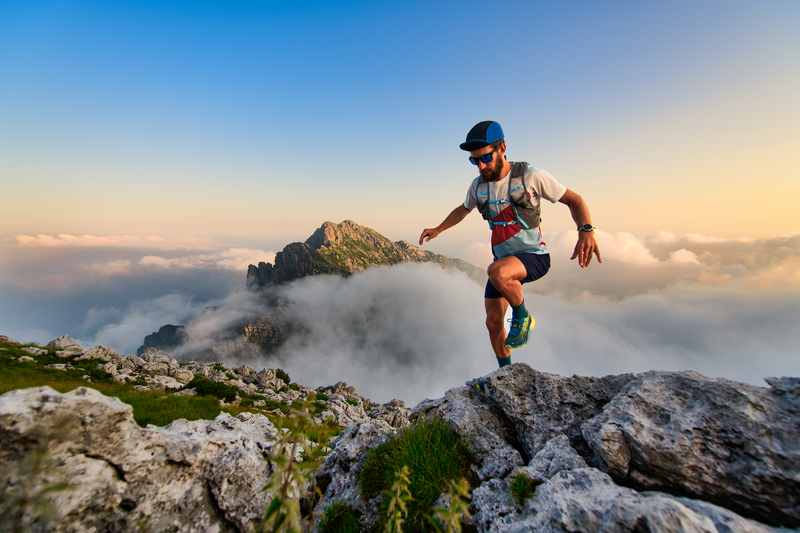
95% of researchers rate our articles as excellent or good
Learn more about the work of our research integrity team to safeguard the quality of each article we publish.
Find out more
REVIEW article
Front. Sustain. Food Syst. , 10 June 2021
Sec. Sustainable Food Processing
Volume 5 - 2021 | https://doi.org/10.3389/fsufs.2021.611835
This article is part of the Research Topic Re-valorization of Food Losses and Food Co-products View all 6 articles
The seafood industry generates large volumes of waste. These include processing discards consisting of shell, head, bones intestine, fin, skin, voluminous amounts of wastewater discharged as effluents, and low-value under-utilized fish, which are caught as by-catch of commercial fishing operations. The discards, effluents, and by-catch are rich in nutrients including proteins, amino acids, lipids containing good proportions of polyunsaturated fatty acids (PUFA), carotenoids, and minerals. The seafood waste is, therefore, responsible for loss of nutrients and serious environmental hazards. It is important that the waste is subjected to secondary processing and valorization to address the problems. Although chemical processes are available for waste treatment, most of these processes have inherent weaknesses. Biological treatments, however, are environmentally friendly, safe, and cost-effective. Biological treatments are based on bioconversion processes, which help with the recovery of valuable ingredients from by-catch, processing discards, and effluents, without losing their inherent bioactivities. Major bioconversion processes make use of microbial fermentations or actions of exogenously added enzymes on the waste components. Recent developments in algal biotechnology offer novel processes for biotransformation of nutrients as single cell proteins, which can be used as feedstock for the recovery of valuable ingredients and also biofuel. Bioconversion options in conjunction with a bio-refinery approach have potential for eco-friendly and economical management of seafood waste that can support sustainable seafood production.
Global food production is growing significantly to meet rising consumer demands. The seafood industry, a major segment of the food industry, provides finfish and shellfish of choice to consumers worldwide. Marine seafood includes finfish (pelagic, anchoveta, pollock, tuna, herring, mackerel, whiting, and others), and shellfish, which include crustaceans such as shrimp, krill, crab, lobster, and, mollusks, consisting of bivalves (mainly mussels, oysters, clams, and scallops), cephalopods (squid and cuttlefish), and gastropods (mainly abalone and snails). In 2018 global seafood production was 178.5 million tons (MT), consisting of 96.4 MT of capture fisheries (FAO, 2020). Of the 71.9 MT of finfish, the most popular marine species were anchoveta (7 MT), followed by Alaska pollock (3.4 MT), skipjack tuna (3.2 MT), herring (1.8 MT), and blue whiting (1.7 MT). Aquaculture production was 82.1 MT in 2018 consisting of finfish, mollusks, and crustacea at 7.3, 17.3, and 5.7 MT, respectively. Seafood amounting to 67.1 MT consisting of marine and farmed species including shrimp, prawns, salmon, mollusks, tilapia, catfish, sea bass, sea bream, and others constituted international trade. It is projected that utilization of fishery products for human consumption will reach 204 MT by the year 2030. It should be noted that percentage of fish stocks within biologically sustainable levels in 2017 was only 65.8% against 90% in the year 1990 (FAO, 2020). This suggests efforts by stakeholders for sustainability of the seafood resources are necessary.
The processing of food generates enormous amounts of waste, which include both solid discards as well as wastewaters containing portions of food being processed, which are released as process effluents. It has been estimated that, on average, about one-third of food produced globally, amounting to 1.3 billion tons, is wasted (Gustavsson et al., 2011). In the case of seafood, all the species harvested are not adequately used as food. Consumers prefer only a few select seafood items. A significant portion of the total harvest, therefore, remains unused or poorly used due to inherent problems related to unattractive color, smaller size, and high fat content. These result in a sizeable amount of catch being treated as by-catch, which is usually a combination of several species, particularly from tropical shrimp fisheries, and is unused or poorly used as food (Venugopal and Shahidi, 1998). Gustavsson et al. (2011) observed that food loss and waste for the whole fisheries sector amounted to 35% of global catches; 9–15% of these losses arise from by-catch.
The seafood industry processes about 80% of total harvest into chilled, frozen, smoked, dried, fermented, or marinated products. The centralized pre-processing operations, which include beheading, de-shelling, skinning, gutting, removal of fins and scales, fileting, washing, and others, leads to significant amounts of solid wastes and wastewater as effluents. The waste, on a wet weight basis, constitutes as high as 50% of whole shellfish, such as shrimp, krill, and crab. Shrimp waste contains about 70% head and 30% shell (Yan and Chen, 2015). Argentine red shrimp (ARS) is a highly popular shellfish, the industrial processing of which yearly yields 18,000 MT of shell waste, which is responsible for environmental pollution and ecological imbalances in Argentine Patagonia (Cretton et al., 2020). Lobster processing generates 50–70% of the shellfish as by-products such as heads, shells, livers, and eggs, which annually amounts to more than 50,000 MT (Nguyen et al., 2017). India produces up to 80,000 MT of shellfish waste (Chandrasekharan, 2015). Discards from finfish constitute 25–50% of the raw material, and is comprised of entrails, heads, skeletal frames, skin, scales, and viscera. Processing of freshwater fish such as trout, carp, pike, and bream generates 40–60% of the fish as waste (Venugopal, 2006). Love et al. (2015) reported that during the period 2009–2013 about 47% of the edible seafood supply was not available in the US for human consumption. This also included 16–32% of the harvest discarded as by-catch. In Europe, for each ton of seafood consumed an almost equal amount is estimated to be discarded as waste. The processing of shrimps and crabs in the EU alone results in more than 100,000 MT of shell waste each year (Sieber et al., 2018). Further, it is cautioned that large amounts of new fish biomass will be generated in European ports following the Landing Obligation Guidelines issued by the EU (Uhlmann et al., 2019).
The seafood industry, apart from solid discards, generates voluminous amounts of wastewater as process effluents as a result of operations such as washing, chilling, blanching, fileting, cooking, marination, and others. It has been estimated that ~10–40 m3 water is required for processing each ton of raw seafood (Arvanitoyannis and Kassaveti, 2008). One of the largest herring-processing factories in Europe, with an annual production of about 50,000 MT, releases ~1,500 m3 of wastewater daily (Steinke and Barjenbruch, 2010). Surimi production, which involves repeated washing of fish mince, uses more water than canning, curing, or freezing (Park, 2013). Water requirements for farmed production per metric ton of fish range from 1.5 to 6 m3 for generic fish (Hall et al., 2011).
Seafood items are known to be rich in nutritionally valuable proteins, essential fatty acids, particularly long chain n-3 polyunsaturated fatty acids (omega-3 PUFA), mainly eicosa pentaenoic acid (EPA, C20:5; n-3) and docosa hexaenoic acid, (DHA,C22:6; n-3), and vitamins and minerals (James, 2013; Venugopal and Gopakumar, 2017; Venugopal, 2018). Analysis of the compositions of more than 40 types of seafood processing discards showed that the discards have average contents of 60% proteins, 19% fat, and 22% ash, as shown in Table 1 (Islam et al., 2004). Shrimp head waste, on a dry weight basis, contains up to 65% proteins, 21% ash, and 18% chitin (Yan and Chen, 2015). Comparative dry-basis proximal analysis of shells of Argentine red shrimp (ARS) and southern king crab (SKC) showed both had 19–20% chitin. However, there were significant differences in contents of proteins and ash (18 and 48% for SKC and 26 and 55% for ARS, respectively). Analysis of shell and heads of ARS showed the highest lipid content (11%), with 5 mg and 158.8 μg of n-3 PUFAs and carotenoids per g, respectively. This suggested potential for recovering n-3-PUFAs and carotenoids from the ARS waste (Cretton et al., 2020). The raw heads, shells, and tails of Northern pink shrimp and spotted shrimp have crude proteins, which were rich in aspartic acid, glutamic acid, phenylalanine, lysine, and arginine. Their lipid contents ranged from 9.3 to 11.6%, while the contents of calcium, phosphorus, sodium, and magnesium were of 3,000, 400, 270, and 100 mg%, respectively. The contents of free amino acids (taurine, threonine, leucine, tryrosine, and phenylalanine) of the processing by-products and edible parts were 2.0 and 1.7 g%, respectively (Heu et al., 2003). Lobster processing waste is responsible for appreciable loss of nutrients. Lobster liver may contain up to 41% proteins, on a dry weight basis, while its head contains meat up to 20% of the shellfish weight (Nguyen et al., 2017).
The characteristic features of seafood processing effluents are contents of total suspended solids (TSS), fats, oils, and grease (FOG), and pigments and minerals. The TSS includes proteinous matter (myofibrillar proteins, collagen, gelatin, enzymes, soluble peptides, and amino acids) in soluble, colloidal, or particulate form (Islam et al., 2004). Ching and Ghufran (2017) reported 2.2% total solids (consisting up to 550 mg% TSS and 260 mg% dissolved solids), 50 mg% each of ammonia and nitrate nitrogen, and up to 100 mg% of phosphate typical effluent. Tuna processing effluents contained TSS, fat, chemical oxygen demand (COD), and biochemical oxygen demand (BOD) at 1,570, 450, 11,100, and 6,600 mg per lg, respectively (Achour et al., 2000). These losses deprive the consumers of significant amounts of nutrients. It has been estimated that annual seafood discards in the US represent a loss of about 208 billion g of proteins and 1.8 trillion mg of n-3 PUFA (Love et al., 2015). These indicate that processing effluents, in addition to solid discards and by-catch, contribute to losses of nutrients.
The food system has generally been considered a threat to the environment. The seafood industries consider huge volumes of by-catch, solid waste, and effluents a burden because of their potential to become environmental hazards. The industry dumps enormous amounts of by-catch in the ocean, while good amounts of solid wastes are disposed off as landfill or subjected to incineration. Ocean dumping causes reduced oxygen levels at the ocean bottom, burial or smothering of living organisms, and introduction of disease to the ecosystem of the sea floor (US EPA, 2017). Nguyen et al. (2017) observed that the disposal of lobster processing costs annually upward of about $7.5 million, and also presents an environmental burden to the lobster processors. Composting and ensilage of waste has been practiced, but they have limitations because of their longer process time, higher costs, and emission of volatile organic compounds. Anaerobic decomposition of seafood in landfill causes formation of methane (CH4), ammonia (NH3), and hydrogen sulfide (H2S), which are detrimental to the environment (Xu et al., 2018). Landfill contributes to climate change, about 10 times larger than other waste disposal options, while composting has the largest impact on carcinogens (Gao et al., 2018). The TSS and FOG of process effluents are responsible for high BOD and COD values, indicative of their adverse influence on the oxygen balance and, in turn, the flora (Gonzalez, 1995). Furthermore, shortage of drinking water, eutrophication (growth of unwanted biota), biotic depletion, algal blooms, habitat destruction, water acidification, disease outbreaks, and extensive siltation of corals are other environmental hazards (Hall et al., 2011). The environmental problems and loss of nutrients associated with seafood process effluents have been pointed out recently (Venugopal and Sasidharan, 2021).
Food sustainability demands optimal uses of resources for maximum benefits, including economic viability. The challenges of sustainable seafood processing are linked to reducing environmental pollution, conservation of water, and prevent losses of nutrients. Improving waste utilization is essential for a sustainable industry to prevent or minimize the environmental impact (López-Pedrouso et al., 2020). The problems of environmental hazards and nutrient losses facing the seafood industry can be addressed by measures such as selective trawling to reduce by-catches, appropriate treatments of wastes and effluents, and valorization of wastes by recovery of useful ingredients. The major advantages with respect to waste treatments are reduction of environmental hazards, conservation of water, and isolation of commercially valuable ingredients and improvement of the economy. In view of seafood-related environmental hazards, a need for a biological solution for the disposal of the seafood processing discards has been recognized (Pal and Suresh, 2016). Failure in these efforts not only leads to loss of potential revenues but also increases the cost of waste disposal and also public health problems (Etemadian et al., 2021). The recent United Nations Conference on Sustainable Development acknowledges the global importance of food losses and food waste. The Conference aims to halve per capita global food waste and reduce food losses along production and supply chains, including post-harvest losses, by 2030 (FAO, 2020).
Conventional processes for valorization of seafood discards and effluents are based on chemical and physical methods. These processes invariably have several limitations. For instance, the process of chitin extraction from crustacean shells involves initial alkali treatment generally with 5 M sodium hydroxide for deproteination followed by hydrochloric acid treatment for demineralization, which involves decomposition of calcium carbonate in the shells. Alkali treatment can result in the hydrolysis of chitin and also partial deacetylation of chitin. The process is also corrosive. Further, it requires large volumes of fresh water to wash off alkali and acid from the treated shells, releasing harmful wastewater (Mao et al., 2017; Yadav et al., 2019). Alkali extraction of proteins can lead to the loss of certain amino acids (Venugopal, 2006). Similarly, the traditional solvent extraction of fish oil can cause its oxidation, which is rich in unsaturated fatty acids. de Oliveira et al. (2016) extracted oil from tuna by employing chemical refining. The process consisted of degumming, neutralization, washing, drying, bleaching, and deodorization. Although chemical refining was successful, temperature and chemical reagents favored the removal of PUFA from the oil. In view of these drawbacks, interest in alternate green processes is growing. Biological processes can have minimal environmental impacts, be cost-effective and safe, and have minimum adverse impacts on the properties of the isolated components. These processes, therefore, offer an economic and versatile way to transform and concentrate waste and wastewater into valuable products.
