- 1School of Agriculture and Food, Faculty of Veterinary and Agricultural Sciences, The University of Melbourne, Parkville, VIC, Australia
- 2Department of Agricultural Chemistry, Bangladesh Agricultural University, Mymensingh, Bangladesh
- 3AgriSci Pty Ltd, Rutherglen, VIC, Australia
- 4Bio21 Molecular Science and Biotechnology Institute, The University of Melbourne, Melbourne, VIC, Australia
Organic waste streams, otherwise known as organic amendments (OA), contain potentially valuable nutrients which may additionally increase legacy nutrient availability in soil. This is particularly the case for phosphorus (P) where declining reserves of rock phosphate add an extra dimension to their utility. In acidic soils, OA have been reported to increase P availability through the action of O-aryl and carbonyl groups (represent organic acid compounds) by substituting previously fixed, legacy P and forming organometallic complexes to reduce P sorption. This study aimed to investigate if signature P (orthophosphate) and C (O-aryl and carbonyl) content of OA could be used to predict soil P availability, to replace traditional ways of testing OA and also for future prescriptive applications. Food waste and biosolid were the sources of OA in this study, with pyrolysis and composting processes used to create a range of functional groups. Nuclear magnetic resonance (NMR) spectroscopy was utilized to identify forms of C (solid-state 13C NMR) and P compounds (solution-state 31P NMR) in these OA. The O-aryl, carbonyl, and orthophosphate content were higher in pyrolysis and composted materials compared to their feedstock substrate. The effect of OA addition on soil P availability was monitored in a 110-day laboratory incubation study. Results showed an increase in soil P availability (Olsen P) and a decrease in soil P buffering capacity (PBC) after incubation. The increase in soil P availability was not predicted well by the NMR-derived orthophosphate content of OA, which may be due to the overestimation of plant-available orthophosphate content by the solution-state 31P NMR. Furthermore, an additional increase in soil ΔOlsen P (difference between observed and expected) was obtained above the Olsen P added from OA indicating substitution of previously fixed soil P. Both indices of P availability namely ΔOlsen P (r = 0.63–0.83) and ΔPBC (difference between treatment—control) (r = −0.50 to −0.80) showed strong (but opposite) correlations with the ratio of O-aryl to carbonyl C content of OA. It was concluded that the ratio of O-aryl and carbonyl C content of OA could be used to predict the P availability in acidic soil.
Introduction
Returning organic waste to the land from which they originated is a logical component of the reuse and recycle mantra. Recycling of organic waste from urban environments as organic amendments (OA) to agricultural land is getting increasingly popular around the world with an aim to recover nutrients especially phosphorus (P). P is an essential and regularly applied nutrient to crops and pastures. Phosphate rock, a geopolitically scarce and declining resource (Smit et al., 2009; Van Kauwenbergh, 2010), is the main inorganic source for phosphate fertilizers applied to these plant systems. In addition, the plant availability of P from most phosphate fertilizers is very low due to the formation of insoluble phosphate complexes with soil cations (White, 1981; Glendinning, 2000; Juo and Franzluebbers, 2003; Price, 2006). Around 50% of the world's agricultural lands are acidic (Von Uexküll and Mutert, 1995) and many of these lands exist in developing countries where complexation of P by the Fe and Al ions is a dominant phenomenon. The oxides and hydroxides of Al and Fe depending on soil pH dissociate into Al3+ and Fe3+ ions which act as P sorption sites in the soil (Haynes and Mokolobate, 2001; Fink et al., 2016; Penn et al., 2018). Besides supplying P, OA have also been reported to increase soil P availability by reducing P sorption and substituting previously fixed, legacy P (Martinez et al., 1984; Bolan et al., 1994; Hansen and Strawn, 2003; Siddique and Robinson, 2003; Huang, 2004). Organic acids which are added from OA or producing during the decomposition process dissociate into organic anions and form organometallic complexes with the soil cations (Al3+ and Fe3+) to reduce P sorption sites and/or undergo ligand exchange reactions to substitute previously cation fixed P to increase soil P availability (Hue et al., 1986; Hue, 1991; Violante et al., 1991; Hu et al., 2005b, Bolan et al., 1994).
Among different forms of C present in OA, the O-aryl, and carbonyl C represent the organic acid compounds. Lignin, lignin-cellulose complexes, flavonoid, and tannins represent the phenolic/aromatic compounds in organic materials (Siqueira et al., 1991; Berg and Mcclaugherty, 2008), and go through a series of slow degradation processes to produce phenolic acids (O-aryl compounds) as an end product (Siqueira et al., 1991). Carbonyl C includes carboxylic and amide compounds in OA. Carboxylic compounds are composed of high and low molecular weight organic acids (Adeleke et al., 2017). The main fate of these organic acids has been reported as the sorption, metal complexation, mobilize, and solubilization of the poorly soluble nutrients by the action of organic anions when dissociating in the soil solution (Hoffland, 1992; Delhaize et al., 1993; Marschner, 1995; Micales, 1997; Jones et al., 2003).
Some OA contain both inorganic (orthophosphate and pyrophosphate) and organic (orthophosphate monoesters including phytic acid and other monoesters, orthophosphate diesters, phosphonates, DNA, and phospholipids and polyphosphates) forms of P (Hansen et al., 2004; Li et al., 2014; Stutter, 2015). However, among all the P compounds in the OA, only orthophosphate ions (H2 and ) are readily available to plants, whereas pyrophosphate (P2) and other organic forms of P are less bioavailable due to their chemical stability and requirement for microbial mineralization (Sutton and Larsen, 1964; Hutzinger and Craig, 1980; Celi et al., 1999; Turner et al., 2005; Leytem and Maguire, 2007).
Many new formulations of OA from different origin in the form of compost, biochar, dehydrated product, manures of plant and animal origin, enter the fertilizer/amendment market every year worldwide and in many cases their efficacy is unknown. It is common to test the effectiveness of OA (for supplying plant-available P, capacity to reduce P sorption and substitute previously fixed P in soil) systematically through laboratory incubation, pot/greenhouse and field experiments by determining several indicators of soil P availability parameters. These traditional approaches are expensive, time-consuming, and laborious. Although highly technical, the C (O-aryl and carbonyl) and P (orthophosphate) signature could be used to screen OA for increasing soil P availability, no such study is available to date where the C and P compounds of the OA were identified and used to understand their effect on soil P availability. This study aims to investigate the changes of C and P compounds in the OA across different sources (food waste and biosolid) and processing (biochar and compost) and to understand if the C (O-aryl and carbonyl) and P (orthophosphate) signature of an OA could be used to predict their behavior in increasing soil P availability.
Materials and Methods
Collection and Processing of Soil and Organic Amendments
A fine sandy clay loam, highly P fixing acidic cropping soil, Haplic, Eutrophic, Red Chromosol (Isbell, 2016) (Abruptic Acrisol according to WRB, 2015) was collected from the top 0–10 cm near Rutherglen, North East Victoria, Australia. This is the dominant acidic soil type within the agricultural zone of Victoria (Isbell et al., 1997) and also extensively used in previous P availability studies (Schefe et al., 2008, 2009, 2011; Schefe and Tymms, 2013). The collected soil was air-dried and sieved to ≤2 mm using a cylinder grinder before further use. The chemical characterization of the soil is presented in Table 1.
Food waste and biosolid and their different processed forms: oven-dried (feedstock); biochar and compost, were used in this study. Food waste was collected from the student union of the University of Melbourne, Parkville campus from a centralized waste collection point from eateries. The collected food waste was separated as best as possible to determine the food groups present. The waste contained on a dry weight basis: mixed vegetables (34%), leafy vegetables (1%), capsicum waste (6%), fruit peel waste (55%), some bread and dairy (2%), and some unidentifiable food waste (2%). Biosolid was collected from the wastewater treatment plant of North East Water, Wodonga, Victoria, Australia. The biosolid was collected into a drying pan on-site and left to air dry to ~75% solid and stockpiled for at least 7 years prior to collection. The biosolid used in this study was classified as T1C2 grade, according to Victorian Environmental Protection Authority regulations (Victoria, 2004), Australia. T1 represents the accepted treatment quality and C2 represents chemical contamination level especially heavy metals.
Oven Drying (Feedstock)
Food waste and biosolid were dried in an oven at 80°C overnight, cooled and ground to <2 mm with a cutting mill and cylinder sieve grinder, respectively and thereafter considered as feedstock of food waste (FW1) and biosolid (BS1). The ground materials were thoroughly mixed and stored in an airtight bucket for future use.
Pyrolysis
The FW1 and BS1 materials were pyrolyzed at 550–600°C for about 30 min in an oxygen-limited condition using a microwave pyrolysis setting at the University of Melbourne, Dookie campus following the procedure described in Kaudal et al. (2018). In this process, organic materials (~1.7 kg of FW1 per batch and in a total of 9 batches and ~3 kg of BS1 per batch and a total of 6 batches) were placed into a tray. In order to trap heat a previously prepared sawdust (Eucalyptus Regnans) biochar at the rate of 5% was added as microwave susceptor (in first batch only and then used the produced own biochar in subsequent batches) and intimately mixed before placing in a 6 kW microwave chamber. After completion of the heating cycle, the product was considered as food waste biochar (FW1B) and biosolid biochar (BS1B).
Compost
Compost was produced from a different feedstock to that used for FW1 and BS1 and therefore the food waste compost (FW2C) and biosolid compost (BS2C) cannot be considered as a production sequence. This was a deliberate strategy owing to the constraints of producing a compost at a small scale with acceptable homogeneity.
FW2C and BS2C were collected from commercial operations: Geelong compost, Geelong, Victoria, Australia and Gippsland water, Gippsland, Victoria, Australia, respectively. At Geelong compost, FW2C was prepared in a windrow composter for 5–6 months and consisted of 80% recycled green organics, 5% fruit waste (pulped fruit from a fruit juice manufacturer), 10% sugar mud (a bi-product of the sugar processing industry) and 5% poultry manure (with rice hulls bedding). At Gippsland water, BS2C was prepared in a combination of in vessel and open windrow composting setting for 12–14 weeks and consisted of biosolid, green waste and other organic waste. The precise makeup of this product was not disclosed for commercial reasons.
Chemical Characterization Procedure for the Soil and Organic Amendments
The electrical conductivity (EC), pH (H2O), pH (CaCl2), Olsen P, CaCl2 extractable P, and cation exchange capacity (CEC) were determined following the methods 3A2, 4A1, 4B2, 9C2a, 9F1, and 15D3 as described in Rayment and Lyons (2011), respectively. Total C and N (≤0.5 mm in size) were determined using a high-frequency induction furnace LECO (Trumac CN analyzer). Total elemental concentration was determined following an Aqua Regia digestion method: about 0.25 ± 0.01 g samples (≤0.5 mm) were placed into a 50 mL Greiner Polypropylene centrifuge tubes. 1.9 mL of concentrated nitric acid, 0.65 mL concentrated hydrochloric, followed by 0.5 mL 30% hydrogen peroxide was added, and the tubes were capped and vortexed for 10 s and then left overnight for pre-digestion. The tubes were heated on a digestion block at 80°C for 30 min and the built-up pressure was released by removing the cap slowly. The cap was then firmly reseated and the temperature was increased to 122°C until the samples were completely bleached. Once the digestion was completed the tubes were cooled down to room temperature and diluted to 25 mL using Milli Q water. The digested extract was analyzed for the total elements (P, Al, Fe, Zn, Mn, Ca, Mg, Na, K, and S) using an ICP-OES (Inductively Coupled Plasma—Optical Emission Spectrometry) (Perkin Elmer Optima 8300 DV-radial view).
