- 1Laboratorio de Microbiología Aplicada, Centro de Estudios Avanzados en Zonas Áridas CEAZA, La Serena, Chile
- 2Departamento de Agronomía, Universidad de La Serena, La Serena, Chile
- 3Biotecnología y Bioquímica, Cinvestav Irapuato, Irapuato, Mexico
- 4Biotecnología e Innovación Agroalimentaria, Instituto Tecnológico de Tepic, Tepic, Mexico
- 5INIA - Instituto de Investigaciones Agropecuarias, Santiago de Chile, Chile
- 6Instituto de Investigación Multidisciplinario en Ciencia y Tecnología, Universidad de la Serena, La Serena, Chile
The importance of phosphate solubilizing rhizobacteria (PSB) has been well-document as an option for enhancing sustainable agriculture. As a particular group of plant growth promoting rhizobacteria (PGPR), PSB play an important role in the soil phosphorus cycle, increasing the bioavailability for growth and plant development. This study analyses the plant growth promoting effects of 5 strains (BN0009, BN0013, BN0015, BN0024, and BN0035) out of 180 isolated from Jarava frigida (Phil.) F.Rojas (Poaceae), a native grass from the Andean Atacama desert from North of Chile. The five bacterial isolated (BN strains) were identified as non-pathogenic Erwinia sp. and show a high phosphate solubilization capacity for Ca(PO4) ranging from 608.9 to 781.4 mg/L. Strains IAA production varies between 23.5 and 35.9 mg/L, siderophores, phosphatase (alkaline and acid) production was also observed, but none of the five isolated presented antagonism against plant pathogens Botrytis sp. and Sclerotinia sp. All isolates enhanced seed germination in Lactuca sativa and Solanum lycopersicum (excepting BN009). Additionally, all strains stimulated the early root elongation and seedling development in lettuce and tomato. Pot experiments displayed that BN0015, BN0024, and BN0035 significantly promote plant growth regarding root and leaf area, root and leaf weight, as well as leaf number compared with non-treated plants. In a field experiment with lettuce and two fertilization treatments (50 and 100% of the recommended crop fertilization), BN0024 application improved crop productivity compared to respective control. P content in plants with bacterial inoculations increased significantly compared to control in either fertilization treatment, suggesting an improved nutrient uptake. Also, lettuce with 50% fertilization and inoculation with BN0024 equate productivity with the control 100% fertilization. Finally, we discuss these results in the context of applicability to enhance the agroecosystem productivity in arid and semiarid zones.
Introduction
The rhizosphere involves dynamic interactions between plant roots and soil microorganisms, where the composition of root exudates influences the nature and structure of the microbial community (Shi et al., 2011; Eisenhauer et al., 2017; López-Angulo et al., 2020). Particularly, beneficial soil microorganisms like mycorrhiza and Plant Growth Promotion Rhizobacteria (PGPR) enhance the adaptive responses and plant development by direct and indirect mechanisms (Backer et al., 2018). PGPR facilitate the bioavailability of nutrients in the soil, e.g., phosphate, considered a major macronutrient for growth and physiological plant performance (Bechtaoui et al., 2020). However, it is well-known that the bioavailable P in the soil is very limited reaching the level of 1.0 mg kg-1 soil (Goldstein, 1994). To raise assimilable P, conventional agriculture has been using chemical fertilizers. Nonetheless, the efficiency of P fertilizer throughout the world is around 10–25%, as a large proportion of the applied P is quickly transformed into insoluble forms, limiting the crop productivity and increasing environmental pollution (Kanter and Brownlie, 2019; Mallin and Cahoon, 2020). Phosphate-solubilizing bacteria (PSB) mobilize insoluble P forms from the mineral matrix to the bulk soil where they can be absorbed by plant roots (Sashidhar and Podile, 2010). In turn, plants liberate several sources of carbohydrates, which can be metabolized for bacterial activity (Vives-Peris et al., 2020). PSB can produce various organic acids with low molecular weight, which alter the soil pH, solubilizing the phosphate from acid or alkaline soils (Kim et al., 1998; Gyaneshwar et al., 2002; Liu et al., 2020). Another possible mechanism is proton-excretion accompanying ammonium ion assimilation, which is assumed to be the most probable explanation for microbial solubilization without acid production (Illmer and Schinner, 1995). Species of the bacterial genera Aerobacter, Acetinobacter, Azospirullum, Bacillus, Burkholderia, Erwinia, Pseudomonas, and Rhizobium, have been widely reported to be able to solubilize various forms of insoluble phosphates (Ranjan et al., 2013; Goswami et al., 2016). Additionally, the use of PSB alone or in combined bioformulations with mycorrhizal fungi has been proven to be highly effective in increasing soil phosphate availability and to improve crop productivity (Singh and Kapoor, 1999; Nacoon et al., 2020). Different authors demonstrated these effects for several crops, like cotton (Qureschi et al., 2012), maize (Yazdani et al., 2009), sunflower Ekin (2010), rice (Rasul et al., 2019), or faba bean (Bechtaoui et al., 2020). Due to the activity that PSB expose by solubilizing the phosphate ion trapped in the soil constituents, the seed bacterization, and the preparation of specific biofertilizer with PSB strains are emerging as a potential strategy to promote the transition to sustainable agriculture (Babalola, 2010; Parani and Saha, 2012).
In Chile, soils of the Atacama Desert and adjacent regions are predominantly calcareous, poor in available phosphate, organic matter, and carbon content (Gómez-Silva et al., 2008). However, the presence of a high diversity of native plants adapted to arid environments and the high percentage of endemic organisms justified the consideration as one of the 35 hotspots of biodiversity around the world (Mittermeier et al., 2005). This fact suggests the presence of ecological networks that harbor bacterial strains with high biotechnical potential and beneficial effects on the productivity and growth of crops, which are grown on arid or semiarid agroecosystem. In fact, some PSB strains, isolated from arid environment, demonstrated promising applicability in agriculture (Goswami et al., 2014; Emami et al., 2020; Wahid et al., 2020).
Despite the benefits that PSB represent to the plants, the information about biotechnological applications of native microorganism, especially from Chile, still is very scarce. Therefore, the present work aims to contribute to our knowledge about PSB, their functional activity as well as potential uses in modern agriculture. We characterize PSB associated to the roots of Jarava frigida (Phil.) F.Rojas (Poaceae), a native grass that dominates the high Andean desert grasslands (Gajardo, 1994). We analyse their P solubilizing capacity and their ability to promote growth of lettuce and tomato plants; and select the best strain for a field experiment with different levels of fertilization. Finally, we discuss their applicability as biofertilizers for agriculture in arid and semi-arid zones.
Materials and Methods
Rhizospheric Soil Sampling
For this study, we sampled a Jarava frigida population (23°02′50"S−67°39′16"W) at an altitude of 4,650 m above sea level, located in the commune of San Pedro de Atacama (Antofagasta, Chile). The sampling site is a little terrain depression with an average vegetation cover of 5%, the mean annual temperature is 7°C, and annual precipitation is ~120–150 mm. Plants of J. frigida were harvested from the study site with a spade. Their root-sediment ball was kept intact, and they were transferred to the laboratory in sterile plastic bags. Besides the rhizoplane-root sample, soil samples were taken. Soil chemistry was analyzed by a commercial laboratory (CTSyC, University of Talca).
Soil chemistry: The pH of Atacama Desert soil samples was moderately acidic (Table 1). The total N and P level was 103 ppm v/s 0.027%, respectively. The soil samples exposed a low level of organic matter (0.63%). However, the organic N level was 0.6 times higher compared to organic P. (16 ppm v/s 10 ppm, respectively). The electric conductivity (EC), expressed as a ds/m measurement was low, but a high concentration of minerals was detected particularly for iron and copper (Table 1). In addition, a high concentration of boron was also measured, a common feature of desert regions.
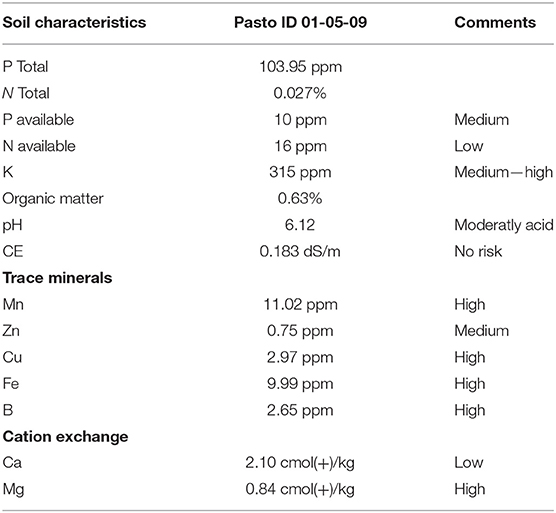
Table 1. Soil characteristics from the isolate collection site in San Pedro de Atacama (as indicated by CTSyC laboratory).