Biotechnology holds promise for novel waste treatment and resource recovery processes, diversification of value-added products, and in quality assurance (Pleissner and Lin, 2013; Chandrasekharan, 2015). Such processes are supported by novel green techniques for industrial recovery of biomolecules from seafood by-products and discards. The extraction efficiency can vary highly depending on the food matrix, the target compounds, and methods of extractions. Therefore, the choice of green extraction technique depends essentially on the matrix and the target compound features (Bruno et al., 2019). Such approaches can also lead to a bio-based economy (Puyol et al., 2017). This article will examine potentials of biological processes depending on bioconversions of components in seafood discards, including effluents. Prospects of algal biotechnology and bio-refinery approaches will also be discussed.
Bioconversion reactions are biological methods, which are carried out to initially detach food components from their matrices. The detached components can be recovered and purified by suitable techniques. Two major bioconversion processes have been recognized, which employ either microorganisms or externally added enzymes. These help detach the components from the food matrices. Both microbial and enzymatic processes are environmentally friendly, cost-effective, and safe, unlike most conventional chemical extraction processes. Microbial fermentation of the waste results in the production of hydrolytic enzymes by the organism, which causes bioconversions of the food components. Exogenous enzymes, on the other hand, cause a direct release of the components from the food matrix. A number of microorganisms, particularly lactic acid bacteria (LAB), have been used for fermentation. The use of algae is a novel approach in this respect. The algae-induced bio-transformations help not only with the treatment of wastewater but also production of nutrient-rich biomass, which is useful for a variety of applications. In addition to microbial and enzymatic methods, biophysical processes such as modifications of pH and temperature can favor the release of components such as proteins and lipids from the food matrices. Figure 1 depicts a schematic representation of the bio-conversion processes for valorization of seafood discards. These aspects will be discussed in detail.
Figure 1. Schematic representation of bioconversion for valorization of seafood discards and the major classes of compounds that can be extracted from seafood processing discards.
The microbe-mediated bioconversion is termed as fermentation, which is safe, environmental-friendly and energy savvy. The process makes use of live microorganisms (bacteria, fungi, mycelium, or microalgae) to convert raw materials into products with desired qualities. Fermentation can be traditional, biomass or precision types. Traditional fermentation has been practiced for a few centuries. Since the 1980s biomass fermentation has emerged in the food industry for the production of cell mass for further use as sources of enzymes, flavors, food, biomaterials, therapeutics, fuels and in recent times, as sources of alternative proteins to develop cultivated meat formulations. Precision fermentation is intended to produce specific functional ingredients using tailor-made microbial hosts (GFI, 2020). Fermentation by lactic acid bacteria (LAB) has been used is a popular method for the development of fermented fishery products (Anihouvi et al., 2012). The efficiency of lactic acid fermentation depends on the type of organism, inoculums size, initial pH and pH attained during fermentation. The lactic acid formed during sugar breakdown creates low pH, which, in turn, suppresses growth of spoilage causing microorganisms and enhances activity of acid proteases, optimally acting on seafood proteins, many of which remain bound to chitin, lipids and carotenoids.
The bioremediation using microorganisms and their aggregates is recognized to be an efficient low-cost green process. The technology of microbial conversion also provides a potential way to isolate and exploit compounds of biotechnological potential (Wang et al., 2019). The microorganisms used for the purpose may be aerobic, anaerobic, or facultative including bacteria, fungi, and protozoa. Microbial fermentation processes can be under solid state, submerged or liquid state, anaerobic, batch, continuous, or fed batch conditions. The process is influenced by factors such as the nature of the starter culture, time, pH, and substrate composition. Fed-batch is a commonly used means for the production of microbial biomass, ethanol, organic acids, antibiotics, vitamins, enzymes, and other compounds in which the culture medium is added continuously or in pulses to reach the maximum volume. The advantages of fed-batch over the conventional batch operation include a higher biodegradation rate, higher productivity, higher dissolved oxygen in the medium, and decrease in fermentation time (Chandrasekharan, 2015; Puyol et al., 2017).
Microbial growth results in the production of hydrolytic enzymes such as proteases, lipases, and chitinases. Proteases and chitinases cause demineralization, deproteination, and proteolysis in the substrate. Chitinases catalyze the cleavage of the β-1,4-O-glycosidic linkages in chitin. Lipases function as triacylglycerol hydrolases and also catalyze synthesis of ester compounds. Fish fermentation, which is traditionally used to increase fish shelf-life, results in the formation of bacteria metabolites of interest. Lactic acid bacteria (LAB) have been used for a long time for the development of fermented fishery products (Anihouvi et al., 2012). Fermentation by LAB produces lactic acid; the low pH enhances acid proteases which act on proteins bound to chitin, lipids, and carotenoids. Fermentation can be applied for the production of cell mass, enzymes, flavors, food additives, and a range of other high value-added products. For example, fermentation of shrimp shell waste by symbiotic LAB such as S. thermophilus, L. acidophilus, and L. bulgaricus rapidly decreased pH to about 4.2 and promoted the removal of calcium and protein, with 91.3% calcium, 97.7% protein, and 32.3% carotenoid removed from shrimp waste after 168 h fermentation activated acid proteases gave bioactive peptides of size between 1,000 and 10,000 Da (Shan et al., 2011). LAB -induced fermentations bring about diversity into foods, make otherwise inedible foods products edible, enhance nutritional value, decrease toxicity, preserve food, and decrease cooking time and energy requirements. The technology is safe, environmentally friendly, and does not consume much energy.
Microbe-assisted bioconversions are ideal for the bioremediation of seafood processing waste and production of aquafeed and fertilizer. Applied to fish by-products, fermentation gives rise to quality protein hydrolysates and oil and produces antioxidant compounds (Marti-Quijal et al., 2020). Fish offal and a mixture of sawdust and wood shavings in equal proportions were subjected to composting by placing them in an open structure with passive aeration. Solid state fermentation converted the waste into a highly nutritive fertilizer with a nitrogen content as high as 12% (Wang et al., 2019). Dried skipjack tuna waste (red meat, gills, viscera, fins, etc.) was mixed with 25% wheat flour and fermented with L. plantarum and B. licheniformis for 14 days. The proximate analysis showed significant changes in the composition of L. plantarum. The fermented product can be used as a nutritive aquafeed ingredient (Hena et al., 2009). Conversion of fish waste to liquid fertilizer was achieved with mixed microorganisms, resulting in about 28% degradation of fish waste. The product was stable against putrefaction for 6 months at ambient temperature (Dao and Kim, 2011). The microbe-assisted aerobic bioprocess of aquaculture solid waste for 15 days at 35 °C and at pH 6.0–6.5 maximized nitrogen bioconversion in the form of ammonium ions () (Khiari et al., 2019). Fermentation is a viable alternative to chemical treatment for the extraction of collagen (Song et al., 2021).
Rashid et al. (2018) fermented shrimp-shell powder with B. cereus to produce sugar, antioxidant, and DNA protective compounds. The fed-batch biodegradation was operated in a 5-L bioreactor for 96 h according to three time pulse-feeding strategy. On the basis of the equal working volume of 3 l, the fed-batch biodegradation showed a better production of the target compounds than the batch biodegradation, with higher cell density and a shortened biodegradation period. The maximum values of the target compounds were about 0.3 mg per ml of reducing sugar and 92 to 98% antioxidant activities. Fed batch fermentation gave ~3–12% higher values compared with batch biodegradation.
Microorganisms can treat seafood industry process effluents in reaction systems such as activated sludge, aerobic lagoons, trickling filters, and rotating disc contactors. In the commonly used activated sludge system, the sludge consisting of an optimized, mixed flora of microorganisms degrades the organic materials in the presence of dissolved oxygen, thereby decreasing the BOD of the effluent (Gonzalez, 1995; Choudhury et al., 2010). An aerobic continuous bioreactor treated high saline fish processing wastewater for 8 h, which removed the offensive odor of the effluent (Ching and Ghufran, 2017). Anaerobic digestion (AD), a popular green technology for waste treatment, involves fermentation of the material in the absence of molecular oxygen with the formation of CO2, hydrogen, and/or acetic acid; reduction of the CO2 and acetate leads to the production of methane. AD of tuna processing effluents involved a decanter to remove the fats and the TSS, an anaerobic digester, and an activated sludge aerated bioreactor. The integrated system helped with the removal of up to 95% of the COD (Achour et al., 2000). AD of seafood industry effluents in a dissolved air flotation system (DAF) removed organic contents. The process flow consists of the separation of effluent in DAF and treatment of clarified water in a double nitrification-de-nitrification stage. AD of solids separated during the DAF process produces biogas and significantly reduces sludge volume (Fluence, 2019).
Microalgae such as Chlorella, Spirulina, Dunaliella, diatoms, and cyanobacteria, commonly referred to as blue green algae, are the main algae grown commercially as sources of functional materials in natural foods. These organisms are bestowed with a high growth rate in nutrient media under phototrophic (light and CO2) conditions. Their digestive actions allow the degradation of organic contents, unused food, and excretory products together with the removal of CO2, NH3-N, CO2, and H2S, thereby ameliorating environmental pollution (Puyol et al., 2017; Gifuni et al., 2019). The phototrophic algae can be cultivated in open ponds or in closed photo-bioreactors, or heterotrophically in closed systems. One of the major advantages associated with the open ponds is its low production and operating costs. However, the limitations include uneven light availability and distribution within the pond. Heterotrophic cultivation in closed systems eliminates the requirement of light, but heterotrophic culture is prone to contamination by other microbial species (Nigam et al., 2020).
Microalgae are promising agents for bioconversions of food, fishery, and agricultural waste into biomass rich in bioactive compounds (Das, 2015). The algal mass (referred as single cell proteins, SCP) can contain up to 60% proteins, good amounts of oil, and also polysaccharides, minerals, and pigments including chlorophylls, carotenoids, and phycobiliproteins. Stringent nitrogen limitations stimulate algae to produce more lipids, as high as 75% with high n-3 PUFA contents (Stengel and Connan, 2015). SCP has found wide applications as sources of bioactive peptides, plant growth stimulants, animal feeds, food additives, cosmeceuticals, drugs, and as probiotics in aquaculture. SCP can also replace expensive soy meal and fishmeal in animal and aquaculture feeds (Sharma and Sharma, 2017; Caporgno and Mathys, 2018; Smárason et al., 2019). Cultivation of microalgae in wastewater offered the highest atmospheric carbon fixation rate (1.83 kg CO2/kg biomass) and rapid biomass productivity-−40–50% higher than terrestrial crops (Shahid et al., 2020). Growth of microalgal biomass has been estimated to require 200–1,000 liter of water per kg of dry biomass. This suggests comparable volumes of seafood industry effluents could be treated for producing equivalent amounts of microalgae as SCP (de Farias and Barbera, 2018). Batch cultivation of Chlorella sp. in seafood processing water gave a biomass yield of 896 mg per lg (Gao et al., 2018). Bacillus sp., Brevibacterium sp., and Vibrio sp. associated with seaweed (Ulva sp.), having a consortium of hydrolytic enzymes including cellulase, protease, and chitinase, degraded crab shells, prawn shells, and fish scales within 4 days in the seawater-based broth. The reducing sugars released during degradation can be used for ethanol fermentation by Saccharomyces cerevisiae (Samant et al., 2019). Fermentation of fish media can result in 3 to 4 fold reduction in treatment costs (Vázquez et al., 2020).
Microalgae have the key advantage to produce third generation biofuel, because of its rapid growth and high lipid contents (Shuba and Kifle, 2018; Koyande et al., 2019). In comparison to petroleum diesel, biodiesel is characterized by lower emissions of carbon dioxide, sulfur dioxide, and harmful air pollutants. Oil from SCP is a plausible choice for biofuel. Therefore, cultivation of oleaginous microorganisms can be a promising approach for valorization low-cost organic waste for energy production (Cho and Park, 2018). Seafood discards, including effluents, can be a promising alternative feedstock for the sustainable production of biodiesel and biogas (Jayasinghe and Hawboldt, 2012). Direct transformation of lipidic biomass into biodiesel has also gained attention. Fadhil et al. (2017) produced liquid biofuels and activated carbons by trans-esterification of fish oil with methanol and ethanol using potassium hydroxide as a base catalyst. Fish oil that contains high levels of free fatty acids may require a modified esterification process. The process comprised rapid purification of the oil, followed by methanol esterification at 60°C for 1 h initially under acidic conditions followed by alkaline conditions. The preparation satisfied required standards, in terms of viscosity, flash point and other parameters (Kara et al., 2018). Anaerobic digestion of seafood processing wastewater by Chlorella sp. supports biogas production (Jehlee et al., 2017). These suggest algal technology has potential for valorization of seafood processing discards and effluents.
Processing with an enzyme holds enormous potential in waste management. Enzymes can mitigate hazards of conventional chemical transformations for resolution of food waste-related environmental problems, help production of novel compounds, and function as analytical tools for food quality assessment. Enzymes can be included as additional processing aids to conventional processes or can be exclusively used to upgrade existing technologies in seafood processing. The advantages of enzymes are their low energy requirements, safety, and low-cost (Venugopal, 2006; Chandrasekharan, 2015; Fernandes, 2016; Yang and Yan, 2018). Hydrolases, which include carbohydrases, proteases, and lipases, are popular enzymes in biotechnology,. Enzymatic hydrolysis of protein from aquatic by-products and livestock, poultry, and plants offer novel products with applications in foods, pet feed, pharmaceutical, and other industries (Etemadian et al., 2021). Specific, energy-efficient, and easily controllable enzymatic techniques using proteases, glycoside hydrolases, lipases, transglutaminases, and other enzymes are emerging as bio-processing techniques for seafood processing (Shah et al., 2016; Huang et al., 2017).
The by-catch and the various seafood processing discards are rich in balanced proteins, collagen, enzymes, lipid, carotenoids such as astaxanthine and β- carotene, polysaccharides including chitin, glycosaminoglicans, and various minerals. These can be recovered by coupling the various bioconversion processes, discussed above, supported by marine biotechnology-based downstream processes. These processes are mostly mild, energy-efficient, safe, and environmentally friendly. Green techniques include pressurized liquid, sub-critical, super-critical, enzyme-mediated, microwave-, and ultrasound-assisted extractions (Muffler and Ulber, 2005; Freitas et al., 2012; Chavez et al., 2013). Membrane bioreactors integrate reaction vessels with membrane separation units for producing materials such as peptides, chito-oligosaccharides, and PUFA from seafood discards (Kim and Senevirathne, 2011).
