- 1Division of Microbiology, Indian Council of Agricultural Research-Indian Agricultural Research Institute, New Delhi, India
- 2Division of Plant Pathology, Indian Institute of Oilseed Research-Indian Council of Agricultural Research, Hyderabad, India
The above ground growth of the plant is highly dependent on the belowground root system. Rhizosphere is the zone of continuous interplay between plant roots and soil microbial communities. Plants, through root exudates, attract rhizosphere microorganisms to colonize the root surface and internal tissues. Many of these microorganisms known as plant growth promoting rhizobacteria (PGPR) improve plant growth through several direct and indirect mechanisms including biological nitrogen fixation, nutrient solubilization, and disease-control. Many PGPR, by producing phytohormones, volatile organic compounds, and secondary metabolites play important role in influencing the root architecture and growth, resulting in increased surface area for nutrient exchange and other rhizosphere effects. PGPR also improve resource use efficiency of the root system by improving the root system functioning at physiological levels. PGPR mediated root trait alterations can contribute to agroecosystem through improving crop stand, resource use efficiency, stress tolerance, soil structure etc. Thus, PGPR capable of modulating root traits can play important role in agricultural sustainability and root traits can be used as a primary criterion for the selection of potential PGPR strains. Available PGPR studies emphasize root morphological and physiological traits to assess the effect of PGPR. However, these traits can be influenced by various external factors and may give varying results. Therefore, it is important to understand the pathways and genes involved in plant root traits and the microbial signals/metabolites that can intercept and/or intersect these pathways for modulating root traits. The use of advanced tools and technologies can help to decipher the mechanisms involved in PGPR mediated determinants affecting the root traits. Further identification of PGPR based determinants/signaling molecules capable of regulating root trait genes and pathways can open up new avenues in PGPR research. The present review updates recent knowledge on the PGPR influence on root architecture and root functional traits and its benefits to the agro-ecosystem. Efforts have been made to understand the bacterial signals/determinants that can play regulatory role in the expression of root traits and their prospects in sustainable agriculture. The review will be helpful in providing future directions to the researchers working on PGPR and root system functioning.
Introduction
Rhizosphere is the ecological niche comprising the soil surrounding the plant roots which is under the influence of root exudates (Hiltner, 1904) and plays a major role in supporting the growth and activity of a large but variable community of microorganisms. Plant growth promoting rhizobacteria (PGPR) are a group of bacteria that colonize the rhizosphere and are capable of augmenting plant growth by a variety of direct and indirect mechanisms. The beneficial microorganisms colonizing the microhabitats, rhizoplane, and root endosphere (Hartman and Tringe, 2019) function as the root microbiome and exhibit plant growth promoting activities. In turn, the secretion of carbon compounds from plants into the surrounding soil results in greater microbial populations (100–1,000 times higher) in the rhizosphere relative to the bulk soil (Lynch, 1987; Bending, 2003; Goswami et al., 2016). Due to the influence of root exudates, the microbial populations present in the rhizosphere are relatively different from those present in the bulk soil (Burdman et al., 2000). The most important and well-documented group of plant beneficial microbes comprises bacteria that form endosymbiotic associations with leguminous plants by inhabiting the root nodules and benefit the host plant mainly by fixing atmospheric nitrogen. PGPR do not need to form endosymbiotic association and exert positive effect on plant through one or more direct and/or indirect mechanisms. A rhizobacteria qualifies as PGPR only if it is able to produce a positive effect on the host plant upon inoculation, hence demonstrating good competitive skills over the native rhizosphere communities. It has been suggested that ~2–5% of rhizosphere bacteria act as PGPR (Antoun and Prévost, 2005; Barriuso et al., 2008). PGPR may affect plant performance through direct and indirect mechanisms (Figure 1). Direct mechanisms operate through the production of plant growth promoting substances (i.e., phytohormones), and enhanced availability and uptake of nutrients in soil through biological nitrogen fixation, solubilization of fixed form of nutrients to plant available form (P, K, Zn), chelation of nutrients (Fe) through siderophore production etc. (Glick, 2014; Goswami et al., 2016). Indirect mechanisms include suppression of plant pathogens (Beneduzi et al., 2012; Ribeiro and Cardoso, 2012) and abiotic stress tolerance (Grover et al., 2011; van Oosten et al., 2017). Credible group of PGPR include bacteria belonging to genera Acinetobacter, Agrobacterium, Arthobacter, Azotobacter, Azospirillum, Burkholderia, Bradyrhizobium, Rhizobium, Frankia, Serratia, Thiobacillus, Pseudomonads, and Bacillus (Vessey, 2003; Goswami et al., 2016).
Positive effect of PGPR inoculation has been reported on aboveground as well as belowground plant part, although, more attention has been given to the above ground part due to economic importance of the aerial parts as food and fodder and, ease of sampling and recording observations. However, accumulating evidences on the role of root traits in ecosystem functioning have attracted the attention of the researchers to understand and explore the root trait based interventions in driving ecosystem processes (Bardgett et al., 2014). The primary function of the roots is to provide anchorage as well as support to the aboveground biomass and uptake water and nutrients (macro and micro) from the soil for plant growth. In addition, roots also play important role in nutrient cycling by providing organic matter and by influencing the activity of soil microbial communities, in soil structure formation through soil aggregation and in carbon sequestration through recalcitrant components etc. Root traits are highly plastic in response to nutrient and water gradient, environmental gradients and to biotic interactions with other species. Hence, the root traits show high variation both between and within the species (Bardgett et al., 2014). Thus, any of these external stimuli may influence the roots traits. In this respect, PGPRs have been reported for altering the root architecture of plants (Mantelin et al., 2006). Similarly, root acquisition of water and nutrients is essential for plant growth and crop productivity. As a primary target, root is the organ that shows the first stimulatory effects of bacterial interactions (Nosheen et al., 2011). This is particularly remarkable in plants inoculated with PGPR belonging to genus Azospirillum (Okon, 1985). Inoculation with Azospirillum has been found to cause remarkable positive alterations in the growth and morphology of the roots accompanied by better water and mineral uptake and marked increase in plant yield (Creus et al., 2005). One of the most striking effects of PGPR inoculation on roots is stimulation of lateral root development (Mantelin et al., 2006). This increase in development of lateral roots causes an increase in root surface which improves plant nutrient uptake and therefore, can be considered as an important factor for promotion of plant growth by PGPR.
An improved understanding of root system development and functioning, to identify root traits contributing to crop yields in various scenarios, as well as mechanisms by which they are enhanced is a research frontier that might enable a second Green Revolution needed to sustain world food security (Lynch, 2007). PGPR mediated modulations of root traits can be advantageously explored for improving the efficiency of agroecosystems. PGPR able to induce desired root traits for harnessing the soil resources can be a way toward sustainable agricultural production. The role of PGPR in improving plant productivity has been reviewed by many workers (Beneduzi et al., 2012; Backer et al., 2018). However, there is limited knowledge on how PGPR modify the root traits. The present review focuses on the PGPR mediated changes in root traits and key determinants responsible for root-microbe cross talk. Contributions of PGPR mediated root traits to ecosystem service and crop productivity are also discussed.
Importance of Root Traits
Root act as interface between plant and soil besides providing anchorage and support to the growing plant. Also, roots act as storage organ in many plants (Smith and De Smet, 2012). Majority of the terrestrial plants have well-developed system of roots for exploration of the soil and for nutrient acquisition to support the growth and development of the growing plant. Root is a complex organ that comprises different regions including root tip, meristem, differentiation and elongation zones, and emerging lateral roots (Scheres et al., 2002). All these regions have discrete roles. For example, the terminal portion of a root or root branch usually including the root cap and the meristematic region behind it is often the region of differentiation, elongation, and root hair formation. The purpose of the root cap is to enable downward growth of the root tip while, root hairs are the differentiated epidermal cells vital for uptake of minerals (Ahn et al., 2004). Lateral roots help in branching of root systems and play a significant role in the uptake of water, ion, and nutrients and also facilitate the diffusion of oxygen from the base to the apex thus contributing significantly in plant development (Colmer, 2003; Kotula et al., 2009a,b). The functional specificity of roots is also known by the extent of interactions between plant and the microbes. For example, in Fabaceae, root tip is the main region where the process of rhizobial colonization is initiated leading to root nodule formation (Desbrosses and Stougaard, 2011). While, PGPR preferentially colonize root hairs and lateral roots, where they might exhibit beneficial properties for the plant (Pothier et al., 2007; Combes-Meynet et al., 2011).
According to Bardgett et al. (2014), kinds of root traits which can potentially affect the rhizosphere functioning, include: (1) architectural root traits, that decide the topology and spatial configuration of the entire root system of a plant, like root number, depth of root, and root length density; (2) morphological root traits that refer to features of individual roots, such as diameter of the roots, specific length of roots, density of root tissues, and dry matter content of the roots; (3) physiological root traits like nutrient uptake kinetics, release of root exudates, and root respiration; and (4) biotic traits which include direct interactions between roots and soil microbiota that affect nutrient capture, such as associations with mycorrhizal fungi and rhizobia (in legumes). However, the biotic interaction of roots with rhizospheric microorganisms also influence the architectural, morphological, and physiological traits of roots thus, influencing the root system functioning as indicated by the recently accumulating evidences (Nosheen et al., 2011; Drogue et al., 2013; Vacheron et al., 2013; Mesa-Marín et al., 2018), although majority of earlier studies on PGPR effect on root traits have reported only effects in terms of root length, biomass and volume.
Root architecture refers to the spatial configuration of the root system that includes topology and distribution of an entire root system or a large subset of the root system of an individual plant. The spatial deployment of the root system helps the plant to explore the unevenly distributed or spatially localized resources in the soil and thus play important role in plant productivity (Lynch, 1995). The architecture of a root system also responds dynamically to the localized availability of soil resources through meristematic activity and transport functions. Indeed, root architecture has been linked with plant acquisition of water and nutrients that move with water, as well as immobile nutrients such as P (Lynch, 1995). The root architecture also determines the role of roots in providing mechanical support to the aboveground parts of the plant (Ennos and Fitter, 1992). The morphological and physiological traits of roots play essential roles in ecological processes (Dong et al., 2016). Morphological changes in roots are often caused due to internal physiological changes, induced in response to external stimuli (Makita et al., 2011; Gong and Zhao, 2019).
PGPR and Root Traits
Understanding and quantifying the impact of PGPR on roots and the whole plant remain challenging (Vacheron et al., 2013). Several in vitro studies conducted to study the effect of PGPR inoculation on plant growth and root traits, reveal that many PGPR reduce the growth of main root, increase the number, and/or length of lateral roots and stimulate root hair elongation thus enhancing the uptake of water and nutrients and resulting in increased plant growth and development (Figure 2; Vacheron et al., 2013; Cassán et al., 2020). Positive effects of PGPR inoculation on root and shoot biomass have also been reported from soil based pot and field studies (El-Zemrany et al., 2006; Minorsky, 2008; Veresoglou and Menexes, 2010; Walker et al., 2011). Table 1 summarizes the reports on PGPR mediated effects on root traits and other plant growth parameters. As evident from the Table 1, majority of the studies have reported the effect in terms of root length and biomass. Members of Azospirillum and Rhizobium are most studied PGPR for their effect on root traits. Azospirillum-root association has been extensively studied all over the world in different crops including cereals, forage, vegetables, and the stimulatory effects of inoculation on root elongation, root biomass, development of lateral and adventitious roots, root hairs and branching of root hairs have been well-documented (Hadas and Okon, 1987; Bashan and de-Bashan, 2010). These cumulative effects on root traits results in significant improvement in the root system functioning. However, these effects have been found to vary with inoculum concentration and environmental conditions (Okon and Kapulnik, 1986).
Among the initial studies on Azospirillum-root interaction, Tien et al. (1979) observed that the morphology of pearl millet (Pennisetum glaucum L.) roots with respect to increased number of lateral roots and increased density of root hair when inoculated with A. brasilense. Umali-Garcia et al. (1980) described the adsorption and colonization of A. brasilense Sp7 on the root surfaces of pearl millet and guinea grass (Megathyrsus maximus) under controlled conditions. Inoculated roots of both the plants produced more mucilaginous sheath, root hairs, and lateral roots as compared to the uninoculated controls. The presence of bacteria was observed within the mucilaginous sheath accumulated on the root cap and along the root axes. Pectolytic activities detected in pure cultures of A. brasilense provided a possible explanation for colonization of intercellular spaces of the outer root cortex. It was also observed that inoculated bacteria showed firm attachment to root hairs when no nitrogen source was added to the culture medium, whereas, no firm attachment of the inoculated bacteria was observed in the culture medium supplemented with potassium nitrate. Okon and Kapulnik (1986) summarized many such studies that documented the presence of Azospirillum in the cortical tissues, in the regions of lateral root emergence, along the inner cortex, inside the xylem vessels and between the pith cells. Inoculation of various crops including wheat (Triticum aestivum L.), corn (Zea mays L.), sorghum (Sorghum bicolor L.), and setaria (Setaria viridis L.) with different strains of Azospirillum resulted in root morphological changes that started immediately after germination. The effect on root length and surface area varied with age and cell level of inoculated bacteria. Inoculation increased the number and branching of root hairs (Jain and Patriquin, 1985) and number of lateral roots during the initial 3 weeks post-germination, but root biomass increased at later stages (Okon and Kapulnik, 1986). Inoculation also induced irregularity in the arrangement of cortical cells. Morphological changes also influenced the physiological traits of inoculated roots as evident from the reduced activities of oxidative enzymes, and low lipid and suberin content. These observations suggested presence of larger proportion of younger roots in inoculated roots than in uninoculated roots. The inoculated seedlings also exhibited higher rate of , K+, and H2 uptake in inoculated seedlings. Field studies revealed faster rates of dry matter, N, P, and K accumulation with high water content in Azospirillum inoculated plants (Okon and Kapulnik, 1986). Inoculation with wild strains of A. brasilense Sp245 and Sp7 in wheat caused significant reduction in root length, but increased the number of root hairs (Dobbelaere et al., 1999). Inoculation of tomato (Lycopersicum esculentum L.) seedlings with A. brasilense Sp245 could promote both lateral and adventitious roots formation. Application of cell free culture supernatants of A. brasilense ATCC 29710 to rice (Oryza sativa L.) roots increased root elongation, root surface area, root dry matter, and development of lateral roots and root hairs (El-Khawas and Adachi, 1999). In a similar study, Molla et al. (2001) reported increased number of roots and root length in soybean (Glycine max L.) plants treated with cell-free supernatant of A. brasilense Cd., indicating the release of root growth promoting substances by the cells into the medium. El-Zemrany et al. (2007) assessed the result of inoculation of maize (Zea mays) seeds grown in a luvisol soil with Azospirillum lipoferum CRT1. The observations recorded after 35 days of plant growth revealed significant increase in the number of root tips and total root length in the inoculated plants. The authors reported that due to changes in root traits, the inoculated roots had a larger surface area to interact with soil particles, soil water and microbes than the uninoculated plants. Further, the mechanical and biochemical (lignin content) properties of the root system of inoculated plants showed less mature characteristics as compared to uninoculated control plants. This observation indicated that the growth of new root tips in the early stages of inoculation resulted in the dominance of primary root tissue. Moreover, roots of inoculated plants showed more stiffness that may contribute to anchorage thus increasing the resistance of the plants to lodging. However, such types of studies need to be conducted at regular intervals during the crop growth period, to evaluate if the differences between inoculated and uninoculated plants endure or vanish with time until the harvest.
Increased formation of lateral roots in barrel medic (Medicago truncatula) by the Nod factor of Sinorhizobium meliloti was observed by Oláh et al. (2005). Inoculation with Bacillus megaterium UMCV1 in Arabidopsis thaliana resulted in inhibition of primary-root growth (due to decreased cell elongation and proliferation in the root meristem,) and an increase in number and length of lateral roots and root-hair (López-Bucio et al., 2007). Similar results were reported by Zhang et al. (2007) and Gutiérrez-Luna et al. (2010) with volatile organic compounds (VOCs) producing Bacillus strains. Co-culturing of Serratia marscescens with Arabidopsis resulted in inhibition of elongation of primary roots, while inducing lateral roots (Shi et al., 2010). On contrary, inoculation with Phyllobacterium brassicacearum STM196 in Arabidopsis exerted a positive effect on the total length of lateral roots but no effect on the primary root length or lateral root density (Mantelin et al., 2006; Contesto et al., 2010). An increased root branching was seen in alder (Alnus glutinosa L.) due to root colonization by Frankia UGL010708 (Orfanoudakis et al., 2010). The root architecture in terms of increased primary length of roots, number of lateral roots and root fresh weight in A. thaliana was modified by the rhizobacterium Pseudomonas aeruginosa PAO1 (Ortiz-Castro et al., 2011). Interestingly, Nosheen et al. (2011) observed inoculation effects of Azotobacter vinelandii Khsr1, Azospirillum brasilense and Pseudomonas stutzeri Khsr3 on physiological root traits in safflower (Carthamus tinctorius L.). The authors observed that the inoculation caused significant increase in root diameter (upto 76% increase), resulting in greater biomass. Ferreira Rêgo et al. (2014) observed significant changes in architectural, morphological and physiological traits in the roots of rice plant, treated individually or as combination with rhizobaterial strains Burkholderia pyrrocinia R-46 and Pseudomonas fluorescens R-55 as individual or combined treatments. Rhizobacterial treatment caused increase in length and diameter of roots along with expansion of cortex and aerenchyma space as compared to uninoculated control treatment. Rhizobacterial inoculation also caused increase in number of protoxylem poles and metaxylem vessel elements and also improved total phenol content and flavonoid content in the roots. Also, treatment with Pseudomonas fluorescens R-55 improved lignin content. The modification caused by rhizobacterial treatment resulted in root plasticity in rice plants. Interestingly, a thicker pericycle was presented in plants inoculated with B. pyrrocinia, which is linked to the development of lateral roots. The process by which lateral roots are formed includes two major stages: pericycle cell cycle reactivation and establishing a new meristem. The PGPR mediated stimulation of pericycle and protoxylem may result in increased lateral root formation and consequently more vigorous establishment of rice plants (Ferreira Rêgo et al., 2014). Co-inoculation of common bean (Phaseolus vulgaris L.) with two rhizobial strains, i.e., IITA-PAU 983 and IITA-PAU 987 significantly increased root dry weights and nodule weight of plants when compared to uninoculated control (Korir et al., 2017). Several PGPR, not classified as mutualisti partners, have also been shown to affect the architecture of plant roots.
Microbial Metabolites/Molecules That Influence Root Trait Pathways in Plants
Phytohormones and Root Traits
Root traits components like branch number, branching pattern, length, orientation, angle, and diameter are controlled by intricate genetic pathways that are also involved in plant's response to environmental stimuli (Jung and McCouch, 2013). These pathways belong to either “intrinsic pathways” or “extrinsic pathways” (Malamy and Ryan, 2001). Intrinsic pathways involve hormones, their receptors, signaling components, and transcription factors (TFs) whereas extrinsic pathways involve receptors for environmental stimuli, their downstream transduction and TFs. Many components of extrinsic pathways are shared with or interregulated by intrinsic pathway and are mediated by hormonal regulation (Jung and McCouch, 2013).
Phytohormones coordinate metabolic activities associated with growth of microorganisms in different plants tissues. Modifications at organ, cellular and molecular level of root systems and plant tissues get triggered by beneficial and detrimental rhizosphere microorganisms, through modifications of phytohormonal balances (Boivin et al., 2016). Many of the PGPR present in rhizosphere are well-known to secrete hormones for uptake by the plant roots or for manipulation of plant hormonal balance, which finally regulate root/shoot growth as well as stress response of the plant (Tien et al., 1979; Gupta et al., 2015). In this respect, the hormones involved majorly include auxins, cytokinins, ethylene, and to a lesser extent gibberellins and abscisic acid (ABA) has also been reported (Vacheron et al., 2013). The auxin-cytokinin balance is definitely the main regulating mechanism for organogenesis in plants that defines the architecture of roots (Aloni et al., 2006). In this respect, particular changes that occur in the structure of roots are: total root growth, length of primary and lateral roots, number of lateral roots, and the manner in which lateral roots are positioned (Contesto et al., 2010).
Role of Microbial Auxins on Root Traits of Plants
Auxins are responsible for division, extension, and differentiation of plant cells and tissues and are known to increase the rate of xylem and root formation (Bashan and de-Bashan, 2010). A brief and simple explanation of the function of auxin in development of roots is the promotion of lateral root development, while inhibiting elongation of roots. The development of lateral roots involves a cycle of cell divisions in the activated endodermal/pericycle cells which form the root primordia that eventually differentiate and emerge as the new lateral roots (Péret et al., 2012). All these processes i.e., initiation, development, emergence, and elongation of lateral roots are regulated by the biosynthesis, transport, and signaling of auxins (Péret et al., 2009; De Smet, 2012). Increase in the amount of auxins can cause increased formation of roots in an amount dependent manner, as evidenced by genetic engineering studies which augment levels of auxin (Malamy, 2005).
