- 1Department of Plant Sciences, Quaid-I-Azam University Islamabad, Islamabad, Pakistan
- 2Department of Biosciences, University of Wah, Wah Cantt, Pakistan
- 3Department of Agronomy, Institute of Food and Agricultural Sciences, University of Florida, Gainesville, FL, United States
During the last two decades the world has experienced an abrupt change in climate. Both natural and artificial factors are climate change drivers, although the effect of natural factors are lesser than the anthropogenic drivers. These factors have changed the pattern of precipitation resulting in a rise in sea levels, changes in evapotranspiration, occurrence of flood overwintering of pathogens, increased resistance of pests and parasites, and reduced productivity of plants. Although excess CO2 promotes growth of C3 plants, high temperatures reduce the yield of important agricultural crops due to high evapotranspiration. These two factors have an impact on soil salinization and agriculture production, leading to the issue of water and food security. Farmers have adopted different strategies to cope with agriculture production in saline and saline sodic soil. Recently the inoculation of halotolerant plant growth promoting rhizobacteria (PGPR) in saline fields is an environmentally friendly and sustainable approach to overcome salinity and promote crop growth and yield in saline and saline sodic soil. These halotolerant bacteria synthesize certain metabolites which help crops in adopting a saline condition and promote their growth without any negative effects. There is a complex interkingdom signaling between host and microbes for mutual interaction, which is also influenced by environmental factors. For mutual survival, nature induces a strong positive relationship between host and microbes in the rhizosphere. Commercialization of such PGPR in the form of biofertilizers, biostimulants, and biopower are needed to build climate resilience in agriculture. The production of phytohormones, particularly auxins, have been demonstrated by PGPR, even the pathogenic bacteria and fungi which also modulate the endogenous level of auxins in plants, subsequently enhancing plant resistance to various stresses. The present review focuses on plant-microbe communication and elaborates on their role in plant tolerance under changing climatic conditions.
Introduction
Drivers of Climate Change
Variations in the atmospheric conditions of a particular region for a long period is known as climate and changes in the atmospheric variables beyond the average value is known as climate change. Different forces are responsible for variations in atmospheric variables. They are broadly categorized into two main groups: anthropogenic and natural drivers. Anthropogenic drivers are well-mixed greenhouse gases (WMGHGs), i.e., CO2, CH4, and nitrous oxide (N2O), whereas solar irradiance and earth orbit, volcanic eruption, and chemical weathering of rocks are examples of natural climate drivers. The greenhouse gasses cause changes in climate by trapping heat, initiating wildfires, and disrupting food supply (Cassia et al., 2018). Volcanic eruptions cause a reduction in the amount of solar radiation, decreasing the temperature in the troposphere and changing atmospheric circulation patterns. Similarly, the chemical weathering of rocks also caused climate cooling by consumption of CO2 (Huh, 2003). The effects of natural drivers on climate change are relatively less compared to anthropogenic impacts (Nelson et al., 2006; Rosa et al., 2015; Myhre et al., 2017). Synthetic fertilizers used in agriculture are responsible for the production of about 80% growth rate of N2O (Ciais et al., 2014). Global rice production is responsible for >10% of total CH4 emission (Smartt et al., 2016).
Variables of Climate Change
The bioindustry of agriculture is greatly influenced by temperature, precipitation, and sunlight. These elements are vulnerable to climate change. A significant increase in global mean temperature (GMT) is induced by artificial drivers as compared to natural drivers of climate (Myhre et al., 2017). It influences precipitation patterns which might lead to drought or inundation. An increase in temperature and decrease in precipitation would affect soil properties (Gelybó et al., 2018). It also affects soil organic carbon as a decrease in water ratio lowers the decomposition process and mineralization of different organic compounds (Karmakar et al., 2016; Onwuka and Mang, 2018). Change in soil temperature also influences soil moisture content, water holding capacity, and soil particle size. Increased soil surface temperature also increases the rate of evaporation and restricts the movement of water in soil profile (Onwuka and Mang, 2018). One study has shown that clay size and organic matter, which decrease in response to increased soil temperature, lead to reduced cation exchange capacity (CEC) (Certini, 2005). Reduced productivity of livestock, horticulture, and cultivated crops is directly related to broad scale alterations in climatic conditions (Malla, 2008).
Negative Impacts of Climate Change on Agricultural Productivity
Climate change and food security are two main issues of the 21st century. The world population is expected to reach 9 billion by the end of 2050, and food requirements are expected to escalate by 85% (FAO, 2017). The agriculture sector is highly threatened by the increased frequency of droughts, heavy rainfall, fluctuations in temperature, salinity, and insect pest attacks on major food crops (Dhankher and Foyer, 2018; Hussain et al., 2019). The FAO declared that 20% of total emissions of GHGs were from agriculture and land use practices. Changes in precipitation patterns, increased frequency of dry spells and drought, rising temperatures, rising sea levels, and temperature variability have negative impacts on agriculture production (Figure 1). Zhao et al. (2017) constructed different simulation models to investigate the effect of increased temperature on yield of four crops by analyzing yield data from 46 research articles and 48 sites on a global scale. He concluded that for each degree increase in global mean temperature (GMT), the production of wheat has been predicted to reduce by 6.0%, rice by 3.2%, maize by 7.4%, and soybean by 3.1% around the world. Depending on geographical regions, the effect of temperature may be positive or negative. In general, a rise in temperature has caused a shift in the phenological development of crops (Teller, 2016), lower water use and nitrogen use efficiency (Ullah et al., 2019), shortening of vegetative and reproductive growth phases of plants (Wang et al., 2018), and decrease in the number of grains and grain-filling duration (Lin et al., 2017). The proliferation of insects and pests at high rates which attack major crops are indirect effects of climate change (Dhankher and Foyer, 2018). The largest grain producers of the world viz. China, the US, and France are facing major infestations of crop pests and consequent yield losses (Shrestha, 2019). This is because increased temperatures and precipitation promote the growth and spread of many pest species.
Climate Change and Salinity
Soil salinity is one of the impacts of climate change in coastal agriculture land, as rises in sea levels has increased salinity from 1 to 33% over 25 consecutive years (Rahman et al., 2018). Sea level rise is associated with the increase in global warming and occurs due to the melting of glaciers and ice sheets as well as the thermal expansion of sea water. Bhuiyan and Dutta (2012) demonstrated a comprehensive understanding of the possible effects of sea level rise with the aid of a hydrodynamic model. Sea level rise includes flooding and salinization and has implications for water resources. Rising sea levels increase the salinity of both surface water and ground water through saltwater intrusion.
Salinity is more evident in semi-arid, coastal agriculture lands, and particularly in arid regions of the world (Kasim et al., 2013; Qadir et al., 2014; Hashem et al., 2018). Changes in weather patterns have resulted in the frequency of recurrent drought or rain falling above the average value for more than a decade (Ayanlade et al., 2018). The upward movement of water in areas with shallow water tables and coastal areas with sea water intrusion resulted in root zone salinity. Changes in precipitation and temperature have a greater influence on soil salinity. In one study conducted by Bannari and Al-Ali (2020), it has been reported that the long-term effects of increased temperatures and decreased precipitation for 30 years showed a positive correlation with increased soil salinity in arid landscape due to less over leaching of salt in the soil as determined by Landsat sensor data including Thematic Mapper (TM), Thematic Mapper Plus (ETM+), and Operational Land Imager (OLI). In coastal agriculture lands, the salinity has increased from 1 to 33% in the last 25 years (Rahman et al., 2018).
On a global level, more than 831 Mha of agriculture land is salt-affected (FAO, 2008); salinity affected 397 M ha, while sodicity affected up to 434 M ha land (Martinez-Beltran, 2005; Setia et al., 2013). Out of the total cultivated and irrigated agriculture land, 50% is affected by high salinity on a global level (Zhu, 2001; Gengmao et al., 2015). Irrigation with saline water, low precipitation, and high evapotranspiration are key factors that cause salinization at a rate of 10% annually to agricultural lands. At this rate more than 50% of arable land would be salinized by 2050 (Jamil et al., 2011). Expansion of salt-affected arable lands has emerged as a major threat toward world food security. About 6% of the cultivated area is destroyed by soil salinization with a continued addition of 1–2% every year around the globe, causing significant yield losses of staple grain crops like maize, rice, and wheat (Munns and Tester, 2008). High rates of evapo-transpiration results in accumulation of salt on the soil surface (Ashraf et al., 2002; Munns, 2002). Most of the underground water used for crops in such conditions become brackish and contains a high content of soluble salt ions, such as Na+ and Cl−, and lower quantities of K+, Ca2+, and NO−3 (Abro et al., 2007; Munns and Tester, 2008). The presence of these ions results in hyper ionic salt stress, which induces metabolic impairment and oxidative stress through the generation of reactive oxygen species (ROS), thus adversely impacting the yield of crops (El-Hendawy et al., 2005; Petrov et al., 2015; Caverzan et al., 2016).
Negative Impacts of Salinity on Crop Physiology
Salinity exerts its detrimental effect on plants by two mechanisms: osmotic stress and ion toxicity. The first effect is short term and occurs due to the uptake of Na+ and Cl− which reduce osmotic potential between root and soil solution and infiltrate water availability (Abbasi et al., 2016). Secondly, high concentrations of Na+, Cl−, or induce ion toxicity that affect nutrient uptake (Tavakkoli et al., 2011). Sodium chloride (NaCl) toxicity is directly related to electrical conductivity (EC), which is an indicator of plant tolerance to salt stress (Isla and Aragüés, 2010). Crop tolerance decreases beyond 2 dSm−1, while some plants grow well even at 8 dSm−1. Beyond this limit, growth and yield are negatively impacted. The adverse effects of salinity on crop morphology, physiology, and yield are listed in Figure 2. Seeds sown in saline conditions are poorly germinated (Ullah and Bano, 2019). Salinity decreases the endogenous level of phytohormones and inhibits seedling establishment (Egamberdieva and Kucharova, 2009). If seeded in the first phase of salinity, shoot growth will be affected in later stages (El Sayed, 2011; Wakeel et al., 2011). Even if seedlings are vigorous and maintain normal growth in the second phase of salinity, yield will be affected at the end. Salinity causes various physiological impairments in plants; due to less stomatal conductivity, C-fixation capacity becomes limited, disturbing the catalytic activities of enzymes that fix C and destroy photosynthetic pigments (Omoto et al., 2012). A significant decrease in shoot and root biomass has been recorded in plants grown in saline soil (Kalhoro et al., 2016; Genc et al., 2019).
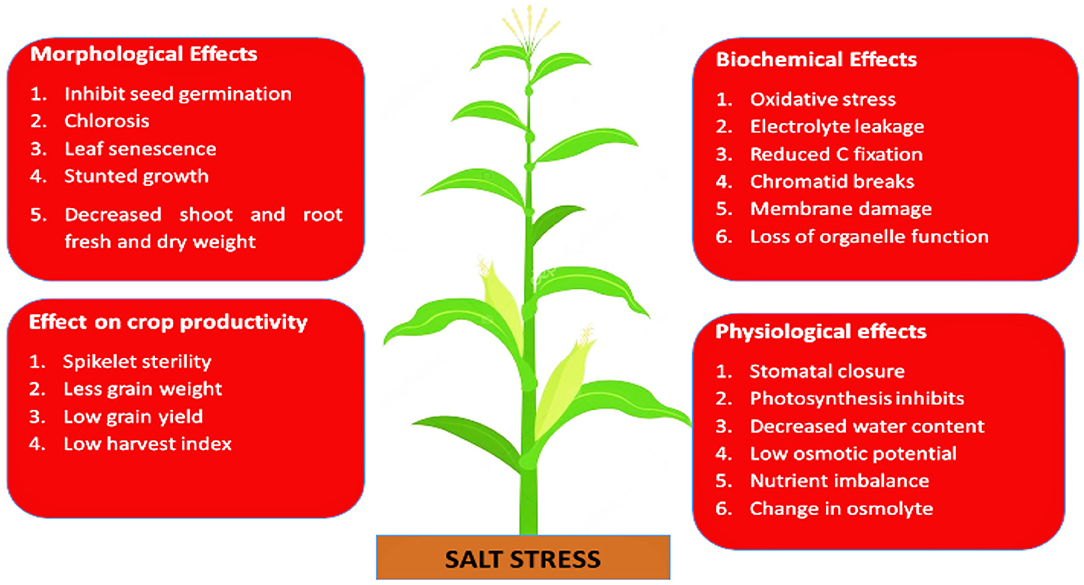
Figure 2. Effects of salt stress on crop morphology and biochemical, physiological, and yield productivity.
