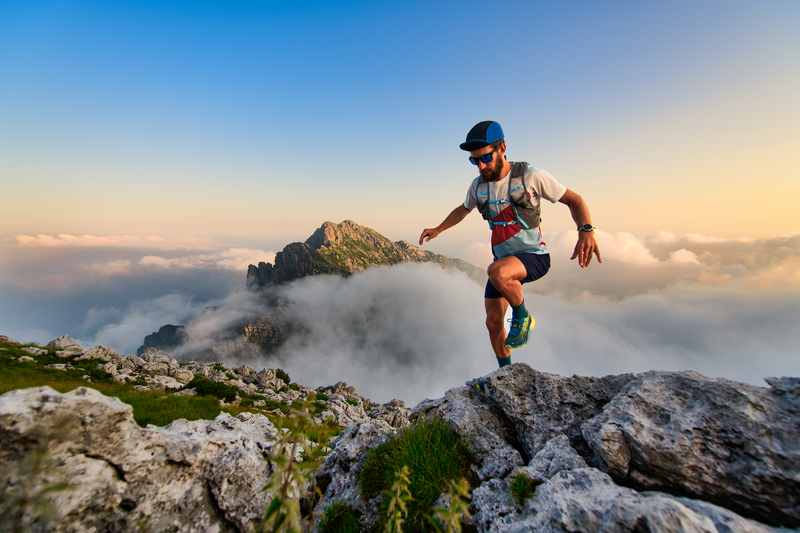
94% of researchers rate our articles as excellent or good
Learn more about the work of our research integrity team to safeguard the quality of each article we publish.
Find out more
ORIGINAL RESEARCH article
Front. Sustain. Food Syst. , 27 January 2021
Sec. Crop Biology and Sustainability
Volume 4 - 2020 | https://doi.org/10.3389/fsufs.2020.617978
This article is part of the Research Topic Plant Growth-Promoting Microorganisms for Sustainable Agricultural Production View all 50 articles
Soybean is the most widely grown legume worldwide, but it is a glycophyte and salinity stress can decrease its yield potential up to 50%. Plant growth promoting rhizobacteria (PGPR) are known to enhance growth and induce tolerance to abiotic stresses including salinity. The aim of this study was to isolate such PGPR from the root nodules of Amphicarpaea bracteata, a North American relative of soybean. Isolated strains were identified, and 15 strains were screened for potential utilization as PGPR of soybean through a series of greenhouse trials. Four isolates that greatly improved shoot and root growth were further selected and screened under a range of salt concentrations. Two of the most promising strains, Rhizobium sp. SL42 and Hydrogenophaga sp. SL48 were ascertained to exert the greatest beneficial effects on soybean growth and salinity tolerance. They were co-inoculated with Bradyrhizobium japonicum 532C (Bj) and the plants were grown up to the harvest stage. The treatment of Bj+SL42 resulted in higher shoot biomass than the control, 18% at the vegetative stage, 16% at flowering, 7.5% at pod-filling, and 4.6% at harvest and seed weight was increased by 4.3% under salt stress (ECe = 7.4 ds/m). Grain yield was raised under optimal conditions by 7.4 and 8.1% with treatments Bj+SL48 and Bj+SL42+SL48, respectively. Nitrogen assimilation and shoot K+/Na+ ratio were also higher in the co-inoculation treatments. This study suggested that inoculation with bacteria from an indigenous legume can induce stress tolerance, improve growth and yield to support sustainability, and encourage ecological adaptability of soybean.
Salinity is a major threat to agricultural sustainability and undermining the crop productivity on arable lands worldwide of which more than 50% is predicted to be affected by 2050 (Ashraf, 1994). It is anticipated that climate changes may hasten the aridisation of the Great Plains of North America during this century, leading to deficits in groundwater level and increased salinization of soil and water resources (Florinsky et al., 2009). The Canadian Prairies are susceptible to salinity, due to their soil type, moisture loss, high evapotranspiration rates and mineral salts in groundwater (Wiebe et al., 2007). The temporal fluctuation of soil salinity in this region is controlled by groundwater depth, which in turn is related to annual precipitation (Florinsky et al., 2009). Excessive use of road salts during winter may risk contamination of water sources and groundwater table in regions above 40°N (EnvironmentCanada, 2001). Soil salinity is also a pressing problem in many states of the USA on both irrigated and rainfed agricultural lands (NRCS, 2002). Salinity stress is mainly caused by uptake of NaCl, the dominant salt in nature, which creates both osmotic and ionic imbalances in plants. These lead to physiological dysfunctions that inhibit plant growth and development, thereby declining crop yield (Munns and Tester, 2008). Soil salinization has caused an estimated annual loss of $257 million CAD to Canadian farmers in 1998. Even though salinization risk has been lowered in the Prairies through better land-soil-water management practices, it persists to be a localized issue (AAFC, 2020).
Soybean [Glycine max (L.) Merrill] is an agriculturally important grain legume and oilseed crop worldwide. Due to the abundant protein (36%) and oil (19%) reserves in its seed, soybean has found uses as food for human consumption, animal feed, edible oil and industrial products (Thoenes, 2004). Soybean is capable of fixing atmospheric nitrogen through its symbiotic association with species of Bradyrhizobium (B. japonicum, B. elkani, B. liaoningense, and B. yuanmingense) in root nodules. Hence, it is an ideal rotation crop with corn, wheat, cotton and other arable crops, to increase soil nitrogen content and reduce production costs. Cultivation of soybean has gained significance in North America after world war II (Cloutier, 2017). Now, Canada and the USA are prominent global suppliers and consumers with the USA ranking first (60% of soybean trade). Soybean production has been steadily on the rise in Canada during recent years (Supplementary Table 1) and cultivation has expanded in the southern regions, bolstered by the introduction of early-maturing varieties (Dorff, 2007).
However, sub-optimal growing conditions are met with environmental challenges other than just low temperatures. Soybean is basically a short-day plant (development is influenced by daylength), relatively resistant to temperature fluctuations (more extreme temperatures affect flowering and pod-setting), grown in a wide range of soils (except very sandy), sensitive to waterlogging and moderately tolerant to drought and salinity (FAO, 2002). According to the FAO crop database, “yield decrease due to soil salinity is: 0% at ECe 5 mmhos/cm, 10% at 5.5, 25% at 6.2, 50% at 7.5 and 100% at ECe 10 mmhos/cm.” Salinity stress may cause physiological and biochemical disorders in soybean that inhibit seed germination and plant growth, aggravate leaf chlorosis and bleaching, decrease biomass accumulation, restrain nodulation and nitrogen fixation, and reduce yield and seed quality (Phang et al., 2008). Salinity has significantly reduced the germination percentage, plant height and shoot dry weight of 45 day-old plants of three soybean cultivars. There was also an increase in sodium and chloride levels in the leaf tissues (Essa, 2002). In soybean cv. Williams, seedling growth declined to 5% at 220 mmolal NaCl and no growth was recorded at 330 mmolal NaCl (Hosseini et al., 2002). Association mapping of soybean seed germination revealed 1,142 single nucleotide polymorphisms associated with salt tolerance. Salinity tolerance is influenced by numerous genetic and environmental factors and a complex trait, such that molecular breeding for salt-tolerant soybean cultivars has been challenging (Kan et al., 2015).
When commercial cultivation of soybean began in North America (early twentieth century), seeds were inoculated with the Bradyrhizobium strains capable of nodulating soybean to facilitate nitrogen fixation because they were not existing in the soil. Thus, populations of bradyrhizobia have become established in soils that had no prior soybean cropping history as a result of selective enrichment over the years by the host plant (Weaver et al., 1972). Subsequently, there has been research interest in exploring the symbiotic associations of native legumes and their relationships with soybean. Amphicarpaea bracteata (American hog peanut) is an annual plant of the family Leguminosae, native to eastern North America, found in a variety of partially shaded, wet habitats (Parker, 1994). A. bracteata is closely related to soybean, confirmed by molecular studies and both genera are classified in the subtribe Glycininae of the tribe Phaseoleae (Zhu et al., 1995). Symbiotic specificity and nodule formation with rhizobia strains are genetically controlled by nodulation restriction alleles in the host legume (Devine et al., 1990; Wilkinson et al., 1996). The inoculation of soybean plants with 10 Bradyrhizobium strains from A. bracteata resulted in nodule formation but no nitrogen fixation (Marr et al., 1997). Micro-evolution was observed within Bradyrhizobium populations from the soils of soybean field sites in eastern Canada and the isolated strains were clustered with isolates from the native legumes (Tang et al., 2012). In a later study by Bromfield et al. (2017), inoculation of soybean with root-zone soils of native legumes including A. bracteata resulted in nodulation. Upon isolation, bacteria of the Bradyrhizobium genus and closely related taxa were inoculated onto soybean, and some of the bacteria containing nodC and nifH gene sequences effectively fixed nitrogen, while the others were ineffective.