PGPR stimulate the root growth mainly due to their potential to secrete indole acetic acid (IAA). Many PGPR are known to synthesize auxins that impart a considerable effect on root growth and architecture (Vacheron et al., 2013). For example, auxin producing PGPRs Pseudomonas extremaustralis IB-κ13-1A and Paenibacillus illinoisensis IB 1087, when inoculated in wheat plants, resulted in increased root biomass and root auxin levels (Kudoyarova et al., 2017). Seed inoculation of wheat plant with P. extremaustralis IB-κ13-1A increased the crop yield under field conditions (Arkhipova et al., 2019). In several studies (as reviewed by Bashan and de-Bashan, 2010; Vacheron et al., 2013), morphological changes induced by Azospirillum in roots were mimicked by applying a combination of plant growth substances, indicating the involvement of bacterial auxin in root proliferation and consequent plant growth promotion. Jain and Patriquin (1984, 1985) identified IAA as the “branching substance” in the culture filtrate of A. brasilense Sp245. Fallik et al. (1989) reported that the levels of IAA and IBA (indole-3-butyric acid) were increased in maize plants inoculated with Azospirillum, thereby justifying the role of auxins in promotion of plant growth. Dobbelaere et al. (1999) reported that wheat plants when inoculated with the wild strains of A. brasilense Sp245 and Sp7 caused a significant reduction of root length and an increase in the formation of root hairs. Addition of tryptophan further enhanced the effect of inoculation on the root morphology. Also, the replacement of Azospirillum cells with exogenous IAA resulted in a similar response in the wheat roots (Dobbelaere et al., 1999). On these lines, when IAA was applied exogeneously to the roots of bean plants, the results were similar to that obtained with A. brasilense Sp245 inoculation (Remans et al., 2008).
Use of IAA-attenuated mutants could provide strong evidence for the role of IAA in PGPR-induced root growth promotion (de-Bashan et al., 2008). Inoculation of canola (Brassica napus L.) plants with auxin deficient Pseudomonas putida GR12-2 strain resulted in reduced root growth (Patten and Glick, 2002a). Likewise, inoculation with IAA-deficient mutants of Pseudomonas moraviensis in wheat caused reduction in root surface area by 13–38% as compared to that with the control strain (Hassan and Bano, 2019). Barbieri and Galli (1993) inoculated wheat seedlings with wild-type strain Azospirillum brasilense Sp6 and a very Iow-lAA producing mutant Azospirillum brasilense SPM7918 and observed reduced ability of the mutant strain to stimulate root system in terms of number and length of lateral roots and the distribution of root hairs. They also observed that by increasing the inoculum concentration of SpM7918, no increase in the number and length of lateral roots or in the density of root hair was observed, thus, indicating the involvement of additional factors in root growth. In another study, Kundu et al. (1997) observed that inoculating pearl millet seedlings with A. brasilense mutant strain with high N2-fixation activity but low phytohormone production ability could not cause any increase in root growth, whereas, the mutant strain able to produce higher levels of phytohormones had a considerable positive effect on the morphology of roots when compared with the uninoculated control plants (Kundu et al., 1997). The wheat seedlings inoculated with A. brasilense Sp245 strain with a mutated IAA synthesis gene, could not cause reduced root length or enhance the formation of root hairs when compared with the control (Dobbelaere et al., 1999). However, the insertion of the heterologous genes for indole acetamide pathway (iaaM and iaaH) into the strain A. brasilense SM significantly enhanced the levels of IAA and inoculation of sorghum with engineered strain resulted in marked increase on the lateral branching of sorghum roots and dry weight of roots as compared to inoculation with wild-type strain (Malhotra and Srivastava, 2006). Hence, it can be inferred that the analysis of mutations for studying the role of auxins in various plant-microbe interactions provide constant results in a widespread bacterial genera and plant species. Sukumar et al. (2013) reviewed the role of auxin pathways in regulating architecture of roots during plant- microbe interactions. Inculation with auxin producing PGPR strain induced transcriptional changesin hormone- and defense-related genes, as well as in plant cell wall-related genes in the host plant (Spaepen et al., 2014), resulting in induction of long roots (Hong et al., 1991), increased root biomass and decreased size and density of stomata (Llorente et al., 2016) as well as induction of auxin responsive genes that stimulate plant growth (Ruzzi and Aroca, 2015).
Auxins are implicated in the process of formation of nodules by rhizobia in leguminous plants, like founder cell specification (auxin transport inhibition mainly by flavonoids), initiation and differentiation of nodules (auxin accumulation), formation of vascular bundles, and the number of nodules (long distance auxin transport). Auxin produced by bacteria may change the balance of auxins in the plant and hence, indirectly affect the nodulation in legumes (Mathesius, 2008). Generally, rhizobia can produce auxins to enhance cell division, differentiation, root growth and increase nodule formation. IAA is most widely produced and studied among different rhizobial species including R. japonicum, R. lupine, R leguminosarum, R. phaseoli, R. meliloti, R. trifolii, Bradyrhizobium japonicum, B. elkanii, and Sinorhizobium sp. (Gopalakrishnan et al., 2015). The co-inoculation of IAA producing strains B. japonicum SB-1 and Bacillus thuringinesis KR1 resulted in enhanced plant growth and nodulation in pea (Pisum sativum L.) (Mishra et al., 2010). Inoculation with IAA overproducing R leguminosarum bv. viciae strain LPR1105 caused 60-fold increase in IAA content in the root nodules of the vetch plant (Camerini et al., 2008). The co-inoculation of Azospirillum and Rhizobium resulted in early and faster nodulation with higher crop yields. Additionally, the number of nodules and biological nitrogen fixation also increased. While, when ipdC mutants of Azospirillum were used for inoculating the plants, they synthesized only 10% of the IAA as compared to wild type strain and exhibited reduced ability to promote root development, without any increase in nodulation as well as nitrogen fixation, thus representing that the IAA biosynthesis by bacteria play significant role in plant-microbe symbiosis (Barbieri and Galli, 1993; Dobbelaere et al., 1999; Remans et al., 2008).
Azospirillum spp. are well-known for their potential to synthesize plant hormones and among them, indoles, specifically IAA and gibberellins (GAs) may play a larger role (Spaepen et al., 2007; Bashan and de-Bashan, 2010). Although auxins are known to cause stimulatory effect, their inhibitory effects on the elongation of roots has also been reported. The inhibitory effects could be dose-dependent (increases with auxin concentration) (Arteca and Arteca, 2008) as the increasing concentration of auxins may stimulate production of ethylene (Glick, 2014). Ethylene is a major phytohormone that causes inhibition of root elongation and transport of auxins, while promoting senescence and organ abscission, leading to ripening of fruits (Glick et al., 2007; Splivallo et al., 2009). Since, the direct precursor of ethylene is 1-aminocyclopropane-1-carboxylate (ACC) and hence bacterial ACC deaminase activity can control excessive ethylene production. The ACC deaminase can alleviate the repressing effect of ethylene, thus enhancing elongation of roots, in spite of high concentrations of potentially inhibitory auxin (Kudoyarova et al., 2019). Enterobacter cloacae UW4 that exhibited IAA and ACC deaminase activity promoted root elongation in canola (Brassica napus L.) plants, whereas its mutant lacking ACC deaminase activity had no effect on root growth and thus, highlighting the significance of ACC deaminase enzyme in elongation of roots in the presence of high auxins (Li et al., 2000). Thus, both IAA and ACC deaminase act together for stimulating elongation of roots (Patten and Glick, 2002b). Over expression of ACC deaminase in rhizobia enhanced the competiveness of the bacterium and increase nodule number in legumes such as pea, Lotus sp. (Lotus japonicus and L. tenuis) (Glick et al., 1998; Conforte et al., 2010). According to Glick (2014), the high level of ACC was observed in IAA producing rhizobia, which promote root and shoot elongation, enhanced mineral uptake, and nodulation. It was also observed that ACC deaminase enzyme producer rhizobia are potent nitrogen fixers. In Mesorhizobium sp., the regulation of ACC deaminase gene (acds) is under the control of nif promoter which also regulates nif gene expression for nitrogen fixation (Nascimento et al., 2012). Even though, auxins produced by microorganisms enhance branching in roots, which in turn stimulate uptake of water and nutrients, their higher concentrations can also be inhibitory for root elongation. Its implication can be harmful during conditions of drought, when longer roots are required for extracting water from deeper layers of soil. However, this can be prevented by PGPR that also have ACC deaminase activity along with high auxin producing capability.
On the other hand, in some studies, application of synthetic IAA could not stimulate the effect on root growth as induced by bacterial inoculation, thus showing that no correlation exists between IAA synthesis and the root growth stimulation (Kapulnik et al., 1985; Harari et al., 1988; Bothe et al., 1992). A. thaliana mutants (aux1-7, axr4-1, eir1, etr1, ein2, and rhd6), defective in either auxin or ethylene signaling, were found to increase the number of lateral roots and to develop long root hairs when inoculated with B. megaterium UMCV1, indicating that plant-growth promotion and root-architectural alterations by B. megaterium may involve auxin- and-ethylene independent mechanisms (López-Bucio et al., 2007). In addition, many studies indicated that the total positive effect on the organogenesis of roots and plant growth cannot be governed by IAA biosynthesis alone (Spaepen et al., 2007). According to Cassán et al. (2001), bacterial phytostimulation is critical in the stages of early development (germination and early seedling growth) and leads to other processes that occur later on during interaction between Azospirillum and plants. Although, IAA production from Azospirillum and other PGPR is well-documented, it is not the only mechanism in bacteria that regulates plant growth.
Microbial Cytokinins and Root Traits of Plants/Nodulation in Legumes
Cytokinins through regulating cell division and differentiation play important role in development of plant and regularly act in conjunction with other phytohormone. Mostly, the equilibrium between the levels of auxin and cytokinins is considered a major regulator of plant organogenesis and root architecture (Vacheron et al., 2013; Liu et al., 2017; Kudoyarova et al., 2019). In the shoot, cytokinins stimulate proliferation of cells, including the apical and axillary meristem activities. Increased levels of cytokinins in shoots have been linked to yield gain (Kieber and Schaller, 2018). In contrast to their stimulatory effect on shoot, cytokinins have inhibitory effect on root growth, which is due to promotion of cell differentiation in root apical meristem and regulation of root branching. By inhibiting initiation of lateral roots and elongation of primary roots, cytokinins control root architecture, and can also regulate functions of roots wherein, they regulate transport of nutrients and uptake of proteins (Argueso et al., 2009; Werner et al., 2010). Further, in concert with auxins, cytokinins are also responsible for the regulation of vascular development, as cytokinins promote phloem and auxins develop xylem through couple of mutually inhibitory interactions. Cytokinin production (particularly zeatin) has been reported in many PGPR like Arthrobacter, Azospirillum, Bradyrhizobium, Bacillus, Pseudomonas, and Paenibacillus (Cacciari et al., 1989; Timmusk et al., 1999; de-García Salamone et al., 2001; Perrig et al., 2007; Cassán et al., 2009; Hussain and Hasnain, 2009; Vacheron et al., 2013). Plants that are inoculated with cytokinin producing bacteria show enhanced growth of shoot, with reduced root to shoot ratio (Arkhipova et al., 2007). Additionally, various PGPRs synthesize cytokinins which cause increased production of root exudates by the plant (Ruzzi and Aroca, 2015) and thus in turn increase the PGPR interactions with the plant. The role of auxin and cytokinin in the regulation of Rhizobium nitrogen-fixing symbiotic interaction has been documented. In this regard, Gonzalez-Rizzo et al. (2006) used RNA interference approach for specifically targeting different putative cytokinin receptors that affect lateral root development in barrel medic roots and also its symbiotic interaction with Sinorhizobium meliloti. The authors observed that RNAi of the cytokinin receptor homolog Cytokinin Response1 (Mt CRE1) led to cytokinin-insensitive roots, which showed an increased number of lateral roots and a strong reduction in nodulation. Further, the development of S. meliloti infection and the formation of nodule primordium were also affected. The authors also identified two cytokinin signaling response regulator genes, Mt RR1 and Mt RR4, which were induced in the beginning of the symbiotic interaction. However, in the mutants that were affected in the NoD factor signaling pathway, the induction of these genes was altered by S. meliloti infection. This indicated that Mt CRE1 is responsible for cytokinin regulation of the early nodulin gene, Nodule Inception1 (Mt NIN). The authors thus concluded that Mt NIN, Mt RR1, and Mt RR4 are activated at the beginning of S. meliloti interaction, and thus permitting interaction between cytokinins of the plant and Nod factor signals of bacteria. Similarly, highly reduced nodulation capacity was observed in the lhk1/hit1 (lotus histidine kinase 1/hyperinfected 1) mutant of lotus (L. japonicas), which affected the closest homolog of cytokine receptor MtCRE1 (Murray et al., 2007). During nodule formation in legumes, the cell division is initiated by cytokinin responsible gene in the cortical cells, which leads to nodule development (Frugier et al., 2008; Plet et al., 2011; Held et al., 2014). Cooper and Long (1994) reported that rhizobia was capable to produce cytokinin in sufficient amount to induce cell division in cortical cells of alfalfa (Medicago sativa L.). Similarly, rhizobia enhanced the synthesis of cytokinin in legumes by expression of Nod factor cascade, which is helpful in understanding the coordination among cortical and epidermal cells during nodulation (Oldroyd, 2007). Interaction of Bradyrhizobium sp. strain ORS285 with legume plants, Aeschynomene afraspera and Indian joint vetch (A. indica L.) and displayed nodule formation by production of cytokinin through organism and also change size and numbers of nodules but initiation of nodule formation was also observed in cytokinin deficient mutants (Podlesakova et al., 2013).
Cytokinins regulate differentiation of root meristem, cause proliferation of root hairs, but have inhibitory effect on the formation of lateral roots (Silverman et al., 1998) and elongation of primary roots (Riefler et al., 2006). Primary root growth in A. thaliana was inhibited by Bacillus amyloliquefaciens UCMB5113, which can be attributed to the production of cytokinin (Asari et al., 2016). However, inoculation of wheat rhizospheres with zeatin producing B. subtilis IB 22 could not cause any reduction in the root biomass, but increased rhizodeposition (Kudoyarova et al., 2014). Arkhipova et al. (2005) observed that after inoculation with rhizobacteria B. subtilis IB22, zeatin riboside were detected in roots in the beginning followed by accumulation in shoots and corresponding decline in their concentration in roots. As the cytokinins produced by the bacterium are ribosylated, they get transported out of the roots. Hence, their accumulation was not observed in the roots and the growth of the roots was normal. It can thus be inferred that introduction of microbes that produce cytokinins into the rhizosphere may not essentially cause inhibition of root growth, provided they are migrated to the shoots.
Role of Microbial ABA and Gibberellins on Root Traits of Plants
Various studies have reported the ability of PGPR to synthesize ABA or gibberellic acid, or to regulate the level of these hormones in plants (Richardson et al., 2009; Dodd et al., 2010). ABA is a well-known phytohormone for its role during conditions of drought stress. The increased ABA levels during water limiting conditions cause closure of stomata and thus help in reducing loss of water (Bauer et al., 2013). Nevertheless, the hormone is also known to act differently during development of lateral roots (De Smet et al., 2006; Dodd et al., 2010). An increase in ABA levels in A. thaliana was observed when inoculated with Azospirillum brasilense Sp245, particularly under osmotic stress conditions (Cohen et al., 2008).
Gibberellins act in combination with other phytohormones and additional regulatory factors, and stimulate primary root elongation along with lateral root extension (Yaxley et al., 2001). Many PGPR including, Achromobacter xylosoxidans, Acinetobacter calcoaceticus, Azospirillum spp., Azotobacter spp., Bacillus spp., Herbaspirillum seropedicae, Gluconobacter diazotrophicus, and rhizobia are known to produce gibberlins (Gutiérrez-Mañero et al., 2001; Bottini et al., 2004; Dodd et al., 2010). In a study, when gibberellic acid at similar levels as those produced by Azospirillum was applied on maize was found to promote root growth. Further, the amount of gibberellins also increased in maize roots inoculated with Azospirillum strains (Fulchieri et al., 1993). Yanni et al. (2001) noted that indigenous Rhizobium leguminosarum bv. trifoli C6 could colonize rice roots in the Egyptian Nile delta where Trifolium alexandrinum L. is grown in rotation with rice. These IAA and gibberellin producing Rhizobium strains promoted root and shoot growth in rice, thereby improving seedling vigor hence resulting in higher grain yield.
The production of phytohormones by PGPR has been well-documented. Inoculation with phytohormone producing PGPR may influence the phytohormone levels in plants and thus resulting in altered growth. However, the role of individual hormone in influencing plant growth is difficult to measure as different phytohormones are co-produced by bacterial strains and they work in combination with other hormones and regulatory factors to produce an effect on plant system. Development of bacterial mutants lacking the ability to produce individual phytohormone from a strain producing multiple phytohormones can help in deducing the role of each phytohormone. However, the effect may vary with the dose and host plant. In future, transcriptomics can help in deciphering pathways up-regulated or down-regulated due to PGPR inoculation.
Role of Microbial VOCs on Root Traits of Plants
A number of VOCs and secondary metabolites produced by PGPRs helps in improving tolerance of plants to stress conditions and also aid in stimulation of plant growth by specifically altering root traits. VOCs are thus considered to be effective mediators of chemical crosstalk be it, attracting, repelling or warning signals. Microbes secrete VOCs for a variety of reasons like, crosstalk and protection (Kai and Piechulla, 2009). Plant root system can quickly and efficiently sense the volatiles released by its allied microbes. Ortíz-Castro et al. (2008) demonstrated that plant roots can perceive quorum sensing signals to modulate the root system architecture. Gutiérrez-Luna et al. (2010) using a divided Petri plate assay, observed the positive effect of certain rhizobacteria isolated from lemon (Citrus limon L.) on root morphogenesis and biomass production in A. thaliana seedlings, indicating the role of VOCs in plant growth modulation. The response of the plant to VOCs via alteration of root architecture may be of ecological significance for increasing colonization of roots and strengthening of beneficial mutual interactions between plants and their coupled bacteria (Gutiérrez-Luna et al., 2010). These chemical cross talks/communications might regulate how plant will perform in various agricultural soils (Camarena-Pozos et al., 2018). The effect is achieved by affecting root growth and improving availability of nutrients in the rhizosphere along with other beneficial contributions w.r.t. plant immunity by the microorganisms (Ortíz-Castro et al., 2009). Inoculation of A. thaliana with Bacillus megaterium UMCV1 resulted in altered root-system architecture with inhibition of primary-root growth and an increase in number and length of lateral roots. The solid-phase microextraction coupled to a gas chromatography—mass spectrometry (SPME-GC-MS) based analysis identified acetoin in the volatiles produced by UMCV1 (López-Bucio et al., 2007). VOCs (aldehydes, ketones and 1-butanol) released by some Bacillus isolates (L254, L263, L265a, L265b, L266, L270, L271, and L272a) resulted in modulation of root-system architecture in A. thaliana plants, consequently resulting in enhanced biomass (Gutiérrez-Luna et al., 2010). Farag et al. (2006) also reported 1-butanol among the VOCs produced by B. subtilis GB03 and B. amyloliquefaciens IN937a by using SPME-GC-MS technique. Ryu et al. (2003) reported the stimulatory effect of acetoin or 2,3-butanediol producing Bacillus strains on A. thaliana growth. The volatiles of B. subtilis GB03 stimulated plant growth via signaling of cytokinin and ethylene, whereas, VOCs of B. amyloliquefaciens IN937a exerted their effect independently without the involvement of cytokinin and ethylene (Ryu et al., 2003; Farag et al., 2006), indicating the role of different VOCs in phytostimulation. Gutiérrez-Luna et al. (2010) detected butyrolactone in the VOCs produced by Bacillus sp. strain L265a. Butyrolactone as an autoinducer of bacterial quorum-sensing (QS) signaling has also been reported (Polkade et al., 2016).
Tobacco (Nicotiana attenuata L.) plants cultivated under sulfur-limiting conditions showed enhanced growth under the influence of dimethyl disulfide produced by Bacillus sp. strain BG55. The observed effect was partially attributed to absorption and assimilation of dimethyl sulphoxide (Meldau et al., 2013). Cordovez et al. (2018) demonstrated that VOCs from Microbacterium sp. strain EC8 caused an increase in root biomass and lateral root density in A. thaliana plants. The authors also reported similar effects in other crop plants like lettuce (Lactuca sativa L.) and tomato. Further, enrichment of sulfur-containing compounds like dimethyl disulfide and dimethyl trisulfide, compounds found usually from bacteria, and also rarer compounds, such as S-methyl 2-methylpropanethioate and S-methyl pentanethioate was also observed in the headspace of EC8. Genome-wide transcriptome analyses further identified 1,361 (698 upregulated and 663 downregulated) differentially expressed genes (DEGs) in root tissues induced by microbial VOCs. In root tissues, DEGs involved in sulfur metabolism were mostly downregulated, DEGs involved in nitrate assimilation were upregulated, whereas DEGs involved in nitrate reduction were downregulated. While, upregulated expression of genes encoding three nitrate transporters (NRT2.1, NRT2.6, and NRT2.7) and a chlorine channel (CLC-A) proteins was observed. Other genes involved in nitrate-related processes, like NIA1 and NIA2, encoding nitrate reductases, were downregulated. It has been reported that nitrate is not a mere nutrient for plants but also acts as a signal for regulating metabolism of carbon and nitrogen (Scheible et al., 1997). The transporter gene of nitrogen, NTR2.1, displayed an upregulation of 14-folds in A. thaliana when exposed to VOCs from EC8, is reported to be regulated by nitrate and act as a negative regulator of lateral root initiation under high-sucrose and low-nitrate conditions, whereas NRT2.6 is reported to be a part of growth stimulation of A. thaliana by the rhizobacterium Phyllobacterium brassicacearum STM196 (Little et al., 2005; Kechid et al., 2013). The transporter gene NRT2.6, along with NRT2.5, was upregulated in the leaves of A. thaliana that were inoculated with the bacteria, thereby inferring that these genes may be a part of the regulation of nitrogen control for root development (Kechid et al., 2013). The identification of the bioactive volatiles produced by PGPR and characterization of their ecological functions can open up new avenues for improving crop productivity in a sustainable way.
