- 1School of Environmental Sciences, University of Guelph, Guelph, ON, Canada
- 2Centre for Nuclear Energy in Agriculture, University of Sāo Paulo, Piracicaba, Brazil
- 3Department of Soil Science, University of Saskatchewan, Saskatoon, SK, Canada
Global population growth poses a threat to food security in an era of increased ecosystem degradation, climate change, soil erosion, and biodiversity loss. In this context, harnessing naturally-occurring processes such as those provided by soil and plant-associated microorganisms presents a promising strategy to reduce dependency on agrochemicals. Biofertilizers are living microbes that enhance plant nutrition by either by mobilizing or increasing nutrient availability in soils. Various microbial taxa including beneficial bacteria and fungi are currently used as biofertilizers, as they successfully colonize the rhizosphere, rhizoplane or root interior. Despite their great potential to improve soil fertility, biofertilizers have yet to replace conventional chemical fertilizers in commercial agriculture. In the last 10 years, multi-omics studies have made a significant step forward in understanding the drivers, roles, processes, and mechanisms in the plant microbiome. However, translating this knowledge on microbiome functions in order to capitalize on plant nutrition in agroecosystems still remains a challenge. Here, we address the key factors limiting successful field applications of biofertilizers and suggest potential solutions based on emerging strategies for product development. Finally, we discuss the importance of biosafety guidelines and propose new avenues of research for biofertilizer development.
Introduction
Soil and plant-associated microbes play a key role in ecosystem functioning by carrying out numerous biogeochemical cycles and organic matter degradation (Paul, 2015). For this reason, biofertilizers (i.e., microbial-based fertilizers) are considered to be crucial components of sustainable agriculture, with long lasting effects on soil fertility (Bargaz et al., 2018; Singh et al., 2019). The term biofertilizer can be defined as formulations comprised of living microbial cells, either a single strain or multiple strains (mixed or consortium), that promote plant growth by increasing nutrient availability and acquisition (Riaz et al., 2020). Nevertheless, the term itself has evolved over the last 30 years receiving many interpretations (El-Ghamry et al., 2018; Macik et al., 2020). As stated by Macik et al. (2020), the greatest misconception occurs when including microbial inoculants with other beneficial applications (e.g., biopesticides and phytostimulators) as biofertilizers. Likewise, plant growth-promoting bacteria or rhizobacteria (PGPB/PGPR) and biofertilizers should not be considered an interchangeable term, since not all PGPB/PGPR are biofertilizers (Riaz et al., 2020). Nonetheless, it is worth mentioning that biofertilizers can also provide other direct and indirect benefits for plant growth, such as phytostimulation, abiotic stress tolerance and biocontrol (Ferreira et al., 2019; Liu et al., 2020; Shirmohammadi et al., 2020).
The commercial history of biofertilizers dates back to 1895 using “Nitragin” by Nobbe and Hiltner with a laboratory culture of Rhizobium sp. (Singh et al., 2019). In the late 1950s, several studies with arbuscular mycorrhizal fungi inoculants reported positive plant growth promotion (PGP) effects through phosphorus (P) uptake (Koide and Mosse, 2004). However, despite their numerous advantages and low cost, the commercialization of biofertilizers is not widespread. The reasons limiting their use are mostly related to inconsistent responses over different soils, crops and environmental conditions, along with practical aspects related to mass production, shelf-life, appropriate recommendations and ease of use for farmers (Debnath et al., 2019).
In the last 10 years, multi-omics technologies enhanced our understanding of the complexity of microbiomes, as they allowed us to better characterize the structure and function of microbial communities (Kaul et al., 2016). These novel approaches are increasingly applied to describe soil microbial communities and their influence on plant nutrient acquisition and other PGP traits (Saad et al., 2020; Tosi et al., 2020a,b). However, they have yet to be successfully applied in the development of novel and improved biofertilizer technologies (Qiu et al., 2019).
In this review, we focus on the direct mechanisms by which microorganisms enhance the availability and acquisition of essential plant nutrients. Subsequently, we assess the current challenges and constraints faced by the implementation of biofertilizers in agriculture, and we discuss emerging strategies for biofertilizer development (e.g., bioprospecting and formulations). Finally, we address the potential risks that biofertilizers pose to human and environmental health, and conclude by highlighting current knowledge gaps and identifying priorities for future research.
An Overview of Biofertilizers: Key Mechanisms of Nutrient Acquisition
Nitrogen: N2-Fixation
Nitrogen (N) is an essential element for life and it is the fourth most abundant element in all living biomass after hydrogen, carbon, and oxygen (Howarth, 2009). For example, N is an essential component of chlorophyll, amino acids, nucleic acids, and the energy transfer molecule adenosine triphosphate (ATP) (Werner and Newton, 2005). One important source of N in soils is organic N which requires microbial mineralization to be converted to plant available inorganic N, a combination of ammonification and nitrification (Paul, 2015). However, the major N reservoir is in the atmosphere as N2, which is not directly used by plants and only becomes available through Biological N2-fixation (BNF) (Figure 1). This is an energy-intensive process by which the enzyme nitrogenase converts atmospheric N2 to ammonia (NH3), which is readily available for assimilation by plants and microbes (Dakora et al., 2008). Nitrogenases can be found in a small and diverse group of microorganisms called diazotrophs (N2-fixing), which includes symbiotic bacteria, and free-living bacteria and archaea (Moreira-Coello et al., 2019). In agriculture, the most studied symbiotic N2-fixing organisms are bacteria known as rhizobia, comprised mostly of the family Rhizobiaceae [i.e., Rhizobium, Bradyrhizobium, Sinorhizobium, Azorhizobium, Mesorhizobium, and Sinorhizobium (Ensifer)] (Shamseldin et al., 2017). Rhizobia can establish symbiotic relationships with legumes (family Fabaceae) by forming nodules on their roots or stems (Masson-Boivin and Sachs, 2018). These nodules provide an advantage for N2-fixation in which nitrogenases are protected in bacteroids from atmospheric O2. The oxygen concentration is an important factor determining the amount of N that is fixed, since oxygen is a negative regulator of nif gene expression and inhibits nitrogenase activity (Glick, 2015). Several rhizobia, such as Rhizobium, Sinorhizobium (Ensifer), and Bradyrhizobium, are commonly used as biofertilizers in agriculture (Carareto Alves et al., 2014). Plants can acquire a significant proportion of their N requirement through associations with the diazotrophs (Dakora et al., 2008). For example, N2-fixation could supply ~20–25% of total N requirement in rice, ~30–50% in wheat and up to 70% in sugarcane (Hurek et al., 2002; Gupta and Paterson, 2006; Santi et al., 2013). Yet, the amount of N provided by BNF will vary depending on the plant species and environmental factors, which will ultimately determine a successful colonization (Parnell et al., 2016), as explained below.
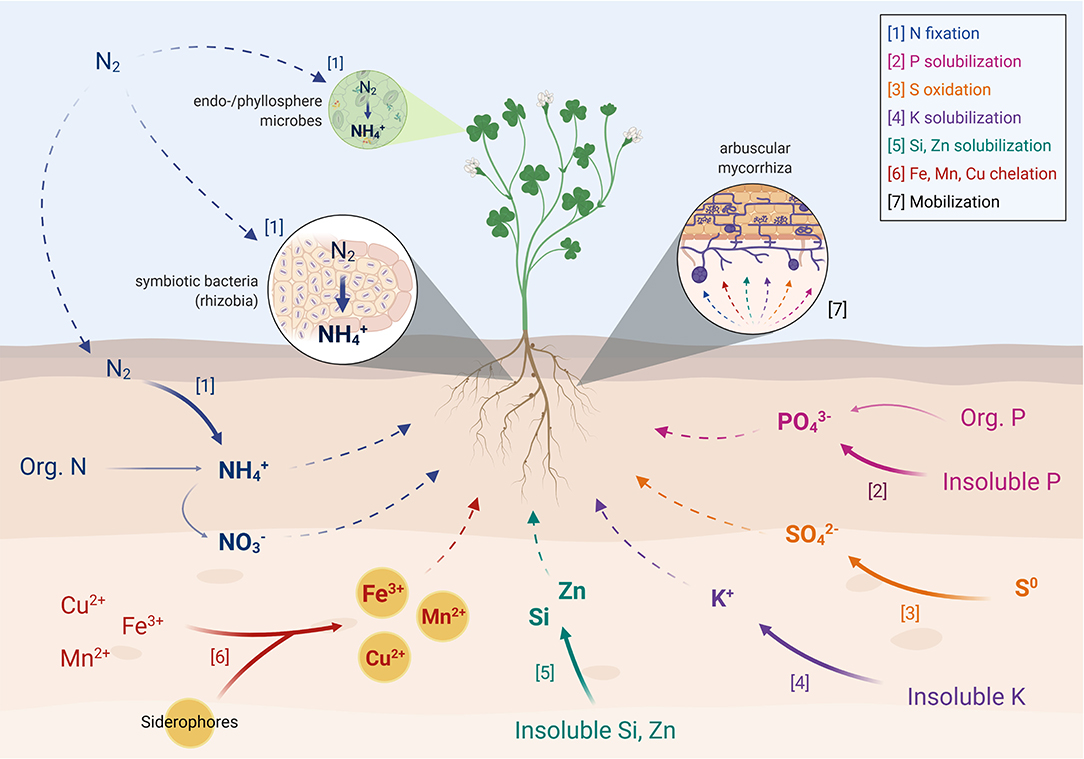
Figure 1. Key microbially-mediated nutrient transformation/acquisition pathways associated with biofertilizers. Full arrows represent microbial transformations whereas dashed arrows represent mobilization/movement of nutrients. Created with BioRender (https://biorender.com/).
In contrast to symbiotic N2-fixing bacteria, several heterotrophic free-living diazotrophic microorganisms such as Azotobacter sp., Azospirillum sp., and cyanobacteria can fix atmospheric N2 in the rhizosphere and bulk soil. Free-living diazotrophs are particularly important for N acquisition in non-legume crops. For example, increased crop yields were observed in cereals (e.g., wheat, rice, and corn) and a variety of other crops such as sunflower, carrot, oak, sugar beet, sugarcane, tomato, eggplant, pepper, and cotton (Garcha and Maan, 2017). Azospirillum species can carry out several PGP functions but are also the most well known free-living diazotrophs, shown to enhance N availability and acquisition in more than 113 plant species (Bashan and De-Bashan, 2010; Pereg et al., 2016; Zeffa et al., 2019).
Phosphorus: Solubilization and Mineralization
Phosphorus is one of the most important plant nutrients that directly or indirectly affects all biological processes. For example, P is key in all major plant metabolic processes such as photosynthesis, energy transfer, signal transduction, biosynthesis of molecules, and respiration. A considerable amount of P is present in soils, in both inorganic and organic forms, but its availability is one of the main factors limiting plant growth in many ecosystems worldwide (Raghothama, 2015). This is because most soil P is in an occluded or insoluble form, and unavailable for plants, which can uptake P from the soil solution as orthophosphate ions H2 and (Soumare et al., 2020). The concentration of soluble phosphates () in the soil solution is generally low, around 0.001–0.4 mg P/L in unfertilized soils, which is equivalent to 0.001–0.01% of total-P (Weihrauch and Opp, 2018). In addition, it is estimated that ~80% of the P applied via fertilization gets quickly fixed into stable forms in the soil, unavailable for plants (Pradhan et al., 2017).
Soil microbes are capable of converting insoluble soil P into plant available form(s) through various mechanisms of solubilization and mineralization (Alori et al., 2017) (Figure 1). Phosphate-solubilizing microbes (PSM) solubilize inorganic P (e.g., tricalcium phosphate, hydroxyapatite, and rock phosphate) via the production and release of different compounds. One mechanism consists in the excretion of organic acids, hydroxyl ions and CO2, which dissolve the insoluble phosphates directly by lowering the soil pH, then leading to ion exchange of by acid ions (Wei et al., 2018). Microbes can also release chelating compounds that capture and mobilize cations from different insoluble phosphates such as Ca+2, Al+3, and Fe+3, resulting in the release of associated soluble phosphates (Riaz et al., 2020). By increasing P bioavailability, PSM reduce the need for mineral P fertilizer inputs, which in excess can lead to negative environmental impacts such as eutrophication of fresh-water bodies. The most studied P solubilizers belong to the genera Pseudomonas, Bacillus, Rhizobium, Enterobacter, Penicillium, and Aspergillus (De Freitas et al., 1997; Anand et al., 2016).
Another important process by which soil microorganisms can increase P bioavailability is by the mineralization of organic phosphate compounds (e.g., inositol hexaphosphate and phytate) (Alori et al., 2017). This process is mediated by phosphatases (e.g., phosphodiesterases and phosphomonoesterases) and phytases that help release phosphate from organic P compounds, which can then be taken up by plants (Pradhan et al., 2017).
Potassium: Solubilization
Potassium (K) is a vital plant macronutrient and a major inorganic cation in the plant cytoplasm, essential for cell constitution and functioning, implicated in photosynthesis, protein synthesis, and many other primary metabolic functions. Potassium is also the second most abundant nutrient in soil after N, and one of the most abundant elements on Earth. However, ~98% of soil K is present in a non-exchangeable form, trapped within crystal structures of the minerals feldspar and mica (e.g., muscovite, biotite). Another 1–2% is adsorbed onto clay particles and organic matter, while only 0.1–0.2% is in the soil solution and directly available for plant uptake (Srivastava et al., 2019). Currently, Canada is the world's largest potash producer with approximately one-third of the world potash reserves located in southern Saskatchewan (Broughton, 2019). However, especially in the Southern Hemisphere, several countries with little or no potash production are highly-dependent on the import of fertilizers and need alternatives to increase soil K availability.
Microorganisms can increase K availability via solubilization, a process that plays a key role in the K cycle by making K available to plants (Sattar et al., 2019; Macik et al., 2020) (Figure 1). Similar to P, the most well-known mechanism of microbial K solubilization involves the synthesis and discharge of organic acids (i.e., tartaric, citric, oxalic, gluconic, lactic, and malic acid) (Sattar et al., 2019). These organic acids lead to the acidification of the surrounding environment and therefore the release (acidolysis) of K+ from minerals (Sattar et al., 2019). Other important K release mechanisms include chelation, and exchange reactions involving organic acids (Sharma et al., 2016). Several groups of soil bacteria (e.g., Bacillus, Rhizobium, Acidithiobacillus, Paenibacillus, Pseudomonas, and Burkholderia) and fungi (Aspergillus, Cladosporium, Macrophomina, Sclerotinia, Trichoderma, Glomus, and Penicillium) can solubilize K minerals (Kour et al., 2020).
Sulfur: Oxidation
Sulfur (S) is an essential nutrient for plant growth, implicated in the conformation of biomolecules such as proteins, glutathione, chloroplast membrane lipids, coenzymes, and vitamins. Most S in soils (~95%) is in an organic form (C-bonded S or sulfate esters), while inorganic forms are less common (5–10%). The most common form of inorganic S is sulfate (), which is readily available for plant uptake and is present either dissolved in the soil solution or adsorbed to soil particles (Scherer, 2009). In the last decades, S deficiency in agricultural soils increased on a worldwide scale, likely as a consequence of the decline in atmospheric deposition of S due to the reductions in SO2 emissions and the use of low-S fertilizers (Ercoli et al., 2012). Consequently, S fertilizers have received increasing attention, with elemental S (S0) as the most common form of S fertilizer. Elemental S constitutes a highly concentrated form of S but needs to be oxidized to in order to become available to plants (Scherer, 2009).
The application of S-oxidizing microbes can help by both optimizing S fertilization and minimizing environmental risks caused by S leaching (Figure 1). Sulfur-oxidizing bacteria can use S0 as an energy source, releasing plant-available sulfate. Hence, their inoculation together with S0 fertilizers can speed up its conversion to sulfates, potentially leading to higher crop yields (Pujar et al., 2014). Sulfur-oxidizing biofertilizers have been recommended for grain crops (e.g., oilseed species, oats) and horticultural crops (e.g., onion, cauliflower, ginger, garlic) (Santra et al., 2015). Sulfur oxidation in soil is carried out by a variety of archaea and bacteria such as the genera Xanthobacter, Alcaligenes, Bacillus, Pseudomonas, Streptomyces, and Thiobacillus, as well as fungi including Fusarium, Aspergillus, and Penicillium (Grayston et al., 1986; Germida and Janzen, 1993; Macik et al., 2020).
Micronutrients: Chelation and Solubilization
Micronutrients such as iron (Fe), zinc (Zn), cooper (Cu), manganese (Mn), boron (B), molybdenum (Mo), chlorine (Cl), nickel (Ni), cobalt (Co), and silicon (Si), are essential for plants (Shukla et al., 2018). These are essential for plant development as they are involved in critical enzymatic reactions including photosynthesis, respiration, water oxidation, and oxidative stress protection (Castro et al., 2018). In fact, several studies revealed that micronutrient deficiencies hamper crop production in many areas of the world; especially in alkaline soils with low organic matter content (Rashid and Ryan, 2004).
One of the most studied mechanisms for increasing micronutrient availability is iron sequestration via siderophores (Rroço et al., 2003). Under Fe-limiting conditions, these low molecular weight chelating compounds scavenge Fe3+ (the most common form in soils) from the mineral phases, forming soluble Fe3+ complexes that are accessible for plant uptake (Figure 1). In general, plant species such as barley, rye, and wheat, can produce high concentrations of siderophores and, thus are more resistant to iron deficiency (Ahmed and Holmström, 2014). However, other crops (e.g., maize, sorghum, and rice) with lower siderophore production can benefit from siderophore-producing microorganisms. There are three main classes of fungal siderophores (i.e., rhodotorulic acid, ferrichromes, and fusarinines) and four classes of bacterial siderophores (i.e., phenol-catecholates, hydroxamates, carboxylate, and pyoverdines) (Crowley, 2006). In agricultural plants, siderophore production by Pseudomonas fluorescens was shown to play a role in Fe nutrition and PGP in tomato (Nagata, 2017), pea (Lurthy et al., 2020) and sorghum (Abbaszadeh-Dahaji et al., 2020) under Fe limiting conditions. Apart from Fe, siderophores are also known to bind other metals (e.g., Al3+, Cd2+, Co2+, Cu2+, Hg2+, Mn2+, Ni2+, and Pb2+) (Saha et al., 2013) (Figure 1).
Zinc deficiency is the most important micronutrient problem in crops, causing root necrosis, reduction of biomass and yield, and high plant mortality (Caldelas and Weiss, 2017). Also, it is estimated that more than 84% of total soil Zn occurs as structurally lattice bound [e.g., zincite (ZnO) and zinc sulfide (ZnS)], while only 1% is in water soluble form and available for plant uptake (Sharma et al., 2013; Prasad et al., 2016). Consequently, Zn is now integrated into chemical fertilizers (i.e., applied along with NPK) for most crops in several countries (Prasad et al., 2016). However, most of this water-soluble Zn (96–99%) is rapidly converted to insoluble forms, and only 1–4% of the total applied Zn can be used by plants (Kushwaha et al., 2020). A solution to this problem is the application of Zn-solubilizing microbes (ZSM) as biofertilizers to increase Zn availability in soils where this micronutrient is present in high concentrations but insoluble forms (Sammauria et al., 2020) (Figure 1). Similarly to other solubilizers, these ZSM are capable of solubilizing Zn by acidification, chelation, and chemical transformation (Kushwaha et al., 2020; Macik et al., 2020).
In 2013, Si was classified as a beneficial nutrient by the Association of American Plant Food Control Officials (AAPFCO), due to the increasing knowledge of Si effects on plant growth and protection (Heckman, 2013). In soils, most Si is in recalcitrant silicate minerals (i.e., aluminum silicates and quartz) and only a much smaller fraction is available for plants (Greger et al., 2018). The plant-available Si is in the form of monosilicic acid (H4SiO4), present both in the soil solution and adsorbed phases (Fe and Al oxides/hydroxides) (Tubaña and Heckman, 2015). Silicate-solubilizing bacteria occurring in soils and rhizosphere act by solubilizing silicates (Figure 1). The most studied species are from the genera Burkholderia, Aeromonas, Rhizobium, Enterobacter, and Bacillus (Santi and Goenadi, 2017; Lee et al., 2019).
