethanol and sodium stearate esterification added with diatomite as adsorbent capable to preserve the quality of fresh Chinese bayberry fruit during refrigerated storage; and anticipated that it could be applied for other perishable fruit because of the appropriate selection of sodium stearate content in agreement with the storage period to reach precise storage goals.
Lipid oxidation is the second cause of food spoilage, after microbial growth. Foods with high lipid content, and especially those with a high unsaturation degree, such as nuts, vegetable and fish oils, meat and fishing products, are susceptible to oxidation (Ganiari et al., 2017). Lipid oxidation in food products results in the development of rancid smells, making the product unacceptable for human consumption. Other negative effects are aldehyde formation and nutritional quality loss produced by the degradation of polyunsaturated fatty acids (Gómez-Estaca et al., 2014). There are two main action methods for antioxidant packages: (i) the addition of antioxidant compounds or (ii) the elimination of undesired compounds that can accelerate oxidation reactions, such as O2, singlet oxygen (1O2), UV light, or metallic ions from the headspace or foods (Islam et al., 2017).
The direct addition of antioxidants to food surface can face the limitation that, once the active compounds are consumed in the reaction, protection ceases, and food quality degrades at a higher speed. Besides, it can change food quality parameters, such as color or taste, and currently consumers prefer the exclusion of additives in food. Thus, the addition of antioxidant agents to packaging formulation became a good alternative (Vilela et al., 2018). In this regard, synthetic antioxidants, such as butylated hydroxytoluene (BHT) or butylated hydroxyanisole (BHA), have been added to PLA (Jamshidian et al., 2012), gelatin (Sai-Ut et al., 2015), carrageenan (Wan Yahaya et al., 2019), gum cordia and carboxymethyl cellulose (Haq et al., 2015) films proving to effectively reduce lipid oxidation in several foods. However, the use of natural antioxidants like pure compounds (e.g., tocopherols, caffeic acid, chlorogenic acid, ferulic acid, rosmarinic acid, salvianolic acid, carnosic acid and ascorbic acid carvacrol, thymol, carnosol, quercetin, luteolin, apigenin, eriodictyol, catechin, rutin), plant and fruit extracts (e.g., rosemary, grape seed, green tea, oregano, murta, mint, marjoram, savory, and pomegranate peel), and essential oils or oleoresins from herbs and spices (e.g., cinnamon, lemongrass, clove, thyme, ginger, sage, oregano, pimento and bergamot) (Amorati et al., 2013; Sanches-Silva et al., 2014; Valdés et al., 2015; Ganiari et al., 2017; Tsimogiannis et al., 2017) is currently preferred. Likewise, it is important to mention the potential use of extracts obtained from plant by-products like barley husks, pomegranate peel, olive leaves, as sources of antioxidant agents (Pereira de Abreu et al., 2012; Barbosa-Pereira et al., 2013; Marcos et al., 2014; Qin et al., 2015; Yuan et al., 2015; de Moraes Crizel et al., 2016). The antioxidant properties of peptides obtained from diverse food proteins were also studied (Salgado et al., 2011; Blanco-Pascual et al., 2014; Rostami et al., 2017; da Rocha et al., 2018; Lima et al., 2018; de Oliveira Filho et al., 2019; Montero et al., 2019). Over the last years, several studies have been published dealing on addition of different antioxidant compounds to biodegradable and biobased polymers and their assessment in diverse food systems (Eça et al., 2014; Sanches-Silva et al., 2014; Ganiari et al., 2017; Lourenço et al., 2019; Sahraee et al., 2019; Jacob et al., 2020).
Acting as antioxidants, free-radical scavengers can donate hydrogen to reactive free radicals and convert them into (fairly) stable products unable to participate in initiation or propagation reactions (Vilela et al., 2018). Table 1 summarized some newly published research on this topic. In addition to primary antioxidants, other additives like chelating agents, ultraviolet absorbers, singlet oxygen quenchers, and oxygen scavengers are also used to reduce the oxidation rate in active packaging (Vilela et al., 2018). Metal pro-oxidants (e.g., iron or copper derivatives) could be converting into stable products using metal chelators such as ethylenediaminetetraacetic acid (EDTA), poly (acrylic acid), citric acid, and lactoferrin (Rawdkuen, 2018). Some examples of UV absorbers are lignin (Aadil et al., 2016; Yang et al., 2016a), naringin, boric acid, bixin, α-tocopherol, ellagic acid, natural extracts rich in phenolic compounds (Vilela et al., 2018), and pigments like phtalocyanine and TiO2 (Tian et al., 2013). On the other hand, carotenoids, tocopherols and polyphenols have been described as singlet oxygen (1O2) quenchers (Vilela et al., 2018). Finally, oxygen scavengers are described latter as affect more than one activity.
Table 1. Recent examples of antioxidant agents incorporated into biobased and biodegradable polymer matrices.
The adequate selection of the antioxidant compound to be incorporated in the polymeric matrix is very important and should be considered for each application. The antioxidant compound and polymer matrix should be compatible to achieve a homogeneous distribution, and the antioxidant partition coefficients in the different phases must that favor their release toward the food or headspace. Once released, the antioxidant solubility features could determine its efficacy, so in turn the antioxidant must be selected depending on food type.
These devices try to control water activity inside the package in order to reduce microbial growth; eliminate defrosting water from frozen products and blood or fluids from flesh food products such as meat and poultry; avoid excessive condensation or dehydration of fresh products such as fruit, vegetables and meat products; keep the properties of dry foods to prevent seizure in powder products such as instant milk or coffee, softening of crunchy products such as biscuits, and dampening of hygroscopic products as candy and sweets; and avoid lipid oxidation in products such as cheese and nuts (Janjarasskul and Suppakul, 2018; Gaikwad et al., 2019).
Moisture absorbents can be classified into: relative humidity (RH) regulators that absorb moisture and control humidity in the headspace of the package, and liquid removers that absorb and hold exuded liquids from food products (Yildirim et al., 2018). Moisture absorbers are hygroscopic substances that absorb and retain water from the surrounding environment through physical adsorption, while moisture absorbers absorb water through a chemical reaction between two functional materials. Common desiccants such as silica gel, CaO, CaSO4, CaCl2, KCl, K2CO3, natural clays (montmorillonite, zeolite, and bentonite), fructose, xylitol, and sorbitol, or superabsorbent polymers like carboxymethyl cellulose and starch can be located between two layers of a microporous polymer (such as sachets, sheets or pads) and are typically placed below fresh products in different packaging concepts (Dobrucka and Cierpiszewski, 2014; Pinming et al., 2016; Yildirim et al., 2018).
These devices could be used together with adequate water-barrier materials without interfering with the external structure of packaging. Furthermore absorbers could be incorporated inside the bulk packaging material or as a coating for commercial food product applications such as fresh fruits, vegetables, fish, and meat (Yildirim et al., 2018). Using biopolymers, Rhim and Wang (2013) proposed agar, carrageenan, and konjac glucomannan films as potential food packaging moisture absorbents because of their safety and large water vapor absorption capacity. Guo et al. (2018) studied the hygroscopic behavior of cellulose nanofiber-based films, Chand and Kumar (2018) analyzed the application of hydroxypropylmethyl cellulose and carboxymethyl cellulose edible coatings on Jaggery cubes. Sängerlaub et al. (2018) developed PLA films with dispersed CaCl2 (2-4 wt%) by extrusion that could absorb up to 15 wt% water vapor at 75% RH at 23°C. The functionality of these devices could be broadened by adding other types of active components such as O2-scavengers, CO2-generators, and antimicrobials (Janjarasskul and Suppakul, 2018). Future research focused in this area is expected.
MAP technology involves the alteration of the gas atmosphere inside a food package to preserve the food quality and extend its shelf-life (Sängerlaub et al., 2018). So, it can consider as an active packaging. This method can extend the conditions of fresh food products and has been applied to perishable food products. High O2 concentrations favor oxidative processes, which can modify food quality, whereas its reduction delays breathing speed, ethylene production, enzymatic browning, and metabolic activity of fruit. Breathing implies the oxidation of starch, sugar, and organic acids to produce simpler molecules such as CO2 and H2O and heat. CO2 in packages prevents microorganism and bacteria growth associated with meat products spoilage. For these reasons, MAP can prolong shelf-life, reduce microbial growth and smells development or delay ripening (Lee et al., 2015). The most utilized gases in MAP are O2, N2, and CO2. These gases are used individually or combined to keep the characteristics and quality of foods on the shelf (Zhang et al., 2015). Other gases such as helium, nitrous oxide, ozone, neon, argon, ethylene oxide, sulfur dioxide, and chlorine have also been used (Lee et al., 2015). Numerous studies have shown the successful application of MAP in products such as fresh vegetables, fruit, meat, and fish (Oliveira et al., 2015; Belay et al., 2016; Saini et al., 2017). Han (2014), for example, describes various bioplastics and MAP combinations to extend shelf-life of lettuce, green pepper, broccoli, mushroom, and blueberries, among other vegetables. Leceta et al. (2015) reported that packaged carrots exhibited better properties when MAP and chitosan coating were combined. Priyadarshi et al. (2020) discussed the applications of various polysaccharides in MAP systems, such as alginate, cellulose, and chitosan, gellan gum, pectin, carrageenan, and xanthan. Finally, it is worth highlighting that the potential uses of MAP increase when it is used in conjunction with natural or chemical additives and biopolymers, and other technologies such as nanotechnology, irradiation, high pressure, and other active and intelligent devices (Tajeddin et al., 2018). For example, the O2 that permeates through the package film from the external environment cannot be eliminated by MAP systems. In these cases, the use of materials with low O2 permeability, such as those based on proteins and PLA (Ebnesajjad, 2012) or the addition of O2 scavengers to the packaging system can be suggested. Also, when a mixture of CO2 and O2 are applied in MAP, CO2 generators presence could be helpful and required for maintaining the desired CO2 concentration in the package to ensure MAP system efficacy, as its concentration dynamically diminish in the package due to its dissolution in food and/or permeation to the external environment through the packaging material (being CO2 permeability 3–5 times higher than that of O2 in most synthetic polymers) (Han et al., 2018a).
Some of these active devices, such as oxygen and ethylene scavengers and carbon dioxide (CO2) generators and scavengers are outlined below.
Oxygen accelerates and eases the oxidative spoilage of foods, the growth of aerobic microorganisms, the development of nasty smells and unwanted color changes and the loss of aroma, flavors and nutritional value of food products, reducing their general stability and shelf-life (Hogan and Kerry, 2008). Consequently, it is important to control oxygen levels into the packages to limit different spoilage reactions rate in foods (Janjarasskul and Suppakul, 2018).
Oxygen scavengers are designed to quickly oxidize to eliminate or decrease the level of free O2 in hermetically packaged food system or to reduce O2 permeation rates through container walls (Janjarasskul and Suppakul, 2018). Most oxygen scavengers currently available are based on bags containing Fe with different catalysts. It reacts with the water provided by food producing a hydrated metal reducing agent that absorbs oxygen and converts it into a stable oxide inside the container (Day, 2008). But packaging industry has become progressively interested in the development and application of novel non-metallic scavenging agents such as reducing organic compounds like ascorbic acid, α-tocopherol, 2-methyl hydroquinone, catechol, sorbose, lignin, gallic acid, polyunsaturated fatty acids, sulfite, thiosulfate, some enzymes (glucose oxidase/catalase in presence of glucose, oxalate oxidase/catalase, ethanol oxidase, and laccase), and some microorganisms and yeasts (Bacillus amyloliquefaciens, Kocuria varians, and Pichia subpelliculosa), among others (Gaikwad et al., 2018; Dey and Neogi, 2019). Besides, there are photosensitive colorants that activate oxygen elimination when they are irradiated with UV light (Lopez-Rubio et al., 2004). Most of these systems are beneficial for food with high water content but need to be triggered by Fe2+, Cu+, and/or UV radiation. In particular, those based on enzymes and microorganisms are highly susceptible to temperature, pH and water activity changes; and polyunsaturated fatty acids based systems, are an excellent option for dry foods, but the formation of by-products like aldehydes, ketones or organic acids may affect the sensory quality of food (Dey and Neogi, 2019). These systems are more expensive, that is why they are not widely used (Dobrucka and Cierpiszewski, 2014).
These systems can be incorporated as part of multilayer films, sandwiched between inert layers, or physical absorbed or coated onto the packaging material surface or also onto food surface. Thus, potential O2 scavenger systems based on biopolymers were developed, such as whey protein edible films added with ascorbic acid (Janjarasskul et al., 2011, 2013), ascorbyl palmitate-β-cyclodextrin inclusion complexes (Byun and Whiteside, 2012), cast extruded PLA films added with α-tocopherol microparticles (Di Maio et al., 2014; Scarfato et al., 2017), carboxymethyl cellulose films incorporated with α-tocopherol nanoparticles (Noronha et al., 2014), fish gelatin films added with α-tocopherol nanoparticles and FeCl2 (Byun et al., 2012), cationic nanofibrillated cellulose and polygalacturonic acid coatings (Mølgaard et al., 2014), starch-based films incorporated with laccase and lignosulfonates (Johansson et al., 2012), and extruded thermoplastic starch films containing ascorbic acid, iron powder and CuCl2 (Mahieu et al., 2015). These systems have better consumers acceptance than sachets or labels traditionally used inside the package (Gaikwad et al., 2018).
CO2 can be added inside the packages to inhibit a wide range of aerobic bacteria and fungi by reducing O2 relative levels in certain products such as fresh meat, poultry, fish, cheese and baked products; reduce breathing rate of fresh products and avoid partial emptying of flexible packaging caused by oxygen scavengers or by CO2 concentration decrease due to its dissolution in food and/or permeation to the external environment through polymeric packaging (Han et al., 2018a; Yildirim et al., 2018). On the contrary, high levels of CO2 can cause adverse effects in food products quality and on package integrity. In those cases, including CO2 scavengers in food packages would be recommended (Dobrucka and Cierpiszewski, 2014). Some CO2 generators and scavengers can be used as bags or labels placed in the package. Dripping losses, in meat for example, can be absorbed in pillows and react with citric acid and sodium bicarbonate present in the pillow, generating carbon dioxide as a result (Kerry et al., 2006). This kind of active package is habitually associated with MAP to balance CO2 losses, due to dissolution in meat and permeation through the package material (Coma, 2008). A device enclosing NaHCO3 and citric acid dry powder could act as both liquid absorber and CO2 generator pad (Biji et al., 2015). Also, dual-function O2-scavenging and CO2-generating sachets and labels based on either FeCO3 or a mixture of NaHCO3 and ascorbic acid were reported (Vilela et al., 2018). However, only some studies have described the development of synthetic films that can produce CO2, scavenge O2, or perform both functions, but none using biodegradable and biobased polymers, as this concept is yet in its early stage of research (Han et al., 2018a). Meanwhile CO2 excess can be eliminated using highly permeable plastics and/or chemical [CaO, MgO, NaOH, KOH, Ca(OH)2, Mg(OH)2, Na2CO3, NaHCO3 and silica gel] and physical (activated carbon and zeolites) absorbers. It should be noted that physical CO2 sorption on the adsorbent can be modified by moisture content (Marx et al., 2013). Although the absorbents are usually enclosed in sachets or pads prepared with synthetic polymers and placed in the food package, other formats have been recently proposed. Agar-based films containing Na2CO3 and/or sodium glycinate were developed by Wang et al. (2015a) for fresh product packaging. This device could modulate CO2 and H2O absorption and be used for MAP of shiitake mushrooms as an insert label, generating the desired internal atmosphere and showing the best quality preservation in terms of color, firmness, flavor, and bacterial growth.
Until now, CO2 scavengers have been applied using a trial-and-error approach rather than a systematic design process. Thus, their optimization should be achieved by characterizing the dynamics of the interactions among the food, the packaging material, the absorber device and the environment in terms of CO2 production, dissolution, absorption and permeation (Lee, 2016).
Ethylene (C2H4) is a phytohormone which accelerates fruit and vegetable breathing and promotes its ripening, softening and senescence, even in a low concentration. Eliminating C2H4 from a package helps to delay the ripening rate of climacteric fruit, a crucial issue for fresh fruit exportation (East et al., 2015). The C2H4 can be controlled in three ways: (i) reduction of the C2H4 concentration through MAP by the exchange of the different gases; (ii) use of micro-perforated packaging materials; and iii) removal of ethylene through the use of C2H4 scavengers or absorbers (Vilela et al., 2018; Gaikwad et al., 2020).
The most common C2H4 scavenger agent is KMnO4 which oxidizes C2H4 –in presence of water– to CO2 and H2O (Gaikwad et al., 2020). Potassium permanganate immobilized on inert matrices (e.g., silica gel or alumina) is available in the form of sachets for packages and in blankets for storage rooms (Janjarasskul and Suppakul, 2018), prepared with C2H4 highly permeable materials (Yildirim et al., 2018). Recently, Warsiki (2018) developed chitosan films activated with KMnO4 to wrap tomatoes that managed to prolong their shelf-life. Nevertheless, KMnO4 cannot be utilized in direct contact with foods due to its high toxicity and has a limited long-term efficacy in high-moisture environments (Yildirim et al., 2018).
The use of 1-methylcyclopropane (1-MCP) is another option to diminish the effect of C2H4. Its action mechanism implies its irreversible union to the C2H4 receptor in fruit, thus blocking C2H4 effects by competitive inhibition. It is encapsulated in cyclodextrins in commercial products and released when the formulation is mixed with water (Ortiz et al., 2013). Ortiz et al. (2013) showed that pillows prepared with soybean proteins could use the RH inside the package to release 1-MCP and delay tomatoes softening and suggested that it could be useful for postharvest treatments in the own package.
Ethylene can also be eliminated by physical adsorption on active surfaces such activated carbon, carbon impregnated with metal catalysts or activated clays (zeolite, vermiculite, and montmorillonite) contained into ethylene-permeable sachets (Álvarez-Hernández et al., 2018) or incorporated into the packaging film (Gaikwad et al., 2020). Biodegradable and biobased polymers have been reinforced by nanoclays addition (Belibi et al., 2013; Echeverría et al., 2014; Azevedo et al., 2018; Souza et al., 2018). Bessa et al. (2015) developed starch-zeolite films able to maintain the natural characteristics of guava fruit during refrigerated storage, and García et al. (2014) showed that chitosan-zeolite coating could delay tomatoes ripening during refrigerated storage, although did not constitute an effective barrier against their weight losses. However, the clay properties as C2H4 capturers were not studied in these studies. Recently, Kaewklin et al. (2018) developed chitosan films containing nanosized TiO2 that exhibited C2H4 photodegradation into CO2 and H2O which might contribute to delay the ripening process and extend the storage life of cherry tomatoes stored at 25 °C and 50% RH. But it should be noted that the C2H4 removal capacity of the chosen material must be considered in conjunction with the proposed application and expected shelf-life when designing a packaging system for ethylene sensitive foods.
These systems absorb unwanted gas molecules such as volatile ingredients of the package, food chemical metabolites, microbial and deterioration reactions products, breathing products or unwanted smells coming from raw foods (Rooney, 2005). Some examples could be sulfides and amines produced from protein degradation, and aldehydes and ketones produced from lipid oxidation or anaerobic glycolysis. These volatile compounds could be selectively eliminated to prevent food product rejecting by the consumer when opening the package (Mane, 2016). These systems use a mass transfer mechanism and in general are films, sachets, tapes, labels, or trays that are placed inside the containers or combined with other permeable materials.
Porous materials such as zeolites, clays, activated charcoal, maltodextrin and cyclodextrin have been used as smell capturers; for example, charcoal and nickel were proposed to absorb fruit smells (Morris, 1999). Volatile amines, such as trimethylamine, from fish flesh could be eliminated by adding citric or ascorbic acid into polymeric material (Hoshino and Osanai, 1986). Polar materials can be used to prevent the absorption of smells with non-polar features in foods (Sajilata et al., 2007). In some products, especially fat-rich or vacuum-packaged foods, nasty smells are absorbed during storage or distribution. These smell-proof packages have been mainly developed based on synthetic polymers such as polyethylene terephthalate, polyethylene, polyvinylidene chloride, and polypropylene (Biji et al., 2015). Nasty smells can also be avoided by improving the barrier properties of films combining different package materials or adding nanoparticles to prepare nanocomposites (Mihindukulasuriya and Lim, 2014). However, it is worth noting that even though eliminating stinking components is recommended to improve packaged foods quality, these technologies should not be used to mask smells produced by dangerous microorganisms that could jeopardize consumers. As far as we know, there is no literature related to the development of smell capturers using biodegradable polymers.