The quality of the chemical analysis was controlled either by using external reference materials (both soil and plant samples) from the Australian Soil and Plant Analysis Council (ASPAC) or by using internal standards.
Nuclear Magnetic Resonance (NMR) Characterization of Organic Amendments
Solution-State 31P NMR
To prepare the sample for solution-state 31P NMR, all the OA (≤0.5 mm in size) were extracted using 0.25 M NaOH and 0.05 M EDTA solution at 1:20 sample to solution ratio following the method described in Turner (2004) and Mackay et al. (2017). The extract was centrifuged at 4,500 rpm for 20 min and filtered through Whatman no. 42 filter paper to collect the supernatant. About 30 ml of aliquot was immediately frozen using liquid nitrogen and freeze-dried. After freeze-drying, 150 mg of sub-sample was ground and re-dissolved in 1.5 ml of deionized water in a 2 ml Eppendorf tube. This was then centrifuged at 1,300 g for 20 min. Precisely 0.7 ml of aliquot of the supernatant was placed in a 5 mm NMR tube with 80 μL of deuterium oxide for analysis in the NMR. Methylene diphosphonic acid solution was added as an external standard at the rate of 6 g L−1. The spectra were acquired at 25°C on an Agilent 500 MHz Solution-state 31P NMR spectrometer at a 31P frequency of 202.34 MHz with a recovery delay of 26 s. A 90° pulse of 23 μs was used with an acquisition time of 1 s and broadband 1H decoupling. 50,000 scans were acquired for each sample. The spectra were processed with a 5 Hz line broadening.
Solid-State 13C NMR Study
Finely ground (≤0.5 mm) materials were used for solid-state 13C NMR study following the method described in Mackay et al. (2017). Solid-state 13C cross-polarization (CP) NMR spectra were acquired with magic angle spinning (MAS) at a 13C frequency of 150.33 MHz on an Agilent 600 MHz spectrometer. Samples were packed in a 4.2 mm diameter cylindrical zirconia rotor with Kel-F end-caps and spun at 10 kHz. Spectra were acquired using a cross-polarization pulse sequence (tancpx). One ms contact time and 1 s of recycling delay were used and 100,000 transients were collected for each spectrum. All spectra were processed with a 50 Hz Lorentzian line broadening. Chemical shifts were externally referenced to the methyl resonance of Adamantane (Morcombe and Zilm, 2003). All spectral processing was completed using Bruker TopSpin 3.6 software. The following chemical shifts were used to provide estimates of broad carbon types: 0–45 ppm (alkyl C), 45–60 ppm (N-alkyl C), 60–110 ppm (O-alkyl C), 110–145 ppm (aryl C), 145–165 ppm (O-aryl-C), and 165–215 ppm (carbonyl C). Signal intensity found in spinning sidebands was allocated back to their parent resonances according to the calculations presented by Baldock and Smernik (2002).
Soil Incubation Experiment With Organic Amendments
About 100 g of soil was incubated in a 250 mL plastic vial for 110 days with FW1, FW1B, FW2C, BS1, BS1B, and BS2C or without the addition of OA (control) at the rate of 0.5 g C (this rate was added based on a preliminary experiment where 0.5 g C had the maximum impact in increasing soil P availability from a range of 0.01–0.5 g C, Supplementary Table 1 and Supplementary Figure 1). The targeted rates of OA also resulted in the addition of different amounts of N and P to the soil (Supplementary Table 2). Vials were incubated at 20°C with moisture content at 70% of soil water holding capacity (WHC). In order to stabilize microbial activity, all vials were pre-incubated with only Milli Q water for 7 days prior to adding treatments (a total of 112 incubation vials). After the pre-incubation, all the treatments were added and mixed well before capping the vials and placing them into the incubator. Vials were destructively sampled at 7, 30, 60, and 110 days. Each sample collection date had a separate control and all the treatments had 4 replications. All the vials were aerated and watered regularly to maintain a similar aerobic condition throughout the experiment. At each time point of sample collection, the soil was oven-dried (40°C) overnight before chemical analyses. A subsample of the dried soil was used to determine the effect of OA addition on soil pH (CaCl2), plant-available P (Olsen P), and Mehlich-3 (M3) extractable soil Al and Fe concentration following the methods 4B2, 9C2a, and 18F1 described in Rayment and Lyons (2011).
Determination of Soil Phosphorus Buffering Capacity (PBC)
Soil PBC was determined following the modified method of 9J2 described in Rayment and Lyons (2011). About 2.5 g of oven-dried incubated soil (all treatments at all times) was placed into a 50 mL centrifuge tube and 25 ml of 0.01 M CaCl2 solution containing differing concentrations of P (KH2PO4) ranging from 0, 10, 20, 40, and 80 mg P/L was added. Two drops of chloroform were added to suppress microbial growth. Then it was shaken for 17 h using an end-over-end shaker, centrifuged and filtered (Whatman no 5 filter paper) to collect the supernatant. The phosphate concentration in the supernatant was determined colorimetrically following Murphy and Riley (1962) method using a segmented flow analyzer (SFA) (Skalar SAN++). P sorption was calculated according to Equation (1).
Where, C0 and Ce were the initial phosphate concentration, and phosphate concentration in the supernatant (mg L−1), respectively. V represents the volume of solution (mL) and M is the mass of the soil taken (g).
Soil Phosphorus Buffering Capacity (PBC) was calculated by plotting P adsorbed (mg/kg) on Y-axis against log10C (initial P concentration) and fitted with a linear equation. The slope of the linear fitting was presented as PBC in mg/kg/log10mg/L, as described in Rayment and Lyons (2011).
Statistical Analysis
Data were statistically analyzed by analysis of variance (ANOVA) using the software package Minitab 18 (Akers, 2018). Treatment means were separated using least significant differences (LSD) value at 5% level of probability at a specific sample collection point. Pearson's correlation coefficient was used in correlation studies.
Results
Chemical Characterization of the Organic Amendments
The pyrolysis had a relatively greater impact on food waste substrate compared to the biosolid substrate (Table 1). Pyrolysis increased the pH, total C, and total P in FW1B relative to FW1, whereas BS1B showed very little to no effect compared to BS1. Similarly, pyrolysis markedly decreased the Olsen P concentration in FW1B compared to FW1 and slightly in BS1B than BS1. In case of FW2C and BS2C, the greatest difference was observed in total Al, Fe, and Mg content compared to FW1, FW1B, BS1, and BS1B due to having different feedstock materials. Among all the food waste materials FW2C had a higher Al, Fe, Ca, and Mg content, whereas BS2C had the lowest Al, Fe, Ca, and Mg content among biosolid materials.
Identification of Forms of P in the Organic Amendments
The solution-state 31P NMR spectrometer identified orthophosphate, orthophosphate monoesters, pyrophosphate and phospholipid compounds from OA in this study (Supplementary Figure 2). In FW1, the dominant forms of P compounds were orthophosphate monoesters P (89.25%) followed by orthophosphate (10%), phospholipid (0.34%) and pyrophosphate (0.28%) (Table 2). Pyrolysis of food waste substrate converted all the P monoesters compounds of FW1 (89%) to orthophosphate (93%) in FW1B, which was the dominant form of P. It was also interesting to note that biochar materials contained a higher amount of pyrophosphate (6.7% in FW1B) compared to other processed forms of organic materials used in this experiment (Table 2). There was little to no effect of pyrolysis on biosolid materials observed. In biosolid materials, orthophosphate and orthophosphate monoester peaks were overlapped with each other (Supplementary Figure 2) due to having a high metal concentration in the biosolid materials, which affected the NMR signals. The presence of Fe and other metal species at higher concentrations exert a paramagnetic effect in NMR signals by affecting instrument relaxation and surface relaxivity (Keating and Knight, 2010). In FW2C and BS2C, the dominant forms of P compounds were orthophosphate, followed by orthophosphate monoesters and pyrophosphate.
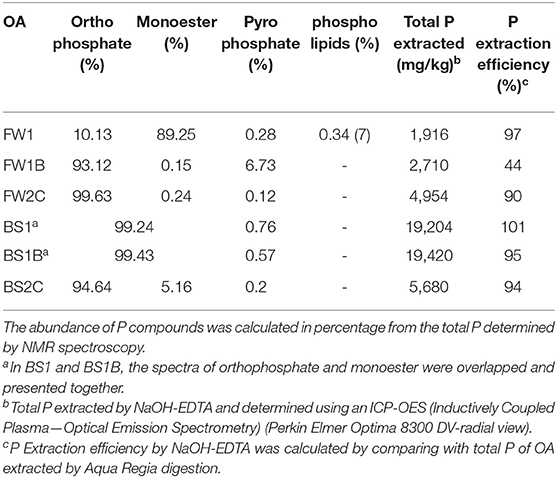
Table 2. The abundance of the identified P compounds in organic amendments using solution-state 31P NMR.
Identification of Forms of C in the Organic Amendments
The solid-state 13C NMR identified alkyl C (represents aliphatic lipids, fatty acids and waxes), N-alkyl C (amino acids and amino sugars), O-alkyl C (oxygenated C in carbohydrate which includes sugars and polysaccharides), aryl C (alkyl-substituted aromatic compounds), O-aryl C (phenolic compounds), and carbonyl C (carboxylic, amide and ketone compounds) (Baldock and Smernik, 2002; Albrecht et al., 2008; Spaccini et al., 2012) in all the OA (Supplementary Figure 3). The O-alkyl C (sugars and polysaccharides) (88.1%) was the major C form in FW1 with the alkyl C to O-alkyl C ratio of 0.1 (Table 3). Pyrolysis of FW1 decreased the abundance of O-alkyl C and increased the abundance of alkyl C, N-alkyl C, aryl-C (aromatic), O-aryl C and carbonyl C forms in FW1B (Table 3). In FW1B, the major form of C was aryl C (aromatic) (46.3%) followed by alkyl C (24.3%), N-alkyl C (4.7%), O-aryl (2.7%) and carbonyl C (11.3%), with O-aryl to carbonyl C ratio of 2.3 and alkyl C to O-alkyl C ratio of 0.9 (Table 3). Similar to FW1, the abundant C form was O-alkyl C (sugars and polysaccharides) (38.9%) in the BS1 with the alkyl to O-alkyl C ratio 0.6. Pyrolysis of the BS1 reduced the alkyl C, N-alkyl C and O-alkyl C and increased the aryl C (aromatic) (27.8%), O-aryl C (7.8%), and carbonyl C (10.3%) content in BS1B (Table 3). In FW2C and BS2C, the major form of C was O-alkyl C (sugars and polysaccharides) with the alkyl C to O-alkyl C ratio of 0.2 and 0.6, respectively (Table 3). Amongst all the OA, the highest abundance of carbonyl C was observed in BS1B (10.3%) and the highest abundance of O-aryl C was observed in FW2C (10.5%). Together O-aryl C and carbonyl C abundance were highest in the FW2C (18.7%) followed by BS1B (18.1%), FW1B (14%), BS2C (9.4%), BS1 (6.2%), and FW1 (4.1%) (Table 3).