The past few years have seen notable interests in seafood-derived compounds for varied applications including food, pharmaceutical, agriculture, and other industries. For example, bioactive compounds from lobster processing by-products can be extracted using microwave, ultrasonic, and supercritical fluid extraction. The proteins, chitin, lipids, minerals, and pigments recovered from lobster processing by-products possess several functionalities and bioactivities, useful for their applications in water treatment, agriculture, food, nutraceutical, pharmaceutical products, and biomedicine (Nguyen et al., 2017). The diverse compounds that can be extracted from seafood processing discards can be grouped into four classes, depending upon their chemical nature. The major classes include nitrogenous, lipid, polysaccharide, and mineral-based compounds. Some of the individual compounds under each class are given in Table 2. In addition, a multitude of derivatives can also be developed from many of these components. These include bioactive peptides, gelatin, n-3 PUFA, glucosamine, chitosan, and its various derivatives. Detailed aspects of preparations, properties, and applications of components of seafood processing discards will not be discussed here; nevertheless, a very brief mention may be made. Fish proteins and protein hydrolyzates have the potential to be used as a protein supplement, in the fortification of foods, and as sources of bioactive peptides. Fish oil has numerous health benefits and can also be used to impart functional properties to food products. Chitosan and their oligosaccharides are applied as antioxidants, antibacterial and antifungal agents, and functional compounds in food, pharmaceutical, and other industries. Gelatin is used for the purposes of gelling, edible coating, emulsification, and microencapsulation. The reader is referred to a few recent articles for details (Karim and and Bhat, 2009; Yu and Gu, 2013; Venugopal and Lele, 2014; Vidanarachchi et al., 2014; Muxika et al., 2017; Sasidharan and Venugopal, 2019; Shahidi et al., 2019; Ashraf et al., 2020). Nawaz et al. (2020) recently observed a need to focus on bioavailability, interaction with other ingredients, nutritional, biotechnological, and sensorial aspects, and other factors of seafood-derived compounds that can significantly favor valorization of fisheries by-products. The following discussion will focus on bioconversion processes for extraction of major classes of compounds seafood discards and effluents.
Diverse proteins, peptides, amino acids, and their co-products constitute the nitrogenous fraction. Scarcity of nutritional proteins in many parts of the world necessitates novel and economical processes to recover them from unexplored sources (Henchion et al., 2017). Seafood processing discards, having a maximum of about 60% proteins, can be good sources of proteins, which can be recovered while retaining most of its native properties. Bioconversion processes for protein recovery from the discards have been developed. These make use of biophysical changes that induce their coagulation and precipitation of the macromolecules. These are discussed below.
Isoelectric solubilization precipitation (ISP) is a gentle bioprocess. This involves homogenization of underutilized fish or processing discards with either dilute acid (pH 2.5–3.5) or alkali (pH 10.8–11.5). The treatment dissolves sarcoplasmic and myofibrillar proteins, while insoluble impurities such as bone, skin, oil, and membranes are removed. Up to 90% of the dissolved proteins are precipitated by raising the pH of the solution to their iso-electric pH of 5.2–6.0. The proteins are concentrated by centrifugation or filtration. The process, as depicted in Figure 2, is ideally performed at 10°C or below, to avoid denaturation of the protein (Hultin et al., 2005). Recovery of proteins by the ISP process can be enhanced by coupling it with high intensity sonication, electro-flocculation, and ultrafiltration. Sasidharan and Venugopal (2019) summarized studies on ISP-based protein recovery from various species of finfish and shellfish, their discards, by-catch, and also process effluents. Some of the fishery sources included mackerel, catfish. rockfish, Pacific whiting, rainbow trout, Atlantic croaker, channel catfish, bullhead catfish, shrimp, crab, mussel, and squid. The fish protein isolates (FPIs) have protein contents of at least 65% and fat below 2%. FPIs differ from conventional surimi, which is a concentrate of fish myofibrillar proteins obtained by repeated washing of fish meat mince (Park, 2013). Unlike surimi, which is only myofibrillar proteins, particularly myosin and actomyosin, FPIs contain sarcoplasmic proteins along with the refined concentrate of myofibrillar proteins. The FPIs generally retain biochemical, nutritional, and functional properties of the native proteins, which make them valuable raw materials for applications such as the development of restructured food products, protein supplements, and bioactive peptides (Sasidharan and Venugopal, 2019).
Figure 2. Recovery of proteins from seafood discards by isoelectric solubilization precipitation (Adapted from Hultin et al., 2005).
Apart from myofibrillar proteins, collagen is another protein from marine sources. Marine collagen is a promising biocompatible alternative to mammalian collagen, particularly in biomedical and food applications. Collagen-based novel functional food ingredients contain a nutritional benefit, such as essential and non-essential amino acid, to improve the quality of different food products. It can also be used as a natural antioxidant and texturizing agent that can reduce the utilization of chemical food additives and may be able to fulfill the consumer demands for safe and green food products (Pal and Suresh, 2016).
Collagen and collagen hydrolysate (CH) were recovered from the bone and skin containing residues emerging during the ISP process from silver carp. Isolated collagen maintained their triple-helical structure and was characterized as type I collagen. Pepsin-hydrolysis and sequential hydrolysis by pepsin and trypsin degraded all heavy molecular weight chains of collagen; sequential enzyme treatment yielded a higher degree of hydrolysis. When CH was added to silver carp protein isolate prior to gelation, the gel properties were dependent on the molecular weight of the added CH. More hydrolyzed collagen emerging from sequential hydrolysis improved the water holding capacity of the gel while reducing its breaking force. The results suggest that residue from pH-shift processing of fish can be used for isolation of functionally active collagen and CH (Abdollahi et al., 2018). Tilapia type I collagen is biocompatible and can be used as an effective biodegradable scaffold biomaterial for regenerative medicine (Hayashi, 2020). Gelatin is extracted from collagen generally by pre-treatment with dilute NaOH, followed by swelling with dilute acetic acid and then by warm (45°C) water (Vázquez et al., 2019). Gelatin prepared from collagen of skins and bones of various marine and freshwater fishery sources have good gelling properties. Gelatin extract of big eye tuna skin had glycine, up to 32% of total amino acids, and hydroxyproline together with proline and alanine. Rheological studies revealed Newtonian and shear thickening properties of the gelatin. The tuna gelatin could be useful for the formulation of functional foods and nutraceutical and biomedical applications (Dara et al., 2020).
This process makes use of the ability of muscle structural proteins to undergo gelation under mild acidic conditions when water is strongly bound to the protein matrices. The process of extraction involves initial mechanical deboning of by-catch or fish discards such as heads and frames. The meat mince is washed twice with chilled (0–5°C) water, followed by homogenization of the washed mince in equal amounts of fresh chilled water. The pH of the homogenate is lowered to 3.5–4.0 by drop-wise addition of weak acid, such as acetic acid, which induces gelation of the proteins. The gelation process is associated with a fall in viscosity of the homogenate; the viscosity fall can be enhanced by mild heating to 50°C. Proteins in the low-viscous dispersion are highly stable as they cannot be precipitated by heating even at temperatures as high as 100°C. Such thermo-stable dispersions have been prepared from Atlantic herring, Atlantic mackerel, threadfin bream, and shark. Shark meat, however, is an exception. The homogenate of washed shark meat in water exhibited an increase in viscosity during the course of acidification to pH 4 to 5 (Venugopal, 2017). In the case of capelin, thermo-stable dispersion could be prepared without the need for acidification. The thermo-stable protein dispersions prepared from different fishery products can have varied applications, such as preparation of fish protein powder, as protein coating of fresh fish to extent their refrigerated shelf life, preparation of fermented sauce, or for the development of edible packaging (Venugopal, 1997). Meat recovered from by-catch fish can be used for protein dispersion or can be a resource for value added food products such as urimi, sausages, or fermented products, among others (Venugopal and Shahidi, 1998).
Proteins, which are present in suspended or dissolved states in process effluents, can be flocculated and precipitated by food grade polysaccharides such as carrageenan, alginate, and carboxy methylcellulose, which are then subjected to concentration by filtration, sedimentation, and/or centrifugation (Forghani et al., 2020). Proteins from herring industry processing effluents were recovered using electroflocculation (EF) and ultrafiltration (UF). EF and UF recovered up to 80% proteins. The highest protein and fatty acid contents of the effluent were 12.7 and 2.5 g per lg, respectively. Leucine and glutamic acid/glutamine were the dominating amino acids while calcium and magnesium were the dominating trace elements. The proteins had good foaming and emulsifying properties, which make them good functional additives (Gringer et al., 2015). Biomass from cooking wastewaters of snow crab was concentrated by membrane filtration. The concentrate had 59% proteins and contained desirable flavor compounds. The extract can be a natural aroma for the food industry (Tremblay et al., 2020).
Proteolytic enzymes from various sources, including microorganisms (such as alcalase, flavourzyme, and protamex), animal (colla genase, proteinase, serine-protease, neutrase, and trypsin), and plants (papain, bromelain, and ficin), can extract proteins from seafood processing discards as fish protein hydrolyzates (FPHs). The ideal treatment conditions are incubation temperature, 35–37°C; enzyme to substrate ratio, 1–50; and incubation up to 24 h. The degree of hydrolysis determines the properties of the hydrolyzate, such as solubility, water-holding capacity, emulsification, and foam-forming ability, and the contents and chemical nature of peptides formed. The FPH can be concentrated by spray drying or ultrafiltration. They generally show a beneficial effect on growth performances and feed utilization at low inclusion levels (Chalamaiah et al., 2012; Vijaykrishnaraj and Prabhasankar, 2015). Fish frames without heads from Atlantic salmon and Atlantic cod were treated with commercial proteases for 2 h. Salmon treated with alcalase and cod treated with pepsin yielded 64 and 68% proteins, respectively (Liaset et al., 2003). Proteins, together with chitin and astaxanthin, were extracted from shrimp using enzymatic treatment with alcalase and pancreatin. Alcalase was more efficient than pancreatin, which increased recovery of proteins from 57.5 to 64.6% and of astaxanthin from 4.7 to 5.7 mg astaxanthin per 100 g of dry waste, at a degree of hydrolysis of 12%. An increase in the DH from 6 to 12% resulted in 26% to 28% protein recovery (Routray et al., 2019). Alcalase hydrolysis of the industrial waste from Xiphopenaeus kroyeri shrimp allowed 65% protein recovery in the form of hydrolysates (Holanda and Netto, 2006).
Bioactive peptides are specific protein fragments that can have high nutraceutical potentials and may be able to address important public health issues like obesity, stress, hypertension, and more. Such peptides have been produced from hydrolyzates of several fish and shellfish. Their potential functions include antimicrobial, antiviral, antitumor, antioxidative, antihypertensive, cardioprotective, anti-amnesiac, immunomodulatory, analgesic, antidiabetic, antiaging, appetite-suppressing, and neuroprotective activities (Chalamaiah et al., 2012; Vijaykrishnaraj and Prabhasankar, 2015). These activities are related to the sequence, composition, and type of amino acids in the peptides. FPHs can be used as a source of bioactive peptides with potentials for use as functional food ingredients industry. Tonon et al. (2016) prepared protein hydrolysate from the shrimp cooking effluents by enzymatic hydrolysis and ultrafiltration. The hydrolyzate prepared at 75°C and at pH of 9.0 have essential amino acids that can satisfy people's recommended daily needs. The preparation had significant antioxidant activities. Hypertensive and antioxidant peptides were prepared by enzymatic hydrolysis of proteins from cuttlefish wastewater. The proteins were initially concentrated by ultrafiltration (Amado et al., 2013). Pepsin soluble collagen (PSC) was enzymatically hydrolyzed, and the resultant hydrolysates were ultrafiltrated and characterized. Electrophoretic patterns showed the typical composition of type I collagen, with denaturation temperatures ranging between 23 and 33°C. In terms of antioxidant capacity, results revealed significant intraspecific differences between hydrolysates, retentate, and permeate fractions when using β-Carotene and DPPH methods (Blanco et al., 2017). The presence of both omega-3 fatty acids and ACE-inhibitory peptides in squid hydrolyzate suggested its nutraceutical potential (Apostolidis et al., 2016). In order to use and commercialize bioactive hydrolysates and peptides as food ingredients, a number of significant challenges must first be overcome. These include high production costs, likely negative sensory attributes in end products, taste modifications of carrier food products, and potential toxicity or allergenicity, among others (Lafarga and Hayes, 2017).
The enormous pool of biodiversity in marine ecosystems offers a reservoir of enzymes with potential biotechnological applications. Enzymes from aquatic animals, particularly from marine habitats, exhibit significant variations in their properties in comparison with enzymes from terrestrial sources. The factors responsible for the variations include molecular weights, amino acid compositions, optimal pH and temperature requirements, inhibition characteristics, and kinetic properties, which facilitate their novel uses for a variety of practical applications. Seafood discards such as viscera, liver, and head are sources of enzymes including proteases including pepsin, gastricin, trypsin, collagenase, elastase, and peptidases, transglutaminases, lipases, phospholipase, chitinases, β-1, 3-glucanase, carrageenases, and others. Methodologies for their isolations from various seafood processing discards have been summarized (Shah et al., 2016; Murthy et al., 2018). Enzymes from seafood discards can be used for various seafood processing operations, as shown in Table 3.
The global production of fish oil is around one million tons, predominated by cod liver oil (Bimbo, 2007). Fish oils are rich in omega-3 PUFA and vitamins A and D. The oil of fish species, such as Atlantic mackerel, shark, anchovies, menhaden, and Atlantic sardine, can have up to 35% omega-3 fatty acids, with EPA and DHA at around 10% of the oil (Venugopal, 2009). Fish processing discards, particularly the livers of albacore, cod, salmon, shark, haddock, and tuna, are good sources of oil. An average production of 10,000 kg filets of cod will generate by-products with more than 1,000 kg marine lipids (Falch et al., 2006). Conventionally, fish oil is extracted by wet reduction method involving cooking, pressing, and filtration. The extracted oil is purified by carbon treatment, degumming, alkali refining, deodorization, and stabilization by antioxidants for prolonged storage, while the protein-rich press liquor is used as animal feed (Venugopal, 2009). There have been concerns fueled by ominous predictions of depletion of several oil-rich oceanic fishes, which necessitates better exploitation of fish discards as oil resources.
Whereas, conventional extraction methods can lead to oxidation of fish oils, rich in unsaturated fatty acids, as pointed out earlier, green processes can help with the recovery of fish oil with minimum oxidation (Ivanovs and Blumberga, 2017). Fish viscera are an important source of lipids, with a content ranging from 19 to 21%. Up to 85% of this could be recovered by natural fermentation. Fermentation using added lactic cultures did not show any advantage over natural fermentation with respect to recovery of oil. Activity of acidic, neutral, and alkaline proteases decreased during fermentation. Even though the degree of protein hydrolysis increased up to 62% during fermentation using Pediococcus acidilactici K7, no differences were observed in the amounts of recovered proteins (Rai et al., 2010). Catfish viscera, a by-product of catfish processing, are industrially used to produce edible oils (Shahidi et al., 2019). The liver of shark is 22–30% of its body weight and its liver may contain oil as high as 90% of its weight. Natural decomposition, ensilage in presence of formic acid, alkali digestion, and steam rendering recovered oil from shark liver (Venugopal, 2009). Salmon frames were hydrolyzed by a mixture of commercial proteases, which helped recovery of 77% of total lipids present in the salmon frames as EPA and DHA rich oil (Liaset et al., 2003).