Symbiotic association with rhizobia has been the primary focus of plant-microbe interaction research on legumes, and more particularly soybean, but there are also other beneficial plant growth promoting rhizobacteria (PGPR) associated with them. Endophytic bacteria were isolated from soybean nodules and co-inoculation of Bacillus subtilis NEB4, B. subtilis NEB5 and B. thuringiensis NEB17 with B. japonicum increased soybean growth and plant dry weight (Bai et al., 2002a). PGPR influence plant growth through direct and indirect mechanisms such as nitrogen fixation, nutrient assimilation, and secretion of exopolysaccharides and signaling molecules (Hynes et al., 2008; Adesemoye and Kloepper, 2009). Distinct genera of PGPR have been known to act as elicitors of induced systemic tolerance to abiotic stress (Yang et al., 2009). Many studies have reported on the beneficial role of PGPR co-inoculated with Bradyrhizobium on growth, yield and stress tolerance of soybean. Co-inoculation with Serratia proteamaculans 1–102 and S. liquefaciens 2–68 increased plant dry weight and nodule number in soybean under sub-optimal root-zone temperatures in a soil-less media (Bai et al., 2002b). In a field study, seed co-inoculation with Azospirillum brasilense increased soybean yield by 14.1% (Hungria et al., 2013). Co-inoculation with Pseudomonas putida TSAU1 improved plant growth, root architecture, nitrogen and phosphorous content of soybean under salt stress in a hydroponic experiment (Egamberdieva et al., 2017).
Diverse PGPR may be associated within the nodules of A. bracteata and they may confer better adaptation of soybean plants to the soil and environmental conditions prevailing in Canada and benefit co-inoculation with B. japonicum for nitrogen fixation. The current study had two objectives. First, bacteria isolated from the root nodules of A. bracteata were screened for their ability to enhance plant growth and salt stress tolerance of soybean by evaluating seed germination and growth parameters in a greenhouse environment. Successive screening was then performed at a range of salt concentrations to determine the threshold salinity tolerance of soybean, inoculated with selected isolates. Second, two of the most promising bacteria were co-inoculated with Bradyrhizobium japonicum 532C, to validate their role as PGPR able to induce salinity tolerance, improve nutrient assimilation and increase growth and yield of soybean plants.
Plants of Amphicarpaea bracteata were collected along the shore of Lac St. Louis on the Macdonald Campus of McGill University, located in Sainte-Anne-de-Bellevue, Quebec, Canada. The nodules present on the roots of A. bracteata were relatively smaller and fewer than those of cultivated soybean plants (Supplementary Figure 1). The nodules were separated from the roots, washed and surface sterilized using 70% (v/v) ethanol for two min. They were crushed using micro pestles and the suspension was serially diluted in sterile water. The dilutions (from 10−2 to 10−7) were plated on Kings B and yeast extract mannitol (YEM) agar plates. The plates were incubated at 25 °C for 24–96 h. Single colonies of bacteria (excluding mold or actinomycetes) that were morphologically different from one another were re-isolated on new agar plates to obtain pure colonies (Supplementary Figure 2). The individual colonies of 15 isolates were grown in liquid broth for culture maintenance and stored in glycerol stocks at −80°C.
The bacteria were grown in Kings B or YEM broth for 48 h, incubated at 25°C and 150 rpm. The cultures were harvested by centrifugation at 5,000 × g for 10 min, room temperature (AwelTM MF 48-R, NuAire, USA) and the supernatant was discarded. The pellet was suspended in 10 mM MgSO4 and the optical density was adjusted to 0.1 at A600nm (Ultraspec 4300 pro UV/Visible Spectrophotometer, Biochrom). The prepared suspension was used in downstream experiments.
The identification of the isolated bacteria was done by Sanger di-deoxy nucleotide sequencing (Genome Quebec, Montreal, Canada) of the 16S rRNA gene. Briefly, the samples were diluted 1:10 with water and the PCR mix was prepared with Taq DNA polymerase (Roche FastStart High Fidelity PCR system 2500 U), 27F (5′-AGAGTTTGATCMTGGCTCAG-3′), and 1492R (3′-TACGGYTACCTTGTTACGACTT-5′) primers and run in the PCR cycler (Eppendorf Mastercycler® ProS) for 40 cycles. The amplified product was sequenced on Applied Biosystems™ 3730XL DNA Analyzer platform. The assembled sequences (in FASTA format) were queried for similarity using the BLAST tool to find reference prokaryotic type strains (https://blast.ncbi.nlm.nih.gov/). Based on the score and percent identity, the isolated strains were classified into specific genus and species and the assembled sequences were then submitted to GenBank, NCBI (https://submit.ncbi.nlm.nih.gov/subs/genbank/). A phylogenetic analysis was performed with EMBL-EBI webservices API tools: multiple sequence alignment was generated using the interface for Clustal Omega program (https://www.ebi.ac.uk/Tools/msa/clustalo/). The output was used to generate a phylogenetic tree with the Simple Phylogeny tool (https://www.ebi.ac.uk/Tools/phylogeny/simple_phylogeny/) using the ClustalW2 program. The phylogeny tree was constructed using iTOL (Interactive Tree of Life https://itol.embl.de/) interface (Letunic and Bork, 2019) with the Phylogenetic tree file.
The isolates were tested for their tolerance capacity of salt stress at 100, 250, and 500 mM NaCl solution. The initial culture was adjusted to 0.01 OD and added to the growth media with added salt in a 96-well plate. The plate was incubated in Cytation5™ reader (BioTek Instruments Inc.,) at 25 °C and the growth curve was measured at A600nm for up to 48 h. The isolates were also screened for PGP activities including biofilm formation, nitrogen fixation, phosphorous solubilization, production of 1-aminocyclopropane-1-carboxylate (ACC) deaminase, indole-3-acetic acid (IAA) and siderophores following standard protocols (Jensen et al., 1960; Schwyn and Neilands, 1987; Bric et al., 1991; Penrose and Glick, 2003; O'Toole, 2011; Goswami et al., 2014).
Soybean seeds (Absolute RR) were soaked in the bacterial cell suspension (at a rate of 100 μL per seed) or 10 mM MgSO4 (control) for 30 min. The seeds were then placed on Petri dishes (10 seeds per plate) lined with P8 filter paper containing 5 mL of sterile water or 100 mM NaCl solution (EC = 9.8 ds m−1). The plates were sealed with parafilm, incubated at 25 °C in the dark inside a growth chamber and germination was counted at 24, 36, and 48 h periods.
Seeds were bacterized with the inoculum at a rate of 500 μL per seed. Bacterized and control seeds (5 seeds per pot) were placed in 15.25 cm (diameter) pots filled with vermiculite (Perlite Canada Inc.) treated with 300 mL water or 100 mM NaCl solution (ECe = 5 ds m−1). The pots were placed in a greenhouse room maintained at 25 ± 2°C and 50% relative humidity (Supplementary Figure 3). Seedling emergence was counted on 7th and 8th DAP (days after planting) and the plants were thinned to one seedling per pot. The plants were irrigated with 50 mL water thrice a week and fertilized with strength Hoagland's solution once a week and sampled at 28th DAP. Plant growth variables including plant height, leaf area, shoot dry weight, and root dry weight were measured. Roots were scanned (EPSON Expression 11000XL) and analyzed using WinRHIZO™ (Regent Instruments Inc.) image analysis platform to measure root volume, length, and surface area.
Based on the previous experiments, four bacterial isolates were selected and tested further for their ability to induce salt stress tolerance in soybean. A seed germination experiment as described above was conducted at different levels of salinity (0, 100, 125, 150, 175, and 200 mM NaCl solution) and at two cell densities (1 × 108 and 1 × 1010 cfu mL−1).