Nutrient Mobilization and the Role of Root-Associated Fungi
The most studied case among root-associated fungi are arbuscular mycorrhizal fungi (AMF), obligate symbionts from the phylum Glomeromycota which can form symbiotic relationships with ~80% of land plant species, including agricultural crops (Berruti et al., 2016). Among other benefits, AMF can enhance the uptake of mineral nutrients (i.e., P, N, S, Cu, and Zn) and water by their host plants (Bücking and Kafle, 2015) in exchange for carbon sources (Hodge and Fitter, 2010; Veresoglou et al., 2012). The extraradical AMF mycelium increases the volume of soil explored not only by reaching far beyond the rhizosphere but also by penetrating smaller soil pores (Berruti et al., 2016). This is particularly important for the acquisition of less mobile nutrients such as P and, in fact, AMF are well-known for their ability to enhance P acquisition, especially in P-deficient soils (Kumar et al., 2018) (Figure 1). Arbuscular mycorrhizae were also shown to facilitate N uptake, mostly in the form of ammonium () (Hodge and Fitter, 2010; Veresoglou et al., 2012). Over the last decades, several companies manufactured and commercialized AMF inoculants and the global mycorrhiza-based biofertilizer market is projected to be worth 621.6 million US dollars by 2025. These products are especially being encouraged in countries of the Asia Pacific region such as India and China (ReportLinker, 2020) but, as will be discussed below, their reliability is still limited (Hart et al., 2018).
Besides AMF, efforts are being made to use other endophytic fungi to improve crop nutrient acquisition and growth (Murphy et al., 2018). Among these, root-colonizing dark septate endophytes (DSE) are a diverse group, mostly belonging to the phylum Ascomycota, which could potentially provide benefits ranging from nutrient acquisition to disease and abiotic stress tolerance (He et al., 2019a; Spagnoletti and Giacometti, 2020). These DSE have been found in over 600 plant species, some of them non-mycorrhizal, and several studies show their potential to enhance N uptake in crops such as rice and tomato (Mandyam and Jumpponen, 2005; Mahmoud and Narisawa, 2013; Vergara et al., 2018). Their effect on P acquisition has been less studied, but evidence suggests they could also assist on the solubilization of Ca, Al, and Fe phosphates (Spagnoletti et al., 2017).
Current Challenges Limiting Biofertilizer Applications
There are several key steps to be overcome by introduced microbe/s before achieving the desired effects in plant growth and fitness: survival, establishment, colonization, and interaction with the plant (e.g., parasitic/symbiotic behavior, PGP performance). This is particularly concerning in the lab-to-field transition, where it is common for a microbial strain with good performance in vitro to perform poorly in greenhouse and/or field trials (Parnell et al., 2016; Hart et al., 2018; Keswani et al., 2019). The inoculation outcome is especially hard to predict because we generally consider and control for a limited number of variables, usually not taking into account their intricate interactions (Moutia et al., 2010; Sasaki et al., 2010; Busby et al., 2017). Here, we summarize the main factors associated with inoculation success by classifying them into plant-related, edaphic/environmental, and inoculant-related (e.g., additives, concentration, viability) (Malusà et al., 2016) (Table 1), as well as the practical aspects that currently limit the applicability of biofertilizers in agriculture.
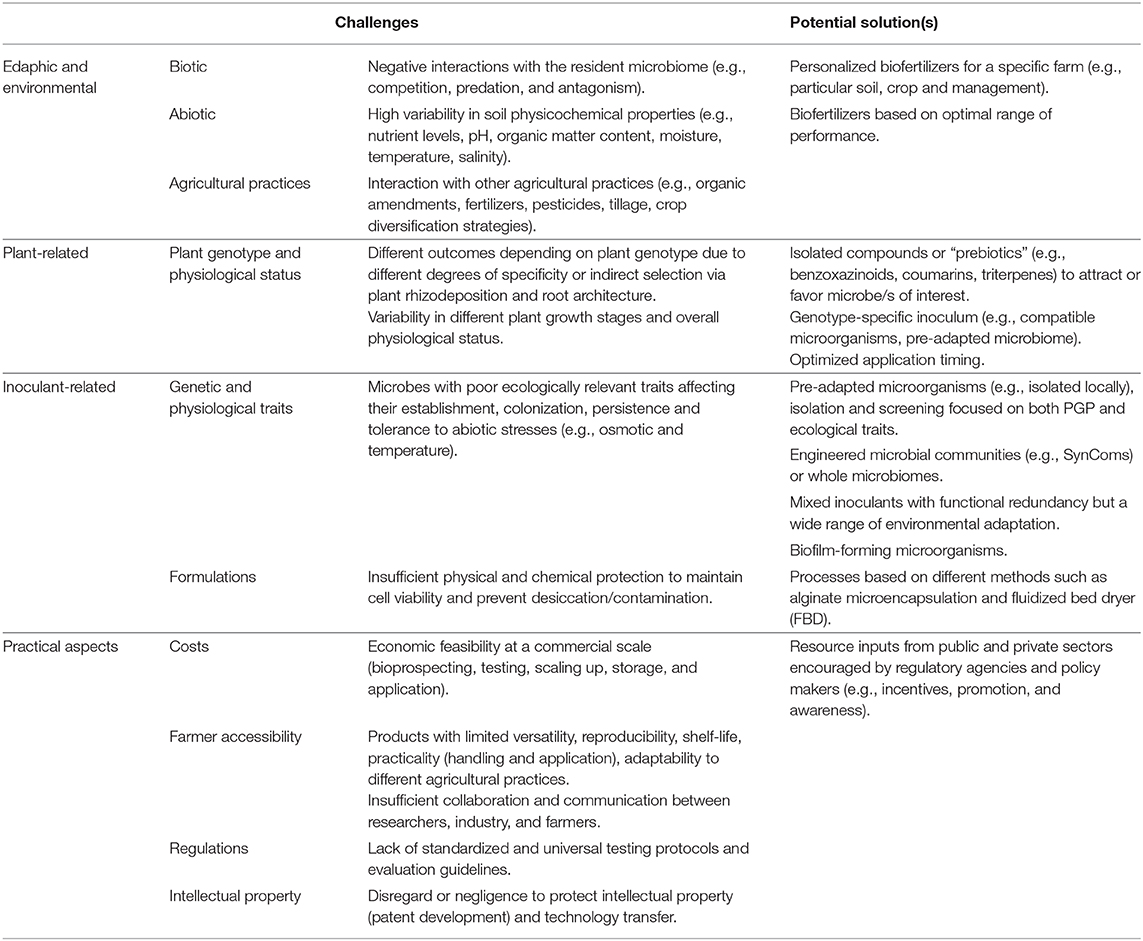
Table 1. Challenges associated with biofertilizer success and potential solutions relying on novel technologies.
Edaphic and Environmental Conditions
Edaphic and environmental conditions are two major factors behind the variability and low reproducibility of biofertilizers in field trials (Hoeksema et al., 2010; Da Costa et al., 2014; Schütz et al., 2018). Initial steps of biofertilizer testing are carried out in aseptic controlled conditions, which allow for an unbiased characterization of the microorganism under study. Scaling up to growth chamber or greenhouse trials, and especially to field conditions, increases the number of uncontrolled biotic and abiotic factors that can interfere with inoculation success (Nkebiwe et al., 2016; Bacilio et al., 2017). Among the biotic factors that can affect the outcome of inoculation, the most discussed is the presence of competitors, predators, or other antagonists within the resident microbiome (i.e., indigenous and previously introduced microorganisms) (Biró et al., 2000; Vargas et al., 2000; Ortas, 2003). Abiotic factors, either climatic or edaphic, can also influence the effectiveness of biofertilization on crop nutrient use efficiency and yield. According to a meta-analysis by Schütz et al. (2018), biofertilizers generally perform better under drier climatic conditions, higher soil P levels (especially for N2-fixers) and, exclusively for AMF, lower soil organic matter content and neutral pH. Yet, higher growth and yield responses to biofertilization are usually observed under low nutrient availability (Ozturk et al., 2003; Da Costa et al., 2014), as the plant can fully benefit from the interaction with the introduced microbe. The negative relationship between biofertilization success and soil nutrient content has been widely studied both for AMF colonization with soil P (Kaeppler et al., 2000; Jansa et al., 2009), and rhizobia nodulation with soil N (Glyan'ko et al., 2009; Thilakarathna and Raizada, 2017).
Some edaphic properties can also be highly susceptible to agricultural practices, therefore generating additional variability in inoculation success. So far, evidence has shown interacting effects of microbial inoculation with chemical fertilizers (Ozturk et al., 2003; Jansa et al., 2009; Da Costa et al., 2014), organic amendments (Manandhar et al., 2017; Ulzen et al., 2019), pesticides (Gaind et al., 2007; Jin et al., 2013b), and tillage (Miller et al., 1995; Mulas et al., 2015). It is also expected that crop diversification practices (e.g., rotations, cover crops) could modulate biofertilization efficiency by modifying resident microbial communities (Maiti et al., 2011; Buysens et al., 2016).
Plant-Related Factors
Biofertilization can lead to different outcomes depending on the selected crop species or genotype. Although some studies are beginning to identify genetic markers (e.g., quantitative trait loci or QTLs) associated with this differential response (Kaeppler et al., 2000; Remans et al., 2008), the plant factors behind them are not yet clearly understood. In general terms, it is known that plants can directly and indirectly alter the rhizosphere habitat through rhizodeposits and changes in the root architecture (Somers et al., 2004; Saleem et al., 2018). Among their many rhizodeposits, plant roots secrete signaling molecules such as secondary metabolites (e.g., flavonoids, hormones, antibiotics), some of which are important in the recognition and interaction with PGP microbes (Bais et al., 2004). The degree of specificity of this signaling process can be high for certain symbioses such as rhizobia-legumes, where the compatibility between microbes and host plants is crucial to establish a successful colonization (Hirsch et al., 2003; Thilakarathna and Raizada, 2017). In the case of arbuscular mycorrhiza, host specificity might not be as critical (Koyama et al., 2017) but colonization and PGP can still be affected by the plant genotype (Yao et al., 2001; Linderman and Davis, 2004; Hoeksema et al., 2010). Studies have explained these different levels of AMF-host plant compatibility through differences in root architecture (Declerck et al., 1995), aerial architecture (Liu et al., 2000), and P utilization and uptake efficiency (Kaeppler et al., 2000; Yao et al., 2001). Significant specificity between microbe and plant genotype was also observed for endophytes (Muñoz-Rojas and Caballero-Mellado, 2003; Yoon et al., 2016; Vujanovic and Germida, 2017) and free-living PGPR such as Azospirillum spp. (Sasaki et al., 2010; Vargas et al., 2012), Pseudomonas sp. (Digat et al., 1990; Safronova et al., 2006), and Azotobacter sp. (Mezei et al., 1997; Anbi et al., 2020). Considering that most plant breeding programs do not address plant-microbe interactions, plant genotype-induced variability in biofertilization outcomes remains a major concern. Furthermore, genotype effects are not isolated, but modulated by plant age and physiological status (Dennis et al., 2010), which will be ultimately determined by surrounding environmental conditions and time of inoculation.
Inoculant-Related Factors
The selected microbial genotype not only determines its PGP functions, but also its compatibility with the plant host genotype (Linderman and Davis, 2004; Vargas et al., 2012; Ehinger et al., 2014). Furthermore, microbial traits also determine key aspects of inoculation such as survival (before and after application), establishment, colonization, and persistence. Generally, inoculant development focuses on genetic and PGP traits, with little or no attention to ecological traits, which will ultimately determine inoculation success under field conditions (Hart et al., 2018; Kaminsky et al., 2019). For example, strains adapted to specific environmental conditions can be selected by focusing on specific traits such as osmotic tolerance (García et al., 2017) or psychrotolerance (Rawat et al., 2019). Another approach consists in the isolation of native strains, which were shown to improve biofertilization performance (Melchiorre et al., 2011; Ahmed et al., 2013; Maltz and Treseder, 2015). Yet, there seems to be a trade-off between establishment and survival traits and PGP traits, posing a challenge at each of the different stages of inoculant development (Parnell et al., 2016; Kaminsky et al., 2019). This trade-off, together with the high specificity of microbial strains to both environment and host genotype, leads to a crucial question: should we aim for more specific and targeted biofertilizers or, instead, pursuit broad-spectrum versatile products? (Parnell et al., 2016; Bell et al., 2019; Tosi et al., 2020a). In the latter, the outcome would imply either utilizing versatile microbes or formulating mixed inoculants that expand the ecological adaptation range (i.e., functionally redundant strains that encompass a wider range of environmental adaptation). In spite of the challenges arising from the interaction and possible interference among members of a mixed inoculant, which will differ on each particular scenario (Xavier and Germida, 2003; Remans et al., 2008; Ballesteros-Almanza et al., 2010), they bear great potential for overcoming the issues of environmental adaptability.
Besides the identity of the inoculated microbe, biofertilizer concentration, formulation, and delivery practices will also determine how tolerant and protected inoculated microbes will be from environmental constraints. The inoculant formulation should be able to support microbial growth, while protecting an amount of viable cells high enough for an effective response in the plant (Herrmann and Lesueur, 2013; Bashan et al., 2014). Additional challenges associated with scaling up and commercializing a microbial product include, but are not limited to, a risk of genetic and physiological changes in the strain, viability loss (particularly by desiccation) and contamination (Parnell et al., 2016; Glick, 2020; Greffe and Michiels, 2020). With the expansion in the biofertilizer industry, novel and more sophisticated formulations have been developed, including a variety of solid, slurry, and liquid carriers, as well as a wide array of additives (e.g., nutrients, stimulants, preservatives) to enhance the physical properties of the inoculant (e.g., adhesives, surfactants) (Bashan et al., 2014, 2016; Preininger et al., 2018). These additives allow microbes to withstand the fluctuating and suboptimal conditions during distribution, storage, and application (Parnell et al., 2016) (further discussed in section New Formulations and Delivery Methods). Even though these carriers and additives seem to be a secondary aspect of biofertilizer development, they can be critical for obtaining successful results (Gomez et al., 1997; Herrmann and Lesueur, 2013; Lee et al., 2016).
Similarly, delivery methods (e.g., on seed or into soil), and application timing and frequency can be critical for inoculation success (Parnell et al., 2016). For example, in a rhizobia inoculation trial on Pisum sativa, soil applications of a granular inoculant resulted in higher PGP than seed applications of a liquid formulation (Clayton et al., 2004). Foliar and flower applications were also being considered as a safer and more effective delivery strategy for endophytic or phyllosphere microorganisms (Mitter et al., 2013; Preininger et al., 2018). Application timing and frequency should be also taken into consideration in relation to plant growth stages (Bashan, 1986; Fallik et al., 1988; Linderman and Davis, 2004), as well as other agricultural practices such as fertilization (Pii et al., 2019) and transplant (Sohn et al., 2003). The time elapsed between application and establishment is critical, as it will determine the survival of the microbe to environmental constraints before it can provide any benefits to the plant.
Practical Aspects
We have discussed major technical limitations that can markedly affect biofertilizer efficiency, but there is also a set of practical aspects that cannot be overlooked in biofertilizer development. One of these concerns is the accessibility and convenience of inoculants for both farmers and manufacturers, especially in comparison with chemical fertilizers. Some key factors related to biofertilizer accessibility are: cost/benefit relationship, versatility, robustness and reproducibility, shelf-life and storage requirements, ease of use (handling and application), adaptability to agricultural practices and machinery, and biosafety (Bashan et al., 2014; Parnell et al., 2016; Bell et al., 2019). Novel products should be accompanied by a better outreach in order to inform farmers on the benefits of biofertilizers, particularly in the longer term, and to facilitate and promote their application (Parnell et al., 2016; Martínez-Hidalgo et al., 2018). A less discussed issue are intellectual property rights and patent development, which can be a valuable tool to transfer technology between the academic and industrial sector, but could also put at risk the free exchange of ideas and materials among researchers (Glick, 2020). Product commercialization also requires proper regulations (Sessitsch et al., 2019) with standardized and universal testing protocols and risk evaluation guidelines (Vílchez et al., 2016; Timmusk et al., 2017). Finally, the research and development sector are still in need of standardized protocols to evaluate inoculation success (Hart et al., 2018; Martínez-Hidalgo et al., 2018; Kaminsky et al., 2019) and to monitor microbes once applied in the field (Schütz et al., 2018; Compant et al., 2019).
New Approaches in Biofertilizer Development
In recent years, product development strategies have shifted from single-strain to microbial consortia inoculation. These strategies are based on a greater chance of at least one strain escaping competitive exclusion, and thus ensuring inoculant survival and function (Rivett et al., 2018; Tosi et al., 2020a). Microbial consortia can consist of two or more strains that are either closely (Kyei-Boahen et al., 2005; Jin et al., 2013a) or distantly (Ramírez-López et al., 2019; El Maaloum et al., 2020) related that provide an overall additive or synergistic biofertilization effect. One of the most common applications is the co-inoculation of rhizobia and AMF on legumes, as a number of studies report a synergistic effect on plant growth promotion (Xavier and Germida, 2003; Ashrafi et al., 2014; Kamaei et al., 2019). Yet, examples in the literature also show negative effects of AMF on nodule development or non-significant effects on crop yield (Antunes et al., 2006; Menéndez and Paço, 2020). Despite the promising beneficial effects of developing biofertilizers consisting of microbial consortia, it is unknown how these inoculants would establish across a range of agricultural field settings (Finkel et al., 2017). Moreover, even if inoculated microbes colonize their new environment initially, their persistence over time is not guaranteed. Here, we discuss new approaches to develop suitable bioinoculants at commercial scale from screening potential candidate microorganisms, designing the inoculant and optimizing formulations.
New Culture-Dependent Methods
Since Antony Van Leeuwenhoek's discovery of microorganisms in the 1670s, isolation and cultivation of microbes are the major pillars of microbiology (Oren and Garrity, 2014). Since then, the best practices for culturing new organisms have been developed and published in guides such as the Bergey's Manual of Systematic Bacteriology (Boone et al., 2001). However, in the last 20 years, the use of diffusion chambers (Kaeberlein, 2002; Bollmann et al., 2007) and isolation chips (Ichip) (Nichols et al., 2010), that mimic natural environments, increased the number of cultured colonies and marked a rebirth of culture dependent techniques. Most recently, studies by Lagier et al. (2015, 2016) used “culturomics” to cultivate previously uncultured members of the human gut microbiota. Culturomics uses multiple culture conditions, MALDI-TOF mass spectrometry and 16S rRNA sequencing for the identification of bacterial species (Lagier et al., 2018). The main objective of this technique is to suppress the culture of fast-growing and highly abundant species and to promote the growth of fastidious and/or less abundant microorganisms (Lagier et al., 2015).
Despite its success on the human microbiota, multiple combinations in culturomics (i.e., various growth media, culturing conditions, atmospheres, and times of incubation) have yet to be extended and developed for the soil and plant-associated microbiome. In order to minimize these challenges, Sarhan et al. (2019) suggested a “plant-tailored culturomic technique” that combines culturomics with plant-based media. According to these authors, most studies continue to use general media containing nutrients of animal origin (e.g., nutrient agar, R2A, and LB) to isolate plant associated microbes, whereas plant materials or dehydrated juices powders should be used instead. In fact, P-solubilizing Bacillus circulans and N2-fixing Azospirillum brasilense have been successfully grown on plant-only-based culture media (Youssef et al., 2016; Mourad et al., 2018). In addition, online platforms such as KOMODO (Known Media Database, http://komodo.modelseed.org) that includes >18,000 strain–media combinations and >3,300 media variants/compound concentrations can be used as a guide for developing suitable lab media for growing microorganisms (Oberhardt et al., 2015). Therefore, new culturing methods to discover novel isolates with biotechnological applications are key for biofertilizer development. Unfortunately, newly culturable microorganisms (e.g., slow growers) may still be less reliable and cost-effective for mass production with our current technology.
How to Artificially Select Microbiomes?
There are two main approaches to artificially select microbiomes: “top-down,” which modifies an existing microbiome, and “bottom-up,” which starts from individual microorganisms to build artificial or engineered microbiomes (e.g., synthetic communities or SynComs). In the “top-down” approach, selected environmental variables (e.g., pH, temperature, redox potential) are used to manipulate an existing microbiome, through ecological selection, to perform desired functions (Lawson et al., 2019). Although this approach is widely used for bioremediation (Atashgahi et al., 2018) and wastewater treatment (Demarche et al., 2012), it has the disadvantage of working with a complex community. In contrast, “bottom-up” approaches offer the advantage of simplifying these interactions by building artificial communities from pre-selected individual organisms (Raaijmakers, 2015).