The enzymatic process disrupts the tissue and membranes under mild conditions to release the oil from fish by-products, such as liver and roe (Dumay et al., 2004). Treatment of salmon heads and other byproducts by commercial proteases (alcalase, neutrase, and flavourzyme) for 2 h released 17% oil, which contained 11.6 and 5.6% of DHA and EPA, respectively (Routray et al., 2019). Oil from sardine was obtained at 5.5, the iso-electric pH the fish meat, which was adjusted by citric acid. The separated oil had good n-3 PUFA contents and exhibited high oxidative stability (Okada and Morrissey, 2007). Alcalase-based extraction of oil from tuna was conducted for 120 min at 60°C and pH 6.5 at an enzyme–substrate ratio of 1:200. The enzyme- extracted oil had lowest acidity and peroxide values and higher levels of EPA and DHA contents than chemically refined oil (de Oliveira et al., 2016). Hydrolysis of shrimp waste with alcalase gave an oil yield of 28.6 μg per g waste (Sachindra and Mahendrakar, 2011). The hydrolyzate of squid processing by-products had EPA and DHA at 16.9 and 29.2% of oil, respectively. About half of the oil was comprised of phospholipids (Apostolidis et al., 2016).
Lipases have grown in importance due to their ease of availability and possibilities for product modifications. Candida rugosa lipase was used to concentrate fatty acids in the glyceride fraction of the oil. By controlling the degree of hydrolysis, two products were obtained, one having 50% n-3 PUFA, and the other having 40% DHA and 7% EPA. The glyceride from these reactions was converted back to triglycerides using Rhizomucor miehei lipase catalyzed partial hydrolysis and esterification (Moore and McNeill, 1996). Lipolysis of salmon oil by a commercial lipase gave a mixture of free fatty acids and acylglycerols. A hydrophobic membrane was used to separate high melting saturated fatty acids from low melting acylglycerols. The sum of total PUFA increased from 42% in the crude oil to 47% in the filtrate with increase of DHA and EPA contents from 9.9 to 11.6%, and from 3.6 to 5.6%, respectively (Linder et al., 2005).
Carotenoids provide red and orange colors to some foods. The pigments are present in shellfish, krill, shrimp, crab, crayfish, and also in salmon and trout. The red-orange color of cooked crustaceans is attributed to partial or complete separation of astaxanthin from the protein moiety to which it is attached in the native state. Carotenoids may be hydrocarbons, such as β-carotene or xanthophylls, or oxygenated derivatives, such as astaxanthin, astacene, canthaxanthin, cryptoxanthin, lutein, neoxanthin, violaxanthin, and zeaxanthin. Astaxanthin (3,3′-dihydroxy-β, β′-carotene-4,4′-dione) and canthaxanthin (β,β-carotene-4,4′-dione) have been used in aquafeed for many years in order to impart the desired flesh color in farmed salmonids. The shells of shrimp, prawn, crawfish, krill, crab, and lobster are important sources of astaxanthin, bound to free protein or chitin, and range from 40 to 200 μg per g, dry weight. Canthaxanthin is present in crayfish, mytiloxanthin in mussel, and mactraxanthin and fucoxanthin are present in clams (Sowmya and Sachindra, 2015; de Carvalho and Caramujo, 2017).
Microbial fermentation-based bioconversion methods can extract carotenoids from crustacean shells, giving better yields than conventional solvent extraction. During bacterial fermentation processes, the proteins and minerals present in the shrimp shells are effectively removed, thereby increasing the extraction efficiency of the pigments without any change in quality. Current extraction methods make use of proteolytic enzymes such as trypsin and alcalase and fermentation by LAB and other microorganisms (Prameela et al., 2017; Routray et al., 2019). Fermentation of shrimp shell waste using the lactic acid bacterium Pediococcus acidolactici under optimal conditions resulted in 98% deproteination, 72% demineralization, and carotenoid recovery of up to 78% (Bhaskar et al., 2007). Shrimp waste was hydrolyzed with alcalase at optimal conditions of 0.75% of enzyme for 150 min at 37°C. The recovered carotenoids were extracted in sunflower oil at an oil to hydrolyzed waste ratio of 2:1 at 70°C for 90 min (Sachindra and Mahendrakar, 2011). Caroteno-protein from pink shrimp (Parapenaeus longirostris) waste was extracted by trypsin treatment for 1 h at 25°C. The recovered caroteno-protein fraction after freeze-drying contained about 71% protein, 16% lipid, 8% ash, 2% chitin, and 87 μg astaxanthin per g of the sample. Enzymatic hydrolysis of the protein–pigment complex allows studies on pigment absorption, stability, and application (Sila et al., 2012). Trypsin from bluefish was used to extract caroteno-proteins from black tiger shrimp shells. The extract also contained 70% protein, 20% lipid, 6.6% ash, 1.5% chitin, and 87.9 μg with total astaxanthin per g sample (Klomklao et al., 2009). Autolysis of shrimp heads resulted in the recovery of 195 μg carotenoids per g wet shells (Cahu et al., 2012).
Processing effluents can also be used as a medium for the production of carotenoids. Employing non-sterilized mussel processing wastewater as a low-cost substrate for yeast fermentation, the green microalga (H. pluvialis) was cultivated in fish effluents for the production of astaxanthin with significantly greater antioxidant capacity than the synthetic one (Shah et al., 2016). Similarly, mussel processing water was used for the production of astaxanthin by Xanthophyllomyces dendrorhous (Amado and Vazquez, 2015). With shrimp waste, being highly perishable and seasonal, the fermented carotenoid-rich liquor can be prepared as per the availability of the waste and can be stored up to 75 days under normal storage conditions. Carotenoids from the liquor can be extracted by ultrasonic or supercritical CO2 extraction, or can be extracted in palm oil or other vegetable oils or by high pressure chemical extraction (Sowmya and Sachindra, 2015). Astaxanthin is stable at 70–90°C in rice bran, ginger, and palm oils. Astaxanthin has important applications in the nutraceuticals, cosmetics, food, and aquaculture industries (Ambati et al., 2014; Shah et al., 2016).
The dry shell discards of crab, shrimp, and lobster may contain up to 70% chitin; dry squid skeleton pen and krill shells have a lower chitin content of 40%. The commercial process of extraction of chitin from crab and shrimp shells involves three steps: demineralization of dried and pulverized shells by dilute hydrochloric acid; deproteinization by dilute alkali; and decoloration, washing, and drying. Chitin is deacetylated to chitosan using 30 to 60% (w/v) sodium or potassium hydroxide at 80–140°C. The yield of chitin is about 25% of dry shell, and the yield of shrimp chitosan about 77% of the crude chitin (Dima et al., 2017). The chemical methods and the high treatment temperatures have influence on molecular weight, degree of deacetylation, and the functional properties of chitosan (Venugopal, 2009). Biological processes give products of better quality, require less energy, and consume less fresh water, unlike chemical processes (Arbia et al., 2013; Kaur and Dhillon, 2015; Mao et al., 2017; Lopes et al., 2018).
Fermentation is beneficial for extraction of chitin from seafood processing discards (Yadav et al., 2019). Fermentation by lactic acid bacteria (LAB) has advantages over conventional methods for chitin extraction. One beneficial LAB is Lactobacillus plantaru. Other LABs include L. paracasei, L. acidophilus, L. lactis, L. paracasei, S. marcescens, and T. turnirae. Non-LAB organisms can also be used for fermentation (Vázquez et al., 2013, 2019). An epiphytic L. acidophilus is isolated from rapidly fermented shrimp waste. The chitin released in the fermented product can be easily transformed by a bleaching treatment. The product had better quality than chemically extracted chitin (Duan et al., 2012). Fermentation of shrimp head by a consortium of LAB for 48 h gave chitin and protein rich liquor; the latter can be used as aquafeed supplement (Ximenes et al., 2019). Jung et al. (2007) employed L. acidophilus for chitin extraction from crab shell waste by two-step fermentation, involving L. paracasei in the first step, followed by a protease producing bacterium Serratia marcescens. The process removed 94% CaCO3 and 70% proteins. The highest deproteinization of 96% and demineralization (68%) were achieved through the combination of two-stage solid state culture by Lactobacillus brevis and Rhizopus oligosporus. Lactic acid was the main organic acid produced along with acetic, succinic, and oxalic acids. The purified chitin presented a molecular weight of 1,313 × 103 Da, preserving a high crystalline index and acetylation of 94% (Aranday-García et al., 2017). Sieber et al. (2018) suggested the use of natural microbial isolates as well as Serratia spp. and Lactobacillus spp. in fermentations that can realize a demineralization of 97%. Younes et al. (2016) used fermentation to extract highly acetylated chitin from crustacean shells, which were initially subjected to demineralization and enzymatic deproteinization prior to the treatment.
Fermentation by LAB for a maximum period of 7 days resulted in extensive deproteination and demineralization of crustacean shells, facilitating chitin recovery. The process can be conducted under conditions such as anaerobic, solid-stat, semi-continuous, or co-fermentation (Vázquez et al., 2020). Ghorbel-Bellaaj et al. (2011) optimized fermentation variables in accordance with Plackett–Burman design, which resulted in 96% demineralization and removal of 89% protein. Fermentation of shrimp head by Bacillus licheniformis released appreciable amounts of polysaccharides and other compounds in the fermented medium (Mao et al., 2017). Bacillus cereus and Exiguobacterium acetylicum accomplished 90% demineralization and deproteination during chitin extraction from shrimp waste (Sorokulova et al., 2009). Autolysis of shrimp heads could recover not only chitin and chitosan, but also protein hydrolyzate, carotenoids, sulfated-, and amino-polysaccharides. An amount of 25 mg of chitin and 17 mg chitosan (60–80% deacetylated) per g of wet shells were recovered (Cahu et al., 2012). A pilot plant study by Vázquez et al. (2017) employed a combination of enzymatic, acid, and alkaline processes for the recovery of chitin and also protein and carotenoprotein from the cephalothorax of Penaeus vannamei. The sequential treatment yielded 30% chitin with 92% acetylation. In another combination process, protease was used to remove Ca2+ and protein, followed by fermentation by Bacillus coagulans to extract chitin from crayfish shell waste, which resulted in recovery of chitin as high as 94% (Dun et al., 2019). Most of these studies reported deproteination and demineralization in the range of 45–90%.
Extraction of chitin from shellfish waste is facilitated by initial deproteinization and demineralization. One of the biological alternatives proposed is the use of proteases for deproteinization of crustacean shells, avoiding alkaline treatments. When using enzymatic deproteinization, previous demineralization is more convenient since it increases enzyme permeability of the tissues and reduces the presence of potential enzyme inhibitors. Crude proteases from fish discards such as viscera can be used for deproteination, which can lower the cost of treatment (Shah et al., 2016). Instead of the conventional hydrochloric acid, the use of organic acids (lactic and acetic) produced by cheese whey fermentation has been suggested for demineralization of shrimp shells. Organic acids were comparable to hydrochloric acid but less harmful when helping to maintain the integrity of chitin (Mahmoud et al., 2007). Proteolytic enzymes remove up to 90% of the protein and carotenoids from demineralized shrimp waste. Hamdi et al. (2017) extracted chitin from blue crab and shrimp shells by crude crab viscera alkaline protease digestion at pH 8.0 and 60°C. The treatment caused up to 91% deproteinization, facilitating the release of chitin.
In recent times there has been increased interest in using broad-specificity chitinases for chitin extraction. These novel enzymes possess two or three different catalytic activities, functioning as exochitinase, endochitinase, and N-acetyl-glucosaminidase. Endochitinases cleave chitin at internal sites, thereby generating low-molecular weight chitin oligosaccharides (COG), while exochitinases or chitobiases catalyze the progressive release of chitin dimmers by cleaving the polysaccharide at external sites (Suresh, 2012). Recently, a cold-adapted chitinase from a marine bacterium was characterized by broad pH stability, high thermo-stability, low Km value, and optimal activity at 30 °C, with 35% activity at 0°C.The enzyme completely degraded colloidal chitin into N-acetyl glucosamine (GlcNAc). The enzyme was suggested to be a superior candidate for producing bioactive oligosaccharides (Fu et al., 2020). A mass production of chitonolytic enzymes by cultures of micro-organisms, such as Trichoderma hamatum, T.viride, Aspergillus niger, and Carica papaya, will be beneficial for large scale extraction of chitin and its transformation into valuable commercial products as a solution to waste management (Yadav et al., 2019). Chitin-degrading enzymes from Serratia marcescens, Amantichitinus ursilacus, and Andreprevotia ripae have been used on a pilot scale to degrade chitin into monomers with yields up to 95% (Sieber et al., 2018).
Chitin deacetylase from fungi such as such as Mucor rouxii, M. mechei, and Aspergillus niger catalyzes the hydrolysis of N-acetylamido linkage of chitin to give chitosan. The crystallized chitin, after pretreatment with 18% formic acid, is amenable to 90% deacetylation by the fungal deacetylase (Suresh, 2012). To enhance the accessibility of chitin deacetylase to acetyl groups of natural crystalline chitin, pretreatment may be needed with physical or chemical methods such as sonication, grinding, heating, and derivatization (Yadav et al., 2019). The various applications of chitin and chitosan are not topics of discussion here.
Crude enzyme from Bacillus cereus was used to hydrolyze chitosan having 66% deacetylation in a membrane reactor, operated at 45 °C and pH 5. The major oligomers were chitobiose, chitotriose, chitotetraose, chitopentaose, and chitohexaose. The system could be operated for 15 h and still maintained a stable product composition (Kuo et al., 2004). Chito-oligomers (COS), the depolymerized products of chitosan, have attracted considerable interest due to their biocompatible, biodegradable, non-toxic, and non-allergenic natures, and potential applications in biomedical, food, pharmaceutical, agricultural, and environmental industries (Ngo et al., 2020). These suggest a need for commercial production of chitinases and chitosanases (Suresh, 2012; Zhou et al., 2019). Chitosan and its derivatives have been reported to possess various biomedical activities including free radical scavenging, antihypertensive, anticoagulant, antidiabetic, antiobesity, antiallergic, anti-inflammatory, antimicrobial, anticancer, and anti-Alzheimer effects. The antibacterial and antifungal properties of chitosan qualify it for use in food packaging films. Its mechanical, gas, and water vapor permeability properties can be enhanced by blending chitosan with other natural polymers such as starch, essential oils, and clay (Venugopal, 2011). Figure 3 depicts the biological process for extraction of chitin and its conversion to chitosan.