Isolation of Phosphate Solubilizing Bacteria
Approximately 5 g of roots and rhizosphere (soil attached to the roots) were placed in an Erlenmeyer flask with 50 ml distilled water and shook at 120 rpm at room temperature for 2 h. Serial diluted (103–105) samples were dispersed with borosilicate glass balls on PVK plates, containing glucose 10 g/l, yeast extract 0.5 g/l, ammonium sulfate 0.5 g/l, magnesium sulfate heptahydrate 0.1 g/l, calcium phosphate 5 g/l, sodium chloride 0.2 g/l, potassium chloride 0.2 g/l, manganese sulfate 0.002 g/l, ferrous sulfate 0.002 g/l, agar 15 g/l, pH adjusted to 7 (Vazquez et al., 2000). Plates were incubated for 3 days at 25°C. Afterward, solubilizing strains were picked and purified. Their solubilizing efficiency on PVK plates (triplicates) was expressed as E = halo diameter/colony diameter × 100 (Nguyen et al., 1992). All isolates with ratio >200 were stored at −80°C.
Biochemical Characterization of PGPR Traits
Quantification of Solubilized Phosphate
The isolates were inoculated into 20 ml liquid PVK medium (as mentioned above but without agar), where Ca3(PO4)2 was replaced by KH2PO4 and incubated at 28°C for 24 h. Bacterial concentration of this liquid culture was adjusted to in 1 × 105 CFU in 500 μl and bacterial cells were cleaned. Finally, respectively, 50 ml of liquid PVK per isolate were inoculated and incubated for 72 h with continuous agitation (125 r/min). Every 12 h 1 ml culture was sampled aseptically for the determination of pH and soluble phosphorus. The pH was measured on a pH meter; the phosphorus availability was determined with the Mo blue method (Watanabe and Olsen, 1965). The solubilizing isolates were compared against a negative control and a not-solubilizing isolate. All measurements were taken in triplicates and the procedure was repeated 3 times independently.
Alkaline and Acid Phosphatase
To detect alkaline and acid phosphatase activity isolates were cultivated on PVK plates (composition mentioned above) for 4 days at 25°C. Afterwards, a 6 mM 4-nitrophenyl phosphate solution was added to the plates containing either acetic acid (100 mM, pH 5.4) for acid phosphatase or Tris (100 mM, pH 9) for alkaline phosphatase. In both cases, plates were incubated for 90 min., inverted and exposed to ammoniac vapor for 2–3 min. under an extractor hood. The formation of a yellow halo around bacterial colonies indicates phosphatase activity by reduction of 4-nitrophenyl phosphate (colorless) to p-nitrophenol (yellow).
Siderophores
To determine the excretion of siderophores by the strains, CAS (chrome azurol sulfur) plates were used as described by Alexander and Zuberer (1991). Bacterial strains were inoculated on the plates and incubated for 72 h. The observation of an orange halo indicates the liberation of iron from the colored Fe-CAS complex due to the presence of siderophores produced by the bacteria. Afterwards CAS-positive bacteria were grown in MM medium and incubated for 24 h (at 25°C, 120 rpm). One milliliter of the culture was centrifuged at 8,000 rpm for 5 min, the cell-free supernatant was mixed with 0.5 ml CAS solution and measured at 630 nm (spectrophotometer), as reference a 0.5 ml uninoculated MM medium and 0.5 ml CAS solution was used (Schwyn and Neilands, 1987). The percentage of siderophore units was estimated as the proportion of CAS color shifted using the formula: % Siderophore units = [(absorbance reference -absorbance sample)/absorbance reference] × 100 (Bholay et al., 2012).
IAA Production
For each strain, 10 ml liquid PY medium containing Tryptophan (0.1% p/v) were inoculated and incubated for 48 h at 25°C and 175 rpm. The production of Indole-3-acetic acid (IAA) was determined after 24 and 48 h with or without tryptophan. For each measurement, 1 ml bacterial culture was centrifuged to 0.5 ml of the supernatant 1 ml of Salkowsky reagent (1 ml FeCl3 0,5 M mixed with 50 ml 35% (p/p) HClO4; Gordon and Weber, 1951) was added and incubated in darkness for 30 min (Glickmann and Dessaeux, 1995). Absorbance was measured at 530 nm against a blank [PY medium + Tryptophan (0.1% p/v)]. The IAA concentration was calculated based on a standard curve calibrated with dilutions (0–100 μg/ml) of a commercial IAA reagent (Merck).
ACC-Desaminase Activity
The capacity to produce ACC deaminase was evaluated qualitatively by placing the isolates on Dworking-Foster plates with ACC as sole nitrogen source (Penrose and Glick, 2003). Bacterial growth on the plate would indicate the presence of the enzyme.
N-Fixation Ability
The capacity to fix aerial nitrogen was determined using NFb semi-solid medium according to Baldani et al. (2014).
Antagonism Capacity
To evaluate the antagonistic capacity of the strains against Sclerotina sp. and Botrytis sp., we employed the methodology of Salvatierra-Martinez et al. (2018). Briefly, PDA medium Petri dishes were used, placing in the center of each plate, a disc of fungal hyphae (7-d-old, 0.5 cm diameter) surrounded at a distance of 4 cm by five 3 μl liquid culture aliquots of each strain. The strain liquid culture was prepared in LB, grown for 24 h, and diluted 1:10. As control, plates with only a disc of Sclerotina sp. or Botrytis sp. in the center were used. All plates were incubated at 25°C for 5 days. The inhibition of the radial growth of the fungi was calculated using the equation: ICM = [(C–T)/C] × 100; where C = the growth area of the fungus in the control plate and T = the fungal growth area in the treatment (Fiume and Fiume, 2006). The experiment was repeated three times with 3 plates per replication for each strain.
Strain Identity
To determine the taxonomical identity of our strains we carried out several tests. For the Gram reaction, we used the commercial stain kit (Merck, Company) and KOH test. Additionally, photographs and observations of cell size and morphology were made for each isolate, using a light microscope and the 100 × objective lens.
Finally, we extracted genomic DNA for each pure strain, following the protocol of Yeates et al. (1998). We amplified 16S region (63F (5′-CAG GCC TAA CAC ATG CAA GTC-3′) and 1387R (5′-GGG CGG WGT GTA CAA GGC-3′; ~1,000 bp) with the primer pair and PCR protocol as reported by Marchesi et al. (1998). Purified fragments were sent to Macrogen for sequencing, and the resulting 16S rRNA gene sequences were compared with the NCBI database using the basic local alignment search tool (Zhang et al., 2000). The phylogenetic analysis was performed using MEGA 7 (Kumar et al., 2016). The obtained sequences are available in the NCBI database (NCBI accession numbers: MW092897, MW092898, MW092899, MW092900, and MW092901; not released yet).
Promotion of Plant Growth
The effects of the bacterial isolates on plant growth were evaluated using Lactuca sativa L. (lettuce) and Solanum lycopersicum L. (tomato).
Seed Germination Assays
Seeds [L. sativa (lettuce) and S. lycopersicum (tomato)] were sterilized with sodium hypochlorite and vernalized for 48 h at 4°C. Afterwards, they were submerged in liquid bacterial culture [LB medium, OD = 0.8 (≈ 107 CFU)] for 5 h at room temperature on a shaker (180 rpm). Twenty-five prepared lettuce or 15 prepared tomato seeds were displaced in a Petri dish on humid filter paper (each isolate in quadruplicate, negative control without bacteria). Petri dishes were incubated horizontally at 20°C for 3.5 days (lettuce) and 14 days (tomato), respectively. Observations on germination progress were made every 4 h for lettuce and every 8 h for tomato. Root length was determined at the end of the respective incubation period, using the scale: no root, radicle emerged, radicle <1 cm and root > 1 cm.
Pot Experiment With Lettuce
For this experiment, the five selected bacterial strains (BN0009, BN0013, BN0015, BN0024, and BN0035) were grown on nutrient agar initially. A single colony was transferred into 250 ml flasks containing nutrient broth and grown aerobically on a shaker (150 pm) for 24 h at 28°C. The bacterial suspension was then diluted in sterile distilled water to a final concentration of 108 CFU/ml. Sterile seeds were submerged for 5 h in this bacterial solution. Inoculated seeds were placed in a pot with a 1:1 sterile mixture of agricultural soil and peat. For each isolate, 10 pots were prepared (replicates) and located randomly. Pots were maintained in a climate chamber at 20°C for 28 days (photoperiod: 16 h light, 8 h darkness). The pots were irrigated daily 5 ml with distillate water. As negative control, 20 pots containing seeds without bacteria were cultured under the same conditions. Photographs were taken every 5 days. At the end of the incubation period, root and leaf area (using WinRhizo), number of leaves, as well as root and leaf fresh and dry weight were recorded. Root and shoot portions of lettuce plants were dried separately for 5 days at 65°C (constant weight).