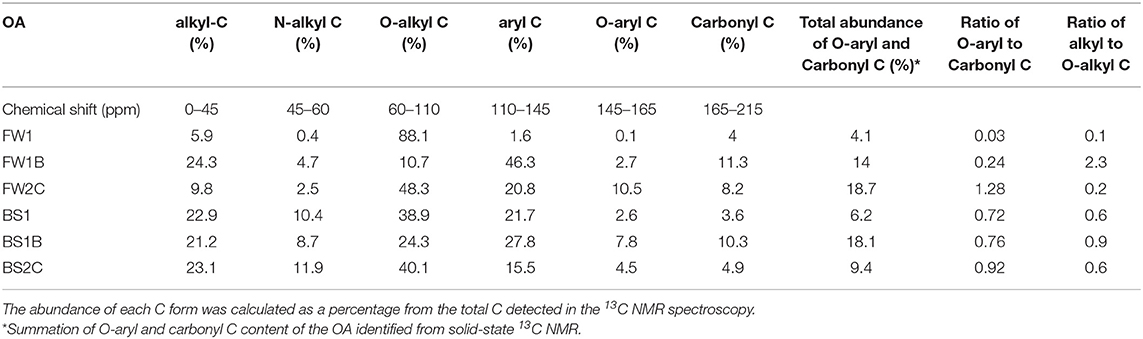
Table 3. The abundance of the forms of C in organic amendments identified using solid-state 13C NMR spectroscopy.
Effect of Incubation of Organic Amendments on Soil pH
The addition of all the OA significantly (P ≤ 0.05) increased soil pH (ranged from 3.9 to 4.7) compared to the control (ranged from 3.7 to 3.8) across all the points of sample collection (Figure 1). No significant difference was observed in soil pH between feedstock and biochar substrates of both food waste and biosolid at most of the sampling times. In composted materials, FW2C significantly increased the soil pH compared to FW1and FW1B at each point of sample collection whereas the BS2C was recorded as the lowest soil pH compared to BS1 and BS1B.
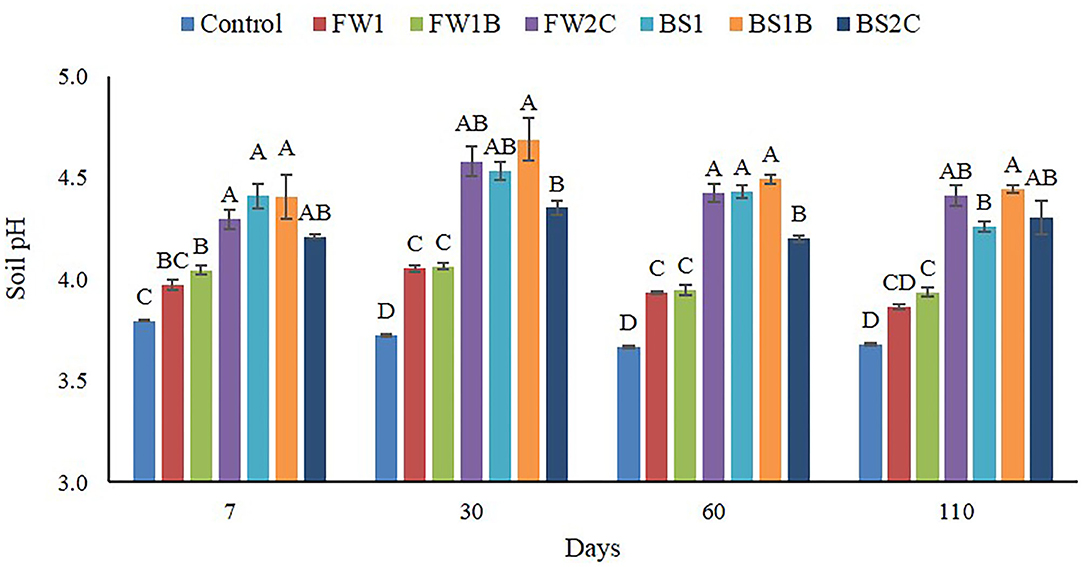
Figure 1. Effect of organic amendments on soil pH across all the times of sample collection. The line on the top of the bar indicates standard error (n = 4) and different letters are indicating statistically significant at P ≤ 0.05 at a specific point of sample collection.
Effect of Incubation of Organic Amendments on Soil Olsen P
The addition of all the organic materials significantly (P ≤ 0.05) increased soil Olsen P at all times relative to the control except for FW1 and FW1B (Figure 2). The FW1 and FW1B did not show any significant difference in soil Olsen P compared to control at any time. FW2C had a significant (P ≤ 0.05) increase of soil Olsen P at all the time points among all the food waste materials. In case of biosolid materials, no significant difference was observed initially (7 days) and later the highest Olsen P increase was observed by BS1B at 30 and 110 days and by BS1 at 60 days. BS2C always had the least effect on soil Olsen P among all the biosolid materials in 30, 60, and 110 days. It is interesting to observe that no significant difference was observed initially among FW2C, BS1, BS1B, and BS2C in most of the cases at 7 and 30 days. In 60 and 110 days, the soil Olsen P was significantly increased by the BS1 and BS1B compared to FW2C and BS2C. In most of the cases, no significant difference was observed between FW1 and FW1B; BS1 and BS1B (except 60 days) and FW2C and BS2C at any time.
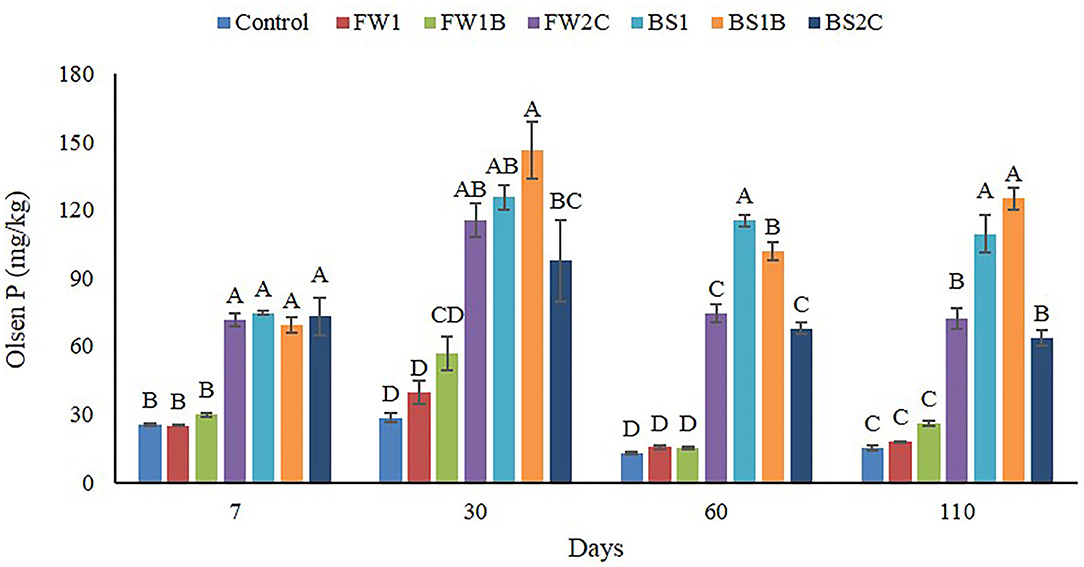
Figure 2. Effect of organic amendments on soil Olsen P across all the times of sample collection. The line on the top of the bar indicates standard error (n = 4) and different letters indicate statistically significant at P ≤ 0.05 at a specific point of sample collection.
Effect of Incubation of Organic Amendments on Soil Mehlich-3 Extractable Cations (Al and Fe)
Compared with control all the OA significantly (P ≤ 0.05) decreased M3 extractable Al concentration in soil at 7 days except BS1 (Figure 3). The BS1 had no significant (P ≤ 0.05) difference in soil M3 extractable Al concentration at 7, 30, and 60 days compared with control. The soil M3 extractable Al was significantly (P ≤ 0.05) decreased as observed by the FW1 and FW2C compared with control at all the time except by FW2C at 110 days. The FW2C showed no significant (P ≤ 0.05) difference in M3 extractable Al concentration at 110 days compared to control. In 30, 60, and 110 days, compared to control, no significant (P ≤ 0.05) difference on soil M3 extractable Al concentration by FW1B, BS1, BS1B, and BS2C was observed except at 110 days. At 110 days, BS1 and BS1B significantly increased (P ≤ 0.05) M3 extractable Al concentration compared with all the treatments.
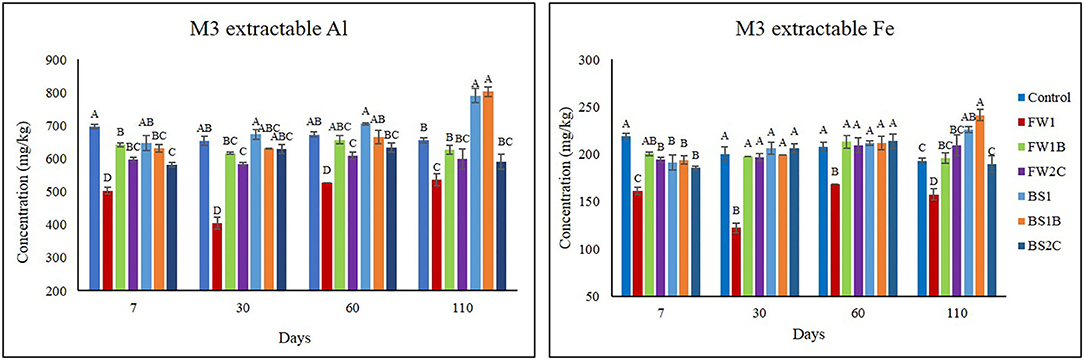
Figure 3. Effect of organic amendments on soil Mehlich-3 (M3) extractable Al and Fe concentration across all the times of sample collection. The line on the top of the bar indicates standard error (n = 4) and different letters are indicating statistically significant at P ≤ 0.05 at a specific point of sample collection.
Similar to soil M3 extractable Al concentration, the addition of OA significantly decreased the soil M3 extractable Fe concentration at 7 days. The FW1 had a significant effect on decreasing soil M3 extractable Fe concentration at all the time compared to control and all other treatments. Compared to control, at 30 and 60 days, no significant difference was observed among any of the treatments except FW1. At 110 days BS1 and BS1B significantly increased the M3 extractable Fe concentration compared to all other treatments, whereas no significant difference was observed among control FW1B, FW2C, and BS2C.