Figure 3. Bioconversion processes for extraction of chitin, chitosan, and protein hydroluzate (adapted from Vázquez et al., 2013).
Glycosaminoglycans (GAGs) are hetero-polysaccharides defined by a repeating disaccharide unit without branched chains, in which one of the two monosaccharides is an amino sugar (N-acetyl-galactosamine or N-acetyl-glucosamine) and the other one is a uronic acid. Based on the disaccharide composition, linkage type, and presence of sulfate groups, GAGs may be chondroitin sulfate (CS), hyaluronic acid (HA), dermatan sulfate, heparin, or keratan sulfate. CS chains have an important function in central nervous system development, wound repair, infection, growth factor signaling, morphogenesis and cell division, differentiation, and migration in addition to osteoarthritis and their conventional structural roles. CS from terrestrial and marine sources contains diverse chain lengths and sulfation. Shark cartilage may contain up to 29% CS, having a molecular weight of 40 kDa. Hyaluronic acid is a linear, high molecular weight linear, and non-sulfated GAG made by alternating disaccharide units of N-acetyl-D-glucosamine and D-glucuronic acids, linked by β-(1 → 3) and β-(1 → 4) glycosidic bonds.). Autolysis of shrimp head waste gave about 8 mg sulfated GAGs per g that exhibited electrophoretic migration similar to mammalian standards. The degradation products of the GAGs suggested the presence of C6-sulfated heparan sulfate (Cahu et al., 2012). Vázquez et al. (2013) reviewed environmentally friendly processes combining microbial, enzymatic, and other strategies to produce CS, HA, chitin, and chitosan. Bacterial production of HA using Streptococci has been industrially developed (Vázquez et al., 2013). Chondroitin sulfate and hyaluronic acid are commercially valuable. The structural similarity of microbial capsular polysaccharides to these biomolecules makes bacteria ideal candidates as non-animal sources of glycosaminoglycan-derived product GAGs because of their high bioactivities and physiological functions. Fish cartilage products, such as shark cartilage and chondroitin sulfate, glucosamine, and other glucosaminoglycans, are able to alleviate rheumatoid arthritis (Venugopal, 2009).
Finfish discards, which contain significant amounts of bone, are rich sources of minerals. The bone is composed of up to 70% minerals, followed by collagen, certain carbohydrates, and lipids. Hydroxyapatite and calcium phosphate have attracted attention for biomedical applications such as implant materials. Grass fish bones were subjected to flavourzyme treatment followed by fermentation with Leuconostoc mesenteroides, giving a preparation with a high content of soluble calcium lactate, calcium acetate, and also small peptides containing calcium. The calcium is bioavailable and therefore can promote growth, as shown by animal studies, suggesting its use as a calcium supplement (Tang et al., 2018). Salmon frames were hydrolyzed by a mixture of commercial proteases. After the procedure, the frames were separated by centrifugation into a bone fraction, which contained 62% of total ash present in the salmon frames. The fraction was high in calcium, phosphorus, and magnesium and also in various trace elements such as copper, iron, selenium, and zinc (Liaset et al., 2003). A fish bone phosphopeptide (FBP) containing up to 24% of phosphorus has a molecular weight of 3.5 kDa and a high calcium-binding activity. The FBP has potential nutraceutical value as a calcium binding agent (Jung et al., 2005). A combination of micro and nano-structured hydroxyapatite (HAp) was isolated from tuna bone. The isolated Hap had comparable physicochemical characteristic with that of standard Hap and was also less toxic (Pallela et al., 2011). Pepsin hydrolyzate of channel catfish bones has antibacterial activity, suggesting that the fish bones are promising resources for generating antibacterial components (Ren et al., 2012).
The various bioconversion processes employing microorganisms and enzymes for the recovery of components from seafood processing discards and effluents are summarized in Tables 4, 5. Table 4 summarizes bioconversion processes using LAB and other microorganisms, while Table 5 summarizes enzymatic bioconversion processes.
The above discussions pointed out various biological processes for the extractions of important compounds from seafood discards. While individual processes may not be economically feasible, an integrated refinery-type process for the extractions of multiple products is more practical. A “refinery” generally means conversion of raw materials into products of higher values, petroleum oil refinery being the most popular example. The International Energy Agency defined bio-refinery as the “sustainable processing of biomass into a spectrum of bio-based products (food, feed, chemicals, and materials) and bio-energy (de Farias and Barbera, 2018). The bio-refinery concept visualizes bio-waste as a potential renewable feedstock that can be valorized through a cascade of various biotechnological processes to produce marketable products and bioenergy on par with petro-chemical refineries. It involves stepwise refining processes using biological methods for the extraction of various high value biomolecules. Such downstream strategies could reduce overall production costs (Das, 2015; Mohan et al., 2016; Mitra and Mishra, 2019; Dineshkukar and Sen, 2020). Bio-refineries, which aim at valorizing biomass from agriculture and aquaculture, into a wide spectrum of products and bio-energy, have been recognized as part of a sustainable economy (Dragone et al., 2020).
Fish processing waste can be a promising renewable biomass for bio-refineries. The bio-refinery approach envisages conversion of fish waste into value-added products such as biofuels, industrial chemicals, animal feed, organic fertilizer, nutraceuticals, and others. Low cost and simplicity of operation by reducing the cost of material, energy consumption, and labor, but maintaining high productivity are some of the important attributes of the process (Sahu et al., 2016). An example is shell refinery, where crustacean shell waste is subjected to sequential treatment to recover chitin, proteins, lipids, carotenoids, calcium carbonate, and chitin monomers (Hülsey, 2018). Vázquez et al. (2019) coupled alcalase hydrolysis with bacterial fermentation to extract gelatin, oils, fish protein hydrolysate including bioactive peptides, and fish peptones from heads, skin, and bones of fish discards. Cahu et al. (2012) reported an integrated process employing autolysis of shrimp heads to recover chitin and chitosan, protein hydrolyzate, and sulfated- and amino-polysaccharides. Lactic acid fermentation followed by green extraction processes including filtration and centrifugation can lead to sequential or simultaneous extractions of astaxanthin, hydrolyzed protein, and chitin from crustacean shell waste (Vázquez et al., 2017; Routray et al., 2019). Similarly, anaerobic fermentation of fish waste resulted in methane and liquid fertilizer as primary products. The purchase price of methane is a crucial factor influencing the economics of the bio-refinery (Ratky and Zamazal, 2020). Another bio-refinery deals with extraction of oil from fish waste, its transesterification with ethanol, and concentration of n-3 PUFA. Fishmeal, glycerol, and saturated and short chain unsaturated fatty acids as liquid bio-fuel are the other products of the refinery. The process can significantly supply thermal energy and reduce CO2 discharge (Fiori et al., 2017). A bio-refinery developed within an EU-funded project combines chitin demineralization by Serratia spp. and Lactobacillus spp. and an enzymatic degradation of chitin by chitin-degrading enzymes from Serratia marcescens, Amantichitinus ursilacus, and Andreprevotia ripae. The resulting N-acetylglucosamine monomers could be used for novel bio-based polymers. Proteins and lipids could be used as feed for biogas production (Sieber et al., 2018). Eurofish processes roughly 200 tons of tuna a day with discharge of at least 1,300 m3 effluents. The company coupled seafood waste-to-energy technology, generating 1,300 m3 methane daily. This reduced wastewater treatment costs by 50% and energy consumption by 35–40%. The plant has been in operation since March 2016, suggesting economic feasibility of bio-refineries based on seafood waste valorization (Fluence, 2019).
Algal biotechnology can be a promising platform for bio-refining of seafood discards. The cultivation of microalgae in bio-wastes is known to produce SCP, as mentioned earlier. The recovery of products from algal biomass is a matter of constant development and progress (Sosa-Hernández et al., 2018). Whereas, the exploitation of SCP for a single product such as biofuel is not economically viable, multiple products such as pigments, antioxidants, and n-3 fatty acids can be extracted from SCP to make the process cost-competitive. The various possibilities are depicted in Figure 4. The approach offers novel ways to utilize wastewater and also help in the promotion of microalgae in the commercial market (Koyande et al., 2019; Mitra and Mishra, 2019). The increasing resource limitations are expected to drive SCP production and improve the economic feasibility in the future (Puyol et al., 2017). On the basis of increasing demands, recently seaweed has been cultivated with improved traits to harvest more than one product through a bio-refinery. Three combination routes have been suggested for production of microalgae-based biodiesel, bio-hydrogen, and SCP (Banu et al., 2020). Cultivation of the microalga H. pluvialis for both SCP and astaxanthin can be an economically sustainable process (Shah et al., 2016; Khoo et al., 2019). food producers must now address environmental concerns, social responsibility and economic viability when designing their food processing techniques food producers must now address environmental concerns, social responsibility and economic viability when designing their food processing techniques. Systematic improvement of the technology readiness level (TRL) could be successful if applied to microalgae cultivation and processing (Caporgno and Mathys, 2018). Recent text mining tools on articles and patents published on algal biotechnology during the period 2012–2017 identified Reinhardtius sp. for wastewater treatment and a Chlorellum strain for biofuel and fatty acids (Parkavi et al., 2020). At present, downstream processing, and in particular the fractionation of microalgal components, remains the most expensive step of the algal processes, demanding novel technologies for SCP processing (Gifuni et al., 2019). Cho and Park (2018) observed that commercialization of the microbial route for fuel production remains uncertain due to the high cost of feedstock or low lipid yield. However, considering the low cost of seafood discards and effluents and potentials for enhancing fuel production through algal technology, as mentioned earlier, this observation may not be realistic. Table 6 summarizes recent bio-refinery approaches for seafood valorization.
Figure 4. Microalgae based bio-refinery for various products from seafood discards (adapted from Koyande et al., 2019).
The article pointed out the major problems associated with discards and process effluents, generated during industrial seafood processing. Besides being responsible for environmental pollution, the discards and effluents represent heavy losses of nutrients and other valuable compounds. There problems can be addressed by biological treatment processes, involving bioconversions of components of the waste by microorganisms and enzymes. Unlike conventional chemical treatments, biological processes are environmentally friendly, safe and economical. Further, biological processes do not adversely affect functional properties of isolated compounds, unlike the chemical processes. Biomass fermentations-using microalgae are emerging as green and economical processes to recover functionally active compounds and also biofuel. Fermentation is ideal to recover functionally active chitin from crustacean shell. Similarly, fermentation or lipase-based processes can replace hazardous solvent extraction techniques for the recovery of fish oil Microorganisms-mediated processes are highly desirable due to ease of handling, lower energy requirements and costs. With the development of tailor made bio-catalysts and advances in green extraction techniques, it is possible to take up challenges of successful bio-processing of seafood discards and effluents. Genetic engineering of microorganisms, enzyme engineering, reactor designs and process optimizations offer strategies leading to a new manufacturing paradigm for successful valorization of seafood waste. The interesting features of microalgae such as their rapid growth, their photosynthetic ability, nutrient-rich characteristics of the cells make microalgae promising bio-platform for seafood waste re-cycling and energy transformation. Although process identification, scaling up and implementation of resource recovery solutions at an industrial level is a challenge, developments in these fields can favor successful waste utilization for sustainable fish processing towards a circular bioeconomy.
The author is the sole contributor of this work and has approved it for publication.
The author declares that the research was conducted in the absence of any commercial or financial relationships that could be construed as a potential conflict of interest.
Abdollahi, M., Rezaei, M., Jafarpour, A., and Underland, I. (2018). Sequential extraction of gel-forming proteins, collagen and collagen hydrolysate from gutted silver carp (Hypophthalmichthys molitrix), a bio-refinery approach. Food Chem. 242, 568–578. doi: 10.1016/j.foodchem.2017.09.045
Achour, M., Khelifi, O., and Hamdi, M. (2000). Design of an integrated bioprocess for the treatment of tuna processing liquid effluents. Proc. Biochem. 35, 1013–1017. doi: 10.1016/S0032-9592(00)00133-3
Amado, I. R., and Vazquez, J. A. (2015). Mussel processing wastewater: a low-cost substrate for the production of astaxanthin by Xanthophyllomyces dendrorhous. Microb. Cell Fact. 14:177. doi: 10.1186/s12934-015-0375-5
Amado, I. R., Vazquez, J. A., Gonzalez, M. P., and Murado, M. A. (2013). Production of antihypertensive and antioxidant activities by enzymatic hydrolysis of protein concentrates recovered by ultrafiltration from cuttlefish processing wastewater. Biochem. Eng. J. 76, 43–54. doi: 10.1016/j.bej.2013.04.009
Ambati, B. R., Moi, P. S., Ravi, S., and Ashwathanarayana, R. G. (2014). Astaxanthin: sources, extraction, stability, biological activities and its commercial applications—A Review. Marine Drugs 12, 128–152. doi: 10.3390/md12010128
Anihouvi, V. B., Kindossi, J., and Hounhouigan, D. J. (2012). Processing and quality characteristics of some major fermented fish products from Africa: a critical review. Int. J. Biol. Sci. 1, 72–84.
Apostolidis, E., Karayannakidis, P. D., and Lee, C. M. (2016). Recovery of bioactive peptides and omega-3 fatty acids-containing phospholipids from squid processing by-product hydrolysate. Aquatic Food Products Technol. 25, 496–506. doi: 10.1080/10498850.2013.878890
Aranday-García, R., Guerrero, A. R., Ifuku, S., and Shirai, K. (2017). Successive inoculation of Lactobacillus brevis and Rhizopus oligosporus on shrimp wastes for recovery of chitin and added-value products. Proc. Biochem. 58, 17–24. doi: 10.1016/j.procbio.2017.04.036
Arbia, W., Arbia, L., Adour, L., and Amrane, A. (2013). Chitin extraction from crustacean shells using biological methods – A Review. Food Technol. Biotechnol. 51, 12–25.