Studies showed that salinity (10 mM NaCl) reduced the yield of Triticum aestivum up to 65% (Khan et al., 2004; Shafi et al., 2010). Elevated levels of salt in the root medium reduced the uptake of K, Ca, and Mg (Keutgen and Pawelzi, 2009; Hussin et al., 2013), leading to disturbances in stomatal conductance and transpiration rate (Sumer, 2004). Iron (Fe) is an important metal activator (co-factor) of different antioxidant enzymes (Kumar et al., 2010; Sharma et al., 2012), which helps in regulating life sustaining processes in plants, i.e., photosynthesis and chloroplast biosynthesis. Rabhi et al. (2008) reported that saline sodic soil is deficient in Fe. Plants grown in such soil showed interveinal chlorosis and a declined yield (Lan et al., 2011). Chloride ion (Cl−) is a predominant ion in saline environments and the uptake of N is indirectly correlated to it. Salinity-induced increased Cl uptake is often associated with decreased uptake of nitrate by crops (Wang et al., 2012; Sadale and Karadge, 2013; Yu et al., 2016; Hasana and Mayake, 2017; Kanagaraj and Desingh, 2017).
Oxidative stress induced by salinity as secondary stress leads to the production of ROS, i.e., O−2, OH, and H2O2 (Apel and Hirt, 2004; Voothuluru and Sharp, 2012; Chawla et al., 2013). As a toxic byproduct of aerobic metabolism, ROS are primarily found in the chloroplast, mitochondria, and peroxisome. Their enhanced production cause lipid peroxidation that affects photosynthesis and membrane permeability, damages nucleic acids, inhibits enzymes, and activates programmed cell death (Apel and Hirt, 2004; Tanou et al., 2009; Mishra et al., 2011; Srivastava and Dubey, 2011). Brassica napus and Triticum aestivum grown in saline conditions experienced poor growth and yield due to elevated levels of saline water (Hasanuzzaman et al., 2011a,b).
Strategies to Cope With Salinity Stress Under Changing Climate
Physical Methods
Some physical methods to reclaim salt-affected soil include scraping, deep plowing, deep tillage, sub soiling, sanding, horizon mixing, profile inversion, flushing, and leaching. Scraping is used by farmers to mechanically remove salt from soil surface; however, it is a temporary measure to improve plant growth, and the ultimate disposal of salt remains a major problem. Flushing is used to desalinate soil with surface salt crusts. This method can flush small amounts of salt but has little practical significance. The most common method to reclaim saline soil involves leaching, in which good quality water is supplied to surface soil to leach salt levels (Ghafoor et al., 2012; Yan and Marschner, 2013). The leached salt is then removed with an adequate drainage system.
Chemical Methods
Reclamation by Inorganic Amendment
Saline sodic soil can be reclaimed by the application of Ca2+-containing chemicals which replace Na+ at soil exchangeable sites, followed by leaching with good quality water supply (Gupta and Abrol, 1990). Application of gypsum in soil with low concentrations of carbonate has been extensively studied (Horney et al., 2005; Gharaibeh et al., 2011). It is commonly used to supply Ca2+. However, for soil containing high carbonate content, sulphuric acid is recommended (Horney et al., 2005), which increases soil Ca2+ levels by dissolving CaCO3 in soils (Zia et al., 2007; Vance et al., 2008). The use of inorganic amendments are costly and labor intensive, and is an unhealthy practice for beneficial microbes.
Reclamation by Organic Amendment
The physico-chemical and biological properties of soil can be improved by the application of organic matter amendment, as they accelerate salt leaching and improve aggregate stability and water holding capacity (Walker and Bernal, 2008), thus enabling better plant growth in salt-affected soils. These are cheap and easily organic amendments which contain soil nutrients, organic matter, and enhance both cation exchange capacity and soluble exchangeable K+, which compete with Na+ in saline sodic soil, and limits its entry at exchangeable sites (Walker and Bernal, 2008). Examples of organic amendments include farmyard manure, poultry manure, municipal solid waste compost, and olive mill waste compost (Ahmad et al., 2006; Tejada et al., 2006; Lakhdar et al., 2009). Application of organic amendments have significant effects in an area with low rain fall but cause secondary salinization in other areas where rainfall is abundant (Diacono and Montemurro, 2011).
Biological Methods
Phyto-Reclamation
Phyto-reclamation is an environment-friendly approach to reclaim saline and saline sodic soils (Rabhi et al., 2008; Ashraf et al., 2010). In this method, salt-tolerant plant species are used to reclaim saline soil. A significant improvement in soil organic matter and water holding capacity and decrease in soluble salts and exchangeable Na+ have been recorded (Mishra and Sharma, 2003; Nosetto et al., 2007). Salt-affected soil contains Ca in the form of calcite which is insoluble and unable to displace Na+ from cation exchange complexes. However, the higher partial pressure of CO2 and enhanced production of carbonic acid in the root zone could assist in the solubilization of calcite which provide soluble Ca2+ for exchanging Na+ (Qadir et al., 2005). Root exudates of salt-tolerant plant species contain H+ which enhances Na+ uptake and its subsequent removal from the field. Examples of halophytes include Atriplex spp., Aegiceras corniculatum, Bruguiera gymnorrhiza, Chenopodium album, Plantago media, Rhizophora mucronata, Suaeda australis, and Salsola vermiculata (Qadir et al., 2007; Hasanuzzaman et al., 2014). Phytoremediation has certain limitations in sustainable agricultural productivity, as it changes the microbial community, and takes several growing seasons to remediate polluted soil.
Plant Growth-Promoting Rhizobacteria for Reclamation of Saline Soils
Use of PGPR to reclaim saline soil is a far better approach than chemical and organic fertilizers because of its environment-friendly and persistent nature, with PGPR proliferating slowly and gradually in inoculated soil and ensuring their survival for decades. For this purpose, PGPR should be isolated from their native stress habitat (Sandhya et al., 2010), and reinoculated into affected fields in order to improve the soil physicochemical properties to reclaim it and in turn improve growth and yield of crops (Nosheen and Bano, 2014; Khan and Bano, 2016). A collective approach to exploit the potential of PGPR to alleviate salinity and sodicity has recently received the attention of modern agriculturalists (Arora, 2015). In the current climate change scenario, exploitation of PGPR could be an eco-friendly strategy to promote organic farming (Figure 3).
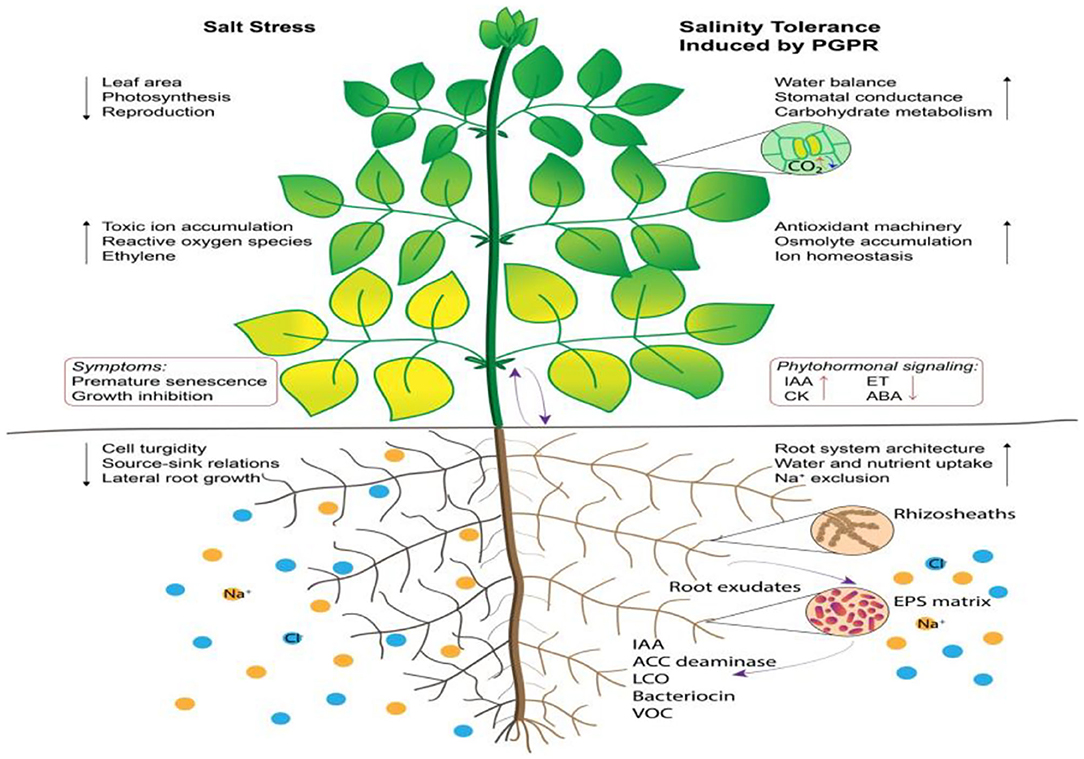
Figure 3. Effect of PGPR on salt tolerant strategy of plants. Source: adapted from Ilangumaran and Smith (2017).
Salt Tolerance Mechanism of PGPR
Under saline habitats, PGPR have developed two potential mechanisms to cope against salt stress (Etesami and Beattie, 2017). The first one is “salt in cytoplasm” while maintaining the osmotic potential of the cell. It was discovered in halobacteria and extreme halophilic archaea (Kunte, 2009). The PGPR accumulates intracellular K+ higher in concentration than Na+ in the environment, as Na+ are exported out through Na/K pumps (Sleator and Hill, 2002; Grant, 2004). The accumulation of K inside the cytoplasm helps in maintaining pH, acts as an activator of intracellular enzymes, and promotes accumulation of other compatible solutes (Epstein, 2003). The second mechanism is termed as “organic osmolyte” in which PGPR accumulate water soluble compatible solutes that maintain turgidity and electrolyte concentration without altering cellular metabolism and protect proteins and membranes at high salt concentration (Roberts, 2005; Tsuzauki et al., 2011; Schroeter et al., 2013; Chen et al., 2015). The osmolytes include glycerols, glutamic acid, glycine betaine, ectoine, hydroxy ectoine, proline, sugars, and trehalose (Khan et al., 2019). PGPR produces a plethora of substances which maintain the composition of outer cellular envelopes. Examples are cyclic lipopeptides (CLP) excreted by Bacillus and Pseudomonas cells (Raaijmakers et al., 2006; Banat et al., 2010).
PGPR, Climate, and Salinity
There are five main reasons why PGPR is an ideal candidate for plants: (i) their participation in C, N, P, and S cycle; (ii) immobilization of toxic ions and solubilization of essential nutrients; (iii) production of plant growth regulators; (iv) use as soil conditioner and nutrition mobilizer (Damodaran et al., 2013); and (v) induction of salinity tolerance in plants by modulating higher enzymatic activities (Kannan et al., 2015). It is a cheap and eco-friendly approach which consists of living inocula of PGPR already inhabiting the soil. However, selection of the best microbe which proliferates well and improves resistance to abiotic stresses should be the first priority.
Jing et al. (2015) and Delgado-Baquerizo et al. (2016) demonstrated that change in climate (seasonal variation or temperature change) can affect diversity and effectiveness of microorganisms. Indirect effects of changes which affect the functionality and microbial community include plant biodiversity, rate, composition of root exudates of host plants, and plant composition. There are also climate-related modulations of nutrient cycling and changes in the duration of the life cycle of plants which affect microbial functioning and community too.
Chemical Communication Between Plants and Microorganisms
Signaling Molecules of PGPR Origin
The complex network of interkingdom signaling between plants and PGPR is governed by a number of secondary metabolites and root exudates (Bukhat et al., 2020; Khan et al., 2020a). The nature of such communication is dynamic and influenced by a number of environmental factors. For survival and proliferation of both plants and PGPR, this cross talk is necessary because it has many advantages (the details of which are given in Figure 4). For interkingdom signaling the first signal that was exchanged between the host plant and its microbial partner was the flavonoid (2-phenyl-1, 4-benzopyrone derivatives) of plant origin (Rossen et al., 1985; AL-Kahtani et al., 2020). This molecule induces bacterial nod gene expression. Upon activation, nod gene code LCOs are secreted into bacteroids and act as a signal for the initiation of nodules. Myc-LCOs have a role in AMF symbiotic association with plants. Another interkingdom signaling system recently identified in plant-associated bacteria is based on HEHEAA molecule, which is spontaneously formed from plant-derived ethanolamine. It acts as a signal for LuxR solo, PipR, which is the co-inducer of pipA in plant root endophytic bacterium Pseudomonas GM79 (Coutinho et al., 2018). Bacteria produce extracellular chemical signals, which control different phenotypes in bacteria and also help in establishing an association with plants. The idea of quorum sensing (QS) signal molecules came from the discovery of autoinduction in marine bacteria Vibrio fischeri. In this phenomenon, bacteria produce an autoinducer signal called homoserine lactones (AHLs) which induces bioluminescence when population density reaches a specific threshold level. So, this phenomenon was named autoinduction. Primary signal molecules generated by bacteria in QS are autoinducers which are either AHLs, quinoline signals, autoinducer-2 (AI-2), auto inducing peptides (AIPs), cyclic dipeptide, indole, short peptides, diffusible signal factors (DSF), or pheromones. AHLs were the first autoinducers identified in gram negative bacteria that regulate the QS phenomenon through LuxI/LuR regulatory proteins. In gram positive bacteria this process is regulated by small peptide autoinducers.