A pot experiment with the different salinity levels and four bacterial isolates at 1 × 108 cfu mL−1 was set in a greenhouse to test the salinity tolerance threshold of soybean. A procedure similar to that described above was followed and the plants were sampled at 28th DAP. All experiments were repeated twice with six replications for each treatment.
Two bacterial isolates were selected and co-inoculated with Bradyrhizobium japonicum 532C (all strains at 1 × 108 cfu mL−1) on soybean seeds (seed bacterization). The seeds were then placed in 25.5 cm (diameter) pots filled with vermiculite and each pot received 1 L water or 150 mM NaCl solution (ECe = 7.4 ds m−1). The pots were placed in a greenhouse room maintained at 25 ± 2°C and 50% relative humidity. Irrigation was set at 50 mL (+25 mL, if light intensity during the day was >1,000 lux) per pot per day during the vegetative stage and increased to 100 mL (+25 mL) during flowering and pod-filling stages and then reduced to 75 mL (+25 mL) during the harvest stage. The plants were given 1 g of water-soluble fertilizer in 1 L water (6-11-31, Hydroponic, Plant Prod, Canada) and 2 g of triple superphosphate per pot, at 2 weeks after seeding and then regularly at every growth stage after sampling the previous growth stage. The plants were sampled at mid-vegetative (~30 DAP), mid-flowering (~60 DAP), mid-pod-filling (~90 DAP) and harvest (~110 DAP) stages, and growth variables were measured (Supplementary Figures 4 and 5). The experiment was repeated twice with 12 replications for each treatment. Dried tissue samples were ground for elemental analysis, measured as mg g−1 dry weight of the plant tissue. N and P were measured on a flow injection analyzer (FIA) (Lachat QuickChem 8000, Hach® USA) and K, Ca and Na were measured after dilutions and appropriate modifier addition on an atomic absorption spectrophotometer (AAS) (Varian 220FS). Seed composition was analyzed at SGS Agrifood laboratories, SGS Canada Inc., Guelph, Canada. Nodules were collected from soybean plants after harvest; bacteria were grown on YEM agar plates similar to the isolation procedure described above and colonies were observed after 48 h of incubation.
Data were analyzed using a generalized linear mixed model that was performed using the GLIMMIX procedure in SAS (v 9.4, SAS Inc., Cary, NC). The SAS PROC GLIMMIX models were “mixed” due to the inclusion of fixed (treatment, salinity, and treatment × salinity) and random (RANDOM Rep) effects. The normal distribution was not assumed for the response (i.e., the observed variables) and therefore the models were “generalized.” Distributions were specified using the “DIST =” option in the MODEL statement and selected from the exponential family of distributions based on model fit statistics, that is, the Bayesian Information Criterion (BIC) that is part of the PROC GLIMMIX output (IC = Q was specified in the PROC GLIMMIX statement). Variance homogeneity was not assumed, and the structure of variance heterogeneity was specified using a “RANDOM _RESIDUAL_/GROUP =” statement and selected based on the BIC. Multiple comparisons were adjusted according to Scheffe's method (i.e., the ADJUST = SCHEFFE option in the LSMEANS statement). Effect slice tables were produced using SLICE and SLICEDIFF in the LSMEANS statement. The data for soybean growth and yield were broken into subsets based on the observed stages of plant development, and data from the respective subsets were analyzed separately using PROC GLIMMIX.
Bacterial colonies from A. bracteata nodules were obtained from 10−3, 10−4, and 10−5 dilutions. Since Bradyrhizobium takes nearly a week to grow on YEM plates, colonies that grew on the agar were selected after 24 to 96 h incubations. These non-Bradyrhizobium colonies of endophytic bacteria were re-isolated and 15 such pure cultures were grown and maintained on Kings B and YEM. One of the strains, SL45, was difficult to culture further and not used in downstream experiments. Of these putative PGPR isolates, there were at least 10 morphologically distinct colonies and they were arbitrarily labeled for identification purposes.
The bacteria identified using partial sequencing of the 16S rRNA gene showed the presence of diverse genera thriving in the nodules of A. bracteata (Supplementary Table 2) that could be isolated successfully. Five strains were belonging to Pseudomonas, two belonging to each of the genera Hydrogenophaga and Variovorax. The isolates SL36 and SL53 could not be identified using Sanger sequencing because of poor quality PCR product. One Rhizobium species was isolated, which is presumed to be one of the associated symbionts of A. bracteata for biological nitrogen fixation. A neighbor-joining phylogenetic tree file was constructed using the 16S rRNA gene sequences (Figure 1). The phylogenetic tree revealed that Hydrogenophaga and Variovorax genera are in the same cluster whereas the Bacillus subtilis, Gemmobacter sp., Flavobacterium sp., Rhizobium sp., and Devosia sp., are in the subsequent nodes of divergence, distant from one another.
Figure 1. Phylogenetic relationships between 14 bacterial strains isolated from the nodules of A. bracteata based on the 16S rRNA gene sequences. Phylogram was generated using iTOL based on the tree file from CLUSTALW2. Values on the lines indicate branch length from the node (tree: Newick/PHYLIP; kimura—false; tossgaps—off; Clustering—Neighbor joining; percent identity matrix—false).
The isolates differed from each other in their growth patterns when grown under salt conditions (Supplementary Figure 6). There was a gradual decrease in growth of strain SL31 with increasing salt concentrations, but it still reached ~1 OD in 500 mM NaCl at 48 h, which is the highest level of growth among all the isolates. Steady growth was observed in strain SL42 up to 250 mM NaCl, but growth was almost negligible at 500 mM NaCl. The salt concentration of 100 mM NaCl increased the growth of strains SL47 and SL48 when compared to 0 mM NaCl, but growth decreased at higher salt concentrations. Growth declined for strain SL52 but progressed for strain SL53 with increasing salt concentrations. Growth was either reduced or inhibited under salt for the other isolates and markedly lower than the isolates mentioned above. Many of the isolates exhibited PGPR characteristics of ACC deaminase and IAA production (Table 1 and Supplementary Figure 7). Rhizobium sp. SL42 and Hydrogenophaga sp. SL48 showed a strong affinity for nitrogen fixation, ACC deaminase activity and biofilm formation. Moreover, Hydrogenophaga sp. SL48 also exhibited profuse IAA synthesis from L-tryptophan.
Seed germination was counted when radicle emergence was observed (Supplementary Figure 8). There were significant differences between optimal and salt stress conditions at various time points and also among treatments (P = 0.002). Under optimal conditions, inoculation with strains SL43, SL47, SL48, and SL49 had significantly increased (P = 0.0001) germination rates at 36 and 48 h (~80 %) compared to the control treatment (60%). Under 100 mM NaCl, the germination rate was negligible at 24 h with 0% for control treatment and <5% for the isolates. Germination rate at 36 h was higher (P = 0.004) for the treatments SL42, SL47, SL48, SL49, and SL53 (~40%) than the control (30%). There was a greater increase (P = 0.0031) in germination rate at 48 h for treatments SL42 and SL48 (65%) than the control (40%) (Figure 2) and treatments with other isolates SL36, SL43, SL47, SL49, SL52, SL53, and SL55 were also higher (50–55%) (Supplementary Figure 9).
Figure 2. Seed germination rate of soybean at 24, 36, and 48 h under (A) optimal (water) and (B) salt (100 mM NaCl) conditions. The seeds were treated with 10 mM MgSO4 as control or bacterized with strains SL42 and SL48. Values represent mean ± SE (n = 6[10]). Significant differences (increase) between the bacterial treatments and the respective control treatments (optimal or salt) are indicated by asterisk above the data points, *p ≤ 0.05 (α = 0.05).