Arvanitoyannis, I. S., and Kassaveti, A. (2008). Fish industry waste: treatments, environmental impacts, current and potential uses. Int. J. Food Sci. Technol. 43, 726–745. doi: 10.1111/j.1365-2621.2006.01513.x
Ashraf, S. A., Adnan, M., Patel, M., Siddiqui, A. J., Sachidanandan, M., Snoussi, M., et al. (2020). Fish-based bioactives as potent nutraceuticals: exploring the therapeutic perspective of sustainable food from the sea. Mar Drugs 18:265. doi: 10.3390/md18050265
Banu, J. R., Preethi, S., Kavitha, S., Gunasekaran, M., and Kumar, G. (2020). Microalgae based bio-refinery promoting circular bio-economy-technology. Economic and life-cycle analysis. Bioresource. Technol. 302:22822. doi: 10.1016/j.biortech.2020.122822
Bhaskar, N., Suresh, P. V., Sakhare, P. Z., and Sachindra, N. M. (2007). Shrimp bio-waste fermentation with Pediococcus acidolactici CFR2182: Optimization of fermentation conditions by response surface methodology and effect of optimized conditions on deproteination/demineralization and carotenoid recovery. Enz. Microb. Biotechnol. 40, 1427–1434. doi: 10.1016/j.enzmictec.2006.10.019
Bimbo, A. P. (2007). Current and future sources of raw materials for the long-chain omega-3 fatty acid market. Lipid Technol. 19, 176–181. doi: 10.1002/lite.200700057
Blanco, M., Vázquez, J. A., Pérez-Martín, R. I., and Sotelo, C. G. (2017). Hydrolysates of fish skin collagen: an opportunity for valorizing fish industry byproducts. Marine Drugs 155:131. doi: 10.3390/md15050131
Bruno, S. F., Ekorong, F. J. A. K., Karkal, S. S., and Cathrine, M. S. B. (2019). Green and innovative techniques for recovery of valuable compounds from seafood by-products and discards: A review. Trends Food Sci. Technol. 85, 10–29. doi: 10.1016/j.tifs.2018.12.004
Cahu, T. B., Santos, S., Mendes, A., Cordula, C. R., Chavante, S. F., Carvalho, J., et al. (2012). Recovery of protein, chitin, carotenoids glycosaminoglycans from Pacific white shrimp (Litopenaeus vannamei) processing discard. Process Biochem. 47, 570–577. doi: 10.1016/j.procbio.2011.12.012
Caporgno, M., and Mathys, A. (2018). Trends in microalgae incorporation into innovative food products with potential health benefits. Front Nutr. 5:58. doi: 10.3389/fnut.2018.00058
Chalamaiah, M., Dinesh Kumar, B., Hemalatha, R., and Jyothirmayi, T. (2012). Fish protein hydrolysates: proximate composition, amino acid composition, antioxidant activities and applications: a review. Food Chem. 135, 3020–3038. doi: 10.1016/j.foodchem.2012.06.100
Chandrasekharan, M. (2015). “Biotechnology for utilization of marine by-products”, in Fish Processing Byproducts, eds. N. M.Sachindra and N. S. Mahendrakar (Houston, TX: Studium Press), 43–62.
Chavez, G. J., Villa, J. A., Ayala-Zavala, F. J., Heredia, J. B., Sepulveda, D., Yahia, F. M., et al. (2013). Technologies for extraction and production of bioactive compounds to be used as nutraceuticals and food ingredients: an overview. Comp Rev. Food Sci. Food Safety 12, 5–23. doi: 10.1111/1541-4337.12005
Ching, Y. C., and Ghufran, R. (2017). Biological treatment of fish processing saline wastewater for reuse as liquid fertilizer. Sustainability 9:1062. doi: 10.3390/su9071062
Cho, H. U., and Park, J. M. (2018). Biodiesel production by various oleaginous microorganisms from organic wastes. Bioresour. Technol. 256, 502–508. doi: 10.1016/j.biortech.2018.02.010
Choudhury, P., VijayRaghavan, T., and Sreenivasan, A. (2010). Biological treatment process for fish processing wastewater. Bioresou. Technol. 101, 439–449. doi: 10.1016/j.biortech.2009.08.065
Cretton, M., Malanga, G., Sobczuk, T. M., and Mazzuca, M. (2020). Lipid Fraction From Industrial Crustacean Waste and Its Potential as a Supplement for the Feed Industry: A Case Study in Argentine Patagonia. Waste Biomass Valor. 12, 2311–2319. doi: 10.1007/s12649-020-01162-7
Dao, Y. T., and Kim, J. K. (2011). Scaled up bioconversion of fish waste to liquid fertilizer using 5L ribbon type reactor. J. Env. Manage 92, 2441–2446. doi: 10.1016/j.jenvman.2011.05.003
Dara, P. K., Raghavankutty, M., Sebastian, N., and Chatterjee, N. K. (2020). Biological, physico-chemical, and surface-active properties of gelatin extracted from bigeye tuna (Thunnus obesus) skin waste. J. Aquatic Food Prod. Technol. 29, 1–17. doi: 10.1080/10498850.2020.1749745
Das, D. (2015). Algal Biorefinery: An Integrated Approach. Berlin; Heidelberg: Springer. doi: 10.1007/978-3-319-22813-6
de Carvalho, C. C. R., and Caramujo, M. J. (2017). Carotenoids in aquatic ecosystems aquaculture: a colorful business with implications for human health. Front. Mar Sci 4, 1–13. doi: 10.3389/fmars.2017.00093
de Farias, S., and Barbera, B. A. (2018). “Bio-refinery as a promising approach to promote ethanol industry from microalgae and cyanobacteria”, in Bioethanol Production From Food Crops: Sustainable Sources, Interventions and Challenges, eds R. C. Ray, and S. Ramachandran (London: Academic Press), 343–359. doi: 10.1016/B978-0-12-813766-6.00017-5
de Oliveira, D. A., Minozzo, S. B., and Licodiedoff, M. G. (2016). Physicochemical and sensory characterization of refined and deodorized tuna (Thunnus albacares) by-product oil obtained by enzymatic hydrolysis, Food Chem. 207, 187–194. doi: 10.1016/j.foodchem.2016.03.069
Dima, J. B., Sequeiros, C., and Zaritzky, N. (2017). “Chitosan from marine crustaceans: production, characterization and applications,” in Biological Activities and Application of Marine Polysaccharides, ed E. A. Shalaby (Rijeka: InTech), 39–56. doi: 10.5772/65258
Dineshkukar, R., and Sen, R. (2020). A sustainable perspective of microalgal biorefinery for co-production and recovery of high value carotenoid and biofuel from CO2 valorization. Biofuel Bioprod Bior. 14, 879–897. doi: 10.1002/bbb.2107
Doan, C. T., Tran, T. N., Nguyen, V. B., and Wang, S.-L. (2020). Bioprocessing of squid pens waste into chitosanase by Paenibacillus sp. TKU047 and its application in low-molecular weight chitosan oligosaccharides production. Polymers 12:1163. doi: 10.3390/polym12051163
Doan, C. T.;, Tran, T. N.;, Nguyen, V. B.;, Vo, T. P. K., Nguyen, A. D., and Wong, S. L. (2019). Chitin extraction from shrimp waste by liquid fermentation using an alkaline protease-producing strain, Brevibacillus parabrevis. Int. J. Biol. Macromol. 131, 706–715. doi: 10.1016/j.ijbiomac.2019.03.117
Dragone, G., Kerssemakers, A. A. J., Driessen, J. S. L. P., Yamakawa, C. K., Brumano, L. P., and Mussatto, S. I. (2020). Innovation and strategic orientations for the development of advanced biorefineries. Bioresource Technol. 302:122847. doi: 10.1016/j.biortech.2020.122847
Duan, S., Zhuang, Z., Wu, W., Hong, S., and Zhou, J. (2012). Improved production of chitin from shrimp waste by fermentation with epiphytic lactic acid bacteria. Carb. Polym. 89, 1283–1288. doi: 10.1016/j.carbpol.2012.04.051
Dumay, J., Barthomeuf, C., and Berge, J. P. (2004). How enzymes may be helpful for upgradaging fish byproducts enhancement of fat extraction. J. Aquatic Food Prod. Technol. 13, 69–84. doi: 10.1300/J030v13n02_07
Dun, Y., Li, Y., Xu, J., Hu, Y., Zhang, C., Liang, Y., et al. (2019). Simultaneous fermentation and hydrolysis to extract chitin from crayfish shell waste. Int. J. Bio. Macromol. 123, 420–426. doi: 10.1016/j.ijbiomac.2018.11.088
Esakkiraj, P., Austin, J. D., Palavesam, A., and Immanuel, G. (2010). Media preparation using tuna-processing wastes for improved lipase production by shrimp gut isolate Staphylococcus epidermidis CMST Pi 2. Appl. Biochem. Biotechnol. 160, 1254–1265. doi: 10.1007/s12010-009-8632-x
Etemadian, Y., Ghaemi, G., Shaviklo, A. R., Pourashouri, P., Sadeghi Mahoonak, A. R., and Rafipour, F. (2021). Development of animal/ plant-based protein hydrolysate and its application in food, feed and nutraceutical industries: state of the art. J. Clean. Prod. 278, 123219. doi: 10.1016/j.jclepro.2020.123219
Fadhil, A. B., Ahmed, A. L., and Salih, H. A. (2017). Production of liquid fuels and activated carbons from fish waste. Fuel 187, 435–447. doi: 10.1016/j.fuel.2016.09.064
Falch, E., Rustad, T., and Aursand, M. (2006). By-products from gadiform species as raw material for production of marine lipids as ingredients in food or feed. Proc. Biochem. 41:666. doi: 10.1016/j.procbio.2005.08.015
FAO. (2020). The State of World Fisheries and Aquaculture: Sustainability in Action. Rome: Food and Agriculture Organization of the United Nations.
Fernandes, P. (2016). Enzymes in fish and seafood processing. Front. Bioeng. Biotech. 4:59. doi: 10.3389/fbioe.2016.00059
Fiori, L., Volpe, M., Lucian, M., Anesi, A., Manfrini, M., and Guella, G. (2017). From fish waste to omega-3 concentrates in a biorefinery concept. Waste Biomass Valor. 8, 2609–2620. doi: 10.1007/s12649-017-9893-1
Fluence. (2019). Waste-to-Energy Technology Helps Fish Processor Save on Operating Costs. Available online at: https://www.fluencecorp.com/case/waste-to-energy-for-fish-processing-plant/ (accessed June 8, 2020).
Forghani, R., Bordes, R., Strom, A., and Undeland, I. (2020). Recovery of a protein-rich biomass from shrimp (Pandalus borealis) boiling water: a colloidal study. Food Chem. 302:125299. doi: 10.1016/j.foodchem.2019.125299
Freitas, A. C., Rodrigues, D., Rocha-Santos, T. A. P., Gomes, A. M. P., and Duarte, A. C. (2012). Marine biotechnology advances towards applications in new functional foods. Biotechnol. Adv. 30, 1506–1515. doi: 10.1016/j.biotechadv.2012.03.006
Fu, G., Gao, Y., Jin, Y., and Ma, M. (2020). Bioconversion of chitin waste using a cold-adapted chitinase to produce chitin oligosaccharides. LWT 133:109863. doi: 10.1016/j.lwt.2020.109863
Gao, F., Peng, Y.-Y., Li, C., Yang, G.-J., Deng, Y.-B., Xu, B., et al. (2018). Simultaneous nutrient removal and biomass/lipid production by Chlorella sp. in seafood processing wastewater. Sci. Total Environ. 105, 943–953. doi: 10.1016/j.scitotenv.2018.05.380
GFI (2020). Fermentation: State of the Industry Report, The Good Food Institute, 2020. Available online at: https://www.gfi.org/files/fermentation/INN-Fermentation-SOTIR-2020-0910.pdf (accessed January 4, 2021).
Ghorbel-Bellaaj, O., Hmidet, N., Hachicha, K. J. R., and Nasri, M. (2011). Shrimp waste fermentation with Pseudomonas aeruginosa A2: optimization of chitin extraction conditions through Plackett–Burman and response surface methodology approaches. Int. J. Biol. Macromol. 48, 596–602. doi: 10.1016/j.ijbiomac.2011.01.024
Gifuni, I., Pollio, A., Safi, C., Marzocchella, A., and Olivieri, G. (2019). Current bottlenecks and challenges of the microalgal bio-refinery. Trends Biotechnol. 37, 242–252. doi: 10.1016/j.tibtech.2018.09.006
Gonzalez, J. F. (1995). “Wastewater treatment in the fishery industry,” in FAO Fisheries Technical Paper. No. 355 (Rome: Food and Agriculture Organization of the United Nations), 52.
Gringer, N., Svendsen, T., Yuan, L., Hosseini, S. V., Baron, C. P., and Undeland, I. (2015). Quantification of biomolecules in herring (Clupea harengus) industry processing waters and their recovery using electroflocculation and ultrafiltration. Food Bioprod. Proc. 96, 198–210. doi: 10.1016/j.fbp.2015.08.002
Gustavsson, J., Cederberg, C., Sonesson, U., van Otterdijk, R., and Meybeck, A. (2011). Global Food Losses and Food Waste: Extent Causes and Prevention. Rome: Food and Agriculture Organization.
Hall, S. J., Delaporte, A., Phillips, M. J., Beveridge, M., and O'Keefe, M. (2011). “Blue frontiers,” in Managing the Environmental Cost of Aquaculture (Penang: The World Fish Center), 92.
Hamdi, M., Hammami, A., Hajji, S., Jidi, M., Nasri, M., and Nasri, R. (2017). Chitin extraction from blue crab (Portunus segnis) and shrimp (Penaeus kerathurus) shells using digestive alkaline proteases from P. segnis viscera. Int. J. Biol. Macromol. 101, 455–463. doi: 10.1016/j.ijbiomac.2017.02.103
Hayashi, Y. (2020). “Fish collagen: characteristics and applications” in Encyclopedia of Marine Biotechnology, ed S. K. Kim (Betreiber: Wiley-VCH GmbH), 1127–1132. doi: 10.1002/9781119143802.ch45
Hena, V., Joseph, I., and Raj, R. P. (2009). Biotransformation of tuna waste by co-fermentation into an aquafeed ingredient. Aquacul. Res. 40, 1047–1053. doi: 10.1111/j.1365-2109.2009.02197.x
Henchion, M., Hayes, M., Mullen, A. M., Fenelon, M., and Tiwari, B. (2017). Future protein supply and demand: strategies and factors influencing a sustainable equilibrium. Foods 6:53. doi: 10.3390/foods6070053
Heu, M. S., Kim, J. S., and Shahidi, F. (2003). Components and nutritional quality of shrimp processing by-products. Food Chem. 82, 235–242. doi: 10.1016/S0308-8146(02)00519-8
Holanda, D. e, H. D., and Netto, F. M. (2006). Recovery of components from shrimp (Xiphopenaeus kroyeri) processing waste by enzymatic hydrolysis. J. Food Sci. 71, C298–C303. doi: 10.1111/j.1750-3841.2006.00040.x
Huang, Y., Ruan, G., Qin, Z., Li, H., and Zheng, Y. (2017). Antioxidant activity measurement and potential antioxidant peptides exploration from hydrolysates of novel continuous microwave-assisted enzymolysis of the Scomberomorus niphonius protein. Food Chem 223, 89–95. doi: 10.1016/j.foodchem.2016.12.026
Hülsey, M. J. (2018). Shell bio-refinery: a comprehensive introduction. Green Energy Environ. 3, 318–327. doi: 10.1016/j.gee.2018.07.007
Hultin, H. O., Kristinsson, H. G., Lanier, T. C., and Park, J. W. (2005). “Process for recovery of functional proteins by pH shifts” in Surimi and Surimi Seafood, ed J. W. Park, (Boca Raton, FL: CRC Press), 107–139,. doi: 10.1201/9781420028041.ch3
Islam, M. D., S., Khan, S., and Tanaka, M. (2004). Waste loading in shrimp and fish processing effluents: potential source of hazards to the coastal and near-shore environments. Mar. Pol. Bull 49, 103–110. doi: 10.1016/j.marpolbul.2004.01.018
Ivanovs, K. and Blumberga, D. (2017). Extraction of fish oil using green extraction methods: A short review. Energy Procedia 128, 477–483. doi: 10.1016/j.egypro.2017.09.033
James, D. (2013). “Risks and benefits of seafood consumption,” in Globefish Research Programme 108 (Rome: FAO), 28.