Given the high complexity of molecular-scale microbiome processes, most of the challenges in designing microbial inoculants is to identify key beneficial microorganisms (e.g., Azotobacter, Rhizobium, Pseudomonas spp.) that are viable and with a greater chance of environmental colonization, resulting in reliable functional outcomes. One approach is to target keystone taxa (i.e., microbial taxa that are highly connected or highly influential on the community) in a pre-existent or artificially built microbiome (Banerjee et al., 2018). These taxa provide an appealing target for microbial screening, followed by isolation and whole genome sequencing to identify their functional capabilities (Kong et al., 2018). Consequently, their role in regulating the growth and function of other members of the microbiome can be exploited to enhance specific desired functions.
An important tool for identifying key taxa and developing ecosystem-wide dynamic models is through network analyses. Based on high-throughput sequencing data, these networks provide an overview of microbial assemblages and microbe-microbe interactions. With network centrality metrics, they can allow to further detect microbial taxa that hold key topological positions within the network (Toju et al., 2018b). Together, detecting ecologically significant microbial interactions, with proper experimentation, will provide powerful methods in developing new biofertilizers.
Creating Synthetic Communities
Recent culture independent techniques and new culture collections have paved the way for developing artificially constructed communities, also known as “artificial microbial consortia” (AMC) or “synthetic communities” (SynComs). Here, core microbiomes are used to recreate the structure and function of the microbiome. A great advantage of SynComs studies is that it allows for a detailed evaluation under controlled conditions, in which members can be added, eliminated, or substituted as needed (Vorholt et al., 2017). These studies can also help to elucidate different aspects of the spatial structure, microbial social interactions and how phenotypes interact and compete for space (Rodríguez Amor and Dal Bello, 2019). Yet, by definition, SynComs attempt to emulate the natural microbiome with less complexity, retaining only the indispensable microbial taxa, which could pose its own limitations (Vorholt et al., 2017; Satyanarayana et al., 2019). For example, SynComs with lower complexity might bypass important associations or inter-relationships, which might be critical at the functional level and, therefore, unsuitable for field applications. Highly complex SynComs, on the other hand, have their own designing limitations but a better chance of keeping associations intact (Satyanarayana et al., 2019). Despite its limitations, these reduced-complexity systems are particularly useful when a metabolic pathway is either too energy intensive or too complex to be accomplished by a single or few taxa.
In plant sciences, SynComs were first introduced in studies using Arabidopsis thaliana under gnotobiotic conditions (Bai et al., 2015; Lebeis et al., 2015). In studies by Castrillo et al. (2017), SynComs were used to investigate links between Arabidopsis phosphate starvation response, immune system function, and root microbiome assembly. In agricultural crops, Armanhi et al. (2018) designed a SynCom comprised of naturally occurring bacterial groups in the sugarcane microbiome and tested using maize as a model plant. These authors found that inoculated plants had an assemblage pattern similar to those found in sugarcane, which demonstrates a successful colonization of the synthetic community. However, Armanhi et al. (2018) conducted their SynCom assembly by choosing highly abundant bacterial groups, and we propose a selection based on microbe-microbe interactions, functional traits (e.g., nutrient solubilization/mineralization) and (or) colonization abilities (e.g., production of antimicrobial compounds). Moreover, biofertilizers can be developed with SynComs designed with high functional redundancy, in order to increase environmental adaptability and overcome some of the challenges of products currently available in the market.
Personalized Microbial Inoculants and Plant Prebiotics
Inspired by the concept of personalized diagnosis in medicine, Schlaeppi and Bulgarelli (2015) proposed a similar strategy in agricultural systems. This strategy consists on customizing tools such as microbial inoculants into farming practices. Similar to fertilizer consultants that advise farmers on application strategies, Bell et al. (2019) proposed a customizable field-scale microbial inoculant that, with appropriate implementation, could have long-lasting effects. Considering that soil conditions might change dramatically over short distances (Peukert et al., 2016), product development strategies of “one formulation applied for all fields” seems unrealistic. One strategy is the on-farm production of mycorrhizae-based inoculants, in which studies have shown their effects on potato (Douds et al., 2007; Goetten et al., 2016) and eggplant (Douds et al., 2017) growth and nutrition. These locally produced inoculants often have low costs and are applied shortly after production, without the need of shipping and storage (Douds et al., 2005). Yet, it is important to consider how these products will be feasible or cost-effective on a global scale. A good starting point could be establishing an optimal range for biofertilizer performance, in which inoculants would be introduced to conditions best resembling the soils they were isolated from. Here, different formulations can be designed for particular soils and(or) plant-root systems with the incorporation of certain aspects of precision farming, thus identifying areas in a particular field that might be more suitable to one formulation or another.
Another strategy is to use root exudates to stimulate the beneficial plant-associated microbiota. These exudates consist predominately of sugars and organic acids, but also flavonoids, amino acids, fatty acids, hormones, antimicrobial compounds, and vitamins (Bulgarelli et al., 2013). They can serve as growth substrates or signals for suitable microbes that strongly influences rhizosphere microbiome composition and dynamics (Philippot et al., 2013; Mitter et al., 2017; Sasse et al., 2018).
For example, benzoxazinoids (BXs) are major secondary defense metabolites in the Poaceae family (e.g., maize, wheat, and barley) that are typically produced during relatively early plant growth stages (Cotton et al., 2019). Benzoxazinoids and their breakdown products are known to be biocidal to some soil-borne bacteria and fungi and act as important regulators of belowground plant–microbe interactions (Schütz et al., 2019). Despite their allelochemical properties, studies by Neal et al. (2012) revealed that BXs may act as recruitment signals for Pseudomonas putida KT2440 in maize plants. In addition, P. putida has been previously studied for their ability to solubilize phosphate and thus promote growth of leguminous (Rosas et al., 2006) and maize (Sarikhani et al., 2020) plants. Hence, BXs could be exploited to recruit beneficial microbes such as P-solubilizing P. putida in field conditions.
Coumarins are a family of plant-derived secondary metabolites exuded by plants that have been extensively studied for their role in induced systemic resistance (ISR) (Stringlis et al., 2018; Pascale et al., 2020). However, Tsai and Schmidt (2017) reported their involvement as Fe-mobilizing compounds in response to Fe deficiency of dicotyledonous plants. Moreover, Voges et al. (2019) demonstrated the role of Fe-mobilizing coumarins in structuring the A. thaliana root bacterial community by inhibiting the growth of Pseudomonas spp. via a redox-mediated mechanism. Other molecules, such as triterpenes, can also promote the enrichment of Bacteroidetes and the depletion of Deltaproteobacteria in A. thaliana (Huang et al., 2019). Similarly, Koprivova et al. (2019) reported that a specific concentrations of plant derived camalexin concentration is necessary for proper interaction with a plant growth-promoting Pseudomonas sp. strain.
Multiple lines of evidence show that root exudates could be used as compounds to stimulate the growth of beneficial microbiota, rather than introducing microbes by inoculation. A similar approach was proposed by Arif et al. (2020), in which the authors suggested that particular soil amendments could act as “prebiotics” to promote microbial functions. Qiu et al. (2019) also suggested that synthesized compounds can be added to crops to attract or favor particular microbes. Yet, we propose that these “plant prebiotics” could be used in combination with microbial inoculants to enhance biofertilizer efficiency. By acting as signaling molecules, these compounds could potentially attract introduced microbes to the rhizosphere, thus giving them an advantage over other microorganisms for early colonization.
Biofilmed Biofertilizers
Biofilms consist of associated microorganisms, either from a single or multiple species, adhering to the biotic or abiotic surfaces in a self-produced matrix of extracellular polymeric substances (EPS) (Rana et al., 2020). This matrix provides the structure and protection by which microbes have the ability to chemically link with each other by quorum sensing (QS) and work as one unit (Li and Tian, 2012; Vlamakis et al., 2013). In soils, microbial communities such as bacteria and fungi can form biofilms on abiotic surfaces such as ore (minerals), water-air interfaces, and dead organisms (Rekadwad and Khobragade, 2017). Moreover, biofilm formation in the rhizosphere is an important trait that prevents microorganisms from being detached from plant roots by various natural processes (Velmourougane et al., 2017).
In recent years, biofilmed biofertilizers (BFBFs) (i.e., biofertilizers containing microbial communities capable of forming biofilms) have emerged as a new inoculant strategy to improve biofertilizer efficiency and sustain soil fertility (Parween et al., 2017). The idea behind BFBFs is that biofilm formation will create a more suitable environment for biofertilizers to compete with resident organisms and to cope with the heterogeneity of biotic and abiotic factors in soil (Ünal Turhan et al., 2019). For example, several studies have shown that biofilmed biofertilizers augmented P-solubilization (Swarnalakshmi et al., 2013), N2 fixation (Wang et al., 2017), siderophore production (Ricci et al., 2019), and Zn solubilization (Triveni and Jhansi, 2017). In addition, studies by Kopycińska et al. (2018) highlighted the role of biofilm formation, by exopolysaccharides (EPS) production, in Rhizobium leguminosarum during Zn stress. These authors found that EPS-deficient R. leguminosarum mutants were more sensitive to Zn exposure, whereas cell viability and root attachment were significantly higher in EPS producing strains.
Multi-species biofilms were also found to be more resilient in comparison to single-species biofilms (Velmourougane et al., 2017). In fact, natural rhizobacterial biofilms are often in mixed communities with interspecies interactions. This assembly is usually more advantageous than single planktonic cells, with optimal and maximal use of nutrients and resources (Nayak et al., 2020). For example, fungal–bacterial biofilms have been shown to enhance nutrient uptake and environmental stress tolerance compared to mono- or mixed-cultures of no biofilm-forming microorganisms (Hassani et al., 2018). Taktek et al. (2017) studied two hyphobacteria (Rhizobium miluonense and Burkholderia anthina) and two mycorrhizobacteria (Rahnella sp. and Burkholderia phenazinium) that strongly attaches to the surface of the arbuscular mycorrhizal fungus Rhizoglomus irregulare. These authors demonstrated that B. anthina can strongly adhere to abiotic and biotic surfaces and allow a higher phosphate solubilization than other isolates tested. Beneficial inter-kingdom biofilm formation were also reported in Bacillus sp. with Gigaspora margarita (Cruz and Ishii, 2012) and Pseudomonas fluorescens with Laccaria bicolor (Noirot-Gros et al., 2018).
Biofilmed biofertilizers have potential applications to improve ecosystem functioning and sustainability, which includes enhancing soil fertility and protecting the host plant under adverse conditions. However, biofilms are known to be problematic in many industrial settings as they often clog pipes and tubing (Vlamakis et al., 2013). Consequently, additional studies are needed to test BFBFs efficiency at a field scale and to determine optimal processes for a large-scale production and reliable results.
New Formulations and Delivery Methods
Following the selection of microorganisms and their functions, a suitable formulation is required to ensure microbial cell viability during storage and application. In fact, most governments regulate quality standards mandating a minimum number of viable cells and a threshold for any potential contaminants (e.g., chemical or microbial) (Herrmann et al., 2015; García de Salamone et al., 2019). Hence, different bioformulations have been developed and are broadly divided into those using solid materials as carriers or liquid formulations.
The most commonly used solid carriers are peat, rock phosphate, charcoal, lignite, vermiculite, clay, diatomaceous earth, talc, cellulose, and polymers such as xanthan gum (Mishra and Arora, 2016). Liquid formulations, also known as flowable or aqueous suspensions, consist of microbial suspensions in water, oils, or emulsions (Mondal and Dalai, 2017). In addition, bioformulations may contain additives such as methyl cellulose, starch and silica gel to improve their physical, chemical, and nutritional properties (Macik et al., 2020). The main disadvantages of liquid bioformulations are that the metabolic activity of beneficial microbes decrease rapidly after manufacturing and they often have higher contamination risks (Kaminsky et al., 2019; Macik et al., 2020; Vassilev et al., 2020). On the other hand, solid formulations (e.g., powders and granules) are challenging for non-sporulating bacteria, as desiccation disrupts cell membranes, causing cell death and overall loss of viability during rehydration (Berninger et al., 2018). This can lead to major setbacks for product commercialization. Two strategies to overcome these limitations are using microbial encapsulation with polymeric hydrogels and drying methods using a fluid bed dryer (FBD).
Polymeric hydrogels consist of crosslinked polymers chains with high affinity for water that are used for a variety of technological applications (Kobayashi, 2018). These hydrogels provide: (i) an aqueous environment that helps maintain the biological function of the encapsulated material and (ii) a diffusion barrier that allows the passage of molecules with a given size threshold (Pérez-Luna and González-Reynoso, 2018). Several naturally occurring (e.g., polysaccharides) and synthetic (e.g., polyacrylamide, polyurethane) polymers have been widely used for microbial encapsulation; yet, alginate has been particularly attractive specially in biomedical applications due to its structural similarity to extracellular matrices of living tissues (Gasperini et al., 2014). Alginate is a polysaccharide derived from different brown algae (e.g., Phaeophyceae) and bacteria (e.g., Azotobacter and Pseudomonas) (Lee and Mooney, 2012), and its main advantages are high biocompatibility in supporting cell survival, low toxicity and ease of gelation (Gasperini et al., 2014).
In biofertilizer applications, microbial cell entrapment with alginate allows a gradual and controlled release of microbial inoculants in the soil (Sahu and Brahmaprakash, 2016). The sticky nature of alginate may also help microbial cells to easily adhere to seeds and resist to environmental stresses (Nayak and Mishra, 2020). Lopes et al. (2020) used alginate beads for encapsulating plant growth-promoting Trichoderma spp., and found higher survival rates in freeze-dried encapsulated cells at different storage temperatures. In addition, studies using microbial cell entrapment with alginate have shown higher PGP rates on cotton (He et al., 2016), maize (Pitaktamrong et al., 2018), and hybrid cabbage (Stella et al., 2019) compared to non-entrapped cells. However, a few drawbacks still limit the use of these formulations at a large-scale in agricultural systems. For example, most natural polymers are heat sensitive and with lower mechanical strength compared to synthetic polymers (Zhu, 2007). In addition, alginate can be relatively costly and the porosity of alginate particles could be a limiting factor for the biofertilizer industry (Reis et al., 2006; Sahu and Brahmaprakash, 2016).
A new approach for developing formulation methods is by using fluid bed or fluidized bed dryer (FBD) to increase inoculant survival rate and reduce contamination. Fluid bed dryer has been extensively used by the pharmaceutical and food industry to reduce the moisture of powders and granules (Dewettinck and Huyghebaert, 1999). In this process, particles to be coated are maintained suspended against gravity in an upward flowing air stream causing them to behave as a fluid (Sahu and Brahmaprakash, 2016). Then, the coating material is sprayed through a nozzle onto particles and electrical heaters are employed for drying the material (Schoebitz et al., 2013). One of the main advantages of this process is that it operates in a temperature of ~37 to 40°C, which is milder than spray-drying and more suitable for mesophilic organisms (Sahu et al., 2018). In fact, Gangaraddi and Brahmaprakash (2018b) reported longer shelf-life of Rhizobium spp. inoculants in a FBD formulation, in which cell viability was maintained for up to 120 days. Higher cell viability using FBD have also been shown for biocontrol (Larena et al., 2003; Sabuquillo et al., 2006; Gotor-Vila et al., 2017) and for plant growth-promoting (Gangaraddi and Brahmaprakash, 2018a) inoculants. In spite of these results, the use of FBD technology in microbial inoculant formulations is not common. Future studies are still needed on optimizing temperature cycles of FBD for inoculant formulations and to assess survival rates after field applications. Yet, both methods using alginate or FBD have promising effects in reducing formulation inconsistency by preserving microbial cell density during storage. Furthermore, these techniques may open new possibilities to extend shelf-life of biofertilizers containing non-spore forming Gram-negative bacteria.
Biosafety of Biofertilizers
As discussed in previous sections, biofertilizers have great potential to replace chemical inputs in agricultural systems but still face several challenges in terms of technical reliability and accessibility to farmers. Yet, an important consideration is their potential impact on both human and ecosystem health. Due to the extensive nature of these risks, we believe that all inoculant research and development should be assessed through a “One Health” approach that regards plant, animal, human, and ecosystem health (van Bruggen et al., 2019). Currently, our understanding of both the introduced microorganism/s and the complex interactions that occur in the plant-soil interface is still limited to fully diagnose the risk posed by a specific product. However, research efforts have been made to fill knowledge gaps and to elaborate guidelines for assessing risks associated with different inoculants (Vílchez et al., 2016). Understanding the mechanisms behind successful PGP is as important as properly assessing the risks associated with the microorganisms that will be introduced in agricultural systems in a large scale.
Human Health Risks
The plant-soil interface hosts a vast number of genetically and functionally diverse microorganisms, some of which interact with the plant in different ways (beneficial, neutral, and pathogenic). Even though these interactions can lead to completely different outcomes in terms of plant fitness, they are known to share many features, to the extent that some symbiotic microbes could switch to a parasitic behavior with the same host (Kogel et al., 2006). In the last decades, various root-associated bacteria, some of them studied for their PGP traits, were found to be opportunistic plant and human pathogens (Berg et al., 2013). Potential human pathogenic bacteria can be found among several genera, including Burkholderia, Pseudomonas, Rhodococcus, Serratia, Acinetobacter, Stenotrophomonas, Enterobacter, Ochrobactrum, Klebsiella, Ralstonia, and Bacillus (Martínez-Hidalgo et al., 2018; Keswani et al., 2019). A well-studied case is that of the Burkholderia cepacia complex, a group of phenotypically associated bacterial species which have known PGP traits, including N2-fixation, but can also be opportunistic human pathogens (Chiarini et al., 2006; Eberl and Vandamme, 2016). Another intensively debated bacterial genus is Pseudomonas, which encompasses several PGP species (e.g., P. fluorescens, P. putida, P. putrefaciens, P. stutzeri, and P. pseudoalcaligenes) but also the pathogenic species P. aeruginosa, an opportunistic pathogen causing respiratory tract infections in humans (Mendes et al., 2013).
Currently, the majority of commercial biofertilizers consist of formulations of N2-fixing organisms (e.g., Actinorhizobium spp., Azospirillum spp., Azotobacter spp., and Rhizobium spp.), which have a low health risk and a history of safe application (Martínez-Hidalgo et al., 2018; Sessitsch et al., 2019). Yet, other bacterial species or strains which could provide useful functions for agriculture could also pose a biosafety threat. For instance, Actinobacteria are thought to have low pathogenic risk, but some species such as Streptomyces somaliensis can cause disease in humans (Martínez-Hidalgo et al., 2018; Olanrewaju and Babalola, 2019). In other cases, the presence of pathogenic species or strains within a taxonomic group can lead to drastic measures that would not be necessary with the proper testing methods and risk evaluation protocols. The B. cepacia complex was restricted for field application in the United States (Martínez-Hidalgo et al., 2018), even though its pathogenicity and ecology were found to be variable among species (Chiarini et al., 2006). The evaluation of pathogenicity and risk associated with a particular species or strain is unclear. More research is needed to identify genotypic and phenotypic differences between a plant or soil opportunistic pathogens and their clinical counterparts (Mendes et al., 2013; Martínez-Hidalgo et al., 2018). Genetic analyses could provide information about presence or absence of known virulence factors such as quorum sensing, motility, siderophore production, and lipopolysaccharide biosynthesis (Guttmann and Ellar, 2000; Eberl and Vandamme, 2016). In this sense, a powerful tool is whole genome sequencing to compare potentially pathogenic and mutualistic members of genus or species (Martínez-Hidalgo et al., 2018; Keswani et al., 2019). Another crucial step is understanding the role of horizontal gene transfer in pathogenicity (Dobrindt et al., 2004), particularly among members of the same species or strain. This information will allow to develop proper screening tests which could be followed by toxicity and pathogenicity testing on model hosts such as plants or nematodes (Martínez-Hidalgo et al., 2018). However, in the case of host-specific pathogens, such as some Burkholderia spp., model hosts might be insufficient to discard potential risks for humans (Eberl and Vandamme, 2016).