Seedling emergence under optimal conditions was not significantly higher for treatments when compared to the control (Supplementary Figure 10). However, under salt stress of 100 mM NaCl emergence rate was significantly increased (P = 0.0002) for all but SL31 of the bacterial treatments at 8th DAP. Growth variables of soybean plants were measured at 28th DAP (Supplementary Figure 11). Significant increases (P < 0.0001) were observed in plant height for treatments SL42, SL43, SL47, SL48, and SL49 compared to the control under optimal conditions. Treatments with SL47 and SL48 showed significant increases (P < 0.0001) under salt stress and slight increases in plant height were also observed for treatments SL42 and SL49. Leaf area was significantly higher (P < 0.0001) for treatments with SL42, SL47, SL48, and SL49 than the control under both optimal and salt stress conditions. A parallel outcome was observed in shoot biomass, with treatments SL42, SL43, SL47, SL49, and SL50 showing significant increases (P < 0.0001) under optimal conditions and treatments SL42, SL44, SL47, SL48, SL49, and SL55 showing significant increases (P < 0.0001) under salt stress. Root dry weight was significantly higher for treatments SL36 and SL43 under optimal conditions (P < 0.0001) and for the treatments SL33, SL36, SL43, SL50, SL55, and SL56 under salt stress (P = 0.0004). However, root volume was significantly increased (P = 0.0003) for treatments SL31, SL33, SL36, SL42, SL48, SL49, SL50, SL55, and SL56 compared to the control treatment under salt stress. Results of the most beneficial strains, SL42 and SL48 are shown in Figure 3. Yet only treatments SL42 and SL50 showed significant increases (P = 0.04) in root length, and SL31 and SL42 showed significant increases (P = 0.01) in root surface area under salt stress (Supplementary Figure 12).
Figure 3. Growth variables of soybean, (A) Plant height, (B) Leaf area index, (C) Shoot dry weight, (D) Root dry weight, (E) Root volume, and (F) Root length measured at 28th DAP under optimal (water) and salt (100 mM NaCl) conditions. The seeds were treated with 10 mM MgSO4 as control or bacterized with strains SL42 and SL48. Values represent mean ± SE (n = 6). Significant differences (increase) between the bacterial treatments and the respective control treatments (optimal or salt) are indicated by asterisk above the data points, *p ≤ 0.05, **p ≤ 0.001, ***p ≤ 0.0001 (α = 0.05).
Based on the results of the first screening, four isolates Rhizobium sp. SL42, Hydrogenophaga sp. SL48, Pseudomonas borealis SL49, and Variovorax sp. SL55 were selected for the next trial. Seed germination of soybean was differentially affected under a range of salt concentrations (Figure 4). All bacterial treatments resulted in increases over the control treatment. The germination rate was 65% for SL42, 80% for SL48, 60% for SL49, and 70% for SL55 at 0 mM NaCl after 72 h compared to the 45% germination rate in control treatment. At 100 and 125 mM NaCl, the germination rate was around 60% for the bacterial treatments and 42% for the control treatment. The germination rate at 1 × 108 cfu mL−1 was 58% for SL42, 55% for SL48, 60% for SL49, and 43% for SL55 at 150 mM, while the control reached about 40%. The germination rate was considerably lower at higher salt concentrations of 175 and 200 mM NaCl. For SL55, no significant increase in germination rate was observed except at 175 mM NaCl (Supplementary Figures 13 and 14). The germination rates for the two inoculums, 1 × 108 and 1 × 1010 cfu mL−1 were mostly parallel to each other but slight variations were present in a few cases and 1 × 108 cfu mL−1 was selected as the inoculum density for successive experiments.
Figure 4. Seed germination of soybean at 72 h under increasing salt concentrations (0, 100, 150, and 200 mM NaCl). The seeds were treated with (A) 10 mM MgSO4 as control or bacterized with strains (B) SL42 and (C) SL48.
Seedling emergence rate at 8th DAP was significantly higher for the treatment SL42 at all the salt concentrations from 100 (P = 0.0326) to 200 mM (P = 0.0153) NaCl than the control treatment. It was also increased by treatments SL48, SL49, and SL55 at different salt levels but the statistical significance varied. Growth variables of soybean treated with the four isolates, grown under a range of salt concentrations were measured at 28th DAP (Supplementary Figure 15) and plant growth was greatly reduced at 175 and 200 mM NaCl. Leaf area was significantly increased by the treatments with SL48 at 0, 125, and 150 mM NaCl (P = 0.01) and SL42 at 100 (P = 0.022) and 125 mM NaCl compared to control treatments. This corresponded to the increase in shoot biomass, which was significantly higher than the control for treatments SL42 at 125, 150 (P = 0.0016), 175, and 200 mM NaCl and SL48 at 150 (P < 0.0001) and 175 mM NaCl. Shoot dry weight was improved by SL48 and SL42 at other NaCl concentrations as well, albeit not significantly. Treatment with SL49 has significantly increased shoot biomass at 150 (P = 0.02) and 175 mM NaCl. Root dry weight was significantly higher at 0 mM NaCl for the treatments SL48 (P = 0.001) and SL49 and at 150 mM for the treatments SL42 (P = 0.0015), SL48 (P = 0.0011), and SL55 (P = 0.002). The root dry weight was also increased by the bacterial treatments at higher salt concentrations (175 and 200 mM NaCl). The results indicated that the strains SL42 and SL48 have greatly improved soybean growth under a range of salt stress conditions (Figure 5). Though salt stress of 100-150 mM NaCl had significant differences between the control and bacterial treatments, 150 mM NaCl provided a much clearer distinction related to salinity stress in the shoot (P = 0.0004) and root biomass (P = 0.0036).
Figure 5. Growth variables of soybean, (A) Seedling emergence rate measured on 8th DAP and growth variables of soybean (B) Leaf area, (C) Shoot dry weight, and (D) Root dry weight measured at 28th DAP under increasing salt concentrations (0, 100, 125, 150, 175, and 200 mM NaCl). The seeds were treated with 10 mM MgSO4 as control or bacterized with strains SL42 and SL48. Values represent mean ± SE (n = 6). Significant differences (increase) between the bacterial treatments and the respective control treatments of a particular salt concentration are indicated by asterisk above the data points, *p ≤ 0.05, **p ≤ 0.001, ***p ≤ 0.0001 (α = 0.05).
Two of the bacterial isolates, Rhizobium sp. SL42 and Hydrogenophaga sp. SL48 were co-inoculated with Bradyrhizobium japonicum 532C and the soybean plants were grown under optimal or 150 mM NaCl conditions. Inoculation with B. japonicum alone served as the control as it is the standard N2-fixing symbiont of soybean. Even though growth variables under optimal conditions were insignificantly different for plants inoculated with B. japonicum because of the uninhibited nitrogen fixation, co-inoculation with SL42 and SL48 enhanced plant growth in most cases. However, under salt stress, there were substantial differences between the co-inoculation treatments and the B. japonicum control and the co-inoculation treatments resulted in higher growth than the control in all respects.
During the vegetative and flowering stages, the growth variables were all significantly different (P < 0.0001) between the optimal and salt stress conditions. At the vegetative stage, plant height (P = 0.0001), shoot biomass (P = 0.2764), and root dry weight (P = 0.0935) were increased by the B. japonicum+SL42 treatment compared to the control, B. japonicum (Bj) under salt stress. Shoot biomass was also increased by the B. japonicum+SL42+SL48 treatment under both optimal and salt stress conditions. During the flowering stage, plant height (P = 0.1934), leaf area (P = 0.1562) (Figure 7), shoot biomass (P = 0.0872), and root dry weight (P = 0.1766) were all higher for the B. japonicum+SL42+SL48 treatment, and also for the other co-inoculation treatments than the B. japonicum control under salt stress. The treatment of B. japonicum+SL42+SL48 was also the highest under optimal conditions (except for plant height), although the difference was more notable under salt stress. At the pod-filling stage, shoot biomass was increased by the treatments of B. japonicum+SL42 (P = 0.1001) and B. japonicum+SL42+SL48 (P = 0.3866), as was the case with other growth variables, compared to the B. japonicum control under salt stress. The treatment of B. japonicum+SL42 also improved plant growth under optimal conditions (except for leaf area index). Plant height (Figure 6) and leaf area (Figure 7) of soybean increased exponentially up to the pod-filling stage and vegetative growth was stationary as the plants reached maturity. During the harvest stage, the shoot biomass was considerably reduced due to the senescence of leaves and not much of a difference among the treatments were observed. Overall, the co-inoculation treatments have resulted in increased shoot dry weight by 1.6 and 18.3% at vegetative, 11.9 and 27% at flowering, 7.1 and 7.5% at pod-filling, 7.5 and 4.6% at harvest under optimal and salt stress conditions, respectively (Figure 8). The root dry weight under salt stress was particularly increased by the treatment of B. japonicum+SL42, by 28% at vegetative, 16% at flowering, 9% at pod-filling, and 24.5% at harvest stages (Figure 9).