These packages do not have the aim of extending the shelf-life of the packaged food, but they are oriented to generate comfort situations such as ready-to-eat food. A self-heating package can heat the food content without external heat sources or energy. They use calcium or magnesium dioxide and water to produce an exothermic reaction in a device that takes a significant amount of package volume (almost half of it). They have been used for coffee packets, military portions, etc. (Lee et al., 2014). Some self-cooling packages involve endothermic chemical reactions and heat pump technology using water vapor as the heat transfer fluid. They have been used to cold single-serve beverage packaging. Active packages for microwaves are designed to improve food heating by means of shielding, field modification and the use of susceptors. Shielding can be applied to attain a more uniform, differential, and controlled heating to different portions of food. Modifiers for microwave heating consist of a series of structures that alter the way microwaves reach food, resulting in a uniform and crunchy heating. Microwaves susceptors consist of aluminum or stainless steel deposited on substrates, such as polyester or cardboard films, that serve to dry, leaving a crunchy texture in food products (Realini and Marcos, 2014; Biji et al., 2015). As these technologies are new, there is no literature related to the development of these materials using biodegradable and biobased polymers.
Intelligent packaging can sense, monitor, record, trace, and provide information about the quality of food (Han et al., 2018a; Schaefer and Cheung, 2018). It can be used with decisions making concerning shelf-life, safety, and quality (Dobrucka and Przekop, 2019). They can disclose the conditions of the packaged product, but cannot interact with the food (Biji et al., 2015).
Intelligent packaging systems can be classified into three main categories: indicators, sensors and data carriers (Ghaani et al., 2016; Müller and Schmid, 2019):
(i) Indicators. They supply immediate information (visual, qualitative, or semi-quantitative) about food through a color (or color intensity) change or by dye diffusion (Kerry et al., 2006). They report a change in the product or its environment (for example, temperature, pH) through visual changes, such as time-temperature indicators (TTI), oxygen indicators, comfort indicators and freshness indicators (Hogan and Kerry, 2008; Lee et al., 2015; Ghaani et al., 2016).
(ii) Sensors. They are devices used to detect, locate, or quantify energy or matter, sending a detection signal or measurement of a physical or chemical property captured by the device. They can detect small molecules of pollutants, pathogens, allergens, or adulterants in food matrixes (Ramos et al., 2015). Sensors are more complex than indicators since they are formed by a receptor that transform the chemical or physical signal into energy, and a transducer that turns that energy into an analytic signal (Ghaani et al., 2016). Commonly, they are gas sensors (for example, for nut respiration products, microorganism metabolites, gases in the headspace of the container) or bio-sensors (used for example in the detection of pesticides in fruits and vegetables) (Biji et al., 2015; Lee et al., 2015).
(iii) Data carriers. They are new devices that provide information or control the flow of products, particularly appropriate for big productions such as supply chains. Compared with indicators and sensors, they do not provide quantitative or qualitative information. They are used for identification, automation, traceability, anti-theft prevention or forgery protection. The most important devices in the packaging industry are radiofrequency identification (RFID) labels, bar codes and QR code (Quick Response) (Robertson, 2012; Ghaani et al., 2016; Kalpana et al., 2019).
Some examples of useful intelligent packages in the food industry are described below.
They provide information on food product quality from biochemical changes or contaminating microorganisms growth (Kuswandi et al., 2013; Realini and Marcos, 2014). Certain metabolites such as glucose, CO2, organic acids, volatile nitrogen compounds, biogenic amines, ethanol, ATP degradation products, and sulfuric compounds, among others, are considered “quality indicators” (Fang et al., 2017). Changes in their concentrations are monitored through indicators and are generally observed as a change in color response that can be sensed and correlated with the freshness of the food product (Fuertes et al., 2016a; Wang et al., 2018b). The reaction between these metabolites and indicators within the packaging system presents visual information about the microbiological quality of the products.
Most indicators are based on pH change due to the production of certain specific metabolites such as n-butyrate, L-lactic acid, D-lactate, acetic acid and volatile amines (Kerry et al., 2006; Yoshida et al., 2014). Eagland and Crowther (2007) developed pH indicators incorporating a mixture of universal indicators in synthetic or polyvinyl alcohol matrixes. Gorski and Booher (2011) included different acid-base indicators such as xylenol blue, bromocresol purple, bromocresol green, cresol red, phenolphthalein, bromothymol blue, neutral red, p-naphtholbenzein and their combinations in polyethylene films, that were used to pack chicken. Chicken, pork and beef spoilage sensors have recently been developed by incorporating pH sensitive material into packaging (Ghaani et al., 2016; Chen et al., 2019a). These reports are basing the measurement on the simple chemical or pH change that can indirectly indicate food spoilage.
Musso et al. (2016) formulated gelatin films with different synthetic acid-base indicators: methyl orange, neutral red and bromocresol green, that could change their color with microbial growth in fish (Musso et al., 2017a). Chen et al. (2018) developed a fresh cut green bell pepper freshness label based on methyl red and bromothymol blue mixtures that changed from yellow-green to orange when pepper decay.
But nowadays the use of natural dyes within biopolymers is prefer considering consumer expectations for food safety (Saliu and Della Pergola, 2018). De La Puerta et al. (2014) developed a solid partially polar adsorbent support (based on paper, cardboard, and polypropylene) impregnated in a vanillin solution, capable of going from colorless to violet when detecting pH changes in food. Anthocyanins, flavonoids responsible for the bright and attractive orange, red, purple, and blue colors of most fruits, vegetables, flowers, and some cereal grains are suitable candidates to be used in pH-sensing labels that can be added inside packages to give simple signals related to food preservation (Singh et al., 2018b; Zhang et al., 2019c; Chen et al., 2020; Sun et al., 2020). Liang et al. (2019) prepare an intelligent Artemisia sphaerocephala Krasch gum-based film capable of anchoring the natural dye extracted from red cabbage that exhibited a color change from pink to green along with pH variations ranging from 3 to 10. Halász and Csóka (2018) immobilized dyes from black chokeberry (Aronia Melanocarpa) pomace extract in chitosan films, that responded well to the pH change and showed a high color difference from pH 1 to pH 10. Medina-Jaramillo et al. (2017) and Yoshida et al. (2014) developed colorimetric pH indicators which consisted of chitosan or starch films formulated with anthocyanin or green tea and basil extracts, respectively. Musso et al. (2017b, 2019) obtained gelatin films added with anthocyanins extracted from red cabbage and curcumin capable of responding to pH changes in the environment. But it should be noted that most of these materials also presented antioxidant activity, due to the presence of active compounds in their formulation. Zhang et al. (2019a) developed an starch/PVA/roselle anthocyanins film that changes its color from red to green when was used to monitor the freshness of pork stored at 25°C, before the TVBN value of the pork gradually increased to the rejection limit (15 mg/100 g) at 36 h.
Betalains are other interesting natural compounds to use as indicators of pH change. They are water-soluble nitrogen containing pigments that can be divided into red and yellow colored ones. Kanatt (2020) developed PVA and gelatin films incorporated with Amaranthus leaf extract to monitor freshness of fish and chicken meat. The film showed a visible color change from red to yellow on spoilage that could be corroborated with the increase in pH, TVBN and microbial counts of the spoiled samples. And Moreira et al. (2018) developed PLA/PEO ultrafine fibers containing phycocyanin (pigment of intense blue color, constituting the biomass of microalga) to be used as pH indicators membranes for food packaging.
Some of these indicators had been used to detect changes in CO2 levels in packages, product of microbial development. Morris et al. (2004) worked with agar and silicone films containing a mixture of bromothymol blue and methyl orange, which turns from green to orange when the pH decreases due to the presence of CO2. Other indicators of CO2 were developed based on aqueous solutions of chitosan or whey protein isolate whose transparence changes depending on pH due to the appearance of CO2 (Puligundla et al., 2012; Lee and Ko, 2014). Perez de Vargas-Sansalvador et al. (2017) developed a CO2 sensor by including anionic liquid (1-ethyl-3-methyl-imidazolium chloride) in a hydroxyethylcellulose matrix that was effective to indicate microbial spoilage in pork. Saliu and Della Pergola (2018) investigated the performance of a food grade mixture constituted of L-Lysine, ε-poly-l-lysine, and anthocyanins as CO2 sensing. Choi and Han (2018) developed a CO2 indicator based on reactions of sodium caseinate and pectin that was applied to kimchi packaging.
Other authors focused on detecting volatile amines, resulting from the degradation of trimethylamine N-oxide, through total volatile basic nitrogen (TVBN) determination as indicators of freshness in fish, poultry, and meat (Kuswandi et al., 2012); as theses amines are responsible for fish odor and taste. Boscher et al. (2014) described the detection of trimethylamine, triethylamine, and dimethylamine using PET films coated with metalloporphyirin. Pacquit et al. (2006, 2007) developed a colorimetric indicator capable of detecting an increase in volatile amines concentration as an indicator of fish spoilage that managed to correlate the response with the total viable count and the growth of pseudomonas. Kuswandi et al. (2012, 2014) also developed indicators of volatile amines based on polyaniline films, cellulose and bacterial cellulose membranes added with methyl red or curcumin, capable of indicating spoilage in fish.
Hydrogen sulfide, which is released by meat during aging because of cysteine decomposition, correlates with the color of myoglobin and is considered a quality attribute for meat products. Smolander et al. (2002) and Smolander (2008) developed a freshness indicator containing myoglobin immobilized in agarose for poultry meat with a modified atmosphere.
In the case of fruit, labels that change their color indicating different maturity stages have been developed. They work through the reaction of the aromas released by the fruit as it ripens (Fuertes et al., 2016a). The aforementioned indicators detect a single compound or parameter, and may have certain limitations, such as lack of specificity giving erroneous results, since false positives or negatives may be generated (Kerry et al., 2006; Nopwinyuwong et al., 2010; Kuswandi et al., 2011).
In addition to colorimetric tests to detect volatile amines, electrochemical sensors have also been used. Bhadra et al. (2015), for example, created a hydrogel-coated pH- electrode passive sensor that reports basic volatile concentration.
As humidity is one of the most important factors to take into account in the shelf life of food, numerous studies have been done on humidity sensors used in real time for packaged foods in order to prevent spoilage (Amin et al., 2013; Feng and Lee, 2016). Vilela et al. (2019) developed an antibacterial conductive nanocomposite films composed of poly(sulfobetaine methacrylate) and bacterial nanocellulose that could shield the food from the effects of UV-radiation, inhibit the growth of pathogenic microorganisms responsible for food spoilage and foodborne illness, absorb moisture and water, and act as conductimetric humidity sensors to control humidity levels in foodstuff.
Package integrity is an essential requirement to maintain the quality and safety of food products along the whole production and distribution chain (Vanderroost et al., 2014). Gas indicators are the most commonly used integrity indicators for packaging applications. They are found in packaging film or can also be found in the form of packaging labels. These labels react in response to changes in the internal atmosphere of the packages (metabolism of microorganisms, permeation phenomena and enzymatic or chemical reactions in food) (Yam et al., 2005). Variation in composition of gases induce color changes that might be attributed to the incorrect package sealing, leaking, and/or has been tempered with (Fuertes et al., 2016a).
Gas indicators for O2, CO2, water vapor, hydrogen sulfide, ethanol, and other gases have been reported (Meng et al., 2014). The leakage in a container with modified atmosphere implies a considerable increase in the O2 concentration and a decrease in CO2 concentration, which could produce a faster deterioration of the packaged food. Therefore, there are leakage indicators for MAP based on the detection of O2 and CO2 (Fuertes et al., 2016a; Matindoust et al., 2016).
Most of the O2 indicators used are colorimetric indicators based on a redox reaction, for example methylene blue in the presence of a reducing agent such as glucose in an alkaline medium (Mills, 2005; Silva-Pereira et al., 2015). Consequently, the dye oxidizes with O2, which causes the color change. However, these dyes have certain limitations, for example, the color change becomes less visible as the O2 level decreases (reversible phenomenon), which can be an inconvenience to detect subsequent microbial growth (Saarinen et al., 2017). To avoid this problem, O2 indicators and oxygen scavengers can be placed together in the packaging (Mitsubishi Gas Chemical, 2014; Realini and Marcos, 2014). Commercially reversible O2 indicators such as Ageless Eye tablets (Mitsubishi Gas Chemical Company Inc) are used in conjunction with AGELESS oxygen absorbers. The tablets change color (from pink to blue) and depends on the temperature. Tell-Tab O2 Indicator Tablets (IMPAK) work the same way (Sorbent Systems, 2014). Likewise, OxySense is a commercial luminescence oxygen indicator label to indicate any leaks in modified atmosphere packaging, and Emco Packaging developed a non-reversible O2 indicator labels for the same purpose.
Lawrie et al. (2013) and Lee et al. (2008) developed UV-activated oxygen indicators. They consist of an ink, composed of a semiconductor such as TiO2, a redox dye such as methylene blue, an electron donor as triethanolamine and an encapsulating polymer like hydroxyethyl cellulose, which can be used as a coating or printed afterwards on a variety of substrates to produce a blue film indicating oxygen concentration that, when is activated by UV light becomes colorless (Ghaani et al., 2016).
Recently, Saarinen et al. (2017) developed a large-scale oxygen indicator, which is based on a colorimetric change and is activated by ultraviolet light. Provides an inexpensive MAP leak indicator that is printed on the packaging material or the label is affixed to the inside of the package lid.
Similarly, Vu and Won (2013) developed an O2 indicator that is activated on exposure to UV-light and can recover its original color in the existence of oxygen. These authors had also developed a way to prevent the dye from seeping out of the O2 indicator labels using alginate. To solve this problem, Jang and Won (2014) also proposed a pressure activated O2 indicator in such a way as to physically separate the components of the O2 indicator. Another type of oxygen sensors are those that are based on phosphorescence. Generally an O2 sensitive probe is encapsulated in an O2 permeable polymer, which allows the dye to access O2 (Banerjee et al., 2016). Optical sensors based on luminescent dyes have also been used to monitor CO2 in food packaging (Wang et al., 2017a). The limitation of these luminescent dyes is that they require the use of sophisticated instrumentation (that can generate excitation and detection) (Wang et al., 2017a; Saliu and Della Pergola, 2018).
Finally, there are also O2 detection systems based on oxidative enzymes (Mattila et al., 1990; Gardiol et al., 1996) which can be formulated in pills (Ageless Eye, Mitsubishi Gas Chemical company), as a printed layer (Emco Packaging), or laminated in a polymeric film (Smolander et al., 1997; Fuertes et al., 2016a).
The time-temperature indicators record the temperature history, either totally or partially, and thus indicate the shelf life of perishable products (Lee and Rahman, 2014; Wang et al., 2015b; Biegańska, 2017). In this way they can indicate and alert consumers to potential microbial growth or possible protein denaturation (Wang et al., 2015b; Zabala et al., 2015).
TTIs are based on irreversible color changes, so consumers can quickly tell if the product is safe (Ahmed et al., 2018).
Three categories of TTI are described: (i) critical temperature indicators, which sense whether the products are at a temperature above or below their corresponding temperature; (ii) indicators of partial history, which indicate if a product has been subjected to temperature at any time, which will cause a change in the quality of the product; (iii) complete history indicator that records temperature throughout the entire food supply chain (O'Grady and Kerry, 2008; Müller and Schmid, 2019).
TTIs are based on sensing time- and temperature-dependent changes in a food product. These changes can be chemical (acid-base reactions or polymerization), electrochemical, mechanical, enzymatic, or microbiological (Pavelková, 2013). The measured values are usually expressed as a visible response, like color changes or mechanical deformations (Dobrucka and Przekop, 2019).
Chun et al. (2013) developed enzymatic TTI to indicate ground pork patty quality based on color changes from green to red due to the cumulative effect of time period and temperature of the enzymatic reactions. Meng et al. (2018) developed an enzymatic TTI consisting of an agar coating and a coating containing microcapsules of sodium alginate, iodine, amylose and glucoamylase, and it was used to control the quality of fresh chilled pork. The thermal history of refrigerated fresh pork could be reflected by the color change of the TTI.
Xu et al. (2017) established a tyrosinase-based TTI for determining the turbot sashimi quality using total plate counts. Park et al. (2013a) employed a prototype of LAB-based TTI to identify the quality changes of vacuum packaged chicken breast during storage. Wang et al. (2017b) developed a plasmonic thermal history indicator taking advantage of the localized surface plasmon resonance of gold nanoparticles (AuNPs) synthesized in situ in alginate. TTI color became more intense with increased storage temperature and time and change from gray to red with time of exposure at high temperature (40°C).
Singh et al. (2018a) developed temperature-sensitive packaging using a eutectic mixture of soybean oil and tetradecane as a thermo-regulating material. This temperature-sensitive packaging is useful to control the temperature of fresh beef from the store to preparation and consumption.
It is worth noting that there are some commercial TTI, small, light, fast, clear, accurate, efficient, easy to interpret, and cost effective for food packaging (Bizerba SE & Co.; DeltaTrak; Thermographic Measurements Ltd; Timestrip UK Ind).
A thermochromic ink is a specialized dynamic ink that changes its color when exposed to different temperatures. They are used to indicate thermal abuse and comfort conditions. Their activation temperatures go from low temperatures of refrigeration, happening through the temperature of the human body until high temperatures that surpass the threshold of pain. They can be activated by cold, by touch or by hot temperatures. Those activated by touch become transparent when are rubbed or touched to reveal an image or other color printed below. Some of them are based on the use of liquid crystals that change their color within the visible spectrum (Vanderroost et al., 2014).
Their color change can be irreversible or reversible. Irreversible ones are colorless until they are exposed to a certain temperature at which an intense color develops and remains constant or changes leaving a permanent mark that indicates the temperature change (Roya and Elham, 2016). They are used as indicators of thermal abuse to show that the storage temperature of a product exceeded a recommended value, for example that the cold chain was broken in a refrigerated product. Therefore, they can be considered as a TTI of partial thermal history. On the other hand, reversible thermochromic inks change their color when they heat up and return to their original color when temperature decreases or vice versa. They are used to indicate comfort situations, for example, to ensure consumers that a drink in a container is perfectly refrigerated or to warn consumers that a package in the microwave has reached the desired temperature or is too hot (Vanderroost et al., 2014). In this sense, Musso et al. (2017a) developed comfort indicators based on gelatin films and different thermochromic inks, whose coloration changes reversibly at different temperatures (15 and 33°C) according to the inks nature.
Recently, Liu and Watts (2019) patented articles and methods for detecting heating patterns within model food compositions containing irreversible thermochromic ink. The irreversible thermochromic inks can exhibit a variable change in at least one-color parameter in response to temperature change across a selected temperature range.
They are used to detect, register and transmit information regarding biological reactions (Yam et al., 2005). In food packaging they can control the freshness of the products, reducing food waste and food-borne illnesses risks (Park et al., 2015).
Biosensors contain bio-receptors (biological or organic materials) that recognizes the desired analyte and transducers (optical, calorimetric, electrochemical, or piezoelectric devices) that turn the biochemical signals into a quantifiable electronic reply (Ahmed et al., 2018). For example, Food Sentinel System (SIRA Technologies Inc.) is a commercial biosensor developed to detect food pathogens with a specific antibody joined to a membrane that is part of a bar code. In the presence of pathogens, a band of dark coloration is formed which prevents the bar code from being read (Yam et al., 2005). ToxinGuard® (Toxin Alert, Canada) is a commercial visual diagnostic that displays antibodies imprinted on a polyethylene-based material that detects specific pathogenic microorganisms such as such as Salmonella spp., Campylobacter spp., E coli., Listeria spp. (Poyatos-Racionero et al., 2018).
Nanotechnology arrival has revolutionized this field of action, and new and more effective nanobiosensors are being developed in order to detect pathogens, chemical polluters, spoilage, product handling, and follow-up of ingredients or products through processing (Srivastava et al., 2018).
For non-destructive evaluation of the aroma of various foods, colorimetric sensors are used. Volatile components react with chemical colorants causing color change and subsequent detection of chemical vapors (Ahmed et al., 2018).
Chen et al. (2017a) reused the food barcode and turned it into a colorimetric sensor integrated with a camera, to sense the state of packaged products and indicate the end of their useful life. This colorimetric sensor is composed of vapor sensitive colorants encapsulated in resin microbeads. With this device it was possible to monitor the deterioration of chicken meat in different temperature ranges.
Hypoxanthine (produced by the metabolic degradation of ATP) and trimethylamine oxide (that degrades to trimethylamine increasing the fishy odor) are ones of the main freshness indicators in fish products (Ashie et al., 1996; Park et al., 2013b; Mustafa and Andreescu, 2018). To detect the amount of hypoxanthine and xanthine, several enzymatic biosensors based on a colorimetric or electrochemical reaction using the enzyme xanthine oxidase have been investigated (Lawal and Adeloju, 2012; Chen et al., 2017b) immobilized on silver, graphite, and platinum electrodes (Devi et al., 2013; Dervisevic et al., 2016). Yan et al. (2017) reported a colorimetric sensor for xanthine detection using a copper nanocluster with peroxidase-like activity, while Chen et al. (2017b) developed a multicolor sensor for hypoxanthine detection by using gold nanorods.