For all the above mentioned, it is clear that proper biosafety screening of any PGPB strain should become a standard practice in biofertilizer production, ensuring the safety of the product before exposing personnel, consumers, and natural resources (Berg et al., 2013; Keswani et al., 2019). More research is needed on potential allergens (Keswani et al., 2019) and fungal biofertilizers, including AMF, which might not be directly harmful for humans but could carry undesired microbial species in their formulation (Agnolucci et al., 2019). Moreover, it is also important to understand the environmental conditions that could promote the proliferation of opportunistic pathogens. For example, while some characteristics of the rhizosphere environment could be favoring opportunistic pathogenic bacteria (e.g., high nutrient availability, UV protection), others, such as high microbial diversity, could limit their survival (Matos et al., 2005; Mendes et al., 2013). Finally, there is a need for improved consistency when evaluating risk and establishing regulatory frameworks such as the Risk Groups (Europe) and Biosafety Level or BSL (United States) (Vílchez et al., 2016). Vílchez et al. (2016) developed an evaluation system, the “Environmental and Human Safety Index (EHSI)” based on a panel of tests which could be used to evaluate the safety of PGP bacteria in an objective and reliable manner, encompassing not only human but also environmental health. More effort must be invested in similar projects, with a special emphasis on international and interdisciplinary exchange and cooperation.
Environmental Health Risks: Effects on the Resident Biota
Ideally, a biofertilizer should cause a minimum and/or controlled impact in terms of dispersion, persistence, alteration of microbial function and biogeochemical cycling, and alteration of macroflora and macrofauna (Bashan et al., 2014). A major concern is the effect of the introduced microorganism/s on the resident microbiome, which can occur from direct ecological interactions, either synergistic or antagonistic (e.g., competition, inhibition), as well as horizontal gene transfer (Glick, 2020; Mawarda et al., 2020). Introduced microorganisms can also modify resident microbial communities indirectly, by modulating plant physiology and morphology. For example, some PGP are known to modify root architecture and exudation (Vacheron et al., 2013), which can alter rhizosphere communities (Jones, 1998; Saleem et al., 2018). In mixed plant communities, indirect effects on the resident microbiome could also be expected if the introduced microorganism induces changes in plant diversity and composition, as was observed with some AMF inoculants (Hart et al., 2018; Keswani et al., 2019).
Inoculation of rhizobia, widely utilized in biofertilizers, have shown significant impacts on soil and plant-associated microbial communities, as found in soybean (Zhong et al., 2019) and alfalfa (Schwieger and Tebbe, 2000) crops. These effects were not limited to the rhizosphere; field inoculation of Phaseolus vulgaris with two indigenous rhizobia strains (separately or combined) modified the structure and increased the phylotype richness of bacterial communities in the bulk soil (Trabelsi et al., 2011). Changes in microbial structure can result from both positive and negative interactions of rhizobia with the rhizosphere microbiome. Azospirillum, a bacterial genus characterized by free-living organisms with N2-fixing capabilities among other PGP traits, has shown variable effects on the resident microbiome (Trabelsi and Mhamdi, 2013). Inoculation of maize with A. lipoferum induced a shift in the composition of rhizosphere bacterial communities which lasted for at least one month (Baudoin et al., 2009). Yet, variable results were observed when inoculating the same or other crops with A. brasilense (Herschkovitz et al., 2005; Lerner et al., 2006; Correa et al., 2007), even though this bacterium can induce physiological and morphological changes in the root system. According to Trabelsi and Mhamdi (2013), effects of Azospirillum inoculation on rhizosphere microorganisms is likely driven by N dynamics, although evidence suggests that a wide array of factors are involved. While rhizobia and Azospirillum have been more widely studied regarding their effects on the resident microbiome, research on other taxa such as Pseudomonas sp. remains limited, even though they could potentially modify both bacterial and fungal communities (Andreote et al., 2009; Gao et al., 2012).
Among fungal biofertilizers, those based on AMF are the most widespread and commercially available, even though ecological risks of field application are not being properly assessed (Hart et al., 2018). Inoculation with foreign AMF can affect native AMF communities, for example displacing them and reducing their diversity (Koch et al., 2011) or modifying their composition (Jin et al., 2013a), although a response in the native AMF community is not always observed (Antunes et al., 2009). In the study by Jin et al. (2013b), a diverse AMF inoculum had less beneficial effects on plant fitness but had a lower impact on the composition of subsequent AMF communities. Besides native mycorrhizal fungi, introduced AMF can disturb other microbial communities in soil, particularly those surrounding their extraradical mycelium, (i.e., the mycorrhizosphere) (Trabelsi and Mhamdi, 2013). So far, the effect of AMF on soil microbial communities seems to be variable and modulated by several factors (Marschner and Timonen, 2005; Cavagnaro et al., 2006; Monokrousos et al., 2020). Remarkably, bacteria inhabiting the mycorrhizosphere, some of which may already be present in commercial inoculants, have shown PGP activity and are thought to act synergistically with AMF (Agnolucci et al., 2019). Lastly, non-AMF fungal inoculants have been less studied but there is some evidence for endophytic fungi (Casas et al., 2011; Rojas et al., 2016) and Trichoderma spp. (Jangir et al., 2019) to induce changes in local microbial communities.
The impact that introduced microbes may have on the resident microbial communities will depend to some extent on their abundance, survival, and permanence in the system (Ambrosini et al., 2016). This means that the same characteristics that will help guarantee a successful plant growth promotion, are also the ones increasing the risk for invasion. Yet, not enough studies focus on the effect of mass and repetitive inoculation, or effects in the long term or in subsequent crops (Trabelsi and Mhamdi, 2013; Mawarda et al., 2020). According to Hart et al. (2018), long-term effects are also important for AMF inoculants, although their complex genetic organization will definitely challenge the assessment of their establishment and persistence. These effects are particularly concerning if we consider that inoculation effects could remain even after the introduced microbial population decreases (Mawarda et al., 2020). One of the mechanisms behind these “legacy” effects is through plant-soil feedbacks, something more likely to occur when the introduced microorganism is symbiotic or has a high affinity for a specific plant (e.g., a non-target plant present in the agroecosystem) (Ambrosini et al., 2016; Keswani et al., 2019).
The variable effects observed on the resident microbiome when introducing a microorganisms via inoculation suggest the outcome is driven by several factors, including the host plant (Marschner and Timonen, 2005). For example, Correa et al. (2007) found that the plant genotype modulated the genetic and physiological response of phyllosphere and rhizoplane bacterial communities to A. brasilense seed inoculation. In another study, the response of rhizosphere bacterial communities to A. brasilense changed with the crop growth stage, being more affected at jointing than grain-filling stage (Di Salvo et al., 2018). On the other hand, the resident microbiome might present different susceptibility and buffering capacity levels depending on its diversity (Eisenhauer et al., 2013; Trabelsi and Mhamdi, 2013) and the presence of specific antagonists or synergistic taxa (Mar Vázquez et al., 2000; Asiloglu et al., 2020). Similarly, environmental conditions may play a key role regulating the impact of introduced microorganisms, as observed for soil moisture (He et al., 2019b; Monokrousos et al., 2020) and light intensity (Marschner and Timonen, 2005).
Overall, most studies analyzing the impact of biofertilizers seem to focus on structural aspects and not enough on functionality (Trabelsi and Mhamdi, 2013). Understanding the functional implications of those changes is crucial, since they will directly impact ecosystem functioning and health. Multi-omics and data analysis are key tools to begin to understand the complex dynamics taking place and to predict microbial response under natural conditions (Ambrosini et al., 2016; Timmusk et al., 2017). Another question that remains is how persistent the environmental impact of the introduced microbes is (i.e., long-term and legacy effects). Moreover, further research is needed in the specific case of genetically modified microorganisms, including effects of metabolic load (Glick, 2020), and risks of horizontal gene transfer and dispersion (Hirsch, 2004; Bellanger et al., 2014). Diverse inoculants could be a safer alternative in terms of environmental impact, as suggested by studies on AMF or Bacillus spp. inoculation (Jin et al., 2013a; Gadhave et al., 2018). Still, further research is needed to fully understand the impact of different types of mixed inoculants (either based on same species, different species or different strains) on the resident microbiome.
Conclusions and Future Perspectives
The global biofertilizer market has an estimated value of 2.3 billion US dollars and it is projected to increase to 3.9 billion in 2025 (Marketandmarkets, 2020). Despite their great potential and long-term effects, biofertilizer products still face major challenges limiting their use in agricultural settings. These are often associated with limited shelf-life and the survival of inoculated strains in vastly different environments.
In this review, we highlighted the application of multi-omics approaches and emerging technologies in biofertilizer development (Figure 2). As discussed by Saad et al. (2020) and Toju et al. (2018a), keystone taxa provide an appealing target to optimize the outcomes of biofertilization due their role in regulating the growth/function of other members in the plant microbiome. We propose that these taxa and other members of the core microbiome could be further explored to manipulate microbiomes or design synthetic microbial communities (e.g., SynComs). At the same time, emerging culture-based methods (e.g., “culturomics”) can be used to discover novel isolates with biofertilizer applications. As an alternative, or in combination with, we suggest the use of “plant prebiotics,” that act as signaling molecules to attract beneficial microbes, thus enhancing biofertilizer efficiency. These studies can be further integrated into a global database systematizing different outcomes, environmental conditions, targeted plant genotypes, soil types, and growing seasons.
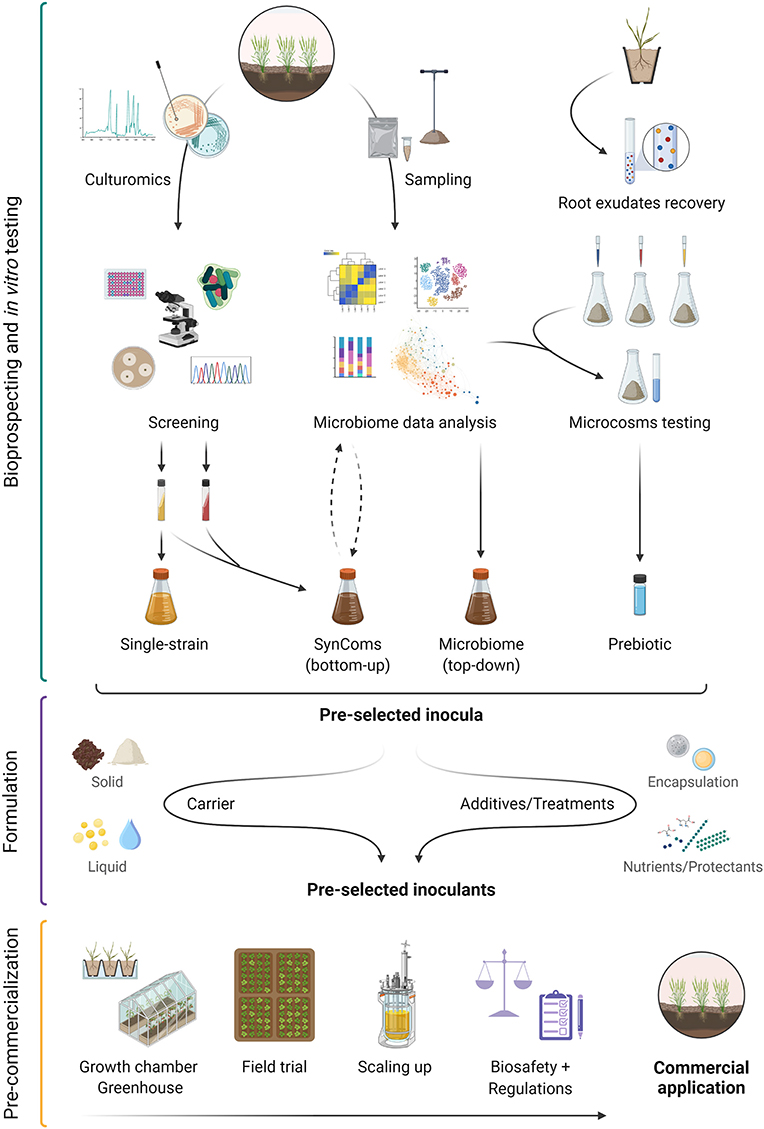
Figure 2. Overview of biofertilizer development workflow from potential candidate microorganisms to commercial applications with four novel approaches: single-strain inoculant obtained using emerging culture-based methods (e.g., culturomics), synthetic microbial communities (SynComs) obtained from a bottom-up approach, whole microbiomes recovered from natural or engineered ecosystems (top-down approach), and prebiotics obtained from root exudates. Following the initial stages of bioprospecting and in vitro testing, selected inocula and/or prebiotics require a proper formulation to ensure shelf life and protection. Finally, product pre-commercialization steps include in planta trials under controlled (growth chamber/greenhouse) and uncontrolled (field) conditions, production scale-up to a commercial scale, proper biosafety screening tests (e.g., toxicity and pathogenicity), and compliance with existing regulations. Created with BioRender (https://biorender.com/).
The success of biofertilizers, however, not only depends on selecting specific microorganisms or functions, but also on developing new formulations to ensure the survival of inoculated strain(s). Here, we reviewed different methods in which bioformulations could be improved by using biofilm-producing strains, microencapsulation with alginate, and processes based on fluidized bed dryer (FBD). Ideally, new technologies should target carriers and additives that are cost-effective and easy to use but, most importantly, able to support a higher number of viable cells during storage and application.
Simultaneously, the biosafety of inoculated microbes should be assessed through a “One Health” approach. This step includes proper screening tests (e.g., toxicity and pathogenicity testing) to ensure their safety before exposing personnel, consumers and natural resources. Moreover, continued studies on ecological interactions and how plants shape their microbiome in agricultural systems are still essential. This is particularly critical in the context of climate change, where key biogeochemical processes carried out by soil microorganisms may be affected.
Due to the complexity and genetic diversity within the soil and plant microbiome, it is unlikely that one formulation will be effective for all fields. Yet, it is unfeasible and unrealistic to design specific biofertilizers for each particular field. For this reason, in agreement with Bell et al. (2019), we suggest that continuous product design, refinement, and validation should be oriented toward optimal and sub-optimal environmental ranges for microbial products (i.e., crop, climate, soil type, and agricultural practices). Finally, significant resource inputs from both public and private sectors are needed to fill critical knowledge gaps in the field. This effort must be accompanied by the encouragement of regulatory agencies and policy makers supporting sustainable practices and biofertilizers.
Author Contributions
EKM, MT, and DO collected literature and wrote the manuscript. EKM edited the manuscript and MT designed the figures. JJG and KED provided critical feedback. All authors conceived and planned the scope, and approved the final version manuscript.
Funding
This work was supported by Food from Thought, a program funded by Canada First Research Excellence Fund (CFREF) and the Natural Sciences and Engineering Research Council (NSERC). Funding was provided by a MITACS Elevate Post-doctoral Fellowship (EKM) and the Canada Research Chairs Program (KED).
Conflict of Interest
The authors declare that the research was conducted in the absence of any commercial or financial relationships that could be construed as a potential conflict of interest.
References
Abbaszadeh-Dahaji, P., Masalehi, F., and Akhgar, A. (2020). Improved growth and nutrition of sorghum (Sorghum bicolor) plants in a low-fertility calcareous soil treated with plant growth–promoting rhizobacteria and Fe-EDTA. J. Soil Sci. Plant Nutr. 20, 31–42. doi: 10.1007/s42729-019-00098-9
Agnolucci, M., Avio, L., Pepe, A., Turrini, A., Cristani, C., Bonini, P., et al. (2019). Bacteria associated with a commercial mycorrhizal inoculum: community composition and multifunctional activity as assessed by illumina sequencing and culture-dependent tools. Front. Plant Sci. 9:1956. doi: 10.3389/fpls.2018.01956
Ahmed, B., Midrarullah, and Sajjad Mirza, M. (2013). Effects of inoculation with plant growth promoting rhizobacteria (PGPRs) on different growth parameters of cold area rice variety, Fakre malakand. Afr. J. Microbiol. Res. 7, 1651–1656. doi: 10.5897/ajmr2013.5351
Ahmed, E., and Holmström, S. J. M. (2014). Siderophores in environmental research: roles and applications. Microb. Biotechnol. 7, 196–208. doi: 10.1111/1751-7915.12117
Alori, E. T., Glick, B. R., and Babalola, O. O. (2017). Microbial phosphorus solubilization and its potential for use in sustainable agriculture. Front. Microbiol. 8:971. doi: 10.3389/fmicb.2017.00971
Ambrosini, A., de Souza, R., and Passaglia, L. M. P. (2016). Ecological role of bacterial inoculants and their potential impact on soil microbial diversity. Plant Soil 400, 193–207. doi: 10.1007/s11104-015-2727-7
Anand, K., Kumari, B., and Mallick, M. A. (2016). Phosphate solubilizing microbes: an effective and alternative approach as biofertilizers. Int. J. Pharm. Pharm. Sci. 8, 37–40.