Microbial Nitric Oxide (NO) in Plant Signaling
Nitric oxide is a small, diffusible, lipophilic, volatile free radical and a ubiquitous bioactive molecule, which participates in a broad spectrum of metabolic, signaling, defense, and developmental pathways (Lamattina et al., 2003). The NO has the potential to operate simultaneously on a number of discrete biochemical nodes via modulation of cell redox status and Ca2+ cytosolic concentrations. This unique property makes NO a molecule with remarkable signaling as well as homeostatic characteristics for the synchronization and organization of cellular metabolism (Lamattina et al., 2003). Many studies suggest extensive linkage between NO and plant hormones (Molina-Favero et al., 2008).
Rhizobacteria can produce NO by different pathways (Molina-Favero et al., 2008; Arasimowicz-Jelonek and Floryszak-Wieczorek, 2014). Bacterial nitric oxide synthase (bNOS) can synthesize NO by oxidation of L-arginine to L-citrulline, in presence of oxygen (Stuehr, 1997). The other major pathway for NO synthesis in free bacteria is through the process of anaerobic denitrification. The established denitrification pathway is a multistep process that reduces nitrate () to nitrogen N2 via activities of nitrate reductase (NR), nitrite reductase (NiR), NO reductase (NoR), and N2O reductase (Meilhoc et al., 2011). Nitric oxide is synthesized as a free intermediate by the enzyme nitrite reductase. In addition, denitrification can also occur under aerobic conditions. The process involves a periplasmic nitrate reductase (Nap) rather than the classical membrane bound respiratory nitrate reductase (Nar) found in anaerobic denitrification. Steenhoudt et al. (2001) identified a Nap in Azospirillum brasilense Sp245 which is not sensitive to oxygen. Another pathway for bacterial NO production is heterotrophic nitrification which causes sequential oxidation of ammonia to hydroxylamine (NH2OH), , and , with NO being produced as an intermediate during the reduction of to N2 (Wrage et al., 2001). Pothier et al. (2007) reported that nirK gene in A. brasilense Sp245 encodes a NO-producing nitrite reductase copper-containing enzyme. Further, the expression of nirK was induced by wheat seed or root extracts (Pothier et al., 2007).
Bloom et al. (2003) have very well-reviewed various molecules and signals which are involved in development of roots. Amongst them, nitrogen species as NO, ammonium and nitrate are evidently occupied in growth and development of roots. NO production by the microbial partner in the legume–Rhizobium symbiosis has been reported by Horchani et al. (2011). Various approaches have also shown that denitrification is responsible for generation of NO in bacteroids, especially during hypoxic conditions (Meakin et al., 2007; Sanchez et al., 2010). In addition, Horchani et al. (2011) also demonstrated that around one-third of the NO generated by Medicago truncatula–Si. meliloti nodules is synthesized by the rhizobial denitrification pathway. Gouvêa et al. (1997) indicated that application of NO donors like sodium nitroprusside (SNP) and nitrosoglutathione stimulated growth and elongation in maize roots, thus postulating the intermediary role of NO in IAA-induced root elongation. Pagnussat et al. (2002, 2003) showed that NO acts as a signal molecule in the IAA-induced signaling pathway, and thus causing development of adventitious roots in cucumber (Cucumis sativus L.). The authors also noted that application of two NO donors, SNP and S-nitroso, N-acetyl penicillamine (SNAP) to hypocotyl cuttings of cucumber could induce de novo root-organogenesis, whereas application of NO scavangers, 2-(4-carboxyphenyl)-4,4,5,5-tetramethylimidazoline-1-oxyl-3-oxide (cPTIO) with SNP or SNAP blocked the effect. Microscopic observation revealed similar anatomical structure in NO- and IAA- induced roots. Moreover, the hypocotyls treated with SNP or SNAP along with IAA showed enhanced response and displayed better root organogenesis as compared to the hypocotyls treated with NO donors or IAA alone (Pagnussat et al., 2002). Pagnussat et al. (2003) strongly indicated the role of endogenous NO as a downstream component in the IAA-mediated root organogenesis. Correa-Aragunde et al. (2004) reported that NO has a major role in lateral root formation (LRF) in tomato. Molina-Favero et al. (2008) analyzed the aerobic NO production in A. brasilense Sp245 and its mutants Faj009 (IAA attenuated) and Faj164 (lacking periplasmic nitrate reductase activity) and correlated it with root-organogenesis in tomato. The strains Sp245 and Faj009 could produce 120 nmols of NO per g bacteria, whereas, strain Faj164 could produce only 5.6 nmols of NO per g bacteria, indicating aerobic denitrification as major source of NO in bacteria. Inoculating tomato seedlings with Sp245 and Faj009 could promote both lateral and adventitious roots formation, whereas no such effect was observed with Faj164 inoculation, indicating the role of NO in Azospirillum-induced root branching, without any regards to the potential of bacteria to synthesize IAA. Creus et al. (2005) investigated the ability of aerobically grown Azospirillum brasilense Sp245 to synthesize NO, using NO specific fluorescent probe 4, 5-diaminofluorescein diacetate (DAF-2 DA), followed by epifluorescence microscopy. Quantification of NO was done by electron paramagnetic resonance (EPR). The production of green fluorescence indicated the production of NO which was drastically reduced in presence of specific NO scavenger 2-(4-carboxyphenyl)-4,4,5,5-tetramethylimidazoline-1-oxyl-3-oxide (cPTIO). They further studied the role of NO as the inducer molecule in the Azospirillum-mediated stimulation of the tomato roots by incubating Azospirillum-inoculated and uninoculated tomato roots with DAF-2 DA followed by fluorescence microscopy. The Azospirillum inoculated roots exhibiting induction of lateral root formation also showed high fluorescence intensity majorly in the vascular tissues and sub-epidermal cells of roots. Treatment with cPTIO completely blocked the Azospirillum-mediated induction of lateral root formation (LRF), while addition of NO donor sodium nitroprusside partly reversed the inhibitory effect of cPTIO. These results strongly indicated the role of NO in the Azospirillum-mediated root growth promotion in tomato seedlings. Other studies have attributed the ion nitrite produced by Azospirillum metabolism to be accountable for the observed effects on the plant growth and other developmental processes on inoculation of roots (Didonet and Magalhaes, 1993).
The auxin competitive inhibitor, p-chlorophenoxyisobutyric acid (PCIB) was found to reduce the stimulating effect of Azospirillum brasilense Sp245 on LRF, indicating the involvement of auxins in the Azospirillum-induced effects (Creus et al., 2005), possibly causing an increase in the levels of NO as reported previously also (Pagnussat et al., 2002). In all together, these findings very well-support the hypothesis that NO is necessary for the Azospirillum-promoted LRF and both auxins and NO, act as cellular messengers that are involved in the close communication between Azospirillum and plant root leading to LRF. The increased build-up of NO in the inoculated plants can also be a result of some events rather than production of NO by Azospirillum itself, viz. (i) induced reduction of nitrite into NO in inoculated plant roots (Stohr and Ullrich, 2002); (ii) acidification of the root apoplast by Azospirillum which eventually induce a non-enzymatic production of NO in plant roots (Bethke et al., 2004). As majority of the studies indicate, effect on the root traits of plants can also result from the indirect stimulation of the plant auxin pathway by microbially produced NO in the rhizosphere.
Contributions of PGPR to Agroecosystem Functioning
Colonization of plant roots with bacteria is mutually beneficial for plant and bacteria. Plants secrete fixed carbon by root exudation and feed the rhizosphere bacteria and in turn bacteria help the plant through exhibiting an array of growth promoting traits. Apart from direct and indirect PGP traits, PGPR mediated altered root traits also contribute to agroecosystem functioning and plant productivity as discussed in the following sub-sections.
PGPR and Soil Structure
Root adhering soil play important role in uptake of nutrients and water for plant growth. Root traits play key role in shaping the physical structure of soils (Leifheit et al., 2013). Morphological root traits, such as root length density and root diameter, strongly influence soil stability because denser, finer root systems bind soil more effectively than do coarse root systems (Loades et al., 2010). As the diameter of larger root increases with growth, the soil particles adjacent to the root get pushed aside thus increasing the soil density. Finer roots on the other hand increase the soil porosity by improving the soil aggregation (Gyssels et al., 2005). Architectural root traits such as branching of roots helps in increasing the anchorage by fixing the soil, hence increasing soil stability and protection against erosion (Bardgett et al., 2014). PGPR strains able to enhance root morphological, physiological, and architectural characteristics thus can help in improving soil structure and stability. However, focussed studies need to be conducted to have direct evidence on PGPR mediated improvement of soil structure mediated through root traits.
Root exudation is another root trait that has strong influence on soil aggregation and stability, as the polysaccharides (mucilaginous substances) and proteins present in the root exudates act like glue, that bind clay and mineral particles together to form soil aggregates (Czarnes et al., 2000; Whalley et al., 2005). PGPR inoculation has been found to enhance rhizodeposition by the host plant (Kudoyarova et al., 2014), that provide nutritional niche for the rhizosphere microbial communities. However, not much information is available on how the PGPR mediated alteration in quality and quantity of rhizodeposits influences soil structure. Nonetheless, many of the rhizosphere microorganisms produce exopolysacharides (EPS) for attachment to other cells, soil particles and root surfaces. EPS bind with the soil particle to form aggregates and stabilize the soil structure. The improved structure increases cation exchange and water holding capacity of the soil (Upadhyay et al., 2011). EPS producing Rhizobium spp. strain YAS34 significantly increased the root adhering soil (RAS) aggregation and soil macropore volume. The inoculation also improved root diameter and overall plant growth of sunflower (Helianthus annuus L.) (Alami et al., 2000). This significant increase in RAS mass around the roots of inoculated plants could be the result of either an increase in soil adhesion to roots or a higher soil aggregate stability around roots, or both (Alami et al., 2000). The improvement in soil aggregation due to microbial inoculation may be attributed to microbial EPS. Seed inoculation of sunflower with an EPS producing Pseudomonas putida strain GAP-P45 resulted in increased root adhering soil/root tissue ratio, increased the stable soil aggregates percentage and also improved plant biomass under drought stress (Sandhya et al., 2009). Two salt-tolerant strains Halomonas variabilis HT1 and Planococcus rifietoensis RT4 able to form biofilm and accumulate EPS, improved chickpea (Cicer arietinum Var. CM-98) plant growth and soil aggregation under salinity stress (Qurashi and Sabri, 2012). Similar effects on RAS improvement have been observed in wheat under P. polymyxa CF43 inoculation (Gouzou et al., 1993). Mutation in sacB gene (encoding levansucrase for synthesis of levan) of P. polymyxa impaired its ability to cause soil aggregation, thus implicating the role of levan produced by P. polymyxa in the aggregation of root-adhering soil in wheat. EPS producing Bacillus amyloliquefaciens p16 improved soil aggregation and soil water retention (Deka et al., 2018). Inoculation of EPS producing Pantoea agglomerans strain NAS206 in wheat increased aggregation and stabilization of root-adhering soil, as indicated by significant increase in aggregate mean weight diameter, aggregate macro-porosity, RAS/RT ratio, and water stable aggregates (Amellal et al., 1998). Similarly, inoculation with EPS producing rhizobacteria (Planomicrobium chinense P1, Bacillus cereus P2, and Pseudomonas fluorescens P3) in wheat significantly enhanced the water holding capacity of sandy soil and improved soil aggregation around the plant roots (Khan et al., 2016). Under field conditions, inoculation with EPS producing Pseudomonas mendocina Palleroni along with Glomus intraradices, an arbuscular mycorrhizal fungus onto lettuce showed stabilization of soil aggregation (Kohler et al., 2006).
PGPR can contribute toward soil structure and stability through EPS production, influencing root exudation and other root traits. However, intricacies of the mechanisms need to be explored through focussed studies on these aspects.
PGPR and Carbon Sequestration
Soil is incorporating 6,000 billion tons of carbon approximately and acts as natural reservoirs of biogeochemical carbon cycle. More carbon is getting emitted into the atmosphere due to industrial development (Cristea et al., 2020). Microorganisms can sequester carbon into the soils and is sustainable method to lowering atmospheric carbon. Selection of suitable microbial inoculant is important to improve agriculture land capability to sequester and store carbon (Ahmed et al., 2019). Microbial consortia of (Pseudomonas protegens, Bacillus paramycoides and Bacillus paramycoides) when applied in tall perennial aromatic grass species including vetiver (Vetiveria zizanioides), lemongrass (Cymbopogon citratus), palmarosa (Cymbopogon martinii), and citronella (Cymbopogon winterianus) improved the carbon sequestration (Maddhesiya et al., 2020). High C: N ratio of soil treated with PGPR highlight the significant role of inoculants in enhancing carbon sequestration. Co-inoculation of rhizobacteria and cyanobacteria significantly increased the grain yield of rice and showed positive influence on carbon sequestration in soil (Prasanna et al., 2011). Under elevated CO2 soil microbe Pseudomonas fluorescens increased the capacity to store carbon and nitrogen in plant (Nie et al., 2015). Combined application of rice husk biochar (RHB) along with PGPR (Pseudomonas species, Azotobacter chroococcum, and Azospirillum brasilense) resulted in significantly higher rice yield, nutrient uptake, and also increased organic content of soil (Singh et al., 2017). Glomalin-Related Soil Protein (GRSP) improves the soil quality, C and N storage. Cell walls of hyphal Arbuscular mycorrhizal fungi (AMF) produce glomalin and, death of hyphae deposited in the soil, further incorporated into soil organic matter (SOM) pool. Interaction of AMF and PGPR improved the glomalin production in rhizosphere of pea (Pisum sativum L.) and further enhanced C and N storage in rhizosphere (Walley et al., 2014). In boreal forest ecosystems root-associated fungi acts as organic decomposers and belowground mediators for C transport and respiration (Clemmensen et al., 2013).
PGPR in Water and Nutrient Acquisition
For efficient nutrition in plants, the acquisition of nutrients by the roots plays the most important role (Gutschick, 1993). Efficiency in acquisition depends on root size and morphology, physiology and biochemistry. Root exudation influence the soil nutrient cycling by exuding compounds rich in organic acids that increase availability of nutrients by desorption and solubilization from mineral surfaces and by priming the rhizospheric microbial communities for enhanced mineralization of organic matter resulting in increased nutrient availability in the soil (Bardgett et al., 2014). However, priming of microbial growth by root exudates can also increase immobilization of nutrients, thereby reducing their availability to the plants (Bengtson et al., 2012). Thus a fine balance between mineralization and immobilization dynamics is probably controlled by variations in the quality of root exudates, the extent of nutrient limitation for microbial growth (Dijkstra et al., 2013; Drake et al., 2013), and the response of specific groups of microbes involved in nutrient transformations (Bremer et al., 2007). The relationships between root traits and nutrient cycling have been studied in the field and mixed results have been reported.
PGPR may improve the plant nutrition by affecting the plant nutrient uptake and/or the plant growth rate (Mantelin and Touraine, 2004). It is generally considered that increase in root surface area triggered by PGPR, results in enhanced nutrient uptake (Vacheron et al., 2013). However, the activity of root ion transporters can be regulated according to the nutritional demand of the plant (Lappartient et al., 1999). Hence, PGPR must interfere with the development and nutrition pathways of the host plant to modulate both nutrient acquisition and growth (Vacheron et al., 2013). Maize cultivar Seiddi inoculated with A. lipoferum CRT1 exhibited increased lateral root growth and enhanced photosynthetic potential unlike non-responsive cultivar FuturiXX (Rozier, 2016). GC-MS based profiling of small organic substances in the ascending xylem sap of plantlets revealed reduced content of 17 substances, including primary metabolites, such as glucose, maltose, sucrose, TCA cycle intermediates, GABA, amino acids and shikimate pathway metabolites in both the cultivars after A. lipoferum CRT1 inoculation. Whereas, the content of 28 substances, including glucose, lactic acid, acidic intermediates of the pentose phosphate and ascorbate/aldarate pathways and defense-related hydroxycinnamic acids, decreased in the xylem sap of the A. lipoferum-phytostimulated plantlets of responsive cultivar (Seiddi) only, suggesting the role of xylem-transported metabolic signaling in A. lipoferum induced phytostimulation of maize. Glucose or other metabolites that are retrograde transported through the xylem to the shoot by transpirational pull may act as feedback signals of the root status. Such signals may stimulate leaves to enhance photosynthesis-mediated C-assimilation that is needed to sustain A. lipoferum-triggered root growth (Rozier, 2016).
Li et al. (2020) reported improved nutrient acquisition of N, P, and K+ in maize by novel PGPR strain Kocuria rhizophila Y1. Inoculation of consortium of Bacillus megaterium BHU1, Arthrobacter chlorophenolicus BHU3, and Enterobacter sp. BHU5 significantly improved Cu, Zn, Mn, and Fe nutrients in wheat (Kumar et al., 2014). According to Sabir et al. (2012) root inoculation with PGPR strains Azospirillum brasilense Sp245 and Bacillus subtilis OSU-142 enhanced growth and nutrient acquisition (N, P, K, Ca, and Mg) in grapevine rootstocks (Vitis spp.). Inoculation of soybean with a consortium of Pseudomonas aeruginosa LSE-2 and Bradyrhizobium sp. LSBR-3 exhibiting multiple PGP traits (IAA, P solubilization, ACC deaminase, biofilm formation) enhanced root dry weight, nodule number, dry weight, leghaemoglobin content, and nutrient (N, P, K) acquisition (Kumawat et al., 2019) adding toward overall plant growth and yield. Safflower (Carthamus tinctorius L.) inoculation with Bacillus strains (B. subtilis OSU-142 and B. megatorium M3) enhanced nutrient acquisition and improved seed and oil yield under semi-arid condition (Ekin, 2020). Treatment with rhizobacteria (Pseudomonas sp. R185) and endophytic bacteria (Pseudomonas mosselii E240) had a positive effect on P, Zn, and Fe contents in wheat grains in phosphorous deficient soils (Emami et al., 2018). Rana et al. (2012) reported 2-fold enhancement in P and 66.7% increase in the N content in leaves of wheat treated with a combination of Bacillus sp. AW1 and Providentia sp. AW5. They also observed significant correlation between plant biomass, grain weight, N, P, and Fe contents with acetylene reduction activity of rhizosphere soil, indicating the role of N fixation in crop productivity.
PGPR can directly increase bioavailability of nutrients through nitrogen fixation and nutrient mobilization of key nutrients (iron, phosphorous, and potassium) to crop plants (Rashid et al., 2016). Phosphate solubilization is an important effect of PGPR on plant nutrition. Although, the soils generally have high levels of phosphorus, most of the soil P exists in fixed forms and only a small proportion is available for plants. Soil microorganisms play important role in the solubilization and mineralization of organic or insoluble forms of P into plant available forms (Richardson et al., 2009; Ramaekers et al., 2010) through the release of organic acids and hydrolytic enzymes (phosphatases and phytases) (Richardson and Simpson, 2011).
Plant phosphate use efficiency (PUE) is generally correlated with P acquisition efficiency and with root length and architectural traits (Ruiz et al., 2019). Root hairs can increase the effective surface area of plant roots and help the roots to exploit non-accessible stocks of P through accessing the finer pores than the main root axis can enter, thus length and density of root hairs play important role in nutrient acquisition (Ma et al., 2001). PGPR able to influence the root traits including root length, lateral branching, and root hair length and density, thus can help in improving plant nutrition acquisition capacity.
Biological nitrogen fixation is another important trait of many PGPR that contributes to fulfill the nutritional demands of the plant. Nitrogen fixing bacteria possess the nitrogenase enzyme that converts atmospheric elemental dinitrogen into ammonia. Nitrogen fixing PGPR can be symbiotic, associative or free living. Symbiotic nitrogen fixers like rhizobia and Fankia form nodules on the plants root by modifying the root architecture and can benefit the plant by directly supplying the fixed form of N in the root nodules (Brock, 2000). Rhizobium-legume symbiosis is most studied for its nitrogen contributions. Rhizobia can fix 180 × 106 tons of nitrogen annually through BNF process at global level (Sahgal and Johri, 2003) which causes efficient increment in the productivity and quality of crops (Herridge et al., 2008; Krapp et al., 2011).
Associative nitrogen fixers like Azospirillum are known for their effect on root architecture and associative nitrogen fixation in many crops (Bashan et al., 2004). The important free living and associative nitrogen fixing genera include Azospirillum, Azotobacter, Gluconacetobacter, Bacillus, Burkholderia, Clostridia, Kelbsiella, Mycobacterium, and Pseudomonas.
With increasing attention on the genetic, molecular and physiological regulation for root architecture as related to plant nutrient efficiency, a number of genes and regulators have been identified and demonstrated to participate in regulatory networks linking root architecture to nutrient efficiency in different crops including Arabidopsis, maize, rice, soybean (Li X. et al., 2016). The expression studies of these genes and regulators under the influence of PGPR inoculation can help in understanding the regulatory role of PGPR in nutrient acquisition.