Effect of Incubation of Organic Amendments on Soil Phosphorus Buffering Capacity
The addition of OA significantly decreased (P ≤ 0.05) soil PBC at each time compared to the control (Figure 4). Compared with all the processed forms of OA, the composted materials of both food waste (FW2C) and biosolid (BS2C) had the highest decrease in soil PBC. Between FW2C and BS2C, FW2C significantly (P ≤ 0.05) decreased soil PBC at all the time compared to BS2C except 7 and 30 days. There was no significant (P ≤ 0.05) difference observed among FW1, FW1B, BS1, and BS1B at any time except by BS1B at 30 and 110 days. The BS1B significantly decreased soil PBC at 30 and 110 days compared to FW1, FW1B, and BS1. It is also interesting to observe that the soil PBC showed a decreasing trend of varying degrees by all the treatments as incubation time progressed.
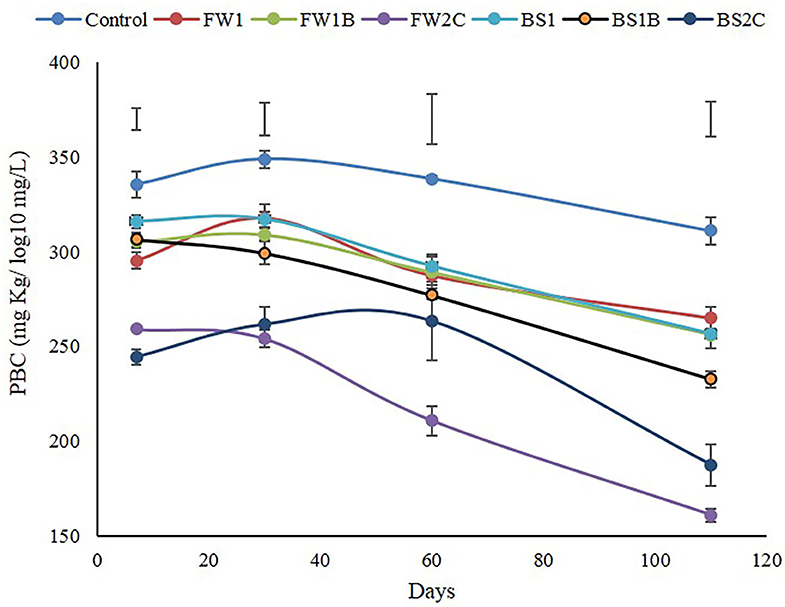
Figure 4. Effect of organic amendments on soil Phosphorus Buffering Capacity (PBC) at each time. The bar on each trend line representing standard error (n = 4) and the floating bar on the top representing l.s.d at P ≤ 0.05 at a specific point of sample collection.
Discussion
Chemical Characterization of the Organic Materials
In this study, a direct comparison can only be made between FW1, BS1, and their biochar materials (FW1B and BS1B) due to having similar feedstock materials compared to composted materials. The increase in pH after the pyrolysis of the OA was attributed to the decrease in acidic functional groups during the thermal treatment of organic materials (Song and Guo, 2012; Dai et al., 2017). The increase in the concentration of C, P and other elements was attributed to the loss of mass of the materials during pyrolysis in the form of volatile hydrocarbons, hydrogen (H2), carbon monoxides (CO) and carbon dioxide (CO2) (Robinson et al., 2018). The increase in the C concentration was also attributed to the formation of stable C compounds especially aromatic C (Baldock and Smernik, 2002; Demirbas, 2004) which was further confirmed from the solid-state 13C NMR analysis of the biochar materials (aryl and O-aryl C content) (Table 3). However, the pyrolysis of biosolid substrate had a relatively small effect in biosolid biochar in this study compared to food waste substrate. This was may be due to the low C content of the BS1 which affected the char production process during pyrolysis. The C content of the organic amendments has been indicated as the key element for the yield of biochar and functional group developments (Weber and Quicker, 2018). Moreover, as the biosolid was stockpiled for seven years, there would have been preferential accumulation of aromatic structures due to selective microbial decomposition of aliphatic structures. The increase in N concentration after pyrolysis in plant-derived materials and decreased in biosolid materials may be due to the high labile form of N in biosolids. Similar results were also observed in a previous study by Robinson et al. (2018). Labile N content of OA vaporizes at a relatively low temperature with a peak at 200 (Surampalli et al., 2015) to 300°C (Baldock and Smernik, 2002) compared to other nutrients such as P at around 1,000°C (Zhang et al., 2012). In this study, the biochar was produced at a temperature ranging from 550 to 600°C.
The decrease in the Olsen P was attributed to the formation of hydroxyapatite and oxyapatite during the pyrolysis process of the organic materials at a high temperature above 450°C (Hunger et al., 2008; Li et al., 2018). Hydroxyapatite and oxyapatite are usually absent in unprocessed feedstock materials (Hunger et al., 2004) and likely to form during the pyrolysis process. They are considered to be only poorly labile in the sequential extraction of the P from organic materials (Li et al., 2018).
Identification of Forms of P Using Solution-State 31P NMR
The P extraction efficiency is an important factor to identify different forms of P in the OA using solution-state 31P NMR. The P extraction efficiency of NaOH and EDTA was more than 90% for all the amendments in this study except for FW1B (44%) (Table 2). A similar P extraction efficiency of NaOH-EDTA ranging from 46 to 86% was observed by Chen et al. (2004) and low extraction efficiency in the case of biochar (25%) by Stutter (2015) compared to other processed forms of OA such as compost, manure and anaerobic digest.
The lowest orthophosphate content and highest monoesters content in FW1 (Table 2) was due to having high lipid and phytate content as also observed in a study by Idowu et al. (2017). The increase in orthophosphate content in FW1B was indicating the conversion of organic P to inorganic orthophosphate during pyrolysis process. The conversion of organic P to inorganic P (orthophosphate) ranging from 67 to 99% during the pyrolysis of organic materials has also been reported in previous studies (Huang et al., 2017; Li et al., 2018). The pyrolysis of the organic materials also increased the abundance of pyrophosphate in this study. The polymerization of orthophosphate to form pyrophosphate during the pyrolysis process has also been reported in a previous study (Xu et al., 2016).
It was interesting to observe that, the abundance of the NMR-derived orthophosphate increased in the biochars compared to their unprocessed feedstock materials, but the Olsen P content decreased. This may be due to the formation of less bioavailable metal orthophosphate compounds such as Al, Fe, Ca, and Mg phosphate during the pyrolysis of organic materials at elevated temperature (Li et al., 2018; Rose et al., 2019). In some recent studies, the use of X-ray absorption near edge structure (XANES) spectroscopy identified the formation of less water-soluble P compounds in biochar such as octacalcium phosphate (Hunger et al., 2008), variscite (Al-phosphate) (Rose et al., 2019) and hydroxyapatite (Robinson et al., 2018) as new dominant forms of P.
The composted product of the food waste and biosolid used in this study was rich in orthophosphate content. A similar higher abundance of orthophosphate was found in food waste compost by Stutter (2015) and in sewage sludge compost by Galvez-Sola et al. (2010).
Identification of Forms of C Using Solid-State 13C NMR
Similar to the chemical characterization of the OA, pyrolysis had a greater impact on food waste substrate to change the abundance of forms of C compared to the biosolid substrate (Table 3). The O-alkyl C (61–110 ppm) usually represents the sugars and polysaccharide compounds in the OA which are the labile forms of C compounds that can quickly convert to the more stable C forms (aromatic C) during the heating process of biochar production (Almendros et al., 2003). An increase in the alkyl C region was observed when olive mill waste was heated at 300°C and a gradual increase in aromatic region (aryl C and O-aryl C) was observed when heated to 500°C to 1,000°C (Marra et al., 2018). This may explain the reason for decrease in O-alkyl C (sugars and polysaccharides) abundance in FW1B and increase in alkyl and aromatic C (aryl C and O-aryl C) region after pyrolysis at 550–600°C compared to FW1 in this study (Table 3).
In the composted materials, FW2C and BS2C, the abundance of O-alkyl, N-alkyl, and alkyl C were greater compared to aryl and O-aryl C. A recent study by Mayans et al. (2019) has also shown the higher abundance of this aliphatic C (O-alkyl, N-alkyl, and alkyl C) forms compared to the aromatic C (aryl and O-aryl C) forms in pruning waste compost, pine bark compost, vermicompost and sheep, and horse manure compost. Furthermore, during the composting process the conversion of the aliphatic compounds (O-alkyl, N-alkyl, and alkyl C) to aromatic (aryl C), O-aryl C and carboxylic (carbonyl) compounds have been reported (Chefetz et al., 1996; Spaccini and Piccolo, 2008; Tapia et al., 2010). This may be the reason for having higher aryl (from 1.6 to 20.8%) and O-aryl C (from 0.1 to 10.5%) and lower O-alkyl C (from 88.1 to 48.3%) compounds in FW2C compared to FW1 (Table 3). In contrast, the BS2C did not follow the decreasing trend in the abundance of O-alkyl and increasing trend in aryl C abundance compared with BS1. This is may be due to having a different feedstock material for FW2C and BS2C compared to FW1 and BS1, respectively.
The carbonyl C range (165–215 ppm) includes the carboxylic, amide, aldehyde and ketone compounds. However, in this study, the presented ranges for carbonyl C (165–215 ppm) compounds will be considered as carboxylic compounds (Table 3). Because the absence of amide, aldehyde and ketone compounds in organic materials has been reported in previous studies (Al-Faiyz, 2017; Marra et al., 2018; Mayans et al., 2019). No signal of amide, aldehyde and ketone compounds were found in olive mill waste and olive mill waste biochar (Marra et al., 2018). A very recent study also demonstrated a complete absence of amide, aldehyde and ketone compounds in the pruning waste compost, vermicompost, and sheep and horse manure compost and a negligible amount (1.6%) in pine bark compost (Mayans et al., 2019).
Effect of Incubation of Organic Amendments on Soil P Availability
In acidic soil, the change in pH with the addition of OA have been reported to increase P availability if the pH was increased from an acidic pH to a neutral pH (6.5–7) (Ritchie and Dolling, 1985; Haynes and Swift, 1989; Hue, 1992; Yan et al., 1996). In this study, the observed soil pH ranged from 3.7 to 4.7 throughout the experiment (Figure 1) which is considered as a zone where soil P sorption/fixation by the soil cations such as Al3+ and Fe3+ is dominant (Price, 2006; Penn and Camberato, 2019). Therefore, the change in native soil pH from 3.7 to 4.7 by the OA is unlikely to be the reason for increased soil P availability in this study.
The addition of OA significantly increased soil Olsen P to a varying degree in this study (Figure 2). The addition of different amounts of P from different OA (Supplementary Table 2) can explain the varying degree of increase of soil Olsen P. The obtained lower soil Olsen P at all times by FW1 and FW1B and higher by BS1 and BS1B compared to control may further support the effect of the addition of different amounts of P ranging from 2.3 (FW1) to 112.8 mg (BS1B) of total P from OA (Supplementary Table 2) and an increase of varying degrees of soil Olsen P.