Jamieson, B. L., Gagnon, G. A., and Gonçalves, A. A. (2017). Physicochemical characterization of Atlantic Canadian seafood processing plant effluent. Mar. Poll. Bull. 116, 137–142. doi: 10.1016/j.marpolbul.2016.12.071
Jayasinghe, P., and Hawboldt, K. (2012). A review of bio-oils from waste biomass: focus on fish processing waste. Renew. Sustain. Energy Rev. 16, 798–821. doi: 10.1016/j.rser.2011.09.005
Jehlee, A., Khongkliang, P., and Thong, S. O. (2017). Biogas production from Chlorella sp. TISTR 8411 biomass cultivated on biogas effluent of seafood processing wastewater. Energy Proc. 138, 853–857. doi: 10.1016/j.egypro.2017.10.095
Jung, W. J., Jo, G. H., Kuk, J. H., Kim, Y. J., Oh, K. T., and Park, R. D. (2007). Production of chitin from red crab shell waste by successive fermentation with Lactobacillus paracasei KCTC-3074 and Serratia marcescens FS-3. Carb. Polym. 68, 746–750. doi: 10.1016/j.carbpol.2006.08.011
Jung, W. K., Park, P. J., Byun, H. G., Moon, S. H., and Kim, S. K. (2005). Preparation of hoki (Johnius belengerii) bone oligophosphopeptide with a high affinity to calcium by carnivorous intestine crude proteinase. Food Chem. 91, 333–340. doi: 10.1016/j.foodchem.2004.06.016
Kara, K., Ouanji, F., Mostapha, L. E., and Mahi, M. E. (2018). Biodiesel production from waste fish oil with high free fatty acid content from Moroccan fish-processing industries. Egyptian J. Petroleum. 27, 249–255. doi: 10.1016/j.ejpe.2017.07.010
Karim, A. A., and Bhat, R. (2009). Fish gelatin: properties, challenges, and prospects as an alternative to mammalian gelatins. Food Hydrocoll. 23, 563–576. doi: 10.1016/j.foodhyd.2008.07.002
Kaur, S., and Dhillon, G. S. (2015). Recent trends in biological extraction of chitin from marine shell wastes: a review. Crit. Rev. Biotechnol. 35, 44–61. doi: 10.3109/07388551.2013.798256
Khiari, Z., Kaluthota, S., and Savidov, N. (2019). Aerobic bioconversion of aquaculture solid waste into liquid fertilizer: effects of bioprocess parameters on kinetics of nitrogen. Aquaculture 500, 492–499. doi: 10.1016/j.aquaculture.2018.10.059
Khoo, K. S., Lee, S. Y., Ooi, C. W., Fu, X., Miao, X., Ling, T. C., et al. (2019). Recent advances in Bio-refinery of astaxanthin from Haematococcus pluvialis. Bioresour. Technol. 288:121606. doi: 10.1016/j.biortech.2019.121606
Kim, S. K., and Senevirathne, M. (2011). Membrane bioreactor technology for the development of functional materials from sea-food processing discards and their potential health benefits. Membranes 1, 327–344. doi: 10.3390/membranes1040327
Klomklao, S., Benjaku, S., Visessanguan, W., Kishimura, H., and Simspon, B. K. (2009). Extraction of carotenoprotein from black tiger shrimp shells with the aid of bluefish trypsin. J. Food Biochem. 33, 201–217. doi: 10.1111/j.1745-4514.2009.00213.x
Koyande, A. K., Show, P.-L., Guo, R., Tang, B., Ogino, C., and Chang, J. S. (2019). Bio-processing of algal bio-refinery: a review on current advances and future perspectives. Bioengineered 10, 574–592. doi: 10.1080/21655979.2019.1679697
Kuo, C. H., Chen, C. C., and Chiang, B. H. (2004). Process characteristics of hydrolysis of chitosan in a continuous enzymatic membrane reactor, J. Food Sci. 69, 332–337. doi: 10.1111/j.1365-2621.2004.tb13638.x
Lafarga, T., and Hayes, M. (2017). Bioactive protein hydrolyzates in the functional food ingredient industry: overcoming current challenges. Food Rev. Int. 33, 217–246. doi: 10.1080/87559129.2016.1175013
Liaset, B., Julshamn, K., and Eape, M. (2003). Chemical composition and theoretical nutrition of the processed fractions from enzyme hydrolysis of salmon with Protamex™. Process Biochem. 38, 1747–1759. doi: 10.1016/S0032-9592(02)00251-0
Linder, M., Fanni, J., and Parmentier, M. (2005). Proteolytic extraction of salmon oil and PUFA Concentration by lipases. Mar. Biotechnol. 7, 70–76. doi: 10.1007/s10126-004-0149-2
Lopes, C., Antelo, L. T., Franco-Uría, A., Alonso, A. A., and Pérez-Martín, R. (2018). Chitin production from crustacean biomass: Sustainability assessment of chemical and enzymatic processes. J. Cleaner Prod. 172, 4140–4151. doi: 10.1016/j.jclepro.2017.01.082
López-Pedrouso, M., Lorenzo, J. M., Cantalapiedra, J., Zapata, C., Franco, J. M., and Franco, D. (2020). Aquaculture and by-products: challenges and opportunities in the use of alternative protein sources and bioactive compounds. Adv. Food Nutr. Res. 92, 127–185. doi: 10.1016/bs.afnr.2019.11.001
Love, D. C., Fry, J. P., Milli, M. C., and Neff, R. A. (2015). Wasted seafood in the United States: quantifying loss from production to consumption and moving toward solutions. Global Environ. Change 35, 116–124. doi: 10.1016/j.gloenvcha.2015.08.013
Mahmoud, N., Ghaly, A., and Arab, F. (2007). Unconventional approach for demineralization of deproteinized crustacean shells for chitin production. Am. J. Biochem. Biotechnol. 3, 1–9. doi: 10.3844/ajbbsp.2007.1.9
Manni, L., Ghorbel-Bellaaj, O., Jellouli, K., Younes, I., and Nasri, M. (2010). Extraction and characterization of chitin, chitosan, and protein hydrolysates prepared from shrimp waste by treatment with crude protease from Bacillus cereus SV1. Appl Biochem. Biotechnol. 162, 345–357. doi: 10.1007/s12010-009-8846-y
Mao, X., Guo, N., Sun, J., and Xue, C. (2017). Comprehensive utilization of shrimp waste based on biotechnological methods: a review. J. Cleaner Prod. 143, 814–823. doi: 10.1016/j.jclepro.2016.12.042
Marti-Quijal, F. J., Remize, F., Meca, G., Ferrer, F., Ruiz, M. J., and Barba, M. J. (2020). Fermentation in fish and by-products processing: An overview of current research and future prospects. Curr. Opin. Food Sci. 31, 9–16. doi: 10.1016/j.cofs.2019.08.001
Mitra, M., and Mishra, S. (2019). Multiproduct Bio-refinery from Arthrospira spp. towards zero waste: Current status and future trends. Bioresource Technol. 291:121928. doi: 10.1016/j.biortech.2019.121928
Mohan, S., d, V., Nikhil, G. N., Chiranjeevi, P., Reddy, C. N., Rohit, M. V., et al. (2016). Waste bio-refinery models towards sustainable circular bioeconomy: Critical review and future perspectives. Bioresource Technol. 215, 2–12. doi: 10.1016/j.biortech.2016.03.130
Moore, S. R. G. P., and McNeill, G. P. (1996). Production of triglycerides enriched in long-chain n-3 polyunsaturated fatty acids from fish oil. J. Am. Oil Chem. Soc 73, 1409–1414. doi: 10.1007/BF02523504
Muffler, K., and Ulber, R. (2005). “Downstream processing in marine biotechnology,” in Marine Biotechnology I. I. Advances in Biochemical Engineering/Biotechnology, eds R. Ulber and Y. Le Gal, (Heidelberg, Berlin: Springer) 97, 63–103. doi: 10.1007/b135823
Murthy, L. N., Phadke, G. G., Unnikrishnan, P., Annamalai, J., Joshy, C. G., Zynudheen, A., et al. (2018). Valorization of fish viscera for crude proteases production and its use in bioactive protein hydrolysate preparation. Waste Biomass Valor. 10, 1735–1746. doi: 10.1007/s12649-017-9962-5
Muxika, A., Etxabide, A., Uranga, J., Guerrero, P., and de la Caba, K. (2017). Chitosan as a bioactive polymer: processing, properties and applications. Int. J. Biol. Macromol. 105, 1358–1368. doi: 10.1016/j.ijbiomac.2017.07.087
Nawaz, A., Li, E., Irshad, S., Xiong, Z., Xiong, H., Shahbaz, H. M., et al. (2020). Valorization of fisheries by-products: challenges and technical concerns to food industry. Trends Food Sci. Technol. 99, 34–43. doi: 10.1016/j.tifs.2020.02.022
Ngo, D. H., Vo, T. S., Ngo, D. N., Thuong, N. T. L., and Kim, S. K. (2020). “Chitosan and its derivatives as potential biomaterials” in Encyclopedia of Marine Biotechnology, ed S. K. Kim (Betreiber: Wiley-VCH GmbH). doi: 10.1002/9781119143802.ch114
Nguyen, T. T., Barber, A. R., Corbin, K., and Zhang, W. (2017). Lobster processing by-products as valuable bioresource of marine functional ingredients, nutraceuticals, and pharmaceuticals. Bioresour. Bioprocess. 4, 27–46. doi: 10.1186/s40643-017-0157-5
Nigam, S., Sinha, S., Srivastava, A., and Srivastava, A. (2020). “Cultivation and production techniques of marine algae,” in Encyclopedia of Marine Biotechnology, ed S. K. Kim (Betreiber: Wiley-VCH GmbH), 327–340. doi: 10.1002/9781119143802.ch9
Okada, T., and Morrissey, M. T. (2007). Recovery and characterization of sardine oil extracted by pH adjustment. J. Agri. Food Chem. 55, 1808–1813. doi: 10.1021/jf062942e
Pal, G. K., and Suresh, P. V. (2016). Sustainable valorisation of seafood by-products: recovery of collagen and development of collagen-based novel functional food ingredients. Inn. Food Sci. Emerg. Technol. 37, 201–215. doi: 10.1016/j.ifset.2016.03.015
Pallela, R., Venkatesan, J., and Kim, S. K. (2011). Polymer assisted isolation of hydroxyapatite from Thunnus obesus bone, Ceramics Int. 37, 3489-3497.