Anbi, A. A., Mirshekari, B., Eivazi, A., Yarnia, M., and Behrouzyar, E. K. (2020). PGPRs affected photosynthetic capacity and nutrient uptake in different Salvia species. J. Plant Nutr. 43, 108–121. doi: 10.1080/01904167.2019.1659342
Andreote, F. D., De Araújo, W. L., De Azevedo, J. L., Van Elsas, J. D., Da Rocha, U. N., and Van Overbeek, L. S. (2009). Endophytic colonization of potato (Solanum tuberosum L.) by a novel competent bacterial endophyte, Pseudomonas putida strain P9, and its effect on associated bacterial communities. Appl. Environ. Microbiol. 75, 3396–3406. doi: 10.1128/AEM.00491-09
Antunes, P. M., de Varennes, A., Zhang, T., and Goss, M. J. (2006). The tripartite symbiosis formed by indigenous arbuscular mycorrhizal fungi, Bradyrhizobium japonicum and soya bean under field conditions. J. Agron. Crop Sci. 192, 373–378. doi: 10.1111/j.1439-037X.2006.00223.x
Antunes, P. M., Koch, A. M., Dunfield, K. E., Hart, M. M., Downing, A., Rillig, M. C., et al. (2009). Influence of commercial inoculation with Glomus intraradices on the structure and functioning of an AM fungal community from an agricultural site. Plant Soil 317, 257–266. doi: 10.1007/s11104-008-9806-y
Arif, I., Batool, M., and Schenk, P. M. (2020). Plant microbiome engineering: expected benefits for improved crop growth and resilience. Trends Biotechnol. 38, 1385–1396. doi: 10.1016/j.tibtech.2020.04.015
Armanhi, J. S. L., de Souza, R. S. C., Damasceno, N. B., de Araújo, L. M., Imperial, J., and Arruda, P. (2018). A community-based culture collection for targeting novel plant growth-promoting bacteria from the sugarcane microbiome. Front. Plant Sci. 8:2191. doi: 10.3389/fpls.2017.02191
Ashrafi, E., Zahedi, M., and Razmjoo, J. (2014). Co-inoculations of arbuscular mycorrhizal fungi and rhizobia under salinity in alfalfa. Soil Sci. Plant Nutr. 60, 619–629. doi: 10.1080/00380768.2014.936037
Asiloglu, R., Shiroishi, K., Suzuki, K., Turgay, O. C., Murase, J., and Harada, N. (2020). Protist-enhanced survival of a plant growth promoting rhizobacteria, Azospirillum sp. B510, and the growth of rice (Oryza sativa L.) plants. Appl. Soil Ecol. 154:103599. doi: 10.1016/j.apsoil.2020.103599
Atashgahi, S., Sánchez-Andrea, I., Heipieper, H. J., van der Meer, J. R., Stams, A. J. M., and Smidt, H. (2018). Prospects for harnessing biocide resistance for bioremediation and detoxification. Science 360, 743–746. doi: 10.1126/science.aar3778
Bacilio, M., Moreno, M., Lopez-Aguilar, D. R., and Bashan, Y. (2017). Scaling from the growth chamber to the greenhouse to the field: demonstration of diminishing effects of mitigation of salinity in peppers inoculated with plant growth-promoting bacterium and humic acids. Appl. Soil Ecol. 119, 327–338. doi: 10.1016/j.apsoil.2017.07.002
Bai, Y., Müller, D. B., Srinivas, G., Garrido-Oter, R., Potthoff, E., Rott, M., et al. (2015). Functional overlap of the Arabidopsis leaf and root microbiota. Nature 528, 364–369. doi: 10.1038/nature16192
Bais, H. P., Park, S. W., Weir, T. L., Callaway, R. M., and Vivanco, J. M. (2004). How plants communicate using the underground information superhighway. Trends Plant Sci. 9, 26–32. doi: 10.1016/j.tplants.2003.11.008
Ballesteros-Almanza, L., Altamirano-Hernandez, J., Peña-Cabriales, J. J., Santoyo, G., Sanchez-Yañez, J. M., Valencia-Cantero, E., et al. (2010). Effect of co-inoculation with mycorrhiza and rhizobia on the nodule trehalose content of different bean genotypes. Open Microbiol. J. 4, 83–92. doi: 10.2174/1874285801004010083
Banerjee, S., Schlaeppi, K., and van der Heijden, M. G. A. (2018). Keystone taxa as drivers of microbiome structure and functioning. Nat. Rev. Microbiol. 16, 567–576. doi: 10.1038/s41579-018-0024-1
Bargaz, A., Lyamlouli, K., Chtouki, M., Zeroual, Y., and Dhiba, D. (2018). Soil microbial resources for improving fertilizers efficiency in an integrated plant nutrient management system. Front. Microbiol. 9:1606. doi: 10.3389/fmicb.2018.01606
Bashan, Y. (1986). Significance of timing and level of inoculation with rhizosphere bacteria on wheat plants. Soil Biol. Biochem. 18, 297–301. doi: 10.1016/0038-0717(86)90064-7
Bashan, Y., and De-Bashan, L. E. (2010). How the plant growth-promoting bacterium Azospirillum promotes plant growth—a critical assessment. Adv. Agron. 108, 77–136. doi: 10.1016/S0065-2113(10)08002-8
Bashan, Y., De-Bashan, L. E., Prabhu, S. R., and Hernandez, J. P. (2014). Advances in plant growth-promoting bacterial inoculant technology: formulations and practical perspectives (1998-2013). Plant Soil 378, 1–33. doi: 10.1007/s11104-013-1956-x
Bashan, Y., Kloepper, J. W., De-Bashan, L. E., and Nannipieri, P. (2016). A need for disclosure of the identity of microorganisms, constituents, and application methods when reporting tests with microbe-based or pesticide-based products. Biol. Fertil. Soils 52, 283–284. doi: 10.1007/s00374-016-1091-y
Baudoin, E., Nazaret, S., Mougel, C., Ranjard, L., and Moënne-Loccoz, Y. (2009). Impact of inoculation with the phytostimulatory PGPR Azospirillum lipoferum CRT1 on the genetic structure of the rhizobacterial community of field-grown maize. Soil Biol. Biochem. 41, 409–413. doi: 10.1016/j.soilbio.2008.10.015
Bell, T. H., Kaminsky, L. M., Gugino, B. K., Carlson, J. E., Malik, R. J., Hockett, K. L., et al. (2019). Factoring ecological, societal, and economic considerations into inoculant development. Trends Biotechnol. 37, 572–573. doi: 10.1016/j.tibtech.2019.02.009
Bellanger, X., Guilloteau, H., Bonot, S., and Merlin, C. (2014). Demonstrating plasmid-based horizontal gene transfer in complex environmental matrices: a practical approach for a critical review. Sci. Total Environ. 493, 872–882. doi: 10.1016/j.scitotenv.2014.06.070
Berg, G., Alavi, M., Schmid, M., and Hartmann, A. (2013). The rhizosphere as a reservoir for opportunistic human pathogenic bacteria. Mol. Microb. Ecol. Rhizosph. 2, 1209–1216. doi: 10.1002/9781118297674.ch116
Berninger, T., González López, Ó., Bejarano, A., Preininger, C., and Sessitsch, A. (2018). Maintenance and assessment of cell viability in formulation of non-sporulating bacterial inoculants. Microb. Biotechnol. 11, 277–301. doi: 10.1111/1751-7915.12880
Berruti, A., Lumini, E., Balestrini, R., and Bianciotto, V. (2016). Arbuscular mycorrhizal fungi as natural biofertilizers: let's benefit from past successes. Front. Microbiol. 6:1559. doi: 10.3389/fmicb.2015.01559
Biró, B., Köves-Péchy, K., Vörös, I., Takács, T., Eggenberger, P., and Strasser, R. J. (2000). Interrelations between Azospirillum and Rhizobium nitrogen-fixers and arbuscular mycorrhizal fungi in the rhizosphere of alfalfa in sterile, AMF-free or normal soil conditions. Appl. Soil Ecol. 15, 159–168. doi: 10.1016/S0929-1393(00)00092-5
Bollmann, A., Lewis, K., and Epstein, S. S. (2007). Incubation of environmental samples in a diffusion chamber increases the diversity of recovered isolates. Appl. Environ. Microbiol. 73, 6386–6390. doi: 10.1128/AEM.01309-07
Boone, D. R., Castenholz, R. W., and Garrity, G. M., (eds.). (2001). Bergey's Manual® of Systematic Bacteriology. New York, NY: Springer. doi: 10.1007/978-0-387-21609-6
Broughton, P. L. (2019). Economic geology of southern Saskatchewan potash mines. Ore Geol. Rev. 113:103117. doi: 10.1016/j.oregeorev.2019.103117
Bücking, H., and Kafle, A. (2015). Role of arbuscular mycorrhizal fungi in the nitrogen uptake of plants: current knowledge and research gaps. Agronomy 5, 587–612. doi: 10.3390/agronomy5040587
Bulgarelli, D., Schlaeppi, K., Spaepen, S., Ver Loren van Themaat, E., and Schulze-Lefert, P. (2013). Structure and functions of the bacterial microbiota of plants. Annu. Rev. Plant Biol. 64, 807–838. doi: 10.1146/annurev-arplant-050312-120106
Busby, P. E., Soman, C., Wagner, M. R., Friesen, M. L., Kremer, J., Bennett, A., et al. (2017). Research priorities for harnessing plant microbiomes in sustainable agriculture. PLoS Biol. 15:e2001793. doi: 10.1371/journal.pbio.2001793
Buysens, C., César, V., Ferrais, F., Dupré de Boulois, H., and Declerck, S. (2016). Inoculation of Medicago sativa cover crop with Rhizophagus irregularis and Trichoderma harzianum increases the yield of subsequently-grown potato under low nutrient conditions. Appl. Soil Ecol. 105, 137–143. doi: 10.1016/j.apsoil.2016.04.011
Caldelas, C., and Weiss, D. J. (2017). Zinc Homeostasis and isotopic fractionation in plants: a review. Plant Soil 411, 17–46. doi: 10.1007/s11104-016-3146-0
Carareto Alves, L. M., de Souza, J. A. M., deVarani, A. M., and de Lemos, E. G. M. (2014). “The Family Rhizobiaceae,” in The Prokaryotes: Alphaproteobacteria and Betaproteobacteria, eds E. Rosenberg, E. F. DeLong, S. Lory, E. Stackebrandt, and F. Thompson (Berlin; Heidelberg: Springer), 419–437. doi: 10.1007/978-3-642-30197-1_297
Casas, C., Omacini, M., Montecchia, M. S., and Correa, O. S. (2011). Soil microbial community responses to the fungal endophyte Neotyphodium in Italian ryegrass. Plant Soil 340, 347–355. doi: 10.1007/s11104-010-0607-8
Castrillo, G., Teixeira, P. J. P. L., Paredes, S. H., Law, T. F., de Lorenzo, L., Feltcher, M. E., et al. (2017). Root microbiota drive direct integration of phosphate stress and immunity. Nature 543, 513–518. doi: 10.1038/nature21417
Castro, P. H., Lilay, G. H., and Assunção, A. G. L. (2018). “Regulation of micronutrient homeostasis and deficiency response in plants,” in Plant Micronutrient Use Efficiency, eds M. A. Hossain, T. Kamiya, D. J. Burritt, L. P. Tran, and T. Fujiwara (Cambridge, MA: Elsevier), 1–15. doi: 10.1016/B978-0-12-812104-7.00002-2
Cavagnaro, T. R., Jackson, L. E., Six, J., Ferris, H., Goyal, S., Asami, D., et al. (2006). Arbuscular mycorrhizas, microbial communities, nutrient availability, and soil aggregates in organic tomato production. Plant Soil 282, 209–225. doi: 10.1007/s11104-005-5847-7
Chiarini, L., Bevivino, A., Dalmastri, C., Tabacchioni, S., and Visca, P. (2006). Burkholderia cepacia complex species: health hazards and biotechnological potential. Trends Microbiol. 14, 277–286. doi: 10.1016/j.tim.2006.04.006
Clayton, G. W., Rice, W. A., Lupwayi, N. Z., Johnston, A. M., Lafond, G. P., Grant, C. A., et al. (2004). Inoculant formulation and fertilizer nitrogen effects on field pea: nodulation, N2 fixation and nitrogen partitioning. Can. J. Plant Sci. 84, 79–88. doi: 10.4141/P02-089
Compant, S., Samad, A., Faist, H., and Sessitsch, A. (2019). A review on the plant microbiome: ecology, functions, and emerging trends in microbial application. J. Adv. Res. 19, 29–37. doi: 10.1016/j.jare.2019.03.004
Correa, O. S., Romero, A. M., Montecchia, M. S., and Soria, M. A. (2007). Tomato genotype and Azospirillum inoculation modulate the changes in bacterial communities associated with roots and leaves. J. Appl. Microbiol. 102, 781–786. doi: 10.1111/j.1365-2672.2006.03122.x
Cotton, T. E. A., Pétriacq, P., Cameron, D. D., Meselmani, M., Al Schwarzenbacher, R., Rolfe, S. A., et al. (2019). Metabolic regulation of the maize rhizobiome by benzoxazinoids. ISME J. 13, 1647–1658. doi: 10.1038/s41396-019-0375-2
Crowley, D. E. (2006). “Microbial siderophores in the plant rhizosphere,” in Iron Nutrition in Plants and Rhizospheric Microorganisms, eds L. L. Barton and J. Abadia (Dordrecht: Springer), 169–198. doi: 10.1007/1-4020-4743-6_8
Cruz, A. F., and Ishii, T. (2012). Arbuscular mycorrhizal fungal spores host bacteria that affect nutrient biodynamics and biocontrol of soil-borne plant pathogens. Biol. Open 1, 52–57. doi: 10.1242/bio.2011014
Da Costa, P. B., Granada, C. E., Ambrosini, A., Moreira, F., De Souza, R., Dos Passos, J. F. M., et al. (2014). A model to explain plant growth promotion traits: a multivariate analysis of 2,211 bacterial isolates. PLoS ONE 9:e116020. doi: 10.1371/journal.pone.0116020
Dakora, F. D., Chimphango, S. B. M., Chimphango, S. B. M., Elmerich, C., and Newton, W. E. (2008). “Biological nitrogen fixation: towards poverty alleviation through sustainable agriculture,” in Proceedings of the 15th International Nitrogen Fixation Congress and the 12th International Conference of the African Association for Biological Nitrogen Fixation (Dordrecht: Springer).
De Freitas, J. R., Banerjee, M. R., and Germida, J. J. (1997). Phosphate-solubilizing rhizobacteria enhance the growth and yield but not phosphorus uptake of canola (Brassica napus L.). Biol. Fertil. Soils 24, 358–364. doi: 10.1007/s003740050258
Debnath, S., Rawat, D., Kumar Mukherjee, A., Adhikary, S., and Kundu, R. (2019). “Applications and constraints of plant beneficial microorganisms in agriculture, biostimulants,” in Plant Science, eds S. M. Mirmajlessi and R. Radhakrishnan (IntechOpen), 1–25. doi: 10.5772/intechopen.89190
Declerck, S., Plenchette, C., and Strullu, D. G. (1995). Mycorrhizal dependency of banana (Musa acuminata, AAA group) cultivar. Plant Soil 176, 183–187. doi: 10.1007/BF00017688
Demarche, P., Junghanns, C., Nair, R. R., and Agathos, S. N. (2012). Harnessing the power of enzymes for environmental stewardship. Biotechnol. Adv. 30, 933–953. doi: 10.1016/j.biotechadv.2011.05.013
Dennis, P. G., Miller, A. J., and Hirsch, P. R. (2010). Are root exudates more important than other sources of rhizodeposits in structuring rhizosphere bacterial communities? FEMS Microbiol. Ecol. 72, 313–327. doi: 10.1111/j.1574-6941.2010.00860.x
Dewettinck, K., and Huyghebaert, A. (1999). Fluidized bed coating in food technology. Trends Food Sci. Technol. 10, 163–168. doi: 10.1016/S0924-2244(99)00041-2
Di Salvo, L. P., Ferrando, L., Fernández-Scavino, A., and García de Salamone, I. E. (2018). Microorganisms reveal what plants do not: wheat growth and rhizosphere microbial communities after Azospirillum brasilense inoculation and nitrogen fertilization under field conditions. Plant Soil 424, 405–417. doi: 10.1007/s11104-017-3548-7
Digat, B., Gaudillat, M., and Labadie, J. M. (1990). Susceptibility of various tomato and lettuce genotypes to plant-growth-promoting Pseudomonas. Symbiosis 9, 295–303.
Dobrindt, U., Hochhut, B., Hentschel, U., and Hacker, J. (2004). Genomic islands in pathogenic and environmental microorganisms. Nat. Rev. Microbiol. 2, 414–424. doi: 10.1038/nrmicro884
Douds, D. D., Carr, E., Shenk, J. E., and Ganser, S. (2017). Positive yield response of eggplant (Solanum melongena L.) to inoculation with AM fungi produced on-farm. Sci. Hortic. 224, 48–52. doi: 10.1016/j.scienta.2017.05.017
Douds, D. D., Nagahashi, G., Pfeffer, P. E., Kayser, W. M., and Reider, C. (2005). On-farm production and utilization of arbuscular mycorrhizal fungus inoculum. Can. J. Plant Sci. 85, 15–21. doi: 10.4141/P03-168
Douds, D. D., Nagahashi, G., Reider, C., and Hepperly, P. R. (2007). Inoculation with arbuscular mycorrhizal fungi increases the yield of potatoes in a high P soil. Biol. Agric. Hortic. 25, 67–78. doi: 10.1080/01448765.2007.10823209
Eberl, L., and Vandamme, P. (2016). Members of the genus Burkholderia: good and bad guys. F1000Res. 5:F1000 Faculty Rev-1007. doi: 10.12688/F1000RESEARCH.8221.1
Ehinger, M., Mohr, T. J., Starcevich, J. B., Sachs, J. L., Porter, S. S., and Simms, E. L. (2014). Specialization-generalization trade-off in a Bradyrhizobium symbiosis with wild legume hosts. BMC Ecol. 14:8. doi: 10.1186/1472-6785-14-8
Eisenhauer, N., Schulz, W., Scheu, S., and Jousset, A. (2013). Niche dimensionality links biodiversity and invasibility of microbial communities. Funct. Ecol. 27, 282–288. doi: 10.1111/j.1365-2435.2012.02060.x
El Maaloum, S., Elabed, A., Alaoui-Talibi, Z., El Meddich, A., Filali-Maltouf, A., Douira, A., et al. (2020). Effect of arbuscular mycorrhizal fungi and phosphate-solubilizing bacteria consortia associated with phospho-compost on phosphorus solubilization and growth of tomato seedlings (Solanum lycopersicum L.). Commun. Soil Sci. Plant Anal. 51, 622–634. doi: 10.1080/00103624.2020.1729376
El-Ghamry, A., Mosa, A., Alshaal, T., and El-Ramady, H. (2018). Nanofertilizers vs. biofertilizers: new insights. Environ. Biodivers. Soil Secur. 2, 40–50. doi: 10.21608/jenvbs.2018.3880.1029
Ercoli, L., Arduini, I., Mariotti, M., Lulli, L., and Masoni, A. (2012). Management of sulphur fertiliser to improve durum wheat production and minimise S leaching. Eur. J. Agron. 38, 74–82. doi: 10.1016/j.eja.2011.12.004
Fallik, E., Okon, Y., and Fischer, M. (1988). Growth response of maize roots to Azospirillum inoculation: effect of soil organic matter content, number of rhizosphere bacteria and timing of inoculation. Soil Biol. Biochem. 20, 45–49. doi: 10.1016/0038-0717(88)90125-3
Ferreira, M. J., Silva, H., and Cunha, A. (2019). Siderophore-producing rhizobacteria as a promising tool for empowering plants to cope with iron limitation in saline soils: a review. Pedosphere 29, 409–420. doi: 10.1016/S1002-0160(19)60810-6
Finkel, O. M., Castrillo, G., Paredes, S. H., Dangl, J. L., Herrera Paredes, S., Salas González, I., et al. (2017). Understanding and exploiting plant beneficial microbes. Curr. Opin. Plant Biol. 38, 155–163. doi: 10.1016/j.pbi.2017.04.018
Gadhave, K. R., Devlin, P. F., Ebertz, A., Ross, A., and Gange, A. C. (2018). Soil inoculation with Bacillus spp. Modifies root endophytic bacterial diversity, evenness, and community composition in a context-specific manner. Microb. Ecol. 76, 741–750. doi: 10.1007/s00248-018-1160-x
Gaind, S., Rathi, M. S., Kaushik, B. D., Nain, L., and Verma, O. P. (2007). Survival of bio-inoculants on fungicides-treated seeds of wheat, pea and chickpea and subsequent effect on chickpea yield. J. Environ. Sci. Heal. Part B 42, 663–668. doi: 10.1080/03601230701465759
Gangaraddi, V., and Brahmaprakash, G. (2018a). Evaluation of selected microbial consortium formulations on growth of green gram (Vigna radiata L.). Int. J. Chem. Stud. 6, 1909–1913.
Gangaraddi, V., and Brahmaprakash, G. P. (2018b). Comparative evaluation of selected formulations of a microbial consortium. Mysore J. Agric. Sci. 52, 255–262.
Gao, G., Yin, D., Chen, S., Xia, F., Yang, J., Li, Q., et al. (2012). Effect of biocontrol agent Pseudomonas fluorescens 2P24 on soil fungal community in cucumber rhizosphere using T-RFLP and DGGE. PLoS ONE 7:e31806. doi: 10.1371/journal.pone.0031806
Garcha, S., and Maan, P. K. (2017). “Biological nitrogen fixation in cereals crops: a bacterial perspective,” in Advances in Soil Microbiology: Recent Trends and Future Prospects, eds T. K. Adhya, K. Annapurna, B. B. Mishra, U. Kumar, and D. K. Verma (Springer), 127–153. doi: 10.1007/978-981-10-7380-9
García de Salamone, I. E., Esquivel-Cote, R., Hernández-Melchor, D. J., and Alarcón, A. (2019). “Manufacturing and quality control of inoculants from the paradigm of circular agriculture,” in Microbial Interventions in Agriculture and Environment (Singapore: Springer), 37–74. doi: 10.1007/978-981-13-8383-0_2
García, J. E., Maroniche, G., Creus, C., Suárez-Rodríguez, R., Ramirez-Trujillo, J. A., and Groppa, M. D. (2017). In vitro PGPR properties and osmotic tolerance of different Azospirillum native strains and their effects on growth of maize under drought stress. Microbiol. Res. 202, 21–29. doi: 10.1016/j.micres.2017.04.007
Gasperini, L., Mano, J. F., and Reis, R. L. (2014). Natural polymers for the microencapsulation of cells. J. R. Soc. Interface 11:20140817. doi: 10.1098/rsif.2014.0817
Germida, J. J., and Janzen, H. H. (1993). Factors affecting the oxidation of elemental sulfur in soils. Fertil. Res. 35, 101–114. doi: 10.1007/BF00750224
Glick, B. R. (2015). Beneficial Plant-Bacterial Interactions, 1st Edn. Cham: Springer International Publishing. doi: 10.1007/978-3-319-13921-0
Glick, B. R. (2020). Beneficial Plant-Bacterial Interactions, 2nd Edn. Cham: Springer International Publishing. doi: 10.1007/978-3-030-44368-9
Glyan'ko, A. K., Vasil'eva, G. G., Mitanova, N. B., and Ishchenko, A. A. (2009). The influence of mineral nitrogen on legume-rhizobium symbiosis. Biol. Bull. 36, 250–258. doi: 10.1134/S1062359009030054
Goetten, L. C., Moretto, G., and Stürmer, S. L. (2016). Influence of arbuscular mycorrhizal fungi inoculum produced on-farm and phosphorus on growth and nutrition of native woody plant species from Brazil. Acta Bot. Bras. 30, 9–16. doi: 10.1590/0102-33062015abb0175
Gomez, M., Silva, N., Hartmann, A., Sagardoy, M., and Catroux, G. (1997). Evaluation of commercial soybean inoculants from Argentina. World J. Microbiol. Biotechnol. 13, 167–173. doi: 10.1023/A:1018533629378
Gotor-Vila, A., Usall, J., Torres, R., Abadias, M., and Teixidó, N. (2017). Formulation of the biocontrol agent Bacillus amyloliquefaciens CPA-8 using different approaches: liquid, freeze-drying and fluid-bed spray-drying. Biocontrol 62, 545–555. doi: 10.1007/s10526-017-9802-3
Grayston, S. J., Nevell, W., and Wainwright, M. (1986). Sulphur oxidation by fungi. Trans. Br. Mycol. Soc. 87, 193–198. doi: 10.1016/S0007-1536(86)80020-1
Greffe, V. R. G., and Michiels, J. (2020). Desiccation-induced cell damage in bacteria and the relevance for inoculant production. Appl. Microbiol. Biotechnol. 104, 3757–3770. doi: 10.1007/s00253-020-10501-6
Greger, M., Landberg, T., and Vaculík, M. (2018). Silicon influences soil availability and accumulation of mineral nutrients in various plant species. Plants 7:41. doi: 10.3390/plants7020041
Gupta, V., and Paterson, J. (2006). Free-living bacteria lift soil nitrogen supply. Farm. Ahead 169:40.