Lettuce Field Experiment
Based on the previous results, one strain was selected for the lettuce field experiment. Just before transplantation, lettuce seedlings (28 days old) were soaked for 1 h in a bacterial suspension. Therefore, the strain was grown in liquid LB medium in a flask on a shaker (150 pm) for 24 h at 28°C and this bacterial culture was diluted afterwards to the final concentration of 108 CFU/ml. The strain was re-inoculated 4 and 8 weeks after the transplant, via drip irrigation adjusting the concentration at the dripper to 108 CFU/ml for 10 min (a separate irrigation system was installed for these applications). We considered two fertilization treatments with respect to the literature indication for crop management of lettuce (INIA, 2012): 100% (NPK ratio 100-100-80) and 50% (NPK ratio 50-50-40), using KNO3, NH4H2PO4, and NH4NO3 diluted in water for applications during drip irrigation. In total, we established four treatments: control 100% fertilization, control 50% fertilization, BN0024 100% fertilization and BN0024 50% fertilization. After 3 months lettuce was cropped and 10 individuals per treatment (randomly picked) were used for analyses. Growth parameters (root and aerial weight, root area) and biochemical parameters (leaf P content (Hylander et al., 1996), protein content (Bradford, 1976), and chlorophyll content (Porra et al., 1989) were measured.
Statistical Analyses
The experimental data were analyzed regarding homogeneity (Pettitt test), variances (Barlett test), descriptive statistics (standard variation and error, median, mean, asymmetric coefficient, range, maximum, and minimum value). Afterwards, Tukey's range test and ANOVA were applied to determine means (from the bacterial treatments) that are significantly different from the control and/or other treatments (P < 0.05). As statistical and graphical software, XLSTAT, and SigmaPlot were used.
Results
Screening for Phosphate Solubilizing Bacteria and Quantification of Solubilized Phosphate
From Jarava frigida rhizosphere samples, 180 isolates were obtained on PVK plates. The isolates showed different colony morphology features such as color, density and colony elevation (Supplementary Table 1). A qualitative determination of the phosphate solubilization capacity was carried with the whole collection using PVK agar plates. Measurements of solubilization efficiency E ranged between 110 and 300, with an average of 172. Twenty-seven isolates demonstrated a solubilization efficiency of over 200 and were further characterized regarding their capacity to solubilize P in liquid medium (Supplementary Table 1). The five most efficient solubilizing isolates were selected for further analyses due to their outstanding E-values of 260 to 300 (Figure 1A). These isolates were labeled as BN0009, BN0013, BN0015, BN0024, and BN0035. Their colonies were described as round and creamy in color (Figure 1B). All BN isolates exposed a high growth capacity on LB medium, with a density peak after 16 h of incubation (Figure 1C). At the same time (16 h), the growth of these five isolates in PVK liquid medium containing a P soluble source is noticeable reduced (Figure 1D). For these selected isolates a quantification of solubilized P in a liquid medium was carried out, which demonstrated different levels in their capacity dissolving insoluble phosphate (Figure 2). The highest solubilization activity was observed between 36 and 48 h when 608.9–781.4 mg/L P were liberated from BN strains. A longer incubation time did not further increase the solubilized phosphate by each isolated. On the contrary, by the end of the experiment (72 h), the concentration of soluble P decreased slightly in all strains. At the same time pH descends from the initial 7.0 to values ranging from 4.3 to 4.0, apparently in negative correlation to the amount of dissolved P. BN0024 and BN0035 solubilized 781.4 and 746.5 mg/L, respectively, the highest amount of P as bacterial culture (Figure 2). Nonetheless, when the capacity of single cells to solubilize phosphate was calculated, the most efficient strain is BN0015 with 10 pg/ CFU after 36 h of growth (Figure 3).
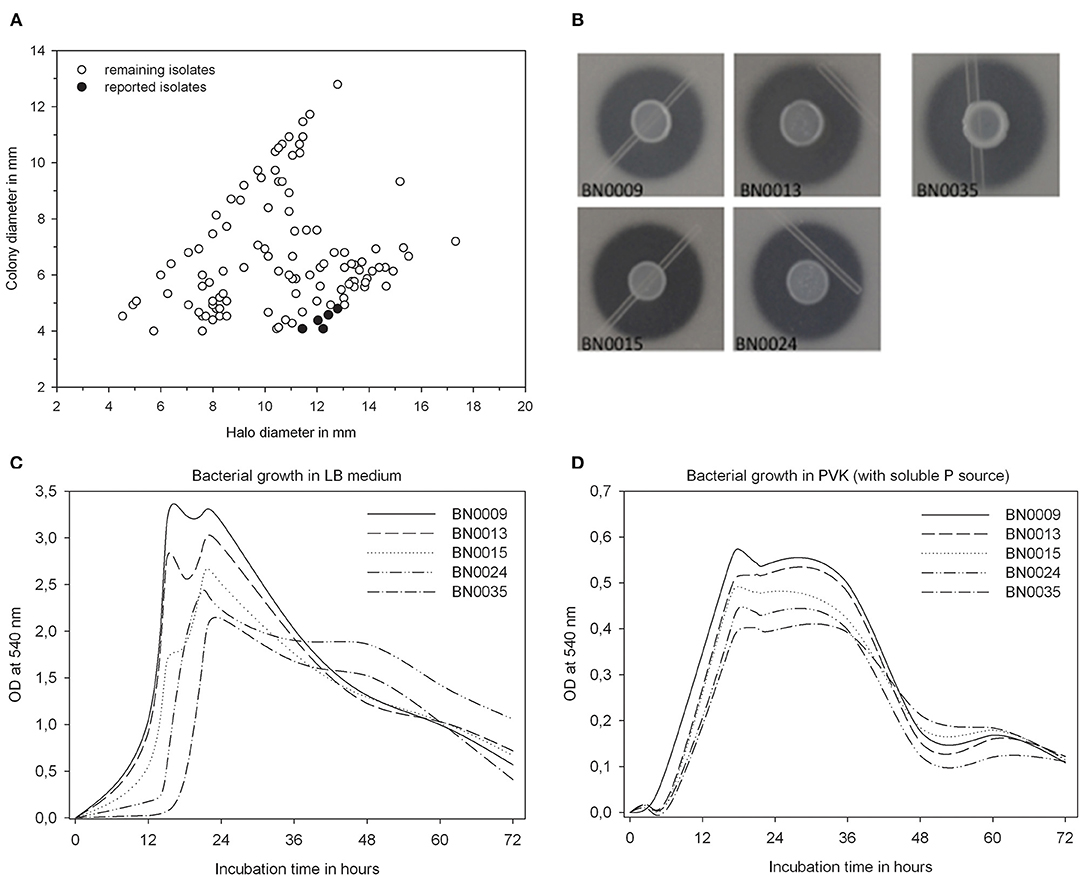
Figure 1. Solubilization capacity, colony morphology, and characterization of the bacterial growth; (A) solubilization capacity of all isolates in the screening, shown as ratio of halo vs. colony diameter (highlighted in black the reported isolates); (B) colony morphology and halo size of the five reported isolates on PVK plates; (C,D) bacterial growth in LB and PVK (with soluble P) liquid media for the reported isolates.
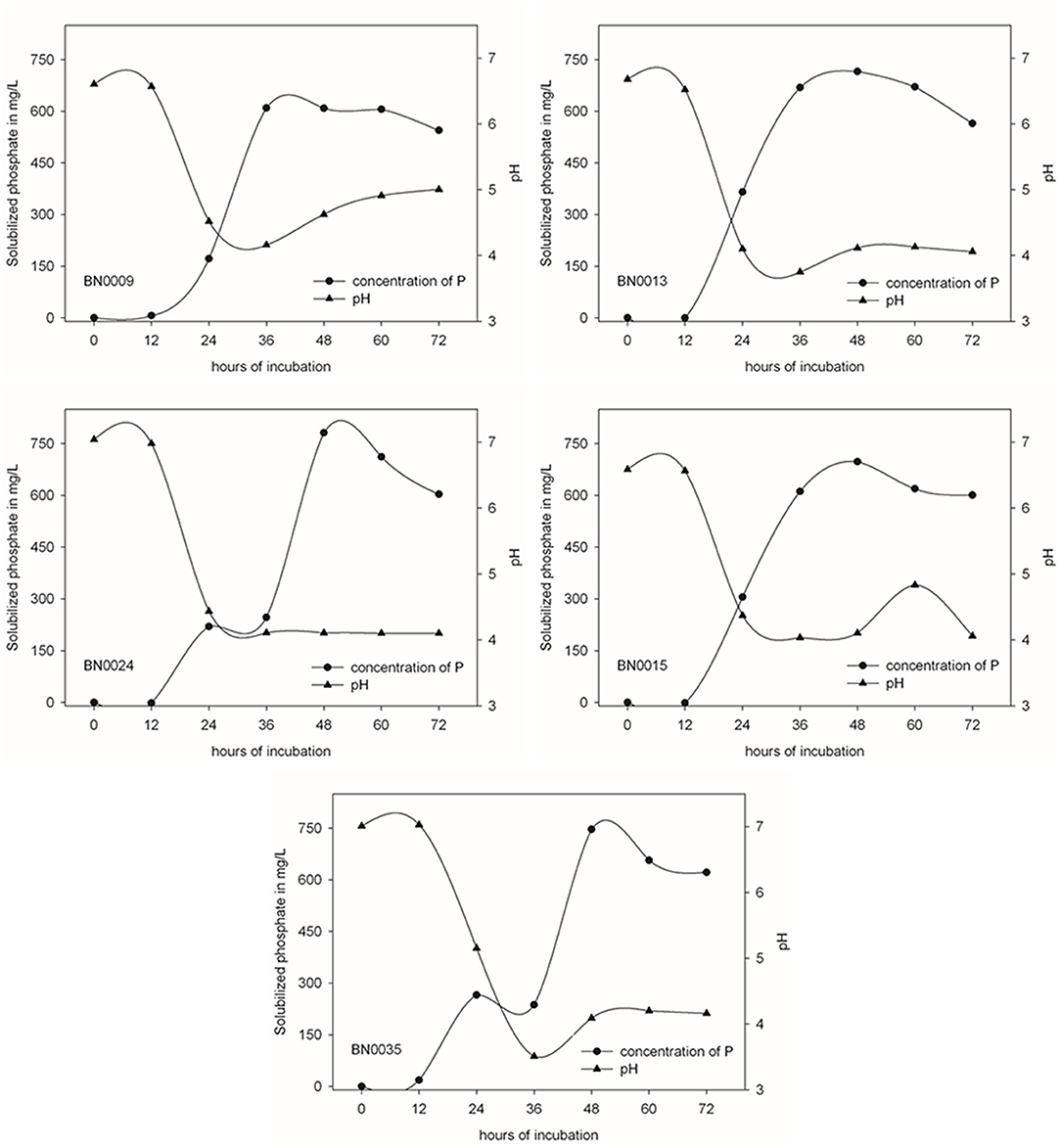
Figure 2. Kinetics of phosphate solubilization and pH changes of the five BN isolates in time, measurements every 12 h during 3 days of incubation.