OA contributed both organic and inorganic P in this study. Inorganic orthophosphate P is considered as readily available and organic P is needed to be mineralized to inorganic orthophosphate P before become available. The organic P content of the OA used in this experiment ranged from 1 to 7% except for FW1 (~90%) (Table 2) as identified solution-state 31P NMR spectroscopy. Among different forms of organic P present in OA, the major form of organic P was orthophosphate monoesters which are considered as less bioavailable due to its slow mineralization in the soil and also due to its high charge density and formation of stable complexes with cations, clay and organic matter in the soils (Celi et al., 1999; Turner et al., 2005; Leytem and Maguire, 2007). The concentration of soil Olsen P in the control treatment was fairly constant throughout the experiment (Figure 2) Therefore, it is highly likely that the mineralization of organic P (from both in soil and OA) would be a non-dominant phenomenon in this experiment for increasing soil Olsen P. Furthermore, the addition of OA to the soil was expected to contribute an initial increase in labile C with a concomitant increase in soil microbial activity and acid phosphatase activity. These phenomena are likely to be responsible for an initial increase in soil P availability after the addition of OA in this study (Schefe et al., 2008; Waldrip et al., 2011; Rahman et al., 2014). However, limitations on the availability of the microbial and phosphatase activity data restrict the option to establish a direct relation on soil P availability in this study.
To determine the contribution of inorganic P content from OA on increasing soil P availability, the expected Olsen P was calculated using both the orthophosphate (NMR recoveries) and Olsen P content measured in OA (Figure 5). The calculation showed that none of the predicted Olsen P was better fitted with the observed Olsen P (Figure 5). The expected Olsen P (when calculated considering orthophosphate content of OA) exceeded observed Olsen P in most of the cases except for FW1. It has been reported that NMR overestimates the bioavailable P concentration (orthophosphate) if orthophosphate present in the form of orthophosphate-metal complexes in the samples (Turner et al., 2003). In some recent studies, as also discussed earlier the use of X-ray absorption near edge structure (XANES) spectroscopy identified less bioavailable orthophosphate compounds such as calcium phosphate (Hunger et al., 2008), variscite (Al-phosphate) (Rose et al., 2019), hydroxyapatite (Robinson et al., 2018) as dominant forms of P in OA, especially in biochar. These compounds were likely to be present in the organic materials used in this study (especially in the biochar and compost) and may have caused the overestimation of the bioavailable orthophosphate content when identified using solution-state 31P NMR.
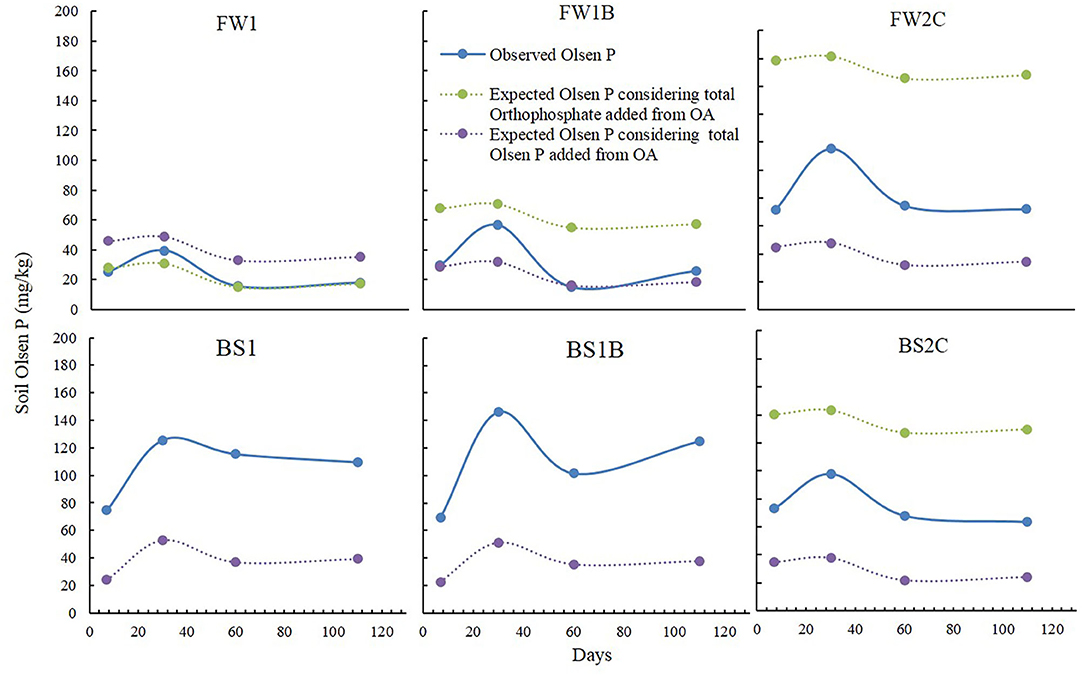
Figure 5. The observed and expected Olsen P concentration in this study. The expected Olsen P was calculated by combining obtained control soil Olsen P + P added from OA in the form of orthophosphate (NMR recoveries) (green dotted line) and Olsen P (purple dotted line) at each time. The blue line represents observed Olsen P in this study. In case of BS1 and BS1B, orthophosphate content was not available due to high Fe content which exerts paramagnetic interference during NMR detection (Table 2).
The observed Olsen P showed an additional increase for FW2C, BS1, BS1B, and BS2C at all times compared to P added from OA in the form of Olsen P (Figure 5). The mechanisms for obtaining this additional Olsen P increase were hypothesized to be due to the substitution of the previously fixed P by the organic anions and the formation of the organometallic complexes in the soil (Hansen and Strawn, 2003; Siddique and Robinson, 2003; Schefe et al., 2009). The representative organic acid producing compounds in the OA are O-aryl and carbonyl C (Albrecht et al., 2008; Spaccini et al., 2012; Eldridge et al., 2013) and are expected to show a correlation with the additional increase of soil Olsen P (ΔOlsen P, the difference between observed and expected Olsen P). In this current study, a correlation study between the ΔOlsen P and O-aryl and carbonyl C content of the OA was conducted (Table 4). The ΔOlsen P was correlated with the individual, total and the ratio of the O-aryl to carbonyl C content of the OA. The correlation coefficient (r) value showed a strong positive significant (P < 0.01) correlation (ranging r value from 0.60 to 0.83) with the ratio of the O-aryl to carbonyl C content of the OA compared to their individual and total abundance (Table 4). The correlation study did not show any significant relationship with the carbonyl C content of the OA whereas it showed a very strong correlation with the O-aryl C content of the OA (Table 4).
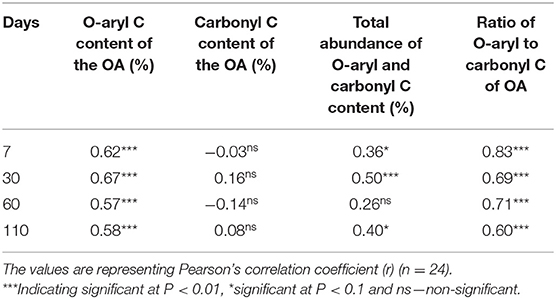
Table 4. Pearson's correlation study between the change in soil Olsen P (mg/kg) (ΔOlsen P, the difference between observed and expected Olsen P, considering Olsen P contribution from OA) and O-aryl and carbonyl C content of the OA at each sampling time.
In general, carbonyl compounds dissociate more readily and are capable of forming stable organo-metal complexes in the soil (Hu et al., 2005a,b; Lindegren and Persson, 2009). However, the microbial degradation of the carbonyl compounds (due to aliphatic structure) is much quicker relative to the O-aryl compounds in the soil ranging from 3 h to several days (Ström et al., 2001) and this may explain why the carbonyl C of OA did not show any significant correlation with ΔOlsen P. The presence of a ring structure in O-aryl compounds make them resistant to microbial degradation, which means they have a longer residence time in the soil and may provide organic anions for a longer period (Schefe and Tymms, 2013). During the degradation process, O-aryl compounds go through a series of slow degradation steps with the production of simple organic acids as an end product (Dagley, 1967; Haider et al., 1975; Shindo and Kuwatsuka, 1976; Kassim et al., 1982) which may supply organic anions for a longer period in soil and also explains the reason for showing significant correlation with the ΔOlsen P. The complementary effect between O-aryl and carbonyl C content of the OA may further explain the reason for obtaining strong correlation between ΔOlsen P and the ratio of O-aryl to carbonyl C. For instance, some O-aryl compounds are known to be toxic to the soil microbes (Hu et al., 2005b; Wu et al., 2016), which may delay the degradation of carbonyl compounds and prolong their effect on soil P availability.
As time progressed in this current study, the Olsen P decreased at 60 days and remained stable until 110 days for most of the treatments (Figure 2). The decrease of Olsen P may be due to the degradation of the organic anions as time progressed, which released the Al or Fe from organometallic complexes and caused re-adsorption of the P in the soil (Schefe and Tymms, 2013). The weaker correlation between the ratio of O-aryl and carbonyl C content of OA and ΔOlsen P as time progressed may also support the degradation of organic anions and re-adsorption of P and reasons for decreasing soil Olsen P.
As the organometallic complexes formed at the beginning of the OA addition, less Al and Fe were expected to be extracted. The addition of OA initially at 7 days significantly decreased the soil M3 extractable Al and Fe concentrations with the highest decrease by FW1 at all times and increased again as time progressed (Figure 3). Although the highest decrease in the soil M3 extractable Al and Fe concentration was obtained by FW1, no increase in soil Olsen P was observed. FW1 was low in organic acid compounds (O-aryl and carbonyl) and was also rich in orthophosphate monoester compounds. As mentioned earlier, the Al and Fe were likely to form strong complexes with the orthophosphate monoesters compounds when FW1 was added to the soil and recorded the lowest M3 extractable Al and Fe.
Effect of Incubation of Organic Amendments on Soil PBC
The results showed that soil PBC decreased with the addition of all the OA (Figure 4) and kept decreasing as time progressed. As discussed earlier, the mechanisms proposed for decreasing PBC after the OA addition were the blocking of the soil P sorption sites by forming organometallic metal complexes with organic anions (Hansen and Strawn, 2003; Siddique and Robinson, 2003) or competition between organic anions with phosphate ions to be fixed (Levesque and Schnitzer, 1967; Borie and Zunino, 1983; Mnkeni and Mackenzie, 1985; Hue et al., 1986; Sibanda and Young, 1986; Iyamuremye et al., 1996; Geckeis et al., 2002; Kwabiah et al., 2003; Hu et al., 2005b; Cheng et al., 2008).