Guttmann, D. M., and Ellar, D. J. (2000). Phenotypic and genotypic comparisons of 23 strains from the Bacillus cereus complex for a selection of known and putative B. thuringiensis virulence factors. FEMS Microbiol. Lett. 188, 7–13. doi: 10.1111/j.1574-6968.2000.tb09160.x
Hart, M. M., Antunes, P. M., Chaudhary, V. B., and Abbott, L. K. (2018). Fungal inoculants in the field: is the reward greater than the risk? Funct. Ecol. 32, 126–135. doi: 10.1111/1365-2435.12976
Hassani, M. A., Durán, P., and Hacquard, S. (2018). Microbial interactions within the plant holobiont. Microbiome 6:58. doi: 10.1186/s40168-018-0445-0
He, C., Wang, W., and Hou, J. (2019a). Characterization of dark septate endophytic fungi and improve the performance of liquorice under organic residue treatment. Front. Microbiol. 10:1364. doi: 10.3389/fmicb.2019.01364
He, C., Wang, W., and Hou, J. (2019b). Plant growth and soil microbial impacts of enhancing licorice with inoculating dark septate endophytes under drought stress. Front. Microbiol. 10:2277. doi: 10.3389/fmicb.2019.02277
He, Y., Wu, Z., Ye, B. C., Wang, J., Guan, X., and Zhang, J. (2016). Viability evaluation of alginate-encapsulated Pseudomonas putida Rs-198 under simulated salt-stress conditions and its effect on cotton growth. Eur. J. Soil Biol. 75, 135–141. doi: 10.1016/j.ejsobi.2016.05.002
Herrmann, L., Atieno, M., Brau, L., and Lesueur, D. (2015). “Microbial quality of commercial inoculants to increase BNF and nutrient use efficiency,” in Biological Nitrogen Fixation, ed F. J. de Bruijn (Hoboken, NJ: John Wiley and Sons, Inc.), 1031–1040. doi: 10.1002/9781119053095.ch101
Herrmann, L., and Lesueur, D. (2013). Challenges of formulation and quality of biofertilizers for successful inoculation. Appl. Microbiol. Biotechnol. 97, 8859–8873. doi: 10.1007/s00253-013-5228-8
Herschkovitz, Y., Lerner, A., Davidov, Y., Okon, Y., and Jurkevitch, E. (2005). Azospirillum brasilense does not affect population structure of specific rhizobacterial communities of inoculated maize (Zea mays). Environ. Microbiol. 7, 1847–1852. doi: 10.1111/j.1462-2920.2005.00926.x
Hirsch, A. M., Bauer, W. D., Bird, D. M., Cullimore, J., Tyler, B., and Yoder, J. I. (2003). Molecular signals and receptors: controlling rhizosphere interactions between plants and other organisms. Ecology 84, 858–868. doi: 10.1890/0012-9658(2003)0840858:MSARCR2.0.CO
Hirsch, P. R. (2004). Release of transgenic bacterial inoculants - rhizobia as a case study. Plant Soil 266, 1–10. doi: 10.1007/s11104-005-4992-3
Hodge, A., and Fitter, A. H. (2010). Substantial nitrogen acquisition by arbuscular mycorrhizal fungi from organic material has implications for N cycling. Proc. Natl. Acad. Sci. U.S.A. 107, 13754–13759. doi: 10.1073/pnas.1005874107
Hoeksema, J. D., Chaudhary, V. B., Gehring, C. A., Johnson, N. C., Karst, J., Koide, R. T., et al. (2010). A meta-analysis of context-dependency in plant response to inoculation with mycorrhizal fungi. Ecol. Lett. 13, 394–407. doi: 10.1111/j.1461-0248.2009.01430.x
Howarth, R. (2009). “Nitrogen,” in Encyclopedia of Inland Waters, ed G. E. Likens (New York, NY: Academic Press), 57–64. doi: 10.1016/B978-012370626-3.00098-3
Huang, A. C., Jiang, T., Liu, Y.-X., Bai, Y.-C., Reed, J., Qu, B., et al. (2019). A specialized metabolic network selectively modulates Arabidopsis root microbiota. Science 364:eaau6389. doi: 10.1126/science.aau6389
Hurek, T., Handley, L. L., Reinhold-Hurek, B., and Piché, Y. (2002). Azoarcus grass endophytes contribute fixed nitrogen to the plant in an unculturable state. Mol. Plant Microbe Interact. 15, 233–242. doi: 10.1094/MPMI.2002.15.3.233
Jangir, M., Sharma, S., and Sharma, S. (2019). “Non-target effects of trichoderma on plants and soil microbial communities,” in Plant Microbe Interface, eds A. Varma, S. Tripathi, and R. Prasad (Cham: Springer International Publishing), 239–251. doi: 10.1007/978-3-030-19831-2_10
Jansa, J., Oberholzer, H. R., and Egli, S. (2009). Environmental determinants of the arbuscular mycorrhizal fungal infectivity of Swiss agricultural soils. Eur. J. Soil Biol. 45, 400–408. doi: 10.1016/j.ejsobi.2009.07.004
Jin, H., Germida, J. J., and Walley, F. L. (2013a). Impact of arbuscular mycorrhizal fungal inoculants on subsequent arbuscular mycorrhizal fungi colonization in pot-cultured field pea (Pisum sativum L.). Mycorrhiza 23, 45–59. doi: 10.1007/s00572-012-0448-9
Jin, H., Germida, J. J., and Walley, F. L. (2013b). Suppressive effects of seed-applied fungicides on arbuscular mycorrhizal fungi (AMF) differ with fungicide mode of action and AMF species. Appl. Soil Ecol. 72, 22–30. doi: 10.1016/j.apsoil.2013.05.013
Kaeberlein, T. (2002). Isolating “uncultivable” microorganisms in pure culture in a simulated natural environment. Science 296, 1127–1129. doi: 10.1126/science.1070633
Kaeppler, S. M., Parke, J. L., Mueller, S. M., Senior, L., Stuber, C., and Tracy, W. F. (2000). Variation among maize inbred lines and detection of quantitative trait loci for growth at low phosphorus and responsiveness to arbuscular mycorrhizal fungi. Crop Sci. 40, 358–364. doi: 10.2135/cropsci2000.402358x
Kamaei, R., Faramarzi, F., Parsa, M., and Jahan, M. (2019). The effects of biological, chemical, and organic fertilizers application on root growth features and grain yield of Sorghum. J. Plant Nutr. 42, 2221–2233. doi: 10.1080/01904167.2019.1648667
Kaminsky, L. M., Trexler, R. V., Malik, R. J., Hockett, K. L., and Bell, T. H. (2019). The inherent conflicts in developing soil microbial inoculants. Trends Biotechnol. 37, 140–151. doi: 10.1016/j.tibtech.2018.11.011
Kaul, S., Sharma, T. K., and Dhar, M. (2016). “Omics” tools for better understanding the plant–endophyte interactions. Front. Plant Sci. 7:955. doi: 10.3389/fpls.2016.00955
Keswani, C., Prakash, O., Bharti, N., Vílchez, J. I., Sansinenea, E., Lally, R. D., et al. (2019). Re-addressing the biosafety issues of plant growth promoting rhizobacteria. Sci. Total Environ. 690, 841–852. doi: 10.1016/j.scitotenv.2019.07.046
Kobayashi, T. (2018). “Cellulose hydrogels; fabrication, properties, and their application to biocompatible and tissue engineering,” in Hydrogels Gels Horizons: From Science to Smart Materials, eds V. K. Thakur and M. K. Thakur (Singapore: Springer). doi: 10.1007/978-981-10-6077-9
Koch, A. M., Antunes, P. M., Barto, E. K., Cipollini, D., Mummey, D. L., and Klironomos, J. N. (2011). The effects of arbuscular mycorrhizal (AM) fungal and garlic mustard introductions on native AM fungal diversity. Biol. Invasions 13, 1627–1639. doi: 10.1007/s10530-010-9920-7
Kogel, K.-H., Franken, P., and Hückelhoven, R. (2006). Endophyte or parasite – what decides? Curr. Opin. Plant Biol. 9, 358–363. doi: 10.1016/j.pbi.2006.05.001
Koide, R. T., and Mosse, B. (2004). A history of research on arbuscular mycorrhiza. Mycorrhiza 14, 145–163. doi: 10.1007/s00572-004-0307-4
Kong, Z., Hart, M., and Liu, H. (2018). Paving the way from the lab to the field: using synthetic microbial consortia to produce high-quality crops. Front. Plant Sci. 9:1467. doi: 10.3389/fpls.2018.01467
Koprivova, A., Schuck, S., Jacoby, R. P., Klinkhammer, I., Welter, B., Leson, L., et al. (2019). Root-specific camalexin biosynthesis controls the plant growth-promoting effects of multiple bacterial strains. Proc. Natl. Acad. Sci. U.S.A. 116, 15735–15744. doi: 10.1073/pnas.1818604116
Kopycińska, M., Lipa, P., Cieśla, J., Kozieł, M., and Janczarek, M. (2018). Extracellular polysaccharide protects Rhizobium leguminosarum cells against zinc stress in vitro and during symbiosis with clover. Environ. Microbiol. Rep. 10, 355–368. doi: 10.1111/1758-2229.12646
Kour, D., Lata, K., Kaur, T., Yadav, N., Kumar, S., Nath, A., et al. (2020). “Potassium solubilizing and mobilizing microbes: biodiversity, mechanisms of solubilization, and biotechnological implication for alleviations of abiotic stress,” in Trends of Microbial Biotechnology for Sustainable Agriculture and Biomedicine Systems: Diversity and Functional Perspective, ed V. K. Gupta (Amsterdam: Elsevier Inc.), 177–202. doi: 10.1016/B978-0-12-820526-6.00012-9
Koyama, A., Pietrangelo, O., Sanderson, L., and Antunes, P. M. (2017). An empirical investigation of the possibility of adaptability of arbuscular mycorrhizal fungi to new hosts. Mycorrhiza 27, 553–563. doi: 10.1007/s00572-017-0776-x
Kumar, S. M., Reddy, C. G., Phogat, M., and Korav, S. (2018). Role of bio-fertilizers towards sustainable agricultural development: a review. J. Pharmacogn. Phytochem. 7, 1915–1921.
Kushwaha, P., Kashyap, P. L., Pandiyan, K., and Bhardwaj, A. K. (2020). “Zinc-solubilizing microbes for sustainable crop production: current understanding, opportunities, and challenges.,” in Phytobiomes: Current Insights and Future Vistas, eds M. Solanki and P. Kashyap (Singapore: Springer) 281–298.
Kyei-Boahen, S., Nleya, T., Hynes, R., and Walley, F. L. (2005). Single and multistrain rhizobial inocula for pinto and black bean cultivars. J. Plant Nutr. 28, 1679–1692. doi: 10.1080/01904160500250664
Lagier, J.-C., Dubourg, G., Million, M., Cadoret, F., Bilen, M., Fenollar, F., et al. (2018). Culturing the human microbiota and culturomics. Nat. Rev. Microbiol. 16, 540–550. doi: 10.1038/s41579-018-0041-0
Lagier, J.-C., Hugon, P., Khelaifia, S., Fournier, P.-E., La Scola, B., and Raoult, D. (2015). The rebirth of culture in microbiology through the example of culturomics to study human gut microbiota. Clin. Microbiol. Rev. 28, 237–264. doi: 10.1128/CMR.00014-14
Lagier, J.-C., Khelaifia, S., Alou, M. T., Ndongo, S., Dione, N., Hugon, P., et al. (2016). Culture of previously uncultured members of the human gut microbiota by culturomics. Nat. Microbiol. 1:16203. doi: 10.1038/nmicrobiol.2016.203
Larena, I., De Cal, A., Linan, M., and Melgarejo, P. (2003). Drying of Epicoccum nigrum conidia for obtaining a shelf-stable biological product against brown rot disease. J. Appl. Microbiol. 94, 508–514. doi: 10.1046/j.1365-2672.2003.01860.x
Lawson, C. E., Harcombe, W. R., Hatzenpichler, R., Lindemann, S. R., Löffler, F. E., O'Malley, M. A., et al. (2019). Common principles and best practices for engineering microbiomes. Nat. Rev. Microbiol. 17, 725–741. doi: 10.1038/s41579-019-0255-9
Lebeis, S. L., Paredes, S. H., Lundberg, D. S., Breakfield, N., Gehring, J., McDonald, M., et al. (2015). Salicylic acid modulates colonization of the root microbiome by specific bacterial taxa. Science 349, 860–864. doi: 10.1126/science.aaa8764
Lee, K. E., Adhikari, A., Kang, S. M., You, Y. H., Joo, G. J., Kim, J. H., et al. (2019). Isolation and characterization of the high silicate and phosphate solubilizing novel strain Enterobacter ludwigii GAK2 that promotes growth in rice plants. Agronomy 9:144. doi: 10.3390/agronomy9030144
Lee, K. Y., and Mooney, D. J. (2012). Alginate: properties and biomedical applications. Prog. Polym. Sci. 37, 106–126. doi: 10.1016/j.progpolymsci.2011.06.003
Lee, S. K., Lur, H. S., Lo, K. J., Cheng, K. C., Chuang, C. C., Tang, S. J., et al. (2016). Evaluation of the effects of different liquid inoculant formulations on the survival and plant-growth-promoting efficiency of Rhodopseudomonas palustris strain PS3. Appl. Microbiol. Biotechnol. 100, 7977–7987. doi: 10.1007/s00253-016-7582-9
Lerner, A., Herschkovitz, Y., Baudoin, E., Nazaret, S., Moenne-Loccoz, Y., Okon, Y., et al. (2006). Effect of Azospirillum brasilense inoculation on rhizobacterial communities analyzed by denaturing gradient gel electrophoresis and automated ribosomal intergenic spacer analysis. Soil Biol. Biochem. 38, 1212–1218. doi: 10.1016/j.soilbio.2005.10.007
Li, Y.-H., and Tian, X. (2012). Quorum sensing and bacterial social interactions in biofilms. Sensors 12, 2519–2538. doi: 10.3390/s120302519
Linderman, R. G., and Davis, E. A. (2004). Varied response of marigold (Tagetes spp.) genotypes to inoculation with different arbuscular mycorrhizal fungi. Sci. Hortic. 99, 67–78. doi: 10.1016/S0304-4238(03)00081-5
Liu, A., Hamel, C., Hamilton, R. I., and Smith, D. L. (2000). Mycorrhizae formation and nutrient uptake of new corn (Zea mays L.) hybrids with extreme canopy and leaf architecture as influenced by soil N and P levels. Plant Soil 221, 157–166. doi: 10.1023/A:1004777821422
Liu, N., Shao, C., Sun, H., Liu, Z., Guan, Y., Wu, L., et al. (2020). Arbuscular mycorrhizal fungi biofertilizer improves American ginseng (Panax quinquefolius L.) growth under the continuous cropping regime. Geoderma 363, 114155. doi: 10.1016/j.geoderma.2019.114155
Lopes, A. R., de, O., Locatelli, G. O., Barbosa, R., de, M., Lobo, M. Junior, et al. (2020). Preparation, characterisation and cell viability of encapsulated Trichoderma asperellum in alginate beads. J. Microencapsul. 37, 270–282. doi: 10.1080/02652048.2020.1729884
Lurthy, T., Cantat, C., Jeudy, C., Declerck, P., Gallardo, K., Barraud, C., et al. (2020). Impact of bacterial siderophores on iron status and ionome in pea. Front. Plant Sci. 11:730. doi: 10.3389/fpls.2020.00730
Macik, M., Gryta, A., and Frac, M. (2020). Biofertilizers in agriculture: an overview on concepts, strategies and effects on soil microorganisms. Adv. Agron. 162, 31–87. doi: 10.1016/bs.agron.2020.02.001
Mahmoud, R. S., and Narisawa, K. (2013). A new fungal endophyte, Scolecobasidium humicola, promotes tomato growth under organic nitrogen conditions. PLoS ONE 8:e78746. doi: 10.1371/journal.pone.0078746
Maiti, D., Toppo, N. N., and Variar, M. (2011). Integration of crop rotation and arbuscular mycorrhiza (AM) inoculum application for enhancing AM activity to improve phosphorus nutrition and yield of upland rice (Oryza sativa L.). Mycorrhiza 21, 659–667. doi: 10.1007/s00572-011-0376-0
Maltz, M. R., and Treseder, K. K. (2015). Sources of inocula influence mycorrhizal colonization of plants in restoration projects: a meta-analysis. Restor. Ecol. 23, 625–634. doi: 10.1111/rec.12231
Malusà, E., Pinzari, F., and Canfora, L. (2016). “Efficacy of biofertilizers: challenges to improve crop production,” in Microbial Inoculants in Sustainable Agricultural Productivity, eds D. P. Singh, H. B. Singh and R. Prabha (New Delhi: Springer), 17–40. doi: 10.1007/978-81-322-2644-4_2
Manandhar, S., Tuladhar, R., Prajapati, K., Singh, A., and Varma, A. (2017). “Effect of Azotobacter chroococcum and Piriformospora indica on Oryza sativa in presence of vermicompost,” in Mycorrhiza - Nutrient Uptake, Biocontrol, Ecorestoration, eds A. Varma, R. Prasad and N. Tuteja (Cham: Springer International Publishing), 327–339. doi: 10.1007/978-3-319-68867-1_18
Mandyam, K., and Jumpponen, A. (2005). Seeking the elusive function of the root-colonising dark septate endophytic fungi. Stud. Mycol. 53, 173–189. doi: 10.3114/sim.53.1.173
Mar Vázquez, M., César, S., Azcón, R., and Barea, J. M. (2000). Interactions between arbuscular mycorrhizal fungi and other microbial inoculants (Azospirillum, Pseudomonas, Trichoderma) and their effects on microbial population and enzyme activities in the rhizosphere of maize plants. Appl. Soil Ecol. 15, 261–272. doi: 10.1016/S0929-1393(00)00075-5
Marketandmarkets (2020). Biofertilizers Market by Form (Liquid, Carrier-Based), Mode of Application (Soil Treatment, Seed Treatment), Crop Type, Type (Nitrogen-Fixing, Phosphate Solubilizing and Mobilizing, Potash Solubilizing and Mobilizing), Dallas, TX: Region – Global Forecast to 2025.