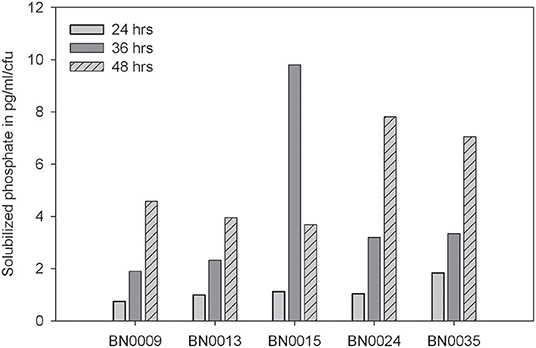
Figure 3. Phosphate solubilization capacity per cell of the five selected phosphate-solubilizing bacteria at three different times of incubation.
Evaluation of PGPR Traits
With respect to the taxonomy and identity, all five BN strains were determined as Gram-negative. Based on the 16S sequences, the strains belong to the genus Erwinia sp, with the highest identity scores (> 98.5%) for Erwinia pyrifoliae strain 8557, Erwinia billingiae strain HMF7415 and strain P3_3, a bacterial endosymbiont of Curculio koreanus (Supplementary Figure 1). Our strains are closely related to one another but are not clones. The phylogenetic analyses did not allow us to determine the species.
Furthermore, several PGPR characteristics were analyzed (Table 2). First, the ability to produce IAA, an important phytohormone for plant growth promotion was assessed and detected for all strains. The IAA production with tryptophan enriched medium ranged from 23 to 54 mg/L (Table 2). Among all BN isolates, BN0009 and BN0035 produced the highest IAA amounts (31 and 54 mg/L, respectively). BN0015, the most efficient P-solubilizer was found to produce an average IAA value (23.5 mg/L). Without tryptophan the IAA production was noticeable lower in all strains, reaching concentrations between 1.4 and 10.3 mg/L (Table 2).
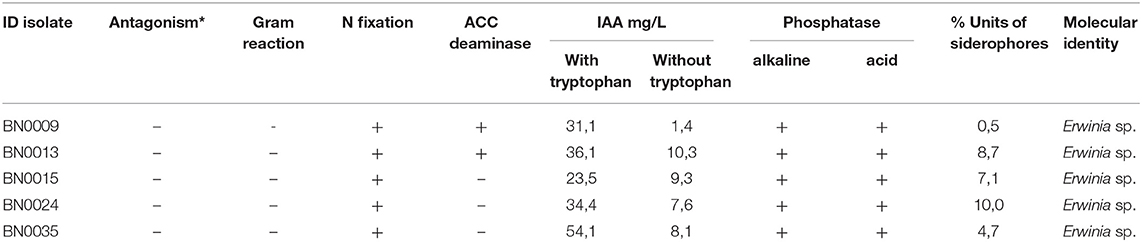
Table 2. Different PGPR traits and molecular identity of the 5 reported isolates (*Antagonism against Sclerotina sp. and Botrytis sp.).
All isolated strains demonstrated activity of alkaline and acid phosphatases and were capable of nitrogen fixation and siderophores production, in the latter values ranged between 0.5 and 10% siderophore units (Table 2). Additionally, only BN0009 and BN0013 were able to grow on ACC medium suggesting that both isolates possess ACC Deaminase activity (Table 2).
None of the five strains presented antagonism against Sclerotina spp and Botrytis spp. (Table 2).
Seed Germination Assays
Seeds of L. sativa (lettuce) and S. lycopersicum (tomato) seeds were inoculated with the selected strains to evaluate their effect on germination. All BN isolates accelerated the germination of L. sativa seed compared to the non-treated control, but the rate of enhancement varied among the bacterial strains (Figure 4A). Nonetheless, BN0013 accelerated the germination most (4.6x), reaching up to 93% after 24 h of incubation vs. the control with 20% at the same time (Figure 4A). Additionally, early root development and extension were analyzed 32 h after inoculation (Figure 4B). In L. sativa seeds, all isolates fostered early root development compared to control. In seeds treated with BN0009 and BN0013 a 78% (51% of seed with radicle <1 cm, 27% with root >1 cm) and 88% (45% of seed with radicle <1 cm, 43% with root >1 cm), respectively, demonstrated an enhanced root development compared to the non-treated control seeds (14% of seed with radicle <1 cm, 0% root >1 cm) (Figure 4B).
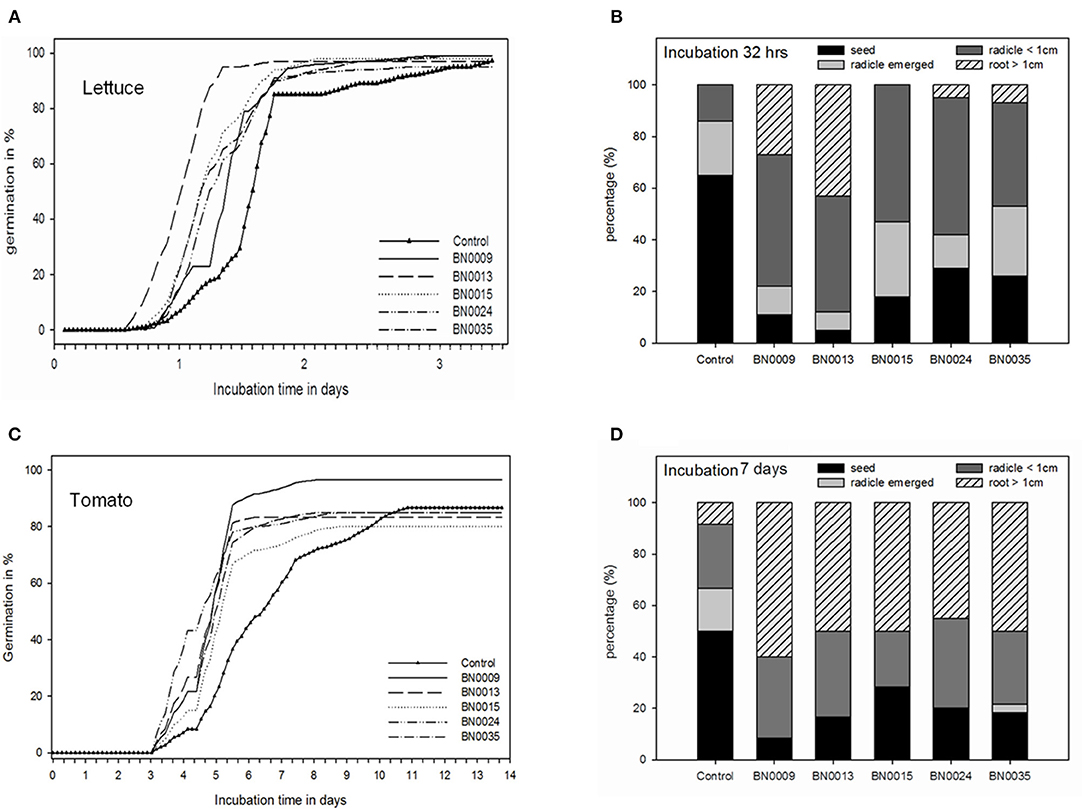
Figure 4. Characterization of the bacterial effect on germination and early root growth in lettuce and tomato; (A) germination over time in lettuce; (B) comparison of bacterial treatments regarding root growth categories in lettuce seedlings at 32 h of incubation; (C) germination over time in tomato; (D) comparison of bacterial treatments regarding root growth categories in tomato seedlings at 7 days of incubation.