Like soil ΔOlsen P, a similar correlation between the ΔPBC (difference between PBCtreatment – PBCControl) and the O-aryl and carbonyl C of OA was expected. A significant strong negative correlation between ΔPBC and the O-aryl and carbonyl compounds of OA at each time was observed (Table 5). The correlation was stronger with the ratio of O-aryl to carbonyl C content of the OA with the ΔPBC at each time point compared to the individual and total abundance of the O-aryl and carbonyl C content of the OA (Table 5). However, unlike Olsen P as time progressed from 7 to 110 days the correlation between ΔPBC and O-aryl and carbonyl C content of the OA became stronger with a range of correlation coefficient values from 0.50 to 0.80 (Table 5). This prolonged effect of OA on decreasing soil PBC may be due to the sequential and slow degradation of O-aryl compounds, which were supplying organic anions for a longer period in the soil. The M3 extractable Al and Fe concentration was expected to decrease as the time progressed in this current study but observed otherwise (Figure 3). This was likely due to the large amounts of Al and Fe that were added to the soil from the OA themselves, which increased the M3 Al and Fe concentration in this study. Furthermore, the degradation of organic anions that are firmly held by soil cations (an organometallic complex) is still controversial (Jones, 1998; Adeleke et al., 2017). Both fast (if held loosely) (Jones and Edwards, 1998; Adeleke et al., 2017) and slow (Ström et al., 2001; Fischer et al., 2010; Andrade et al., 2013) bio-degradation of organometallic complexes by soil microbes have been reported. As the continuous decrease of the soil PBC was observed, a slower degradation of the organic metallic complexes is likely to be happening in this study as time progressed. Therefore, the decrease in soil Olsen P at 60 and 110 days was not likely due to the degradation of organometallic complexes and re-adsorption of P by Al and Fe as discussed earlier. Another hypothesis would be the immobilization of P from the soil, which possibly explains the decrease in soil Olsen P at 60 and 110 days. Because the addition of OA with a low O-alkyl to alkyl C ratio is likely to inspire microbial activity due to the availability of mineralizable C in the soil (Skene et al., 1996; Baldock et al., 1997; Bernal et al., 1998). The range of O-alkyl to alkyl C ratio of the OA used in this study was 0.1 to 2.3 (Table 3) and this was likely to increase microbial activity and immobilize P from soil.
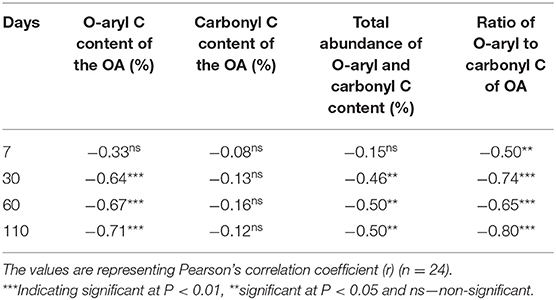
Table 5. Pearson's correlation study between the change in soil PBC (ΔPBC, difference between PBCtreatment – PBCcontrol) and O-aryl and carbonyl C content of the OA at each time.
Furthermore, in this study, the OA with a lower ratio of O-aryl to carbonyl C content (FW1 and FW1B) have shown little effect on increasing Olsen P and decreasing soil PBC compared to the OA with a higher ratio of O-aryl to carbonyl C content. It is also interesting to note that, the OA with the equal abundance of O-aryl and carbonyl C content (FW2C and BS2C) had maximum effect on decreasing soil PBC may be due to the complementary effect between O-aryl and carbonyl compounds as discussed earlier.
Conclusions
The primary aim of this work was to produce materials from two different feedstocks with differing combinations of organic functional groups. This was not achieved due to having different feedstock materials between biochar and compost. The comparison was made only between unprocessed feedstock and biochar materials due to the use of similar feedstock materials. The nutrient and total metal concentrations were higher in the biosolid materials compared to the food waste materials. The aromatic C compounds (aryl and O-aryl C) were abundant in the biochar materials (FW1B and BS1B) and the aliphatic C compounds (O-alkyl, N-alkyl and alkyl C) were abundant in the unprocessed feedstock and composted materials (FW1, FW2C, BS1, and BS2C). The abundance of organic anion producing C compounds (O-aryl and carbonyl) was higher in biochar and composted material compared to their unprocessed feedstocks. The processing of unprocessed feedstock materials to biochar and compost also increased the abundance of the inorganic forms of P (orthophosphate). However, the orthophosphate content identified by solution-state 31P NMR was not able to predict the P fertilization value of the organic materials, which may be due to the overestimation through NMR recoveries of the plant-available orthophosphate content. For obtaining an accurate prediction of the contribution of bioavailable orthophosphate content from OA, other spectroscopic techniques such as XANES with the NMR analysis needed to be combined to identify P species.
The addition of OA in this acidic soil increased soil P availability by supplying P, also by a series of other mechanisms such as substituting previously fixed P and reducing P sorption through forming organometallic complexes. The O-aryl and carbonyl C content represents organic acid compounds of the OA and could be used to predict their role in increasing P availability in the soil. The OA with a higher or equal abundance of O-aryl C compounds relative to carbonyl C compounds had a superior effect on soil P availability compared to the lower abundance. Therefore, we recommend that the O-aryl and carbonyl C content of OA could be used to understand the effectiveness of an OA on soil P availability. The ratio of O-aryl to carbonyl C content would better predict the role of OA in increasing soil P availability compared to their total abundance.
In future, it would be interesting to verify the findings from this study with a wider range of organic materials against soil having different properties. It would also be important to evaluate the sorption capacity of the P by the organic materials itself. The inclusion of modern techniques for the generation of data regarding microbial and phosphatase activity would be useful to understand completely the effect of OA on soil P availability. At present, the accessibility of NMR facilities and the requirement for specialist knowledge to interpret spectra may prohibit the routine use of the findings from this study. However, if the benefits of this technique for prediction of the appropriateness of organic amendment were more broadly established and coupled with the ever-decreasing cost of such technology, then farmers and other land managers would have greater confidence in the long-term benefit of returning waste streams to agricultural systems.
Data Availability Statement
The raw data supporting the conclusions of this article will be made available by the authors, without undue reservation.
Author Contributions
This research was planned, designed, and written by MSR with the contribution from CS and AW. The NMR characterization and data interpretation were conducted by SR and DK. This project was supervised by CS and AW. All authors contributed to the article and approved the submitted version.
Conflict of Interest
CS was employed by the company AgriSci Pty Ltd, Rutherglen, Victoria, Australia.
The remaining authors declare that the research was conducted in the absence of any commercial or financial relationships that could be construed as a potential conflict of interest
Acknowledgments
We acknowledge the Melbourne Research Scholarship to support this research and Melbourne TrACEES platform (soil) to assist and help in the analysis of the sample. We also wish to thank Associate Professor Graham Brodie for assistance in the pyrolysis preparation of the organic materials. We also wish to extend our gratitude to Professor Ji-Zheng He for supporting the publication fees.
Supplementary Material
The Supplementary Material for this article can be found online at: https://www.frontiersin.org/articles/10.3389/fsufs.2020.609788/full#supplementary-material
References
Adeleke, R., Nwangburuka, C., and Oboirien, B. (2017). Origins, roles and fate of organic acids in soils: a review. S. Afr. J. Bot. 108, 393–406. doi: 10.1016/j.sajb.2016.09.002
Akers, M. D. (2018). Exploring, Analysing and Interpeting Data with Minitab 18. Seoul: Compass Publishing.
Albrecht, R., Ziarelli, F., Alarcón-Gutiérrez, E., Le Petit, J., Terrom, G., and Perissol, C. (2008). 13C solid-state NMR assessment of decomposition pattern during co-composting of sewage sludge and green wastes. Eur. J. Soil Sci. 59, 445–452. doi: 10.1111/j.1365-2389.2007.00993.x
Al-Faiyz, Y. S. (2017). CPMAS 13C NMR characterization of humic acids from composted agricultural Saudi waste. Arabian J. Chem. 10, S839–S853. doi: 10.1016/j.arabjc.2012.12.018
Almendros, G., Knicker, H., and González-Vila, F. J. (2003). Rearrangement of carbon and nitrogen forms in peat after progressive thermal oxidation as determined by solid-state 13C -and 15N-NMR spectroscopy. Org. Geochem. 34, 1559–1568. doi: 10.1016/S0146-6380(03)00152-9
Andrade, F. V., Mendonça, E. D. S., and Silva, I. R. D. (2013). Organic acid adsorption and mineralization in oxisols with different textures. Rev. Bras. Ciência do Solo 37, 976–985. doi: 10.1590/S0100-06832013000400015
Baldock, J., Oades, J., Nelson, P., Skene, T., Golchin, A., and Clarke, P. (1997). Assessing the extent of decomposition of natural organic materials using solid-state 13C NMR spectroscopy. Soil Res. 35, 1061–1084. doi: 10.1071/S97004
Baldock, J. A., and Smernik, R. J. (2002). Chemical composition and bioavailability of thermally altered Pinus resinosa (Red pine) wood. Org. Geochem. 33, 1093–1109. doi: 10.1016/S0146-6380(02)00062-1
Berg, B., and Mcclaugherty, C. (2008). Plant litter. Decomposition, Humus Formation, Carbon Sequestration, 2nd Edn. Berlin; Heidelberg: Springer. doi: 10.1007/978-3-540-74923-3
Bernal, M., Sanchez-Monedero, M., Paredes, C., and Roig, A. (1998). Carbon mineralization from organic wastes at different composting stages during their incubation with soil. Agric. Ecosystems Environ. 69, 175–189. doi: 10.1016/S0167-8809(98)00106-6
Bolan, N. S., Naidu, R., Mahimairaja, S., and Baskaran, S. (1994). Influence of low-molecular-weight organic acids on the solubilization of phosphates. Biol. Fertil. Soils 18, 311–319. doi: 10.1007/BF00570634
Borie, F., and Zunino, H. (1983). Organic matter-phosphorus associations as a sink in P-fixation processes in allophanic soils of Chile. Soil Biol. Biochem. 15, 599–603. doi: 10.1016/0038-0717(83)90056-1
Celi, L., Lamacchia, S., Marsan, F. A., and Barberis, E. (1999). Interaction of inositol hexaphosphate on clays: adsorption and charging phenomena. Soil Sci. 164, 574–585. doi: 10.1097/00010694-199908000-00005
Chefetz, B., Hatcher, P. G., Hadar, Y., and Chen, Y. (1996). Chemical and biological characterization of organic matter during composting of municipal solid waste. J. Environ. Qual. 25, 776–785. doi: 10.2134/jeq1996.00472425002500040018x
Chen, C., Condron, L., Turner, B., Mahieu, N., Davis, M., Xu, Z., et al. (2004). Mineralisation of soil orthophosphate monoesters under pine seedlings and ryegrass. Soil Res. 42, 189–196. doi: 10.1071/SR03018
Cheng, C.-H., Lehmann, J., and Engelhard, M. H. (2008). Natural oxidation of black carbon in soils: changes in molecular form and surface charge along a climosequence. Geochim. Cosmochim. Acta 72, 1598–1610. doi: 10.1016/j.gca.2008.01.010
Dai, Z., Zhang, X., Tang, C., Muhammad, N., Wu, J., Brookes, P. C., et al. (2017). Potential role of biochars in decreasing soil acidification-a critical review. Sci. Total Environ. 581, 601–611. doi: 10.1016/j.scitotenv.2016.12.169
Delhaize, E., Ryan, P. R., and Randall, P. J. (1993). Aluminum tolerance in wheat (Triticum aestivum L.) (II. Aluminum-stimulated excretion of malic acid from root apices). Plant Physiol. 103, 695–702. doi: 10.1104/pp.103.3.695
Demirbas, A. (2004). Effects of temperature and particle size on bio-char yield from pyrolysis of agricultural residues. J. Anal. Appl. Pyrolysis 72, 243–248. doi: 10.1016/j.jaap.2004.07.003
Eldridge, S. M., Chen, C., Xu, Z., Nelson, P., Boyd, S., Meszaros, I., et al. (2013). Molecular composition of recycled organic wastes, as determined by solid-state 13C NMR and elemental analyses. Waste Manag. 33, 2157–2169. doi: 10.1016/j.wasman.2013.06.013
Fink, J. R., Inda, A. V., Tiecher, T., and Barrón, V. (2016). Iron oxides and organic matter on soil phosphorus availability. Ciencia e Agrotecnologia 40, 369–379. doi: 10.1590/1413-70542016404023016
Fischer, H., Eckhardt, K. U., Meyer, A., Neumann, G., Leinweber, P., Fischer, K., et al. (2010). Rhizodeposition of maize: short-term carbon budget and composition. J. Plant Nutr. Soil Sci. 173, 67–79. doi: 10.1002/jpln.200800293
Galvez-Sola, L., Morales, J., Mayoral, A., Marhuenda-Egea, F., Martinez-Sabater, E., Perez-Murcia, M., et al. (2010). Estimation of phosphorus content and dynamics during composting: use of near infrared spectroscopy. Chemosphere 78, 13–21. doi: 10.1016/j.chemosphere.2009.09.059
Geckeis, H., Rabung, T., Manh, T. N., Kim, J., and Beck, H. (2002). Humic colloid-borne natural polyvalent metal ions: dissociation experiment. Environ. Sci. Technol. 36, 2946–2952. doi: 10.1021/es010326n
Glendinning, J. (2000). Australian Soil Fertility Manual. Collingwood, VIC: Fertilizer Industry Federation of Australia. Inc. and CRISO.