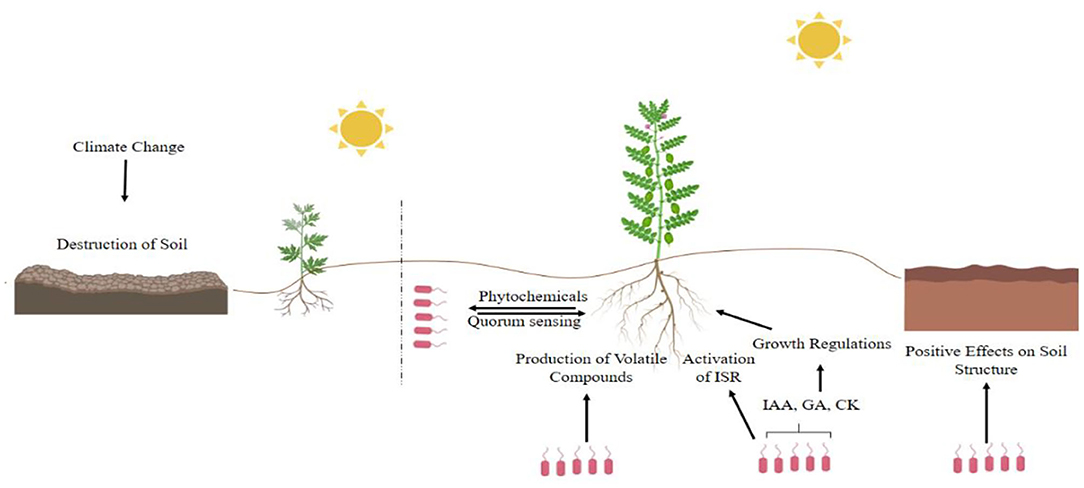
Figure 4. Signaling and communication between host plants and rhizosphere bacteria. This chemical communication results in the formation of improved plant growth and stress resistance and also improves the soil structure and porosity under saline conditions.
Antibiotics are another potential class of bacterial signaling molecule. Ethanolamine acetamide is an active inducer for the PipR transcription regulator in root endophyte Pseudomonas sp. GM79 (Coutinho et al., 2018). It is also present in leaf macerates of Populus. Microbes secrete phytohormone that act as growth and defense regulators to plants, i.e., gibberellic acid (GA), cytokinin (CK), abscisic acid (ABA), indole acetic acid (IAA), and jasmonic acid (JA). Volatile compounds (VOCs) are another class of molecules present in extracellular products of microbes that modulate plant growth and maintain soil health (Figure 5). These include terpenes, nitrogen compounds, pyrazines, indole, and volatile sulfur compounds (Pandey et al., 2013; Groenhagen et al., 2014; Song et al., 2015; Tyc et al., 2015). EPS secreting PGPR helps in stabilizing soil physicochemical properties, improving water holding capacity and CEC (Upadhyay et al., 2012). Some secondary metabolites act as signaling molecules, i.e., in response to flavonoids present in root exudates, and PGPR secrete nod factor which initiates nodule formation in host plants (Miransari and Smith, 2009). A thuricin compound extracted from B. thuringiensis enhances tolerance of Arabidopsis thaliana against 250 mM NaCl (Subramanian, 2013). Polyamine secreting PGPR if inoculated to plants and promotes root architecture and stomatal conductance against stress conditions (Zhou et al., 2016). The above-mentioned examples of signaling molecules along with thousands of others mediate a complex network of signaling in rhizosphere which may be (i) quorum sensing-based microbial intra or inter species signaling, (ii) plant to microbe via plant based signals, or (iii) signaling from microbes to plants.
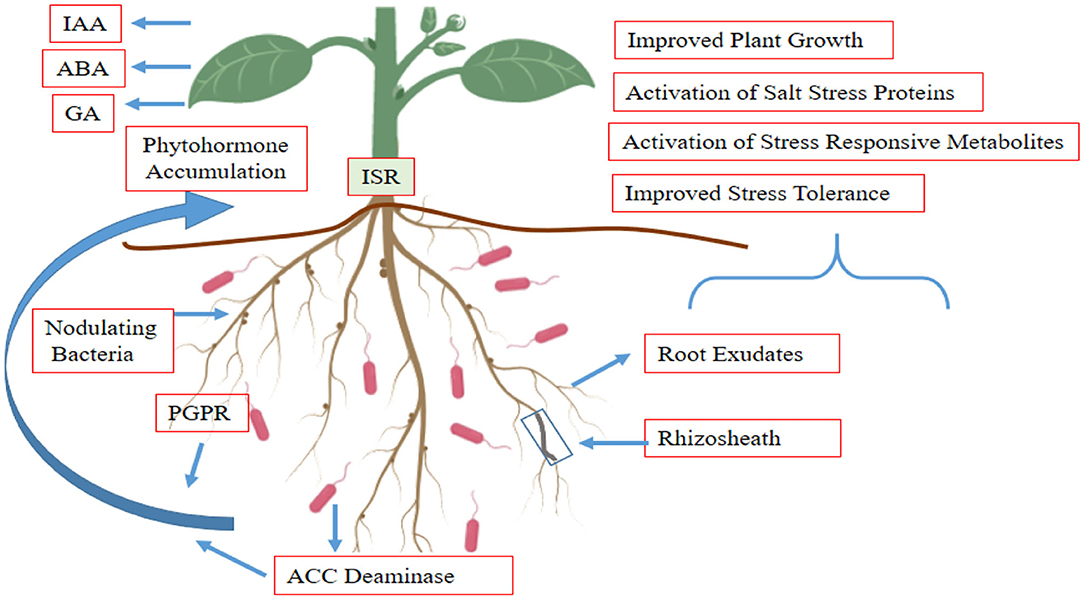
Figure 5. Interactive effects of PGPR and growth substances in maintaining growth under saline conditions.
Signaling Molecules of Plants Origin
Many compounds of root exudates act as general chemoattractant i.e., arabinogalactan protein exuded from plant root tips into the rhizosphere to attract beneficial microbes and repel pathogens. Sugars and organic acids present in the root exudates serve as growth factors and mediate antifungal activities in certain microbes (Khan et al., 2018a). Citric acid and fumaric acid present in root exudates attract microbes and help in biofilm formation (Neal et al., 2012; Zhang et al., 2014). Flavonoids present in root exudates act as an important signal for nodule formation by inducing bacterial nod genes.
Strigolactones (SLs) and cutin monomers are plant-derived signals perceived by both beneficial and pathogenic bacteria (Smith, 2014). SLs belong to plant terpenoid lactones and are involved in plant fungus symbiosis. In Pi-deficient soil, SLs induce the inhibition of shoot branching in host plants and stimulate the growth of arbuscular mycorrhiza fungi (AMF) by increasing hyphal branching toward the host. During AMF infection, Myc factor is also secreted by AMF which stimulates common symbiosis signaling pathway (CSSP) or Sym (Shahid et al., 2020). Myc factors induce lateral root development by increasing root area and by providing starch to fungi to establish symbiosis. As the CSSP pathway triggers, intracellular Ca2+ spiking occurs, which activates CCaMK, which in turn activates transcription of certain genes responsible for symbiosis establishment. The next step is the formation of a pre-symbiotic structure known as hyphopodium by mature hyphae. It facilitates the penetration of AMF into the roots. RAM2 gene is responsible for the formation of hyphopodium. This gene encodes glycerol-3-phosphate acyl transferase enzyme which degrades cutin monomers in the plant cell wall. Thus, cutin monomers act as a plant signal for the formation of hyphopodium. At het same time, host root cells form PPA (Pre-penetration apparatus) which direct the path for AMF colonization from hyphopodium to intracellular space, and a new structure is formed called arbuscule. The entering of AMF hyphae into the root cortex and formation of arbuscule is controlled by the Sym pathway.
Another important molecule present in root exudate is lysophosphatidylcholine which regulates the phosphate transporter gene in fungi and ensures the monitoring of Pi available to plants. In the case of ectomycorrhizal fungi (EMF), the signaling molecules that control the association with host plants are of different classes i.e., abietic acid, flavonoid rutin, hesperidin, quercetin, and chrysin compounds in root exudates lead to germination of EMF. These fungi also secrete phytohormones which should influence root morphology before the establishment of a symbiotic structure, as they reduce the growth of lateral roots where EMF establish. EMF also secrete a signaling molecule hypaphorine, an amino acid derivative which induces these morphological changes. Phenolic acids synthesized by plants are involved in plant-microbe symbiotic association, and AMF symbiosis also plays a role in plant defense. Phytoalexins are secreted by wounded plants to repel or kill microbes. Flavonoids act as chemoattractant to rhizobia and induce the expression of nod gene by synthesis of LCOs. Coumarins are phenolic compounds found across a wide variety of plant species and have a role in iron acquisition and modulating root microbiome composition. Using synthetic community (SynCom) inoculation, it was found that the abundance of Pseudomonas was higher in coumarin deficient Arabidopsis as compared to wild type, because wild type generated a specific coumarin, sideretin, which had a toxic effect by generating H2O2 so it caused toxicity to Pseudomonas strain (Khan et al., 2019; Voges et al., 2019).
Benzoxazinoids belong to indole-derived metabolites in plants that control belowground and above ground interactions. Correlating LC-MS metabolite profiling with operation taxonomic unit (OUT) sequencing data showed that benzoxazinoid stimulates the abundance of Methylophilaceae bacteria while repressing the abundance of Xanthomonadaceae bacteria. Camalexin is another indole compound which can modulate the functionality of root associated microbes (Khan et al., 2020b). Plant microbe interaction assay showed that this compound plays a key role in modulating the effectiveness of growth promoting bacteria because camalexin deficient Arabidopsis mutants were unable to receive growth promoting potentials that were provided by mutualistic strains. As root exudate shape microbe community, at the same time microbes also influence the root exudate composition. For example, microbe's metabolite 2,4-DAPG and zearalenone alter the amino acid composition of tomato in order to maintain osmoregulation (Weller et al., 2007).
Secondary Metabolites Nature on the Basis of Function
A diverse group of bioactive metabolites are produced by PGPR which are not important for normal growth and development but play an important role in stress conditions. On the basis of function, they are categorized into growth hormones, chelating compounds, antibiotics, antifungal, antitumor, and pigment compounds (Guo et al., 2013; Olanrewaju et al., 2017; Asadullah et al., 2020). Examples of such bioactive metabolites include 2, 4-diacetylphloroglucinol (DAPG), amphisin, HCN, phenazine, pyoluteorin, tensin, oligomycin A, xanthobaccin, and zwittermicin. One important role of siderophore secreting PGPR is their ability to reduce Fe availability to phytopathogens and render their proliferation (Olanrewaju et al., 2017). P. putida secretes Putidacin siderophore which is effective against phytopathogens (Parret et al., 2003). Some metabolites are soluble in nature, i.e., ribosomal peptides, non-ribosomal peptides, and polyketides. Pyoverdine is an example of a non-ribosomal peptide, which acts as an iron chelating compound (Hider and Kong, 2010; Khan et al., 2018b).
Positive Impacts of Chemical Communication on Agricultural Productivity
Literature has reported on the potential role of PGPR in marinating the growth and yields of crops under different stress conditions (Table 1). GC-MS analysis showed that Cronobacter sp. JZ38 produce indole and sulfur VOCs which possess volatile mediated antagonistic activity against Phytophthora infestans in plate assay and also act as growth inducer by promoting root and shoot biomass in Arabidopsis thaliana under contact assay (Eida et al., 2020). Due to the difference in AHLs structure, plant response also varies. AHL with short acyl chains increase root growth and primary root elongation in Arabidopsis thaliana, while AHL with long acyl chains induce resistance. This phenomenon is known as AHL priming. Rodríguez et al. (2020) evaluated the antagonistic behavior of Pseudomonas segetis P6 (isolated from Salicornia europaea rhizosphere) against phytopathogens. This bacterium produced an enzyme penicillin acylase which had degraded AHLs extracted from crude extract of bacterial phytopathogens. The strain P6 inhibited the virulence caused by pathogens through secretions of AHLs. When plants were observed, no symptoms of soft rot were found in potato tuber and carrot slice assay. An experiment was conducted on Trichoderma asperellum and B. amyloliquefaciens following simultaneous and sequential inoculation method (Karuppiah et al., 2019). The expression of defense-related vital genes and secondary metabolites were upregulated in Trichoderma and down regulated in Bacillus. Thiamine, an important cofactor for the biosynthesis of IAA in plants, was upregulated in Trichoderma and increased root length in maize plants' under biotic stress condition. Pipecolic acid was detected under a simultaneous inoculation of plants with beneficial microbes that induced systemic acquired resistance (SAR). Aminoglycoside is a low molecular weight antibiotic and pyocyanin is a pigment producing molecule secreted by P. aeruginosa; both act as signaling molecules and induce the development of biofilm by rhizobia species. Cis-2-dodecenoic acid is a type of diffusive signal factor (DSF) secreted by Burkholderia cenocepacia. It inhibits the growth of fungal pathogens such as Candida albicans. Luteolin is a strigolactone present in root exudates of Medicago truncatula and acts as a chemoattractant for rhizobia to induce nod gene expression. Wild type DIMBOA maize produces more benzoxazinoid and attracts more P. putida than DIMBOA deficient mutant maize. It was demonstrated in a study that the spraying of leaf spot pathogen of tomato (P. syringae) on Arabidopsis thaliana resulted in a higher proportion of malic acid in root exudates. The presence of aromatic acid in root exudates recruited a large number of plant growth-promoting B. subtilis. Root exudates of Ocimum basilicum contain rosmarinic acid which showed antagonistic activity against pathogenic Pythium. Hao et al. (2016) reported that volatiles released from B. amyloliquefaciens FZB42 promoted biomass of Arabidopsis thaliana, and a study conducted by Asari et al. (2016) revealed that seedlings of Arabidopsis thaliana exhibited a 2-fold increase in fresh and dry weight after 18 days of exposition to volatiles emitted from B. amyloliquefaciens.