In the germination assay with S. lycopersicum seeds, again all isolates accelerated the germination in 2.5 and 2 times in 6 days with respect to the non-treated control (Figure 4C). Meanwhile, BN0024 anticipated germination, reaching the first peak after only 4 days of incubation (45% of the seeds germinated), BN0009 significantly increased the germination rate compared to the non-treated control. After 7 days of incubation, in the non-treated control 50% of the seeds were germinated, whereas the germination rate in inoculated seeds varied between 72 and 92% (Figure 4D). Similar to lettuce, all BN isolates enhanced early root development in S. lycopersicum seeds. 45% (BN0024) to 60% (BN0009) of seeds with bacterial treatments already presented roots >1 cm, compared to the control with only 8% of the seeds (Figure 4D).
Finally, the root length of lettuce and tomato seedlings was measured after the respective incubation period of 3.5 and 14 days, respectively (Figure 5). In both crops, the BN isolates induced a significant root elongation compared to the non-treated control. For lettuce, BN0013 caused the longest root extension with 2.3 times compared with the control (Figures 5A,B), followed by BN009 and BN0015. In tomato, the bacterial effect is more equal. However, isolates BN0013 and BN0024 rise with an average root length of 2.0 and 2.2 times compared to the control (Figures 5C,D).
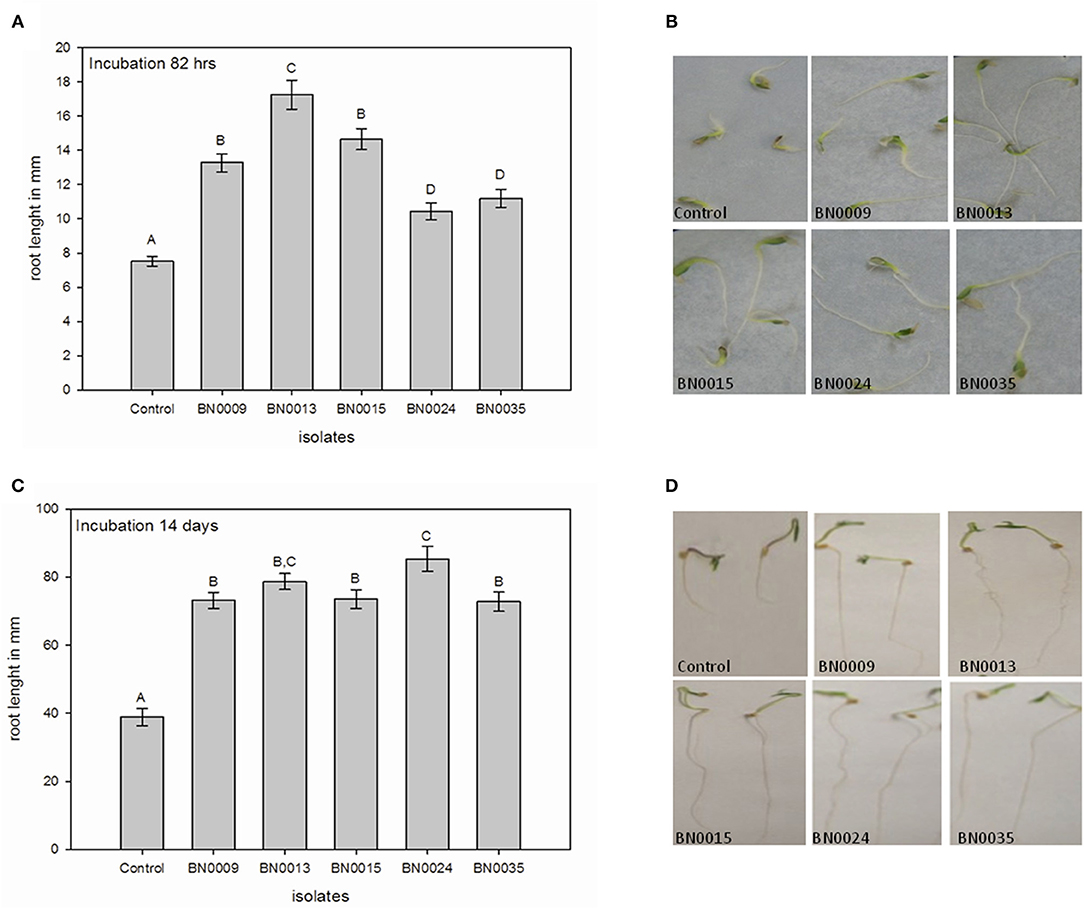
Figure 5. Characterization of the bacterial effects on root extension in lettuce and tomato seedlings; (A) lettuce root length after 82 h with 3 statistical groups different from the control (ANOVA-test); (B) photographs of lettuce seedlings after 82 h of incubation; (C) tomato root length after 14 days with 2 statistical groups different from the control (ANOVA-test); (D) photographs of tomato seedlings after 14 days of incubation.
Pot Experiment With Lettuce
Further insights about growth promotion beyond germination produced by the five strains were evaluated in a lettuce pot experiment. Inoculated seeds were planted and maintained for 35 days under controlled conditions. At harvest, root area, leaf area, number of leaves, fresh, and dry weight were measured. All isolates significantly increased root area (1.4–1.8x), leaf area (1.5–1.7x), and the number of developed leaves (1–2 leaves) compared to the control (Figure 6A, Table 3). Also, the effects were similar when comparing the strains. Seeds treated with BN0024 developed on average one leave more than control, which implies an advance in seedling development of ~1 week. The results for fresh and dry weight after inoculation with BN isolates were more differential (Figure 6B, Table 3). All isolates presented a significantly higher root fresh weight (1.9–2.3x), but the root dry weight was similar to the control. Three strains (BN0015, BN0024, and BN0035) stimulated significantly the leaf development in both fresh and dry weight compared with the control (1.8, and 2x, respectively) (Figure 6B, Table 3). Overall, the inoculation with BN0015, BN0024, and BN0035 produced the strongest promotion of plant growth and development on Lactuca sativa during the pot experiment.
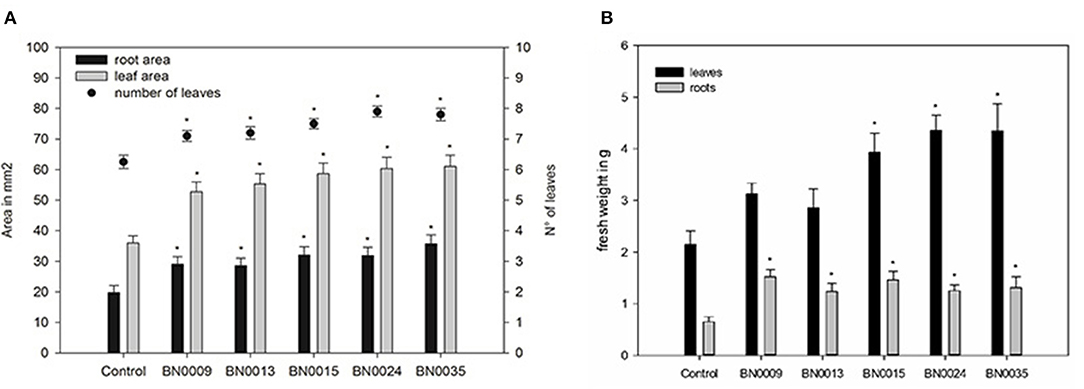
Figure 6. Bacterial effects on the development of lettuce seedlings in pots (vs. control; after 28 days of growth); (A) root and leaf area, number of formed leaves; (B) fresh weight of roots and leaves; asterisks indicate significant difference between respective isolate and control in ANOVA-test.
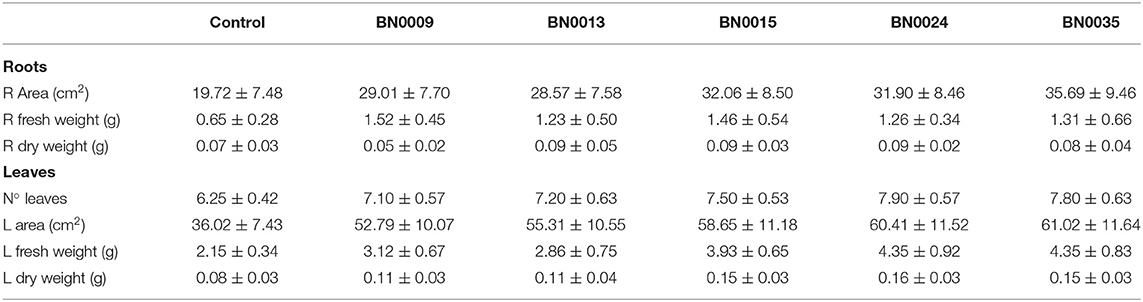
Table 3. Growth parameters from the lettuce pot experiment with seed inoculation of the five selected strains BN0009, BN0013, BN0015, BN0024, and BN0035.