Figure 6. Height of soybean plants measured at (A) mid-vegetative, (B) mid-flowering, and (C) mid-pod-filling stages under optimal (water) and salt (150 mM NaCl) conditions. The seeds were bacterized with Bradyrhizobium japonicum (Bj), Bj+SL42, Bj+SL48 and Bj+SL42+SL48. Values represent mean ± SE (n=12). Scheffe grouping for least square means was used for multiple means comparison and means with the same letter(s) are not significantly different (α = 0.05).
Figure 7. Leaf area of soybean plants measured at (A) mid-vegetative, (B) mid-flowering, and (C) mid-pod-filling stages under optimal (water) and salt (150 mM NaCl) conditions. The seeds were bacterized with Bradyrhizobium japonicum (Bj), Bj+SL42, Bj+SL48 and Bj+SL42+SL48. Values represent mean ± SE (n = 12). Scheffe grouping for least square means was used for multiple means comparison and means with the same letter(s) are not significantly different (α = 0.05).
Figure 8. Shoot biomass of soybean plants measured at (A) mid-vegetative, (B) mid-flowering, (C) mid-pod-filling, and (D) harvest stages under optimal (water) and salt (150 mM NaCl) conditions. The seeds were bacterized with Bradyrhizobium japonicum (Bj), Bj+SL42, Bj+SL48, and Bj+SL42+SL48. Values represent mean ± SE (n = 12). Scheffe grouping for least square means was used for multiple means comparison and means with the same letter(s) are not significantly different (α = 0.05).
Figure 9. Root dry weight of soybean plants measured at (A) mid-vegetative, (B) mid-flowering, (C) mid-pod-filling, and (D) harvest stages under optimal (water) and salt (150 mM NaCl) conditions. The seeds were bacterized with Bradyrhizobium japonicum (Bj), Bj+SL42, Bj+SL48, and Bj+SL42+SL48. Values represent mean ± SE (n = 12). Scheffe grouping for least square means was used for multiple means comparison and means with the same letter(s) are not significantly different (α = 0.05).
The yield variables, seed weight and seed number were increased by all three co-inoculation treatments compared to B. japonicum alone under both optimal and salt stress conditions (Figure 10). The treatment of B. japonicum+SL42 increased seed weight by 4.3% (P = 0.7207) and seed number by 10.5% (P = 0.2788) under salt stress. The other treatments, B. japonicum+SL48 and B. japonicum+SL42+SL48 increased seed weight by 7.4% (P = 0.449) and 8.1% (P = 0.3347), under optimal conditions and 3.6% (P = 0.7145) and 3.1% (P = 0.8686) under salt stress, respectively. Even though seed weight and seed number were less in salt stress than the optimal conditions, the difference between the corresponding treatments was small. The harvest index is the proportion of seed dry weight to the aboveground biomass and the treatments with B. japonicum+SL48 and B. japonicum+SL42+SL48 had higher harvest indices (P = 0.1621) than that of the treatments with B. japonicum and B. japonicum+SL42, under both optimal and salt stress conditions.
Figure 10. Yield variables of soybean plants measured after harvest (A) seed weight, (B) seed number, and (C) harvest index under optimal (water) and salt (150 mM NaCl) conditions. The seeds were bacterized with Bradyrhizobium japonicum (Bj), Bj+SL42, Bj+SL48, and Bj+SL42+SL48. Values represent mean ± SE (n = 12). Scheffe grouping for least square means was used for multiple means comparison and means with the same letter(s) are not significantly different (α = 0.05).
The nutrient analysis provided an interesting perspective on how the nutrients were translocated between various plant tissues throughout the developmental stages. Nitrogen concentration was largely unvarying in the vegetative and flowering stages, except that shoot N concentration was greater than that of roots. No significant difference was observed in nitrogen concentration between the treatments since all of them were inoculated with B. japonicum. At pod-filling, N concentration under salt stress was lower than optimal in leaves, shoot, and roots but almost equal in the pods and at harvest, it was less in the shoot and pods but more or less equal in the roots (Supplementary Table 3). This is reflected in the seed quality where the protein concentration was lower under salt stress (34%) than optimal (37%) conditions. Nitrogen assimilation was calculated as a ratio of total N concentration in the tissues to the dry weight, and it was significantly reduced (P < 0.0001) with the salt stress at all the developmental stages. At the vegetative stage, N assimilation did not vary among treatments and was corresponding to the amount of fertilizer applied under optimal conditions (60 mg per plant) since the nodules will be still developing at this stage and not fully functional yet. As the plants developed, biological nitrogen fixation was actively occurring, evident by the high N assimilation. During the flowering stage, treatment of B. japonicum+SL42+SL48 had increased N assimilation under optimal and salt conditions. At the pod-filling stage, inoculation with B. japonicum+SL42 showed higher N assimilation under optimal conditions, yet the treatment of B. japonicum+SL42+SL48 resulted in the highest N assimilation under salt stress. At the harvest stage, N assimilation was relatively higher for the B. japonicum+SL42 treatment under both optimal and salt-stressed conditions (Table 2).
Table 2. Total Nitrogen assimilation in shoot and root tissues of soybean through the developmental stages.
Phosphorous concentration was higher under salt stress than optimal conditions at the flowering and pod-filling stages, but more or less equal at the vegetative and harvest stages (Supplementary Table 4). The treatment of B. japonicum+SL42+SL48 had the lowest P concentration in the shoot and pods at the harvest stage but highest in the seeds under salt stress. Potassium concentration under salt stress was lower and sodium concentration was higher than optimal conditions at the vegetative stage (Supplementary Tables 5, 6). The plants at this stage had higher salt accumulation relative to their biomass and hence, the K+/Na+ ratio (Table 3) was lower. The treatment of B. japonicum+SL42 had higher K concentration and lower Na concentration in the shoot than the B. japonicum control, and so, the shoot K+/Na+ ratio was significantly higher (P = 0.006) under salt stress. Potassium concentration under salt stress was higher in the leaves, shoot, and pods but lower in the roots at flowering, pod-filling, and harvest stages than optimal conditions. Sodium concentration was relatively higher under salt stress and much of the Na was accumulated in the roots, as compared to the shoot and leaves. This explains the low K+/Na+ ratio in roots versus the high K+/Na+ ratio in the shoot and leaves. The treatment of B. japonicum+SL48 had lower Na concentration in the leaves and significantly higher (P < 0.0001) K+/Na+ ratio than the B. japonicum control under salt stress at the flowering stage. The K+/Na+ ratio was significantly increased (P < 0.01) by the B. japonicum+SL42 treatment under salt stress in the leaves during the pod-filling stage. Treatment with B. japonicum+SL48 increased K concentration and decreased Na concentration in the shoot and pods, which resulted in higher K+/Na+ ratios at the harvest stage. Calcium concentration in the shoot and roots were relatively higher under salt stress than optimal conditions at the flowering, pod-filling and harvest stages, indicating that the plants were also utilizing Ca2+ to maintain ionic balance under salinity stress (Supplementary Table 7).
Table 3. Distribution of K +/Na + in different plant tissues through the developmental stages of soybean.
Seed nutritional composition analysis showed that the moisture, protein, and fiber concentrations decreased, and fat, ash, and potassium concentrations increased under salt stress conditions. Sodium concentration was also significantly higher under salt stress (P = 0.0079). The treatment of B. japonicum+SL42+SL48 had the highest protein, fiber, phosphorus, potassium, calcium, sodium, and magnesium concentrations and the lowest moisture, fat, and ash concentrations under salt stress (Supplementary Table 8). Diversity in the nodule bacteria of soybean was observed at 10−4 and 10−5 dilutions and the colonies were disparate between optimal and salt-stressed plants (Supplementary Figure 16). Colonies similar to the morphology of SL42 and SL48 were prominent in the co-inoculation treatments, specifically, the B. japonicum+SL42 treatment had predominantly SL42 colonies under salt stress. Also, the bacterial population was higher in the B. japonicum+SL42+SL48 treatment than the control, B. japonicum.