PGPR secrete and modulate the concentration of auxin (IAA), cytokinin, gibberellins (GA), ethylene, and abscisic acids (ABA) in plants which regulate various metabolic activities (Egamberdieva and Kucharova, 2009; Waadt et al., 2015; Wani et al., 2016; Khan et al., 2018a). IAA, a physiologically active hormone produced by almost 80% soil microflora, utilizes tryptophan as precursor molecule (Li et al., 2018; Khan et al., 2020a). This hormone is primarily involved in cell division, morphogenesis, elongation of plants, and ultimately improved growth of shoots and yield (Asim et al., 2013; Ahemad and Kibret, 2014; Bhardwaj et al., 2014). Pseudomonas sp. are well-known IAA producers (Reetha et al., 2014; Kumar et al., 2015; Khan et al., 2020b), along with the secretion of organic acids like gluconic acid which solubilize insoluble phosphate and ease their availability to plants (Otieno et al., 2015; Mishra et al., 2018; Sarkar et al., 2018). Spaepen et al. (2008) reported morpho-physiological changes in root architecture upon inoculation with Azospirillum strain which was tested in vitro for phytohormone (IAA, GA, and Cytokinin) production potential. A similar outcome on wheat root morphology was demonstrated by Sadeghi et al. (2012) after being treated with Streptomyces. Another study to strengthen PGPR‘s role in plant growth promotion by signaling and regulating phytohormone level was presented by Arkhipova et al. (2007), who found remarkable signaling of cytokinin from root to shoot after root inoculation with cytokinin synthesizing bacteria B. subtilis.
GA facilitates germination and elongation of stem, flower, and fruit setting (Cassán et al., 2001; Hayat et al., 2010; Pandya and Desai, 2014). PGPR also secrete abscisic acid, which stimulates growth activities under stress. It is secreted by many PGPR such as Azospirillum brasilense, Bacillus subtilis, Bacillus licheniformis, and Pseudomonas putida (Cohen et al., 2008; Dos Santos et al., 2020; Kousar et al., 2020). Ethylene is a gaseous hormone which controls fruit ripening (Masood et al., 2012; Nazar et al., 2014). However, under salt stress elevated levels of ethylene arrest different physiological activities of plants. PGPR modulate ethylene level by hydrolyzing ACC into NH3 and α-ketobutyrate (Siddikee et al., 2010; Gamalero and Glick, 2015; Ali and Kim, 2018) and reduce the deleterious effect of ethylene under salinity stress (Bal et al., 2013; Han et al., 2015). Under salinity stress, rice growth is significantly increased when treated with ABA producing PGPR over control (Shahzad et al., 2017; Billah et al., 2020). It was confirmed by Zhou et al. (2017) when he inoculated Chrysanthemum with ABA producing B. licheniformis under saline condition; a significant increase in seedling establishment, biomass, and photosynthesis was recorded over control plants.
One of the most important mechanisms to combat salinity stress is the production of compatible solutes. These are low molecular weight compounds that have a key role in mitigating the adverse effect of salt stress. Proline is the most studied osmolyte under salt stress, which has been reported in plants inoculated with PGPR. It has a synergetic effect on the production of other computable solutes under induced salt stress. For example, proline is involved in the synthesis of trehalose, another osmolyte in plants when inoculated with PGPR (Chen et al., 2007). Similarly, an increased concentration of total soluble sugar modulated by leaf protein content has been recorded in plants treated with PGPR (Upadhyay and Singh, 2015). Another class of osmoprotectant is glycine betaine which maintains higher leaf water potential during stress conditions (Hoque et al., 2007; Peng et al., 2008). Inoculation of maize with rhizobacteria significantly improved proline, total soluble sugar, and phenolic contents under salt-affected field condition (Iqbal et al., 2016). De novo synthesis of proline in Azospirillum can boost the wheat plant‘s endogenous proline accumulation (Han and Lee, 2005). Paul et al. (2005) found that P. pseudoalcaligenes combat high salt concentration via the production of Ala, Gly, Glu, Ser, Thr, and Asp amino acids, which help in osmotic adjustments as well as in the correct folding of polypeptide chains for maintaining structurally stable proteins under adverse environmental conditions (Streets et al., 2006). The strategy adapted for stress alleviation by these microbes includes modification of chemical constituents through deposition or alteration in capsular compounds to enhance water retention and carbon diffusion by providing micro-environment as reported in P. fluorescens (Ali et al., 2009). Moreover, salt-responsive genes' expression in a halophilic PGPR, such as P. pseudoalcaligenes and B. subtilis, encode a wide range of stress proteins which behave as chaperones for cellular macromolecules (Volker et al., 1994; Paul et al., 2005).
Climate change has adversely affected soil nutrient and fertility status (Pareek, 2017). This problem can be overcome by the application of PGPR which improve soil nutrient status and their uptake into plants through the release of nitrogenase, phosphatase, dehydrogenase, siderophores, and exopolysaccharides (Arshad et al., 2008; Cohen et al., 2009). Decline in CO2 fixation due to hyper osmosis cause the production of reactive oxygen species (ROS), which alter the physiology and histology of plants through changes in normal metabolic activities, damage to the phospholipid bilayer, and ultimately cell apoptosis (Johnson et al., 2003; Hichem et al., 2009; Miller et al., 2010; Halo et al., 2015). PGPR activates antioxidants and defense-related enzymes in plants and help in the mitigation of ROS (Chakraborty et al., 2013). To protect plants against oxidative stress and mimic the harmful effect of H2O2, plants have been inoculated with PGPR (Kim et al., 2005; Upadhyay et al., 2012). The inoculation has significantly enhanced the antioxidant enzymatic system and mitigated the adverse effect of salt stress as compared to control (Damodaran et al., 2013; Jha and Subramanian, 2013). B. megaterium and Enterobacter sp. modulate ROS scavenging enzyme (SOD, CAT, APX) in Abelmoschus esculentus when grown under saline condition (Habib et al., 2016; Khan et al., 2018b).
Conclusion and Future Perspectives
Climate change has negatively impacted the duration and intensity of seasonal changes e.g., a reduced winter season with less snow fall followed by abrupt run off in early spring, subsequently resulting in flood inundation in different areas of the world. The increased temperature during daytime in summer resulted in high evapo-transpiration that resulted in the accumulation of salt on the soil surface. It has adversely impacted physicochemical properties of agricultural land. PGPR had stimulatory effects on the growth and physiology of the plant under induced salt stress. They increased the availability of essential nutrients K and Ca, enhanced Fe and Zn in the rhizosphere soil, and enhanced the uptake by roots and its translocation to leaves and grain. The PGPR induced salt tolerances is mediated by the production of secondary metabolites which have antimicrobial and anti-larvicidal and act as signaling molecules. It is mediated by the presence of ACC deaminase and IAA production. PGPR induces tolerance against salinity by enhancing root proliferation and root biomass and improving the water use efficiency of wheat. This minimizes the oxidative stresses but improves osmoregulation. PGPR, the natural microflora, have adopted different strategies to cope with the changing climate. Under salt stress they produce different kinds of bioactive metabolites which help in their persistence as well as providing a synergetic effect to plant growth and yield. Therefore, ideal candidates of PGPR should be selected for better agriculture productivity in salt-affected areas.
Author Contributions
AU: formal analysis, investigation, methodology, software, validation, visualization, writing-original draft, writing-review, and editing. AB: conceptualization, data curation, formal analysis, investigation, project administration, resources, supervision, writing-original draft, writing-review, and editing. NK: conceptualization, data curation, formal analysis, project administration, resources, software, supervision, validation, writing-original draft, writing-review, and editing. All authors contributed to the article and approved the submitted version.
Conflict of Interest
The authors declare that the research was conducted in the absence of any commercial or financial relationships that could be construed as a potential conflict of interest.
References
Abbasi, H., Jamil, M., Haq, A., Ali, S., Ahmad, R., and MaliK, Z. (2016). Salt stress manifestation on plants, mechanism of salt tolerance and potassium role in alleviating it: a review. Zemdirbyste-Agricul. 103, 229–238. doi: 10.13080/z-a.2016.103.030
Abro, S. A., Mahar, A. R., and Talpur, K. H. (2007). Effective use of brackish water on saline-sodic soils for rice and wheat production. Pakistan J. Botany 39, 2601–2606.
Ahemad, M., and Kibret, M. (2014). Mechanisms and applications of plant growth promoting rhizobacteria: current perspective. J. King Saud University-Sci. 26, 1–20. doi: 10.1016/j.jksus.2013.05.001
Ahmad, S., Ghafoor, A., Qadir, M., and Aziz, M. A. (2006). Amelioration of a calcareous saline-sodic soil by gypsum application and different crop rotations. Int. J. Agric. Biol. 8, 142–146.
Ali, S., and Kim, W. C. (2018). Plant growth promotion under water: decrease of waterlogging-induced ACC and ethylene levels by ACC deaminase-producing bacteria. Front. Microbiol. 9:1096. doi: 10.3389/fmicb.2018.01096
Ali, S. Z., Sandhya, V., Grover, M., Kishore, N., Rao, L. V., and Venkateswarlu, B. (2009). Pseudomonas sp. strain AKM-P6 enhances tolerance of sorghum seedlings to elevated temperatures. Biol. Fertility Soils 46, 45–55. doi: 10.1007/s00374-009-0404-9
AL-Kahtani, M. D., Fouda, A., Attia, K. A., Al-Otaibi, F., Eid, A. M., Ewais, E. E., et al. (2020). Isolation and characterization of plant growth promoting endophytic bacteria from desert plants and their application as bioinoculants for sustainable agriculture. Agronomy 10:1325. doi: 10.3390/agronomy10091325
Apel, K., and Hirt, H. (2004). Reactive oxygen species: metabolism, oxidative stress, and signal transduction. Annu. Rev. Plant Biol. 55, 373–399. doi: 10.1146/annurev.arplant.55.031903.141701
Arkhipova, T. N., Prinsen, E., Veselov, S. U., Martinenko, E. V., Melentiev, A. I., and Kudoyarova, G. R. (2007). Cytokinin producing bacteria enhance plant growth in drying soil. Plant Soil 292, 305–315. doi: 10.1007/s11104-007-9233-5
Arora, N. K. (2015). Plant Microbes Symbiosis (eds): Applied Facets. New Delhi: Springer. doi: 10.1007/978-81-322-2068-8
Arshad, M., Shaharoona, B., and Mahmood, T. (2008). Inoculation with Pseudomonas spp. containing ACC-deaminase partially eliminates the effects of drought stress on growth, yield, and ripening of pea (Pisum sativum L.). Pedosphere 18, 611–620. doi: 10.1016/S1002-0160(08)60055-7
Asadullah, Bano, A., and Janjua, H. T. (2020). “Microbial secondary metabolites and defense of plant stress,” in Microbial Service in Restoration Ecology, vol 1, eds J. S. Singh and S. R. Vimal (Amsterdam: Elsevier), 37–46. doi: 10.1016/B978-0-12-819978-7.00003-8
Asari, S., Matzén, S., Petersen, M. A., Bejai, S., and Meijer, J. (2016). Multiple effects of Bacillus amyloliquefaciens volatile compounds: plant growth promotion and growth inhibition of phytopathogens. FEMS Microbiol. Ecol. 92:fiw070. doi: 10.1093/femsec/fiw070
Ashraf, M. Y., Akhtar, K., Sarwar, G., and Ashraf, M. (2002). Evaluation of arid and semi-arid ecotypes of guar (Cyamopsis tetragonoloba L.) for salinity (NaCl) tolerance. J. Arid Environ. 52, 473–482. doi: 10.1006/jare.2002.1017
Ashraf, M. Y., Ashraf, M., Mahmood, K., Akhter, J., Hussain, F., and Arshad, M. (2010). “Phytoremediation of saline soils for sustainable agricultural productivity,” in Plant adaptation and Phytoremediation (Dordrecht: Springer), 335–355. doi: 10.1007/978-90-481-9370-7_15
Asim, M., Aslam, M., Bano, A., Munir, M., Majeed, A., and Abbas, S. H. (2013). Role of phytohormones in root nodulation and yield of soybean under salt stress. Am. J. Res. Commun. 1, 191–208.