PGPR and Abiotic Stress Tolerance in Plants
Abiotic stresses include excessive or deficient water, high or low temperature, high salinity, heavy metals, and ultraviolet radiation, are malicious for growth and development of plant, lead to crop yield losses (He et al., 2018). PGPR plays defensive role against abiotic stresses (Kaushal and Wani, 2016). While it is difficult to elucidate the explicit mechanism by which PGPR act to enhance drought tolerance by affecting root traits of the plant, in certain cases it has been demonstrated. For example, Sandhya et al. (2009) reported production of extracellular matrices by PGPR for the maintenance of a hydrated root environment and thus, increasing root-adhering soil and stability. In drought stressed A. thaliana plants, inoculation with spermidine producing Bacillus megaterium BOFC15 exhibited reduction in reactive oxygen species, upregulation of ABA biosynthesis and response genes, and augmented photosynthesis and root system architecture (Zhou et al., 2016). In a study, thuricin produced by Bacillus thuringiensis NEB17 was applied to soybean (Glycine max) under drought conditions and it caused modification in root structures and increase in root length and biomass, nodule biomass, ABA content, and total nitrogen content (Prudent et al., 2015). IAA producing strains Pseudomonas putida, Pseudomonas sp., and Bacillus megaterium could stimulate shoot, root biomass and water content in white clover plants (Trifolium repens) under drought condition. Bacillus megaterium was found to be more effective when applied along with arbuscular mycorrhizal fungi against drought (Marulanda et al., 2009). Limited water availability results in low rate of photosynthesis and insufficient nutrient uptake. In wheat, inoculation of phosphorus-solubilizing and drought-tolerant PGPR Pseudomonas libanensis EU-LWNA-33 improved plant growth and phosphorus uptake under water deficient condition (Kour et al., 2019). Inoculation with Bacillus sp. 12D6 and Enterobacter sp. 16i delayed onset of drought symptoms in wheat and maize seedlings. Inoculation of wheat with Bacillus sp. (12D6) increased the root length, whereas, in maize inoculation with both Bacillus sp. strain 12D6 and Enterobacter sp. strain 16i increased the root length and surface area (Jochum et al., 2019). Seed treatment with endophytic bacteria Bacillus subtilis MSEB78 and Corynebacterium hansenii MSEB3 improved chlorophyll content, relative water content, shoot fresh and dry biomass under drought stress (Bodhankar et al., 2019).
Among the various PGP traits implicated in conferring drought tolerance, synthesis of enzyme ACC deaminase is most studied. Through production of ACC deaminase, PGPR maintain the level of plant stress hormone ethylene below inhibitory levels, maintaining normal root growth by diverting the excess of auxins and delaying senescence under drought (Marasco et al., 2013). ACC deaminase producing PGPR strains Ochrobactrum sp. SB2.ACC2, Bacillus sp. SB1.ACC3, and Alcaligens sp. SB1.ACC2 reduced ethylene production and promoted the plant growth by inducing salinity tolerance in rice (Bal et al., 2012). Inoculation of Jerusalem artichoke (Helianthus tuberosus L.) with IAA and ACC deaminase producing Bacillus aquimaris strain 3.13 and Micrococcus luteus strain 4.43 drought conditions increased shoot height and weight, length, diameter, volume, area of root ultimately improving harvest index (Namwongsa et al., 2019). Similarly, Rice (Oryza sativa) seedlings inoculated with IAA and ACC deaminase producing Pseudomonas fluorescens strain REN, exhibited enhanced root length under flooding (Etesami and Alikhani, 2016). The ability of PGPR to produce both auxins and ACC deaminase becomes particularly important under stress conditions (Glick, 2014). Phytohormones mediated increase in root length, root surface area, and the number of root tips, causing increased uptake of water and nutrients, represents a major contributor for the improvement of survival chances of the plant under water limiting conditions (Glick, 2011; Marasco et al., 2013). ACC deaminase producing Pseudomonas lini DT6 and Serratia plymuthica DT8 mitigated drought stress and promoted the growth of jujube plant by regulating ABA and IAA (Zhang et al., 2020). ACC demainase producing rhizobacteria Enterobacter cloacae and Achromobacter xylosoxidans amended with biochar improved the maize growth and productivity under drought stress (Danish et al., 2020). Root colonization of wheat plants with IAA and ACC deaminase producing Stenotrophomonas maltophila SBP-9 improved root, shoot length and biomass, chlorophyll content under salinity stress. Inoculation decreased level of proline and malondialdehyde (MAD), and increased activities of antioxidant enzymes, superoxide dismutase (SOD), catalase (CAT), peroxidase (POX) (Singh and Jha, 2017). The PGPR exhibit enzymatic and non-enzymatic antioxidant responses to scavenge the toxic compounds like reactive oxygen species produced under stress conditions. In Okra (Abelmoschus esculentus) combined inoculation with Bacillus megaterium UPMR2 and Enterobacter sp. UPMR18 improved germination percentage, plant growth parameters (shoot fresh weight and root dry weight) and adapted to salinity stress by eliminating reactive oxygen species (ROS) through SOD (superoxide dismutase), CAT (catalase) and APX (Ascorbate peroxidase) activities (Habib et al., 2016). PGPR inoculation increased proline accumulation in soybean, lettuce, wheat, maize plants under abiotic stress conditions (Han and Lee, 2005; Kohler et al., 2009; Zarea et al., 2012; Bodhankar et al., 2019). Salt tolerant PGPR Bacillus aquimaris SU8 accumulated total soluble sugars, improved growth and yield of wheat under saline soil (Upadhyay and Singh, 2014). Priming of wheat seeds with Bacillus safensis (NCBI JX660689) and Ochrobactrum pseudogrignonense (NCBI JX660688) improved temperature stress tolerance of wheat seedlings by lowering ROS levels, increasing chlorophyll content and accumulating osmolytes (Sarkar et al., 2018).
Role of VOCs released by PGPB have been implicated in stress tolerance. VOCs produced by Bacillus subtilis GB03 stimulated choline synthesis in A. thaliana that helped in maintaining cell turgor through preventing the water loss (Zhang et al., 2010). Moreover, Pseudomonas chlororaphis O6 induced stomata closure in the colonized plants, by producing VOC, 2R, 3R-butanediol (Cho et al., 2011). In mungbean [Vigna radiate (L.) R. Wilczek] inoculation with Pseudomonas aeruginosa GGRJ21 strain elicited drought tolerance as indicated from better plant growth, osmolyte accumulation, antioxidant enzyme status, and up-regulation of drought responsive genes i.e., dehydrin (DHN), catalase (CAT1), and dehydration-responsive element binding protein (DREB2A) (Sarma and Saikia, 2013). Sheibani-Tezerji et al. (2015) reported up-regulation of genes involved in detoxification of reactive oxygen species (ROS) in the drought-exposed potato (Solanum tuberosum) plants colonized with root endophytic bacteria Burkholderia phytofirmans PsJN. Similarly, Pseudomonas putida MTCC5279 inoculation improved drought stress response of chick pea as indicated from gene expression (stress responsive genes), membrane stability, water status, plant growth, antioxidant enzymes (Tiwari et al., 2016). Treatment of wheat seedlings with Bacillus velezensis 5113 exposed to cold/freezing, heat or drought stress, improved the plant survival under stress conditions. Protein profile of wheat leaves showed differential expression of several proteins with cold stress showing strong impact on protein profile than heat and drought (Abd El-Daim et al., 2019). PGPR also help the plant under stress conditions by nutrient cycling through diazotrophy, phosphorus solubilization, and siderophore synthesis, by enhancing photosynthesis, increasing fine root production and overall root surface area and reducing the stress ethylene emission, thus contributing toward better plant performance (Casanovas et al., 2002; Timmusk et al., 2014; Gagné-Bourque et al., 2016). Thus, PGPR through various direct and indirect mechanisms (Figure 3) can enhance plant's tolerance to abiotic stress, hence contributing toward sustainable production.
PGPR and Biotic Stress Tolerance in Plants
Use of chemical inputs to control phytopathogens poses a serious threat to soil fertility, crop productivity, and nutritional value of farm produce. Application of PGPR can help in mitigating biotic stresses and thus improving crop yield in a sustainable way (Sayyed, 2019). The antagonistic relationship between microbes and plant pathogens may be complex. It may involve the competition for nutrient and space, production of antimicrobial compounds or triggering of the host defensive response (Figure 3). PGPR eliciting ISR may modulate defense responses of the plant to their own benefit (Zamioudis and Pieterse, 2012). In the absence of pathogens ISR poses slight fitness costs on the plant, however, the benefits of ISR greatly outweigh the costs when the plant is attacked by pathogens. The cross communication between the plant and the ISR-eliciting PGPR, reduce the susceptibility of the plant to pathogen attack (Choudhary et al., 2007; Bakker et al., 2013).
Many PGPR especially Pseudomonas and Bacillus species have been well-reported to play important roles in plant growth promotion through the biocontrol of a broad range of plant pathogens, by eliciting ISR, production of antimicrobial compounds (lipopeptides, antibiotics, and enzymes) and acting as competitors for growth factors (space and nutrients) with other pathogenic microorganisms through colonization (Pal et al., 2001; Haas and Defago, 2005; Weller, 2007; Loganathan et al., 2014; Singh et al., 2014; Bodhankar et al., 2017; Shafi et al., 2017; Hashem et al., 2019; Wang et al., 2019). Certain PGPR like Azospirillum spp. are reported to reduce the damage caused by plant pathogens through indirect mechanisms like competition and displacement of pathogens, general enhancement of plant health to resist pathogen attack, and possible inhibition of fungal growth via the production of toxic substances (Bashan and de-Bashan, 2010). The role of Rhizobium spp. in disease management has been associated with production of lytic enzymes, antimicrobial metabolites and ISR. Also, rhizobial mediated plant growth promotion and/or symbiotic efficiency also contribute toward disease suppression by the host plant (Volpiano et al., 2019).
Umpteen literature repots provide evidence for PGPR mediated pathogen control in different plant. For example, a PGPR Bacillus subtilis BS2 was found to be effective under field condition against a pathogen Fusarium oxysporum f.sp. lycopersici causing tomato wilt. Tomato plants pretreated with B. subtilis BS2 has significantly induced defense mechanisms viz., polyphenol oxidase, peroxidase, chitinase, phenolics and phenylalanine ammonialyase. Inoculation also improved the nutritional quality and fruit yield (Loganathan et al., 2014). Bacillus amyloliquefaciens SQR9 a beneficial bacteria showed strong antifungal activity against six tested soil-borne pathogens Fusarium solani, Verticillium dahliae Kleb, Rhizoctonia solani Kahn, Sclerotinia sclerotiovum, Fusarium oxysporum f. sp. cucumerinum, and Phytophthora parasitica var. nicotianae the bacterium produces a different spectrum of antifungal compounds in response to different fungal species (Li et al., 2014). In rice plants, inoculationwith B. amyloliquefaciens (SN13) enhanced immune response against R. solani by modulating various molecular, metabolic and physiological functions (Srivastava et al., 2016). Treatment with PGPR Bacillus amyloliquefaciens improved plant growth and induces resistance in chili (Capsicum annum L.) against anthracnose disease (Gowtham et al., 2018). Inoculation with Peanibacillus lentimorbus B-30488 in Nicotiana tabacum cv. white burley significantly reduced the level of cucumber mosaic virus RNA in the leaves. Inoculation caused an increase in the expression of pathogenesis-related (PR) genes and antioxidant enzymes suggesting induced resistance against the virus. Colonization by B-30488 improved tissue health and physiology of cucumber plants, which produced more flowers and seeds (Kumar et al., 2016). P. lentimorbus B-30488 also produced ACC deaminase and could induce tolerance against southern blight disease in tomato caused by S. rolfsii. The inoculated plants showed modulation of the ethylene pathway and antioxidant enzyme activities; systemic tolerance was corroborated by PR gene expression analysis (Dixit et al., 2016). PGPR strains fluorescent Pseudomonas sp. EM85 and two Bacillus spp. MR-11(2) and MRF isolated from maize rhizhosphere showed strong antagonistic activity against Fusarium graminearum, Macrophomina phaseolina, and Fusarium moniliforme causal agents of wilting and root rot, stalk rot/collar rot, and charcoal rot of maize, respectively (Pal et al., 2001). Seed treatment of chickpea with combined triple potential rhizosphere microbes, viz., Trichoderma harzianum THU0816, fluorescent Pseudomonas aeruginosa PHU094, and Mesorhizobium sp. RL091 significantly improved plant growth parameters and also triggered the defense in chickpea against the attack of Sclerotium rolfsii infection (Singh et al., 2014). In french bean (Phaseolus vulgaris) combined inoculation of Pseudomonas fluorescens along with AMF (arbuscular mycorrhizal fungi) has reduced the root rot incidence caused by Rhizoctonia solani apart from promoting plant growth and yield (Neeraj and Singh, 2011). Intercropping of cotton with Sesbania aculeata with PGPR (Azospirillum AZ204 and Pseudomonas fluorescens) inoculation significantly improved cotton yield and reduced the root rot incidence caused by Rhizoctonia bataticola (Marimuthu et al., 2013). Inoculation with Methylobacterium spp. reduced the disease intensity in tomato plants challenged with Ralstonia solanacearum through reduced ethylene levels and ACC accumulation in tomato plants and enhanced accumulation of PR proteins/defense enzymes (Yim et al., 2013). Inoculation with Enterobacter asburiae BQ9 induced resistance of tomato plants against yellow leaf curl virus through enhanced expression of antioxidant enzymes, including catalase, superoxide dismutase, peroxidase and phenylalanine ammonia lyase, and defense-related genes (Li H. et al., 2016). Acyl-homoserine lactones (AHL)-producing Serratia liquefaciens MG1 and P. putida IsoF elicited induced systemic resistance (ISR) in tomato against Alternaria alternata whereas AHL-null mutant strains of both PGPR resulted in reduced ISR (Schuhegger et al., 2006). Root exudates have been found to contain chemicals that mimic AHL signals, stimulating beneficial rhizosphere associations while inhibiting pathogenic bacteria (Teplitski et al., 2000). Treatment of PGPR Pseudomonas aeruginosa and Burkholderia gladioli in tomato plants controlled root-knot nematode infection through alteration in the synthesis of different secondary metabolites in plants (Khanna et al., 2019).
Studies have reported that plants under pathogen attack recruit beneficial microorganisms in their rhizospheres (Rudrappa et al., 2008; Lee et al., 2012) Weller et al. (2002) reported that in monocultures of wheat, after an initial outbreak of take-all disease, recruitment of 2,4-diacetylphoroglucinol producing pseudomonads occurs that results in increased disease suppressiveness. Mavrodi et al. (2012) also reported evidence in support of cry for help hypothesis in the wheat rhizosphere, in which 2,4-diacetylphloroglucinol producing pseudomonads were recruited under irrigated conditions (G. graminis var. tritici, sensitive to 2,4-diacetylphloroglucinol, is the major pathogen under irrigated conditions) and phenazine producing pseudomonads were recruited under dry conditions where R. solani, sensitive to phenazines, is the major pathogen.
As indicated by several studies, PGPR have tremendous potential as biocontrol agents and thus can contribute to sustainable agricultural production by reducing the pesticides load and thus saving the soil health.
Conclusions and Future Prospects
Benefits of PGPR to plant growth and yield are well-documented and majority of the studies have emphasized on plant parameters aboveground. However, interaction of PGPR with the plant starts in the rhizosphere through root exudation followed by attachment, colonization on the rhizoplane, and/or in the endorhizosphere. PGPR, by releasing various metabolites including phytohormones, and volatile organic compounds influence the root traits through influencing hormonal balance and/or root trait pathways. The microbial effect on the altered root traits may manifest in terms of root morphological, physiological, architectural, and biotic traits. The altered toot traits may contribute toward plant growth and development and ecosystem functioning through cumulative effects in terms of enhanced anchorage due to root branching, better uptake of soil resources due to increased root surface area as presented in Figure 4. PGPR inoculation improves soil aggregation due to increased microbial polysaccharide production. In addition, PGP traits exhibited by the PGPR communities like biological nitrogen fixation, solubilization of nutrients like P and K, biocontrol of plant pathogens and abiotic stress management etc. also contribute significantly to plant growth and yield. The application of fixing/mobilizing/acquisitioning PGPR can save significant amount of chemical fertilizers, besides contributing toward sustainable agriculture. However, the PGPR mediated effect on root traits and subsequent benefits to the plants and ecosystem services need to be estimated/calculated through systematic and focused research/methodologies. Seedling stage screening of presumptive PGPR strains for inducing beneficial root traits can be advantageously used for selecting promising PGPR strains. It is an interesting field of research with direct applications in sustainable agriculture.
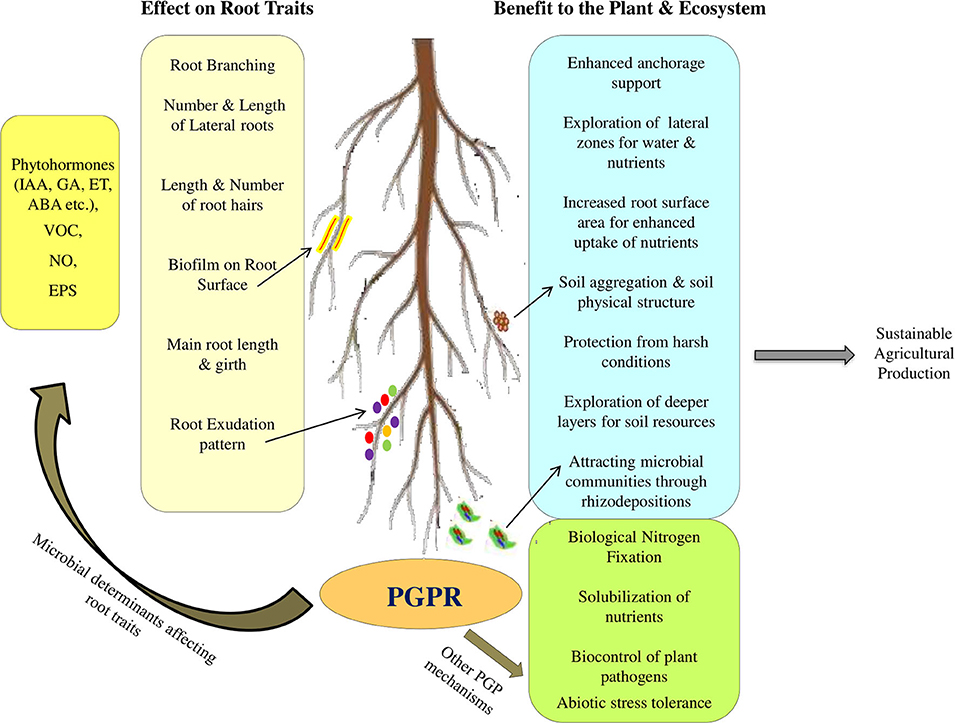
Figure 4. Conceptual presentation of PGPR mediated changes in root traits and the corresponding effects on plant growth and ecosystem functioning.
Author Contributions
MG and SB have written the major portions of the review article. AS, PS, and JS have written sections of the article. SB and JS have made the Table. LN has given her expertise in the field with relevant suggestions, and knowledge along with editing and improvising the review article. All authors contributed to the article and approved the submitted version.
Funding
The authors acknowledge Indian Agricultural Research Institute-ICAR for providing the necessary facilities and financial support.
Conflict of Interest
The authors declare that the research was conducted in the absence of any commercial or financial relationships that could be construed as a potential conflict of interest.