Schaude et al. (2017) developed a colorimetric system for the detection of trimethylamine oxide based on pH indicator dyes immobilized on cellulose microparticles embedded into food-grade silicone and were safely integrated into food packaging, that change color from green to red when the food is spoiled.
Gluten is another interesting component that can be detected, since some people are intolerant of this ingredient, which can cause serious disorders of the digestive system (Malvano et al., 2017). It is usually detected by the conventional enzyme-linked immunosorbent assay (Nassef et al., 2008). White et al. (2018) developed an electronic sensor based on antibodies that were used as selective receptors to bind wheat and barley gluten.
Biosensors are a good choice for the detection of pathogenic microorganisms due to their portability and potential for in situ detection. Most of these biosensors are based on immunological or DNA recognition, but they have the disadvantage that the preparation procedures are time consuming and require labeling and specialized facilities (Palumbo et al., 2003; Cai et al., 2014; Mustafa and Andreescu, 2018). A variant that has been proposed to avoid the aforementioned drawbacks is to use synthetic antimicrobial peptides as recognition agents, which ensure the detection and quantification of pathogens such as Staphylococcus aureus, Escherichia coli, Pseudomonas aeruginosa, and Staphylococcus epidermidis (Liu et al., 2016). Synthetic antimicrobial peptides are inexpensive, can be produced on a large scale and have high stability and furthermore, they could also be used to inactivate pathogens. The development of colorimetric biosensor strips based on immobilized peptides that were used for the detection of Listeria monocytogenes in milk and meat samples has been reported (Alhogail et al., 2016). Recently, a fluorescent DNAzyme probe that specifically binds E. coli was developed and printed on a cyclo-olefin polymer transparent package with meat and apple-juice samples (Yousefi et al., 2018a).
Over the past few years, researchers have attempted to create bacteria-detecting devices easy to read that required minimal user intervention; however, most of them are destructive and require to open the food package for analysis. Some authors developed lateral-flow strip assays (Yang et al., 2016b; Ramos et al., 2017; Tominaga, 2017, 2018) based on antibodies labeled to colloidal gold or palladium nanoparticles (PdNPs) applied to a test strip. This process takes approximately 15 min and does not require special equipment and has been used to detect Listeria monocytogenes and bacteria in the Klebsiella group (Tominaga, 2017, 2018).
Portable microfluidic devices were also developed for bacterial detection. Thus, Tokel et al. (2015) created a device that has antibodies capable of responding to antigens found on the surface of Escherichia coli.
Bright-field imaging allowed to visualize bacteria accumulation. Thus, Altintas et al. (2018) used a mechanism similar to that described above, but also incorporated sensor chips that allow electrochemical detection in real time. Other devices included E. coli sensors on graphene based flexible acetate sheets, that used electrodes as the main method of detection (Basu et al., 2014).
DuVall et al. (2015) created a phone application to calculate bacteria's presence based on paramagnetic silica beads aggregation. Cells were first lysed, the target DNA strand was then amplified through loop-mediated isothermal amplification, subsequently causing beads aggregation.
Techniques such as surface functionalization, microcontact printing and automated printers have been extensively used to target cells detection (Didar et al., 2015; Shakeri et al., 2017; Yousefi et al., 2018a). Recently, Yousefi et al. (2018b) created a simple E. coli-detecting device based on the use of DNAzymes capable of performing a specific reaction, that could be incorporated into food packaging.
Gas detection within the package has acted as a driving force in the development of many biosensors (Meng et al., 2014; Banerjee et al., 2016). Luminescence-based optical fiber O2 sensor had been fabricated via layer-by-layer nanoassembly technique. The resulting sensor was enhanced by tuning the spacing between fluorophore layers by the introduction of poly(acrylic acid) (de Acha et al., 2017). Mao et al. (2017) developed a new optical O2 sensor using microstructured pillar detection layer arrays, which improves sensitivity (detection in the nanometric range). Likewise, Lee and Park (2017) also observed highly improved sensitivity using luminescent O2 sensors based on porous sensing films with augmented photoluminescence and O2 accessibility. Antropov et al. (2018) proposed a new material associating the O2 permeability of fluorinated polymers with an even dissemination of the photostable dye on the surface. The mesoporous structures allowed the determination of O2 at a very low level as well as avoided dye leaching and microbiological fouling.
CO2 sensors are being of great interest in detecting spoilage in food. There are optochemical detection techniques involving a colorimetric pH indicator and a phosphorescent dye (Neethirajan et al., 2009; Puligundla et al., 2012; Lochman et al., 2017). There are several devices on the market that detect both dissolved and gaseous CO2, such as Severinghaus-type sensors and non-distributive infrared (NDIR). Their disadvantage is that they are large, expensive, and susceptible to contamination and water vapor, which is why cheaper and more compact sensors are preferred (Ahmed et al., 2018). Sun et al. (2016) developed a highly sensitive squaraine-based system for colorimetrically and fluorescently sense CO2 gas in dimethyl sulfoxide (DMSO). Xia et al. (2015) synthesized an unsymmetrical squaraine-based chemosensor for sensing CO2 gas by proton nuclear magnetic resonance and UV-visible spectroscopies in DMSO. Similarly, Sun et al. (2017) developed a CO2 squaraine-based cationic chemosensor that allows the change to be seen with the naked eye and is highly sensitive.
These devices are extremely sensitive to the products released by food when it deteriorates. Their sensing system could consist of a single device or an array of different sensing elements that produce time-dependent electrical signals in response to volatile components composition that then are analyzed by pattern recognition system (Kodogiannis, 2017; Szulczyński et al., 2017; Wojnowski et al., 2017). There are also other types of sensors, in addition to conductimetrics ones, such as surface acoustic wave sensors, chemoresistor sensors, quartz crystal microbalance, and optical sensors for detecting odors (Alizadeh, 2010; Papadopoulou et al., 2013; Verma and Yadava, 2015; Zhao et al., 2016).
Multivariate statistics and artificial neuron networks are used as statistical tools for data analysis, and the results are then compared with the reference methods data based on chemical determination of spoilage indicators or sensory and microbiological analyses (Salinas et al., 2014; Górska-Horczyczak et al., 2016). Specifically, electronic noses interact with volatile compounds present in the container headspace, while electronic tongues react with non-volatile compounds dispersed in a liquid. These instruments could be packaged to provide data on quality and safety controls (Zhong, 2019).
The electronic nose, designed to simulate human olfactory sense, should distinguish complex odors via an array of sensors. The common e-nose consisted of an array of gas sensors, an analog to digital converter, and a computer equipped with a pattern recognition algorithm (Ali et al., 2020). This technology has been successfully used to detect food additives in fruit juices (Qiu and Wang, 2017) and fruit spoilage (Wen et al., 2019). Chen et al. (2019b) used the electronic nose to monitor the freshness of fresh-cut broccoli during refrigerated storage, while Tan and Kerr (2019) use it for monitor the volatile compounds of cocoa samples undergoing refining. It has been also used to determine meat freshness of refrigerated, frozen, and chill-stored pork meat (Górska-Horczyczak et al., 2017; Ramírez et al., 2018).
The electronic tongue has shown its potential to be used as a complementary tool with human tasters in the sensor technology field because of fast, highly sensitive and selective methods. It is an analytical sensory array unit that can detect specific substances by different artificial membranes and electrochemical techniques (Titova and Nachev, 2020). The sensor array responds to the liquid sample and outputs a signal that is then processed by the computer system and patterned by the recognition system. Subsequently, the sample taste characteristics are obtained (Wang and Liu, 2019). Electronic tongues have found a wide range of applications for different food products such as wines (Wang et al., 2019a), fruit flavors (Németh et al., 2019; Zhu et al., 2020), coffee (Arrieta et al., 2019), and milk (Pan et al., 2019).
Although these electronics have been developed for smart packaging, current devices are usually complicated and expensive. Therefore they are not yet ready to be integrated with real packages (Zou et al., 2015; Poyatos-Racionero et al., 2018).
RFID tags use electromagnetic fields of radiofrequency to store and communicate product information in real time for their identification and traceability (Lee and Rahman, 2014; Magalhães et al., 2019). They consist of an integrated circuit connected to an antenna for the transmission of information stored on the chip to a reader. They could be basically active or passive, depending on battery requirement (Kalpana et al., 2019). Active types use the battery as power sources and passive type generate power from signals received. Generally, low and high frequency (125–134 or 13.56 MHz) ranges are used in food packaging applications (Bibi et al., 2017). The functions of RFID are to collect RF energy from the interrogator with its antenna, activate the RFID chip in the tag, and transmit an ID code back to the interrogator, being the ID code a fixed number used as a product unique identifier (Cui et al., 2019). The reader is a small component whose primary function is to serve as a platform for analyzing and receiving information from the tag for further processing. Electronic chips are used for encoding information in the form of bits in the Electronic Product Code (Bibi et al., 2017). RFID middleware, as the vital part, performs several functions like filtering, integrating of data, coordination of reader and proper management of scheduled processes (Chen et al., 2008).
The use of RFID systems is very important for traceability and food chain monitoring, as storage time and temperature are the two main factors affecting food quality (Dobrucka and Przekop, 2019). Main advantages of RFID on “bar codes” are that they allow remote control, store different information (origin, process parameters, commercial information, etc.), and multiple elements monitoring at the same time allowing a unique identification of the product (Kuswandi et al., 2011).
There are many companies manufacturing RFID labels, which have been used during years in high value products, such as electronics or clothing. In the food industry, these devices can be attached to a container or box, thus they can be identified and tracked. There is a series of RFID suppliers such as EPSILIA (Canada), RFID Enabled Solutions Inc. (USA) and HRAFN Ltd. (Sweden), which have worked together with meat and fish industries to implement RFID systems (Fuertes et al., 2016a).
The most evolved RFID systems allow the integration of other functions in the label, such as TTI or biosensors to monitor and communicate the thermal history of the product, as well as to provide information about quality. Numerous advances have been achieved in this field, for example, the development of a pH sensor incorporated in a radiofrequency transmitter without batteries to monitor in situ spoilage processes of fishing products (Huang et al., 2011); RFID labels to control meat freshness (Eom et al., 2014) and traceability (Cappai et al., 2018). RFID labels with an O2 indicator could be used in MAP systems (Martínez-Olmos et al., 2013) and as pork freshness sensors (Sen et al., 2013). Feng et al. (2014) and Fernandez-Salmeron et al. (2015) proposed the application of a humidity sensor integrated in a fully printed RFID tags for varying humidity levels. Lorite et al. (2017) developed a novel and functional critical temperature indicator-smart sensor which combines irreversible visual color changes and RFID technologies.
RFID labels with CO2 and O2 sensors were developed to control vegetables freshness (Eom et al., 2012; Potyrailo et al., 2012) and the quality and safety of dairy products (Zhang et al., 2020).
Figure 8 resumes the classification of smart food packaging described in this review.
Food packaging represents about half of all packaging area in developed countries, with a reported market size of USD 304.98 billion in 2019 that is projected to reach USD 463.65 billion by 2027, growing at a CAGR of 5.9% (Food Packaging Market - Fortune Business Insights), while smart packaging is expected to grow at a CAGR of 8.3% during the same period, starting from USD 6.87 Bn in 2018 (Smart Packaging Market - Credence Research). However, food and beverage is the largest sector for smart packaging (Smart Packaging Market 2018-2023 – OpenPR; de Oliveira and de Melo, 2019) and currently, it is estimated that within the global market, most of the smart packaging corresponds to active packaging (with MAPs showing the greatest growth in the market) (ReportLinker).
The increasing demand for fresh and quality packaged food, consumer convenience and manufacturers' concern for longer shelf-life of the food products are driving the market for global active and intelligent packaging technology for food and beverage market. These technologies offer tremendous potential to fulfill the growing demand of food safety in various applications which include dairy products, meat and poultry, ready-to-eat meal segment, etc. Smart packaging's advantages over their conventional counterparts are reduced counterfeiting, increased shelf-life, improved safety, and easy implementation (Sohail et al., 2018). Although many of these products have been considered unnecessary, the development of technology that allows the low-cost production of electronics and sensors and the elimination of specialized electronic readers in favor of smart-phones (adopting QR codes) will allow them to enter into this tendency (Industry Market Research - Freedonia Group; Fuertes et al., 2016a).
Market's growth has also evolved due to the exploration of nanotechnology (Smart Packaging Market - Mordor Intelligence; He et al., 2019). Nanomaterials have various applications; both in active and intelligent packaging. In active packaging, nanostructures can enhance plastics permeabilities to gases and flavors, and can also be used to improve food safety and fortification, as to improve bioavailability, processing, and encapsulation of active compounds (Pereda et al., 2018). In intelligent packages, nanosensors improve the rate, sensitivity and specificity of the detection of pathogens, toxins, and chemicals, and incorporated inside the packaging, they can inform the consumers about food's freshness level and nutrition status (Fuertes et al., 2016b; Caon et al., 2017).
Furthermore, developments in printing practices are providing their added functionality for the potential development of intelligent packaging and smart labels, associated with the use of RFID technology and printed electronics (Smart Packaging Market - Mordor Intelligence; Liao et al., 2019).
Moreover, the use of new technologies such as thermo and photochromic inks, oxygen scavenging, electronic labels and RFID by packaging industries would also increase the market (Smart Packaging Market - Credence Research).
Although, high research and development cost could be expected to hamper the growth of the market in coming years, important companies such as 3M Company, Temp Time Corporation, PakSense, American Thermal Instruments, Avery Dennison, R.R. Donnelly Sons & Company, BASF SE, International Paper, Stora Enso, Huhtamaki Group and Smartrac N.V., are currently included in the global smart packaging market (Smart Packaging Market 2018-2023 – OpenPR).
Food smart packaging should be highly regulated with strict guidelines for packaging materials, testing, and labeling (Madhusudan et al., 2018; Drago et al., 2020). The most important regulation is to obtain food contact approval. Even though legislations about food contact material vary between countries, regulations are mainly based on restrictions as to the material compositions or migration limits. Package's components, whether the bioplastic or the additives added to give the container a particular functionality, should not migrate into food in quantities that could be a risk to human health or change its sensory properties (Restuccia et al., 2010; Drago et al., 2020).
On the other hand, the labeling of the components of smart packaging, such as the contents of the envelopes and sachets (i.e., O2 removal sachets) should be provided, clearly mentioning “do not eat” on the labels (Hurme et al., 2002). But the most important concern in the use of sachets is the accidental leakage of the components within the food package that can spoil the food and be a threat to the consumer. Thus, efforts are aimed at replacing the use of envelopes in the package by directly fusing the active components within or on the package (Restuccia et al., 2010).
Another aspect to consider is the environmental regulations. These regulations cover the use, reuse, recycling, and identification of packaging material to guarantee the recycling or energy recovery of the components of the materials. For end-of-life management of food and waste, industries in the USA follow the US EPA's Waste Reduction Model (WARM; version 13) [US EPA (U.S. Environmental Protection Agency), 2015]. The companies under European Union are required to make sure the environmental influence of their packaging operations as given in the Packaging and Packaging Waste Directive (European Commission, 2018).
Finally, it is necessary to consider and evaluate the possible risks that the antimicrobial agents used in smart packaging technologies may produce due to the possible development of antimicrobial resistance. This can cause an alteration in the microbial ecology and put food security at risk (Restuccia et al., 2010; Ahmed et al., 2017).
Smart packaging has great possibilities to food and beverage industry, giving response to consumers that demand for convenient packages that ensure products quality and safety, with a longer shelf- life. Nowadays, their development at industrial level is focused on the use of synthetic plastic materials because they are economical, functional, light and very versatile. However, the interest in replacing these polymers by bioplastics tries to meet the society's demands regarding environmental care and sustainability.
Over the last years, much progress has been achieved in improving the behavior of bioplastics, through modifications of biopolymers functionality by physical, chemical and/or enzymatic treatments; by addition of additives that modify mechanisms of materials formation or extend their functionality, by modifications in the processing conditions and technologies, by applying post-treatments after materials formation, by using polymer blends or preparing multilayer materials, composite and nanocomposites. The resulting materials properties are suitable for numerous applications in smart food packaging. However, most of these materials are not commercialized yet, possibly because a great part of the studies is made at a laboratory scale. Therefore, it is essential to study and advance in the scaling of the processing of these materials, as well as to evaluate their behavior as real food packaging systems in order to achieve a real application.
A better understanding of interactions, regulation and safety issues and proper design will certainly increase consumer confidence in this technology. Future studies should also be focalized on complete product evaluation, ensuring that the developed packaging systems are suitable to be in contact with food. Natural bioactive agents have been extensively explored as antimicrobial/antioxidant packaging and are currently preferred (Aziz and Karboune, 2018; Zanetti et al., 2018; Jamróz and Kopel, 2020). A huge challenge in the research area of the quality indicators is to find the indicators that are sensitive (ppm–ppb levels) and specific, as most of the current pH-based indicators lack these properties. Recent studies have explored the ability of biopolymer-based food packaging materials to carry, encapsulate and control-release these bioactive compounds (Chalier et al., 2019; Almasi et al., 2020). The current research trends are moving toward employment of emerging technologies such as nanotechnology packaging and antimicrobial packaging to facilitate effective incorporation of bioactive ingredients and enhance designed functions (Sharma et al., 2017; da Silva Barbosa and dos Santos Rosa, 2019; Salgado et al., 2019b; Zhong et al., 2020).
The next level of active and intelligent packaging should be the bioactive and bio-intelligent packaging. These novel packaging will open new frontiers and opportunities for preserving the quality of food products and in monitoring the status of product quality, saving fossil resources through the use of biomass that regenerates and provides the unique potential for carbon neutrality. The biodegradability of certain types of bioplastics also offers the advantage a potential solution for controlling food waste as well as preventing plastic packaging from leaking into the environment (Dilkes-Hoffman et al., 2018; Kakadellis and Harris, 2020).
All authors contributed to the design and writing of this manuscript, as well as to the bibliographic search used for its completion.
The authors would like to thank the National Agency of Scientific and Technological Support of Argentina (ANPCyT, PICT-2013-2124, PICT-2015-2822, and PICT-2018-4040), and La Plata National University (UNLP, Project 2016-11/X750, and 2020-11/X923) for their financial support.
The authors declare that the research was conducted in the absence of any commercial or financial relationships that could be construed as a potential conflict of interest.
Aadil, K. R., Prajapati, D., and Jha, H. (2016). Improvement of physcio-chemical and functional properties of alginate film by Acacia lignin. Food Packag. Shelf Life 10, 25–33. doi: 10.1016/j.fpsl.2016.09.002
Adilah, Z. A. M., Jamilah, B., and Hanani, Z. A. N. (2018). Functional and antioxidant properties of protein-based films incorporated with mango kernel extract for active packaging. Food Hydrocoll. 74, 207–218. doi: 10.1016/j.foodhyd.2017.08.017
Ahmed, I., Lin, H., Zou, L., Brody, A. L., Li, Z., Qazi, I. M., et al. (2017). A comprehensive review on the application of active packaging technologies to muscle foods. Food Control 82, 163–178. doi: 10.1016/j.foodcont.2017.06.009
Ahmed, I., Lin, H., Zou, L., Li, Z., Brody, A. L., Qazi, I. M., et al. (2018). An overview of smart packaging technologies for monitoring safety and quality of meat and meat products. Packag. Technol. Sci. 31, 449–471. doi: 10.1002/pts.2380
Alhogail, S., Suaifan, G. A. R. Y., and Zourob, M. (2016). Rapid colorimetric sensing platform for the detection of Listeria monocytogenes foodborne pathogen. Biosens. Bioelectron. 86, 1061–1066. doi: 10.1016/j.bios.2016.07.043
Ali, M. M., Hashim, N., Abd Aziz, S., and Lasekan, O. (2020). Principles and recent advances in electronic nose for quality inspection of agricultural and food products. Trends Food Sci. Technol. 99, 1–10. doi: 10.1016/j.tifs.2020.02.028
Alizadeh, T. (2010). Chemiresistor sensors array optimization by using the method of coupled statistical techniques and its application as an electronic nose for some organic vapors recognition. Sensors Actuators B Chem. 143, 740–749. doi: 10.1016/j.snb.2009.10.018
Almasi, H., Jahanbakhsh Oskouie, M., and Saleh, A. (2020). A review on techniques utilized for design of controlled release food active packaging. Crit. Rev. Food Sci. Nutr. 1–21. doi: 10.1080/10408398.2020.1783199
Al-Tayyar, N. A., Youssef, A. M., and Al-Hindi, R. (2020). Antimicrobial food packaging based on sustainable bio-based materials for reducing foodborne Pathogens: a review. Food Chem. 310:125915. doi: 10.1016/j.foodchem.2019.125915
Altintas, Z., Akgun, M., Kokturk, G., and Uludag, Y. (2018). A fully automated microfluidic-based electrochemical sensor for real-time bacteria detection. Biosens. Bioelectron. 100, 541–548. doi: 10.1016/j.bios.2017.09.046
Álvarez-Hernández, M. H., Artés-Hernández, F., Ávalos-Belmontes, F., Castillo-Campohermoso, M. A., Contreras-Esquivel, J. C., Ventura-Sobrevilla, J. M., et al. (2018). Current scenario of adsorbent materials used in ethylene scavenging systems to extend fruit and vegetable postharvest life. Food Bioprocess Technol. 11, 511–525. doi: 10.1007/s11947-018-2076-7
Amin, E. M., Bhuiyan, M. S., Karmakar, N. C., and Winther-Jensen, B. (2013). Development of a low cost printable chipless RFID humidity sensor. IEEE Sens. J. 14, 140–149. doi: 10.1109/JSEN.2013.2278560
Amorati, R., Foti, M. C., and Valgimigli, L. (2013). Antioxidant activity of essential oils. J. Agric. Food Chem. 61, 10835–10847. doi: 10.1021/jf403496k
Andrade, M. A., Ribeiro-Santos, R., Bonito, M. C. C., Saraiva, M., and Sanches-Silva, A. (2018). Characterization of rosemary and thyme extracts for incorporation into a whey protein based film. LWT 92, 497–508. doi: 10.1016/j.lwt.2018.02.041
Antropov, A. P., Ragutkin, A. V., Melnikov, P. V., Luchnikov, P. A., and Zaitsev, N. K. (2018). “Composite material for optical oxygen sensor,” in IOP Conference Series: Materials Science and Engineering (Tomsk: IOP Publishing), 12031.