Ayanlade, A., Radeny, M., Morton, J. F., and Muchaba, T. (2018). Rainfall variability and drought characteristics in two agro-climatic zones: an assessment of climate change challenges in Africa. Sci. Total Environ. 630, 728–737. doi: 10.1016/j.scitotenv.2018.02.196
Bal, H. B., Naya, K. L., Das, S., and Adhya, T. K. (2013). Isolation of ACC deaminase producing PGPR from rice rhizosphere and evaluating their plant growth promoting activity under salt stress. Plant Soil 366, 93–105. doi: 10.1007/s11104-012-1402-5
Banat, I. M., Franzetti, A., Gandolfi, I., Bestetti, G., Martinotti, M. G., Fracchia, L., et al. (2010). Microbial biosurfactants production, applications and future potential. Appl. Microbiol. Biotechnol. 87, 427–444. doi: 10.1007/s00253-010-2589-0
Bannari, A., and Al-Ali, Z. M. (2020). Assessing climate change impact on soil salinity dynamics between 1987–2017 in arid landscape using Landsat TM, ETM+ and OLI data. Remote Sensing 12:2794. doi: 10.3390/rs12172794
Bhardwaj, D., Ansari, M. W., Sahoo, R. K., and Tuteja, N. (2014). Biofertilizers function as Key player in sustainable agriculture by improving soil fertility, plant tolerance and crop productivity. Microb. Cell Fact. 13:66. doi: 10.1186/1475-2859-13-66
Bhuiyan, M., and Dutta, D. (2012). Assessing impacts of sea level rise on river salinity in the gorai river network, Bangladesh. Estuarine Coast. Shelf Sci. 96, 219–227. doi: 10.1016/j.ecss.2011.11.005
Billah, M., Khan, M., Bano, A., Nisa, S., Hussain, A., Dawar, K. M., et al. (2020). Rock phosphate-enriched compost in combination with rhizobacteria; a cost-effective source for better soil health and wheat (Triticum aestivum) productivity. Agronomy 10:1390. doi: 10.3390/agronomy10091390
Bukhat, S., Imran, A., Javaid, S., Shahid, M., Majeed, A., and Naqqash, T. (2020). Communication of plants with microbial world: exploring the regulatory networks for PGPR mediated defense signaling. Microbiol. Res. 238:126486. doi: 10.1016/j.micres.2020.126486
Cassán, F., Bottini, R., Schneider, G., and Piccoli, P. (2001). Azospirillum brasilense and Azospirillum lipoferum hydrolyze conjugates of GA20 and metabolize the resultant aglycones to GA1 in seedlings of rice dwarf mutants. Plant Physiol. 125, 2053–2058. doi: 10.1104/pp.125.4.2053
Cassia, R., Nocioni, M., Correa-Aragunde, N., and Lamattina, L. (2018). Climate change and the impact of greenhouse gasses: CO2 and NO, friends and foes of plant oxidative stress. Front. Plant Sci. 9:273. doi: 10.3389/fpls.2018.00273
Caverzan, A., Casassola, A., and Brammer, S. P. (2016). Antioxidant responses of wheat plants under stress. Genet. Mol. Biol. 39, 1–6. doi: 10.1590/1678-4685-GMB-2015-0109
Certini, G. (2005). Effects of fire in properties of forest soils: a review. Oecologia 143, 1–10. doi: 10.1007/s00442-004-1788-8
Chakraborty, U., Chakraborty, B. N., Chakraborty, A. P., and Dey, P. L. (2013). Water stress amelioration and plant growth promotion in wheat plants by osmotic stress tolerant bacteria. World J. Microbiol. Biotechnol. 29, 789–803. doi: 10.1007/s11274-012-1234-8
Chawla, S., Jain, S., and Jain, V. (2013). Salinity induced oxidative stress and antioxidant system in salt-tolerant and salt-sensitive cultivars of rice (Oryza sativa L.). J. Plant Biochem. Biotechnol. 22, 27–34. doi: 10.1007/s13562-012-0107-4
Chen, J. H., Chi, M. C., Lin, M. G., Lin, L. L., and Wang, T. F. (2015). Beneficial effect of sugar osmolytes on the refolding of guanidine hydrochloride-denatured trehalose-6-phosphate hydrolase from Bacillus licheniformis. BioMed Res. Int. 2015:806847. doi: 10.1155/2015/806847
Chen, M., Wei, H., Cao, J., Liu, R., Wang, Y., and Zheng, C. (2007). Expression of Bacillus subtilis proBA genes and reduction of feedback inhibition of proline synthesis increases proline production and confers osmotolerance in transgenic Arabidopsis. BMB Rep. 40, 396–403. doi: 10.5483/BMBRep.2007.40.3.396
Ciais, P., Sabine, C., Bala, G., Bopp, L., Brovkin, V., Canadell, J., et al. (2014). “Carbon and other biogeochemical cycles,” in Climate change 2013: the physical science basis. Contribution of Working Group I to the Fifth Assessment Report of the Intergovernmental Panel on Climate Change (Cambridge: Cambridge University Press), 465–570.
Cohen, A. C., Bottini, R., and Piccoli, P. N. (2008). Azospirillum brasilense Sp 245 produces ABA in chemically-defined culture medium and increases ABA content in arabidopsis plants. Plant Growth Regul. 54, 97–103. doi: 10.1007/s10725-007-9232-9
Cohen, A. C., Travaglia, C. N., Bottini, R., and Piccoli, P. N. (2009). Participation of abscisic acid and gibberellins produced by endophytic Azospirillum in the alleviation of drought effects in maize. Botany 87, 455–462. doi: 10.1139/B09-023
Coutinho, B. G., Mevers, E., Schaefer, A. L., Pelletier, D. A., Harwood, C. S., Clardy, J., et al. (2018). A plant-responsive bacterial-signaling system senses an ethanolamine derivative. Proc. Natl. Acad. Sci. U.S.A. 115, 9785–9790. doi: 10.1073/pnas.1809611115
Dabrowska, G., Hrynkiewicz, K., Trejgell, A., and Baum, C. (2017). The effect of plant growth-promoting rhizobacteria on the phytoextraction of Cd and Zn by Brassica napus L. Int. J. Phytoremed. 19, 597–604. doi: 10.1080/15226514.2016.1244157
Damodaran, T., Rai, R. B., Jha, S. K., Sharma, D. K., Mishra, V. K., Dhama, K., et al. (2013). Impact of social factors in adoption of CSR BIO-A cost effective, eco-friendly bio-growth enhancer for sustainable crop production. South Asian J. Experi. Biol. 3, 158–165.
Darma, R., Purnamasari, I., Agustina, D., Pramudito, T. E., Sugiharti, M., and Suwanto, A. (2016). A strong antifungal-producing bacteria from bamboo powder for biocontrol of Sclerotium rolfsii in Melon (Cucumis melo var. amanta). J. Plant Pathol. Microbiol. 7:334. doi: 10.4172/2157-7471.1000334
Delgado-Baquerizo, M., Maestre, F. T., Reich, P. B., Jeffries, T. C., Gaitan, J. J., Encinar, D., et al. (2016). Microbial diversity drives multifunctionality in terrestrial ecosystems. Nat. Commun. 7, 1–8. doi: 10.1038/ncomms10541
Dhankher, O. P., and Foyer, C. H. (2018). Climate resilient crops for improving global food security and safety. Plant Cell Environ. 41, 877–884. doi: 10.1111/pce.13207
Diacono, M., and Montemurro, F. (2011). Long-term effects of organic amendments on soil fertility. Sustain. Agric. 2, 761–786. doi: 10.1007/978-94-007-0394-0_34
Dos Santos, R. M., Diaz, P. A., Lobo, L. L., and Rigobelo, E. C. (2020). Use of plant growth-promoting rhizobacteria in maize and sugarcane: characteristics and applications. Front. Sustain. Food Syst. 4:136. doi: 10.3389/fsufs.2020.00136
Egamberdieva, D., and Kucharova, Z. (2009). Selection for root colonising bacteria stimulating wheat growth in saline soils. Biol. Fertil. Soils 45, 563–571. doi: 10.1007/s00374-009-0366-y
Eida, A. A., Bougouffa, S., L'Haridon, F., Alam, I., Weisskopf, L., Bajic, V. B., et al. (2020). Genome insights of the plant-growth promoting bacterium Cronobacter muytjensii JZ38 with volatile-mediated antagonistic activity against phytophthora infestans. Front. Microbiol. 11:369. doi: 10.3389/fmicb.2020.00369
El Sayed, H. E. S. A. (2011). Influence of salinity stress on growth parameters, photosynthetic activity and cytological studies of Zea mays, L. plant using hydrogel polymer. Agric. Biol. J. Am. 2, 907–920. doi: 10.5251/abjna.2011.2.6.907.920
El-Hendawy, S. E., Hu, Y., Yakout, G. M., Awad, A. M., Hafiz, S. E., and Schmidhalter, U. (2005). Evaluating salt tolerance of wheat genotypes using multiple parameters. Eur. J. Agronomy 22, 243–253. doi: 10.1016/j.eja.2004.03.002
Epstein, W. (2003). The roles and regulation of potassium in bacteria. Prog. Nucleic Acid Res. Mol. Biol. 75, 293–320. doi: 10.1016/S0079-6603(03)75008-9
Etesami, H., and Beattie, G. A. (2017). “Plant-microbe interactions in adaptation of agricultural crops to abiotic stress conditions,” in Probiotics and Plant Health, eds V. Kumar, M. Kumar, S. Sharma, and R. Prasad (Singapore: Springer), 163–200. doi: 10.1007/978-981-10-3473-2_7
FAO (2008). Land and Plant Nutrition Management Service. Available online at: htpp://www.fao.org/ag/agl/agll/spush (accessed September 17, 2020).
Gamalero, E., and Glick, B. R. (2015). Bacterial modulation of plant ethylene levels. Plant Physiol. 169, 13–22. doi: 10.1104/pp.15.00284
Gelybó, G., Tóth, E., Farkas, C., Horel, Á., Kása, I., and Bakacsi, Z. (2018). Potential impacts of climate change on soil properties. AgroKémiaés Talajtan 67, 121–141. doi: 10.1556/0088.2018.67.1.9
Genc, Y., Taylor, J., Lyons, G. H., Li, Y., Cheong, J., Appelbee, M., et al. (2019). Bread wheat with high salinity and sodicity tolerance. Front. Plant Sci. 10:1280. doi: 10.3389/fpls.2019.01280
Gengmao, Z., Yu, H., Xing, S., Shihui, L., Quanmei, S., and Changhai, W. (2015). Salinity stress increases secondary metabolites and enzyme activity in safflower. Indust. Crops Prod. 64, 175–181. doi: 10.1016/j.indcrop.2014.10.058
Ghafoor, A., Murtaza, G., Rehman, M. Z., and Sabir, M. (2012). Reclamation and salt leaching efficiency for tile drained saline-sodic soil using marginal quality water for irrigating rice and wheat crops. Land Degrad. Dev. 23, 1–9. doi: 10.1002/ldr.1033
Gharaibeh, M. A., Eltaif, N. I., and Albalasmeh, A. A. (2011). Reclamation of highly calcareous saline sodic soil using Atriplex halimus and by-product gypsum. Int. J. Phytoremed. 13, 873–883. doi: 10.1080/15226514.2011.573821
Grant, W. D. (2004). Life at low water activity. Philos. Trans. R. Soc. Lond. Series B: Biol. Sci. 359, 1249–1267. doi: 10.1098/rstb.2004.1502
Groenhagen, U., Maczka, M., Dickschat, J. S., and Schulz, S. (2014). Streptopyridines, volatile pyridine alkaloids produced by Streptomyces sp. FORM5. Beilst. J. Organic Chem. 10, 1421–1432. doi: 10.3762/bjoc.10.146
Guo, R. F., Yuan, G. F., and Wang, Q. M. (2013). Effect of NaCl treatments on glucosinolate metabolism in broccoli sprouts. J. Zhejiang University Sci. B 14, 124–131. doi: 10.1631/jzus.B1200096
Gupta, R. K., and Abrol, I. P. (1990). “Salt-affected soils: their reclamation and management for crop production,” in Advances in Soil Science (New York, NY: Springer), 223–228. doi: 10.1007/978-1-4612-3322-0_7
Habib, S. H., Kausar, H., and Saud, H. M. (2016). Plant growth-promoting rhizobacteria enhance salinity stress tolerance in okra through ROS-scavenging enzymes. BioMed Res. Int. 2016:628547. doi: 10.1155/2016/6284547
Halo, B. A., Khan, A. L., Waqas, M., Al-Harrasi, A., Hussain, J., Ali, L., et al. (2015). Endophytic bacteria (Sphingomonas sp. LK11) and gibberellin can improve Solanum lycopersicum growth and oxidative stress under salinity. J. Plant Interact. 10, 117–125. doi: 10.1080/17429145.2015.1033659
Han, H. S., and Lee, K. D. (2005). Plant growth promoting rhizobacteria effect on antioxidant status, photosynthesis, mineral uptake and growth of lettuce under soil salinity. Res. J. Agric. Biol. Sci. 1, 210–215.