Rhizobia and legumes have established their mutualistic association over 100 million years of coevolution and the association between different lineages of both the rhizobia and the legume has diverged to be species-specific and spatially-specific (Parker, 1999). This mutualistic specificity also holds true for the host relationship with other members of the phytomicrobiome, including other bacteria in the nodules. The nodules of Amphicarpaea bracteata have endophytic bacteria other than its Bardyrhizobium symbiont. The vastness in the diversity of these bacteria suggests that they might be effectively functioning as plant growth promoting rhizobacteria (PGPR) in their host. The tested isolates do not coexist with B. japonicum in nature (or at least not known yet) and in this study, they were introduced to a related host intended to exert beneficial effects. Co-inoculation of PGPR with rhizobia was reported in various legume plants and proposed to be used as inoculants (Bai et al., 2002a).
Seed germination is the initiation of plant growth and favorable conditions are necessary for successful germination and subsequent seedling emergence. The rate of germination and the time to seedling emergence are important in terms of crop establishment at the beginning of the growing season. Seedling emergence and younger seedlings are more prone to risk from salinity since root development occurs in the topsoil, which generally has higher accumulation of soluble salts (Almansouri et al., 2001). Salt was pre-applied to vermiculite before planting the soybean seeds in the greenhouse, so as to mimic the salinity stress under field conditions where salt is already present in the topsoil and the seeds have to undergo the process of germination and development in the presence of salt. The seedling stage of the soybean plant is considered to be more sensitive than seed germination (Hosseini et al., 2002) and that is why the effect of salinity stress was acute and precise in the screening experiments where the plants were grown up to the mid-vegetative stage. Salinity stress inhibited seed germination, affected seedling growth, reduced biomass accumulation and decreased seed weight of soybean (Essa, 2002). The plants exhibited symptoms of salinity stress, the seedling emergence was slower, and the growth was less compared to the optimal conditions. The mechanisms underlying the inhibition of soybean seed germination and growth by salinity stress are only partially understood (Zhang et al., 2014). Salt stress leads to the up-regulation of ABA and ethylene biosynthesis and down-regulation of GA during seed germination and auxin and cytokinin during plant growth (Shu et al., 2017). The PGPR are reported to modulate phytohormone signaling involved in salinity stress; a rhizobacterium Sphingomonas sp. LK11, known to secrete phytohormones (auxins and gibberellins) and trehalose had significantly increased plant growth under drought-induced osmotic stress in soybean (Asaf et al., 2017). Another rhizobacterium, Arthrobacter woluwensis AK1 was shown to ameliorate salinity stress by decreasing ABA content, regulating antioxidant activities and salt tolerance genes and reduced sodium uptake in soybean (Khan et al., 2019). Several isolates, including SL42, SL48, and SL49 have significantly improved seed germination and shoot biomass under salt stress and similar results were observed in the consecutive greenhouse trial as well. The isolates also produced IAA and ACC deaminase, which at least partly explains the observed plant growth promotion and stress tolerance.
Since the bacteria were isolated from the nodules of A. bracteata that has Bradyrhizobium sp. as the symbiont, they have co-existed in the nodules. Hence, the behavior of nodule bacteria was speculated to be potentially complementary when co-inoculated with a related symbiont in soybean. The plants were grown up to the harvest stage and samples were collected at every growth stage to discern the effect of the isolates on the salinity response of soybean. Soybean has varying water requirements throughout its growing season and rapid root and shoot growth are noted from mid-vegetative to mid-pod-filling stages when the water requirements are also the highest. Though the plant is moderately tolerant and able to withstand short periods of drought and salinity stress, they affect development and crop yield and the plant is most susceptible to the stressors during the vegetative and flowering stages (FAO, 2002). Shoot dry weight of soybean was more affected by salt stress than root dry weight, as reported previously (Essa, 2002) and above-ground plant growth was significantly reduced (P < 0.0001) by salinity during the vegetative and flowering stages when the plant was suffering from chronic salt stress. At the later development stages (pod-filling and harvest) this difference was seldom noticed because the plants would have developed tolerance mechanisms and acclimatized to the stress with time (Munns and Tester, 2008). The degree of salt tolerance in soybean differs among developmental stages (Phang et al., 2008). The plant is sensitive to salinity at early growth stages, but this doesn't necessarily mean it will also be sensitive at later growth stages. The results would probably vary if the plants were exposed to another surge of salinity stress at the later growth stages. For soybean, both flowering and pod-filling stages are responsive to water availability and significant yield loss occurred when the plants were exposed to drought at these developmental stages (Westgate and Peterson, 1993). Soluble salts are usually localized in the sub-surface layers and the concentration of these salts reduces water availability and the roots may be exposed to salt-contaminated soil water table (Rengasamy, 2002). Nevertheless, it is interesting to note that the salt stress is contained in a closed system in the greenhouse and salt volume was applied proportionately to the field capacity of the pot volume. Under field conditions, the intensity of the stress fluctuates depending on other environmental factors such as precipitation or evapotranspiration.
The plants were supplied with a low N fertilizer and the nitrogen fixation was predominantly carried out by Bradyrhizobium japonicum. The decrease in nitrogen accumulation under salinity stress was due to the inhibition of root nodulation and biological nitrogen fixation. The number of root nodules and root hair curling were constrained by salt stress (Tu, 1981). The N content of the pods dramatically decreased from the pod-filling to harvest stages indicating the translocation of N to the pods and then to the seeds. The protein content of the seeds was reduced under salinity stress whereas, the oil content was increased. Despite the decline in photosynthesis, translocation of assimilates to the sink tissues were largely maintained in soybean under drought stress (Huber et al., 1984). Phytohormone signaling coordinates partitioning of the assimilates between source and sink, and thereby maintains growth, development and function (Perez-Alfocea et al., 2010). The co-inoculation treatments resulted in higher seed weight and seed number than the control under salt stress and allowed the plants to at least partially overcome the effects of stress on reproduction. The isolates might regulate signaling events in the plants during the initial osmotic phase but later shift towards balancing ionic stress under salinity. Potassium is a key nutrient in maintaining ion homeostasis under salinity and the ratio of K+ to Na+ is determined by the rate of K+ assimilation. The high cytosolic K+/Na+ ratio is critical for plant salinity tolerance and the function of K+ transporters is regulated by osmolytes and calcium signaling. Ionic homeostasis is maintained by excluding Na+ and Cl− and restricting their accumulation in plant tissues and compartmentalizing the toxic ions in vacuoles (Shabala and Cuin, 2008). The ability of the plants treated with isolates SL42 and SL48 to maintain a high K+/Na+ ratio through various growth stages is indeed an indication of induced salinity tolerance. Follow-up studies are in progress to understand the mode of action of the isolates and the adaptive mechanisms elicited in the plants. The primary reason for using vermiculite as the sole potting medium is that it is inert, ruling out the possibility of interference by organic matter (including microflora) usually present in the soil or peat-based potting medium. This has proved to be an effective testing tool for salt stress mitigation by the bacterial inoculation and plant nutrient uptake from the added fertilizer. The observations of nodule bacterial colonies indicated that SL42 and SL48 predominantly inhabited the nodules of soybean and also supported the resident nodule phytomicrobiome population. Indigenous microbial communities influence the survival of inoculated bacteria and vice-versa (Trabelsi and Mhamdi, 2013). However, the tested strains are a part of the native habitat, so that the potential concern for altering the ecosystem function of soil microbial communities is diminished. They have a competitive advantage over the resident soil microbiota since they provide a synergistic plant-microbe interaction with soybean. Considering that many other factors are at play under a field condition, extensive field trials are needed to determine the beneficial effects of these microbes on soybean growth and yield in local agriculture production systems.
Soybean cultivation has reached its northern hemisphere limit and expansion/extension of cultivation both spatially and temporally will be possible when the plants can further acclimatize to the native conditions. Co-inoculation with native nodule bacterial strains can help in the adaptation and expression of particular traits such as salt/drought tolerance or cold acclimatization induced by the bacteria can benefit the associated plant. Early plant response mechanisms to these stresses overlap each other, which means inoculation with these bacteria can be an asset to sustainable soybean production under the Canadian agricultural scenario. Moreover, such growth promoting technology as this might invigorate native soil properties (both abiotic and biotic), create synergy with the native soil microflora, assist in the reduction of chemical inputs and advance crop productivity. However, multiple field trials are required to demonstrate the potential of these isolates to boost yield by growth promotion and stress alleviation. Even though adaptation to salinity stress depends on various factors including the plant's innate potential and the environmental conditions, implementing a cost-effective strategy of PGPR inoculation to enhance stress tolerance will be fruitful and helpful to meet the rising demands for global food production.