Arfat, Y. A., Ahmed, J., Hiremath, N., Auras, R., and Joseph, A. (2017). Thermo-mechanical, rheological, structural and antimicrobial properties of bionanocomposite films based on fish skin gelatin and silver-copper nanoparticles. Food Hydrocoll. 62, 191–202. doi: 10.1016/j.foodhyd.2016.08.009
Arrieta, A. A., Arrieta, P. L., and Mendoza, J. M. (2019). Analysis of coffee adulterated with roasted corn and roasted soybean using voltammetric electronic tongue. Acta Sci. Pol. Technol. Aliment. 18, 35–41. doi: 10.17306/J.AFS.2019.0619
Ashie, I. N. A., Smith, J. P., Simpson, B. K., and Haard, N. F. (1996). Spoilage and shelf-life extension of fresh fish and shellfish. Crit. Rev. Food Sci. Nutr. 36, 87–121. doi: 10.1080/10408399609527720
Assis, R. Q., Lopes, S. M., Costa, T. M. H., Flôres, S. H., and de Rios, A. O. (2017). Active biodegradable cassava starch films incorporated lycopene nanocapsules. Ind. Crops Prod. 109, 818–827. doi: 10.1016/j.indcrop.2017.09.043
Azevedo, V. M., Dias, M. V., de Siqueira Elias, H. H., Fukushima, K. L., Silva, E. K., de Deus Souza Carneiro, J., et al. (2018). Effect of whey protein isolate films incorporated with montmorillonite and citric acid on the preservation of fresh-cut apples. Food Res. Int. 107, 306–313. doi: 10.1016/j.foodres.2018.02.050
Aziz, M., and Karboune, S. (2018). Natural antimicrobial/antioxidant agents in meat and poultry products as well as fruits and vegetables: a review. Crit. Rev. Food Sci. Nutr. 58, 486–511. doi: 10.1080/10408398.2016.1194256
Aziz, S. G.-G., and Almasi, H. (2018). Physical characteristics, release properties, and antioxidant and antimicrobial activities of whey protein isolate films incorporated with thyme (Thymus vulgaris L.) extract-loaded nanoliposomes. Food Bioprocess Technol. 11, 1552–1565. doi: 10.1007/s11947-018-2121-6
Banerjee, S., Kelly, C., Kerry, J. P., and Papkovsky, D. B. (2016). High throughput non-destructive assessment of quality and safety of packaged food products using phosphorescent oxygen sensors. Trends Food Sci. Technol. 50, 85–102. doi: 10.1016/j.tifs.2016.01.021
Barbosa-Pereira, L., Cruz, J. M., Sendón, R., de Quirós, A. R. B., Ares, A., Castro-López, M., et al. (2013). Development of antioxidant active films containing tocopherols to extend the shelf life of fish. Food Control 31, 236–243. doi: 10.1016/j.foodcont.2012.09.036
Bastarrachea, L. J., Wong, D. E., Roman, M. J., Lin, Z., and Goddard, J. M. (2015). Active packaging coatings. Coatings 5, 771–791. doi: 10.3390/coatings5040771
Basu, P. K., Indukuri, D., Keshavan, S., Navratna, V., Vanjari, S. R. K., Raghavan, S., et al. (2014). Graphene based E. coli sensor on flexible acetate sheet. Sens. Actuat. B Chem. 190, 342–347. doi: 10.1016/j.snb.2013.08.080
Belay, Z. A., Caleb, O. J., and Opara, U. L. (2016). Modelling approaches for designing and evaluating the performance of modified atmosphere packaging (MAP) systems for fresh produce: a review. Food Packag. Shelf Life 10, 1–15. doi: 10.1016/j.fpsl.2016.08.001
Belibi, P. C., Daou, T. J., Ndjaka, J.-M. B., Michelin, L., Brendlé, J., Nsom, B., et al. (2013). Tensile and water barrier properties of cassava starch composite films reinforced by synthetic zeolite and beidellite. J. Food Eng. 115, 339–346. doi: 10.1016/j.jfoodeng.2012.10.027
Bessa, R. A., de Oliveira, L. H., Arraes, D. D., Batista, E. S., Nogueira, D. H., Silva, M. S., et al. (2015). Starch and starch/zeolite films used in coating and conservation of guava fruit (Psidium guajava). Rev. Virtual Quim. 7, 2190–2201. doi: 10.5935/1984-6835.20150130
Bhadra, S., Narvaez, C., Thomson, D. J., and Bridges, G. E. (2015). Non-destructive detection of fish spoilage using a wireless basic volatile sensor. Talanta 134, 718–723. doi: 10.1016/j.talanta.2014.12.017
Bhargava, N., Sharanagat, V. S., Mor, R. S., and Kumar, K. (2020). Active and intelligent biodegradable packaging films using food and food waste-derived bioactive compounds: a review. Trends Food Sci. Technol. 105, 385–401. doi: 10.1016/j.tifs.2020.09.015
Bibi, F., Guillaume, C., Gontard, N., and Sorli, B. (2017). A review: RFID technology having sensing aptitudes for food industry and their contribution to tracking and monitoring of food products. Trends Food Sci. Technol. 62, 91–103. doi: 10.1016/j.tifs.2017.01.013
Biegańska, M. (2017). Shelf-life monitoring of food using time-temperature indicators (TTI) for application in intelligent packaging. Towarozn. Probl. Jakości 2, 75–85. doi: 10.19202/j.cs.2017.02.07
Biji, K. B., Ravishankar, C. N., Mohan, C. O., and Gopal, T. K. S. (2015). Smart packaging systems for food applications: a review. J. Food Sci. Technol. 52, 6125–6135. doi: 10.1007/s13197-015-1766-7
Bizerba SE & Co. Onvu. Available online at: http://www.onvu.com/ (accessed March 2021).
Blanco-Pascual, N., Alemán, A., Gómez-Guillén, M. C., and Montero, M. P. (2014). Enzyme-assisted extraction of κ/ι-hybrid carrageenan from Mastocarpus stellatus for obtaining bioactive ingredients and their application for edible active film development. Food Funct. 5, 319–329. doi: 10.1039/C3FO60310E
Bonilla, J., Poloni, T., Lourenço, R. V., and Sobral, P. J. A. (2018). Antioxidant potential of eugenol and ginger essential oils with gelatin/chitosan films. Food Biosci. 23, 107–114. doi: 10.1016/j.fbio.2018.03.007
Bonomo, R. C. F., Santos, T. A., Santos, L. S., da Costa Ilhéu Fontan, R., Rodrigues, L. B., dos Santos Pires, A. C., et al. (2018). Effect of the incorporation of lysozyme on the properties of jackfruit starch films. J. Polym. Environ. 26, 508–517. doi: 10.1007/s10924-016-0902-4
Boscher, N. D., Bohn, T., Heier, P., Moisy, F., Untereiner, B., Heinze, K., et al. (2014). Optical sensing responses of CrIIICl (TPP)(H2O)-based coatings obtained by an atmospheric pressure plasma method–application to the detection of volatile amines. Sens. Actuat. B Chem. 191, 553–560. doi: 10.1016/j.snb.2013.10.044
Bouarab Chibane, L., Degraeve, P., Ferhout, H., Bouajila, J., and Oulahal, N. (2019). Plant antimicrobial polyphenols as potential natural food preservatives. J. Sci. Food Agric. 99, 1457–1474. doi: 10.1002/jsfa.9357
Brandelli, A., Brum, L. F. W., and dos Santos, J. H. Z. (2017). Nanostructured bioactive compounds for ecological food packaging. Environ. Chem. Lett. 15, 193–204. doi: 10.1007/s10311-017-0621-7
Brockgreitens, J., and Abbas, A. (2016). Responsive food packaging: recent progress and technological prospects. Compr. Rev. Food Sci. Food Saf. 15, 3–15. doi: 10.1111/1541-4337.12174
Byun, Y., Bae, H. J., and Whiteside, S. (2012). Active warm-water fish gelatin film containing oxygen scavenging system. Food Hydrocoll. 27, 250–255. doi: 10.1016/j.foodhyd.2011.06.010
Byun, Y., and Whiteside, S. (2012). Ascorbyl palmitate-β-cyclodextrin inclusion complex as an oxygen scavenging microparticle. Carbohydr. Polym. 87, 2114–2119. doi: 10.1016/j.carbpol.2011.10.037
Cai, H. Y., Caswell, J. L., and Prescott, J. F. (2014). Nonculture molecular techniques for diagnosis of bacterial disease in animals: a diagnostic laboratory perspective. Vet. Pathol. 51, 341–350. doi: 10.1177/0300985813511132
Caon, T., Martelli, S. M., and Fakhouri, F. M. (2017). “New trends in the food industry: application of nanosensors in food packaging,” in Nanobiosensors, ed A. M. Grumezescu (Academic Press), 773–804.
Cappai, M. G., Rubiu, N. G., and Pinna, W. (2018). Economic assessment of a smart traceability system (RFID+ DNA) for origin and brand protection of the pork product labelled “suinetto di Sardegna.” Comput. Electron. Agric. 145, 248–252. doi: 10.1016/j.compag.2018.01.003
Cazón, P., Velazquez, G., Ramírez, J. A., and Vázquez, M. (2017). Polysaccharide-based films and coatings for food packaging: a review. Food Hydrocoll. 68, 136–148. doi: 10.1016/j.foodhyd.2016.09.009
Chalier, P., Ruiz, T., and Gastaldi, E. (2019). Active food packaging based on biopolymer and aroma compounds: how to design and control the release. Front. Chem. 7:398. doi: 10.3389/fchem.2019.00398
Chand, K., and Kumar, S. (2018). Effect of moisture absorber and packaging materials on quality parameters of Jaggery cubes. IJCS 6, 1398–1404.
Chen, H., Zhang, M., Bhandari, B., and Guo, Z. (2018). Applicability of a colorimetric indicator label for monitoring freshness of fresh-cut green bell pepper. Postharvest Biol. Technol. 140, 85–92. doi: 10.1016/j.postharvbio.2018.02.011
Chen, H., Zhang, M., Bhandari, B., and Yang, C. (2019a). Development of a novel colorimetric food package label for monitoring lean pork freshness. LWT 99, 43–49. doi: 10.1016/j.lwt.2018.09.048
Chen, H., Zhang, M., and Guo, Z. (2019b). Discrimination of fresh-cut broccoli freshness by volatiles using electronic nose and gas chromatography-mass spectrometry. Postharvest Biol. Technol. 148, 168–175. doi: 10.1016/j.postharvbio.2018.10.019
Chen, R.-S., Chen, C. C., Yeh, K. C., Chen, Y.-C., and Kuo, C. W. (2008). Using RFID technology in food produce traceability. WSEAS Trans. Inf. Sci. Appl. 5, 1551–1560.
Chen, S., Wu, M., Lu, P., Gao, L., Yan, S., and Wang, S. (2020). Development of pH indicator and antimicrobial cellulose nanofibre packaging film based on purple sweet potato anthocyanin and oregano essential oil. Int. J. Biol. Macromol. 149, 271–280. doi: 10.1016/j.ijbiomac.2020.01.231
Chen, Y., Fu, G., Zilberman, Y., Ruan, W., Ameri, S. K., Zhang, Y. S., et al. (2017a). Low cost smart phone diagnostics for food using paper-based colorimetric sensor arrays. Food Control 82, 227–232. doi: 10.1016/j.foodcont.2017.07.003
Chen, Z., Lin, Y., Ma, X., Guo, L., Qiu, B., Chen, G., et al. (2017b). Multicolor biosensor for fish freshness assessment with the naked eye. Sens. Actuat. B Chem. 252, 201–208. doi: 10.1016/j.snb.2017.06.007
Choi, I., and Han, J. (2018). Development of a novel on–off type carbon dioxide indicator based on interactions between sodium caseinate and pectin. Food Hydrocoll. 80, 15–23. doi: 10.1016/j.foodhyd.2018.01.028
Chun, J.-Y., Choi, M.-J., Lee, S. J., and Hong, G.-P. (2013). Applications of time-temperature integrator (TTI) as a quality indicator of grounded pork patty. Korean J. Food Sci. An 33, 439–447. doi: 10.5851/kosfa.2013.33.4.439
Coban, H. B. (2020). Organic acids as antimicrobial food agents: applications and microbial productions. Bioprocess Biosyst. Eng. 43, 569–591. doi: 10.1007/s00449-019-02256-w
Çoban, O. E., Patir, B., Özpolat, E., and Kuzgun, N. K. (2016). Improving the quality of fresh rainbow trout by sage essential oil and packaging treatments. J. Food Saf. 36, 299–307. doi: 10.1111/jfs.12242
Coma, V. (2008). Bioactive packaging technologies for extended shelf life of meat-based products. Meat Sci. 78, 90–103. doi: 10.1016/j.meatsci.2007.07.035
Cui, L., Zhang, Z., Gao, N., Meng, Z., and Li, Z. (2019). Radio frequency identification and sensing techniques and their applications—a review of the state-of-the-art. Sensors 19:4012. doi: 10.3390/s19184012
da Rocha, M., Alemán, A., Romani, V. P., López-Caballero, M. E., Gómez-Guillén, M. C., Montero, P., et al. (2018). Effects of agar films incorporated with fish protein hydrolysate or clove essential oil on flounder (Paralichthys orbignyanus) fillets shelf-life. Food Hydrocoll. 81, 351–363. doi: 10.1016/j.foodhyd.2018.03.017
da Silva Barbosa, R. F., and dos Santos Rosa, D. (2019). New Trends of Antimicrobial Packaging Applying Nanotechnology. São Paulo.
Dainelli, D., Gontard, N., Spyropoulos, D., Zondervan-van den Beuken, E., and Tobback, P. (2008). Active and intelligent food packaging: legal aspects and safety concerns. Trends Food Sci. Technol. 19, S103–S112. doi: 10.1016/j.tifs.2008.09.011
Day, B. P. F. (2008). Active packaging of food. Smart Packag. Technol. fast Mov. Consum. Goods 1, 1–18. doi: 10.1002/9780470753699.ch1
de Acha, N., Elosúa, C., Matías, I. R., and Arregui, F. J. (2017). Enhancement of luminescence-based optical fiber oxygen sensors by tuning the distance between fluorophore layers. Sens. Actuat. B Chem. 248, 836–847. doi: 10.1016/j.snb.2016.12.081
de Araújo, G. K. P., de Souza, S. J., da Silva, M. V., Yamashita, F., Gonçalves, O. H., Leimann, F. V, et al. (2015). Physical, antimicrobial and antioxidant properties of starch-based film containing ethanolic propolis extract. Int. J. Food Sci. Technol. 50, 2080–2087. doi: 10.1111/ijfs.12869
De La Puerta, M. C. N., Bartolome, L. G., and Jarabo, C. S. (2014). Smart packaging for detecting microorganisms. U.S. Patent No. 8,741,596. Washington, DC: U.S. Patent and Trademark Office.
de Moraes Crizel, T., Costa, T. M. H., de Oliveira Rios, A., and Flôres, S. H. (2016). Valorization of food-grade industrial waste in the obtaining active biodegradable films for packaging. Ind. Crops Prod. 87, 218–228. doi: 10.1016/j.indcrop.2016.04.039
de Oliveira Filho, J. G., Rodrigues, J. M., Valadares, A. C. F., de Almeida, A. B., de Lima, T. M., Takeuchi, K. P., et al. (2019). Active food packaging: alginate films with cottonseed protein hydrolysates. Food Hydrocoll. 92, 267–275. doi: 10.1016/j.foodhyd.2019.01.052
de Oliveira, A. S. B., and de Melo, N. R. (2019). Market and sustainability of food packaging: a review. Bol. do Cent. Pesqui. Process. Aliment. 36. doi: 10.5380/bceppa.v36i1.57846
DeltaTrak. WarmMark. Available online at: https://www.deltatrak.com/warmmark-time-temperature-indicator#overview (accessed March 2021).
Dervisevic, M., Dervisevic, E., Azak, H., Cevik, E., Senel, M., and Yildiz, H. B. (2016). Novel amperometric xanthine biosensor based on xanthine oxidase immobilized on electrochemically polymerized 10-[4H-dithieno (3, 2-b: 2′, 3′-d) pyrrole-4-yl] decane-1-amine film. Sens. Actuat. B Chem. 225, 181–187. doi: 10.1016/j.snb.2015.11.043
Devi, R., Batra, B., Lata, S., Yadav, S., and Pundir, C. S. (2013). A method for determination of xanthine in meat by amperometric biosensor based on silver nanoparticles/cysteine modified Au electrode. Process Biochem. 48, 242–249. doi: 10.1016/j.procbio.2012.12.009
Dey, A., and Neogi, S. (2019). Oxygen scavengers for food packaging applications: a review. Trends Food Sci. Technol. 90, 26–34. doi: 10.1016/j.tifs.2019.05.013
Di Giorgio, L., Salgado, P. R., and Mauri, A. N. (2018). Flavored oven bags for cooking meat based on proteins. LWT. 101, 374–381. doi: 10.1016/j.lwt.2018.11.002
Di Giorgio, L., Salgado, P. R., and Mauri, A. N. (2019). Encapsulation of fish oil in soybean protein particles by emulsification and spray drying. Food Hydrocoll. 87, 891–901. doi: 10.1016/j.foodhyd.2018.09.024
Di Maio, L., Scarfato, P., Avallone, E., Galdi, M. R., and Incarnato, L. (2014). Preparation and characterization of biodegradable active PLA film for food packaging. AIP Conf. Proc. 1593, 338–341. doi: 10.1063/1.4873795
Didar, T. F., Cartwright, M. J., Rottman, M., Graveline, A. R., Gamini, N., Watters, A. L., et al. (2015). Improved treatment of systemic blood infections using antibiotics with extracorporeal opsonin hemoadsorption. Biomaterials 67, 382–392. doi: 10.1016/j.biomaterials.2015.07.046
Dilkes-Hoffman, L. S., Lane, J. L., Grant, T., Pratt, S., Lant, P. A., and Laycock, B. (2018). Environmental impact of biodegradable food packaging when considering food waste. J. Clean. Prod. 180, 325–334. doi: 10.1016/j.jclepro.2018.01.169
Dobrucka, R., and Ankiel, M. (2019). Possible applications of metal nanoparticles in antimicrobial food packaging. J. Food Saf. 39:e12617. doi: 10.1111/jfs.12617
Dobrucka, R., and Cierpiszewski, R. (2014). Active and intelligent packaging food–research and development–a review. Polish J. Food Nutr. Sci. 64, 7–15. doi: 10.2478/v10222-012-0091-3
Dobrucka, R., and Przekop, R. (2019). New perspectives in active and intelligent food packaging. J. Food Process. Preserv. 43:e14194. doi: 10.1111/jfpp.14194
Ðordević, V., Balanč, B., Belščak-Cvitanović, A., Lević, S., Trifković, K., Kalušević, A., et al. (2015). Trends in encapsulation technologies for delivery of food bioactive compounds. Food Eng. Rev. 7, 452–490. doi: 10.1007/s12393-014-9106-7
Drago, E., Campardelli, R., Pettinato, M., and Perego, P. (2020). Innovations in smart packaging concepts for food: an extensive review. Foods 9:1628. doi: 10.3390/foods9111628
DuVall, J. A., Borba, J. C., Shafagati, N., Luzader, D., Shukla, N., Li, J., et al. (2015). Optical imaging of paramagnetic bead-DNA aggregation inhibition allows for low copy number detection of infectious pathogens. PLoS ONE 10:e0129830. doi: 10.1371/journal.pone.0129830
Eagland, D., and Crowther, N. (2007). Polymeric Materials Incorporating a Ph Indicator Dye. U.S. Patent Application No. 10/580,383.