References
Abd El-Daim, I. A., Bejai, S., and Meijer, J. (2019). Bacillus velezensis 5113 induced metabolic and molecular reprogramming during abiotic stress tolerance in wheat. Sci. Rep. 9:16282. doi: 10.1038/s41598-019-52567-x
Ahmed, A. A. Q., Odelade, K. A., and Babalola, O. O. (2019). “Microbial inoculants for improving carbon sequestration in agroecosystems to mitigate climate change,” in Handbook of Climate Change Resilience, ed W. Leal Filho (Cham: Springer), 1–21. doi: 10.1007/978-3-319-71025-9_119-1
Ahn, S. J., Rengel, Z., and Matsumoto, H. (2004). Aluminum-induced plasma membrane surface potential and H+-ATPase activity in near-isogenic wheat lines differing in tolerance to aluminum. New Phytologist. 162, 71–79. doi: 10.1111/j.1469-8137.2004.01009.x
Alami, Y., Achouak, W., Marol, C., and Heulin, T. (2000). Rhizosphere soil aggregation and plant growth promotion of sunflowers by an exopolysaccharide-producing Rhizobium sp. strain isolated from Sunflower roots. Appl. Environ. Microbiol. 66, 3393–3398. doi: 10.1128/aem.66.8.3393-3398.2000
Aloni, R., Aloni, E., Langhans, M., and Ullrich, C. I. (2006). Role of cytokinin and auxin in shaping root architecture: regulating vascular differentiation, lateral root initiation, root apical dominance and root gravitropism. Ann. Botany 97, 883–893. doi: 10.1093/aob/mcl027
Amellal, N., Burtin, G., Bartoli, F., and Heulin, T. (1998). Colonization of wheat roots by an exopolysaccharide-producing Pantoea agglomerans strain and its effect on rhizosphere soil aggregation. Appl. Environ. Microbiol. 64, 3740–3747. doi: 10.1128/aem.64.10.3740-3747.1998
Anderson, A. J., and Guerra, D. (1985). Responses of bean to root colonization with Pseudomonas putida in a hydroponic system. Phytopathology 75, 992–995. doi: 10.1094/phyto-75-992
Antoun, H., and Prévost, D. (2005). “Ecology of plant growth promoting rhizobacteria,” in PGPR: Biocontrol and Biofertilization, ed Z. A. Siddiqui (Dordrecht: Springer), 1–38. doi: 10.1007/1-4020-4152-7_1
Arasimowicz-Jelonek, M., and Floryszak-Wieczorek, J. (2014). Nitric oxide: an effective weapon of the plant or the pathogen? Mol. Plant Pathol. 15, 406–416. doi: 10.1111/mpp.12095
Argaw, A. (2012). Characterization of symbiotic effectiveness of rhizobia nodulating Faba bean (Vicia faba L.) isolated from central Ethiopia. Res. J. Microbiol. 7, 280–296. doi: 10.3923/jm.2012.280.296
Argueso, C. T., Ferreira, F. J., and Kieber, J. J. (2009). Environmental perception avenues: the interaction of cytokinin and environmental response pathways. Plant Cell Environ. 32, 1147–1160. doi: 10.1111/j.1365-3040.2009.01940.x
Arkhipova, T. N., Galimsyanova, N. F., Yu, K. L., Vysotskaya, L. B., Sidorova, L. V., Gabbasova, I. M., et al. (2019). Effect of seed bacterization with plant growth promoting bacteria on wheat productivity and phosphorus mobility in the rhizosphere. Plant Soil Environ. 65, 313–319. doi: 10.17221/752/2018-pse
Arkhipova, T. N., Prinsen, E., Veselov, S. U., Martinenko, E. V., Melentiev, A. I., and Kudoyarova, G. R. (2007). Cytokinin producing bacteria enhance plant growth in drying soil. Plant Soil 292, 305–315. doi: 10.1007/s11104-007-9233-5
Arkhipova, T. N., Veselov, S. U., Melentiev, A. I., Martynenko, E. V., and Kudoyarova, G. R. (2005). Ability of bacterium Bacillus subtilis to produce cytokinins and to influence the growth and endogenous hormone content of lettuce plants. Plant Soil 272, 201–209. doi: 10.1007/s11104-004-5047-x
Arteca, R. N., and Arteca, J. M. (2008). Effects of brassinosteroid, auxin, and cytokinin on ethylene production in Arabidopsis thaliana plants. J. Exp. Bot. 59, 3019–3026. doi: 10.1093/jxb/ern159
Arumugam, R., Rajasekaran, S., and Nagarajan, S. (2010). Response of arbuscular mycorrhizal fungi and Rhizobium inoculation on growth and chlorophyll content of Vigna unguiculata (L) Walp Var. Pusa 151. J. Appl. Sci. Environ. Manag. 14, 113–115. doi: 10.4314/jasem.v14i4.63282
Asari, S., Tarkowska, D., Rolcik, J., Novak, O., Palmero, D. V., Bejai, S., et al. (2016). Analysis of plant growth-promoting properties of Bacillus amyloliquefaciens UCMB5113 using Arabidopsis thaliana as host plant. Planta 245, 15–30. doi: 10.1007/s00425-016-2580-9
Backer, R., Rokem, J. S., Ilangumaran, G., Lamont, J., Praslickova, D., Ricci, E., et al. (2018). Plant growth-promoting rhizobacteria: context, mechanisms of action, and roadmap to commercialization of biostimulants for sustainable agriculture. Front. Plant Sci. 9:1473. doi: 10.3389/fpls.2018.01473
Badar, R., and Qureshi, S. A. (2012). Comparative effect of Trichoderma hamatum and host-specific Rhizobium species on growth of Vigna mungo. J. Appl. Pharm. Sci. 128–132. doi: 10.7324/japs.2012.2409
Bakker, P.A, Doornbos, R. F., Zamioudis, C., Berendsen, R. L., and Pieterse, C. M. J. (2013). Induced systemic resistance and the rhizosphere microbiome. Plant Pathol. J. 29, 136–143. doi: 10.5423/PPJ.SI.07.2012.0111
Bal, H. B., Nayak, L., Das, S., and Adhya, T. K. (2012). Isolation of ACC deaminase producing PGPR from rice rhizosphere and evaluating their plant growth promoting activity under salt stress. Plant Soil 366, 93–105. doi: 10.1007/s11104-012-1402-5
Barbieri, P., and Galli, E. (1993). Effect on wheat root development of inoculation with an Azospirillum brasilense mutant with altered indole-3-acetic acid production. Res. Microbiol. 144, 69–75. doi: 10.1016/0923-2508(93)90216-O
Bardgett, R. D., Mommer, L., and De Vries, F. T. (2014). Going underground: root traits as drivers of ecosystem processes. Trends Eco. Evol. 29, 692–699. doi: 10.1016/j.tree.2014.10.006
Barriuso, J., Ramos Solano, B., Lucas, J. A., Lobo, A. P., Garca-Villaraco, A., and Gutirrez Maero, F. J. (2008). “Ecology, genetic diversity and screening strategies of plant growth promoting rhizobacteria (PGPR),” in Plant-Bacteria Interactions: Strategies and Techniques to Promote Plant Growth, eds I. Ahmad, J. Pichtel, and S. Hayat (WILEY-VCH Verlag GmbH & Co). 1–17. doi: 10.1002/9783527621989.ch1
Bashan, Y., and de-Bashan, L. E. (2010). How the plant growth-promoting bacterium Azospirillum promotes plant growth – a critical assessment. Adv. Agron. 108, 77–136. doi: 10.1016/S0065-2113(10)08002-8
Bashan, Y., Holguin, G., and de-Bashan, L. E. (2004). Azospirillum–plant relationships: physiological, molecular, agricultural, and environmental advances (1997–2003). Can. J. Microbiol. 50, 521–577. doi: 10.1139/w04-035
Bauer, H., Ache, P., Lautner, S., Fromm, J., Hartung, W., Al-Rasheid Khaled, A. S., et al. (2013). The stomatal response to reduced relative humidity requires guard cell-autonomous ABA synthesis. Curr. Biol. 1, 53–57. doi: 10.1016/j.cub.2012.11.022
Bending, G. D. (2003). “The rhizosphere and its microorganisms,” in Encyclopaedia of Applied Plant Sciences, eds B. Thomas, D. J. Murphy, and B. G. Murray (London: Academic Press), 1123–1129.
Beneduzi, A., Ambrosini, A., and Passaglia, L. M. P. (2012). Plant growth-promoting rhizobacteria (PGPR): their potential as antagonists and biocontrol agents. Genet. Mol. Biol. 35, 1044–1051. doi: 10.1590/s1415-47572012000600020
Bengtson, P., Barker, J., and Grayston, S. J. (2012). Evidence of a strong coupling between root exudation, C and N availability, and stimulated SOM decomposition caused by rhizosphere priming effects. Ecol. Evol. 2, 1843–1852. doi: 10.1002/ece3.311
Benhamou, N., Belanger, R., and Paulitz, T. (1996). Pre-inoculation of Ri T-DNA-transformed pea roots with Pseudomonas fluorescens inhibits colonization by Pythium ultimum trow: an ultrastructural and cytochemical study. Planta 199, 105–117. doi: 10.1007/bf00196887
Bethke, P. C., Badger, M. R., and Jones, R. L. (2004). Apoplastic synthesis of nitric oxide by plant tissues. Plant Cell 16, 332–341. doi: 10.1105/tpc.017822
Bloom, A. J., Meyerhoff, P. A., Taylor, A. R., and Rost, T. L. (2003). Root development and absorption of ammonium and nitrate from the rhizosphere. J. Plant Growth Regul. 21, 416–431. doi: 10.1007/s00344-003-0009-8
Bodhankar, S., Grover, M., Hemanth, S., Reddy, G., Rasul, S., Yadav, S. K., et al. (2017). Maize seed endophytic bacteria: dominance of antagonistic, lytic enzyme-producing Bacillus spp. 3 Biotech. 7:232. doi: 10.1007/s13205-017-0860-0
Bodhankar, S., Grover, M., and Reddy, G. (2019). In planta screening of maize seed endophytic bacteria for potential applications under dryland conditions. Indian J. Dryland Agri. Res. Develop. 34, 53–62. doi: 10.5958/2231-6701.2019.00009.5
Boivin, S., Fonouni-Farde, C., and Frugier, F. (2016). How auxin and cytokinin phytohormones modulate root microbe interactions. Front. Plant Sci. 7:1240. doi: 10.3389/fpls.2016.01240
Bothe, H., Körsgen, H., Lehmacher, T., and Hundeshagen, B. (1992). Differential effects of Azospirillum, auxin and combined nitrogen on the growth of the roots of wheat. Symbiosis 13, 167–179.
Bottini, R., Cassán, F., and Piccoli, P. (2004). Gibberellin production by bacteria and its involvement in plant growth promotion and yield increase. Appl. Microbiol. Biotechnol. 65, 497–503. doi: 10.1007/s00253-004-1696-1
Bremer, C., Braker, G., Matthies, D., Reuter, A., Engels, C., and Conrad, R. (2007). Impact of plant functional group, plant species, and sampling time on the composition of nirK-Type denitrifier communities in soil. Appl. Environ. Microbiol. 73, 6876–6884. doi: 10.1128/aem.01536-07
Brock. (2000). “Microbiol ecology,” in Biology of Microorganisms, eds M. T. Madigan, J. M. Martinko, and J. Parker (Upper Saddle River, NJ), 642–720.
Burdman, S., Jurkevitch, E., and Okon, Y. (2000). “Recent advances in the use of plant growth promoting rhizobacteria (PGPR) in agriculture,” in Microbial Interactions in Agriculture and Forestry, eds N. S. Subba Rao, and Y. R. Dommergues (Enfield, NH: Science Publishers), 229–250.
Cacciari, I., Lippi, D., Pietrosanti, T., and Pietrosanti, W. (1989). Phytohormone-like substances produced by single and mixed diazotrophic cultures of Azospirillum spp. and Arthrobacter. Plant Soil. 115, 151–153. doi: 10.1023/A:1004658000815
Camarena-Pozos, D. A., Flores-Núñez, V. M., López, M. G., López- Bucio, J., and Partida Martínez, L. P. (2018). Smells from the desert: Microbial volatiles that affect plant growth and development of native and non-native plant species. Plant Cell Environ. 42, 1368–1380. doi: 10.1111/pce.13476
Camerini, S., Senatore, B., Lonardo, E., Imperlini, E., Bianco, C., Moschetti, G., et al. (2008). Introduction of a novel pathway for IAA biosynthesis to rhizobia alters vetch root nodule development. Arch. Microbiol. 190, 67–77. doi: 10.1007/s00203-008-0365-7
Casanovas, E. M., Barassi, C. A., and Sueldo, R. J. (2002). Azospirillum inoculation mitigates water stress effects in maize seedlings. Cereal Res. Commun. 30, 343–350. doi: 10.1007/BF03543428
Cassán, F., Bottini, R., Schneider, G., and Piccoli, P. (2001). Azospirillum brasilense and Azospirillum lipoferum hydrolyze conjugates of GA20 and metabolize the resultant aglycones to GA1 in seedlings of rice dwarf mutants. Plant Physiol. 125, 2053–2058. doi: 10.1104/pp.125.4.2053
Cassán, F., Coniglio, A., López, G., et al. (2020). Everything you must know about Azospirillum and its impact on agriculture and beyond. Biol. Fertil. Soil 56, 461–479. doi: 10.1007/s00374-020-01463-y
Cassán, F., Perrig, D., Sgroy, V., Masciarelli, O., Penna, C., and Luna, V. (2009). Azospirillum brasilense Az39 and Bradyrhizobium japonicum E109 inoculated singly or in combination, promote seed germination and early seedling growth in corn (Zea mays L.) and soybean (Glycine max L.). Eur. J. Soil Biol. 45, 28–35. doi: 10.1016/j.ejsobi.2008.08.005
Cho, S. M., Park, J. Y., Han, S. H., Anderson, A. J., Yang, K. Y., McSpadden Gardener, B., et al. (2011). Identification and transcriptional analysis of priming genes in Arabidopsis thaliana induced by root colonization with Pseudomonas chlororaphis O6. Plant Pathol. J. 27, 272–279.
Choudhary, D. K., Prakash, A., and Johri, B. N. (2007). Induced systemic resistance (ISR) in plants: mechanism of action. Indian J. Microbiol. 47, 289–297. doi: 10.1007/s12088-007-0054-2
Clemmensen, K. E., Bahr, A., Ovaskainen, O., Dahlberg, A., Ekblad, A., Wallander, H., et al. (2013). Roots and associated fungi drive long-term carbon sequestration in boreal forest. Science 339, 1615–1618. doi: 10.1126/science.1231923
Cohen, A. C., Bottini, R., and Pic-coli, P. N. (2008). Azospirillum brasilense sp 245 produces ABA in chemically-defined culture medium and increases ABA content in Arabidopsis plants. Plant Growth Regul. 54, 97–103. doi: 10.1007/s10725-007-9232-9
Colmer, T. D. (2003). Long-distance transport of gases in plants: a perspective on internal aeration and radial oxygen loss from roots. Plant Cell Environ. 26, 17–36. doi: 10.1046/j.1365-3040.2003.00846.x
Combes-Meynet, E., Pothier, J. F., Moënne-Loccoz, Y., and Prigent-Combaret, C. (2011). The Pseudomonas secondary metabolite 2,4-diacetylphloroglucinol is a signal inducing rhizoplane expression of Azospirillum genes involved in plant-growth promotion. Mol. Plant Microbe. Interact. 24, 271–284. doi: 10.1094/mpmi-07-10-0148
Conforte, V. P., Echeverria, M., Sánchez, C., Ugalde, R. A., Menéndez, A. B., and Lepek, V. C. (2010). Engineered ACC deaminase-expressing free-living cells of Mesorhizobium loti show increased nodulation efficiency and competitiveness on Lotus spp. J. Gen. Appl. Microbiol. 56, 331–338. doi: 10.2323/jgam.56.331
Contesto, C., Milesi, S., Mantelin, S., Zancarini, A., Desbrosses, G., Varoquaux, F., et al. (2010). The auxin-signaling pathway is required for the lateral root response of Arabidopsis to the rhizobacterium Phyllobacterium brassicacearum. Planta 232, 1455–1470. doi: 10.1007/s00425-010-1264-0
Cooper, J. B., and Long, S. R. (1994). Morphogenetic rescue of Rhizobium meliloti nodulation mutants by trans-zeatin secretion. Plant Cell 6, 215–225. doi: 10.1105/tpc.6.2.215
Cordovez, V., Schop, S., Hordijk, K., Dupré de Boulois, H., Coppens, F., Hanssen, I., et al. (2018). Priming of plant growth promotion by volatiles of root-associated Microbacterium spp. Appl. Environ. Microbiol. 84:e01865-18. doi: 10.1128/aem.01865-18
Correa-Aragunde, N., Graziano, M., and Lamattina, L. (2004). Nitric oxide plays a central role in determining lateral root development in tomato. Planta 218, 900–905. doi: 10.1007/s00425-003-1172-7
Creus, C. M., Graziano, M., Casanovas, E. M., Pereyra, M. A., Simontacchi, M., Puntarulo, S., et al. (2005). Nitric Oxide is involved in the Azospirillum brasilense-induced lateral root formation in tomato. Planta 221, 297–303. doi: 10.1007/s00425-005-1523-7
Cristea, O. D., Vlădu,t, V., Ungureanu, N., Popa, D. L., Isticioaia, S., Dumitrescu, L., et al. (2020). Research on methods, techniques and technologies of carbon sequestration in soil. E3S Web Conf. 180:03021. doi: 10.1051/e3sconf/202018003021
Czarnes, S., Hallett, P. D., Bengough, A. G., and Young, A. M. (2000). Root- and microbial-derived mucilages affect soil structure and water transport. Eur. J. Soil Sci. 51, 435–443. doi: 10.1046/j.1365-2389.2000.00327.x
Dahmani, M. A., Desrut, A., Moumen, B., Verdon, J., Mermouri, L., Kacem, M., et al. (2020). Unearthing the plant growth-promoting traits of Bacillus megaterium RmBm31, an endophytic bacterium isolated from root nodules of retama monosperma. Front. Plant Sci. 11:124. doi: 10.3389/fpls.2020.00124
Danish, S., Zafar-Ul-Hye, M., Mohsin, F., and Hussain, M. (2020). ACC-deaminase producing plant growth promoting rhizobacteria and biochar mitigate adverse effects of drought stress on maize growth. PLoS ONE. 15:e0230615. doi: 10.1371/journal.pone.0230615
De Smet, I. (2012). Lateral root initiation: one step at a time. New Phytol. 193, 867–873. doi: 10.1111/j.1469-8137.2011.03996.x
De Smet, I., Zhang, H., Inz,é, D., and Beeckman, T. (2006). A novel role for abscisic acid emerges from nderground. Trends Plant Sci. 11, 434–439. doi: 10.1016/j.tplants.2006.07.003
de-Bashan, L. E., Antoun, H., and Bashan, Y. (2008). Involvement of indole-3-acetic-acid produced by the growth-promoting bacterium Azospirillum spp. in promoting growth of Chlorella vulgaris. J. Phycol. 44, 938–947. doi: 10.1111/j.1529-8817.2008.00533.x
de-García Salamone, I. E., Hynes, R. K., and Nelson, L. M. (2001). Cytokinin production by plant growth promoting rhizobacteria and selected mutants. Can. J. Microbiol. 47, 404–411. doi: 10.1139/w01-029
Deka, P., Goswami, G., Das, P., Gautom, T., Chowdhury, N., Boro, R. C., et al. (2018). Bacterial exopolysaccharide promotes acid tolerance in Bacillus amyloliquefaciens and improves soil aggregation. Molecular Biol. Rep. 46, 1079–1091. doi: 10.1007/s11033-018-4566-0
Desbrosses, G. J., and Stougaard, J. (2011). Root nodulation: a paradigm for how plant-microbe symbiosis influences host developmental pathways. Cell Host Microbe 10, 348–358. doi: 10.1016/j.chom.2011.09.005
Desta, Y., Habtegebrial, K., and Weldu, Y. (2015). Inoculation, phosphorous and zinc fertilization effects on nodulation, yield and nutrient uptake of Faba bean (Vicia faba L.) grown on calcaric cambisol of semiarid Ethiopia. J. Soil Sci.Environ. Manage. 6, 9–15. doi: 10.5897/JSSEM2013.0406
Didonet, A. D., and Magalhaes, A. C. (1993). The role of auxin like compounds in plant growth promoting rhizobacteria: the wheat-Azospirillum association. Revista Brasileira de Fisiologia Vegetal, Londrina. 5, 179–183.