The datasets presented in this study can be found in online repositories. The names of the repository/repositories and accession number(s) can be found in the article/Supplementary Material.
GI conducted the research, collected data, and prepared the manuscript. TS helped GI for statistical analysis of the data. DS helped GI in editing the manuscript and providing feedback. All authors contributed to the article and approved the submitted version.
Publication of this article has been made possible by the Biomass Canada Cluster. The Biomass Canada Cluster is managed by BioFuelNet Canada and was funded through the Canadian Agricultural Partnership's, AgriScience Program, Agriculture and Agri-Food, Canada.
The authors declare that the research was conducted in the absence of any commercial or financial relationships that could be construed as a potential conflict of interest.
We sincerely thank the members of Smith lab, especially Dr. Sowmyalakshmi Subramanian, Dr. Selvakumari Arunachalam, Dr. Alfred Souleimanov, and Dr. Yoko Takishita for their valuable inputs, and the summer students who helped with experimental setup and sample collection. We are grateful for the support given by Mr. Guy Rimmer, Mr. Ian Ritchie, Ms. Melissa LaRiviere, and Mr. Drew Anthony of the Department of Plant sciences and Ms. Hélène Lalande, Environmental Chemistry Lab, McGill university, Canada. We greatly appreciate the services provided by Genome Québec, Montreal, Canada and SGS Canada Inc., Guelph, Canada.
The Supplementary Material for this article can be found online at: https://www.frontiersin.org/articles/10.3389/fsufs.2020.617978/full#supplementary-material
AAFC (2020). Soil Salinization Indicator. Agriculture and Agri-Food Canada. Available online at: https://www.agr.gc.ca/eng/agriculture-and-climate/agricultural-practices/soil-and-land/soil-salinization-indicator/?id=1462912470880 (accessed April 12, 2020).
Adesemoye, A. O., and Kloepper, J. W. (2009). Plant-microbes interactions in enhanced fertilizer-use efficiency. Appl. Microbiol. Biotechnol. 85, 1–12. doi: 10.1007/s00253-009-2196-0
Almansouri, M., Kinet, J. M., and Lutts, S. (2001). Effect of salt and osmotic stresses on germination in durum wheat (Triticum durum Desf.). Plant Soil 231, 243–254. doi: 10.1023/A:1010378409663
Asaf, S., Khan, A. L., Khan, M. A., Imran, Q. M., Yun, B. W., and Lee, I. J. (2017). Osmoprotective functions conferred to soybean plants via inoculation with Sphingomonas sp LK11 and exogenous trehalose. Microbiol. Res. 205, 135–145. doi: 10.1016/j.micres.2017.08.009
Ashraf, M. (1994). Breeding for salinity tolerance in plants. Crit. Rev. Plant Sci. 13, 17–42. doi: 10.1080/07352689409701906
Bai, Y. M., D'Aoust, F., Smith, D. L., and Driscoll, B. T. (2002a). Isolation of plant-growth-promoting Bacillus strains from soybean root nodules. Can. J. Microbiol. 48, 230–238. doi: 10.1139/w02-014
Bai, Y. M., Pan, B., Charles, T. C., and Smith, D. L. (2002b). Co-inoculation dose and root zone temperature for plant growth promoting rhizobacteria on soybean [Glycine max (L.) Merr] grown in soil-less media. Soil Biol. Biochem. 34, 1953–1957. doi: 10.1016/S0038-0717(02)00173-6
Bric, J. M., Bostock, R. M., and Silverstone, S. E. (1991). Rapid in situ assay for indoleacetic-acid production by bacteria immobilized on a nitrocellulose membrane. Appl. Environ. Microbiol. 57, 535–538. doi: 10.1128/AEM.57.2.535-538.1991
Bromfield, E. S. P., Cloutier, S., Tambong, J. T., and Thi, T. V. T. (2017). Soybeans inoculated with root zone soils of Canadian native legumes harbour diverse and novel Bradyrhizobium spp. that possess agricultural potential (vol 40, pg 440, 2017). Syst. Appl. Microbiol. 40:517. doi: 10.1016/j.syapm.2017.10.003
Cloutier, J. (2017). Soy Story: A Short History of Glycine max in Canada. Statistics Canada. Available online at: https://www150.statcan.gc.ca/n1/pub/21-004-x/2017001/article/14779-eng.htm (accessed April 12, 2020).
Devine, T. E., Kuykendall, L. D., and Oneill, J. J. (1990). The Rj4 allele in soybean represses nodulation by chlorosis-inducing bradyrhizobia classified as DNA homology group-ii by antibiotic-resistance profiles. Theor. Appl. Genet. 80, 33–37. doi: 10.1007/BF00224012
Dorff, E. (2007). The Soybean, Agriculture's Jack-of-all-Trades, Is Gaining Ground Across Canada. Statistics Canada. Available online at: https://www150.statcan.gc.ca/n1/en/pub/96-325-x/2007000/article/10369-eng.pdf?st=6wd-Sqjq (accessed April 12, 2020).
Egamberdieva, D., Wirth, S., Jabborova, D., Rasanen, L. A., and Liao, H. (2017). Coordination between Bradyrhizobium and Pseudomonas alleviates salt stress in soybean through altering root system architecture. J. Plant Interact. 12, 100–107. doi: 10.1080/17429145.2017.1294212
EnvironmentCanada (2001). Priority Substances List Assessment Report for Road Salts. EnvironmentCanada. Available online at: https://www.canada.ca/en/health-canada/services/environmental-workplace-health/reports-publications/environmental-contaminants/canadian-environmental-protection-act-1999-priority-substances-list-assessment-report-road-salts.html#a342 (accessed April 12, 2020).
Essa, T. A. (2002). Effect of salinity stress on growth and nutrient composition of three soybean (Glycine max L. Merrill) cultivars. J. Agron. Crop Sci. 188, 86–93. doi: 10.1046/j.1439-037X.2002.00537.x
FAO (2002). Soybean. Food and Agriculture Organization of the United Nations. Available online at: http://www.fao.org/land-water/databases-and-software/crop-information/soybean/en/ (accessed April 12, 2020).
Florinsky, I. V., Eilers, R. G., Wiebe, B. H., and Fitzgerald, M. M. (2009). Dynamics of soil salinity in the Canadian prairies: application of singular spectrum analysis. Environ. Model Softw. 24, 1182–1195. doi: 10.1016/j.envsoft.2009.03.011
Goswami, D., Dhandhukia, P., Patel, P., and Thakker, J. N. (2014). Screening of PGPR from saline desert of Kutch: growth promotion in Arachis hypogea by Bacillus licheniformis A2. Microbiol. Res. 169, 66–75. doi: 10.1016/j.micres.2013.07.004
Hosseini, M. K., Powell, A. A., and Bingham, I. J. (2002). Comparison of the seed germination and early seedling growth of soybean in saline conditions. Seed Sci. Res. 12, 165–172. doi: 10.1079/SSR2002108
Huber, S. C., Rogers, H. H., and Mowry, F. L. (1984). Effects of water-stress on photosynthesis and carbon partitioning in soybean (Glycine-max [L] Merr) plants grown in the field at different Co2 levels. Plant Physiol. 76, 244–249. doi: 10.1104/pp.76.1.244
Hungria, M., Nogueira, M. A., and Araujo, R. S. (2013). Co-inoculation of soybeans and common beans with rhizobia and azospirilla: strategies to improve sustainability. Biol. Fertil. Soils 49, 791–801. doi: 10.1007/s00374-012-0771-5
Hynes, R. K., Leung, G. C., Hirkala, D. L., and Nelson, L. M. (2008). Isolation, selection, and characterization of beneficial rhizobacteria from pea, lentil, and chickpea grown in western Canada. Can. J. Microbiol. 54, 248–258. doi: 10.1139/W08-008
Jensen, H. L., Petersen, E. J., De, P. K., and Bhattacharya, R. (1960). A new nitrogen-fixing bacterium - Derxia gummosa nov-gen-nov-spec. Arch. Mikrobiol. 36, 182–195. doi: 10.1007/BF00412286
Kan, G. Z., Zhang, W., Yang, W. M., Ma, D. Y., Zhang, D., Hao, D. R., et al. (2015). Association mapping of soybean seed germination under salt stress. Mol. Genet. Genomics 290, 2147–2162. doi: 10.1007/s00438-015-1066-y
Khan, M. A., Asaf, S., Khan, A. L., Jan, R., Kang, S. M., Kim, K. M., et al. (2019). Rhizobacteria AK1 remediates the toxic effects of salinity stress via regulation of endogenous phytohormones and gene expression in soybean. Biochem. J. 476, 2393–2409. doi: 10.1042/BCJ20190435
Letunic, I., and Bork, P. (2019). Interactive Tree Of Life (iTOL) v4: recent updates and new developments. Nucleic Acids Res. 47, W256–W259. doi: 10.1093/nar/gkz239
Marr, D. L., Devine, T. E., and Parker, M. A. (1997). Nodulation restrictive genotypes of Glycine and Amphicarpaea: a comparative analysis. Plant Soil 189, 181–188. doi: 10.1023/A:1004203018770
Munns, R., and Tester, M. (2008). Mechanisms of salinity tolerance. Annu. Rev. Plant Biol. 59, 651–681. doi: 10.1146/annurev.arplant.59.032607.092911
NRCS (2002). Salinity in Agriculture. Natural Resources Conservation Service, United States Department of Agriculture. Available online at: https://www.nrcs.usda.gov/wps/portal/nrcs/detailfull/national/water/quality/tr/?cid=nrcs143_010914 (accessed April 12, 2020).