East, A. R., Samarakoon, H. C., Pranamornkith, T., and Bronlund, J. E. (2015). A review of ethylene permeability of films. Packag. Technol. Sci. 28, 732–740. doi: 10.1002/pts.2137
Ebnesajjad, S. (2012). Handbook of Biopolymers and Biodegradable Plastics: Properties, Processing and Applications. Massachusetts, MA: William Andrew.
Eça, K. S., Sartori, T., and Menegalli, F. C. (2014). Films and edible coatings containing antioxidants - a review. Brazilian J. Food Technol. 17, 98–112. doi: 10.1590/bjft.2014.017
Echeverría, I., Eisenberg, P., and Mauri, A. N. (2014). Nanocomposites films based on soy proteins and montmorillonite processed by casting. J. Memb. Sci. 449, 15–26. doi: 10.1016/j.memsci.2013.08.006
Eghbal, N., Dumas, E., Yarmand, M. S., Mousavi, M. E., Oulahal, N., and Gharsallaoui, A. (2019). Antimicrobial films based on pectin and sodium caseinate for the release of antifungal natamycin. J. Food Process. Preserv. 43:e13953. doi: 10.1111/jfpp.13953
Ehsani, A., Hashemi, M., Aminzare, M., Raeisi, M., Afshari, A., Mirza Alizadeh, A., et al. (2019). Comparative evaluation of edible films impregnated with sage essential oil or lactoperoxidase system: impact on chemical and sensory quality of carp burgers. J. Food Process. Preserv. 43:e14070. doi: 10.1111/jfpp.14070
Emco Packaging. Oxygen Indicator Labels - Oxygen Indicating Color Change Chemistry. Available online at: https://www.emcotechnologies.co.uk/category/oxygen-indicator-labels (accessed March 2021).
Eom, K.-H., Hyun, K.-H., Lin, S., and Kim, J.-W. (2014). The meat freshness monitoring system using the smart RFID tag. Int. J. Distrib. Sens. Netw. 10:591812. doi: 10.1155/2014/591812
Eom, K. H., Kim, M. C., Lee, S., and Lee, C. W. (2012). The vegetable freshness monitoring system using RFID with oxygen and carbon dioxide sensor. Int. J. Distrib. Sens. Netw. 8:472986. doi: 10.1155/2012/472986
European Bioplastics Organization. Available online at: https://www.european-bioplastics.org/
Ezhilarasi, P. N., Karthik, P., Chhanwal, N., and Anandharamakrishnan, C. (2012). Nanoencapsulation techniques for food bioactive components: a review. Food Bioprocess Technol. 6, 628–647. doi: 10.1007/s11947-012-0944-0
Fang, Z., Zhao, Y., Warner, R. D., and Johnson, S. K. (2017). Active and intelligent packaging in meat industry. Trends Food Sci. Technol. 61, 60–71. doi: 10.1016/j.tifs.2017.01.002
Farshidi, M., Yousefi, M., and Ehsani, A. (2018). The combined effects of lactoperoxidase system and whey protein coating on microbial, chemical, textural, and sensory quality of shrimp (Penaeus merguiensis) during cold storage (4 ± 1°C). Food Sci. Nutr. 6, 1378–1386. doi: 10.1002/fsn3.669
Feng, Y., and Lee, Y. (2016). Surface modification of zein colloidal particles with sodium caseinate to stabilize oil-in-water pickering emulsion. Food Hydrocoll. 56, 292–302. doi: 10.1016/j.foodhyd.2015.12.030
Feng, Y., Xie, L., Chen, Q., and Zheng, L.-R. (2014). Low-cost printed chipless RFID humidity sensor tag for intelligent packaging. IEEE Sens. J. 15, 3201–3208. doi: 10.1109/JSEN.2014.2385154
Fernandez-Salmeron, J., Rivadeneyra, A., Rodríguez, M. A. C., Capitan-Vallvey, L. F., and Palma, A. J. (2015). HF RFID tag as humidity sensor: two different approaches. IEEE Sens. J. 15, 5726–5733. doi: 10.1109/JSEN.2015.2447031
Food Packaging Forum (2015). Putting the CSS Into Action. Available online at: https://www.foodpackagingforum.org/
Food Packaging Market - Fortune Business Insights. Available online at: https://www.fortunebusinessinsights.com/industry-reports/food-packaging-market-101941
Fuertes, G., Soto, I., Carrasco, R., Vargas, M., Sabattin, J., and Lagos, C. (2016a). Intelligent packaging systems: sensors and nanosensors to monitor food quality and safety. J. Sensors 2016:4046061. doi: 10.1155/2016/4046061
Fuertes, G., Soto, I., Vargas, M., Valencia, A., Sabattin, J., and Carrasco, R. (2016b). Nanosensors for a monitoring system in intelligent and active packaging. J. Sens. 2016 7980476. doi: 10.1155/2016/7980476
Gaikwad, K. K., Singh, S., and Ajji, A. (2019). Moisture absorbers for food packaging applications. Environ. Chem. Lett. 17, 609–628. doi: 10.1007/s10311-018-0810-z
Gaikwad, K. K., Singh, S., and Lee, Y. S. (2018). Oxygen scavenging films in food packaging. Environ. Chem. Lett. 16, 523–538. doi: 10.1007/s10311-018-0705-z
Gaikwad, K. K., Singh, S., and Negi, Y. S. (2020). Ethylene scavengers for active packaging of fresh food produce. Environ. Chem. Lett. 18, 1–16. doi: 10.1007/s10311-019-00938-1
Gallego, M. G., Gordon, M. H., Segovia, F., and Almajano Pablos, M. P. (2016). Gelatine-based antioxidant packaging containing Caesalpinia decapetala and Tara as a coating for ground beef patties. Antioxidants 5:10. doi: 10.3390/antiox5020010
Ganiari, S., Choulitoudi, E., and Oreopoulou, V. (2017). Edible and active films and coatings as carriers of natural antioxidants for lipid food. Trends Food Sci. Technol. 68, 70–82. doi: 10.1016/j.tifs.2017.08.009
Garcia, C. V., Shin, G. H., and Kim, J. T. (2018). Metal oxide-based nanocomposites in food packaging: applications, migration, and regulations. Trends Food Sci. Technol. 82, 21–31. doi: 10.1016/j.tifs.2018.09.021
García, M., Casariego, A., Diaz, R., and Roblejo, L. (2014). Effect of edible chitosan/zeolite coating on tomatoes quality during refrigerated storage. Emirates J. Food Agric. 26, 238–246. doi: 10.9755/ejfa.v26i3.16620
Gardiol, A. E., Hernandez, R. J., Reinhammar, B., and Harte, B. R. (1996). USA-Gas-phase oxygen biosensor. Biosens. Bioelectron. 11, vii–viii. doi: 10.1016/0956-5663(96)87678-0
Gautam, R. K., Kakatkar, A. S., and Karani, M. N. (2016). Development of protein-based biodegradable films from fish processing waste. Int. J. Curr. Microbiol. Appl. Sci. 5, 878–888. doi: 10.20546/ijcmas.2016.508.099
Ghaani, M., Cozzolino, C. A., Castelli, G., and Farris, S. (2016). An overview of the intelligent packaging technologies in the food sector. Trends Food Sci. Technol. 51, 1–11. doi: 10.1016/j.tifs.2016.02.008
Gómez-Estaca, J., López-de-Dicastillo, C., Hernández-Muñoz, P., Catalá, R., and Gavara, R. (2014). Advances in antioxidant active food packaging. Trends Food Sci. Technol. 35, 42–51. doi: 10.1016/j.tifs.2013.10.008
Górska-Horczyczak, E., Guzek, D., MOLEDA, Z., Wojtasik-Kalinowska, I., Brodowska, M., and Wierzbicka, A. (2016). Applications of electronic noses in meat analysis. Food Sci. Technol. 36, 389–395. doi: 10.1590/1678-457X.03615
Górska-Horczyczak, E., Wojtasik-Kalinowska, I., Guzek, D., Sun, D., and Wierzbicka, A. (2017). Differentiation of chill-stored and frozen pork necks using electronic nose with ultra-fast gas chromatography. J. Food Process Eng. 40:e12540. doi: 10.1111/jfpe.12540
Gorski, J. R., and Booher, J. (2011). Processes for Preparing a Polymeric Indicator Film. U.S. Patent Application No. 13/010,725.
Goswami, P., and O'Haire, T. (2016). “3 - developments in the use of green (biodegradable), recycled and biopolymer materials in technical nonwovens,” in Advances in Technical Nonwovens Woodhead Publishing Series in Textiles, ed G. Kellie (Woodhead Publishing), 97–114.
Guo, X., Liu, L., Hu, Y., and Wu, Y. (2018). Water vapor sorption properties of TEMPO oxidized and sulfuric acid treated cellulose nanocrystal films. Carbohydr. Polym. 197, 524–530. doi: 10.1016/j.carbpol.2018.06.027
Haghighi-Manesh, S., and Azizi, M. H. (2017). Active packaging systems with emphasis on its applications in dairy products. J. Food Process Eng. 40:e12542. doi: 10.1111/jfpe.12542
Halász, K., and Csóka, L. (2018). Black chokeberry (Aronia melanocarpa) pomace extract immobilized in chitosan for colorimetric pH indicator film application. Food Packag. Shelf Life 16, 185–193. doi: 10.1016/j.fpsl.2018.03.002
Halonen, N. J., Palvölgyi, P. S., Bassani, A., Fiorentini, C., Nair, R., Spigno, G., et al. (2020). Bio-based smart materials for food packaging and sensors–a review. Front. Mater. 7:82. doi: 10.3389/fmats.2020.00082
Han, J., Ruiz-Garcia, L., Qian, J., and Yang, X. (2018a). Food packaging: a comprehensive review and future trends. Compr. Rev. Food Sci. Food Saf. 17, 860–877. doi: 10.1111/1541-4337.12343
Han, J. H. (2014). “Edible films and coatings: a review,” in Innovations in Food Packaging, 2nd Edn. (Texas, TX: Elsevier), 213–255.
Han, Y., Yu, M., and Wang, L. (2018b). Preparation and characterization of antioxidant soy protein isolate films incorporating licorice residue extract. Food Hydrocoll. 75, 13–21. doi: 10.1016/j.foodhyd.2017.09.020
Hanani, Z. A. N., Yee, F. C., and Nor-Khaizura, M. A. R. (2019). Effect of pomegranate (Punica granatum L.) peel powder on the antioxidant and antimicrobial properties of fish gelatin films as active packaging. Food Hydrocoll. 89, 253–259. doi: 10.1016/j.foodhyd.2018.10.007
Haq, M. A., Azam, M., and Hasnain, A. (2015). Gum cordia as carrier of antioxidants: effects on lipid oxidation of peanuts. J. Food Sci. Technol. 52, 2366–2372. doi: 10.1007/s13197-013-1199-0
He, X., Deng, H., and Hwang, H. (2019). The current application of nanotechnology in food and agriculture. J. Food Drug Anal. 27, 1–21. doi: 10.1016/j.jfda.2018.12.002
Hempel, A. W., Papkovsky, D. B., and Kerry, J. P. (2013). Use of optical oxygen sensors in non-destructively determining the levels of oxygen present in combined vacuum and modified atmosphere packaged pre-cooked convenience-style foods and the use of ethanol emitters to extend product shelf-life. Foods 2, 507–520. doi: 10.3390/foods2040507
Hogan, S. A., and Kerry, J. P. (2008). Chapter 3: Smart packaging of meat and poultry products. Smart Packag. Technol. Fast Mov. Consum. Goods 33–54. doi: 10.1002/9780470753699.ch3
Holcapkova, P., Hurajova, A., Bazant, P., Pummerova, M., and Sedlarik, V. (2018). Thermal stability of bacteriocin nisin in polylactide-based films. Polym. Degrad. Stab. 158, 31–39. doi: 10.1016/j.polymdegradstab.2018.10.019
Hoseinnejad, M., Jafari, S. M., and Katouzian, I. (2018). Inorganic and metal nanoparticles and their antimicrobial activity in food packaging applications. Crit. Rev. Microbiol. 44, 161–181. doi: 10.1080/1040841X.2017.1332001
Huang, T., Qian, Y., Wei, J., and Zhou, C. (2019). Polymeric antimicrobial food packaging and its applications. Polymers 11:560. doi: 10.3390/polym11030560
Huang, W.-D., Deb, S., Seo, Y.-S., Rao, S., Chiao, M., and Chiao, J. C. (2011). A passive radio-frequency pH-sensing tag for wireless food-quality monitoring. IEEE Sens. J. 12, 487–495. doi: 10.1109/JSEN.2011.2107738
Hurme, E., Smolander, M., and Ahvenainen, R. (2002). “Intelligent systems in food packaging: Advances in the supply chain,” in New Technologies and Strategies to Enhance Packaging Supply Chains (London), 14–15.
Industry Market Research - Freedonia Group. Reliable Unbiased Industry Market Research. Available online at: https://www.freedoniagroup.com/
Islam, R. U., Khan, M. A., and Islam, S. U. (2017). Plant derivatives as promising materials for processing and packaging of meat-based products–focus on antioxidant and antimicrobial effects. J. Food Process. Preserv. 41:e12862. doi: 10.1111/jfpp.12862
Jacob, J., Thomas, S., Loganathan, S., and Valapa, R. B. (2020). “Chapter 10 - antioxidant incorporated biopolymer composites for active packaging,” in Processing and Development of Polysaccharide-Based Biopolymers for Packaging Applications, ed Y. Zhang (Lethbridge: Elsevier), 239–260.
Jamróz, E., and Kopel, P. (2020). Polysaccharide and protein films with antimicrobial/antioxidant activity in the food industry: a review. Polymers 12:1289. doi: 10.3390/polym12061289
Jamshidian, M., Arab Tehrany, E., Cleymand, F., Leconte, S., Falher, T., and Desobry, S. (2012). Effects of synthetic phenolic antioxidants on physical, structural, mechanical and barrier properties of poly lactic acid film. Carbohydr. Polym. 87, 1763–1773. doi: 10.1016/j.carbpol.2011.09.089
Jang, N. Y., and Won, K. (2014). New pressure-activated compartmented oxygen indicator for intelligent food packaging. Int. J. Food Sci. Technol. 49, 650–654. doi: 10.1111/ijfs.12310
Janjarasskul, T., Min, S. C., and Krochta, J. M. (2013). Triggering mechanisms for oxygen-scavenging function of ascorbic acid-incorporated whey protein isolate films. J. Sci. Food Agric. 93, 2939–2944. doi: 10.1002/jsfa.6120
Janjarasskul, T., and Suppakul, P. (2018). Active and intelligent packaging: the indication of quality and safety. Crit. Rev. Food Sci. Nutr. 58, 808–831. doi: 10.1080/10408398.2016.1225278
Janjarasskul, T., Tananuwong, K., and Krochta, J. M. (2011). Whey protein film with oxygen scavenging function by incorporation of ascorbic acid. J. Food Sci. 76, E561–E568. doi: 10.1111/j.1750-3841.2011.02409.x
Jha, P. (2020). Effect of plasticizer and antimicrobial agents on functional properties of bionanocomposite films based on corn starch-chitosan for food packaging applications. Int. J. Biol. Macromol. 160, 571–582. doi: 10.1016/j.ijbiomac.2020.05.242
Johansson, K., Winestrand, S., Johansson, C., Järnström, L., and Jönsson, L. J. (2012). Oxygen-scavenging coatings and films based on lignosulfonates and laccase. J. Biotechnol. 161, 14–18. doi: 10.1016/j.jbiotec.2012.06.004
Johnson, E. M., Jung, D. Y.-G., Jin, D. Y.-Y., Jayabalan, D. R., Yang, D. S. H., and Suh, J. W. (2018). Bacteriocins as food preservatives: challenges and emerging horizons. Crit. Rev. Food Sci. Nutr. 58, 2743–2767. doi: 10.1080/10408398.2017.1340870
Jridi, M., Boughriba, S., Abdelhedi, O., Nciri, H., Nasri, R., Kchaou, H., et al. (2019). Investigation of physicochemical and antioxidant properties of gelatin edible film mixed with blood orange (Citrus sinensis) peel extract. Food Packag. Shelf Life 21:100342. doi: 10.1016/j.fpsl.2019.100342
Kaewklin, P., Siripatrawan, U., Suwanagul, A., and Lee, Y. S. (2018). Active packaging from chitosan-titanium dioxide nanocomposite film for prolonging storage life of tomato fruit. Int. J. Biol. Macromol. 112, 523–529. doi: 10.1016/j.ijbiomac.2018.01.124
Kakadellis, S., and Harris, Z. M. (2020). Don't scrap the waste: the need for broader system boundaries in bioplastic food packaging life-cycle assessment–a critical review. J. Clean. Prod. 274:122831. doi: 10.1016/j.jclepro.2020.122831
Kalpana, S., Priyadarshini, S. R., Leena, M., Maria Moses, J. A., and Anandharamakrishnan, C. (2019). Intelligent packaging: trends and applications in food systems. Trends Food Sci. Technol. 93, 145–157. doi: 10.1016/j.tifs.2019.09.008
Kanatt, S. R. (2020). Development of active/intelligent food packaging film containing Amaranthus leaf extract for shelf life extension of chicken/fish during chilled storage. Food Packag. Shelf Life 24:100506. doi: 10.1016/j.fpsl.2020.100506
Kapetanakou, A. E., and Skandamis, P. N. (2016). Applications of active packaging for increasing microbial stability in foods: natural volatile antimicrobial compounds. Curr. Opin. Food Sci. 12, 1–12. doi: 10.1016/j.cofs.2016.06.001
Kaya, M., Khadem, S., Cakmak, Y. S., Mujtaba, M., Ilk, S., Akyuz, L., et al. (2018). Antioxidative and antimicrobial edible chitosan films blended with stem, leaf and seed extracts of Pistacia terebinthus for active food packaging. RSC Adv. 8, 3941–3950. doi: 10.1039/C7RA12070B
Kerry, J. P. (2012). “Application of smart packaging systems for conventionally packaged muscle-based food products,” in Advances in Meat, Poultry and Seafood Packaging, ed J. P. Kerry (Ireland: Woodhead Publishing), 522–564.
Kerry, J. P., O'grady, M. N., and Hogan, S. A. (2006). Past, current and potential utilisation of active and intelligent packaging systems for meat and muscle-based products: a review. Meat Sci. 74, 113–130. doi: 10.1016/j.meatsci.2006.04.024
Kodogiannis, V. S. (2017). Application of an electronic nose coupled with fuzzy-wavelet network for the detection of meat spoilage. Food Bioprocess Technol. 10, 730–749. doi: 10.1007/s11947-016-1851-6
Kowalczyk, D., Kordowska-Wiater, M., Karaś, M., Zieba, E., Mezyńska, M., and Wiacek, A. E. (2020). Release kinetics and antimicrobial properties of the potassium sorbate-loaded edible films made from pullulan, gelatin and their blends. Food Hydrocoll. 101:105539. doi: 10.1016/j.foodhyd.2019.105539
Kraśniewska, K., Galus, S., and Gniewosz, M. (2020). Biopolymers-based materials containing silver nanoparticles as active packaging for food applications–a review. Int. J. Mol. Sci. 21:698. doi: 10.3390/ijms21030698
Kuorwel, K. K., Cran, M. J., Orbell, J. D., Buddhadasa, S., and Bigger, S. W. (2015). Review of mechanical properties, migration, and potential applications in active food packaging systems containing nanoclays and nanosilver. Compr. Rev. Food Sci. Food Saf. 14, 411–430. doi: 10.1111/1541-4337.12139
Kurek, M., Garofulić, I. E., Bakić, M. T., Ščetar, M., Uzelac, V. D., and Galić, K. (2018). Development and evaluation of a novel antioxidant and pH indicator film based on chitosan and food waste sources of antioxidants. Food Hydrocoll. 84, 238–246. doi: 10.1016/j.foodhyd.2018.05.050
Kuswandi, B., Maryska, C., Abdullah, A., and Heng, L. Y. (2013). Real time on-package freshness indicator for guavas packaging. J. Food Meas. Charact. 7, 29–39. doi: 10.1007/s11694-013-9136-5
Kuswandi, B., Oktaviana, R., Abdullah, A., and Heng, L. Y. (2014). A novel on-package sticker sensor based on methyl red for real-time monitoring of broiler chicken cut freshness. Packag. Technol. Sci. 27, 69–81. doi: 10.1002/pts.2016
Kuswandi, B., Restyana, A., Abdullah, A., Heng, L. Y., and Ahmad, M. (2012). A novel colorimetric food package label for fish spoilage based on polyaniline film. Food Control 25, 184–189. doi: 10.1016/j.foodcont.2011.10.008
Kuswandi, B., Wicaksono, Y., Abdullah, A., Heng, L. Y., and Ahmad, M. (2011). Smart packaging: sensors for monitoring of food quality and safety. Sens. Instrum. Food Qual. Saf. 5, 137–146. doi: 10.1007/s11694-011-9120-x
Larson, E. L. (1991). “Chapter 11: Alcohols,” in Disinfection, Sterilization and Preservation, Lea and Febiger, 4th edn, ed S. S. Block (Philadelphia, PA), 642–654.