Dijkstra, F. A., Carrillo, Y., Pendall, E., and Morgan, J. A. (2013). Rhizosphere priming: a nutrient perspective. Front. Microbiol. 4:216. doi: 10.3389/fmicb.2013.00216
Dixit, R., Agrawal, L., Gupta, S., Kumar, M., Yadav, S., Chauhan, P. S., et al. (2016). Southern blight disease of tomato control by 1-aminocyclopropane-1-carboxylate (ACC) deaminase producing Paenibacillus lentimorbus B-30488. Plant Signal.Behav. 11:e1113363. doi: 10.1080/15592324.2015.111336
Dobbelaere, S., Croonenborghs, A., Thys, A., Broek, A. V., and Vanderleyden, J. (1999). Phytostimulatory effect of A. brasilense wild type and mutant strains altered in IAA production on wheat. Plant Soil. 212, 155–164. doi: 10.1023/a:1004658000815
Dodd, I. C., Zinovkina, N. Y., Safronova, V. I., and Belimov, A. A. (2010). Rhizobacterial mediation of plant hormone status. Ann. Appl. Biol. 157, 361–379. doi: 10.1111/j.1744-7348.2010.00439.x
Dong, L., Mao, Z., and Sun, T. (2016). Condensed tannin effects on decomposition of very fine roots among temperate tree species. Soil Biol. Biochem. 103, 489–492. doi: 10.1016/j.soilbio.2016.10.003
Drake, J. E., Darby, B. A., Giasson, M. A., Kramer, M. A., Phillips, R. P., and Finnzi, A. C. (2013). Stoichiometry constrains microbial response to root exudation-insights from a model and a field experiment in a temperate forest. Biogeosciences 10, 821–838. doi: 10.5194/bg-10-821-2013
Drogue, B., Combes-Meynet, E., Moënne-Loccoz, Y., Wisniewski-Dyé, F., and Prigent-Combaret, C. (2013). “Control of the cooperation between plant growth-Promoting rhizobacteria and crops by rhizosphere signals,” in Molecular Microbial Ecology of the Rhizosphere, ed F. J. de Bruijn (John wiley and Sons), 279–293. Available online at: https://onlinelibrary.wiley.com/doi/abs/10.1002/9781118297674.ch27
Egamberdieva, D., Wirth, S., Jabborova, D., Räsänen, L. A., and Liao, H. (2017). Coordination between Bradyrhizobium and Pseudomonas alleviates salt stress in soybean through altering root system architecture. J. Plant Interact. 12, 100–107. doi: 10.1080/17429145.2017.1294212
Ekin, Z. (2020). Co-application of humic acid and Bacillus strains enhances seed and oil yields by mediating nutrient acquisition of safflower (Carthamus tinctorius L.) plants in a semi-arid region. Appl. Ecol. Environ. Res. 18, 1883–1900. doi: 10.15666/aeer/1801_18831900
El-Khawas, H., and Adachi, K. (1999). Identification and quantification of auxins in culture media of Azospirillum and Klebsiella and their effect on rice roots. Biol. Fertil. Soils 28, 377–381. doi: 10.1007/s003740050507
El-Zemrany, H., Cortet, J., Lutz, M. P., Chabert, A., Baudoin, E., Haurat, J., et al. (2006). Field survival of the phytostimulator Azospirillum lipoferum CRT1 and functional impact on maize crop, biodegradation of crop residues, and soil faunal indicators in a context of decreasing nitrogen fertilisation. Soil Biol. Biochem. 38, 1712–1726. doi: 10.1016/j.soilbio.2005.11.025
El-Zemrany, H., Czarnes, S., Hallett, P. D., Alamercery, S., Bally, R., and Jocteur Monrozier, L. (2007). Early changes in root characteristics of maize (Zea mays) following seed inoculation with the PGPR Azospirillum lipoferum CRT1. Plant Soil 291, 109–118. doi: 10.1007/s11104-006-9178-0
Emami, S., Alikhani, H. A., Pourbabaei, A. A., Etesami, H., Motashare-Zadeh, B., and Sarmadian, F. (2018). Improved growth and nutrient acquisition of wheat genotypes in phosphorus deficient soils by plant growth-promoting rhizospheric and endophytic bacteria. Soil Sci. Plant Nutr. 64, 719–727. doi: 10.1080/00380768.2018.1510284
Ennos, A. R., and Fitter, A. H. (1992). Comparative functional morphology of the anchorage systems of annual dicots. Funct. Ecol. 6, 71–78. doi: 10.2307/2389773
Erturk, Y., Ercisli, S., Haznedar, A., and Cakmakci, R. (2010). Effects of plant growth promoting rhizobacteria (PGPR) on rooting and root growth of kiwifruit (Actinidia deliciosa) stem cuttings. Biol. Res. 43, 91–98. doi: 10.4067/s0716-97602010000100011
Etesami, H., and Alikhani, H. A. (2016). Co-inoculation with endophytic and rhizosphere bacteria allows reduced application rates of N-fertilizer for rice plant. Rhizosphere 2, 5–12. doi: 10.1016/j.rhisph.2016.09.003
Fallik, E., Okon, Y., Epstein, E., Goldman, A., and Fischer, M. (1989). Identification and quantification of IAA and IBA in Azospirillum brasilense-inoculated maize roots. Soil Biol. Biochem. 21, 147–153. doi: 10.1016/0038-0717(89)90024-2
Farag, M. A., Ryu, C. M., Sumner, L. W., and Par,é, P. W. (2006). GC–MS SPME profiling of rhizobacterial volatiles reveals prospective inducers of growth promotion and induced systemic resistance in plants. Phytochemistry 67, 2262–2268. doi: 10.1016/j.phytochem.2006.07.021
Ferreira Rêgo, M. C., Ilkiu-Borges, F., Filippi, M. C. C., de, Gonçalves, L. A., and Silva, G. B. (2014). Morphoanatomical and biochemical changes in the roots of rice plants induced by plant growth-promoting microorganisms. J. Bot. 2014:818797. doi: 10.1155/2014/818797
Fierro-Coronado, R. A., Quiroz-Figueroa, F. R., García-Pérez, L. M., Ramírez-Chávez, E., Molina-Torres, J., and Maldonado-Mendoza, I. E. (2014). IAA-producing rhizobacteria from chickpea (Cicer arietinum L.) induce changes in root architecture and increase root biomass. Can. J. Microbiol. 60, 639–648. doi: 10.1139/cjm-2014-0399
Franzini, V. I., Azcón, R., Mendes, F. L., and Aroca, R. (2010). Interactions between Glomus species and Rhizobium strains affect the nutritional physiology of drought-stressed legume hosts. J. Plant Physiol. 167, 614–619. doi: 10.1016/j.jplph.2009.11.010
Frugier, F., Kosuta, S., Murray, J. D., Crespi, M., and Szczyglowski, K. (2008). Cytokinin: secret agent of symbiosis. Trends Plant Sci. 13, 115–120. doi: 10.1016/j.tplants.2008.01.003
Fulchieri, M., Lucangeli, C., and Bottini, R. (1993). Inoculation with Azospirillum lipoferum affects growth and gibberellin status of corn seedling roots. Plant Cell Physiol. 34, 1305–1309. doi: 10.1093/oxfordjournals.pcp.a078554
Gagné-Bourque, F., Bertrand, A., Claessens, A., Aliferis, K. A., and Jabaji, S. (2016). Alleviation of drought stress and metabolic changes in timothy (Phleum pratense L.) Colonized with Bacillus subtilis B26. Front. Plant Sci. 7:584. doi: 10.3389/fpls.2016.00584
Glick, B. R. (2011). The enhancement of plant growth by free-living bacteria. Can. J. Microbiol. 41, 109–117. doi: 10.1139/m95-015
Glick, B. R. (2014). Bacteria with ACC deaminase can promote plant growth and help to feed the world. Microbiol. Res. 169, 30–39. doi: 10.1016/j.micres.2013.09.009
Glick, B. R., Cheng, Z., Czarny, J., and Duan, J. (2007). Promotion of plant growth by ACC deaminase-producing soil bacteria. Eur. J. Plant Pathol. 119, 329–339. doi: 10.1007/s10658-007-9162-4
Glick, B. R., Penrose, D. M., and Li, J. (1998). A model for the lowering of plant ethylene concentrations by plant growth promoting bacteria. J. Theor. Biol. 190, 63–68. doi: 10.1006/jtbi.1997.0532
Gong, L., and Zhao, J. (2019). The response of fine root morphological and physiological traits to added nitrogen in Schrenk's spruce (Picea schrenkiana) of the Tianshan mountains, China. Peer J. 7:e8194. doi: 10.7717/peerj.8194
Gonzalez-Rizzo, S., Crespi, M., and Frugier, F. (2006). The Medicago truncatula CRE1 cytokinin receptor regulates lateral root development and early symbiotic interaction with Sinorhizobium meliloti. Plant Cell. 18, 2680–2693. doi: 10.1105/Ftpc.106.043778
Gopalakrishnan, S., Sathya, A., Vijayabharathi, R., Varshney, R. K., Gowda, C. L., and Krishnamurthy, L. (2015). Plant growth promoting rhizobia: challenges and opportunities. 3 Biotech. 5, 355–377. doi: 10.1007/s13205-014-0241-x
Goswami, D., Thakker, J. N., and Dhandhukia, P. C. (2016). Portraying mechanics of plant growth promoting rhizobacteria (PGPR): a review. Cogent. Food Agri. 2:1127500. doi: 10.1080/23311932.2015.1127500
Gouvêa, C. M. C. P., Souza, J. F., Magalhaês, C. A. N., and Martins, I. S. (1997). NO-releasing substances that induce growth elongation in maize root segments. J. Plant Growth Regul. 21, 183–187. doi: 10.1023/A:1005837012203
Gouzou, L., Burtin, G., Philippy, R., Bartoli, F., and Heulin, T. (1993). Effect of inoculation with Bacillus polymyxa on soil aggregation in the wheat rhizosphere: preliminary examination. Geoderma 56, 479–491. doi: 10.1016/0016-7061(93)90128-8
Gowtham, H. G., Murali, M., Singh, S. B., Lakshmeesha, T. R., Narasimha Murthy, K., Amruthesh, K. N., et al. (2018). Plant growth promoting rhizobacteria- Bacillus amyloliquefaciens improves plant growth and induces resistance in chilli against anthracnose disease. Biol. Cont. 126, 209–217. doi: 10.1016/j.biocontrol.2018.05.022
Grover, M., Ali, S.k.Z, Sandhya, V., Rasul, A., and Venkateswarlu, B. (2011). Role of microorganisms in adaptation of agriculture crops to abiotic stresses. World J. Microbiol. Biotechnol. 27, 1231–1240. doi: 10.1007/s11274-010-0572-7
Gupta, G., Parihar, S. S., Ahirwar, N. K., Snehi, S. K., and Singh, V. (2015). Plant growth promoting rhizobacteria (PGPR): current and future prospects for development of sustainable agriculture. J. Microb. Biochem. Technol. 7, 096–102. doi: 10.4172/1948-5948.1000188
Gutiérrez-Luna, F. M., López-Bucio, J., Altamirano-Hernández, J., Valencia-Cantero, E., de la Cruz, H. R., and Macías-Rodríguez, L. (2010). Plant growth-promoting rhizobacteria modulate root-system architecture in Arabidopsis thaliana through volatile organic compound emission. Symbiosis 51, 75–83. doi: 10.1007/s13199-010-0066-2
Gutiérrez-Mañero, F. J., Ramos-Solano, B., Probanza, A., Mehouachi, J., Tadeo, F. R., and Talon, M. (2001). The plant-growth promoting rhizobacteria Bacillus pumilus and Bacillus licheniformis produce high amounts of physiologically active gibberellins. Physiol. Plant 111, 206–211. doi: 10.1034/j.1399-3054.2001.1110211.x
Gutschick, V. P. (1993). Nutrient-limited growth rates: roles of nutrient-use efficiency and of adaptations to increase uptake rate. J. Exp. Bot. 44, 41–51. doi: 10.1093/jxb/44.1.41
Gyssels, G., Poesen, J., Bochet, E., and Li, Y. (2005). Impact of plant roots on the resistance of soils to erosion by water: a review. Prog. Phys. Geog. Earth Environ. 29, 189–217. doi: 10.1191/0309133305pp443ra
Haas, D., and Defago, G. (2005). Biological control of soil-borne pathogens by fluorescent Pseudomonads. Nat. Rev. Microbiol. 3, 307–319. doi: 10.1038/nrmicro1129
Habib, S. H., Kausar, H., and Saud, H. M. (2016). Plant growth-promoting Rhizobacteria enhance salinity stress tolerance in Okra through ROS-scavenging enzymes. BioMed Res. Int. 2016:6284547. doi: 10.1155/2016/6284547
Hadas, R., and Okon, Y. (1987). Effect of Azospirillum brasilense inoculation on root morphology and respiration in tomato seedlings. Biol. Fertil. Soils. 5, 241–247. doi: 10.1007/BF00256908
Han, H. S., and Lee, K. D. (2005). Physiological responses of soybean - inoculation of Bradyrhizobium japonicum with PGPR in saline soil conditions. Res. J. Agric. Biol. Sci. 1, 216–221.
Harari, A., Kigel, J., and Okon, Y. (1988). Involvement of IAA in the interaction between Azospirillum brasilense and Panicum miliaceum roots. Plant Soil. 110, 275–282.
Hartman, K., and Tringe, S. G. (2019). Interactions between plants and soil shaping the root microbiome under abiotic stress. Biochem. J. 476, 2705–2724. doi: 10.1042/BCJ20180615
Hashem, A., Tabassum, B., and FathiAbd-Allah, E. (2019). Bacillus subtilis: a plant-growth promoting rhizobacterium that also impacts biotic stress. Saudi J. Biol. Sci. 26, 1291–1297. doi: 10.1016/j.sjbs.2019.05.004
Hassan, T. U., and Bano, A. (2019). Construction of IAA-deficient mutants of Pseudomonas moraviensis and their comparative effects with wild type strains as bio-inoculant on wheat in saline sodic soil. Geomicrobiol. J. 36, 376–384. doi: 10.1080/01490451.2018.1562498
He, M., He, C. Q., and Ding, N. Z. (2018). Abiotic stresses: general defenses of land plants and chances for engineering multistress tolerance. Front. Plant Sci. 9:1771. doi: 10.3389/fpls.2018.01771
Held, M., Hou, H., Miri, M., Huynh, C., Ross, L., Hossain, M. S., et al. (2014). Lotus japonicus cytokinin receptors work partially redundantly to mediate nodule formation. Plant Cell. 26, 678–694. doi: 10.1105/Ftpc.113.119362
Herridge, D. F., Peoples, M. B., and Boddey, R. M. (2008). Global inputs of biological nitrogen fixation in agricultural systems. Plant Soil. 311, 1–18. doi: 10.1007/s11104-008-9668-3
Hiltner, L. (1904). Uber neuere erfahrungen und probleme auf dem gebiete der bodenbakteriologie unter besonderden berucksichtigung und Brache. Arb. Dtsch. Landwirtsch. Gesellschaft. 98, 59–78.
Hong, Y., Glick, B. R., and Pasternak, J. J. (1991). Plant-microbial interaction under gnotobotic conditions: a scanning electron microscope study. Curr. Microbiol. 23, 111–114. doi: 10.1007/bf02092259
Horchani, F., Prevot, M., Boscari, A., Evangelisti, E., Meilhoc, E., Bruand, C., et al. (2011). Both plant and bacterial nitrate reductases contribute to nitric oxide production in Medicago truncatula nitrogen-fixing nodules. Plant Physiol. 155, 1023–1036. doi: 10.1104/pp.110.166140
Hussain, A., and Hasnain, S. (2009). Cytokinin production by some bacteria: its impact on cell division in cucumber cotyledons. Afr. J. Microbiol. Res. 3, 704–712.
Jain, D. K., and Patriquin, D. G. (1984). Root hair deformation, bacterial attachment, and plant growth in wheat–Azospirillum associations. Appl. Environ. Microbiol. 48, 1208–1213.
Jain, D. K., and Patriquin, D. G. (1985). Characterization of a substance produced by Azospirillum which causes branching of wheat root hairs. Can. J. Microbiol. 31, 206–210.
Jochum, M. D., McWilliams, K. L., Borrego, E. J., Kolomiets, M. V., Niu, G., Pierson, E. A., et al. (2019). Bioprospecting plant growth-promoting rhizobacteria that mitigate drought stress in grasses. Front. Microbiol. 10:2106. doi: 10.3389/fmicb.2019.02106
Jung, J. K. H., and McCouch, S. (2013). Getting to the roots of it: genetic and hormonal control of root architecture. Front. Plant Sci. 4:186. doi: 10.3389/fpls.2013.00186
Kai, M., and Piechulla, B. (2009). Plant growth promotion due to rhizobacterial volatiles - an effect of CO2? FEBS Lett. 583, 3473–3477. doi: 10.1016/j.febslet.2009.09.053
Kapulnik, Y., Okon, Y., and Henis, Y. (1985). Changes in root morphology of wheat caused by Azospirillum inoculation. Can. J. Microbiol. 31, 881–887. doi: 10.1139/m85-165
Karaca, U., and Uyanoz, R. (2012). Effectiveness of native Rhizobium on nodulation and growth properties of dry bean (Phaseolus vulgaris L.). Afr. J. Biotechnol. 11, 37, 8986–8991. doi: 10.5897/AJB11.4087
Kaushal, M., and Wani, S. P. (2016). Plant growth promoting rhizobacteria: drought stress alleviators to ameliorate crop production in drylands. Ann. Microbiol. 66, 35–42. doi: 10.1007/s13213-015-1112-3
Kechid, M., Desbrosses, G., Rokhsi, W., Varoquaux, F., Djekoun, A., and Touraine, B. (2013). The NRT2.5 and NRT2.6 genes are involved in growth promotion of Arabidopsis by the plant growth-promoting rhizobacterium (PGPR) strain Phyllobacterium brassicacearum STM196. New Phytologist. 198, 514–524. doi: 10.1111/nph.12158
Khaitov, B., Kurbonov, A., Abdiev, A., and Adilov, M. (2016). Effect of chickpea in association with Rhizobium to crop productivity and soil fertility. Euras. J. Soil Sci. 5, 105–112. doi: 10.18393/ejss.2016.2.105-112
Khan, N., Bano, A., and Babar, M. A. (2016). The root growth of wheat plants, the water conservation and fertility status of sandy soils influenced by plant growth promoting rhizobacteria. Symbiosis 72, 195–205. doi: 10.1007/s13199-016-0457-0
Khanna, K., Jamwal, V. L., Sharma, A., Gandhi, S. G., Ohri, P., Bhardwaj, R., et al. (2019). Evaluation of the role of Rhizobacteria in controlling root knot nematode (RKN) infection in Lycopersicon esculentum plants by modulation in the secondary metabolite profiles. AoB Plants. 11:plz069. doi: 10.1093/aobpla/plz069
Kieber, J. J., and Schaller, G. E. (2018). Cytokinin signaling in plant development. Development 145:dev149344. doi: 10.1242/dev.149344
Kohler, J., Caravaca, F., Carrasco, L., and Roldán, A. (2006). Contribution of Pseudomonas mendocina and Glomus intraradices to aggregate stabilization and promotion of biological fertility in rhizosphere soil of lettuce plants under field conditions. Soil Use Manage. 22, 298–304. doi: 10.1111/j.1475-2743.2006.00041.x
Kohler, J., Hernández, J. A., Caravaca, F., and Roldán, A. (2009). Induction of antioxidant enzymes is involved in the greater effectiveness of a PGPR versus AM fungi with respect to increasing the tolerance of lettuce to severe salt stress. Environ. Exp. Bot. 65, 245–252. doi: 10.1016/j.envexpbot.2008.09.008
Korir, H., Mungai, N. W., Thuita, M., Hamba, Y., and Masso, C. (2017). Co-inoculation effect of Rhizobia and plant growth promoting Rhizobacteria on common bean growth in a low phosphorus soil. Front. Plant Sci. 8:141. doi: 10.3389/fpls.2017.00141
Kotula, L., Ranathunge, K., Schreiber, L., and Steudle, E. (2009b). Functional and chemical comparison of apoplastic barriers to radial oxygen loss in roots of rice (Oryza sativa L.) grown in aerated or deoxygenated solution. J. Exp. Bot. 60, 2155–2167. doi: 10.1093/jxb/erp089
Kotula, L., Ranathunge, K., and Steudle, E. (2009a). Apoplastic barriers effectively block oxygen permeability across outer cell layers of rice roots under deoxygenated conditions: roles of apoplastic pores and of respiration. New Phytologist. 184, 909–917. doi: 10.1111/j.1469-8137.2009.03021.x
Kour, D., Rana, K. L., Sheikh, I., Kumar, V., Yadav, A. N., Dhaliwal, H. S., et al. (2019). Alleviation of drought stress and plant growth promotion by Pseudomonas libanensis EU-LWNA-33, a drought-adaptive phosphorus-solubilizing bacterium. Proc. Natl. Acad. Sci. India B Biol. Sci. 90, 785–795. doi: 10.1007/s40011-019-01151-4
Krapp, A., Berthomé, R., Orsel, M., Mercey-Boutet, S., Yu, A., Castaings, L., et al. (2011). Arabidopsis roots and shoots show distinct temporal adaptation patterns toward nitrogen starvation. Plant Physiol. 157, 1255–1282. doi: 10.1104/pp.111.179838
Kudoyarova, G., Arkhipova, T., Korshunova, T., Bakaeva, M., Loginov, O., and Dodd Ian, C. (2019). Phytohormone mediation of interactions between plants and non- symbiotic growth promoting bacteria under edaphic stresses. Front. Plant Sci. 10:1368. doi: 10.3389/fpls.2019.01368
Kudoyarova, G. R., Melentiev, A. I., Martynenko, E. V., Timergalina, L. N., Arkhipova, T. N., Shendel, G. V., et al. (2014). Cytokinin producing bacteria stimulate amino acid deposition by wheat roots. Plant Physiol. Biochem. 83, 285–291. doi: 10.1016/j.plaphy.2014.08.015
Kudoyarova, G. R., Vysotskaya, L. B., Arkhipova, T. N., Kuzmina, L. Y., Galimsyanova, N. F., Sidorova, L. V., et al. (2017). Effect of auxin producing and phosphate solubilizing bacteria on mobility of soil phosphorus, growth rate, and P acquisition by wheat plants. Acta Physiologiae Plantarum. 39:253. doi: 10.1007/s11738-017-2556-9
Kumar, A., Maurya, B. R., and Raghuwanshi, R. (2014). Isolation and characterization of PGPR and their effect on growth, yield and nutrient content in wheat (Triticum aestivum L.). Biocatal. Agri. Biotechnol. 3, 121–128. doi: 10.1016/j.bcab.2014.08.003
Kumar, S., Chauhan, P. S., Agrawal, L., Raj, R., Srivastava, A., Gupta, S., et al. (2016). Paenibacillus lentimorbus inoculation enhances tobacco growth and extenuates the virulence of cucumber mosaic virus. PLoS ONE 11:e0149980. doi: 10.1371/journal.pone.0149980
Kumawat, K., Sharma, P., Sirari, A., Singh, I., Gill, B., Singh, U., et al. (2019). Synergism of Pseudomonas aeruginosa (LSE-2) nodule endophyte with Bradyrhizobium sp.(LSBR-3) for improving plant growth, nutrient acquisition and soil health in soybean. World J. Microbiol. Biotechnol. 35:47. doi: 10.1007/s11274-019-2622-0
Kundu, B. S., Sangwan, P., Sharma, P. K., and Nandwal, A. S. (1997). Response of pearlmillet to phytohormones produced by Azospirillum brasilense. Indian J. Plant Physiol. 2, 101–104.