Haider, K., Martin, J., and Filip, Z. (1975). “Humus biochemistry” in Soil Biochemistry, Vol. 4, eds E. A. Paul, and A.D. Me Laren (New York, NY: Marcel Dekker Inc), 195–244.
Hansen, J. C., Cade-Menun, B. J., and Strawn, D. G. (2004). Phosphorus speciation in manure-amended alkaline soils. J. Environ. Qual. 33, 1521–1527. doi: 10.2134/jeq2004.1521
Hansen, J. C., and Strawn, D. G. (2003). Kinetics of phosphorus release from manure-amended alkaline soil. Soil Sci. 168, 869–879. doi: 10.1097/01.ss.0000106408.84926.8f
Haynes, R., and Swift, R. (1989). Effect of rewetting air-dried soils on pH and accumulation of mineral nitrogen. Eur. J. Soil Sci. 40, 341–347. doi: 10.1111/j.1365-2389.1989.tb01278.x
Haynes, R. J., and Mokolobate, M. S. (2001). Amelioration of Al toxicity and P deficiency in acid soils by additions of organic residues: a critical review of the phenomenon and the mechanisms involved. Nutr. Cycling Agroecosyst. 59, 47–63. doi: 10.1023/A:1009823600950
Hoffland, E. (1992). Quantitative evaluation of the role of organic acid exudation in the mobilization of rock phosphate by rape. Plant Soil 140, 279–289. doi: 10.1007/BF00010605
Hu, H., Tang, C., and Rengel, Z. (2005a). Influence of phenolic acids on phosphorus mobilisation in acidic and calcareous soils. Plant Soil 268. 173–180. doi: 10.1007/s11104-004-0280-x
Hu, H., Tang, C., and Rengel, Z. (2005b). Role of phenolics and organic acids in phosphorus mobilization in calcareous and acidic soils. Journal Plant Nutr. 28, 1427–1439. doi: 10.1081/PLN-200067506
Huang, P. (2004). Soil mineral–organic matter–microorganism interactions: fundamentals and impacts. Adv. Agron. 82, 391–472. doi: 10.1016/S0065-2113(03)82006-0
Huang, R., Fang, C., Lu, X., Jiang, R., and Tang, Y. (2017). Transformation of phosphorus during (hydro) thermal treatments of solid biowastes: reaction mechanisms and implications for P reclamation and recycling. Environ. Sci. Technol. 51, 10284–10298. doi: 10.1021/acs.est.7b02011
Hue, N. (1991). Effects of organic acids/anions on P sorption and phytoavailability in soils with different mineralogies. Soil Sci. 152, 463–471. doi: 10.1097/00010694-199112000-00009
Hue, N. (1992). Correcting soil acidity of a highly weathered Ultisol with chicken manure and sewage sludge. Commun. Soil Sci. Plant Anal. 23, 241–264. doi: 10.1080/00103629209368586
Hue, N., Craddock, G., and Adams, F. (1986). Effect of organic acids on aluminum toxicity in subsoils. Soil Sci. Soc. Am. J. 50, 28–34. doi: 10.2136/sssaj1986.03615995005000010006x
Hunger, S., Cho, H., Sims, J. T., and Sparks, D. L. (2004). Direct speciation of phosphorus in alum-amended poultry litter: solid-state 31P NMR investigation. Environ. Sci. Technol. 38, 674–681. doi: 10.1021/es034755s
Hunger, S., Sims, J. T., and Sparks, D. L. (2008). Evidence for struvite in poultry litter: effect of storage and drying. J. Environ. Qual. 37, 1617–1625. doi: 10.2134/jeq2007.0331
Idowu, I., Li, L., Flora, J. R., Pellechia, P. J., Darko, S. A., Ro, K. S., et al. (2017). Hydrothermal carbonization of food waste for nutrient recovery and reuse. Waste Manag. 69, 480–491. doi: 10.1016/j.wasman.2017.08.051
Isbell, R. (2016). The Australian Soil Classification. Collingwood, VIC: CSIRO Publishing. doi: 10.1071/9781486304646
Isbell, R., Mcdonald, W. S., and Ashton, L. J. (1997). Concepts and Rationale of the Australian soil Classification. Canberra, ACT: CSIRO Land and Water, 152.
Iyamuremye, F., Dick, R., and Baham, J. (1996). Organic amendments and phosphorus dynamics: I. Phosphorus chemistry and sorption. Soil Sci. 161, 426–435. doi: 10.1097/00010694-199607000-00002
Jones, D., Dennis, P., Owen, A., and Van Hees, P. (2003). Organic acid behavior in soils–misconceptions and knowledge gaps. Plant Soil 248, 31–41. doi: 10.1023/A:1022304332313
Jones, D., and Edwards, A. (1998). Influence of sorption on the biological utilization of two simple carbon substrates. Soil Biol. Biochem. 30, 1895–1902. doi: 10.1016/S0038-0717(98)00060-1
Jones, D. L. (1998). Organic acids in the rhizosphere–a critical review. Plant Soil 205, 25–44. doi: 10.1023/A:1004356007312
Juo, A. S., and Franzluebbers, K. (2003). Tropical Soils: Properties and Management for Sustainable Agriculture. Oxford: Oxford University Press. doi: 10.1093/oso/9780195115987.001.0001
Kassim, G., Stott, D. E., Martin, J., and Haider, K. (1982). Stabilization and Incorporation into biomass of phenolic and benzenoid carbons during biodegradation in soil. Soil Sci. Soc. Am. J. 46, 305–309. doi: 10.2136/sssaj1982.03615995004600020018x
Kaudal, B. B., Aponte, C., and Brodie, G. (2018). Biochar from biosolids microwaved-pyrolysis: characteristics and potential for use as growing media amendment. J. Anal. Appl. Pyrolysis 130, 181–189. doi: 10.1016/j.jaap.2018.01.011
Keating, K., and Knight, R. (2010). A laboratory study of the effect of Fe (II)-bearing minerals on nuclear magnetic resonance (NMR) relaxation measurements. Geophysics 75, F71–F82. doi: 10.1190/1.3386573
Kwabiah, A., Stoskopf, N., Palm, C., Voroney, R., Rao, M., and Gacheru, E. (2003). Phosphorus availability and maize response to organic and inorganic fertilizer inputs in a short term study in western Kenya. Agric. Ecosyst. Environ. 95, 49–59. doi: 10.1016/S0167-8809(02)00167-6
Levesque, M., and Schnitzer, M. (1967). Organometallic interactions in soils: 6. Preparation and properties of fulvic acid-metal phosphates. Soil Sci. 103, 183–190. doi: 10.1097/00010694-196703000-00006
Leytem, A. B., and Maguire, R. O. (2007). “Environmental implications of inositol phosphates in animal manures,” in Inositol Phosphates, eds B. L. Turner, A. E. Richardson, and E. J. Mullaney (Wallingford: CABI), 150. doi: 10.1079/9781845931520.0150
Li, G., Li, H., Leffelaar, P. A., Shen, J., and Zhang, F. (2014). Characterization of phosphorus in animal manures collected from three (dairy, swine, and broiler) farms in China. PLoS ONE 9:e102698. doi: 10.1371/journal.pone.0102698
Li, W., Feng, X., Song, W., and Guo, M. (2018). Transformation of phosphorus in speciation and bioavailability during converting poultry litter to biochar. Front. Sust. Food Syst. 2:20. doi: 10.3389/fsufs.2018.00020
Lindegren, M., and Persson, P. (2009). Competitive adsorption between phosphate and carboxylic acids: quantitative effects and molecular mechanisms. Eur. J. Soil Sci. 60, 982–993. doi: 10.1111/j.1365-2389.2009.01171.x
Mackay, J., Macdonald, L., Smernik, R., and Cavagnaro, T. (2017). Organic amendments as phosphorus fertilisers: chemical analyses, biological processes and plant P uptake. Soil Biol. Biochem. 107, 50–59. doi: 10.1016/j.soilbio.2016.12.008
Marra, R., Vinale, F., Cesarano, G., Lombardi, N., D'errico, G., Crasto, A., et al. (2018). Biochars from olive mill waste have contrasting effects on plants, fungi and phytoparasitic nematodes. PloS ONE 13:e0198728. doi: 10.1371/journal.pone.0198728
Martinez, M., Romero, C., and Gavilan, J. (1984). Solubilization of phosphorus by humic acids from lignite. Soil Sci. 138, 257–261. doi: 10.1097/00010694-198410000-00001
Mayans, B., Pérez-Esteban, J., Escolástico, C., Eymar, E., and Masaguer, A. (2019). Evaluation of commercial humic substances and other organic amendments for the immobilization of copper through 13C CPMAS NMR, FT-IR, and DSC Analyses. Agronomy 9:762. doi: 10.3390/agronomy9110762
Micales, J. A. (1997). Localization and induction of oxalate decarboxylase in the brown-rot wood decay fungus Postia placenta. Int. Biodeterior. Biodegradation 39, 125–132. doi: 10.1016/S0964-8305(97)00009-7
Mnkeni, P., and Mackenzie, A. (1985). Retention of ortho-and polyphosphates in some Quebec soils as affected by added organic residues and calcium carbonate. Can. J. Soil Sci. 65, 575–585. doi: 10.4141/cjss85-061
Morcombe, C. R., and Zilm, K. W. (2003). Chemical shift referencing in MAS solid state NMR. J. Magn. Reson. 162, 479–486. doi: 10.1016/S1090-7807(03)00082-X
Murphy, J. A., and Riley, J. P. (1962). A modified single solution method for the determination of phosphate in natural waters. Anal. Chim. Acta 27, 31–36. doi: 10.1016/S0003-2670(00)88444-5
Penn, C. J., and Camberato, J. J. (2019). A critical review on soil chemical processes that control how soil pH affects phosphorus availability to plants. Agriculture 9:120. doi: 10.3390/agriculture9060120
Penn, C. J., Rutter, E. B., Arnall, D. B., Camberato, J., Williams, M., and Watkins, P. (2018). A discussion on mehlich-3 phosphorus extraction from the perspective of governing chemical reactions and phases: impact of soil pH. Agriculture 8:106. doi: 10.3390/agriculture8070106
Rahman, M. S., Quadir, Q. F., Rahman, A., Asha, M. N., and Chowdhury, M. A. K. (2014). Screening and characterization of Phosphorus solubilizing Bacteria and their effect on Rice seedlings. Res. Agric. Livestock Fisheries 1, 27–35. doi: 10.3329/ralf.v1i1.22353