Lawal, A. T., and Adeloju, S. B. (2012). Progress and recent advances in fabrication and utilization of hypoxanthine biosensors for meat and fish quality assessment: a review. Talanta 100, 217–228. doi: 10.1016/j.talanta.2012.07.085
Lawrie, K., Mills, A., and Hazafy, D. (2013). Simple inkjet-printed, UV-activated oxygen indicator. Sens. Actuat. B Chem. 176, 1154–1159. doi: 10.1016/j.snb.2012.10.125
Leceta, I., Molinaro, S., Guerrero, P., Kerry, J. P., and De la Caba, K. (2015). Quality attributes of map packaged ready-to-eat baby carrots by using chitosan-based coatings. Postharvest Biol. Technol. 100, 142–150. doi: 10.1016/j.postharvbio.2014.09.022
Lee, D. S. (2016). Carbon dioxide absorbers for food packaging applications. Trends Food Sci. Technol. 57, 146–155. doi: 10.1016/j.tifs.2016.09.014
Lee, D. S., Yam, K. L., and Piergiovanni, L. (2008). Food Packaging Science and Technology. CRC Press.
Lee, K., and Ko, S. (2014). Proof-of-concept study of a whey protein isolate based carbon dioxide indicator to measure the shelf-life of packaged foods. Food Sci. Biotechnol. 23, 115–120. doi: 10.1007/s10068-014-0015-6
Lee, K. K., Kim, M. J., and Seo, E. H. (2014). Bag for Food Packing. U.S. Patent Application No. 29/465,038.
Lee, M. H., Kim, S. Y., and Park, H. J. (2018). Effect of halloysite nanoclay on the physical, mechanical, and antioxidant properties of chitosan films incorporated with clove essential oil. Food Hydrocoll. 84, 58–67. doi: 10.1016/j.foodhyd.2018.05.048
Lee, S., and Park, J.-W. (2017). Luminescent oxygen sensors with highly improved sensitivity based on a porous sensing film with increased oxygen accessibility and photoluminescence. Sens. Actuat. B Chem. 249, 364–377. doi: 10.1016/j.snb.2017.04.112
Lee, S. J., and Rahman, A. T. M. M. (2014). “Intelligent packaging for food products,” in Innovations in Food Packaging, ed H. Jung (Elsevier), 171–209.
Lee, S. Y., Lee, S. J., Choi, D. S., and Hur, S. J. (2015). Current topics in active and intelligent food packaging for preservation of fresh foods. J. Sci. Food Agric. 95, 2799–2810. doi: 10.1002/jsfa.7218
Liang, T., Sun, G., Cao, L., Li, J., and Wang, L. (2019). A pH and NH3 sensing intelligent film based on Artemisia sphaerocephala Krasch. gum and red cabbage anthocyanins anchored by carboxymethyl cellulose sodium added as a host complex. Food Hydrocoll. 87, 858–868. doi: 10.1016/j.foodhyd.2018.08.028
Liao, Y., Zhang, R., and Qian, J. (2019). Printed electronics based on inorganic conductive nanomaterials and their applications in intelligent food packaging. RSC Adv. 9, 29154–29172. doi: 10.1039/C9RA05954G
Lima, H. L. S., Gonçalves, C., Cerqueira, M. Â., do Nascimento, E. S., Gama, M. F., Rosa, M. F., et al. (2018). Bacterial cellulose nanofiber-based films incorporating gelatin hydrolysate from tilapia skin: production, characterization and cytotoxicity assessment. Cellulose 25, 6011–6029. doi: 10.1007/s10570-018-1983-0
Limpisophon, K., and Schleining, G. (2017). Use of gallic acid to enhance the antioxidant and mechanical properties of active fish gelatin film. J. Food Sci. 82, 80–89. doi: 10.1111/1750-3841.13578
Liu, H., and Watts, M. R. (2019). Detection of Multi-Dimensional Heating Patterns in Thermal Food Processes Using Thermochromic Inks. U.S. Patent Application No. 16/414,159.
Liu, J., Yong, H., Liu, Y., Qin, Y., Kan, J., and Liu, J. (2019). Preparation and characterization of active and intelligent films based on fish gelatin and haskap berries (Lonicera caerulea L.) extract. Food Packag. Shelf Life 22:100417. doi: 10.1016/j.fpsl.2019.100417
Liu, X., Marrakchi, M., Xu, D., Dong, H., and Andreescu, S. (2016). Biosensors based on modularly designed synthetic peptides for recognition, detection and live/dead differentiation of pathogenic bacteria. Biosens. Bioelectron. 80, 9–16. doi: 10.1016/j.bios.2016.01.041
Lochman, L., Zimcik, P., Klimant, I., Novakova, V., and Borisov, S. M. (2017). Red-emitting CO2 sensors with tunable dynamic range based on pH-sensitive azaphthalocyanine indicators. Sens. Actuat. B Chem. 246, 1100–1107. doi: 10.1016/j.snb.2016.10.135
Lopez-Rubio, A. (2011). “Bioactive food packaging strategies,” in Multifunctional and Nanoreinforced Polymers for Food Packaging, ed J.-M. Lagarón (Cambridge: Elsevier), 460–482.
Lopez-Rubio, A., Almenar, E., Hernandez-Muñoz, P., Lagarón, J. M., Catalá, R., and Gavara, R. (2004). Overview of active polymer-based packaging technologies for food applications. Food Rev. Int. 20, 357–387. doi: 10.1081/FRI-200033462
Lorite, G. S., Selkälä, T., Sipola, T., Palenzuela, J., Jubete, E., Viñuales, A., et al. (2017). Novel, smart and RFID assisted critical temperature indicator for supply chain monitoring. J. Food Eng. 193, 20–28. doi: 10.1016/j.jfoodeng.2016.06.016
Lourenço, S. C., Moldão-Martins, M., and Alves, V. D. (2019). Antioxidants of natural plant origins: from sources to food industry applications. Molecules 24:4132. doi: 10.3390/molecules24224132
Luís, Â., Pereira, L., Domingues, F., and Ramos, A. (2019). Development of a carboxymethyl xylan film containing licorice essential oil with antioxidant properties to inhibit the growth of foodborne pathogens. LWT 111, 218–225. doi: 10.1016/j.lwt.2019.05.040
Lunkov, A. P., Ilyina, A. V., and Varlamov, V. P. (2018). Antioxidant, antimicrobial, and fungicidal properties of chitosan based films. Appl. Biochem. Microbiol. 54, 449–458. doi: 10.1134/S0003683818050125
Madhusudan, P., Chellukuri, N., and Shivakumar, N. (2018). Smart packaging of food for the 21st century–a review with futuristic trends, their feasibility and economics. Mater. Today Proc. 5, 21018–21022. doi: 10.1016/j.matpr.2018.06.494
Magalhães, A. E. V., Rossi, A. H. G., Zattar, I. C., Marques, M. A. M., and Seleme, R. (2019). Food traceability technologies and foodborne outbreak occurrences. Br. Food J. 121, 3362–3379. doi: 10.1108/BFJ-02-2019-0143
Mahieu, A., Terrié, C., and Youssef, B. (2015). Thermoplastic starch films and thermoplastic starch/polycaprolactone blends with oxygen-scavenging properties: influence of water content. Ind. Crops Prod. 72, 192–199. doi: 10.1016/j.indcrop.2014.11.037
Majid, I., Nayik, G. A., Dar, S. M., and Nanda, V. (2018). Novel food packaging technologies: innovations and future prospective. J. Saudi Soc. Agric. Sci. 17, 454–462. doi: 10.1016/j.jssas.2016.11.003
Malhotra, B., Keshwani, A., and Kharkwal, H. (2015). Antimicrobial food packaging: potential and pitfalls. Front. Microbiol. 6:611. doi: 10.3389/fmicb.2015.00611
Malvano, F., Albanese, D., Pilloton, R., and Di Matteo, M. (2017). A new label-free impedimetric aptasensor for gluten detection. Food Control 79, 200–206. doi: 10.1016/j.foodcont.2017.03.033
Mane, K. A. (2016). A review on active packaging: an innovation in food packaging. Int. J. Environ. Agric. Biotechnol. 1:238566. doi: 10.22161/ijeab/1.3.35
Mao, Y., Gao, Y., Wu, S., Wu, S., Shi, J., Zhou, B., et al. (2017). Highly enhanced sensitivity of optical oxygen sensors using microstructured PtTFPP/PDMS-pillar arrays sensing layer. Sens. Actuat. B Chem. 251, 495–502. doi: 10.1016/j.snb.2017.05.081
Marcos, B., Sárraga, C., Castellari, M., Kappen, F., Schennink, G., and Arnau, J. (2014). Development of biodegradable films with antioxidant properties based on polyesters containing α-tocopherol and olive leaf extract for food packaging applications. Food Packag. Shelf Life 1, 140–150. doi: 10.1016/j.fpsl.2014.04.002
Martínez-Olmos, A., Fernández-Salmerón, J., Lopez-Ruiz, N., Rivadeneyra Torres, A., Capitan-Vallvey, L. F., and Palma, A. J. (2013). Screen printed flexible radiofrequency identification tag for oxygen monitoring. Anal. Chem. 85, 11098–11105. doi: 10.1021/ac4028802
Marx, D., Joss, L., Hefti, M., Pini, R., and Mazzotti, M. (2013). The role of water in adsorption-based CO2 capture systems. Energy Procedia 37, 107–114. doi: 10.1016/j.egypro.2013.05.090
Matindoust, S., Baghaei-Nejad, M., Abadi, M. H. S., Zou, Z., and Zheng, L.-R. (2016). Food quality and safety monitoring using gas sensor array in intelligent packaging. Sens. Rev. 36, 169–183. doi: 10.1108/SR-07-2015-0115
Mattila, T., Tawast, J., and Ahvenainen, R. (1990). New possibilities for quality control of aseptic packages: microbiological spoilage and seal defect detection using head-space indicators. Leb. Technol. 23, 246–251.
Medina-Jaramillo, C., Ochoa-Yepes, O., Bernal, C., and Fam,á, L. (2017). Active and smart biodegradable packaging based on starch and natural extracts. Carbohydr. Polym. 176, 187–194. doi: 10.1016/j.carbpol.2017.08.079
Meira, S. M. M., Zehetmeyer, G., Werner, J. O., and Brandelli, A. (2017). A novel active packaging material based on starch-halloysite nanocomposites incorporating antimicrobial peptides. Food Hydrocoll. 63, 561–570. doi: 10.1016/j.foodhyd.2016.10.013
Mellinas, C., Valdés, A., Ramos, M., Burgos, N., Garrigos, M., del, C., et al. (2016). Active edible films: current state and future trends. J. Appl. Polym. Sci. 133. doi: 10.1002/app.42631
Meng, J., Qian, J., and Tang, Y. (2018). A solid-state time-temperature indicator used in chilled fresh pork monitoring. Packag. Technol. Sci. 31, 353–360. doi: 10.1002/pts.2328
Meng, X., Kim, S., Puligundla, P., and Ko, S. (2014). Carbon dioxide and oxygen gas sensors-possible application for monitoring quality, freshness, and safety of agricultural and food products with emphasis on importance of analytical signals and their transformation. J. Korean Soc. Appl. Biol. Chem. 57, 723–733. doi: 10.1007/s13765-014-4180-3
Menzel, C., González-Martínez, C., Chiralt, A., and Vilaplana, F. (2019). Antioxidant starch films containing sunflower hull extracts. Carbohydr. Polym. 214, 142–151. doi: 10.1016/j.carbpol.2019.03.022
Mihindukulasuriya, S. D. F., and Lim, L.-T. (2014). Nanotechnology development in food packaging: a review. Trends Food Sci. Technol. 40, 149–167. doi: 10.1016/j.tifs.2014.09.009
Mills, A. (2005). Oxygen indicators and intelligent inks for packaging food. Chem. Soc. Rev. 34, 1003–1011. doi: 10.1039/b503997p
Mir, S. A., Dar, B. N., Wani, A. A., and Shah, M. A. (2018). Effect of plant extracts on the techno-functional properties of biodegradable packaging films. Trends Food Sci. Technol. 80, 141–154. doi: 10.1016/j.tifs.2018.08.004
Mitsubishi Gas Chemical Company Inc. AGELESS EYE, Oxygen Indicator. Available online at: https://www.mgc.co.jp/eng/products/sc/ageless-eye.html (accessed March 2021).
Moghadam, M., Salami, M., Mohammadian, M., Khodadadi, M., and Emam-Djomeh, Z. (2020). Development of antioxidant edible films based on mung bean protein enriched with pomegranate peel. Food Hydrocoll. 104:105735. doi: 10.1016/j.foodhyd.2020.105735
Mølgaard, S. L., Henriksson, M., Cárdenas, M., and Svagan, A. J. (2014). Cellulose-nanofiber/polygalacturonic acid coatings with high oxygen barrier and targeted release properties. Carbohydr. Polym. 114, 179–182. doi: 10.1016/j.carbpol.2014.08.011
Montero, P., Mosquera, M., Marín-Peñalver, D., Alemán, A., Martínez-Álvarez, Ó., and Gómez-Guillén, M. C. (2019). Changes in structural integrity of sodium caseinate films by the addition of nanoliposomes encapsulating an active shrimp peptide fraction. J. Food Eng. 244, 47–54. doi: 10.1016/j.jfoodeng.2018.09.024
Moreira, J. B., Terra, A. L. M., Costa, J. A. V., and de Morais, M. G. (2018). Development of pH indicator from PLA/PEO ultrafine fibers containing pigment of microalgae origin. Int. J. Biol. Macromol. 118, 1855–1862. doi: 10.1016/j.ijbiomac.2018.07.028
Morris, R., Mcmorris, I. J. A., Acosta, G., Hill, J., Tank, A. R., Bishop, A., et al. (2004). Food-Borne Pathogen and Spoilage Detection Device and Method. U.S. Patent Application No. 10/659,222.
Mousavi Khaneghah, A., Hashemi, S. M. B., and Limbo, S. (2018). Antimicrobial agents and packaging systems in antimicrobial active food packaging: an overview of approaches and interactions. Food Bioprod. Process. 111, 1–19. doi: 10.1016/j.fbp.2018.05.001
Mu, H., Gao, H., Chen, H., Fang, X., and Han, Q. (2017). A novel controlled release ethanol emitter: preparation and effect on some postharvest quality parameters of Chinese bayberry during storage. J. Sci. Food Agric. 97, 4929–4936. doi: 10.1002/jsfa.8369
Müller, P., and Schmid, M. (2019). Intelligent packaging in the food sector: a brief overview. Foods 8:16. doi: 10.3390/foods8010016
Musso, Y. S., Salgado, P. R., and Mauri, A. N. (2016). Gelatin based films capable of modifying its color against environmental pH changes. Food Hydrocoll. 61, 523–530. doi: 10.1016/j.foodhyd.2016.06.013
Musso, Y. S., Salgado, P. R., and Mauri, A. N. (2017a). Desarrollo de Películas Proteicas Para el Envasado Activo e Inteligente de Alimentos. Universidad Nacional de La Plata. Available online at: http://sedici.unlp.edu.ar/handle/10915/60885.
Musso, Y. S., Salgado, P. R., and Mauri, A. N. (2017b). Smart edible films based on gelatin and curcumin. Food Hydrocoll. 66, 8–15. doi: 10.1016/j.foodhyd.2016.11.007
Musso, Y. S., Salgado, P. R., and Mauri, A. N. (2019). Smart gelatin films prepared using red cabbage (Brassica oleracea L.) extracts as solvent. Food Hydrocoll. 89, 674–681. doi: 10.1016/j.foodhyd.2018.11.036
Mustafa, F., and Andreescu, S. (2018). Chemical and biological sensors for food-quality monitoring and smart packaging. Foods 7:168. doi: 10.3390/foods7100168
Nassef, H. M., Bermudo Redondo, M. C., Ciclitira, P. J., Ellis, H. J., Fragoso, A., and O'Sullivan, C. K. (2008). Electrochemical immunosensor for detection of celiac disease toxic gliadin in foodstuff. Anal. Chem. 80, 9265–9271. doi: 10.1021/ac801620j
Neethirajan, S., Jayas, D. S., and Sadistap, S. (2009). Carbon dioxide (CO 2) sensors for the agri-food industry—a review. Food Bioprocess Technol. 2, 115–121. doi: 10.1007/s11947-008-0154-y
Németh, D., Balázs, G., Daood, H. G., Kovács, Z., Bodor, Z., Zinia Zaukuu, J.-L., et al. (2019). standard analytical methods, sensory evaluation, NIRS and electronic tongue for sensing taste attributes of different melon varieties. Sensors 19:5010. doi: 10.3390/s19225010
Nilsuwan, K., Benjakul, S., and Prodpran, T. (2018). Properties and antioxidative activity of fish gelatin-based film incorporated with epigallocatechin gallate. Food Hydrocoll. 80, 212–221. doi: 10.1016/j.foodhyd.2018.01.033
Nisa, I., Ashwar, B. A., Shah, A., Gani, A., Gani, A., and Masoodi, F. A. (2015). Development of potato starch based active packaging films loaded with antioxidants and its effect on shelf life of beef. J. Food Sci. Technol. 52, 7245–7253. doi: 10.1007/s13197-015-1859-3
Nopwinyuwong, A., Trevanich, S., and Suppakul, P. (2010). Development of a novel colorimetric indicator label for monitoring freshness of intermediate-moisture dessert spoilage. Talanta 81, 1126–1132. doi: 10.1016/j.talanta.2010.02.008
Noronha, C. M., de Carvalho, S. M., Lino, R. C., and Barreto, P. L. M. (2014). Characterization of antioxidant methylcellulose film incorporated with α-tocopherol nanocapsules. Food Chem. 159, 529–535. doi: 10.1016/j.foodchem.2014.02.159
Noshirvani, N., Ghanbarzadeh, B., Gardrat, C., Rezaei, M. R., Hashemi, M., Le Coz, C., et al. (2017). Cinnamon and ginger essential oils to improve antifungal, physical and mechanical properties of chitosan-carboxymethyl cellulose films. Food Hydrocoll. 70, 36–45. doi: 10.1016/j.foodhyd.2017.03.015
O'Grady, M. N., and Kerry, J. P. (2008). “Smart packaging technologies and their application in conventional meat packaging systems,” in Meat Biotechnology, ed F. Toldrá (New York, NY: Springer), 425–451.
Oliveira, M., Abadias, M., Usall, J., Torres, R., Teixidó, N., and Viñas, I. (2015). Application of modified atmosphere packaging as a safety approach to fresh-cut fruits and vegetables–a review. Trends Food Sci. Technol. 46, 13–26. doi: 10.1016/j.tifs.2015.07.017
Ortiz, C. M., Mauri, A. N., and Vicente, A. R. (2013). Use of soy protein based 1-methylcyclopropene-releasing pads to extend the shelf life of tomato (Solanum lycopersicum L.) fruit. Innov. Food Sci. Emerg. Technol. 20, 281–287. doi: 10.1016/j.ifset.2013.07.004
Ortiz, C. M., Vicente, A. R., and Mauri, A. N. (2014). Combined use of physical treatments and edible coatings in fresh produce: moving beyond. Stewart Postharvest Rev. 10, 1–6.