Lamattina, L., García-Mata, C., Graziano, M., and Pagnussat, G. (2003). Nitric oxide: the versatility of an extensive signal molecule. Annu. Rev. Plant Biol. 54, 109–136. doi: 10.1146/annurev.arplant.54.031902.134752
Lappartient, A. G., Vidmar, J. J., Leustek, T., Glass, A. D., and Touraine, B. (1999). Inter-organ signalling in plants: regulation of ATP sulfurylase and sulphate transporter genes expression in roots mediated by phloem- translocated compound. Plant J. 18, 89–95. doi: 10.1046/j.1365-313X.1999.00416.x
Lee, B., Lee, S., and Ryu, M. R. (2012). Foliar aphid feeding recruits rhizosphere bacteria and primes plant immunity against pathogenic and non-pathogenic bacteria in pepper. Ann Bot. 110, 281–290. doi: 10.1093/Faob/Fmcs055
Leifheit, E. F., Veresoglou, S. D., Lehmann, A., Morris, E. K., and Rillig, M. C. (2013). Multiple factors influence the role of arbuscular mycorrhizal fungi in soil aggregation—a meta-analysis. Plant Soil. 374, 523–537. doi: 10.1007/s11104-013-1899-2
Li, B., Li, Q., Xu, Z., Zhang, N., Shen, Q., and Zhang, R. (2014). Responses of beneficial Bacillus amyloliquefaciens SQR9 to different soil borne fungal pathogens through the alteration of antifungal compounds production. Front. Microbiol. 5:636. doi: 10.3389/fmicb.2014.00636
Li, H., Ding, X., Wang, C., Ke, H., Wu, Z., Wang, Y., et al. (2016). Control of tomato yellow leaf curl virus disease by Enterobacterasburiae BQ9 as a result of priming plant resistance in tomatoes. Turk. J. Biol. 40, 150–159. doi: 10.3906/biy-1502-12
Li, J., Ovakim, D. H., Charles, T. C., and Glick, B. R. (2000). An ACC Deaminase minus mutant of Enterbacter cloacae UW4 no longer promotes root elongation. Curr. Microbiol. 41, 101–105. doi: 10.1007/s00284001010c
Li, X., Sun, P., Zhang, Y., Jin, C., and Guan, C. (2020). A novel PGPR strain Kocuria rhizophila Y1 enhances salt stress tolerance in maize by regulating phytohormone levels, nutrient acquisition, redox potential, ion homeostasis, photosynthetic capacity and stress-responsive genes expression. Environ. Exp. Botany 174:104023. doi: 10.1016/j.envexpbot.2020.104023
Li, X., Zeng, R., and Liao, H. (2016). Improving crop nutrient efficiency through root architecture modifications. J. Integr. Plant Biol. 58, 193–202. doi: 10.1111/jipb.12434
Little, D. Y., Rao, H., Oliva, S., Daniel-Vedele, F., Krapp, A., and Malamy, J. E. (2005). The putative high-affinity nitrate transporter NRT2.1 represses lateral root initiation in response to nutritional cues. Proc. Natl. Acad. Sci. U.S.A. 102, 13693–13698. doi: 10.1073/pnas.0504219102
Liu, J., Moore, S., Chen, C., and Lindsey, K. (2017). Crosstalk complexities between auxin, cytokinin, and ethylene in Arabidopsis root development: from experiments to systems modeling, and back again. Mol. Plant 10, 1480–1496. doi: 10.1016/j.molp.2017.11.002
Llorente, B. E., Alasia, M. A., and Larraburu, E. E. (2016). Biofertilization with Azospirillum brasilense improves in vitro culture of Handroanthus ochraceus, a forestry, ornamental and medicinal plant. N. Biotechnol. 33, 32–40. doi: 10.1016/j.nbt.2015.07.006
Loades, K. W., Bengough, A. G., Bransby, M. F., and Hallett, P. D. (2010). Planting density influence on fibrous root reinforcement of soils. Ecol. Eng. 36, 276–284. doi: 10.1016/j.ecoleng.2009.02.005
Loganathan, M., Garg, R., Venkataravanappa, V., Saha, S., and Rai, A. B. (2014). Plant growth promoting rhizobacteria (PGPR) induces resistance against Fusarium wilt and improves lycopene content and texture in tomato. Afr. J. Microbiol. Res. 8, 1105–1111. doi: 10.5897/AJMR2013.5653
López-Bucio, J., Campos-Cuevas, J. C., Hernández-Calderón, E., Velásquez-Becerra, C., Farías-Rodríguez, R., Macías-Rodríguez, L. I., et al. (2007). Bacillus megaterium rhizobacteria promote growth and alter root-system architecture through an auxin- and ethylene-independent signaling mechanism in Arabidopsis thaliana. Mol Plant-Microbe. Inter. 20, 207–217. doi: 10.1094/mpmi-20-2-0207
Lynch, J. M. (1987). Microbial interactions in the rhizosphere. Japanese Soc. Soil Microbiol. 30, 33–41.
Lynch, J. P. (2007). Roots of the second green revolution. Aust. J. Bot. 55, 493–512. doi: 10.1071/BT06118
Ma, Z., Walk, T. C., Marcus, A., and Lynch, J. P. (2001). Morphological synergism in root hair length, density, initiation and geometry for phosphorus acquisition in Arabidopsis thaliana: a modeling approach. Plant Soil. 236, 221–235. doi: 10.1023/a:1012728819326
Maddhesiya, P. K., Singh, K., and Singh, R. P. (2020). Effects of perennial aromatic grass species richness and microbial consortium on soil properties of marginal lands and on biomass production. Land. Degrad. Dev. doi: 10.1002/ldr.3742
Makita, N., Hirano, Y., Mizoguchi, T., Kominami, Y., Dannoura, M., Ishii, H., et al. (2011). Very fine roots respond to soil depth: biomass allocation, morphology, and physiology in a broad-leaved temperate forest. Ecol. Res. 26, 95–104. doi: 10.1007/s11284-010-0764-5
Malamy, J. E. (2005). Intrinsic and environmental response pathways that regulate root system architecture. Plant, Cell Environ. 28, 67–77. doi: 10.1111/j.1365-3040.2005.01306.x
Malamy, J. E., and Ryan, K. S. (2001). Environmental regulation of lateral root initiation in Arabidopsis. Plant Physiol. 127, 899–909. doi: 10.1104/pp.010406
Malhotra, M., and Srivastava, S. (2006). Targeted engineering of Azospirillum brasilense SM with indole acetamide pathway for indoleacetic acid over-expression. Can. J. Microbiol. 52, 1078–1084. doi: 10.1139/w06-071
Malhotra, M., and Srivastava, S. (2009). Stress-responsive indole-3-acetic acid biosynthesis by Azospirillum brasilense SM and its ability to modulate plant growth. Eur. J. Soil Biol. 45, 73–80. doi: 10.1016/j.ejsobi.2008.05.006
Mantelin, S., Desbrosses, G., Larcher, M., Tranbarger, T. J., Cleyet-Marel, J. C., and Touraine, B. (2006). Nitrate-dependent control of root architecture and N nutrition are altered by a plant growth- promoting Phyllobacterium sp. Planta 223, 591–603. doi: 10.1007/s00425-005-0106-y
Mantelin, S., and Touraine, B. (2004). Plant growth-promoting bacteria and nitrate availability :impacts on root development and nitrate uptake. J. Exp. Bot. 55, 27–34. doi: 10.1093/jxb/erh010
Marasco, R., Rolli, E., Vigani, G., Borin, S., Sorlini, C., Ouzari, H., et al. (2013). Are drought-resistance promoting bacteria cross-compatible with different plant models? Plant Sig. Behav. 8:10. doi: 10.4161/psb.26741
Marimuthu, S., Ramamoorthy, V., Samiyappan, R., and Subbian, P. (2013). Intercropping system with combined application of Azospirillum and Pseudomonas fluorescens reduces root rot incidence caused by Rhizoctonia bataticola and increases seed cotton yield. J. Phytopathol. 161, 405–411. doi: 10.1111/jph.12084
Marulanda, A., Barea, J. M., and Azcón, R. (2009). Stimulation of plant growth and drought tolerance by native microorganisms (AM fungi and bacteria) from dry environments: mechanisms related to bacterial effectiveness. J. Plant Growth Regul. 28, 115–124. doi: 10.1007/s00344-009-9079-6
Mathesius, U. (2008). Auxin: at the root of nodule development? Funct. Plant Biol. 35, 651. doi: 10.1071/fp08177
Mavrodi, O. V., Mavrodi, D. V., Parejko, J. A., Thomashow, L. S., and Weller, D. M. (2012). Irrigation differentially impacts populations of indigenous antibiotic-producing Pseudomonas spp. in the rhizosphere of wheat. Appl. Environ. Microbiol. 78, 3214–3220. doi: 10.1128/AEM.07968-11
Meakin, G. E., Bueno, E., Jepson, B., Bedmar, E. J., Richardson, D. J., and Delgado, M. J. (2007). The contribution of bacteroidal nitrate and nitrite reduction to the formation of nitrosylleghaemoglobin complexes in soybean root nodules. Microbiology. 153, 411–419 doi: 10.1099/mic.0.2006/000059-0
Meilhoc, E., Boscari, A., Bruand, C., Puppo, A., and Brouquisse, R. (2011). Nitric oxide in legume–Rhizobium symbiosis. Plant Sci. 181, 573–581. doi: 10.1016/j.plantsci.2011.04.007
Meldau, D. G., Meldau, S., Hoang, L. H., Underberg, S., Wunsche, H., and Baldwin, I. T. (2013). Dimethyl disulfide produced by the naturally associated bacterium Bacillus sp B55 promotes Nicotiana attenuata growth by enhancing sulfur nutrition. Plant Cell 25, 2731–2747. doi: 10.1105/tpc.113.114744
Mesa-Marín, J., Del-Saz, N. F., Rodríguez-Llorente, I. D., Redondo-Gómez, S., Pajuelo, E., Ribas-Carbó, M., et al. (2018). PGPR reduce root respiration and oxidative stress enhancing Spartina maritima root growth and heavy metal rhizoaccumulation. Front. Plant Sci. 9:1500. doi: 10.3389/fpls.2018.01500
Mishra, P. K., Bisht, S. C., Ruwari, P., Joshi, G. K., Singh, G., Bisht, J. K., et al. (2011). Bioassociative effect of cold tolerant Pseudomonas spp. and Rhizobium leguminosarum-PR1 on iron acquisition, nutrient uptake and growth of lentil (Lens culinaris L.). Eur. J. Soil Biol. 47, 35–43. doi: 10.1016/j.ejsobi.2010.11.005
Mishra, R. K., Prakash, O., Alam, M., and Dikshit, A. (2010). Influence of Plant Growth Promoting Rhizobacteria (PGPR) on the productivity of Pelargonium Graveolens L. Herit. Recent Res. Sci. Technol. 2, 53–57.
Molina-Favero, C., Creus, C. M., Simontacchi, M., Puntarulo, S., and Lamattina, L. (2008). Aerobic nitric oxide production by Azospirillum brasilense Sp245 and its influence on root architecture in tomato. Mol. Plant Microbe Interact. 21, 1001–1009. doi: 10.1094/mpmi-21-7-1001
Molla, A. H., Shamsuddin, Z. H., and Saud, H. M. (2001). Mechanism of root growth and promotion of nodulation in vegetable soybean by Azospirillum brasilense. Commun. Soil Sci. Plant Anal. 32, 2177–2187. doi: 10.1081/css-120000276
Murray, J. D., Karas, B. J., Sato, S., Tabata, S., Amyot, L., and Szczyglowski, K. (2007). A cytokinin perception mutant colonized by Rhizobium in the absence of nodule organogenesis. Science 315, 101–104. doi: 10.1126/science.1132514
Namwongsa, J., Jogloy, S., Vorasoot, N., Boonlue, S., Riddech, N., and Mongkolthanaruk, W. (2019). Endophytic bacteria improve root traits, biomass and yield of Helianthus tuberosus L. under normal and deficit water conditions. J. Microbiol. Biotechnol. 29, 1777–1789. doi: 10.4014/jmb.1903.03062
Nascimento, F. X., Brígido, C., Glick, B. R., and Oliveira, S. (2012). ACC deaminase genes are conserved among Mesorhizobium species able to nodulate the same host plant. FEMS Microbiol. Lett. 336, 26–37. doi: 10.1111/j.1574-6968.2012.02648.x
Neeraj, and Singh, K. (2011). Organic amendments to soil inoculated arbuscularmycorrhizal fungi and Pseudomonas fluorescens treatments reduce the development of root-rot disease and enhance the yield of Phaseolus vulgaris L. Euro J. Soil Biol. 47, 288–295. doi: 10.1016/j.ejsobi.2011.07.002
Nie, M., Bell, C., Wallenstein, M. D., and Pendall, E. (2015). Increased plant productivity and decreased microbial respiratory C loss by plant growth-promoting rhizobacteria under elevated CO2. Sci. Rep. 5:9212. doi: 10.1038/srep09212
Nosheen, A., Bano, A., Ullah, F., Farooq, U., Yasmin, H., and Hussain, I. (2011). Effect of plant growth promoting rhizobacteria on root morphology of Safflower (Carthamus tinctorius L.). Afr. J. Biotechnol. 10, 12639–12649. doi: 10.5897/AJB11.1647
Okon, Y. (1985). Azospirillum as a potential inoculant for agriculture. Trends Biotechnol. 3, 223–228.
Okon, Y., and Kapulnik, Y. (1986). Development and function of Azospirillum-inoculated roots. Plant and Soil. 90, 3–16.
Oláh, B., Brière, C., Bécard, G., Dénarié, J., and Gough, C. (2005). Nod factors and a diffusible factor from arbuscular mycorrhizal fungi stimulate lateral root formation in Medicago truncatula via the DMI1/DMI2 signalling pathway. Plant J. 44, 195–207. doi: 10.1111/j.1365-313x.2005.02522.x
Orfanoudakis, M., Wheeler, C. T., and Hooker, J. E. (2010). Both the arbuscular mycorrhizal fungus Gigaspora rosea and Frankia increase root system branching and reduce root hair frequency in Alnus glutinosa. Mycorrhiza 20, 117–126. doi: 10.1007/s00572-009-0271-0
Ortíz-Castro, R., Contreras-Cornejo, H. A., Macías-Rodríguez, L., and LópezBucio, J. (2009). The role of microbial signals in plant growth and development. Plant Signal. Behav.4, 701–712. doi: 10.4161/psb.4.8.9047
Ortiz-Castro, R., Diaz-Perez, C., Martinez-Trujillo, M., del Rio, R. E., Campos-Garcia, J., and Lopez-Bucio, J. (2011). Transkingdom signaling based on bacterial cyclodipeptides with auxin activity in plants. Proc. Nat. Acad. Sci. U.S.A. 108, 7253–7258. doi: 10.1073/pnas.1006740108
Ortíz-Castro, R., Martínez-Trujillo, M., and López-Bucio, J. (2008). N-acyl-Lhomoserine lactones: a class of bacterial quorum-sensing signals alter post-embryonic root development in Arabidopsis thaliana. Plant Cell Environ. 31, 1497–1509. doi: 10.1111/j.1365-3040.2008.01863.x
Pagnussat, G. C., Lanteri, M. L., and Lamattina, L. (2003). Nitric oxide and cyclic GMP are messengers in the indole acetic acid-induced adventitious rooting process. Plant Physiol. 132, 1241–1248. doi: 10.1104/pp.103.022228
Pagnussat, G. C., Simontacchi, M., Puntarulo, S., and Lamattina, L. (2002). Nitric Oxide is required for root organogenesis. Plant Physiol. 129, 954–956. doi: 10.1104/pp.004036
Pal, K. K., Tilak, K. V. B. R., Saxena, A. K., Dey, R., and Singh, C. S. (2001). Suppression of maize root diseases caused by Macrophomina phaseolina, Fusarium moniliforme and Fusarium graminearum by plant growth promoting rhizobacteria. Microbiol. Res. 156, 209–223. doi: 10.1078/0944-5013-00103
Patten, C. L., and Glick, B. R. (2002a). Regulation of indoleacetic acid production in Pseudomonas putidaGR12-2 by tryptophan and the stationary-phase sigma factor RpoS. Can. J. Microbiol. 48, 635–642. doi: 10.1139/w02-053
Patten, C. L., and Glick, B. R. (2002b). Role of Pseudomonas putida indoleacetic acid in development of the host plant root system. Appl. Environ. Microbiol. 68, 3795–3801. doi: 10.1128/aem.68.8.3795-3801.2002
Péret, B., De Rybel, B., Casimiro, I., Benkova, E., Swarup, R., Laplaze, L., et al. (2009). Arabidopsis lateral root development: an emerging story. Trends Plant Sci. 14, 399–408. doi: 10.1016/j.tplants.2009.05.002
Péret, B., Li, G., Zhao, J., Band, L. R., Vob, U., Postaire, O., et al. (2012). Auxin regulates aquaporin function to facilitate lateral root emergence. Nature Cell Biol. 14, 991–998. doi: 10.1038/ncb2573
Perrig, D., Boiero, M. L., Masciarelli, O. A., Penna, C., Ruiz, O. A., Cassán, F. D., et al. (2007). Plant-growth- promoting compounds produced by two agronomically important strains of Azospirillum brasilense, and implications for inoculant formulation. Appl. Microbiol. Biotechnol. 75, 1143–1150. doi: 10.1007/s00253-007-0909-9
Plet, J., Wasson, A., Ariel, F., Le Signor, C., Baker, D., Mathesius, U., et al. (2011). MtCRE1-dependent cytokinin signaling integrates bacterial and plant cues to coordinate symbiotic nodule organogenesis in Medicago truncatula. Plant J. 65, 622–633. doi: 10.1111/j.1365-313x.2010.04447.x
Podlesakova, K., Fardoux, J., Patrel, D., Bonaldi, K., Novak, O., Strnad, M., et al. (2013). Rhizobial synthesized cytokinins contribute to but are not essential for the symbiotic interaction between photosynthetic Bradyrhizobia and Aeschynomene legumes. Mol. Plant Microbe Interact. 26, 1232–1238. doi: 10.1094/mpmi-03-13-0076-r
Polkade, A. V., Mantri, S. S., Patwekar, U. J., and Jangid, K. (2016). Quorum sensing: an under-explored phenomenon in the phylum Actinobacteria. Front. Microbiol. 7:131. doi: 10.3389/fmicb.2016.00131
Pothier, J. F., Wisniewski-Dyé, F., Weiss-Gayet, M., Moënne-Loccoz, Y., and Prigent-Combaret, C. (2007). Promoter-trap identification of wheat seed extract-induced genes in the plant-growth-promoting rhizobacterium Azospirillum brasilense Sp245. Microbiology 153, 3608–3622. doi: 10.1099/mic.0.2007/009381-0
Prasanna, R., Joshi, M., Rana, A., Shivay, Y. S., and Nain, L. (2011). Influence of co-inoculation of bacteria-cyanobacteria on crop yield and C–N sequestration in soil under rice crop. World J. Microbiol. Biotechnol. 28, 1223–1235. doi: 10.1007/s11274-011-0926-9
Prudent, M., Salon, C., Souleimanov, A., Emery, R. J. N., and Smith, D. L. (2015). Soybean is less impacted by water stress using Bradyrhizobium japonicum and thuricin-17 from Bacillus thuringiensis. Agron. Sustain. Dev. 35, 749–757. doi: 10.1007/s13593-014-0256-z
Qurashi, A. W., and Sabri, A. N. (2012). Bacterial exopolysaccharide and biofilm formation stimulate chickpea growth and soil aggregation under salt stress. Braz. J. Microbiol. 43, 1183–1191. doi: 10.1590/S1517-83822012000300046
Ramaekers, L., Remans, R., Rao, I. M., Blair, M.W, and Vanderleyden, J. (2010). Strategies for improving phosphorus acquisition efficiency of crop plants. Field Crops Res. 117, 169–176. doi: 10.1016/j.fcr.2010.03.001
Rana, A., Saharan, B., Nain, L., Prasanna, R., and Shivay, Y. S. (2012). Enhancing micronutrient uptake and yield of wheat through bacterial PGPR consortia. Soil Sci. Plant Nutr. 58, 573–582, doi: 10.1080/00380768.2012.716750
Rashid, M. I., Mujawar, L. H., Shahzad, T., Almeelbi, T., Ismail, I. M. I., and Oves, M. (2016). Bacteria and fungi can contribute to nutrients bioavailability and aggregate formation in degraded soils. Microbiol. Res. 183, 26–41. doi: 10.1016/j.micres.2015.11.007
Ravikumar, R. (2012). Growth effects of Rhizobium inoculation in some legume plants. Int. J. Curr. Sci. 2012, 1–6.