Rayment, G. E., and Lyons, D. J. (2011). Soil Chemical Methods: Australasia. Collingwood, VIC: CSIRO Publishing. doi: 10.1071/9780643101364
Ritchie, G., and Dolling, P. (1985). The role of organic matter in soil acidification. Soil Res. 23, 569–576. doi: 10.1071/SR9850569
Robinson, J. S., Baumann, K., Hu, Y., Hagemann, P., Kebelmann, L., and Leinweber, P. (2018). Phosphorus transformations in plant-based and bio-waste materials induced by pyrolysis. Ambio 47, 73–82. doi: 10.1007/s13280-017-0990-y
Rose, T. J., Schefe, C., Weng, Z. H., Rose, M. T., Van Zwieten, L., Liu, L., et al. (2019). Phosphorus speciation and bioavailability in diverse biochars. Plant Soil 443, 233–244. doi: 10.1007/s11104-019-04219-2
Schefe, C., Kappen, P., Zuin, L., Pigram, P. J., and Christensen, C. (2009). Addition of carboxylic acids modifies phosphate sorption on soil and boehmite surfaces: a solution chemistry and XANES spectroscopy study. J. Colloid Interface Sci. 330, 51–59. doi: 10.1016/j.jcis.2008.10.044
Schefe, C. R., Kappen, P., and Pigram, P. J. (2011). Carboxylic acids affect sorption and micro-scale distribution of phosphorus in an acidic soil. Soil Sci. Soc. Am. J. 75, 35–44. doi: 10.2136/sssaj2010.0068
Schefe, C. R., Patti, A. F., Clune, T. S., and Jackson, R. (2008). Organic amendments increase soil solution phosphate concentrations in an acid soil: a controlled environment study. Soil science. 173, 267–276. doi: 10.1097/SS.0b013e31816d1e3b
Schefe, C. R., and Tymms, K. (2013). Phased addition of organic and phenolic acids with phosphate fertiliser increases P availability in an acid soil. Soil Res. 51:437. doi: 10.1071/SR13126
Shindo, H., and Kuwatsuka, S. (1976). Behavior of phenolic substances in the decaying process of plants: IV. Adsorption and movement of phenolic acids in soils. Soil Sci. Plant Nutr. 22, 23–33. doi: 10.1080/00380768.1976.10432964
Sibanda, H., and Young, S. (1986). Competitive adsorption of humus acids and phosphate on goethite, gibbsite and two tropical soils. Eur. J. Soil Sci. 37, 197–204. doi: 10.1111/j.1365-2389.1986.tb00020.x
Siddique, M. T., and Robinson, J. S. (2003). Phosphorus sorption and availability in soils amended with animal manures and sewage sludge. J. Environ. Qual. 32, 1114–1121. doi: 10.2134/jeq2003.1114
Siqueira, J. O., Nair, M. G., Hammerschmidt, R., Safir, G. R., and Putnam, A. R. (1991). Significance of phenolic compounds in plant-soil-microbial systems. Crit. Rev. Plant Sci. 10, 63–121. doi: 10.1080/07352689109382307
Skene, T., Skjemstad, J., Oades, J., and Clarke, P. (1996). The influence of inorganic matrices on the decomposition of straw. Soil Res. 34, 413–426. doi: 10.1071/SR9960413
Smit, A. L., Bindraban, P. S., Schröder, J. J., Conijn, J. G., and Van Der Meer, H. G. (2009). Phosphorus in agriculture: global resoources, trends and developments: report to the Steering Committee Technology Assessment of the Ministery of Agriculture, Nature and Food Quality, The Netherlands, and in collaboration with the Nutrient Flow Task Group (NFTG), supported by DPRN (Development Policy review Network) (No. 282). Plant Research International.
Song, W., and Guo, M. (2012). Quality variations of poultry litter biochar generated at different pyrolysis temperatures. J. Anal. Appl. Pyrolysis 94, 138–145. doi: 10.1016/j.jaap.2011.11.018
Spaccini, R., Mazzei, P., Squartini, A., Giannattasio, M., and Piccolo, A. (2012). Molecular properties of a fermented manure preparation used as field spray in biodynamic agriculture. Environ. Sci. Pollut. Res. 19, 4214–4225. doi: 10.1007/s11356-012-1022-x
Spaccini, R., and Piccolo, A. (2008). Spectroscopic characterization of compost at different maturity stages. Clean Soil Air Water 36, 152–157. doi: 10.1002/clen.200720012
Ström, L., Owen, A., Godbold, D., and Jones, D. (2001). Organic acid behaviour in a calcareous soil: sorption reactions and biodegradation rates. Soil Biol. Biochem. 33, 2125–2133. doi: 10.1016/S0038-0717(01)00146-8
Stutter, M. I. (2015). The composition, leaching, and sorption behavior of some alternative sources of phosphorus for soils. Ambio 44(Suppl. 2), S207–S216. doi: 10.1007/s13280-014-0615-7
Surampalli, R. Y., Zhang, T. C., Tyagi, R. D., Naidu, R., Gurjar, B. R., Ojha, C. S. P., et al. (2015). Carbon Capture and Storage: Physical, Chemical, and Biological Methods. Reston, VA: American Society of Civil Engineers (ASCE). doi: 10.1061/9780784413678
Sutton, C., and Larsen, S. (1964). Pyrophosphate as a source of phosphorus for plants. Soil Sci. 97, 196–201. doi: 10.1097/00010694-196403000-00008
Tapia, Y., Cala, V., Eymar, E., Frutos, I., Gárate, A., and Masaguer, A. (2010). Chemical characterization and evaluation of composts as organic amendments for immobilizing cadmium. Biores. Technol. 101, 5437–5443. doi: 10.1016/j.biortech.2010.02.034
Turner, B. L. (2004). Optimizing phosphorus characterization in animal manures by solution phosphorus-31 nuclear magnetic resonance spectroscopy. J. Environ. Qual. 33, 757–766. doi: 10.2134/jeq2004.7570
Turner, B. L., Cade-Menun, B. J., and Westermann, D. T. (2003). Organic phosphorus composition and potential bioavailability in semi-arid arable soils of the western United States. Soil Sci. Soc. Am. J. 67, 1168–1179. doi: 10.2136/sssaj2003.1168
Turner, B. L., Mahieu, N., Condron, L. M., and Chen, C. (2005). Quantification and bioavailability of scyllo-inositol hexakisphosphate in pasture soils. Soil Biol. Biochem. 37, 2155–2158. doi: 10.1016/j.soilbio.2005.03.005
Van Kauwenbergh, S. J. (2010). World Phosphate Rock Reserves and Resources. Muscle Shoals, AL: IFDC.
Victoria, E. P. A. (2004). Guidelines for Environmental Management: Biosolids Land Application. Carlton, VIC: EPA Victoria.
Violante, A., Colombo, C., and Buondonno, A. (1991). Competitive adsorption of phosphate and oxalate by aluminum oxides. Soil Sci. Soc. Am. J. 55, 65–70. doi: 10.2136/sssaj1991.03615995005500010011x
Von Uexküll, H., and Mutert, E. (1995). Global extent, development and economic impact of acid soils. Plant Soil 171, 1–15. doi: 10.1007/BF00009558
Waldrip, H. M., He, Z., and Erich, M. S. (2011). Effects of poultry manure amendment on phosphorus uptake by ryegrass, soil phosphorus fractions and phosphatase activity. Biol. Fertil. Soils 47, 407–418. doi: 10.1007/s00374-011-0546-4
Weber, K., and Quicker, P. (2018). Properties of biochar. Fuel 217, 240–261. doi: 10.1016/j.fuel.2017.12.054
White, R. (1981). Retention and Release of Phosphate by Soil and Soil Constituents [Prediction of phosphorus fertilizer requirements]. Soils and agriculture.
WRB (2015). World Reference Base for Soil Resources 2014, Update 2015: International Soil Classification System for Naming Soils and Creating Legends for Soil Maps. Rome: Fao.
Wu, H., Wu, L., Wang, J., Zhu, Q., Lin, S., Xu, J., et al. (2016). Mixed phenolic acids mediated proliferation of pathogens Talaromyces helicus and Kosakonia sacchari in continuously monocultured Radix pseudostellariae rhizosphere soil. Front. Microbiol. 7:335. doi: 10.3389/fmicb.2016.00335
Xu, G., Zhang, Y., Shao, H., and Sun, J. (2016). Pyrolysis temperature affects phosphorus transformation in biochar: chemical fractionation and 31P NMR analysis. Sci. Total Environ. 569, 65–72. doi: 10.1016/j.scitotenv.2016.06.081
Yan, F., Schubert, S., and Mengel, K. (1996). Soil pH increase due to biological decarboxylation of organic anions. Soil Biol. Biochem. 28, 617–624. doi: 10.1016/0038-0717(95)00180-8
Keywords: organic waste, microwave pyrolysis, biochar, compost, solid-state 13C NMR, solution-state 31P NMR
Citation: Rahman MS, Schefe C, Rajput S, Keizer D and Weatherley A (2021) O-aryl and Carbonyl Carbon Contents of Food Waste and Biosolid Predict P Availability in an Acidic Soil. Front. Sustain. Food Syst. 4:609788. doi: 10.3389/fsufs.2020.609788
Received: 24 September 2020; Accepted: 30 November 2020;
Published: 13 January 2021.
Edited by:
José Martinez, National Research Institute of Science and Technology for Environment and Agriculture (IRSTEA), FranceReviewed by:
Teresa Gea, Autonomous University of Barcelona, SpainJoão Coutinho, University of Trás-os-Montes and Alto Douro, Portugal
Copyright © 2021 Rahman, Schefe, Rajput, Keizer and Weatherley. This is an open-access article distributed under the terms of the Creative Commons Attribution License (CC BY). The use, distribution or reproduction in other forums is permitted, provided the original author(s) and the copyright owner(s) are credited and that the original publication in this journal is cited, in accordance with accepted academic practice. No use, distribution or reproduction is permitted which does not comply with these terms.
*Correspondence: Md. Shahinur Rahman, bXNyYmF1QGdtYWlsLmNvbQ==; Anthony Weatherley, YW50aG9ueUB1bmltZWxiLmVkdS5hdQ==