Han, Y., Wang, R., Yang, Z., Zhan, Y., Ma, Y., Ping, S., et al. (2015). 1-Aminocyclopropane-1-carboxylate deaminase from Pseudomonas stutzeri A1501 facilitates the growth of rice in the presence of salt or heavy metals. J. Microbiol. Biotechnol. 25, 1119–1128. doi: 10.4014/jmb.1412.12053
Hao, H. T., Zhao, X., Shang, Q. H., Wang, Y., Guo, Z. H., Zhang, Y. B., et al. (2016). Comparative digital gene expression analysis of the Arabidopsis response to volatiles emitted by Bacillus amyloliquefaciens. PLoS ONE 11:e0158621. doidoi: 10.1371/journal.pone.0158621
Hasana, R., and Mayake, H. (2017). Salinity stress alters nutrient uptake and causes the damage of root and leaf anatomy in maize. KnE Life Sci. 3, 219–225. doi: 10.18502/kls.v3i4.708
Hasanuzzaman, M., Hossain, M. A., and Fujita, M. (2011a). Nitric oxide modulates antioxidant defense and the methylglyoxal detoxification system and reduces salinity-induced damage of wheat seedlings. Plant Biotechnol. Rep. 5:353. doi: 10.1007/s11816-011-0189-9
Hasanuzzaman, M., Hossain, M. A., and Fujita, M. (2011b). Selenium-induced up-regulation of the antioxidant defense and methylglyoxal detoxification system reduces salinity-induced damage in rapeseed seedlings. Biol. Trace Elem. Res. 143, 1704–1721. doi: 10.1007/s12011-011-8958-4
Hasanuzzaman, M., Nahar, K., Alam, M., Bhowmik, P. C., Hossain, M., Rahman, M. M., et al. (2014). Potential use of halophytes to remediate saline soils. BioMed Res. Int. 2014:589341. doi: 10.1155/2014/589341
Hashem, A., Alqarawi, A. A., Radhakrishnan, R., Al-Arjani, A. B. F., Aldehaish, H. A., Egamberdieva, D., et al. (2018). Arbuscular mycorrhizal fungi regulate the oxidative system, hormones and ionic equilibrium to trigger salt stress tolerance in Cucumis sativus L. Saudi J. Biol. Sci. 25, 1102–1114. doi: 10.1016/j.sjbs.2018.03.009
Hassan, T. U., Bano, A., and Naz, I. (2017). Alleviation of heavy metals toxicity by the application of plant growth promoting rhizobacteria and effects on wheat grown in saline sodic field. Int. J. Phytoremed. 19, 522–529. doi: 10.1080/15226514.2016.1267696
Hayat, R., Ali, S., Amara, U., Khalid, R., and Ahmed, I. (2010). Soil beneficial bacteria and their role in plant growth promotion: a review. Ann. Microbiol. 60, 579–598. doi: 10.1007/s13213-010-0117-1
Hichem, H., El Naceur, A., and Mounir, D. (2009). Effects of salt stress on photosynthesis, PSII photochemistry and thermal energy dissipation in leaves of two corn (Zea mays L.) varieties. Photosynthetica 47, 517–526. doi: 10.1007/s11099-009-0077-5
Hider, R. C., and Kong, X. (2010). Chemistry and biology of siderophores. Nat. Prod. Rep. 27, 637–657. doi: 10.1039/b906679a
Hoque, M. A., Hoque, M. M., and Ahmed, K. M. (2007). Declining groundwater level and aquifer dewatering in Dhaka metropolitan area, Bangladesh causes and quantification. Hydrogeol. J. 15, 1523–1534. doi: 10.1007/s10040-007-0226-5
Horney, R. D., Taylor, B., Munk, D. S., Roberts, B. A., Lesch, S. M., and Plant, R. E. (2005). Development of practical site-specific management methods for reclaiming salt-affected soil. Comp. Electron. Agricul. 46, 379–397. doi: 10.1016/j.compag.2004.11.008
Huh, Y. (2003). Chemical weathering and climate—a global experiment: a review. Geosci. J. 7, 277–288. doi: 10.1007/BF02910294
Hussain, H. A., Men, S., Hussain, S., Chen, Y., Ali, S., Zhang, S., et al. (2019). Interactive effects of drought and heat stresses on morpho-physiological attributes, yield, nutrient uptake and oxidative status in maize hybrids. Sci. Rep. 9, 1–12. doi: 10.1038/s41598-019-40362-7
Hussin, S., Geissler, N., and Koyro, H. W. (2013). Effect of NaCl salinity on Atriplex nummularia (L.) with special emphasis on carbon and nitrogen metabolism. Acta Physiol. Plantar. 35, 1025–1038. doi: 10.1007/s11738-012-1141-5
Ilangumaran, G., and Smith, D. L. (2017). Plant growth promoting rhizobacteria in amelioration of salinity stress: a systems biology perspective. Front. Plant Sci. 8:1768. doi: 10.3389/fpls.2017.01768
Iqbal, M. A., Khalid, M., Zahir, Z. A., and Ahmad, R. (2016). Auxin producing plant growth promoting rhizobacteria improve growth, physiology and yield of maize under saline field conditions. Int. J. Agric. Biol. 18, 37–45. doi: 10.17957/IJAB/15.0059
Isla, R., and Aragüés, R. (2010). Yield and plant ion concentrations in maize (Zea mays L.) subject to diurnal and nocturnal saline sprinkler irrigations. Field Crops Res. 116, 175–183. doi: 10.1016/j.fcr.2009.12.008
Jamil, A., Riaz, S., Ashraf, M., and Foolad, M. R. (2011). Gene expression profiling of plants under salt stress. CRC Crit. Rev. Plant Sci. 30, 435–458. doi: 10.1080/07352689.2011.605739
Jha, Y., and Subramanian, R. B. (2013). Paddy plants inoculated with PGPR show better growth physiology and nutrient content under saline condition. Chilean J. Agri. Res. 73, 213–219. doi: 10.4067/S0718-58392013000300002
Jing, X., Sanders, N. J., Shi, Y., Chu, H., Classen, A. T., Zhao, K., et al. (2015). The links between ecosystem multifunctionality and above-and belowground biodiversity are mediated by climate. Nat. Commun. 6, 1–8. doi: 10.1038/ncomms9159
Johnson, H. E., Broadhurst, D., Goodacre, R., and Smith, A. R. (2003). Metabolic fingerprinting of salt-stressed tomatoes. Phytochemistry 62, 919–928. doi: 10.1016/S0031-9422(02)00722-7
Kalhoro, N. A., Rajpar, I., Kalhoro, S. A., Ali, A., Raza, S., Ahmed, M., et al. (2016). Effect of salts stress on the growth and yield of wheat (Triticum aestivum L.). Am. J. Plant Sci. 7:2257. doi: 10.4236/ajps.2016.715199
Kanagaraj, G., and Desingh, R. (2017). Salinity influences physiological traits of seven Sesame (Sesamum indicum L.) varieties. J. Sci. Agri. 1, 188–196. doi: 10.25081/jsa.2017.v1.59
Kannan, R., Damodaran, T., and Umamaheswari, S. (2015). Sodicity tolerant polyembryonic mango root stock plants: a putative role of endophytic bacteria. Afr. J. Biotechnol. 14, 350–359. doi: 10.5897/AJB2014.14259
Karlidag, H., Yildirim, E., Turan, M., Pehluvan, M., and Donmez, F. (2013). Plant growth-promoting rhizobacteria mitigate deleterious effects of salt stress on strawberry plants (Fragaria× ananassa). Hortscience 48, 563–567. doi: 10.21273/HORTSCI.48.5.563
Karmakar, R., Das, I., Dutta, D., and Rakshit, A. (2016). Potential effects of climate change on soil properties: a review. Sci. Int. 4, 51–73. doi: 10.17311/sciintl.2016.51.73
Karuppiah, V., Vallikkannu, M., Li, T., and Chen, J. (2019). Simultaneous and sequential based co-fermentations of Trichoderma asperellum GDFS1009 and Bacillus amyloliquefaciens 1841: a strategy to enhance the gene expression and metabolites to improve the bio-control and plant growth promoting activity. Microb. Cell Fact. 18:185. doi: 10.1186/s12934-019-1233-7
Kasim, W. A., Osman, M. E., Omar, M. N., El-Daim, I. A. A., Bejai, S., and Meijer, J. (2013). Control of drought stress in wheat using plant-growth-promoting bacteria. J. Plant Growth Regul. 32, 122–130. doi: 10.1007/s00344-012-9283-7
Keutgen, A. J., and Pawelzi, K. E. (2009). Impacts of NaCl stress on plant growth and mineral nutrient assimilation in two cultivars of strawberry. Environ. Exp. Bot. 65, 170–176. doi: 10.1016/j.envexpbot.2008.08.002
Khan, M. A., Hussain, N., Abid, M., and Imran, T. (2004). Screening of wheat (Triticum aestivum L.) cultivars for saline conditions under irrigated arid environment. J. Res. 11, 471–477.
Khan, N., and Bano, A. (2016). Role of plant growth promoting rhizobacteria and Ag-nano particle in the bioremediation of heavy metals and maize growth under municipal wastewater irrigation. Int. J. Phytoremed. 18:211–21. doi: 10.1080/15226514.2015.1064352
Khan, N., Bano, A., Ali, S., and Babar, M. A. (2020a). Crosstalk amongst phytohormones from planta and PGPR under biotic and abiotic stresses. Plant Growth Regul. 16, 1–5. doi: 10.1007/s10725-020-00571-x
Khan, N., Bano, A., and Curá, J. A. (2020b). Role of Beneficial microorganisms and salicylic acid in improving rainfed agriculture and future food safety. Microorganisms 8:1018. doi: 10.3390/microorganisms8071018
Khan, N., Bano, A., Rahman, M. A., Rathinasabapathi, B., and Babar, M. A. (2019). UPLC-HRMS-based untargeted metabolic profiling reveals changes in chickpea (Cicer arietinum) metabolome following long-term drought stress. Plant Cell Environ. 42, 115–132. doi: 10.1111/pce.13195
Khan, N., Bano, A., and Zandi, P. (2018a). Effects of exogenously applied plant growth regulators in combination with PGPR on the physiology and root growth of chickpea (Cicer arietinum) and their role in drought tolerance. J. Plant Interact. 13, 239–247. doi: 10.1080/17429145.2018.1471527
Khan, N., Zandi, P., Ali, S., Mehmood, A., Adnan Shahid, M., and Yang, J. (2018b). Impact of salicylic acid and PGPR on the drought tolerance and phytoremediation potential of Helianthus annus. Front. Microbiol. 9:2507. doi: 10.3389/fmicb.2018.02507
Kim, S. Y., Lim, J. H., Park, M. R., Kim, Y. J., Park, T. I., Seo, Y. W., et al. (2005). Enhanced antioxidant enzymes are associated with reduced hydrogen peroxide in barley roots under saline stress. BMB Rep. 38, 218–224. doi: 10.5483/BMBRep.2005.38.2.218
Kousar, B., Bano, A., and Khan, N. (2020). PGPR Modulation of secondary metabolites in tomato infested with Spodoptera litura. Agronomy 10:778. doi: 10.3390/agronomy10060778
Kumar, P., Tewari, R. K., and Sharma, P. N. (2010). Sodium nitroprusside-mediated alleviation of iron deficiency and modulation of antioxidant responses in maize plants. AoB Plants 2010:plq002. doi: 10.1093/aobpla/plq002
Kumar, V., Kumar, A., Pandey, K. D., and Roy, B. K. (2015). Isolation and characterization of bacterial endophytes from the roots of Cassia tora L. Ann. Microbiol. 65, 1391–1399. doi: 10.1007/s13213-014-0977-x
Lakhdar, A., Rabhi, M., Ghnaya, T., Montemurro, F., Jedidi, N., and Abdelly, C. (2009). Effectiveness of compost use in salt-affected soil. J. Hazard. Mater. 171, 29–37. doi: 10.1016/j.jhazmat.2009.05.132
Lan, P., Li, W., Wen, T. N., Shiau, J. Y., Wu, Y. C., Lin, W., et al. (2011). iTRAQ protein profile analysis of Arabidopsis roots reveals new aspects critical for iron homeostasis. Plant Physiol. 155, 821–834. doi: 10.1104/pp.110.169508
Li, M., Guo, R., Yu, F., Chen, X., Zhao, H., Li, H., et al. (2018). Indole-3-acetic acid biosynthesis pathways in the plant-beneficial bacterium Arthrobacter pascens ZZ21. Int. J. Mol. Sci. 19:443. doi: 10.18483/ijSci.1537
Lin, Y., Feng, Z., Wu, W., Yang, Y., Zhou, Y., and Xu, C. (2017). Potential impacts of climate change and adaptation on maize in northeast China. Agron. J. 109, 1476–1490. doi: 10.2134/agronj2016.05.0275
Malla, G. (2008). Climate change and its impact on nepalese agriculture. J. Agricul. Environ. 9, 62–65. doi: 10.3126/aej.v9i0.2119
Martinez-Beltran, J. (2005). “Overview of salinity problems in the world and FAO strategies to address the problem,” in Managing Saline Soils and Water: Science, Technology and Social Issues. Proceedings of the International Salinity Forum (Riverside, CA).