Lettuce Field Experiment
Based on the previous data, BN0024 was selected for a lettuce field experiment with root inoculation during transplant from nursery to field and two fertilization levels. The lettuce plants responded to both factors (inoculation/fertilization) of the field experiment (Figure 7, Table 4). The effect of bacterial inoculation on plant growth was higher at 50% fertilization, where all evaluated parameters increased significantly (Table 4). With 100% fertilization the evaluated parameters also improved in plants with application of BN0024, nonetheless only root area, P content and protein content were significantly higher compared to control. The P content in plants with bacterial inoculations was significantly elevated compared to control in either fertilization treatment.
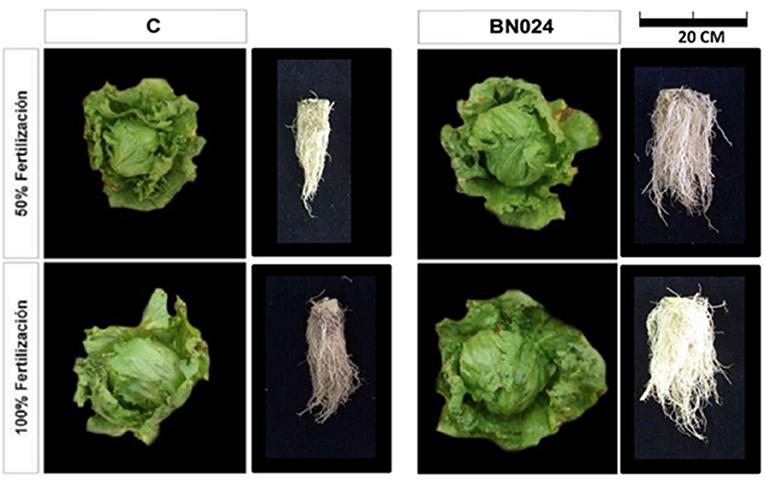
Figure 7. Photographs from the lettuce field trail; left: Control (C) aerial and root lettuce development at harvest with 50 and 100% fertilization; right: BN0024 (BN024) aerial and root lettuce development at harvest with 50 and 100% fertilization.
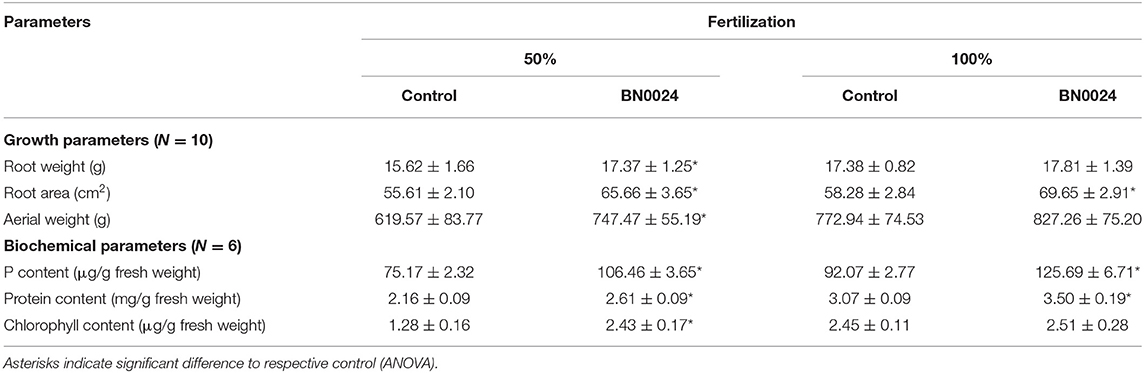
Table 4. Growth parameters from the lettuce field experiment, carried out with two levels of fertilization (50 and 100%) and root application of strain BN0024 at transplant.
Finally, the application of the strain BN0024 with 50% fertilization reached a crop productivity and nutrient content similar to the control with 100% fertilization (Table 4).
Discussion
In this work, rhizosphere soil samples from Jarava frigida (Phil.) F.Rojas (Poaceae) were screened for phosphate solubilizing rhizobacteria, which exhibit additional PGPR traits. The rhizospheric soil showed a low content of organic matter (0.63%), lower electric conductivity and high content of iron and copper, as described for Andean desert soils (Mandakovic et al., 2018).
Among the 180 phosphate solubilizing isolates, five efficient BN isolates were selected for further studies. Each of these five isolates demonstrated a high P-solubilization capacity, ability to N fixation, as well as the production of siderophores and IAA, which are used as indicators for a positive growth promotion aptitude (Backer et al., 2018; Wahid et al., 2020). However, but none of them showed antagonism response against fungal pathogens. The five strains, denominated as BN0009, BN0013, BN0015, BN0024, and BN0035 were identified as Erwinia sp., and did not demonstrate pathogenic characteristics. In fact, non-pathogenic Erwinia sp. strains were previously described as phosphate solubilizes by different authors, like Goswami et al. (2016) and Sagar et al. (2018).
In liquid medium each of five BN strains, induced an inverse relationship between pH and the solubilized P concentration, suggesting that the acidification facilitates the inorganic phosphate solubilization (Khan et al., 2007). This capacity is probably complemented with the enzymatic activity of alkaline and acid phosphatases that use organic phosphate as a substrate to convert it into inorganic form (Walpola and Yoon, 2012; Zhao et al., 2013). Additionally, the increased plant elongation (plant height and root length) can be associated with higher nutrient absorption, particularly phosphorous, which can induce cell elongation and multiplication Richardson and Simpson (2011).
Another important feature in the rhizobacteria—plant interaction, is their ability to produce phytohormones. We looked especially at IAA, which is involved in the formation of lateral roots and root hair, enhancing the nutrient absorption from soil (Qin and Huang, 2018). At least four pathways have been described for the biosynthesis of IAA in PGPR strains, being one of them tryptophan Trp-independent and two of them (Trp)-dependent (Gusain and Bhandari, 2019). Our five BN isolates produced between 23 and 54 mg IAA /L under the presence of tryptophan, concentrations which could cause important effects on the growth of the host plant. Actually, up to 80% of IAA-producing bacteria colonize root surfaces and stimulate the root system as a response to higher IAA concentrations (plant exogenous and endogenous synthesis) (Patten and Glick, 2002). The five isolated strains also were able to produce IAA in absence of tryptophan, which suggests the presence of a tryptophan-independent pathway (Ribeiro and Cardoso, 2012).
Compared to other studies (e.g., Sayyed et al., 2005), the siderophore production of our strains is relatively low, values are between 0.5 and 10 % siderophore units (Table 2). However, the concentration of available iron in their natural environment is high regarding the soil analysis (Table 1), which could result in lower selective pressure for this bacterial capacity.
All selected isolates demonstrated stimulation on lettuce and tomato germination (Figure 4). All strains accelerated the germination significantly compared to the control and generated a fast root extension in their early growth phase. These results coincide with other PSB studies, using strains alone or in combination, where a strong stimulation in radicle emergence from the seed is observed in plants with economical interest, e.g., rice, asparagus bean, and mung bean (Duarah et al., 2011; Arun et al., 2012).
In the lettuce greenhouse experiments, all five strains enhanced significantly the plant growth regarding root and leaf area, fresh, and dry weight as well as leaf numbers. These results are concordant with other authors who studied the effects of PSB on different crops: canola (Pandey et al., 2006), peanuts Goswami et al. (2014), cotton (Qureschi et al., 2012), maize (Yazdani et al., 2009; Shirinbayan et al., 2019), and rice (Rasul et al., 2019).
Despite the variety of in vitro and pot experiments with PSB and PGPR, few studies are available, where their effects were evaluated under productive conditions in field. Here we challenged Erwinia sp. strain BN0024, selected due to its constant growth promotion results, in a field experiment with lettuce, comparing two fertilization treatments (50 and 100% of the recommended crop fertilization). The application of BN0024 improved crop productivity in both fertilization treatments. Particularly, the P content in plants with bacterial inoculations was significantly elevated compared to control in either fertilization treatment, suggesting an improved nutrient uptake (Rezakhani et al., 2019; Bechtaoui et al., 2020). In addition, lettuce with 50% fertilization and inoculation with BN0024 equate the productivity of the control plants with 100% fertilization. Recently, similar experiences reducing the phosphate fertilizer requirements of crops between 50 and 75% have been reported for other soils, e.g., Brazilian Latosol with sugarcane (Saccharum sp.) production integrating PSB (Rosa et al., 2020), Chinese Troporthents and Rhodustuts with tea (Camellia sinensis (L.) O. Kuntze) production integrating PGPR (Tennakoon et al., 2019). Our results gain special importance in the context of P availability in agricultural soil of semiarid and arid zones, which could be mediated integrating PSB application in the crop management (Bechtaoui et al., 2020; Wahid et al., 2020) reducing environmental pollution (Mallin and Cahoon, 2020) and production costs for agricultures.
Beyond aridity, soils of arid zones possess other complex characteristics (pH, low soil organic matter, low availability of macronutrients, bound by soil chemistry), which need to be addressed in agriculture management. While aridity is compensated by the use of irrigation systems, the low availability of macronutrients like phosphorus, quickly bound to the soil matrix and not accessible for plant growth, still is a major issue in agriculture and the use of P solubilizing PGPR can offer an alternative to improve fertilization efficiency—as demonstrated by our results. Our results encourage the use of PGPR for these purposes, improving not only the efficiency of P-fertilization, but also allowing to improve crop quality and productivity as well as reduce costs.