Marschner, P., and Timonen, S. (2005). Interactions between plant species and mycorrhizal colonization on the bacterial community composition in the rhizosphere. Appl. Soil Ecol. 28, 23–36. doi: 10.1016/j.apsoil.2004.06.007
Martínez-Hidalgo, P., Maymon, M., Pule-Meulenberg, F., and Hirsch, A. M. (2018). Engineering root microbiomes for healthier crops and soils using beneficial, environmentally safe bacteria. Can. J. Microbiol. 65, 91–104. doi: 10.1139/cjm-2018-0315
Masson-Boivin, C., and Sachs, J. L. (2018). Symbiotic nitrogen fixation by rhizobia — the roots of a success story. Curr. Opin. Plant Biol. 44, 7–15. doi: 10.1016/j.pbi.2017.12.001
Matos, A., Kerkhof, L., and Garland, J. L. (2005). Effects of microbial community diversity on the survival of Pseudomonas aeruginosa in the wheat rhizosphere. Microb. Ecol. 49, 257–264. doi: 10.1007/s00248-004-0179-3
Mawarda, P. C., Le Roux, X., Dirk van Elsas, J., and Salles, J. F. (2020). Deliberate introduction of invisible invaders: a critical appraisal of the impact of microbial inoculants on soil microbial communities. Soil Biol. Biochem. 148:107874. doi: 10.1016/j.soilbio.2020.107874
Melchiorre, M., de Luca, M. J., Anta, G. G., Suarez, P., Lopez, C., Lascano, R., et al. (2011). Evaluation of Bradyrhizobia strains isolated from field-grown soybean plants in Argentina as improved inoculants. Biol. Fertil. Soils 47, 81–89. doi: 10.1007/s00374-010-0503-7
Mendes, R., Garbeva, P., and Raaijmakers, J. M. (2013). The rhizosphere microbiome: significance of plant beneficial, plant pathogenic, and human pathogenic microorganisms. FEMS Microbiol. Rev. 37, 634–663. doi: 10.1111/1574-6976.12028
Menéndez, E., and Paço, A. (2020). Is the application of plant probiotic bacterial consortia always beneficial for plants? Exploring synergies between rhizobial and non-rhizobial bacteria and their effects on agro-economically valuable crops. Life 10, 1–18. doi: 10.3390/life10030024
Mezei, S., Popović, M., Kovačev, L., Mrkovački, N., Nagl, N., and Malenčić, D. (1997). Effect of Azotobacter strains on sugar beet callus proliferation and nitrogen metabolism enzymes. Biol. Plant. 40, 277–283. doi: 10.1023/A:1001028922433
Miller, M. H., McGonigle, T. P., and Addy, H. D. (1995). Functional ecology of vesicular arbuscular mycorrhizas as influenced by phosphate fertilization and tillage in an agricultural ecosystem. Crit. Rev. Biotechnol. 15, 241–255. doi: 10.3109/07388559509147411
Mishra, J., and Arora, N. K. (2016). “Bioformulations for plant growth promotion and combating phytopathogens: a sustainable approach,” in Bioformulations: for Sustainable Agriculture, eds N. K. Arora, S. Mehnaz, and R. Balestrini (New Delhi: Springer), 3–33. doi: 10.1007/978-81-322-2779-3_1
Mitter, B., Sessitsch, A., and Naveed, M. (2013). Method for Producing Plant Seed Containing Endophytic Micro-Organisms. U.S. Patent No US 2015/0335029 A1. Washington, DC: U.S. Patent and Trademark Office.
Mitter, E. K., de Freitas, J. R., and Germida, J. J. (2017). Bacterial root microbiome of plants growing in oil sands reclamation covers. Front. Microbiol. 8:849. doi: 10.3389/fmicb.2017.00849
Mondal, P., and Dalai, A. K., (eds.). (2017). Sustainable Utilization of Natural Resources. Boca Raton, FL: CRC Press; Taylor and Francis. doi: 10.1201/9781315153292
Monokrousos, N., Papatheodorou, E. M., Orfanoudakis, M., Jones, D. G., Scullion, J., and Stamou, G. P. (2020). The effects of plant type, AMF inoculation and water regime on rhizosphere microbial communities. Eur. J. Soil Sci. 71, 265–278. doi: 10.1111/ejss.12882
Moreira-Coello, V., Mouriño-Carballido, B., Marañón, E., Fernández-Carrera, A., Bode, A., Sintes, E., et al. (2019). Temporal variability of diazotroph community composition in the upwelling region off NW Iberia. Sci. Rep. 9:3737. doi: 10.1038/s41598-019-39586-4
Mourad, E. F., Sarhan, M. S., Daanaa, H.-S. A., Abdou, M., Morsi, A. T., Abdelfadeel, M. R., et al. (2018). Plant materials are sustainable substrates supporting new technologies of plant-only-based culture media for in vitro culturing of the plant microbiota. Microbes Environ. 33, 40–49. doi: 10.1264/jsme2.ME17135
Moutia, J. F. Y., Saumtally, S., Spaepen, S., and Vanderleyden, J. (2010). Plant growth promotion by Azospirillum sp. in sugarcane is influenced by genotype and drought stress. Plant Soil 337, 233–242. doi: 10.1007/s11104-010-0519-7
Mulas, D., Seco, V., Casquero, P. A., Velázquez, E., and González-Andrés, F. (2015). Inoculation with indigenous rhizobium strains increases yields of common bean (Phaseolus vulgaris L.) in northern Spain, although its efficiency is affected by the tillage system. Symbiosis 67, 113–124. doi: 10.1007/s13199-015-0359-6
Muñoz-Rojas, J., and Caballero-Mellado, J. (2003). Population dynamics of Gluconacetobacter diazotrophicus in sugarcane cultivars and its effect on plant growth. Microb. Ecol. 46, 454–464. doi: 10.1007/s00248-003-0110-3
Murphy, B., Doohan, F., and Hodkinson, T. (2018). From concept to commerce: developing a successful fungal endophyte inoculant for agricultural crops. J. Fungi 4:24. doi: 10.3390/jof4010024
Nagata, T. (2017). Effect of Pseudomonas fluorescens inoculation on the improvement of iron deficiency in tomato. Plant Root 11, 1–9. doi: 10.3117/plantroot.11.1
Nayak, S. K., and Mishra, B. B. (2020). Frontiers in Soil and Environmental Microbiology. Boca Raton, FL: CRC Press. doi: 10.1201/9780429485794
Nayak, S. K., Nayak, S., and Patra, J. K. (2020). “Rhizobacteria and its biofilm for sustainable agriculture: a concise review,” in New and Future Developments in Microbial Biotechnology and Bioengineering: Microbial Biofilms (Amsterdam: Elsevier), 165–175. doi: 10.1016/B978-0-444-64279-0.00013-X
Neal, A. L., Ahmad, S., Gordon-Weeks, R., and Ton, J. (2012). Benzoxazinoids in root exudates of maize attract Pseudomonas putida to the rhizosphere. PLoS ONE 7:e35498. doi: 10.1371/journal.pone.0035498
Nichols, D., Cahoon, N., Trakhtenberg, E. M., Pham, L., Mehta, A., Belanger, A., et al. (2010). Use of ichip for high-throughput in situ cultivation of “uncultivable” microbial species. Appl. Environ. Microbiol. 76, 2445–2450. doi: 10.1128/AEM.01754-09
Nkebiwe, P. M., Weinmann, M., and Müller, T. (2016). Improving fertilizer-depot exploitation and maize growth by inoculation with plant growth-promoting bacteria: from lab to field. Chem. Biol. Technol. Agric. 3, 1–16. doi: 10.1186/s40538-016-0065-5
Noirot-Gros, M.-F., Shinde, S., Larsen, P. E., Zerbs, S., Korajczyk, P. J., Kemner, K. M., et al. (2018). Dynamics of aspen roots colonization by pseudomonads reveals strain-specific and mycorrhizal-specific patterns of biofilm formation. Front. Microbiol. 9:853. doi: 10.3389/fmicb.2018.00853
Oberhardt, M. A., Zarecki, R., Gronow, S., Lang, E., Klenk, H.-P., Gophna, U., et al. (2015). Harnessing the landscape of microbial culture media to predict new organism–media pairings. Nat. Commun. 6:8493. doi: 10.1038/ncomms9493
Olanrewaju, O. S., and Babalola, O. O. (2019). Streptomyces: implications and interactions in plant growth promotion. Appl. Microbiol. Biotechnol. 103, 1179–1188. doi: 10.1007/s00253-018-09577-y
Oren, A., and Garrity, G. M. (2014). Then and now: a systematic review of the systematics of prokaryotes in the last 80 years. Antonie Van Leeuwenhoek 106, 43–56. doi: 10.1007/s10482-013-0084-1
Ortas, I. (2003). Effect of selected mycorrhizal inoculation on phosphorus sustainability in sterile and non-sterile soils in the harran plain in South Anatolia. J. Plant Nutr. 26, 1–17. doi: 10.1081/PLN-120016494
Ozturk, A., Caglar, O., and Sahin, F. (2003). Yield response of wheat and barley to inoculation of plant growth promoting rhizobacteria at various levels of nitrogen fertilization. J. Plant Nutr. Soil Sci. 166, 262–266. doi: 10.1002/jpln.200390038
Parnell, J. J., Berka, R., Young, H. A., Sturino, J. M., Kang, Y., Barnhart, D. M., et al. (2016). From the lab to the farm: an industrial perspective of plant beneficial microorganisms. Front. Plant Sci. 7:1110. doi: 10.3389/fpls.2016.01110
Parween, T., Bhandari, P., Siddiqui, Z. H., Jan, S., Fatma, T., and Patanjali, P. K. (2017). “Biofilm: a next-generation biofertilizer,” in Mycoremediation and Environmental Sustainability, ed R. Prasad (Cham: Fungal Biology; Springer), 39–51. doi: 10.1007/978-3-319-68957-9_3
Pascale, A., Proietti, S., Pantelides, I. S., and Stringlis, I. A. (2020). Modulation of the root microbiome by plant molecules: the basis for targeted disease suppression and plant growth promotion. Front. Plant Sci. 10:1741. doi: 10.3389/fpls.2019.01741
Paul, E. A. (2015). Soil Microbiology, Ecology and Biochemistry. London: Elsevier. doi: 10.1016/C2011-0-05497-2
Pereg, L., De-Bashan, L. E., and Bashan, Y. (2016). Assessment of affinity and specificity of Azospirillum for plants. Plant Soil 399, 389–414. doi: 10.1007/s11104-015-2778-9
Pérez-Luna, V., and González-Reynoso, O. (2018). Encapsulation of biological agents in hydrogels for therapeutic applications. Gels 4:61. doi: 10.3390/gels4030061
Peukert, S., Griffith, B. A., Murray, P. J., Macleod, C. J. A., and Brazier, R. E. (2016). Spatial variation in soil properties and diffuse losses between and within grassland fields with similar short-term management. Eur. J. Soil Sci. 67, 386–396. doi: 10.1111/ejss.12351
Philippot, L., Raaijmakers, J. M., Lemanceau, P., and van der Putten, W. H. (2013). Going back to the roots: the microbial ecology of the rhizosphere. Nat. Rev. Microbiol. 11, 789–799. doi: 10.1038/nrmicro3109
Pii, Y., Aldrighetti, A., Valentinuzzi, F., Mimmo, T., and Cesco, S. (2019). Azospirillum brasilense inoculation counteracts the induction of nitrate uptake in maize plants. J. Exp. Bot. 70, 1313–1324. doi: 10.1093/jxb/ery433
Pitaktamrong, P., Kingkaew, J., Yooyongwech, S., Cha-um, S., and Phisalaphong, M. (2018). Development of arbuscular mycorrhizal fungi-organic fertilizer pellets encapsulated with alginate film. Eng. J. 22, 65–79. doi: 10.4186/ej.2018.22.6.65
Pradhan, A., Pahari, A., Mohapatra, S., and Mishra, B. B. (2017). “Phosphate-solubilizing microorganisms in sustainable agriculture: genetic mechanism and application,” in Advances in Soil Microbiology: Recent Trends and Future Prospects, eds T. K. Adhya, B. B. Mishra, K. Annapurna, D. K. Verma, and U. Kumar (Singapore: Springer) 81–97.
Prasad, R., Shivay, Y., and Kumar, D. (2016). Interactions of zinc with other nutrients in soils and plants-a review. Indian J. Fertil. 16, 16–26. doi: 10.1007/978-94-011-0878-2_9
Preininger, C., Sauer, U., Bejarano, A., and Berninger, T. (2018). Concepts and applications of foliar spray for microbial inoculants. Appl. Microbiol. Biotechnol. 102, 7265–7282. doi: 10.1007/s00253-018-9173-4
Pujar, A. M., Kumar, B. N. A., and Geeta, G. S. (2014). Response of Sunflower (Helianthus annuus L.) to gradedlevels of Sulphur and sulphur oxidising biofertilizer (Thiobacillus Thiooxidans). Biochem. Cell. Arch. 14, 339–342.
Qiu, Z., Egidi, E., Liu, H., Kaur, S., and Singh, B. K. (2019). New frontiers in agriculture productivity: optimised microbial inoculants and in situ microbiome engineering. Biotechnol. Adv. 37:107371. doi: 10.1016/j.biotechadv.2019.03.010
Raaijmakers, J. M. (2015). “The Minimal Rhizosphere Microbiome,” in Principles of Plant-Microbe Interactions, ed B. Lugtenberg (Cham: Springer International Publishing), 411–417. doi: 10.1007/978-3-319-08575-3_43
Raghothama, K. G. (2015). “Phosphorus and plant nutrition: an overview,” in In Phosphorus: Agriculture and the Environment, eds T. Sims, A. N. Sharpley, and M. L. Cabrera (Madison, WI: American Society of Agronomy; Crop Science Society of America; Soil Science Society of America), 2992–2999. doi: 10.2134/agronmonogr46.c11
Ramírez-López, C., Esparza-García, F. J., Ferrera-Cerrato, R., Alarcón, A., and Cañizares-Villanueva, R. O. (2019). Short-term effects of a photosynthetic microbial consortium and nitrogen fertilization on soil chemical properties, growth, and yield of wheat under greenhouse conditions. J. Appl. Phycol. 31, 3617–3624. doi: 10.1007/s10811-019-01861-2
Rana, K. L., Kour, D., Yadav, A. N., Yadav, N., and Saxena, A. K. (2020). “Agriculturally important microbial biofilms: biodiversity, ecological significances, and biotechnological applications,” in New and Future Developments in Microbial Biotechnology and Bioengineering: Microbial Biofilms, eds M. K. Yadav and B. P. Singh (Amsterdam: Elsevier), 221–265. doi: 10.1016/B978-0-444-64279-0.00016-5
Rashid, A., and Ryan, J. (2004). Micronutrient constraints to crop production in soils with mediterranean-type characteristics: a review. J. Plant Nutr. 27, 959–975. doi: 10.1081/PLN-120037530
Rawat, N., Sharma, M., Suyal, D. C., Singh, D. K., Joshi, D., Singh, P., et al. (2019). Psyhcrotolerant bio-inoculants and their co-inoculation to improve Cicer arietinum growth and soil nutrient status for sustainable mountain agriculture. J. Soil Sci. Plant Nutr. 19, 639–647. doi: 10.1007/s42729-019-00064-5
Reis, C. P., Neufeld, R. J., Vilela, S., Ribeiro, A. J., and Veiga, F. (2006). Review and current status of emulsion/dispersion technology using an internal gelation process for the design of alginate particles. J. Microencapsul. 23, 245–257. doi: 10.1080/02652040500286086
Rekadwad, B. N., and Khobragade, C. N. (2017). “Microbial biofilm: role in crop productivity,” in Microbial Applications Vol. 2, ed V. C. Kalia (Cham: Springer International Publishing), 107–118. doi: 10.1007/978-3-319-52669-0_5
Remans, R., Ramaekers, L., Schelkens, S., Hernandez, G., Garcia, A., Reyes, J. L., et al. (2008). Effect of Rhizobium–Azospirillum coinoculation on nitrogen fixation and yield of two contrasting Phaseolus vulgaris L. genotypes cultivated across different environments in Cuba. Plant Soil 312, 25–37. doi: 10.1007/s11104-008-9606-4
ReportLinker (2020). Mycorrhiza-Based Biofertilizer Market - Growth, Trends, and Forecast (2020 - 2025). 97.
Riaz, U., Mehdi, S. M., Iqbal, S., Khalid, H. I., Qadir, A. A., Anum, W., et al. (2020). “Bio-fertilizers: eco-friendly approach for plant and soil environment,” in Bioremediation and Biotechnology: Sustainable Approaches to Pollution Degradation, eds K. R. Hakeem, R. A. Bhat, and H. Qadri (Cham: Springer), 188–214. doi: 10.1007/978-3-030-35691-0_8
Ricci, E., Schwinghamer, T., Fan, D., Smith, D. L., and Gravel, V. (2019). Growth promotion of greenhouse tomatoes with Pseudomonas sp. and Bacillus sp. biofilms and planktonic cells. Appl. Soil Ecol. 138, 61–68. doi: 10.1016/j.apsoil.2019.02.009
Rivett, D. W., Jones, M. L., Ramoneda, J., Mombrikotb, S. B., Ransome, E., and Bell, T. (2018). Elevated success of multispecies bacterial invasions impacts community composition during ecological succession. Ecol. Lett. 21, 516–524. doi: 10.1111/ele.12916
Rodríguez Amor, D., and Dal Bello, M. (2019). Bottom-up approaches to synthetic cooperation in microbial communities. Life 9:22. doi: 10.3390/life9010022
Rojas, X., Guo, J., Leff, J. W., McNear, D. H., Fierer, N., and McCulley, R. L. (2016). Infection with a shoot-specific fungal endophyte (Epichloë) alters tall fescue soil microbial communities. Microb. Ecol. 72, 197–206. doi: 10.1007/s00248-016-0750-8
Rosas, S. B., Andrés, J. A., Rovera, M., and Correa, N. S. (2006). Phosphate-solubilizing Pseudomonas putida can influence the rhizobia–legume symbiosis. Soil Biol. Biochem. 38, 3502–3505. doi: 10.1016/j.soilbio.2006.05.008
Rroço, E., Kosegarten, H., Harizaj, F., Imani, J., and Mengel, K. (2003). The importance of soil microbial activity for the supply of iron to sorghum and rape. Eur. J. Agron. 19, 487–493. doi: 10.1016/S1161-0301(02)00185-5
Saad, M. M., Eida, A. A., and Hirt, H. (2020). Tailoring plant-associated microbial inoculants in agriculture: a roadmap for successful application. J. Exp. Bot. 71, 3878–3901. doi: 10.1093/jxb/eraa111
Sabuquillo, P., De Cal, A., and Melgarejo, P. (2006). Biocontrol of tomato wilt by Penicillium oxalicum formulations in different crop conditions. Biol. Control 37, 256–265. doi: 10.1016/j.biocontrol.2006.02.009
Safronova, V. I., Stepanok, V. V., Engqvist, G. L., Alekseyev, Y. V., and Belimov, A. A. (2006). Root-associated bacteria containing 1-aminocyclopropane-1-carboxylate deaminase improve growth and nutrient uptake by pea genotypes cultivated in cadmium supplemented soil. Biol. Fertil. Soils 42, 267–272. doi: 10.1007/s00374-005-0024-y
Saha, R., Saha, N., Donofrio, R. S., and Bestervelt, L. L. (2013). Microbial siderophores: a mini review. J. Basic Microbiol. 53, 303–317. doi: 10.1002/jobm.201100552
Sahu, P. K., and Brahmaprakash, G. P. (2016). “Formulations of biofertilizers – approaches and advances,” in Microbial Inoculants in Sustainable Agricultural Productivity, D. P. Singh, H. B. Singh and R. Prabha (New Delhi: Springer), 179–198. doi: 10.1007/978-81-322-2644-4_12
Sahu, P. K., Gupta, A., Singh, M., Mehrotra, P., and Brahmaprakash, G. P. (2018). “Bioformulation and fluid bed drying: a new approach towards an improved biofertilizer formulation,” in Eco-Friendly Agro-Biological Techniques for Enhancing Crop Productivity, eds R. S. Sengar and A. Singh (Singapore: Springer), 47–62. doi: 10.1007/978-981-10-6934-5_3
Saleem, M., Law, A. D., Sahib, M. R., Pervaiz, Z. H., and Zhang, Q. (2018). Impact of root system architecture on rhizosphere and root microbiome. Rhizosphere 6, 47–51. doi: 10.1016/j.rhisph.2018.02.003
Sammauria, R., Kumawat, S., Kumawat, P., Singh, J., and Jatwa, T. K. (2020). Microbial inoculants: potential tool for sustainability of agricultural production systems. Arch. Microbiol. 202, 677–693. doi: 10.1007/s00203-019-01795-w
Santi, C., Bogusz, D., and Franche, C. (2013). Biological nitrogen fixation in non-legume plants. Ann. Bot. 111, 743–767. doi: 10.1093/aob/mct048
Santi, L. P., and Goenadi, D. H. (2017). Solubilization of silicate from quartz mineral by potential silicate solubilizing bacteria. E J. Menara Perkeb. 85, 95–104. doi: 10.22302/iribb.jur.mp.v85i2.247
Santra, S. C., Mallick, A., and Samal, A. C. (2015). “Biofertilizer for bioremediation,” in Recent Trends in Biofertiizer, eds B. R. Pati, S. M. Mandal (New Delhi: IK International Publishing House Pvt), 205–234.