Masood, A., Iqbal, N., and Khan, N. A. (2012). Role of ethylene in alleviation of cadmium-induced photosynthetic capacity inhibition by sulphur in mustard. Plant Cell Environ. 35, 524–533. doi: 10.1111/j.1365-3040.2011.02432.x
Miller, G. A. D., Suzuki, N., Ciftci-Yilmaz, S., and Mittler, R. O. N. (2010). Reactive oxygen species homeostasis and signalling during drought and salinity stresses. Plant Cell Environ. 33, 453–467. doi: 10.1111/j.1365-3040.2009.02041.x
Miransari, M., and Smith, D. (2009). Rhizobial lipo-chitooligosaccharides and gibberellins enhance barley (Hordeum vulgare L.) seed germination. Biotechnology 8, 270–275. doi: 10.3923/biotech.2009.270.275
Mishra, A., and Sharma, S. D. (2003). Leguminous trees for the restoration of degraded sodic wasteland in eastern Uttar Pradesh, India. Land Degrad. Dev. 14, 245–261. doi: 10.1002/ldr.544
Mishra, J., Fatima, T., and Arora, N. K. (2018). “Role of secondary metabolites from plant growth-promoting rhizobacteria in combating salinity stress,” in Plant Microbiome: Stress Response, eds D. Egamberdieva, and A. Parvaiz (Singapore: Springer), 127–163. doi: 10.1007/978-981-10-5514-0_6
Mishra, S., Jha, A. B., and Dubey, R. S. (2011). Arsenite treatment induces oxidative stress, upregulates antioxidant system, and causes phytochelatin synthesis in rice seedlings. Protoplasma 248, 565–577. doi: 10.1007/s00709-010-0210-0
Munns, R. (2002). Comparative physiology of salt and water stress. Plant Cell Environ. 25, 239–250. doi: 10.1046/j.0016-8025.2001.00808.x
Munns, R., and Tester, M. (2008). Mechanisms of salinity tolerance. Annu. Rev. Plant Biol. 59, 651–681. doi: 10.1146/annurev.arplant.59.032607.092911
Myhre, G., Aas, W., Cherian, R., Collins, W., Faluvegi, G., Flanner, M., et al. (2017). Multi-model simulations of aerosol and ozone radiative forcing due to anthropogenic emission changes during the period 1990-2015. Atmosp. Chem. Phys. 17, 2709–2720. doi: 10.5194/acp-17-2709-2017
Nautiyal, C. S., Srivastava, S., Chauhan, P. S., Seem, K., Mishra, A., and Sopory, S. K. (2013). Plant growth-promoting bacteria Bacillus amyloliquefaciens NBRISN13 modulates gene expression profile of leaf and rhizosphere community in rice during salt stress. Plant Physiol. Biochem. 66, 1–9. doi: 10.1016/j.plaphy.2013.01.020
Nazar, R., Khan, M. I. R., Iqbal, N., Masood, A., and Khan, N. A. (2014). Involvement of ethylene in reversal of salt-inhibited photosynthesis by sulfur in mustard. Physiol. Plantarum 152, 331–344. doi: 10.1111/ppl.12173
Neal, A. L., Ahmad, S., Gordon-Weeks, R., and Ton, J. (2012). Benzoxazinoids in root exudates of maize attract Pseudomonas puçtida to the rhizosphere. PLoS ONE 7:e35498. doi: 10.1371/journal.pone.0035498
Nelson, G. C., Bennett, E., Berhe, A. A., Cassman, K., DeFries, R., Dietz, T., et al. (2006). Anthropogenic drivers of ecosystem change: an overview. Ecol. Soc. 11:29. doi: 10.5751/ES-01826-110229
Nosetto, M. D., Jobbágy, E. G., Tóth, T., and Di Bella, C. M. (2007). The effects of tree establishment on water and salt dynamics in naturally salt-affected grasslands. Oecologia 152, 695–705. doi: 10.1007/s00442-007-0694-2
Nosheen, A., and Bano, A. (2014). Growth enrichment of Carthamusç tinctorius (L) and reduction in dosage of chemical fertilizers with application of plant growth promoting rhizobacteria. Int. J. Agron. Agric. Res. 4, 75–84.
Olanrewaju, O. S., Glic, K. B. R., and Babalola, O. O. (2017). Mechanisms of action of plant growth promoting bacteria. World J. Microbiol. Biotechnol. 33:197. doi: 10.1007/s11274-017-2364-9
Omoto, E., Taniguchi, M., and Mayake, H. (2012). Adaptation responses in C4 photosynthesis of maize under salinity. J. Plant Physiol. 169, 469–477. doi: 10.1016/j.jplph.2011.11.009
Onwuka, B. M., and Mang, B. (2018). Effects of soil temperature on some soil properties and plant growth. Adv. Plants Agric. Res. 8, 34–37. doi: 10.15406/apar.2018.08.00288
Otieno, N., Lally, R. D., Kiwanuka, S., Lloyd, A., Ryan, D., Germaine, K. J., et al. (2015). Plant growth promotion induced by phosphate solubilizing endophytic Pseudomonas isolates. Front. Microbiol. 6:745. doi: 10.3389/fmicb.2015.00745
Pandey, R., Swamy, K. V., and Khetmalas, M. B. (2013). Indole: A novel signaling molecule and its applications. Indian J. Biotechnol. 12, 297–310.
Pandya, N. D., and Desai, P. V. (2014). Screening and characterization of GA3 producing Pseudomonas monteilii and its impact on plant growth promotion. Int. J. Curr. Microbiol. Appl. Sci. 3, 110–115.
Pareek, N. (2017). Climate change impact on soils: adaptation and mitigation. MOJ Eco. Environ. Sci. 2:00026. doi: 10.15406/mojes.2017.02.00026
Parret, A. H., Schoofs, G., Proost, P., and De Mot, R. (2003). Plant lectin-like bacteriocin from a rhizosphere-colonizing Pseudomonas isolate. J. Bacteriol. 185, 897–908. doi: 10.1128/JB.185.3.897-908.2003
Paul, D. I. B. Y., Bharathkumar, S., and Sudh, N. (2005). Osmotolerance in biocontrol strain of Pseudomonas pseudoalcaligenes MSP-538: a study using osmolyte, protein and gene expression profiling. Ann. Microbiol. 55, 243–247.
Peng, Y. L., Gao, Z. W., Gao, Y., Liu, G. F., Sheng, L. X., and Wang, D. L. (2008). Eco-physiological characteristics of Alfalfa seedlings in response to various mixed salt-alkaline stresses. J. Integr. Plant Biol. 50, 29–39. doi: 10.1111/j.1744-7909.2007.00607.x
Petrov, V., Hille, J., Mueller-Roeber, B., and Gechev, T. S. (2015). ROS-mediated abiotic stress-induced programmed cell death in plants. Front. Plant Sci. 6:69. doi: 10.3389/fpls.2015.00069
Qadir, M., Noble, A. D., Oster, J. D., Schubert, S., and Ghafoor, A. (2005). Driving forces for sodium removal during phytoremediation of calcareous sodic and saline–sodic soils: a review. Soil Use Manage. 21, 173–180. doi: 10.1079/SUM2005312
Qadir, M., Oster, J. D., Schubert, S., Noble, A. D., and Sahrawat, K. L. (2007). Phytoremediation of sodic and saline-sodic soils. Adv. Agronomy 96, 197–247. doi: 10.1016/S0065-2113(07)96006-X
Qadir, M., Quillérou, E., Nangia, V., Murtaza, G., Singh, M., Thomas, R. J., et al. (2014). Economics of salt-induced land degradation and restoration. Nat. Res. Forum 38, 282–295. doi: 10.1111/1477-8947.12054
Raaijmakers, J. M., De Bruijn, I., and de Kock, M. J. (2006). Cyclic lipopeptide production by plant-associated Pseudomonas spp.: diversity, activity, biosynthesis, and regulation. Mol. Plant-Microbe Interact. 19, 699–710. doi: 10.1094/MPMI-19-0699
Rabhi, M., Talbi, O., Atia, A., Abdelly, C., and Smaoui, A. (2008). “Selection of a halophyte that could be used in the bioreclamation of salt-affected soils in arid and semi-arid regions,” in Biosaline Agriculture and High Salinity Tolerance, eds C. Abdelly, M. Öztürk, M. Ashraf, and C. Grignon (Basel: BirKhäuser), 241–246. doi: 10.1007/978-3-7643-8554-5_22
Rahman, A. K. M. M., Ahmed, K. M., Butler, A. P., and Hoque, M. A. (2018). Influence of surface geology and micro-scale land use on the shallow subsurface salinity in deltaic coastal areas: a case from southwest Bangladesh. Environ. Earth Sci. 77:423. doi: 10.1007/s12665-018-7594-0
Reetha, S., Bhuvaneswari, G., Thamizhiniyan, P., and Mycin, T. R. (2014). Isolation of indole acetic acid (IAA) producing rhizobacteria of Pseudomonas fluorescens and Bacillus subtilis and enhance growth of onion (Allim cepa L.). Int. J. Curr. Microbiol. Appl. Sci. 3, 568–574.
Roberts, M. F. (2005). Organic compatible solutes of halotolerant and halophilic microorganisms. Saline Syst. 1:5. doi: 10.1186/1746-1448-1-5
Rodríguez, M., Torres, M., Blanco, L., Béjar, V., Sampedro, I., and Llamas, I. (2020). Plant growth-promoting activity and quorum quenching-mediated biocontrol of bacterial phytopathogens by Pseudomonas segetis strain P6. Sci. Rep. 10, 1–2. doi: 10.1038/s41598-020-61084-1
Roman-Ponce, B., Reza-vázquez, D. M., Gutierrez-Paredes, S., María de Jesús, D. E., Maldonado-Hernandez, J., Bahena-Osorio, Y., et al. (2017). Plant growth-promoting traits in rhizobacteria of heavy metal-resistant plants and their effects on Brassica nigra seed germination. Pedosphere 27, 511–526. doi: 10.1016/S1002-0160(17)60347-3
Rosa, E. A., Rudel, T. K., York, R., Jorgenson, A. K., and Dietz, T. (2015). The human (anthropogenic) driving forces of global climate change. Clim. Change Soc. Sociol. Perspect. 2, 32–60. doi: 10.1093/acprof:oso/9780199356102.003.0002
Rossen, L., Shearman, C. A., Johnston, A. W., and Downy, J. A. (1985). The nodD gene of Rhizobium leguminosarum is autoregulatory and in the presence of plant exudate induces the nodA, B, C genes. EMBO J. 4, 3369–3373. doi: 10.1002/j.1460-2075.1985.tb04092.x
Sadale, A. N., and Karadge, B. A. (2013). Effect of salinity and water stress on nitrogen metabolism in Sesbania grandiflora (L.) Poir. Bioinfolet-A Q. J. Life Sci. 10, 814–818.
Sadeghi, A., Karimi, E., Dahaji, P. A., Javid, M. G., Dalvand, Y., and Askari, H. (2012). Plant growth promoting activity of an auxin and siderophore producing isolate of Streptomyces under saline soil conditions. World J. Microbiol. Biotechnol. 28, 1503–1509. doi: 10.1007/s11274-011-0952-7
Sandhya, V. S. K. Z., Ali, S. Z., Grover, M., Reddy, G., and Venkateswarlu, B. (2010). Effect of plant growth promoting Pseudomonas spp. on compatible solutes, antioxidant status and plant growth of maize under drought stress. Plant Growth Regul. 62, 21–30. doi: 10.1007/s10725-010-9479-4
Sarkar, A., Ghosh, P. K., Pramanik, K., Mitra, S., Soren, T., Pandey, S., et al. (2018). A halotolerant Enterobacter sp. displaying ACC deaminase activity promotes rice seedling growth under salt stress. Res. Microbiol. 169, 20–32. doi: 10.1016/j.resmic.2017.08.005
Schroeter, R., Hoffmann, T., Voigt, B., Meyer, H., Bleisteiner, M., Muntel, J., et al. (2013). Stress responses of the industrial work horse Bacillus licheniformis to osmotic challenges. PLoS ONE 8:80956. doi: 10.1371/journal.pone.0080956
Setia, R., Gottschalk, P., Smith, P., Marschner, P., Baldock, J., Setia, D., et al. (2013). Soil salinity decreases global soil organic carbon stocks. Sci. Total Environ. 465, 267–272. doi: 10.1016/j.scitotenv.2012.08.028
Shafi, M., Bakhat, J., Khan, M. J., Khan, M. A., and Anwar, S. (2010). Effect of salinity on yield and ion accumulation of wheat genotypes. Pakistan J. Botany 42, 4113–4121.