O'Toole, G. A. (2011). Microtiter dish biofilm formation assay. J. Vis. Exp. 47:2437. doi: 10.3791/2437
Parker, M. A. (1994). Evolution in natural and experimental populations of Amphicarpaea-bracteata. J. Evol. Biol. 7, 567–579. doi: 10.1046/j.1420-9101.1994.7050567.x
Parker, M. A. (1999). Mutualism in metapopulations of legumes and rhizobia. Am. Nat. 153, S48–S60. doi: 10.1086/303211
Penrose, D. M., and Glick, B. R. (2003). Methods for isolating and characterizing ACC deaminase-containing plant growth-promoting rhizobacteria. Physiol. Plant 118, 10–15. doi: 10.1034/j.1399-3054.2003.00086.x
Perez-Alfocea, F., Albacete, A., Ghanem, M. E., and Dodd, I. C. (2010). Hormonal regulation of source-sink relations to maintain crop productivity under salinity: a case study of root-to-shoot signalling in tomato. Funct. Plant Biol. 37, 592–603. doi: 10.1071/FP10012
Phang, T. H., Shao, G. H., and Lam, H. M. (2008). Salt tolerance in soybean. J. Integr. Plant Biol. 50, 1196–1212. doi: 10.1111/j.1744-7909.2008.00760.x
Rengasamy, P. (2002). Transient salinity and subsoil constraints to dryland farming in Australian sodic soils: an overview. Aust. J. Exp. Agric. 42, 351–361. doi: 10.1071/EA01111
Schwyn, B., and Neilands, J. B. (1987). Universal chemical-assay for the detection and determination of siderophores. Anal. Biochem. 160, 47–56. doi: 10.1016/0003-2697(87)90612-9
Shabala, S., and Cuin, T. A. (2008). Potassium transport and plant salt tolerance. Physiol. Plant 133, 651–669. doi: 10.1111/j.1399-3054.2007.01008.x
Shu, K., Qi, Y., Chen, F., Meng, Y. J., Luo, X. F., Shuai, H. W., et al. (2017). Salt stress represses soybean seed germination by negatively regulating GA biosynthesis while positively mediating ABA biosynthesis. Front. Plant Sci. 8:1372. doi: 10.3389/fpls.2017.01372
Tang, J., Bromfield, E. S. P., Rodrigue, N., Cloutier, S., and Tambong, J. T. (2012). Microevolution of symbiotic Bradyrhizobium populations associated with soybeans in east North America. Ecol. Evol. 2, 2943–2961. doi: 10.1002/ece3.404
Thoenes, P. (2004). “The role of soybean in fighting world hunger,” in VIIth World Soybean Research Conference (Foz do Iguassu: Food and Agriculture Organization of the United Nations).
Trabelsi, D., and Mhamdi, R. (2013). Microbial inoculants and their impact on soil microbial communities: a review. Biomed Res. Int. 2013:863240. doi: 10.1155/2013/863240
Tu, J. C. (1981). Effect of salinity on rhizobium-root-hair interaction, nodulation and growth of soybean. Can. J. Plant Sci. 61, 231–239. doi: 10.4141/cjps81-035
Weaver, R. W., Dumenil, L. C., and Frederic, LR (1972). Effect of soybean cropping and soil properties on numbers of Rhizobium-japonicum in Iowa soils. Soil Sci. 114:137. doi: 10.1097/00010694-197208000-00009
Westgate, M. E., and Peterson, C. M. (1993). Flower and pod development in water-deficient soybeans (Glycine-max L Merr). J. Exp. Bot. 44, 109–117. doi: 10.1093/jxb/44.1.109
Wiebe, B. H., Eilers, R. G., Eilers, W. D., and Brierley, J. A. (2007). Application of a risk indicator for assessing trends in dryland salinization risk on the Canadian Prairies. Can. J. Soil Sci. 87, 213–224. doi: 10.4141/S06-068
Wilkinson, H. H., Spoerke, J. M., and Parker, M. A. (1996). Divergence in symbiotic compatibility in a legume-Bradyrhizobium mutualism. Evolution 50, 1470–1477. doi: 10.1111/j.1558-5646.1996.tb03920.x
Yang, J., Kloepper, J. W., and Ryu, C. M. (2009). Rhizosphere bacteria help plants tolerate abiotic stress. Trends Plant Sci. 14, 1–4. doi: 10.1016/j.tplants.2008.10.004
Zhang, W. J., Niu, Y., Bu, S. H., Li, M., Feng, J. Y., Zhang, J., et al. (2014). Epistatic association mapping for alkaline and salinity tolerance traits in the soybean germination stage. PLoS ONE 9:e84750. doi: 10.1371/journal.pone.0084750
Zhu, T., Shi, L., Doyle, J. J., and Keim, P. (1995). A single nuclear locus phylogeny of soybean based on DNA-sequence. Theor. Appl. Genet. 90, 991–999. doi: 10.1007/BF00222912
Resource Identification Initiative
NCBI BLAST (NCBI BLAST, RRID:SCR_004870)
Clustal Omega (Clustal Omega, RRID:SCR_001591)
ClustalW2 (ClustalW2, RRID:SCR_002909)
iTOL (iTOL, RRID:SCR_018174)
WinRHIZO (WinRHIZO, RRID:SCR_017120)
SAS 9.4 (Statistical Analysis System, RRID:SCR_008567)
Keywords: co-inoculation, PGPR, stress tolerance, salinity, soybean, native legume
Citation: Ilangumaran G, Schwinghamer TD and Smith DL (2021) Rhizobacteria From Root Nodules of an Indigenous Legume Enhance Salinity Stress Tolerance in Soybean. Front. Sustain. Food Syst. 4:617978. doi: 10.3389/fsufs.2020.617978
Received: 15 October 2020; Accepted: 22 December 2020;
Published: 27 January 2021.
Edited by:
Saveetha Kandasamy, A&L Canada Laboratories, CanadaReviewed by:
Aria Dolatabadian, University of Western Australia, AustraliaCopyright © 2021 Ilangumaran, Schwinghamer and Smith. This is an open-access article distributed under the terms of the Creative Commons Attribution License (CC BY). The use, distribution or reproduction in other forums is permitted, provided the original author(s) and the copyright owner(s) are credited and that the original publication in this journal is cited, in accordance with accepted academic practice. No use, distribution or reproduction is permitted which does not comply with these terms.
*Correspondence: Donald Lawrence Smith, ZG9uYWxkLnNtaXRoQG1jZ2lsbC5jYQ==
Disclaimer: All claims expressed in this article are solely those of the authors and do not necessarily represent those of their affiliated organizations, or those of the publisher, the editors and the reviewers. Any product that may be evaluated in this article or claim that may be made by its manufacturer is not guaranteed or endorsed by the publisher.
Research integrity at Frontiers
Learn more about the work of our research integrity team to safeguard the quality of each article we publish.