Park, J. W. (ed). (2013). Surimi and Surimi Seafood. 3rd ed. Boca Raton, Fl., CRC Press. doi: 10.1201/b16009
Parkavi, K., Raja, R., Arunkumar, K., Coehlo, A., Hemaiswarya, S., and Carvallo, I. S. (2020). “Recent insights into algal biotechnology: An update using text mining tool,” in Encyclopedia of Marine Biotechnology, ed S. K. Kim (Betreiber: Wiley-VCH GmbH), 569–584. doi: 10.1002/9781119143802.ch19
Pleissner, D., and Lin, C. S. K. (2013). Valorisation of food waste in biotechnological processes. Sustain. Chem. Process 1:21. doi: 10.1186/2043-7129-1-21
Prameela, K., Venkatesh, K., Immandi, S.B., Kasturi, A. P. K., Rama Krishna, C., and Murali Mohan, C. (2017). Next generation nutraceutical from shrimp waste: The convergence of applications with extraction methods. Food Chem. 237, 121–132. doi: 10.1016/j.foodchem.2017.05.097
Puyol, D., Batstone, D. J., Hulsen, T., Astals, S., peces, M., and Krömer, J. O. (2017). Resource recovery from wastewater by biological technologies: Opportunities, challenges, and prospects. Front. Microbiol. 7:2106. doi: 10.3389/fmicb.2016.02106
Rai, A. K., Swapna, H. C., Bhaskar, N., Halami, P. M., and Sachindra, N. M. (2010). Effect of fermentation ensilaging on recovery of oil from fresh water fish viscera. Enz. Microb. Biotechnol. 46, 9–13. doi: 10.1016/j.enzmictec.2009.09.007
Rashid, H. A., Jung, H. Y., and Kim, J. K. (2018). Enhanced reutilization value of shrimp-shell waste via fed-batch biodegradation with higher production of reducing sugar, antioxidant, and DNA protective compounds. Fish Aquatic Sci. 21:33. doi: 10.1186/s41240-018-0109-9
Ratky, L., and Zamazal, P. (2020). Economic feasibility and sensitivity analysis of fish waste processing bio-refinery. J. Clean. Prod. 243:118677. doi: 10.1016/j.jclepro.2019.118677
Ren, X., Ma, L., Chu, J., Wang, Y., Zhuang, Y. P., and Zhang, S. (2012). Optimization of enzymatic hydrolysis of channel catfish bones for preparing antimicrobial agents. J. Aquatic Food Prod.Technol. 21, 99–110. doi: 10.1080/10498850.2011.586136
Routray, W., Dave, D., Cheema, S. K., Ramakrishnan, V. V., and Pohling, J. I. (2019). Bio-refinery approach and environment-friendly extraction for sustainable production of astaxanthin from marine wastes. Crit. Rev. Biotechnol. 39, 469–488. doi: 10.1080/07388551.2019.1573798
Sachindra, N. M., n and Mahendrakar, N. S. (2011). Effect of protease treatment on oil extractability of carotenoids from shrimp waste. J. Aquatic Food Prod. Technol. 20, 22–31. doi: 10.1080/10498850.2010.526754
Sahu, B. B., Barik, N. K., Paikaray, A., Agnibesh, A., and Jayasankar, M. P. (2016). Fish waste bio-refinery products: its application in organic farming. J. Env. Agri. Biotechnol. 1, 837–843,. doi: 10.22161/ijeab/1.4.30
Samant, S., Naik, M. M., Vaingankar, D. C., Mujawar, S. Y., Parab, P., and Meena, S. N. (2019). Biodegradation of seafood waste by seaweed-associated bacteria and application of seafood waste for ethanol production. Adv. Biol. Sci. Res. 149–159. doi: 10.1016/B978-0-12-817497-5.00010-0
Sanchart, C., Watthanasakphuban, N., Boonseng, B., et al. (2018). Tuna condensate as a promising low-cost substrate for glutamic acid and GABA formation using Candida rugosa and Lactobacillus futsaii. Process Biochem. 70, 29–35. doi: 10.1016/j.procbio.2018.04.013
Sasidharan, A., and Venugopal, V. (2019). Proteins and co-products from seafood processing discards: their recovery, functional properties and applications. Waste Biomass Valor. 11, 5647–5663. doi: 10.1007/s12649-019-00812-9
Shah, M. M. R., Lian, Y., Cheng, J. J., and Daroch, M. (2016). Astaxanthin-producing green microalga Haematococcus pluvialis: from single cell to high value commercial products. Front Plant Sci. 28:531. doi: 10.3389/fpls.2016.00531
Shahid, A., Malik, S., Zhu, H., Xu, J., Nawaz, M. Z., Nawaz, Z., et al. (2020). Cultivating microalgae in wastewater for biomass production, pollutant removal, and atmospheric carbon mitigation; a review. Sci. Tot. Env. 704:135303. doi: 10.1016/j.scitotenv.2019.135303
Shahidi, F., Varatharajan, V., Peng, H., and Senadheera, R. (2019). Utilization of marine by-products for the recovery of value-added products. J. Food Bioact. 6, 10–61. doi: 10.31665/JFB.2019.5183
Shan, D., Zhang, Y. X., Lu, T. T., Cao, D. X., and Chen, J. D. (2011), Shrimp waste fermentation using symbiotic lactic acid bacteria, Adv. Mater. Sci. 194–196, 2156–2163. doi: 10.4028/www.scientific.net/AMR.194-196.2156
Sharma, N., and Sharma, P. (2017). Industrial and biotechnological applications of algae: a review. J Adv Plant Biol.1:1–26. doi: 10.14302/issn.2638-4469.japb-17-1534
Shuba, E. S., and Kifle, D. (2018). Microalgae to biofuels: promising alternative and renewable energy, review. Renew. Sustain. Energy Rev. 81, 743–755. doi: 10.1016/j.rser.2017.08.042
Sieber, V., Hofer, M., Brück, W. M., Garbe, D., Brück, T., and Lynch, C. A. (2018). “ChiBio: an integrated bio-refinery for processing chitin-rich bio-waste to specialty chemicals,” in Grand Challenges in Marine Biotechnology Grand Challenges in Biology and Biotechnology, eds P. Rampelotto and A. Trincone (Berlin; Heidelberg; Cham: Springer), 555–578. doi: 10.1007/978-3-319-69075-9_14
Sila, A., Nassri, M., and Bougatef, A. (2012). Isolation and characterisation of carotenoproteins from deep-water pink shrimp processing waste. Int. J. Biol. Macromol. 51, 953–959. doi: 10.1016/j.ijbiomac.2012.07.011
Smárason, B. O., Alriksson, B., and Jóhannsson, R. (2019). Safe and sustainable protein sources from the forest industry – The case of fish feed. Trends Food Sci. Technol. 84, 12–14. doi: 10.1016/j.tifs.2018.03.005
Song, Z., Liu, H., Chen, L., Chen, L., Zhou, C., Hong, P., and Deng, C. (2021). Characterization and comparison of collagen extracted from the skin of the Nile tilapia by fermentation and chemical pretreatment. Food Chem. 340. doi: 10.1016/j.foodchem.2020.128139
Sorokulova, I., Krumnow, A., Globa, L., and Vodyanoy, V. (2009). Efficient decomposition of shrimp shell waste using Bacillus cereus and Exiguobacterium acetylicum. J. Ind. Microbiol. Biotechnol. 36, 1123–1126. doi: 10.1007/s10295-009-0587-y
Sosa-Hernández, J. E, Escobedo-Avellaneda, Z, Iqbal, H. M. N, and Welti-Chanes, J. (2018). State-of-the-art extraction methodologies for bioactive compounds from algal biome to meet bio-economy challenges and opportunities. Molecules. 23:2953. doi: 10.3390/molecules23112953
Sowmya, R., and Sachindra, N. M. (2015). “Carotenoids from fishery resources” in Fish processing byproducts: quality assessment and applications, eds N. M. Sachindra and N. S.Mahendraka. (Houston, TX, Studium Press), 273–329.
Steinke, M., and Barjenbruch, M. (2010). Full-scale experiences of nitrogen removal of fish-processing wastewater with flotation and anoxic-aerobic activated sludge system. Water Sci. Technol. 61, 2227–2233. doi: 10.2166/wst.2010.984
Stengel, D. B., and Connan, S. (2015). “Marine Algae: a Source of biomass for biotechnological applications,” in Natural Products from Marine Algae. Methods in Molecular Biology, eds D. Stengel and S. Connan (New York, NY, Humana Press), 1–37. doi: 10.1007/978-1-4939-2684-8_1
Suresh, P. V. (2012). Biodegradation of shrimp processing bio-waste and concomitant production of chitinase enzyme and N-acetyle-D-glucosamine by marine bacteria: production and process optimization. World J. Microbiol. Biotechnol. 28, 2945–2962. doi: 10.1007/s11274-012-1106-2
Tang, S., Dong, S., Chen, M., Gao, R., Chen, S., Zhao, Y., Liu, Z., and Sun, B. (2018). Preparation of a fermentation solution of grass fish bones and its calcium bioavailability in rats. Food Funct. 9, 4135–4142. doi: 10.1039/C8FO00674A
Tonon, R. V., dos Santos, R. A., Couto, C. C., Mellinger-Silva, C., and Band Cabral, L. M. (2016). Coupling of ultrafiltration and enzymatic hydrolysis aiming at valorizing shrimp wastewater. Food Chem. 198, 20–27. doi: 10.1016/j.foodchem.2015.11.094
Tremblay, A., Corcuff, R., Goulet, C., Godfroy, S. B., Doyen, A., and Beaulieu, L. (2020). Valorization of snow crab (Chionoecetes opilio) cooking effluents for food applications. J. Sci. Food Agri. 100, 384–393. doi: 10.1002/jsfa.10066
Uhlmann, S. S., Ulrich, C., and Kennelly, S. J. (2019). The European Landing Obligation Reducing Discards in Complex, Multi-SPECIES and Multi-Jurisdictional Fisheries. Berlin, Germany, Springer Open. doi: 10.1007/978-3-030-03308-8
US EPA. (2017). United States Environmental Protection Agency, Ocean Disposal of Fish Wastes. Available online at: https://www.epa.gov/ocean-dumping/ocean-disposal-fish-wastes, accessed July 12, 2020
Vázquez, J., Patrícia Ramos, R., Mirón, J., Valcarcel, J., and Sotelo, C. G. (2017). Production of chitin from Penaeus vannamei by-products to pilot plant scale using a combination of enzymatic chemical processes and subsequent optimization of the chemical production of chitosan by response surface methodology. Mar Drugs 15:180. doi: 10.3390/md15060180
Vázquez, J. A., Durán, A. I., Menduíña, A., Nogueira, M., Gomes, A. M., Antunes, J., et al. (2020). Bioconversion of fish discards through the production of lactic acid bacteria and metabolites: sustainable application of fish peptones in nutritive fermentation media. Foods 9:1239. doi: 10.3390/foods9091239
Vázquez, J. A., Meduíña, A., Durán, A. I., Nogueira, M., Fernández-Compás, A., and Perez-Martin, R. I. (2019). Production of valuable compounds and bioactive metabolites from by-products of fish discards using chemical processing, enzymatic hydrolysis, and bacterial fermentation. Mar. Drugs 17, 139. doi: 10.3390/md17030139
Vázquez, J. A., Rodríguez-Amado, I., Montemayor, M. I., Fraguas, J., González Mdel, P., and Murado, M. A. (2013). Chondroitin sulfate, hyaluronic acid chitin/chitosan production using marine discard sources: characteristics, applications and eco-friendly processes: a review. Marine Drugs 11, 747–777. doi: 10.3390/md11030747
Venugopal, V. (1997). Functionality and potential applications of thermostable water dispersions of fish meat. Trends Food Sci. Technol. 8, 271–276. doi: 10.1016/S0924-2244(97)01038-8
Venugopal, V. (2006). Seafood Processing: Adding Value Through Quick Freezing, Retortable Packaging, and Cook-Chilling. Boca Raton, FL: CRC Press.
Venugopal, V. (2009). Marine Products for Healthcare: Functional and Bioactive Compounds From the Ocean. Boca Raton, FL: CRC Press. doi: 10.1201/9781420052640
Venugopal, V. (2016): Enzymes from seafood processing waste and their applications in seafood processing. Adv.Food Nutr. Res. 78, 47–69. doi: 10.1016/bs.afnr.2016.06.004.
Venugopal, V. (2017). Weak Acid-induced gel from shark meat and its food applications. EC Nutrition 10, 87–101
Venugopal, V. (2018). “Nutrients and nutraceuticals from seafood” in Bioactive Molecules in Food, Reference Series in Phytochemistry, eds AG J.-M. Mérillon, and K.G. Ramawat (Berlin, Heidelberg: Springer), 1–45.
Venugopal, V., and Gopakumar, K. (2017). Shellfish: nutritive value, health benefits, and consumer safety. Comp. Rev. Food Sci. Food Saf. 16, 1219–1242. doi: 10.1111/1541-4337.12312
Venugopal, V., and Lele, S. S. (2014). “Nutraceuticals and bioactive compounds from seafood processing waste”, in Handbook of Marine Biotechnology, ed. S.K.Kim (Berlin, Heidelberg: Springer), 1405–1425,. doi: 10.1007/978-3-642-53971-8_65
Venugopal, V. and Sasidharan, A. (2021). Seafood industry effluents: Environmental hazards, treatment and resource recovery. J. Env. Chem. Eng. 2021:104758. doi: 10.1016/j.jece.2020.104758
Venugopal, V., and Shahidi, F. (1998). Traditional methods to process underutilized fish species for human consumption. Food Rev. Int. 14, 35–97. doi: 10.1080/87559129809541149
Vidanarachchi, J. K., Ranadheera, C. S., Wijerathne, T. D., Udayangani, R. M. C., Himali, S. M. C., and Pickova, J. (2014). “Applications of seafood by-products in the food industry and human nutrition,” in Seafood Processing By-Products, ed. S. K. Kim (New York, NY, Springer), 463–528. doi: 10.1007/978-1-4614-9590-1_23
Vijaykrishnaraj, M., and Prabhasankar, P. (2015). Marine protein hydrolyzates: their present and future perspectives in Food Chemistry – a review. RSC Adv. 5, 34864–34867. doi: 10.1039/C4RA17205A
Wang, C. H., Doan, C. T., Nguyen, A. D., and Wang, S. L. (2019). Reclamation of fishery processing waste: a mini-review. Molecules 24:2234. doi: 10.3390/molecules24122234
Wang, S. L., Nguyen, V. B., Doan, C. T., Tran, T. N., Nguyen, M. T., and Nguyen, A. D. (2020). Production and potential applications of bioconversion of chitin and protein-containing fishery byproducts into prodigiosin: a review Molecules 25:2744. doi: 10.3390/molecules25122744
Ximenes, I., Hissa, J. D., Ribeiro, D. L., Rocha, M., Oliveira, E. G., and Melo, V. M. M. (2019). Sustainable recovery of protein-rich liquor from shrimp farming waste by lactic acid fermentation for application in tilapia feed, Brazilian. J. Microbiol. 50, 195–203. doi: 10.1007/s42770-018-0024-3
Xu, io, F., Li, Y., Ge, X., Yang, L., and Li, Y. (2018). Anaerobic digestion of food waste–challenges and opportunities. Bioresour Technol. 247, 1047–1058. doi: 10.1016/j.biortech.2017.09.020
Yadav, M., Goswami, P., Paritosh, K., Kumar, M., Pareekh, N., and Vivekanand, V. (2019). Seafood waste: a source for preparation of commercially employable chitin/chitosan materials. Bioresour. Bioprocess. 6:8. doi: 10.1186/s40643-019-0243-y
Yan, N., and Chen, X. (2015). Sustainability: don't waste seafood waste. Nature 524, 155–157. doi: 10.1038/524155a
Yang, H., and Yan, N. (2018). “Transformation of seafood wastes into chemicals and materials,” in Encyclopedia of Sustainability Science and Technology, ed R. Meyers (New York, NY: Springer), 555–558. doi: 10.1007/978-1-4939-2493-6_1012-1
Younes, I., Hajji, S., Rinaudo, M., Chaabouni, M., Jellouli, K., and Nasri, M. (2016). Optimization of proteins and minerals removal from shrimp shells to produce highly acetylated chitin. Int. J. Biol. Macromol. 84, 246–253. doi: 10.1016/j.ijbiomac.2015.08.034
Yu, P., and Gu, H. (2013). Bioactive substances from marine fishes, shrimps and algae and their functions: present and future. Crit. Rev. Food Sci. Nutr. 55, 1114–1136. doi: 10.1080/10408398.2012.686933
Zhang, A., Gao, C., Wang, J., Chen, K., and Ouyvang, P. (2016). An efficient enzymatic production of N-acetyl-D-glucosamine from crude chitin powders. Green Chem. 18, 2147–2154. doi: 10.1039/C5GC02242H
Keywords: seafood by-products, seafood waste treatment, valorization, bioconversions, bio-refinery, marine biotechnology
Citation: Venugopal V (2021) Valorization of Seafood Processing Discards: Bioconversion and Bio-Refinery Approaches. Front. Sustain. Food Syst. 5:611835. doi: 10.3389/fsufs.2021.611835
Received: 29 September 2020; Accepted: 30 March 2021;
Published: 10 June 2021.
Edited by:
Tripti Agarwal, National Institute of Food Technology Entrepreneurship and Management, IndiaReviewed by:
Glaucia Falcao Aragao, Federal University of Santa Catarina, BrazilCopyright © 2021 Venugopal. This is an open-access article distributed under the terms of the Creative Commons Attribution License (CC BY). The use, distribution or reproduction in other forums is permitted, provided the original author(s) and the copyright owner(s) are credited and that the original publication in this journal is cited, in accordance with accepted academic practice. No use, distribution or reproduction is permitted which does not comply with these terms.
*Correspondence: V. Venugopal, dnZlbnVnb3BhbG1lbm9uQGdtYWlsLmNvbQ==
Disclaimer: All claims expressed in this article are solely those of the authors and do not necessarily represent those of their affiliated organizations, or those of the publisher, the editors and the reviewers. Any product that may be evaluated in this article or claim that may be made by its manufacturer is not guaranteed or endorsed by the publisher.
Research integrity at Frontiers
Learn more about the work of our research integrity team to safeguard the quality of each article we publish.