Pacquit, A., Frisby, J., Diamond, D., Lau, K. T., Farrell, A., Quilty, B., et al. (2007). Development of a smart packaging for the monitoring of fish spoilage. Food Chem. 102, 466–470. doi: 10.1016/j.foodchem.2006.05.052
Pacquit, A., Lau, K. T., McLaughlin, H., Frisby, J., Quilty, B., and Diamond, D. (2006). Development of a volatile amine sensor for the monitoring of fish spoilage. Talanta 69, 515–520. doi: 10.1016/j.talanta.2005.10.046
Palumbo, J. D., Borucki, M. K., Mandrell, R. E., and Gorski, L. (2003). Serotyping of Listeria monocytogenes by enzyme-linked immunosorbent assay and identification of mixed-serotype cultures by colony immunoblotting. J. Clin. Microbiol. 41, 564–571. doi: 10.1128/JCM.41.2.564-571.2003
Pan, M., Tong, L., Chi, X., Ai, N., Cao, Y., and Sun, B. (2019). Comparison of sensory and electronic tongue analysis combined with HS-SPME-GC-MS in the evaluation of skim milk processed with different preheating treatments. Molecules 24:1650. doi: 10.3390/molecules24091650
Papadopoulou, O. S., Panagou, E. Z., Mohareb, F. R., and Nychas, G.-J. E. (2013). Sensory and microbiological quality assessment of beef fillets using a portable electronic nose in tandem with support vector machine analysis. Food Res. Int. 50, 241–249. doi: 10.1016/j.foodres.2012.10.020
Park, H. R., Kim, Y. A., Jung, S. W., Kim, H. C., and Lee, S. J. (2013a). Response of microbial time temperature indicator to quality indices of chicken breast meat during storage. Food Sci. Biotechnol. 22, 1145–1152. doi: 10.1007/s10068-013-0196-4
Park, Y.-H., Choi, K.-J., Bae, J.-Y., Yoon, S.-K., Jang, H.-I., and Lee, C.-S. (2013b). Development of a detection sensor for mixed trimethylamine and ammonia gas. J. Ind. Eng. Chem. 19, 1703–1707. doi: 10.1016/j.jiec.2013.02.009
Park, Y. W., Kim, S. M., Lee, J. Y., and Jang, W. (2015). Application of biosensors in smart packaging. Mol. Cell. Toxicol. 11, 277–285. doi: 10.1007/s13273-015-0027-1
Pavelková, A. (2013). Time temperature indicators as devices intelligent packaging. Acta Univ. Agric. Silvic. Mendelianae Brun. 61, 245–251. doi: 10.11118/actaun201361010245
Pereda, M., Marcovich, N., and Ansorena, M. R. (2018). “Nanotechnology in food packaging applications: barrier materials, antimicrobial agents, sensors, and safety assessment,” in Handbook of Ecomaterials (Springer), 1–22.
Pereira de Abreu, D. A., Cruz, J. M., and Paseiro Losada, P. (2012). Active and intelligent packaging for the food industry. Food Rev. Int. 28, 146–187. doi: 10.1080/87559129.2011.595022
Pérez Córdoba, L. J., and Sobral, P. J. A. (2017). Physical and antioxidant properties of films based on gelatin, gelatin-chitosan or gelatin-sodium caseinate blends loaded with nanoemulsified active compounds. J. Food Eng. 213, 47–53. doi: 10.1016/j.jfoodeng.2017.05.023
Perez de Vargas-Sansalvador, I. M., Erenas, M. M., Diamond, D., Quilty, B., and Capitan-Vallvey, L. F. (2017). Water based-ionic liquid carbon dioxide sensor for applications in the food industry. Sens. Actuat. B Chem. 253, 302–309. doi: 10.1016/j.snb.2017.06.047
Petersen, K., Væggemose Nielsen, P., Bertelsen, G., Lawther, M., Olsen, M. B., Nilsson, N. H., et al. (1999). Potential of biobased materials for food packaging. Trends Food Sci. Technol. 10, 52–68. doi: 10.1016/S0924-2244(99)00019-9
Piñeros-Hernandez, D., Medina-Jaramillo, C., López-Córdoba, A., and Goyanes, S. (2017). Edible cassava starch films carrying rosemary antioxidant extracts for potential use as active food packaging. Food Hydrocoll. 63, 488–495. doi: 10.1016/j.foodhyd.2016.09.034
Pinming, C., Sukgorn, N., Suhatcho, T., Saetang, B., Kerdkhong, P., Maboonchuay, T., et al. (2016). “Humidity sensor using carboxymethyl cellulose hydrogel membrane,” in 2016 13th International Conference on Electrical Engineering/Electronics, Computer, Telecommunications and Information Technology (ECTI-CON) (Chiang Mai: IEEE), 1–3.
Potyrailo, R. A., Nagraj, N., Tang, Z., Mondello, F. J., Surman, C., and Morris, W. (2012). Battery-free radio frequency identification (RFID) sensors for food quality and safety. J. Agric. Food Chem. 60, 8535–8543. doi: 10.1021/jf302416y
Poyatos-Racionero, E., Ros-Lis, J. V., Vivancos, J.-L., and Martínez-Máñez, R. (2018). Recent advances on intelligent packaging as tools to reduce food waste. J. Clean. Prod. 172, 3398–3409. doi: 10.1016/j.jclepro.2017.11.075
Priyadarshi, R., Deeba, F., and Sauraj Negi, Y. S. (2020). “Chapter 11 - modified atmosphere packaging development,” in Processing and Development of Polysaccharide-Based Biopolymers for Packaging Applications, ed Y. Zhang (Elsevier), 261–280.
Puligundla, P., Jung, J., and Ko, S. (2012). Carbon dioxide sensors for intelligent food packaging applications. Food Control 25, 328–333. doi: 10.1016/j.foodcont.2011.10.043
Qin, Y., Liu, Y., Yong, H., Liu, J., Zhang, X., and Liu, J. (2019). Preparation and characterization of active and intelligent packaging films based on cassava starch and anthocyanins from Lycium ruthenicum Murr. Int. J. Biol. Macromol. 134, 80–90. doi: 10.1016/j.ijbiomac.2019.05.029
Qin, Y.-Y., Zhang, Z.-H., Li, L., Yuan, M.-L., Fan, J., and Zhao, T.-R. (2015). Physio-mechanical properties of an active chitosan film incorporated with montmorillonite and natural antioxidants extracted from pomegranate rind. J. Food Sci. Technol. 52, 1471–1479. doi: 10.1007/s13197-013-1137-1
Qiu, S., and Wang, J. (2017). The prediction of food additives in the fruit juice based on electronic nose with chemometrics. Food Chem. 230, 208–214. doi: 10.1016/j.foodchem.2017.03.011
Rambabu, K., Bharath, G., Banat, F., Show, P. L., and Cocoletzi, H. H. (2019). Mango leaf extract incorporated chitosan antioxidant film for active food packaging. Int. J. Biol. Macromol. 126, 1234–1243. doi: 10.1016/j.ijbiomac.2018.12.196
Ramírez, H. L., Soriano, A., Gómez, S., Iranzo, J. U., and Briones, A. I. (2018). Evaluation of the Food Sniffer electronic nose for assessing the shelf life of fresh pork meat compared to physicochemical measurements of meat quality. Eur. Food Res. Technol. 244, 1047–1055. doi: 10.1007/s00217-017-3021-0
Ramos, A. C., Gales, A. C., Monteiro, J., Silbert, S., Chagas-Neto, T., Machado, A. M. O., et al. (2017). Evaluation of a rapid immunochromatographic test for detection of distinct variants of Klebsiella pneumoniae carbapenemase (KPC) in Enterobacteriaceae. J. Microbiol. Methods 142, 1–3. doi: 10.1016/j.mimet.2017.08.016
Ramos, M., Valdés, A., Mellinas, A. C., and Garrigós, M. C. (2015). New trends in beverage packaging systems: a review. Beverages 1, 248–272. doi: 10.3390/beverages1040248
Rawdkuen, S. (2018). “Edible films incorporated with active compounds: their properties and application,” in Active Antimicrobial Food Packaging, eds V. Isıl and S. Uzunlu (IntechOpen), 71–85.
Realini, C. E., and Marcos, B. (2014). Active and intelligent packaging systems for a modern society. Meat Sci. 98, 404–419. doi: 10.1016/j.meatsci.2014.06.031
Remedio, L. N., Silva dos Santos, J. W., Vieira Maciel, V. B., Yoshida, C. M. P., and Aparecida de Carvalho, R. (2019). Characterization of active chitosan films as a vehicle of potassium sorbate or nisin antimicrobial agents. Food Hydrocoll. 87, 830–838. doi: 10.1016/j.foodhyd.2018.09.012
ReportLinker. Smart Packaging Market Research. Available online at: https://www.reportlinker.com/p05941977/Smart-Packaging-Market-Research-Report-by-Technology-by-Application-Global-Forecast-to-Cumulative-Impact-of-COVID-19.html?utm_source=GNW (accessed March 2021).
Restuccia, D., Spizzirri, U. G., Parisi, O. I., Cirillo, G., Curcio, M., Iemma, F., et al. (2010). New EU regulation aspects and global market of active and intelligent packaging for food industry applications. Food Control 21, 1425–1435. doi: 10.1016/j.foodcont.2010.04.028
Rhim, J.-W., and Wang, L.-F. (2013). Mechanical and water barrier properties of agar/κ-carrageenan/konjac glucomannan ternary blend biohydrogel films. Carbohydr. Polym. 96, 71–81. doi: 10.1016/j.carbpol.2013.03.083
Riaz, A., Lei, S., Akhtar, H. M. S., Wan, P., Chen, D., Jabbar, S., et al. (2018). Preparation and characterization of chitosan-based antimicrobial active food packaging film incorporated with apple peel polyphenols. Int. J. Biol. Macromol. 114, 547–555. doi: 10.1016/j.ijbiomac.2018.03.126
Ribeiro-Santos, R., Andrade, M., de Melo, N. R., and Sanches-Silva, A. (2017). Use of essential oils in active food packaging: recent advances and future trends. Trends Food Sci. Technol. 61, 132–140. doi: 10.1016/j.tifs.2016.11.021
Rooney, M. L. (2005). “Introduction to active food packaging technologies,” in Innovations in Food Packaging (Elsevier), 63–79.
Rostami, A. H., Motamedzadegan, A., Hosseini, S. E., Rezaei, M., and Kamali, A. (2017). Evaluation of plasticizing and antioxidant properties of silver carp protein hydrolysates in fish gelatin film. J. Aquat. Food Prod. Technol. 26, 457–467. doi: 10.1080/10498850.2016.1213345
Roya, A. Q., and Elham, M. (2016). Intelligent food packaging: concepts and innovations. Int. J. ChemTech Res 9, 669–676.
Saarinen, J. J., Remonen, T., Tobjörk, D., Aarnio, H., Bollström, R., Österbacka, R., et al. (2017). Large-scale roll-to-roll patterned oxygen indicators for modified atmosphere packages. Packag. Technol. Sci. 30, 219–227. doi: 10.1002/pts.2295
Sahraee, S., Milani, J. M., Regenstein, J. M., and Kafil, H. S. (2019). Protection of foods against oxidative deterioration using edible films and coatings: a review. Food Biosci. 32:100451. doi: 10.1016/j.fbio.2019.100451
Said, N. S., and Sarbon, N. M. (2019). Chapter 4: Protein-based active film as antimicrobial food packaging: a review. Act. Antimicrob. Food Packag. 53, 53–69.
Saini, R. K., Ko, E. Y., and Keum, Y.-S. (2017). Minimally processed ready-to-eat baby-leaf vegetables: production, processing, storage, microbial safety, and nutritional potential. Food Rev. Int. 33, 644–663. doi: 10.1080/87559129.2016.1204614
Sai-Ut, S., Benjakul, S., and Rawdkuen, S. (2015). Retardation of lipid oxidation using gelatin film incorporated with longan seed extract compared with BHT. J. Food Sci. Technol. 52, 5842–5849. doi: 10.1007/s13197-014-1631-0
Sajilata, M. G., Savitha, K., Singhal, R. S., and Kanetkar, V. R. (2007). Scalping of flavors in packaged foods. Compr. Rev. Food Sci. Food Saf. 6, 17–35. doi: 10.1111/j.1541-4337.2007.00014.x
Salgado, P. R., Di Giorgio, L., Musso, Y. S., and Mauri, A. N. (2019a). “Bioactive packaging: combining nanotechnologies with packaging for improved food functionality,” in Nanomaterials for Food Applications, eds A. L. Rubio, M. M. Sanz, M. J. Fabra Rovira, and L. G. Gómez-Mascaraque (Massachusetts, MA: Elsevier), 233–270.
Salgado, P. R., Di Giorgio, L., Musso, Y. S., and Mauri, A. N. (2019b). “Chapter 9 - bioactive packaging: combining nanotechnologies with packaging for improved food functionality,” in Nanomaterials for Food Applications Micro and Nano Technologies, eds A. López Rubio, M. J. Fabra Rovira, M. Martínez Sanz, and L. G. Gómez-Mascaraque (Amnsterdam: Elsevier), 233–270.
Salgado, P. R., Fernández, G. B., Drago, S. R., and Mauri, A. N. (2011). Addition of bovine plasma hydrolysates improves the antioxidant properties of soybean and sunflower protein-based films. Food Hydrocoll. 25, 1433–1440. doi: 10.1016/j.foodhyd.2011.02.003
Salgado, P. R., Ortiz, C. M., Musso, Y. S., Di Giorgio, L., and Mauri, A. N. (2015). Edible films and coatings containing bioactives. Curr. Opin. Food Sci. 5, 86–92. doi: 10.1016/j.cofs.2015.09.004
Salinas, Y., Ros-Lis, J. V., Vivancos, J.-L., Martínez-Máñez, R., Marcos, M. D., Aucejo, S., et al. (2014). A novel colorimetric sensor array for monitoring fresh pork sausages spoilage. Food Control 35, 166–176. doi: 10.1016/j.foodcont.2013.06.043
Saliu, F., and Della Pergola, R. (2018). Carbon dioxide colorimetric indicators for food packaging application: applicability of anthocyanin and poly-lysine mixtures. Sensors Actuators B Chem. 258, 1117–1124. doi: 10.1016/j.snb.2017.12.007
Sanches-Silva, A., Costa, D., Albuquerque, T. G., Buonocore, G. G., Ramos, F., Castilho, M. C., et al. (2014). Trends in the use of natural antioxidants in active food packaging: a review. Food Addit. Contam. Part A 31, 374–395. doi: 10.1080/19440049.2013.879215
Sängerlaub, S., Seibel, K., Miesbauer, O., Pant, A., Kiese, S., Rodler, N., et al. (2018). Functional properties of foamed and/or stretched polypropylene-films containing sodium chloride particles for humidity regulation. Polym. Test. 65, 339–351. doi: 10.1016/j.polymertesting.2017.12.002
Santos, J. C. P., Sousa, R. C. S., Otoni, C. G., Moraes, A. R. F., Souza, V. G. L., Medeiros, E. A. A., et al. (2018). Nisin and other antimicrobial peptides: production, mechanisms of action, and application in active food packaging. Innov. Food Sci. Emerg. Technol. 48, 179–194. doi: 10.1016/j.ifset.2018.06.008
Scarfato, P., Avallone, E., Galdi, M. R., Di Maio, L., and Incarnato, L. (2017). Preparation, characterization, and oxygen scavenging capacity of biodegradable α-tocopherol/PLA microparticles for active food packaging applications. Polym. Compos. 38, 981–986. doi: 10.1002/pc.23661
Schaefer, D., and Cheung, W. M. (2018). Smart packaging: opportunities and challenges. Procedia CIRP 72, 1022–1027. doi: 10.1016/j.procir.2018.03.240
Schaude, C., Meindl, C., Fröhlich, E., Attard, J., and Mohr, G. J. (2017). Developing a sensor layer for the optical detection of amines during food spoilage. Talanta 170, 481–487. doi: 10.1016/j.talanta.2017.04.029
Schumann, B., and Schmid, M. (2018). Packaging concepts for fresh and processed meat–recent progresses. Innov. Food Sci. Emerg. Technol. 47, 88–100. doi: 10.1016/j.ifset.2018.02.005
Sen, L., Hyun, K. H., Kim, J. W., Shin, J. W., and Eom, K. H. (2013). The design of smart RFID system with gas sensor for meat freshness monitoring. Adv. Sci. Technol. Lett. 41, 17–20. doi: 10.14257/astl.2013.41.05
Shakeri, A., Sun, N., Badv, M., and Didar, T. F. (2017). Generating 2-dimensional concentration gradients of biomolecules using a simple microfluidic design. Biomicrofluidics 11:44111. doi: 10.1063/1.4991550
Sharma, C., Dhiman, R., Rokana, N., and Panwar, H. (2017). Nanotechnology: an untapped resource for food packaging. Front. Microbiol. 8:1735. doi: 10.3389/fmicb.2017.01735
Shokri, S., and Ehsani, A. (2017). Efficacy of whey protein coating incorporated with lactoperoxidase and α-tocopherol in shelf life extension of Pike-Perch fillets during refrigeration. LWT-Food Sci. Technol. 85, 225–231. doi: 10.1016/j.lwt.2017.07.026
Silva, M., de, F., Lopes, P. S., da Silva, C. F., and Yoshida, C. M. P. (2016). Active packaging material based on buriti oil – Mauritia flexuosa L.f. (Arecaceae) incorporated into chitosan films. J. Appl. Polym. Sci. 133:43210. doi: 10.1002/app.43210
Silva, N. H. C. S., Vilela, C., Almeida, A., Marrucho, I. M., and Freire, C. S. R. (2018). Pullulan-based nanocomposite films for functional food packaging: exploiting lysozyme nanofibers as antibacterial and antioxidant reinforcing additives. Food Hydrocoll. 77, 921–930. doi: 10.1016/j.foodhyd.2017.11.039
Silva-Pereira, M. C., Teixeira, J. A., Pereira-Júnior, V. A., and Stefani, R. (2015). Chitosan/corn starch blend films with extract from Brassica oleraceae (red cabbage) as a visual indicator of fish deterioration. LWT-Food Sci. Technol. 61, 258–262. doi: 10.1016/j.lwt.2014.11.041
Singh, M., and Sahareen, T. (2017). Investigation of cellulosic packets impregnated with silver nanoparticles for enhancing shelf-life of vegetables. LWT 86, 116–122. doi: 10.1016/j.lwt.2017.07.056
Singh, S., Gaikwad, K. K., Lee, M., and Lee, Y. S. (2018a). Temperature sensitive smart packaging for monitoring the shelf life of fresh beef. J. Food Eng. 234, 41–49. doi: 10.1016/j.jfoodeng.2018.04.014
Singh, S., Gaikwad, K. K., and Lee, Y. S. (2018b). Anthocyanin–a natural dye for smart food packaging systems. Korean J Packag Sci Technol 24, 167–180. doi: 10.20909/kopast.2018.24.3.167
Sirocchi, V., Devlieghere, F., Peelman, N., Sagratini, G., Maggi, F., Vittori, S., et al. (2017). Effect of Rosmarinus officinalis L. essential oil combined with different packaging conditions to extend the shelf life of refrigerated beef meat. Food Chem. 221, 1069–1076. doi: 10.1016/j.foodchem.2016.11.054
Smart Packaging Market - Credence Research. Available online at: https://www.credenceresearch.com/report/smart-packaging-market
Smart Packaging Market - Mordor Intelligence. Smart Packaging Market - Growth, Trends and Forecast. (2020- 2025). Mordor Intelligence.
Smart Packaging Market 2018-2023 – OpenPR. Smart Packaging Market 2018-2023 | Global Key Players : 3M, Temp Time, PakSense, American Thermal, Avery Dennison, BASF, International Paper, Huhtamaki. Available online at: https://www.openpr.com/news/1210771/smart-packaging-market-2018-2023-global-key-players-3m-temp-time-paksense-american-thermal-avery-dennison-basf-international-paper-huhtamaki.html (accessed March 2021).
Smolander, M. (2008). “Freshness indicators for food packaging,” in Smart Packaging Technologies for Fast Moving Consumer Goods, eds J. Kerry and P. Butler (Chichester, UK), 111–127.