Future studies will focus on two aspects: (1) further biochemical, physiological, and molecular characterization of the five Erwinia sp. isolates to understand more entirely their metabolism and interaction with plants; (2) development of application strategies for these strains as part of integrated crop management. This information will enable the design of eco-friendly alternatives to stimulate the productivity of agriculture carried out under the limiting conditions of semi-arid and arid zones.
Data Availability Statement
The datasets presented in this study can be found in online repositories. The names of the repository/repositories and accession number(s) can be found below: NCBI accession numbers: MW092897, MW092898, MW092899, MW092900, and MW092901.
Author Contributions
AS, JB, and VO designed the research. SM, AR, BA, and PM sampled and performed laboratory work under the guidance of AS and CJ. JA, MG, and CS carried out data analysis. All authors wrote the manuscript, designed Tables and Figures, revised the manuscript, and approved the final version.
Conflict of Interest
The authors declare that the research was conducted in the absence of any commercial or financial relationships that could be construed as a potential conflict of interest.
Acknowledgments
Authors thank Project BIP 30127532-0, Regional Fund of Innovation for Competitiveness Coquimbo Region (FIC-R), Chile and technical support from professionals of the field station INIA Intihuasi Pan de Azucar (PEPA).
Supplementary Material
The Supplementary Material for this article can be found online at: https://www.frontiersin.org/articles/10.3389/fsufs.2020.607355/full#supplementary-material
References
Alexander, D. B., and Zuberer, D. A. (1991). Use of chrome azurol S reagents to evaluate siderophore production by rhizosphere bacteria. Biol. Fertil. Soils 45, 12–39. doi: 10.1007/BF00369386
Arun, B., Gopinath, B., and Sharma, S. (2012). Plant growth promoting potential of bacteria isolated on N free media from rhizosphere of Cassia occidentalis. World J. Microbiol. Biotechnol. 9, 2849–2857. doi: 10.1007/s11274-012-1095-1
Babalola, O. O. (2010). Beneficial bacteria of agricultural importance. Biotechnol. Lett. 32, 1559–1570. doi: 10.1007/s10529-010-0347-0
Backer, R., Rokem, J. S., Ilangumaran, G., Lamont, J., Praslickova, D., Ricci, E., et al. (2018). Plant growth-promoting rhizobacteria: context, mechanisms of action, and roadmap to commercialization of biostimulants for sustainable agriculture. Front. Plant Sci. 9:1473. doi: 10.3389/fpls.2018.01473
Baldani, J. I., Reis, V. M., Videira, S. S., Boddey, L. H., and Baldani, V. L. D. (2014). The art of isolating nitrogen-fixing bacteria from non-leguminous plants using N-free semi-solid media: a practical guide for microbiologists. Plant Soil 384, 413–431. doi: 10.1007/s11104-014-2186-6
Bechtaoui, N., Raklami, A., Benidire, L., Tahiri, A., Göttfert, M., and Oufdou, K. (2020). Effects of PGPR co-inoculation on growth, phosphorus nutrition and phosphatase/phytase activities of faba bean under different phosphorus availability conditions. Polish J. Environ. Stud. 29, 1557–1565. doi: 10.15244/pjoes/110345
Bholay, A. D., Jadhav Priyanka, U., Borkhataria, B. V., and Dhalkari, M. V. (2012). Fluorescent pseudomonas as plant growth promoting rhizobacteria and their siderophoregenesis CAS assay solutions. J. Pharm. Biol. Sci. 3, 27–32 doi: 10.9790/3008-0312732
Bradford, M. (1976). A rapid and sensitive method for the quantitation of microgram quantities of protein utilizing the principle of protein-dye binding. Anal. Biochem. 72, 248–254. doi: 10.1016/0003-2697(76)90527-3
Duarah, I., Deka, M., Saikia, N., and Boruah Deka, H. P. (2011). Phosphate solubilizers enhance NPK fertilizer use efficiency in rice and legume cultivation. Biotech 1, 227–238. doi: 10.1007/s13205-011-0028-2
Eisenhauer, N., Lanoue, A., Strecker, T., Scheu, S., Steinauer, K., Thakur, M. P., et al. (2017). Root biomass and exudates link plant diversity with soil bacterial and fungal biomass. Sci. Rep. 7:44641. doi: 10.1038/srep44641
Ekin, Z. (2010). Performance of phosphate solubilizing bacteria for improving growth and yield of sunflower (Helianthus annuus L.) in the presence of phosphorus fertilizer. Afr. J. Biotechnol. 9, 3794–3800. Available online at: http://www.academicjournals.org/AJB
Emami, S., Alikhani, H. A., Pourbabaee, A. A., Etesami, H., Motasharezadeh, B., and Sarmadian, F. (2020). Consortium of endophyte and rhizosphere phosphate solubilizing bacteria improves phosphorous use efficiency in wheat cultivars in phosphorus deficient soils. Rhizosphere 14:100196. doi: 10.1016/j.rhisph.2020.100196
Fiume, F., and Fiume, F. (2006). Biological control of Botrytis gray mould on tomato cultivated in greenhouse. Commun. Agric. Appl. Biol. Sci. 71, 897–908.
Gajardo, R. (1994) La Vegetación Natural de Chile. Clasificación y Distribución Geográfica. Santiago: Editorial Universitaria. 165p.
Glickmann, E., and Dessaeux, Y. (1995). A critical examination of the specificity of the salkowski reagent for indolic compounds produced by phytopathogenic bacteria. Appl. Environ. Microbiol. 61, 793–796. doi: 10.1128/AEM.61.2.793-796.1995
Goldstein, A. H. (1994). “Involvement of the quinoprotein glucose dehydrogenises in the solubilization of exogenous phosphates by gram-negative bacteria,” in Phosphate in Microorganisms: Cellular and Molecular Biology, eds A. Torriani, E. Gorini, and S. Yagil (Washington, DC: ASM Press), 197–203.
Gómez-Silva, B., Rainey, F. A., Warren-Rhodes, K. A., McKay, C. P., and Navarro-González, R. (2008). Atacama desert soil microbiology. Soil Biol. 13, 117–132. doi: 10.1007/978-3-540-74231-9_6
Gordon, S. A., and Weber, R. P. (1951). Colorimetric estimation of indoleacetic acid. Plant Physiol. 26, 192–195. doi: 10.1104/pp.26.1.192
Goswami, D., Dhandhukia, P., Patel, P., and Thakker, J. N. (2014). Screening of PGPR from saline desert of Kutch: growth promotion in Arachis hypogea by Bacillus licheniformis A2. Microbiol. Res. 169, 66–75. doi: 10.1016/j.micres.2013.07.004
Goswami, D., Thakker, J. N., and Dhandhukia, P. C. (2016). Portraying mechanics of plant growth promoting rhizobacteria (PGPR). a review. Cogent. Food Agric. 2, 1–19. doi: 10.1080/23311932.2015.1127500
Gusain, P., and Bhandari, P. S. (2019). Rhizosphere associated PGPR functioning. J. Pharm. Phytochem. 8, 1181–1191. Available online at: http://www.phytojournal.com/
Gyaneshwar, P., Kumar, N. J., Pareka, L. J., and Podle, P. S. (2002). Role of soil microorganisms in improving P nutrition of plants. Plant Soil 245, 83–93. doi: 10.1023/A:1020663916259
Hylander, L. D., Svensson, H. I., and Simán, G. (1996). Different methods for determination of plant available soil phosphorus. Commun. Soil Sci. Plant Anal. 27, 1501–1512.
Illmer, P., and Schinner, F. (1995). Solubilization of inorganic calcium phosphates– solubilization mechanisms. Soil Biol. Biochem. 27, 257–263. doi: 10.1016/0038-0717(94)00190-C
INIA (2012). BOLETÍN INIAN°246: Nuevas Fichas Hortícolas. 3ª Edición. Chillán: Centro Regional de Investigación Quilamapu. 59.