Remans, R., Beebe, S., Blair, M., Manrique, G., Tovar, E., Rao, I. M., et al. (2008). Physiological and genetic analysis of root responsiveness to auxin-producing plant growth-promoting bacteria in common bean (Phaseolus vulgaris L.). Plant Soil 302, 149–161. doi: 10.1007/s11104-007-9462-7
Remans, R., Croonenborghs, A., Torres Gutierrez, R., Michiels, J., and Vanderleyden, J. (2007). Effects of plant growth-promoting rhizobacteria on nodulation of Phaseolus vulgaris L. are dependent on plant P nutrition. Eur. J. Plant Pathol. 119, 341–351. doi: 10.1007/978-1-4020-6776-1_9
Ribeiro, C. M., and Cardoso, E. J. B. N. (2012). Isolation, selection and characterization of root- associated growth-promoting bacteria in Brazil Pine (Araucaria angustifolia). Microbiol. Res. 167, 69–78. doi: 10.1016/j.micres.2011.03.003
Richardson, A. E., Baréa, J. M., McNeill, A. M., and Prigent-Combaret, C. (2009). Acquisition of phosphorus and nitrogen in the rhizosphere and plant growth promotion by microorganisms. Plant Soil 321, 305–339. doi: 10.1007/s11104-009-9895-2
Richardson, A. E., and Simpson, R. J. (2011). Soil microorganisms mediating phosphorus availability update on microbial phosphorus. Plant Physiol. 156, 989–996. doi: 10.1104/pp.111.175448
Riefler, M., Novak, O., Strnad, M., and Schmülling, T. (2006). Arabidopsis cytokinin receptor mutants reveal functions in shoot growth, leaf senescence, seed size, germination, root development, and cytokinin metabolism. Plant Cell 18, 40–54. doi: 10.1105/tpc.105.037796
Rozier, C. (2016). Xylem sap metabolite profile changes during phytostimulation of maize by the plant growth-promoting rhizobacterium, Azospirillum lipoferum CRT1. J. Postgenomics Drug Biomark. Dev. 6:3. doi: 10.4172/2153-0769.1000182
Rudrappa, T., Czymmek, K. J., Pare, P. W., and Bais, H. P. (2008). Root-secreted malic acid recruits beneficial soil bacteria. Plant Physiol. 148, 1547–1556. doi: 10.1104/pp.108.127613
Ruiz, S., Kobernick, N., Duncan, S., Fletcher, Y., McKay, S. C., Boghi, A., et al. (2019). Significance of root hairs at the field scale-modelling root water and phosphorus uptake under different field conditions. Plant Soil 47, 281–304. doi: 10.1007/s11104-019-04308
Ruzzi, M., and Aroca, R. (2015). Plant growth-promoting rhizobacteria act as biostimulants in horticulture. Sci. Hort. 196, 124–134. doi: 10.1016/j.scienta.2015.08.042
Ryu, C. M., Farag, M. A., Hu, C. H., Reddy, M. S., Wei, H. X., Par,é, P. W., et al. (2003). Bacterial volatiles promote growth in Arabidopsis. Proc. Natl. Acad. Sci. U.S.A. 100, 4927–4932. doi: 10.1073/pnas.0730845100
Sabir, A., Yazici, M. A., Kara, Z., and Sahin, F. (2012). Growth and mineral acquisition response of grapevine rootstocks (Vitis spp.) to inoculation with different strains of Plant Growth-Promoting Rhizobacteria (PGPR). J. Sci. Food Agric. 92, 2148–2153. doi: 10.1002/jsfa.5600
Sahgal, M., and Johri, B. N. (2003). The changing face of rhizobial systematics. Curr. Sci. 84, 43–48. Available online at: http://www.jstor.org/stable/24107372
Sanchez, C., Gates, A. J., Meakin, G. E., Uchiumi, T., Girard, L., Richardson, D. J., et al. (2010). Production of nitric oxide and nitrosylleghemoglobin complexes in soybean nodules in response to flooding. Mol. Plant Microbe Interact. 23, 702–711. doi: 10.1094/MPMI-23-5-0702
Sandhya, V., Sk, Z. A., Grover, M., Reddy, G., and Venkateswarlu, B. (2009). Alleviation of drought stress effects in sunflower seedlings by the exopolysaccharides producing Pseudomonas putida strain GAP-P45. Biol. Fert. Soil 46, 17–26. doi: 10.1007/s00374-009-0401-z
Sarkar, J., Chakraborty, B., and Chakraborty, U. (2018). Plant growth promoting Rhizobacteria protect wheat plants against temperature stress through antioxidant signalling and reducing chloroplast and membrane injury. J. Plant Growth Regul. 37, 1396–1412. doi: 10.1007/s00344-018-9789-8
Sarma, R. K., and Saikia, R. (2013). Alleviation of drought stress in mung bean by strain Pseudomonas aeruginosa GGRJ21. Plant Soil 377, 111–126. doi: 10.1007/s11104-013-1981-9
Sayyed, R. Z. (2019). “Plant growth promoting rhizobacteria for sustainable stress management,” in Microorganisms for Sustainability 1 & 2, ed N. K. Arora (Springer Nature Singapur Pte Ltd.). doi: 10.1007/978-981-13-6986-5
Scheible, W. R., Gonzalez-Fontes, A., Lauerer, M., Muller-Rober, B., Caboche, M., and Stitt, M. (1997). Nitrate acts as a signal to induce organic acid metabolism and repress starch metabolism in Tobacco. Plant Cell 9, 783–798. doi: 10.1105/tpc.9.5.783
Scheres, B., Benfey, P., and Dolan, L. (2002). Root development. Arabidopsis Book 1:e0101. doi: 10.1199/tab.0101
Schuhegger, R., Ihring, A., Gantner, S., Bahnweg, G., Knappe, C., Vogg, G., et al. (2006). Induction of systemic resistance in tomato by N-acyl-L-homoserine lactone-producing rhizosphere bacteria. Plant Cell Environ. 29, 909–918. doi: 10.1111/j.1365-3040.2005.01471.x
Schwartz, A., Ortiz, I., Maymon, M., Herbold, C., Fujishige, N., Vijanderan, J., et al. (2013). Bacillus simplex—A little known PGPB with anti-fungal activity—alters pea legume root architecture and nodule morphology when coinoculated with Rhizobium leguminosarum bv. viciae. Agronomy 3, 595–620. doi: 10.3390/agronomy3040595
Sekar, C., Prasad, N. N., and Sundaram, M. D. (2000). Enhancement of polygalacturonase activity during auxin induced para nodulation and endorhizosphere colonization of Azospirillum in rice roots. Indian J. Exp. Biol. 38, 80–83.
Shafi, J., Tian, H., and Ji, M. (2017). Bacillus species as versatile weapons for plant pathogens: a review. Biotechnol. Biotechnol. Equip. 31, 446–459. doi: 10.1080/13102818.2017.1286950
Shahzad, S. M., Khalid, A., Arshad, M., Tahir, J., and Mahmood, T. (2010). Improving nodulation, growth and yield of Cicer arietinum L. through bacterial ACC-deaminase induced changes in root architecture. Euro J. Soil Biol. 46, 342–347. doi: 10.1016/j.ejsobi.2010.05.007
Sheibani-Tezerji, R., Rattei, T., Sessitsch, A., Trognitz, F., and Mitter, B. (2015). Transcriptome profiling of the endophyte Burkholderia phytofirmans PsJN indicates sensing of the plant environment and drought stress. mBio 6, e00621–e00615. doi: 10.1128/mBio.00621-15
Shi, C., Park, H., Lee, J. S., Ryu, S., and Ryu, C. M. (2010). Inhibition of primary roots and stimulation of lateral root development in Arabidopsis thaliana by the rhizobacterium Serratia marcescens 90–166 is through both auxin-dependent and -independent signaling pathways. Mol. Cell 29, 251–258 (2010). doi: 10.1007/s10059-010-0032-0
Silverman, F. P., Assiamah, A. A., and Bush, D. S. (1998). Membrane transport and cytokinin action in root hairs of Medicago sativa. Planta 205, 23–31. doi: 10.1007/s004250050292
Singh, A., Jain, A., Sarma, B. K., Upadhyay, R. S., and Singh, H. B. (2014). Rhizosphere competent microbial consortium mediates rapid changes in phenolic profiles in chickpea during Sclerotium rolfsii infection. Microbiol. Res. 169, 353–360. doi: 10.1016/j.micres.2013.09.014
Singh, A., Singh, A. P., Singh, S. K., Rai, S., and Kumar, D. (2017). Impact of addition of biochar along with PGPR on rice yield, availability of nutrients and their uptake in alluvial soil. J. Pure Appl. Microbiol. 10, 2181–2188. doi: 10.5958/0974-0228.2018.00035.x
Singh, R. P., and Jha, P. N. (2017). The PGPR Stenotrophomonas maltophilia SBP-9 augments resistance against biotic and abiotic stress in wheat plants. Front. Microbiol. 8:1945. doi: 10.3389/fmicb.2017.01945
Smith, S., and De Smet, I. (2012). Root system architecture: insights from Arabidopsis and cereal crops. Philos. Trans. R. Soc. B Biol. Sci. 367, 1441–1452. doi: 10.1098/rstb.2011.0234
Solaiman, A., Talukder, M., and Rabbani, M. (1970). Influence of some Rhizobium strains on Chickpea: nodulation, dry matter yield and nitrogen uptake. Bangladesh J. Microbiol. 27, 61–64. doi: 10.3329/bjm.v27i2.9174
Spaepen, S., Bossuyt, S., Engelen, K., Marchal, K., and Vanderleyden, J. (2014). Phenotypical and molecular responses of Arabidopsis thaliana roots as a result of inoculation with the auxin producing bacterium Azospirillum brasilense. New Phytol. 201, 850–861. doi: 10.1111/nph.12590
Spaepen, S., Vanderleyden, J., and Remans, R. (2007). Indole-3-acetic acid in microbial and microorganism–plant signaling. FEMS Microbiol. Rev. 31, 425–448. doi: 10.1111/j.1574-6976.2007.00072.x
Splivallo, R., Fischer, U., Göbel, C., Feussner, I., and Karlovsky, P. (2009). Truffles regulate plant root morphogenesis via the production of auxin and ethylene. Plant Physiol. 150, 2018–2029. doi: 10.1104/pp.109.141325
Srivastava, S., Bist, V., Srivastava, S., Singh, P. C., Trivedi, P. K., Asif, M. H., et al. (2016). Unraveling aspects of Bacillus amyloliquefaciens mediated enhanced production of rice under biotic stress of Rhizoctoniasolani. Front. Plant Sci. 7:587. doi: 10.3389/fpls.2016.00587
Stajković, O., Delić, D., Josic, D., Kuzmanović, Ã., Rasulić, N., and KneŽević- Vukčević, J. (2011). Improvement of common bean growth by co-inoculation with Rhizobium and plant growth promoting bacteria. Rom. Biotechnol. Lett. 16, 5919–5926.
Steenhoudt, O., Keijers, V., Okon, Y., and Vanderleyden, J. (2001). Identification and characterization of a periplasmic nitrate reductase in Azospirillum brasilense Sp245. Arch. Microbiol. 175, 344–352. doi: 10.1007/s002030100271
Stohr, C., and Ullrich, W. (2002). Generation and possible roles of NO in plant roots and their apoplastic space. J. Exp. Botany 53, 2293–2303. doi: 10.1093/jxb/erf110
Stuehr, D. J. (1997). Structure-function aspects in the nitric oxide synthases. Ann. Rev. Pharmacol. Toxicol. 37, 339–359. doi: 10.1146/annurev.pharmtox.37.1.339
Sukumar, P., Legué, V., Vayssières, A., Martin, F., Tuskan, G. A., and Kalluri, U. C. (2013). Involvement of auxin pathways in modulating root architecture during beneficial plant-microorgansim interactions. Plant Cell Environ. 28, 67–77. doi: 10.1111/pce.12036
Teplitski, M., Robinson, J. B., and Bauer, W. D. (2000). Plants secrete substances that mimic bacterial N-acyl homoserine lactone signal activities and affect population density-dependent behaviors in associated bacteria. Mol. Plant Microbe Interact. 13, 637–648. doi: 10.1094/MPMI.2000.13.6.637
Tien, T. M., Gaskins, M. H., and Hubell, D. H. (1979). Plant growth substances produced by Azospirillum brasilense and their effect on the growth of pearl millet (Pennisetum americanum L). Appl. Environ. Microbiol. 37, 1016–1024. doi: 10.1128/aem.37.5.1016-1024.1979
Timmusk, S., Abd El-Daim, I. A., Copolovici, L., Tanilas, T., Kännaste, A., Behers, L., et al. (2014). Drought-tolerance of wheat improved by rhizosphere bacteria from harsh environments: enhanced biomass production and reduced emissions of stress volatiles. PLoS ONE 9:e96086. doi: 10.1371/journal.pone.0096086
Timmusk, S., Nicander, B., Granhall, U., and Tillberg, E. (1999). Cytokinin production by Paenibacillus polymyxa. Soil Biol. Biochem. 31, 1847–1852. doi: 10.1016/s0038-0717(99)00113-3
Tiwari, S., Lata, C., Chauhan, P. S., and Nautiyal, C. S. (2016). Pseudomonas putida attunes morphophysiological, biochemical and molecular responses in Cicer arietinum L. during drought stress and recovery. Plant Physiol. Biochem. 99, 108–117. doi: 10.1016/j.plaphy.2015.11.001
Turan, M., Ekinci, M., Yildirim, E., Güne,ş, A., Karagöz, K., Kotan, R., et al. (2014). Plant growth-promoting rhizobacteria improved growth, nutrient, and hormone content of cabbage (Brassica oleracea) seedlings. Turk. J. Agric. Fores. 38, 327–333. doi: 10.3906/tar-1308-62
Umali-Garcia, M., Hubbell, D. H., Gaskins, M. H., and Dazzo, F. B. (1980). Association of Azospirillum with grass roots. Appl. Environ. Microbiol. 39, 219–226. doi: 10.1128/aem.39.1.219-226.1980
Upadhyay, S. K., and Singh, D. P. (2014). Effect of salt-tolerant plant growth-promoting rhizobacteria on wheat plants and soil health in a saline environment. Plant Biol. 17, 288–293. doi: 10.1111/plb.12173
Upadhyay, S. K., Singh, J. S., and Singh, D. P. (2011). Exopolysaccharide-producing plant growth-promoting rhizobacteria under salinity condition. Pedosphere 21, 214–222. doi: 10.1016/s1002-0160(11)60120-3
Vacheron, J., Desbrosses, G., Bouffaud, M. L., Touraine, B., Moënne-Loccoz, Y., Muller, D., et al. (2013). Plant growth-promoting rhizobacteria and root system functioning. Front. Plant Sci. 4:356. doi: 10.3389/fpls.2013.00356
van Oosten, M. J., Pepe, O., De Pascale, S., Silletti, S., and Maggio, A. (2017). The role of biostimulants and bioeffectors as alleviators of abiotic stress in crop plants. Chem. Biol. Technol. Agric. 4:5. doi: 10.1186/s40538-017-0089-5
Veresoglou, S. D., and Menexes, G. (2010). Impact of inoculation with Azospirillum spp. on growth properties and seed yield of wheat: a meta-analysis of studies in the ISI Web of science from 1981 to 2008. Plant Soil 337, 469–480 doi: 10.1007/s11104-010-0543-7
Vessey, J. K. (2003). Plant growth promoting rhizobacteria as biofertilizers. Plant Soil 255, 571–586. doi: 10.1023/a:1026037216893
Volpiano, C. G., Lisboa, B. B., Granada, E., São Jos,é, J. B. F., de Oliveira, A. M. R., Beneduzi, A., et al. (2019). “Rhizobia for biological control of plant diseases,” in Microbiome in Plant Health and Disease, eds V. Kumar, R. Prasad, M. Kumar, and D. Choudhary (Singapore: Springer), 315–336.
Walker, V., Couillerot, O., Von Felten, A., Bellvert, F., Jansa, J., Maurhofer, M., et al. (2011). Variation of secondary metabolite levels in maize seedling roots induced by inoculation with Azospirillum, Pseudomonas and Glomus consortium under field conditions. Plant Soil 356, 151–163. doi: 10.1007/s11104-011-0960-2
Walley, F. L., Gillespie, A. W., Adetona, A. B., Germida, J. J., and Farrell, R. E. (2014). Manipulation of rhizosphere organisms to enhance glomalin production and C sequestration: Pitfalls and promises. Can. J. Plant Sci. 94, 1025–1032. doi: 10.4141/cjps2013-146
Wang, J., Zhang, Y., Li, Y., Wang, X., Nan, W., Hu, Y., et al. (2015). Endophytic microbes Bacillus sp. LZR216-regulated root development is dependent on polar auxin transport in Arabidopsis seedlings. Plant Cell Rep. 34, 1075–1087. doi: 10.1007/s00299-015-1766-0
Wang, N., Wang, L., Zhu, K.ai., Hou, S., Chen, L.in., Mi, D., et al. (2019). Plant root exudates are involved in Bacillus cereus AR156 mediated biocontrol against Ralstonia solanacearum. Front. Microbiol. 10:98. doi: 10.3389/fmicb.2019.00098
Weller, D. M. (2007). Pseudomonas biocontrol agents of soil borne pathogens: Looking back over 30 years. Phytopathology 97, 250–256. doi: 10.1094/PHYTO-97-2-0250
Weller, D. M., Raaijmakers, J. M., McSpadden Gardener, B. B., and Thomashow, L. S. (2002). Microbia populations responsible for specific soil suppressiveness to plant pathogens. Ann. Rev. Phytopathol. 40, 309–348. doi: 10.1146/annurev.phyto.40.030402.110010
Werner, T., Nehnevajova, E., Kollmer, I., Novak, O., Strnad, M., Kramer, U., et al. (2010). Root-specific reduction of cytokinin causes enhanced root growth, drought tolerance, and leaf mineral enrichment in Arabidopsis and tobacco. Plant Cell 22, 3905–392. doi: 10.1105/tpc.109.072694
Whalley, W. R., Riseley, B., Leeds-Harrison, P. B., Bird, N. R. A., Leech, P. K., and Adderley, W. P. (2005). Structural differences between bulk and rhizosphere soil. Euro J. Soil Sci. 56, 353–360. doi: 10.1111/j.1365-2389.2004.00670.x
Wrage, N., Velthof, G. L., van Beusichem, M. L., and Oenema, O. (2001). Role of nitrifier denitrification in the production of nitrous oxide. Soil Biol. Biochem. 33, 1723–1732. doi: 10.1016/s0038-0717(01)00096-7
Yanni, Y. G., Rizk, R. Y., El-Fattah, F. K. A., Squartini, A., Corich, V., Giacomini, A., et al. (2001). The beneficial plant growth-promoting association of Rhizobium leguminosarum bv. trifolii with rice roots. Funct. Plant Biol. 28:845. doi: 10.1071/pp01069
Yaxley, J. R., Ross, J. J., Sherriff, L. J., and Reid, J. B. (2001). Gibberellin biosynthesis mutations and root development in pea. Plant Physiol. 125, 627–633. doi: 10.1104/pp.125.2.627
Yim, W., Seshadri, S., Kim, K., Lee, G., and Sa, T. (2013). Ethylene emission and PR protein synthesis in ACC deaminase producing Methylobacterium spp. inoculated tomato plants (Lycopersicon esculentum Mill.) challenged with Ralstonia solanacearum under greenhouse conditions. Plant Physiol. Biochem. 67, 95–104. doi: 10.1016/j.plaphy.2013.03.002
Yuttavanichakul, W., Lawongsa, P., Wongkaew, S., Teaumroong, N., Boonkerd, N., Nomura, N., et al. (2012). Improvement of peanut rhizobial inoculant by incorporation of Plant Growth Promoting Rhizobacteria (PGPR) as biocontrol against the seed borne fungus, Aspergillus niger. Biol. Control 63, 87–97. doi: 10.1016/j.biocontrol.2012.06.008
Zamioudis, C., Mastranesti, P., Dhonukshe, P., Blilou, I., and Pieterse, C. M. J. (2013). Unraveling root developmental programs initiated by beneficial Pseudomonas spp. bacteria. Plant Physiol. 162, 304–318. doi: 10.1104/pp.112.212597
Zamioudis, C., and Pieterse, C. M. J. (2012). Modulation of host immunity by beneficial microbes. Mol. Plant Microbe Interact. 25, 139–150. doi: 10.1094/MPMI-06-11-0179
Zarea, M., Hajinia, S., Karimi, N., Goltapeh, E. M., Rejali, F., and Varma, A. (2012). Effect of Piriformospora indica and Azospirillum strains from saline or non-saline soil on mitigation of the effects of NaCl. Soil Biol. Biochem. 45, 139–146. doi: 10.1016/j.soilbio.2011.11.006
Zhang, H., Kim, M. S., Krishnamachari, V., Payton, P., Sun, Y., Grimson, M., et al. (2007). Rhizobacterial volatile emissions regulate auxin homeostasis and cell expansion in Arabidopsis. Planta 226, 839–851. doi: 10.1007/s00425-007-0530-2
Zhang, H., Murzello, C., Sun, Y., Kim, X., Mi, -S R, Jeter, R. M., et al. (2010). Choline and osmotic-stress tolerance induced in Arabidopsis by the soil microbe Bacillus subtilis (GB03). Mol. Plant Microb. Interact. 23, 1097–1104. doi: 10.1094/MPMI-23-8-1097
Zhang, M., Yang, L., Hao, R., Bai, X., Wang, Y., and Yu, X. (2020). Drought-tolerant plant growth-promoting rhizobacteria isolated from jujube (Ziziphus jujuba) and their potential to enhance drought tolerance. Plant Soil 452, 423–440. doi: 10.1007/s11104-020-04582-5
Keywords: root architecture, microbial determinants, root system functioning, gene regulation, hormonal regulation
Citation: Grover M, Bodhankar S, Sharma A, Sharma P, Singh J and Nain L (2021) PGPR Mediated Alterations in Root Traits: Way Toward Sustainable Crop Production. Front. Sustain. Food Syst. 4:618230. doi: 10.3389/fsufs.2020.618230
Received: 16 October 2020; Accepted: 08 December 2020;
Published: 08 January 2021.
Edited by:
Everlon Cid Rigobelo, Universidade Estadual Paulista, BrazilReviewed by:
Claire Prigent-Combaret, UMR5557 Ecologie Microbienne (LEM), FranceGayathri Ilangumaran, McGill University, Canada
Copyright © 2021 Grover, Bodhankar, Sharma, Sharma, Singh and Nain. This is an open-access article distributed under the terms of the Creative Commons Attribution License (CC BY). The use, distribution or reproduction in other forums is permitted, provided the original author(s) and the copyright owner(s) are credited and that the original publication in this journal is cited, in accordance with accepted academic practice. No use, distribution or reproduction is permitted which does not comply with these terms.
*Correspondence: Lata Nain, bGF0YXJhamF0JiN4MDAwNDA7eWFob28uY28uaW4=; orcid.org/000-0001-9551-0940