Sarhan, M. S., Hamza, M. A., Youssef, H. H., Patz, S., Becker, M., ElSawey, H., et al. (2019). Culturomics of the plant prokaryotic microbiome and the dawn of plant-based culture media – A review. J. Adv. Res. 19, 15–27. doi: 10.1016/j.jare.2019.04.002
Sarikhani, M. R., Aliasgharzad, N., and Khoshru, B. (2020). P solubilizing potential of some plant growth promoting bacteria used as ingredient in phosphatic biofertilizers with emphasis on growth promotion of Zea mays L. Geomicrobiol. J. 37, 327–335. doi: 10.1080/01490451.2019.1700323
Sasaki, K., Ikeda, S., Eda, S., Mitsui, H., Hanzawa, E., Kisara, C., et al. (2010). Impact of plant genotype and nitrogen level on rice growth response to inoculation with Azospirillum sp. strain B510 under paddy field conditions. Soil Sci. Plant Nutr. 56, 636–644. doi: 10.1111/j.1747-0765.2010.00499.x
Sasse, J., Martinoia, E., and Northen, T. (2018). Feed your friends: do plant exudates shape the root microbiome? Trends Plant Sci. 23, 25–41. doi: 10.1016/j.tplants.2017.09.003
Sattar, A., Naveed, M., Ali, M., Zahir, Z. A., Nadeem, S. M., Yaseen, M., et al. (2019). Perspectives of potassium solubilizing microbes in sustainable food production system: a review. Appl. Soil Ecol. 133, 146–159. doi: 10.1016/j.apsoil.2018.09.012
Satyanarayana, T., Das, S. K., and Johri, B. N., (eds.). (2019). Microbial Diversity in Ecosystem Sustainability and Biotechnological Applications. Singapore: Springer. doi: 10.1007/978-981-13-8315-1
Scherer, H. W. (2009). Sulfur in soils. J. Plant Nutr. Soil Sci. 172, 326–335. doi: 10.1002/jpln.200900037
Schlaeppi, K., and Bulgarelli, D. (2015). The Plant Microbiome at Work. Mol. Plant Microbe Interact. 28, 212–217. doi: 10.1094/MPMI-10-14-0334-FI
Schoebitz, M., López, M. D., and Roldán, A. (2013). Bioencapsulation of microbial inoculants for better soil–plant fertilization. A review. Agron. Sustain. Dev. 33, 751–765. doi: 10.1007/s13593-013-0142-0
Schütz, L., Gattinger, A., Meier, M., Müller, A., Boller, T., Mäder, P., et al. (2018). Improving crop yield and nutrient use efficiency via biofertilization—A global meta-analysis. Front. Plant Sci. 8:2204. doi: 10.3389/fpls.2017.02204
Schütz, V., Bigler, L., Girel, S., Laschke, L., Sicker, D., and Schulz, M. (2019). Conversions of benzoxazinoids and downstream metabolites by soil microorganisms. Front. Ecol. Evol. 7:238. doi: 10.3389/fevo.2019.00238
Schwieger, F., and Tebbe, C. C. (2000). Effect of field inoculation with Sinorhizobium meliloti L33 on the composition of bacterial communities in rhizospheres of a target plant (Medicago sativa) and a non-target plant (Chenopodium, album) - Linking of 16S rRNA gene-. Appl. Environ. Microbiol. 66, 3556–3565. doi: 10.1128/AEM.66.8.3556-3565.2000
Sessitsch, A., Pfaffenbichler, N., and Mitter, B. (2019). Microbiome applications from lab to field: facing complexity. Trends Plant Sci. 24, 194–198. doi: 10.1016/j.tplants.2018.12.004
Shamseldin, A., Abdelkhalek, A., and Sadowsky, M. J. (2017). Recent changes to the classification of symbiotic, nitrogen-fixing, legume-associating bacteria: a review. Symbiosis 71, 91–109. doi: 10.1007/s13199-016-0462-3
Sharma, A., Patni, B., Shankhdhar, D., and Shankhdhar, S. C. (2013). Zinc - An Indispensable Micronutrient. Physiol. Mol. Biol. Plants 19, 11–20. doi: 10.1007/s12298-012-0139-1
Sharma, A., Shankhdhar, D., and Shankhdhar, S. C. (2016). “Potassium-solubilizing microorganisms: mechanism and their tole in potassium solubilization and uptake,” in Potassium Solubilizing Microorganisms for Sustainable Agriculture, eds V. S. Meena, B. R. Maurya, J. P. Verma, and R. S. Meena (New Delhi: Springer), 1–331. doi: 10.1007/978-81-322-2776-2
Shirmohammadi, E., Alikhani, H. A., Pourbabaei, A. A., and Etesami, H. (2020). Improved phosphorus (P) uptake and yield of rainfed wheat fed with p fertilizer by drought-tolerant phosphate-solubilizing fluorescent pseudomonads strains: a field study in drylands. J. Soil Sci. Plant Nutr. 20, 2195–2211. doi: 10.1007/s42729-020-00287-x
Shukla, A. K., Behera, S. K., Pakhre, A., and Chaudhari, S. K. (2018). Micronutrients in soils, plants, animals and Humans. Indian J. Fertil. 14, 30–54.
Singh, M., Singh, D., Gupta, A., Pandey, K. D., Singh, P. K., and Kumar, A. (2019). “Plant growth promoting rhizobacteria,” in PGPR Amelioration in Sustainable Agriculture, eds A. K. Singh, A. Kumar, and P. K. Singh (Cambridge, MA: Elsevier), 41–66. doi: 10.1016/B978-0-12-815879-1.00003-3
Sohn, B. K., Kim, K. Y., Chung, S. J., Kim, W. S., Park, S. M., Kang, J. G., et al. (2003). Effect of the different timing of AMF inoculation on plant growth and flower quality of chrysanthemum. Sci. Hortic. 98, 173–183. doi: 10.1016/S0304-4238(02)00210-8
Somers, E., Vanderleyden, J., and Srinivasan, M. (2004). Rhizosphere bacterial signalling: a love parade beneath our feet. Crit. Rev. Microbiol. 30, 205–240. doi: 10.1080/10408410490468786
Soumare, A., Boubekri, K., Lyamlouli, K., Hafidi, M., Ouhdouch, Y., and Kouisni, L. (2020). From Isolation Of Phosphate Solubilizing Microbes To Their Formulation And Use As Biofertilizers: Status And Needs. Front. Bioeng. Biotechnol. 7:425. doi: 10.3389/fbioe.2019.00425
Spagnoletti, F. N., and Giacometti, R. (2020). “Dark septate endophytic fungi (DSE) response to global change and soil contamination,” in Plant Ecophysiology and Adaptation Under Climate Change: Mechanisms and Perspectives II, ed M. Hasanuzzaman (Singapore: Springer), 629–642. doi: 10.1007/978-981-15-2172-0_23
Spagnoletti, F. N., Tobar, N. E., Fernández Di Pardo, A., Chiocchio, V. M., and Lavado, R. S. (2017). Dark septate endophytes present different potential to solubilize calcium, iron and aluminum phosphates. Appl. Soil Ecol. 111, 25–32. doi: 10.1016/j.apsoil.2016.11.010
Srivastava, A. K., Shankar, A., Nalini Chandran, A. K., Sharma, M., Jung, K.-H., Suprasanna, P., et al. (2019). Emerging concepts of potassium homeostasis in plants. J. Exp. Bot. 71, 608–619. doi: 10.1093/jxb/erz458
Stella, M., Theeba, M., and Illani, Z. I. (2019). Organic fertilizer amended with immobilized bacterial cells for extended shelf-life. Biocatal. Agric. Biotechnol. 20:101248. doi: 10.1016/j.bcab.2019.101248
Stringlis, I. A., Yu, K., Feussner, K., de Jonge, R., Van Bentum, S., Van Verk, M. C., et al. (2018). MYB72-dependent coumarin exudation shapes root microbiome assembly to promote plant health. Proc. Natl. Acad. Sci. U.S.A. 115, E5213–E5222. doi: 10.1073/pnas.1722335115
Swarnalakshmi, K., Prasanna, R., Kumar, A., Pattnaik, S., Chakravarty, K., Shivay, Y. S., et al. (2013). Evaluating the influence of novel cyanobacterial biofilmed biofertilizers on soil fertility and plant nutrition in wheat. Eur. J. Soil Biol. 55, 107–116. doi: 10.1016/j.ejsobi.2012.12.008
Taktek, S., St-Arnaud, M., Piché, Y., Fortin, J. A., and Antoun, H. (2017). Igneous phosphate rock solubilization by biofilm-forming mycorrhizobacteria and hyphobacteria associated with Rhizoglomus irregulare DAOM 197198. Mycorrhiza 27, 13–22. doi: 10.1007/s00572-016-0726-z
Thilakarathna, M. S., and Raizada, M. N. (2017). A meta-analysis of the effectiveness of diverse rhizobia inoculants on soybean traits under field conditions. Soil Biol. Biochem. 105, 177–196. doi: 10.1016/j.soilbio.2016.11.022
Timmusk, S., Behers, L., Muthoni, J., Muraya, A., and Aronsson, A.-C. (2017). Perspectives and challenges of microbial application for crop improvement. Front. Plant Sci. 8:49. doi: 10.3389/fpls.2017.00049
Toju, H., Peay, K. G., Yamamichi, M., Narisawa, K., Hiruma, K., Naito, K., et al. (2018a). Core microbiomes for sustainable agroecosystems. Nat. Plants 4, 247–257. doi: 10.1038/s41477-018-0139-4
Toju, H., Tanabe, A. S., and Sato, H. (2018b). Network hubs in root-associated fungal metacommunities. Microbiome 6, 1–16. doi: 10.1186/s40168-018-0497-1
Tosi, M., Gaiero, J., Linton, N., Mafa-Attoye, T., CastiIlo, A., and Dunfield, K. (2020b). “Bacterial endophytes: diversity, functional importance, and potential for manipulation,” in Rhizosphere Biology: Interactions Between Microbes and Plants, eds V. V. S. R. Gupta and A. K. Sharma (Singapore), 1–49. doi: 10.1007/978-981-15-6125-2_1
Tosi, M., Mitter, E. K., Gaiero, J., and Dunfield, K. E. (2020a). It takes three to tango: the importance of microbes, host plant and soil management to elucidate manipulation strategies for the plant microbiome. Can. J. Microbiol. 66, 413–433. doi: 10.1139/cjm-2020-0085
Trabelsi, D., Mengoni, A., Ben Ammar, H., and Mhamdi, R. (2011). Effect of on-field inoculation of Phaseolusvulgaris with rhizobia on soil bacterial communities. FEMS Microbiol. Ecol. 77, 211–222. doi: 10.1111/j.1574-6941.2011.01102.x
Trabelsi, D., and Mhamdi, R. (2013). Microbial inoculants and their impact on soil microbial communities: a review. Biomed. Res. Int. 2013:863240. doi: 10.1155/2013/863240
Triveni, Y. N. P., and Jhansi, R. S. (2017). Biofilm formation of zinc solubilizing, potassium releasing bacteria on the surface of fungi. Int. J. Curr. Microbiol. Appl. Sci. 6, 2037–2047. doi: 10.20546/ijcmas.2017.604.241
Tsai, H. H., and Schmidt, W. (2017). Mobilization of iron by plant-borne coumarins. Trends Plant Sci. 22, 538–548. doi: 10.1016/j.tplants.2017.03.008
Tubaña, B. S., and Heckman, J. R. (2015). “Silicon in Soils and Plants,” in Silicon and Plant Diseases, eds F. A. Rodrigues and L. E. Datnoff (Cham: Springer International Publishing), 1–148. doi: 10.1007/978-3-319-22930-0
Ulzen, J., Abaidoo, R. C., Ewusi-Mensah, N., and Masso, C. (2019). Combined application of inoculant, phosphorus and organic manure improves grain yield of cowpea. Arch. Agron. Soil Sci. 00, 1–15. doi: 10.1080/03650340.2019.1669786
Ünal Turhan, E., Erginkaya, Z., Korukluoglu, M., and Konuray, G. (2019). “Beneficial biofilm applications in food and agricultural industry,” in Health and Safety Aspects of Food Processing Technologies, eds A. Malik, Z. Erginkaya and H. Erten (Cham: Springer International Publishing), 445–469. doi: 10.1007/978-3-030-24903-8_15
Vacheron, J., Desbrosses, G., Bouffaud, M.-L., Touraine, B., Moënne-Loccoz, Y., Muller, D., et al. (2013). Plant growth-promoting rhizobacteria and root system functioning. Front. Plant Sci. 4:356. doi: 10.3389/fpls.2013.00356
van Bruggen, A. H. C., Goss, E. M., Havelaar, A., van Diepeningen, A. D., Finckh, M. R., and Morris, J. G. (2019). One Health - Cycling of diverse microbial communities as a connecting force for soil, plant, animal, human and ecosystem health. Sci. Total Environ. 664, 927–937. doi: 10.1016/j.scitotenv.2019.02.091
Vargas, L., de Carvalho, T. L. G., Ferreira, P. C. G., Baldani, V. L. D., Baldani, J. I., and Hemerly, A. S. (2012). Early responses of rice (Oryza sativa L.) seedlings to inoculation with beneficial diazotrophic bacteria are dependent on plant and bacterial genotypes. Plant Soil 356, 127–137. doi: 10.1007/s11104-012-1274-8
Vargas, M. A. T., Mendes, I. C., and Hungria, M. (2000). Response of field-grown bean (Phaseolus vulgaris l.) to Rhizobium inoculation and nitrogen fertilization in two cerrados soils. Biol. Fertil. Soils 32, 228–233. doi: 10.1007/s003740000240
Vassilev, N., Vassileva, M., Martos, V., Garcia del Moral, L. F., Kowalska, J., Tylkowski, B., et al. (2020). Formulation of microbial inoculants by encapsulation in natural polysaccharides: focus on beneficial properties of carrier additives and derivatives. Front. Plant Sci. 11:270. doi: 10.3389/fpls.2020.00270
Velmourougane, K., Prasanna, R., and Saxena, A. K. (2017). Agriculturally important microbial biofilms: present status and future prospects. J. Basic Microbiol. 57, 548–573. doi: 10.1002/jobm.201700046
Veresoglou, S. D., Chen, B., and Rillig, M. C. (2012). Arbuscular mycorrhiza and soil nitrogen cycling. Soil Biol. Biochem. 46, 53–62. doi: 10.1016/j.soilbio.2011.11.018
Vergara, C., Araujo, K. E. C., Alves, L. S., Souza, S. R., de Santos, L. A., Santa-Catarina, C., et al. (2018). Contribution of dark septate fungi to the nutrient uptake and growth of rice plants. Braz. J. Microbiol. 49, 67–78. doi: 10.1016/j.bjm.2017.04.010
Vílchez, J. I., Navas, A., González-López, J., Arcos, S. C., and Manzanera, M. (2016). Biosafety test for plant growth-promoting bacteria: proposed Environmental and Human Safety Index (EHSI) Protocol. Front. Microbiol. 6:1514. doi: 10.3389/fmicb.2015.01514
Vlamakis, H., Chai, Y., Beauregard, P., Losick, R., and Kolter, R. (2013). Sticking together: building a biofilm the Bacillus subtilis way. Nat. Rev. Microbiol. 11, 157–168. doi: 10.1038/nrmicro2960
Voges, M. J. E. E. E., Bai, Y., Schulze-Lefert, P., and Sattely, E. S. (2019). Plant-derived coumarins shape the composition of an Arabidopsis synthetic root microbiome. Proc. Natl. Acad. Sci. U.S.A. 116, 12558–12565. doi: 10.1073/pnas.1820691116
Vorholt, J. A., Vogel, C., Carlström, C. I., and Müller, D. B. (2017). Establishing causality: opportunities of synthetic communities for plant microbiome research. Cell Host Microbe 22, 142–155. doi: 10.1016/j.chom.2017.07.004
Vujanovic, V., and Germida, J. (2017). Seed endosymbiosis: a vital relationship in providing prenatal care to plants. Can. J. Plant Sci. 97:CJPS-2016-0261. doi: 10.1139/CJPS-2016-0261
Wang, D., Xu, A., Elmerich, C., and Ma, L. Z. (2017). Biofilm formation enables free-living nitrogen-fixing rhizobacteria to fix nitrogen under aerobic conditions. ISME J. 11, 1602–1613. doi: 10.1038/ismej.2017.30
Wei, Y., Zhao, Y., Shi, M., Cao, Z., Lu, Q., Yang, T., et al. (2018). Effect of organic acids production and bacterial community on the possible mechanism of phosphorus solubilization during composting with enriched phosphate-solubilizing bacteria inoculation. Bioresour. Technol. 247, 190–199. doi: 10.1016/j.biortech.2017.09.092
Weihrauch, C., and Opp, C. (2018). Ecologically relevant phosphorus pools in soils and their dynamics: the story so far. Geoderma 325, 183–194. doi: 10.1016/j.geoderma.2018.02.047
Werner, D., and Newton, W. E. (2005). Nitrogen Fixation in Agriculture, Forestry, Ecology, and the Environment. (Dordrecht: Springer). doi: 10.1007/1-4020-3544-6
Xavier, L. J. C., and Germida, J. J. (2003). Selective interactions between arbuscular mycorrhizal fungi and Rhizobium leguminosarum bv. viceae enhance pea yield and nutrition. Biol. Fertil. Soils 37, 261–267. doi: 10.1007/s00374-003-0605-6
Yao, Q., Li, X., and Christie, P. (2001). Factors affecting arbuscular mycorrhizal dependency of wheat genotypes with different phosphorus efficiencies. J. Plant Nutr. 24, 1409–1419. doi: 10.1081/PLN-100106991
Yoon, V., Tian, G., Vessey, J. K., Macfie, S. M., Dangi, O. P., Kumer, A. K., et al. (2016). Colonization efficiency of different sorghum genotypes by Gluconacetobacter diazotrophicus. Plant Soil 398, 243–256. doi: 10.1007/s11104-015-2653-8
Youssef, H. H., Hamza, M. A., Fayez, M., Mourad, E. F., Saleh, M. Y., Sarhan, M. S., et al. (2016). Plant-based culture media: efficiently support culturing rhizobacteria and correctly mirror their in-situ diversity. J. Adv. Res. 7, 305–316. doi: 10.1016/j.jare.2015.07.005
Zeffa, D. M., Perini, L., Silva, M. B., de Sousa, N. V., Scapim, C. A., de Oliveira, A. L. M., et al. (2019). Azospirillum brasilense promotes increases in growth and nitrogen use efficiency of maize genotypes. PLoS ONE 14:e0215332. doi: 10.1371/journal.pone.0215332
Zhong, Y., Yang, Y., Liu, P., Xu, R., Rensing, C., Fu, X., et al. (2019). Genotype and rhizobium inoculation modulate the assembly of soybean rhizobacterial communities. Plant Cell Environ. 42, 2028–2044. doi: 10.1111/pce.13519
Keywords: plant growth promotion, microbiome, plant nutrition, bioprospecting, soil health, sustainable agriculture, inoculation, bioformulation
Citation: Mitter EK, Tosi M, Obregón D, Dunfield KE and Germida JJ (2021) Rethinking Crop Nutrition in Times of Modern Microbiology: Innovative Biofertilizer Technologies. Front. Sustain. Food Syst. 5:606815. doi: 10.3389/fsufs.2021.606815
Received: 15 September 2020; Accepted: 18 January 2021;
Published: 19 February 2021.
Edited by:
Maryke T. Labuschagne, University of the Free State, South AfricaReviewed by:
Tahira Fatima, Purdue University, United StatesSenthilkumar Murugaiyan, Tamil Nadu Agricultural University, India
Copyright © 2021 Mitter, Tosi, Obregón, Dunfield and Germida. This is an open-access article distributed under the terms of the Creative Commons Attribution License (CC BY). The use, distribution or reproduction in other forums is permitted, provided the original author(s) and the copyright owner(s) are credited and that the original publication in this journal is cited, in accordance with accepted academic practice. No use, distribution or reproduction is permitted which does not comply with these terms.
*Correspondence: Kari E. Dunfield, ZHVuZmllbGRAdW9ndWVscGguY2E=; James J. Germida, amltLmdlcm1pZGFAdXNhc2suY2E=