Shahid, M. A., Sarkhosh, A., Khan, N., Bilal, R. M., Ali, S., Rossi, L., et al. (2020). Insights into the physiological and biochemical impacts of salt stress on plant growth and development. Agronomy 10:938. doi: 10.3390/agronomy10070938
Shahzad, R., Khan, A. L., Bilal, S., Waqas, M., Kang, S. M., and Lee, I. J. (2017). Inoculation of abscisic acid-producing endophytic bacteria enhances salinity stress tolerance in Oryza sativa. Environ. Exp. Bot. 136, 68–77. doi: 10.1016/j.envexpbot.2017.01.010
Sharma, P., Jha, A. B., Dubey, R. S., and Pessarakli, M. (2012). Reactive oxygen species, oxidative damage, and antioxidative defense mechanism in plants under stressful conditions. J. Botany 2012:217037. doi: 10.1155/2012/217037
Shrestha, S. (2019). Effects of climate change in agricultural insect pest. Acta Sci. Agric. 3, 74–80. doi: 10.31080/ASAG.2019.03.0727
Siddikee, M. A., Chauhan, P. S., Anandham, R., Han, G. H., and Sa, T. (2010). Isolation, characterization, and use for plant growth promotion under salt stress, of ACC deaminase-producing halotolerant bacteria derived from coastal soil. J. Microbiol. Biotechnol. 20, 1577–1584. doi: 10.4014/jmb.1007.07011
Singh, R. P., and Jha, P. N. (2017). The PGPR Stenotrophomonas maltophilia SBP-9 augments resistance against biotic and abiotic stress in wheat plants. Front. Microbiol. 8:1945. doi: 10.3389/fmicb.2017.01945
Sleator, R. D., and Hill, C. (2002). Bacterial osmoadaptation: the role of osmolytes in bacterial stress and virulence. FEMS Microbiol. Rev. 26, 49–71. doi: 10.1111/j.1574-6976.2002.tb00598.x
Smartt, A. D., Brye, K. R., and Norman, R. J. (2016). Methane emissions from rice production in the United States—a review of controlling factors and summary of research. Greenhouse Gases 30, 179–207. doi: 10.5772/62025
Smith, S. M. (2014). What are Strigolactones and why are they important to plants and soil microbes? BMC Biol. 12, 1–7. doi: 10.1186/1741-7007-12-19
Song, C., Schmidt, R., de Jager, V., Krzyzanowska, D., Jongedijk, E., Cankar, K., et al. (2015). Exploring the genomic traits of fungus-feeding bacterial genus collimonas. BMC Genom. 16:1103. doi: 10.1186/s12864-015-2289-3
Sopheareth, M., Chan, S., Naing, K. W., Lee, Y. S., Hyun, H. N., Kim, Y. C., et al. (2013). Biocontrol of late blight (Phytophthora capsici) disease and growth promotion of pepper by Burkholderia cepacia MPC-7. Plant Pathol. J. 29:67. doi: 10.5423/PPJ.OA.07.2012.0114
Spaepen, S., Dobbelaere, S., Croonenborghs, A., and Vanderleyden, J. (2008). Effects of Azospirillum brasilense indole-3-acetic acid production on inoculated wheat plants. Plant Soil 312, 15–23. doi: 10.1007/s11104-008-9560-1
Srivastava, S., and Dubey, R. S. (2011). Manganese-excess induces oxidative stress, lowers the pool of antioxidants and elevates activities of Key antioxidative enzymes in rice seedlings. Plant Growth Regul. 64, 1–16. doi: 10.1007/s10725-010-9526-1
Streets, A. J., Moon, D. J., Kane, M. E., Obara, T., and Ong, A. C. (2006). Identification of an N-terminal glycogen synthase Kinase 3 phosphorylation site which regulates the functional localization of polycystin-2 in vivo and in vitro. Hum. Mol. Genet. 15, 1465–1473. doi: 10.1093/hmg/ddl070
Subramanian, S. (2013). Mass Spectrometry Based Proteome Profiling to Understand the Effects of Lipo-Chito-Oligosaccharide and Thuricin 17 in Arabidopsis thaliana and Glycine Max Under Salt Stress. Montreal, QC: McGill University.
Sumer, A. (2004). Evidence of sodium toxicity for the vegetative growth of maize during the first phase of salt stress. J. App. Bot. 78, 135–139.
Tanou, G., Molassiotis, A., and Diamantidis, G. (2009). Induction of reactive oxygen species and necrotic death-like destruction in strawberry leaves by salinity. Environ. Exp. Bot. 65, 270–281. doi: 10.1016/j.envexpbot.2008.09.005
Tavakkoli, E., Fatehi, F., Coventry, S., Rengasamy, P., and McDonald, G. K. (2011). Additive effects of Na+ and Cl− ions on barley growth under salinity stress. J. Exp. Bot. 62, 2189–2203. doi: 10.1093/jxb/erq422
Tejada, M., Garcia, C., Gonzalez, J. L., and Hernandez, M. T. (2006). Use of organic amendment as a strategy for saline soil remediation: influence on the physical, chemical and biological properties of soil. Soil Biol. Biochem. 38, 1413–1421. doi: 10.1016/j.soilbio.2005.10.017
Teller, A. S. (2016). Moving the conversation on climate change and inequality to the local: socio-ecological vulnerability in agricultural Tanzania. Sociol. Dev. 2, 25–50. doi: 10.1525/sod.2016.2.1.25
Tsuzauki, M., Moskvin, O. V., Kuribayashi, M., Sato, K., Retamal, S., Abo, M., et al. (2011). Salt stress-induced changes in the transcriptome, compatible solutes, and membrane lipids in the facultatively phototrophic bacterium Rhodobacter sphaeroides. Appl. Environ. Microbiol. 77, 7551–7559. doi: 10.1128/AEM.05463-11
Tyc, O., Zweers, H., de Boer, W., and Garbeva, P. (2015). Volatiles in inter-specific bacterial interactions. Front. Microbiol. 6:1412. doi: 10.3389/fmicb.2015.01412
Ullah, A., and Bano, A. (2019). Role of PGPR in the reclamation and revegetation of saline land. Pak. J. Bot. 51, 27–35. doi: 10.30848/PJB2019-1(43)
Ullah, H., Santiago-Arenas, R., Ferdous, Z., Attia, A., and Datta, A. (2019). Improving water use efficiency, nitrogen use efficiency, and radiation use efficiency in field crops under drought stress: A review. Adv. Agronomy 156, 109–157. doi: 10.1016/bs.agron.2019.02.002
Upadhyay, S. K., and Singh, D. P. (2015). Effect of salt-tolerant plant growth-promoting rhizobacteria on wheat plants and soil health in a saline environment. Plant Biol. 17, 88–293. doi: 10.1111/plb.12173
Upadhyay, S. K., Singh, J. S., Saxena, A. K., and Singh, D. P. (2012). Impact of PGPR inoculation on growth and antioxidant status of wheat under saline conditions. Plant Biol. 14, 605–611. doi: 10.1111/j.1438-8677.2011.00533.x
Vance, G. F., King, L. A., and Ganjegunte, G. K. (2008). Soil and plant responses from land application of saline–sodic waters: implications of management. J. Environ. Qual. 37:139. doi: 10.2134/jeq2007.0442
Voges, M. J., Bai, Y., Schulze-Lefert, P., and Sattely, E. S. (2019). Plant-derived coumarins shape the composition of an Arabidopsis synthetic root microbiome. Proc. Natl. Acad. Sci. U.S.A. 116, 12558–12565. doi: 10.1073/pnas.1820691116
Volker, U., Engelmann, S., Maul, B., Riethdorf, S., Völker, A., Schmid, R., et al. (1994). Analysis of the induction of general stress proteins of Bacillus subtilis. Microbiology 140, 741–752. doi: 10.1099/00221287-140-4-741
Voothuluru, P., and Sharp, R. E. (2012). Apoplastic hydrogen peroxide in the growth zone of the maize primary root under water stress. Increased levels are specific to the apical region of growth maintenance. J. Experi. Botany 64, 1223–1233. doi: 10.1093/jxb/ers277
Waadt, R., Hsu, P. K., and Schroeder, J. I. (2015). Abscisic acid and other plant hormones: methods to visualize distribution and signaling. Bio Essays 37, 1338–1349. doi: 10.1002/bies.201500115
Wakeel, A., Sümer, A., Hanstein, S., Yan, F., and Schubert, S. (2011). In vitro effect of different Na+/K+ ratios on plasma membrane H+-ATPase activity in maize and sugar beet shoot. Plant Physiol. Biochem. 49, 341–345. doi: 10.1016/j.plaphy.2011.01.006
Walker, D. J., and Bernal, M. P. (2008). The effects of olive mill waste compost and poultry manure on the availability and plant uptake of nutrients in a highly saline soil. Bioresour. Technol. 99, 396–403. doi: 10.1016/j.biortech.2006.12.006
Wang, C. J., Yang, W., Wang, C., Gu, C., Niu, D. D., Liu, H. X., et al. (2012). Induction of drought tolerance in cucumber plants by a consortium of three plant growth-promoting rhizobacterium strains. PLoS ONE 7:e52565. doi: 10.1371/journal.pone.0052565
Wang, N., Wang, E., Wang, J., Zhang, J., Zheng, B., Huang, Y., et al. (2018). Modelling maize phenology, biomass growth and yield under contrasting temperature conditions. Agric. Forest Meteorol. 250, 319–329. doi: 10.1016/j.agrformet.2018.01.005
Wani, S. H., Kumar, V., Shriram, V., and Sah, S. K. (2016). Phytohormones and their metabolic engineering for abiotic stress tolerance in crop plants. Crop J. 4, 162–176. doi: 10.1016/j.cj.2016.01.010
Weller, D. M., Landa, B. B., Mavrodi, O. V., Schroeder, K. L., et al. (2007). Role of 2, 4-diacetylphloroglucinol-producing fluorescent Pseudomonas spp. in the defense of plant roots. Plant Biol. 9, 4–20. doi: 10.1055/s-2006-924473
Yan, N., and Marschner, P. (2013). Microbial activity and biomass recover rapidly after leaching of saline soils. Biol. Fertil. Soils 49, 367–371. doi: 10.1007/s00374-012-0733-y
Yu, Y., Xu, T., Li, X., Tang, J., Ma, D., Li, Z., et al. (2016). NaCl-induced changes of ion homeostasis and nitrogen metabolism in two sweet potato (Ipomoea batatas L.) cultivars exhibit different salt tolerance at adventitious root stage. Environ. Exp. Bot. 129, 23–36. doi: 10.1016/j.envexpbot.2015.12.006
Zhang, N., Wang, D., Liu, Y., Li, S., Shen, Q., and Zhang, R. (2014). Effects of different plant root exudates and their organic acid components on chemotaxis, biofilm formation and colonization by beneficial rhizosphere-associated bacterial strains. Plant Soil 374, 689–700. doi: 10.1007/s11104-013-1915-6
Zhao, C., Liu, B., Piao, S., Wang, X., Lobell, D. B., Huang, Y., et al. (2017). Temperature increase reduces global yields of major crops in four independent estimates. Proc. Natl. Acad. Sci. 114, 9326–9331. doi: 10.1073/pnas.1701762114
Zhou, C., Ma, Z., Zhu, L., Xiao, X., Xie, Y., Zhu, J., et al. (2016). Rhizobacterial strain Bacillus megaterium BOFC15 induces cellular polyamine changes that improve plant growth and drought resistance. Int. J. Mol. Sci. 17:976. doi: 10.3390/ijms17060976
Zhou, D. Y., Liu, Y. X., Xu, Z. L., Yin, F. W., Song, L., Wan, X. L., et al. (2017). Effects of long-term intake of Antarctic Krill oils on artery blood pressure in spontaneously hypertensive rats. J. Sci. Food Agric. 97, 1143–1148. doi: 10.1002/jsfa.7840
Zhou, J. Y., Zhao, X. Y., and Dai, C. C. (2014). Antagonistic mechanisms of endophytic Pseudomonas fluorescens against Athelia rolfsii. J. Appl. Microbiol. 117, 1144–1158. doi: 10.1111/jam.12586
Zhu, J. K. (2001). Plant salt tolerance. Trends Plant Sci. 6, 66–71. doi: 10.1016/S1360-1385(00)01838-0
Keywords: climate change, salinity, halotolerant PGPR, biofertilizers, stress tolerance
Citation: Ullah A, Bano A and Khan N (2021) Climate Change and Salinity Effects on Crops and Chemical Communication Between Plants and Plant Growth-Promoting Microorganisms Under Stress. Front. Sustain. Food Syst. 5:618092. doi: 10.3389/fsufs.2021.618092
Received: 16 October 2020; Accepted: 22 April 2021;
Published: 10 June 2021.
Edited by:
Duraisamy Saravanakumar, The University of the West Indies St. Augustine, Trinidad and TobagoReviewed by:
Ifigeneia Mellidou, Institute of Plant Breeding and Genetic Resources, GreeceEverlon Cid Rigobelo, São Paulo State University, Brazil
Copyright © 2021 Ullah, Bano and Khan. This is an open-access article distributed under the terms of the Creative Commons Attribution License (CC BY). The use, distribution or reproduction in other forums is permitted, provided the original author(s) and the copyright owner(s) are credited and that the original publication in this journal is cited, in accordance with accepted academic practice. No use, distribution or reproduction is permitted which does not comply with these terms.
*Correspondence: Asghari Bano, YmFuby5hc2doYXJpJiN4MDAwNDA7Z21haWwuY29t; Naeem Khan, bmFlZW1raGFuJiN4MDAwNDA7dWZsLmVkdQ==