Smolander, M., Hurme, E., and Ahvenainen, R. (1997). Leak indicators for modified-atmosphere packages. Trends Food Sci. Technol. 8, 101–106. doi: 10.1016/S0924-2244(97)01017-0
Smolander, M., Hurme, E., Latva-Kala, K., Luoma, T., Alakomi, H.-L., and Ahvenainen, R. (2002). Myoglobin-based indicators for the evaluation of freshness of unmarinated broiler cuts. Innov. Food Sci. Emerg. Technol. 3, 279–288. doi: 10.1016/S1466-8564(02)00043-7
Sofi, S. A., Singh, J., Rafiq, S., Ashraf, U., Dar, B. N., and Nayik, G. A. (2018). A comprehensive review on antimicrobial packaging and its use in food packaging. Curr. Nutr. Food Sci. 14, 305–312. doi: 10.2174/1573401313666170609095732
Sohail, M., Sun, D.-W., and Zhu, Z. (2018). Recent developments in intelligent packaging for enhancing food quality and safety. Crit. Rev. Food Sci. Nutr. 58, 2650–2662. doi: 10.1080/10408398.2018.1449731
Souza, V. G. L., Pires, J. R. A., Rodrigues, P. F., Lopes, A. A. S., Fernandes, F. M. B., Duarte, M. P., et al. (2018). Bionanocomposites of chitosan/montmorillonite incorporated with Rosmarinus officinalis essential oil: development and physical characterization. Food Packag. Shelf Life 16, 148–156. doi: 10.1016/j.fpsl.2018.03.009
Srivastava, A. K., Dev, A., and Karmakar, S. (2018). Nanosensors and nanobiosensors in food and agriculture. Environ. Chem. Lett. 16, 161–182. doi: 10.1007/s10311-017-0674-7
Sun, G., Chi, W., Zhang, C., Xu, S., Li, J., and Wang, L. (2019). Developing a green film with pH-sensitivity and antioxidant activity based on κ-carrageenan and hydroxypropyl methylcellulose incorporating Prunus maackii juice. Food Hydrocoll. 94, 345–353. doi: 10.1016/j.foodhyd.2019.03.039
Sun, J., Jiang, H., Wu, H., Tong, C., Pang, J., and Wu, C. (2020). Multifunctional bionanocomposite films based on konjac glucomannan/chitosan with nano-ZnO and mulberry anthocyanin extract for active food packaging. Food Hydrocoll. 107:105942. doi: 10.1016/j.foodhyd.2020.105942
Sun, J., Ye, B., Xia, G., Zhao, X., and Wang, H. (2016). A colorimetric and fluorescent chemosensor for the highly sensitive detection of CO2 gas: experiment and DFT calculation. Sens. Actuat. B Chem. 233, 76–82. doi: 10.1016/j.snb.2016.04.052
Sun, J., Zheng, X., Wu, X., Li, D., Xia, G., Yu, S., et al. (2017). A squaraine-based sensor for colorimetric detection of CO 2 gas in an aqueous medium through an unexpected recognition mechanism: experiment and DFT calculation. Anal. Methods 9, 6830–6838. doi: 10.1039/C7AY02218B
Syngai, G. G., and Ahmed, G. (2019). “Chapter 11 - lysozyme: a natural antimicrobial enzyme of interest in food applications,” in Enzymes in Food Biotechnology, ed M. Kuddus (London: Academic Press), 169–179.
Szulczyński, B., Namieśnik, J., and Gebicki, J. (2017). Determination of odour interactions of three-component gas mixtures using an electronic nose. Sensors 17:2380. doi: 10.3390/s17102380
Tajeddin, B., Ahmadi, B., Sohrab, F., and Chenarbon, H. A. (2018). “Chapter 14 - polymers for modified atmosphere packaging applications,” in Food Packaging and Preservation Handbook of Food Bioengineering, eds A. M. Grumezescu and A. M. Holban (London: Academic Press), 457–499.
Tan, J., and Kerr, W. L. (2019). Characterizing cocoa refining by electronic nose using a Kernel distribution model. LWT 104, 1–7. doi: 10.1016/j.lwt.2019.01.028
Thermographic Measurements Ltd. Thermax. Available online at: http://www.tmchallcrest.com/Default.aspx?A=ProductSearchandPageID=17554121 (accessed March 2021).
Tian, F., Decker, E. A., and Goddard, J. M. (2013). Controlling lipid oxidation of food by active packaging technologies. Food Funct. 4, 669–680. doi: 10.1039/c3fo30360h
Timestrip UK Ind. Timestrip PLUS Duo. Available online at: https://timestrip.com/products/timestrip-duo/ (accessed March 2021).
Titova, T., and Nachev, V. (2020). “Electronic tongue” in the Food Industry. Food Sci. Appl. Biotechnol. 3, 71–76. doi: 10.30721/fsab2020.v3.i1.74
Tokel, O., Yildiz, U. H., Inci, F., Durmus, N. G., Ekiz, O. O., Turker, B., et al. (2015). Portable microfluidic integrated plasmonic platform for pathogen detection. Sci. Rep. 5, 1–9. doi: 10.1038/srep09152
Tominaga, T. (2017). Enhanced sensitivity of lateral-flow test strip immunoassays using colloidal palladium nanoparticles and horseradish peroxidase. LWT 86, 566–570. doi: 10.1016/j.lwt.2017.08.027
Tominaga, T. (2018). Rapid detection of Klebsiella pneumoniae, Klebsiella oxytoca, Raoultella ornithinolytica and other related bacteria in food by lateral-flow test strip immunoassays. J. Microbiol. Methods 147, 43–49. doi: 10.1016/j.mimet.2018.02.015
Tsimogiannis, D., Bimpilas, A., and Oreopoulou, V. (2017). DPPH radical scavenging and mixture effects of plant o-diphenols and essential oil constituents. Eur. J. Lipid Sci. Technol. 119:16003473. doi: 10.1002/ejlt.2016003473
US EPA (U.S. Environmental Protection Agency) (2015). Documentation for Greenhouse Gas Emission and Energy Factors Used in the Waste Reduction Model (WARM), version 13. Available online at: https://archive.epa.gov/epawaste/conserve/tools/warm/pdfs/WARM_Documentation.pdf
Valdés, A., Mellinas, A. C., Ramos, M., Burgos, N., Jiménez, A., Garrigós, M., et al. (2015). Use of herbs, spices and their bioactive compounds in active food packaging. RSC Adv. 5, 40324–40335. doi: 10.1039/C4RA17286H
Vanden Braber, N. L., Di Giorgio, L., Aminahuel, C. A., Díaz Vergara, L. I., Martin Costa, A. O., Montenegro, M. A., et al. (2021). Antifungal whey protein films activated with low quantities of water soluble chitosan. Food Hydrocoll. 110:106156. doi: 10.1016/j.foodhyd.2020.106156
Vanderroost, M., Ragaert, P., Devlieghere, F., and De Meulenaer, B. (2014). Intelligent food packaging: the next generation. Trends Food Sci. Technol. 39, 47–62. doi: 10.1016/j.tifs.2014.06.009
Verma, P., and Yadava, R. D. S. (2015). Polymer selection for SAW sensor array based electronic noses by fuzzy c-means clustering of partition coefficients: Model studies on detection of freshness and spoilage of milk and fish. Sens. Actuat. B Chem. 209, 751–769. doi: 10.1016/j.snb.2014.11.149
Vilela, C., Kurek, M., Hayouka, Z., Röcker, B., Yildirim, S., Antunes, M. D. C., et al. (2018). A concise guide to active agents for active food packaging. Trends Food Sci. Technol. 80, 212–222. doi: 10.1016/j.tifs.2018.08.006
Vilela, C., Moreirinha, C., Domingues, E., Figueiredo, F. M. L., Almeida, A., and Freire, C. S. R. (2019). “Bacterial nanocellulose-based films with antibacterial and conductive properties for active and intelligent food packaging,” in 4th International Symposium on Bacterial Nanocellulose. Porto-Portugal.
Vilela, C., Pinto, R. J. B., Coelho, J., Domingues, M. R. M., Daina, S., Sadocco, P., et al. (2017). Bioactive chitosan/ellagic acid films with UV-light protection for active food packaging. Food Hydrocoll. 73, 120–128. doi: 10.1016/j.foodhyd.2017.06.037
Vu, C. H. T., and Won, K. (2013). Novel water-resistant UV-activated oxygen indicator for intelligent food packaging. Food Chem. 140, 52–56. doi: 10.1016/j.foodchem.2013.02.056
Wan Yahaya, W. A., Abu Yazid, N., Mohd Azman, N. A., and Almajano, M. P. (2019). Antioxidant activities and total phenolic content of Malaysian herbs as components of active packaging film in beef patties. Antioxidants 8:204. doi: 10.3390/antiox8070204
Wang, D., Lv, R., Ma, X., Zou, M., Wang, W., Yan, L., et al. (2018a). Lysozyme immobilization on the calcium alginate film under sonication: development of an antimicrobial film. Food Hydrocoll. 83, 1–8. doi: 10.1016/j.foodhyd.2018.04.021
Wang, H. J., An, D. S., Rhim, J.-W., and Lee, D. S. (2015a). A multi-functional biofilm used as an active insert in modified atmosphere packaging for fresh produce. Packag. Technol. Sci. 28, 999–1010. doi: 10.1002/pts.2179
Wang, J., Wen, Z., Yang, B., and Yang, X. (2017a). Optical carbon dioxide sensor based on fluorescent capillary array. Results Phys. 7, 323–326. doi: 10.1016/j.rinp.2016.12.044
Wang, J., Zhu, L., Zhang, W., and Wei, Z. (2019a). Application of the voltammetric electronic tongue based on nanocomposite modified electrodes for identifying rice wines of different geographical origins. Anal. Chim. Acta 1050, 60–70. doi: 10.1016/j.aca.2018.11.016
Wang, L.-F., and Rhim, J.-W. (2015). Preparation and application of agar/alginate/collagen ternary blend functional food packaging films. Int. J. Biol. Macromol. 80, 460–468. doi: 10.1016/j.ijbiomac.2015.07.007
Wang, S., Liu, X., Yang, M., Zhang, Y., Xiang, K., and Tang, R. (2015b). Review of time temperature indicators as quality monitors in food packaging. Packag. Technol. Sci. 28, 839–867. doi: 10.1002/pts.2148
Wang, W., Li, M., Li, H., Liu, X., Guo, T., Zhang, G., et al. (2018b). A renewable intelligent colorimetric indicator based on polyaniline for detecting freshness of tilapia. Packag. Technol. Sci. 31, 133–140. doi: 10.1002/pts.2358
Wang, W., and Liu, Y. (2019). “Electronic tongue for food sensory evaluation,” in Evaluation Technologies for Food Quality, eds J. Zhong and X. Wang (Duxford: Elsevier), 23–36.
Wang, X., Yong, H., Gao, L., Li, L., Jin, M., and Liu, J. (2019b). Preparation and characterization of antioxidant and pH-sensitive films based on chitosan and black soybean seed coat extract. Food Hydrocoll. 89, 56–66. doi: 10.1016/j.foodhyd.2018.10.019
Wang, Y.-C., Lu, L., and Gunasekaran, S. (2017b). Biopolymer/gold nanoparticles composite plasmonic thermal history indicator to monitor quality and safety of perishable bioproducts. Biosens. Bioelectron. 92, 109–116. doi: 10.1016/j.bios.2017.01.047
Warsiki, E. (2018). Application of chitosan as biomaterial for active packaging of ethylene absorber. {IOP} Conf. Ser. Earth Environ. Sci. 141:12036. doi: 10.1088/1755-1315/141/1/012036
Wen, T., Zheng, L., Dong, S., Gong, Z., Sang, M., Long, X., et al. (2019). Rapid detection and classification of citrus fruits infestation by Bactrocera dorsalis (Hendel) based on electronic nose. Postharvest Biol. Technol. 147, 156–165. doi: 10.1016/j.postharvbio.2018.09.017
White, S. P., Frisbie, C. D., and Dorfman, K. D. (2018). Detection and sourcing of gluten in grain with multiple floating-gate transistor biosensors. ACS sensors 3, 395–402. doi: 10.1021/acssensors.7b00810
Wojnowski, W., Majchrzak, T., Dymerski, T., Gebicki, J., and Namieśnik, J. (2017). Electronic noses: powerful tools in meat quality assessment. Meat Sci. 131, 119–131. doi: 10.1016/j.meatsci.2017.04.240
Wrona, M., Cran, M. J., Nerín, C., and Bigger, S. W. (2017). Development and characterisation of HPMC films containing PLA nanoparticles loaded with green tea extract for food packaging applications. Carbohydr. Polym. 156, 108–117. doi: 10.1016/j.carbpol.2016.08.094
Xia, G., Liu, Y., Ye, B., Sun, J., and Wang, H. (2015). A squaraine-based colorimetric and F– dependent chemosensor for recyclable CO 2 gas detection: highly sensitive off–on–off response. Chem. Commun. 51, 13802–13805. doi: 10.1039/C5CC04755B
Xu, F., Ge, L., Li, Z., Lin, H., and Mao, X. (2017). Development and application of a tyrosinase-based time-temperature indicator (TTI) for determining the quality of turbot sashimi. J. Ocean Univ. China 16, 847–854. doi: 10.1007/s11802-017-3220-0
Yam, K. L., and Lee, D. S. (2012). Emerging Food Packaging Technologies: Principles and Practice. Cambridge, UK: Elsevier.
Yam, K. L., Takhistov, P. T., and Miltz, J. (2005). Intelligent packaging: concepts and applications. J. Food Sci. 70, R1–R10. doi: 10.1111/j.1365-2621.2005.tb09052.x
Yan, Z., Niu, Q., Mou, M., Wu, Y., Liu, X., and Liao, S. (2017). A novel colorimetric method based on copper nanoclusters with intrinsic peroxidase-like for detecting xanthine in serum samples. J. Nanoparticle Res. 19:235. doi: 10.1007/s11051-017-3904-9
Yang, W., Owczarek, J. S., Fortunati, E., Kozanecki, M., Mazzaglia, A., Balestra, G. M., et al. (2016a). Antioxidant and antibacterial lignin nanoparticles in polyvinyl alcohol/chitosan films for active packaging. Ind. Crops Prod. 94, 800–811. doi: 10.1016/j.indcrop.2016.09.061
Yang, X., Tang, Y., Alt, R. R., Xie, X., and Li, F. (2016b). Emerging techniques for ultrasensitive protein analysis. Analyst 141, 3473–3481. doi: 10.1039/C6AN00059B
Yildirim, S., Röcker, B., Pettersen, M. K., Nilsen-Nygaard, J., Ayhan, Z., Rutkaite, R., et al. (2018). Active packaging applications for food. Compr. Rev. Food Sci. Food Saf. 17, 165–199. doi: 10.1111/1541-4337.12322
Yong, H., Wang, X., Bai, R., Miao, Z., Zhang, X., and Liu, J. (2019a). Development of antioxidant and intelligent pH-sensing packaging films by incorporating purple-fleshed sweet potato extract into chitosan matrix. Food Hydrocoll. 90, 216–224. doi: 10.1016/j.foodhyd.2018.12.015
Yong, H., Wang, X., Zhang, X., Liu, Y., Qin, Y., and Liu, J. (2019b). Effects of anthocyanin-rich purple and black eggplant extracts on the physical, antioxidant and pH-sensitive properties of chitosan film. Food Hydrocoll. 94, 93–104. doi: 10.1016/j.foodhyd.2019.03.012
Yoshida, C. M. P., Maciel, V. B. V., Mendonça, M. E. D., and Franco, T. T. (2014). Chitosan biobased and intelligent films: monitoring pH variations. LWT Food Sci. Technol. 55, 83–89. doi: 10.1016/j.lwt.2013.09.015
Yousefi, H., Ali, M. M., Su, H.-M., Filipe, C. D. M., and Didar, T. F. (2018a). Sentinel wraps: real-time monitoring of food contamination by printing DNAzyme probes on food packaging. ACS Nano 12, 3287–3294. doi: 10.1021/acsnano.7b08010
Yousefi, H., Su, H., Ali, M., Filipe, C. D. M., and Didar, T. F. (2018b). Producing covalent microarrays of amine-conjugated DNA probes on various functional surfaces to create stable and reliable biosensors. Adv. Mater. Interfaces 5:1800659. doi: 10.1002/admi.201800659
Yuan, G., Lv, H., Yang, B., Chen, X., and Sun, H. (2015). Physical properties, antioxidant and antimicrobial activity of chitosan films containing carvacrol and pomegranate peel extract. Molecules 20, 11034–11045. doi: 10.3390/molecules200611034
Zabala, S., Castán, J., and Martínez, C. (2015). Development of a time–temperature indicator (TTI) label by rotary printing technologies. Food Control 50, 57–64. doi: 10.1016/j.foodcont.2014.08.007
Zanetti, M., Carniel, T. K., Dalcanton, F., dos Anjos, R. S., Riella, H. G., de Araujo, P. H. H., et al. (2018). Use of encapsulated natural compounds as antimicrobial additives in food packaging: a brief review. Trends Food Sci. Technol. 81, 51–60. doi: 10.1016/j.tifs.2018.09.003
Zhang, J., Zou, X., Zhai, X., Huang, X., Jiang, C., and Holmes, M. (2019a). Preparation of an intelligent pH film based on biodegradable polymers and roselle anthocyanins for monitoring pork freshness. Food Chem. 272, 306–312. doi: 10.1016/j.foodchem.2018.08.041
Zhang, M., Meng, X., Bhandari, B., Fang, Z., and Chen, H. (2015). Recent application of Modified Atmosphere Packaging (MAP) in fresh and fresh-cut foods. Food Rev. Int. 31, 172–193. doi: 10.1080/87559129.2014.981826
Zhang, T., Cao, C., Yu, H., and Liu, Y. (2020). “Design and implementation of dairy food tracking system based on RFID,” in 2020 International Wireless Communications and Mobile Computing (IWCMC) (Limassol: IEEE), 2199–2203.
Zhang, X., Liu, J., Qian, C., Kan, J., and Jin, C. (2019b). Effect of grafting method on the physical property and antioxidant potential of chitosan film functionalized with gallic acid. Food Hydrocoll. 89, 1–10. doi: 10.1016/j.foodhyd.2018.10.023
Zhang, X., Liu, Y., Yong, H., Qin, Y., Liu, J., and Liu, J. (2019c). Development of multifunctional food packaging films based on chitosan, TiO2 nanoparticles and anthocyanin-rich black plum peel extract. Food Hydrocoll. 94, 80–92. doi: 10.1016/j.foodhyd.2019.03.009
Zhao, Z., Tian, F., Liao, H., Yin, X., Liu, Y., and Yu, B. (2016). A novel spectrum analysis technique for odor sensing in optical electronic nose. Sens. Actuat. B Chem. 222, 769–779. doi: 10.1016/j.snb.2015.08.128
Zhao, Z., Xiong, X., Zhou, H., and Xiao, Q. (2019). Effect of lactoferrin on physicochemical properties and microstructure of pullulan-based edible films. J. Sci. Food Agric. 99, 4150–4157. doi: 10.1002/jsfa.9645
Zheng, K., Xiao, S., Li, W., Wang, W., Chen, H., Yang, F., et al. (2019). Chitosan-acorn starch-eugenol edible film: physico-chemical, barrier, antimicrobial, antioxidant and structural properties. Int. J. Biol. Macromol. 135, 344–352. doi: 10.1016/j.ijbiomac.2019.05.151
Zhong, Y. (2019). “2 - Electronic nose for food sensory evaluation,” in Evaluation Technologies for Food Quality Woodhead Publishing Series in Food Science, Technology and Nutrition, eds J. Zhong and X. Wang (London: Woodhead Publishing), 7–22.
Zhong, Y., Godwin, P., Jin, Y., and Xiao, H. (2020). Biodegradable polymers and green-based antimicrobial packaging materials: a mini-review. Adv. Ind. Eng. Polym. Res. 3, 27–35. doi: 10.1016/j.aiepr.2019.11.002
Zhu, D., Ren, X., Wei, L., Cao, X., Ge, Y., Liu, H., et al. (2020). Collaborative analysis on difference of apple fruits flavour using electronic nose and electronic tongue. Sci. Hortic. 260:108879. doi: 10.1016/j.scienta.2019.108879
Zimet, P., Mombrú, Á. W., Mombrú, D., Castro, A., Villanueva, J. P., Pardo, H., et al. (2019). Physico-chemical and antilisterial properties of nisin-incorporated chitosan/carboxymethyl chitosan films. Carbohydr. Polym. 219, 334–343. doi: 10.1016/j.carbpol.2019.05.013
Keywords: biobased, biodegradable, smart, packaging, food, renewable, active, intelligent
Citation: Salgado PR, Di Giorgio L, Musso YS and Mauri AN (2021) Recent Developments in Smart Food Packaging Focused on Biobased and Biodegradable Polymers. Front. Sustain. Food Syst. 5:630393. doi: 10.3389/fsufs.2021.630393
Received: 17 November 2020; Accepted: 26 March 2021;
Published: 29 April 2021.
Edited by:
Bambang Kuswandi, University of Jember, IndonesiaReviewed by:
Luis Cabedo, University of Jaume I, SpainCopyright © 2021 Salgado, Di Giorgio, Musso and Mauri. This is an open-access article distributed under the terms of the Creative Commons Attribution License (CC BY). The use, distribution or reproduction in other forums is permitted, provided the original author(s) and the copyright owner(s) are credited and that the original publication in this journal is cited, in accordance with accepted academic practice. No use, distribution or reproduction is permitted which does not comply with these terms.
*Correspondence: Pablo R. Salgado, cHNhbGdhZG83OEBnbWFpbC5jb20=; Adriana N. Mauri, YW5tYXVyaUBxdWltaWNhLnVubHAuZWR1LmFy
Disclaimer: All claims expressed in this article are solely those of the authors and do not necessarily represent those of their affiliated organizations, or those of the publisher, the editors and the reviewers. Any product that may be evaluated in this article or claim that may be made by its manufacturer is not guaranteed or endorsed by the publisher.
Research integrity at Frontiers
Learn more about the work of our research integrity team to safeguard the quality of each article we publish.