Kanter, D. R., and Brownlie, W. J. (2019). Joint nitrogen and phosphorus management for sustainable development and climate goals. Environ. Sci. Policy 92, 1–8. doi: 10.1016/j.envsci.2018.10.020
Khan, M. S., Zaidi, A., and Wani, P. A. (2007). Role of phosphate-solubilizing microorganisms in sustainable agriculture a review. Agron. Sustain. Dev. 27, 29–43. doi: 10.1051/agro:2006011
Kim, K., Jordan, D., and McDonald, G. (1998). Enterobacter agglomerans, phosphate solubilizing bacteria and microbial activity in soil: effect of carbon sources. Soil Biol. Biochem. 30, 995–1003. doi: 10.1016/S0038-0717(98)00007-8
Kumar, S., Stecher, G., and Tamura, K. (2016). MEGA7: molecular evolutionary genetics analysis version 7.0 for bigger datasets. Mol. Biol. Evol. 33, 1870–1874. doi: 10.1093/molbev/msw054
Liu, J., Qi, W., Li, Q., Wang, S. G., Song, C., and Yuan, X. Z. (2020). Exogenous phosphorus-solubilizing bacteria changed the rhizosphere microbial community indirectly. 3 Biotech 10:164. doi: 10.1007/s13205-020-2099-4
López-Angulo, J., de la Cruz, M., Chacón-Labella, J., Illuminati, A., Matesanz, S., Pescador, D. S., et al. (2020). The role of root community attributes in predicting soil fungal and bacterial community patterns. New Phytol. 228, 1070–82. doi: 10.1111/nph.16754
Mallin, M. A., and Cahoon, L. B. (2020). The hidden impacts of phosphorus pollution to streams and rivers. BioScience 70, 315–329. doi: 10.1093/biosci/biaa001
Mandakovic, D., Rojas, C., Maldonado, J., Latorre, M., Travisany, D., Delage, E., et al. (2018). Structure and co-occurrence patterns in microbial communities under acute environmental stress reveal ecological factors fostering resilience. Sci. Rep. 8:5875. doi: 10.1038/s41598-018-23931-0
Marchesi, J. R., Sato, T., Weightman, A. J., Martin, T. A., Fry, J. C., Hiom, S. J., et al. (1998). Design and evaluation of useful bacterium-specific PCR primers that amplify genes coding for bacterial 16S rRNA. Appl. Environ. Microbiol. 64, 795–799. doi: 10.1128/AEM.64.2.795-799.1998
Mittermeier, R. A., Robles Gil, P., Hoffmann, M., et al. (2005). Hotspots Revisited: Earth's Biologically Richest and Most Threatened Terrestrial Ecoregions. Chicago, IL: Univ of Chicago Press.
Nacoon, S., Jogloy, S., Riddech, N., Mongkolthanaruk, W., Kuyper, T. K., and Boonlue, S. (2020). Interaction between phosphate solubilizing bacteria and arbuscular mycorrhizal fungi on growth promotion and tuber inulin content of Helianthus tuberosus L. Sci. Rep. 10:4916. doi: 10.1038/s41598-020-61846-x
Nguyen, C., Yan, W., Le Tacon, F., and Lapayrie, F. (1992). Genetic variability of phosphate solubilizing activity by monocaryotic and dicaryotic mycelia of the ectomycorrhizal fungus Laccaria bicolor (Maire) P.D.Orton. Plant Soil 143, 193–199. doi: 10.1007/BF00007873
Pandey, A., Trivedi, P., Kumar, B., and Palni, L. M. S. (2006). Characterization of a phosphate solubilizing and antagonistic strain of Pseudomonas putida (B0) isolated from a sub-alpine location in the Indian central Himalaya. Curr. Microbiol. 53, 102–107. doi: 10.1007/s00284-006-4590-5
Parani, K., and Saha, B. K. (2012). Prospects of using phosphate solubilizing Pseudomonas as bio-fertilizer. Eur. J. Biol. Sci. 4, 40–44. doi: 10.5829/idosi.ejbs.2012.4.2.63117
Patten, C. L., and Glick, B. R. (2002). Role of Pseudomonas putida indoleacetic acid in development of the host plant root system. Appl. Environ. Microbiol. 68, 3795–3801. doi: 10.1128/aem.68.8.3795-3801.2002
Penrose, D. M., and Glick, B. R. (2003). Methods for isolating and characterizing ACC deaminase-containing plant growth-promoting rhizobacteria. Physiol. Plant 118, 10–15. doi: 10.1034/j.1399-3054.2003.00086.x
Porra, R. J., Thompson, W. A., and Kriedemann, P. E. (1989). Determination of accurate extinction coefficients and simultaneous equations for assaying chlorophylls a and b extracted with four different solvents: verification of the concentration of chlorophyll standards by atomic absorption spectroscopy. Biochim. Biophys. Acta Bioener. 975, 384–394. doi: 10.1016/S0005-2728(89)80347-0
Qin, H., and Huang, R. (2018). Auxin controlled by ethylene steers root development. Int. J. Mol. Sci. 19:3656. doi: 10.3390/ijms19113656
Qureschi, M. A., Ahmad, Z. A., Akhtar, N., Iqbal, A., Mujeeb, F., and Shakir, M. A. (2012). Role on phosphate solubilizing bacteria (PSB) in enhancing availability and Promoting cotton growth. J. Anim. Plant Sci. 22, 204–210. Available online at: http://www.thejaps.org.pk/docs/v-22-1/6.pdf
Ranjan, A., Mahalakshmi, M. R., and Sridevi, M. (2013). Isolation and characterization of phosphate-solubilizing bacterial species from different crop fields of Salem, Tamil Nadu, India. Int. J. Nutr. Pharmacol. Neurol. Dis. 3, 29–33. doi: 10.4103/2231-0738.106982
Rasul, M., Yasmin, S., Suleman, M., Zaheer, A., Reitz, T., Tarkka, M. T., et al. (2019). Glucose dehydrogenase gene containing phosphobacteria for biofortification of Phosphorus with growth promotion of rice. Microbiol. Res. 223, 1–12. doi: 10.1016/j.micres.2019.03.004
Rezakhani, L., Motesharezadeh, B., Tehrani, M. M., Etesami, H., and Hosseini, H. M. (2019). Phosphate-solubilizing bacteria and silicon synergistically augment phosphorus (P) uptake by wheat (Triticum aestivum L.) plant fertilized with soluble or insoluble P source. Ecotoxicol. Environ. Saf. 173, 504–513. doi: 10.1016/j.ecoenv.2019.02.060
Ribeiro, C. M., and Cardoso, E. J. B. N. (2012). Isolation, selection and characterization of root-associated growth promoting bacteria in Brazil Pine (Araucaria angustifolia). Microbiol. Res. 167, 69–78. doi: 10.1016/j.micres.2011.03.003
Richardson, A. E., and Simpson, R. J. (2011). Soil microorganisms mediating phosphorus availability. Plant Physiol. 156, 989–996. doi: 10.1104/pp.111.175448
Rosa, P. A. L., Mortinho, E. S., Jalal, A., Galindo, F. S., Buzetti, S., Fernandes, G. C., et al. (2020). Inoculation with growth-promoting bacteria associated with the reduction of phosphate fertilization in sugarcane. Front. Environ. Sci. 8:32. doi: 10.3389/fenvs.2020.00032
Sagar, A., Thomas, G., Rai, S., Mishra, R. K., and Ramteke, P. W. (2018). Enhancement of growth and yield parameters of wheat variety AAI-W6 by an organic farm isolate of plant growth promoting erwinia species (KP226572). Int. J. Agric. Environ. Biotechnol. 11, 159–71. doi: 10.30954/0974-1712.2018.00178.21
Salvatierra-Martinez, R., Arancibia, W., Araya, M., Aguilera, S., Olalde, V., Bravo, J., et al. (2018). Colonization ability as an indicator of enhanced biocontrol capacity-An example using two Bacillus amyloliquefaciens strains and botrytis cinerea infection of tomatoes. J. Phytopathol. 166, 601–612. doi: 10.1111/jph.12718
Sashidhar, B., and Podile, A. R. (2010). Mineral phosphate solubilization by rhizosphere bacteria and scope for manipulation of the direct oxidation pathway involving glucose dehydrogenase. J. Appl. Microbiol. 109, 1–12. doi: 10.1111/j.1365-2672.2009.04654.x
Sayyed, R. Z., Badgujar, M. D., Sonawane, H. M., Mhaske, M. M., and Chincholkar, S. B. (2005). Production of microbial iron chelators (siderophores) by fluorescent Pseudomonads. Indian J. Biotechnol. 4, 484–490. Available online at: http://hdl.handle.net/123456789/5776
Schwyn, B., and Neilands, B. (1987). Universal chemical assay for the detection and determination of siderophores. Anal. Biochem. 160, 47–56. doi: 10.1016/0003-2697(87)90612-9
Shi, S., Richardson, A. E., O'Callaghan, M., DeAngelis, K. M., Jones, E. E., Stewart, A., et al. (2011). Effects of selected root exudate components on soil bacterial communities. FEMS Microbiol. Ecol. 77, 600–610. doi: 10.1111/j.1574-6941.2011.01150.x
Shirinbayan, S., Khosravi, H., and Malakouti, M. J. (2019). Alleviation of drought stress in maize (Zea mays) by inoculation with Azotobacter strains isolated from semi-arid regions. Appl. Soil Ecol. 133, 138–145. doi: 10.1016/j.apsoil.2018.09.015
Singh, S., and Kapoor, K. K. (1999). Inoculation with phosphate solubilizing microorganisms and a vesicular arbuscular mycorrhizal fungus improves dry matter yield and nutrient uptake by wheat grown in a sandy soil. Biol. Fertil. Soils 28, 139–144. doi: 10.1007/s003740050475
Tennakoon, P. L. K., Rajapaksha, R. M. C. P., and Hettiarachchi, L. S. K. (2019). Tea yield maintained in PGPR inoculated field plants despite significant reduction in fertilizer application. Rhizosphere 10:100146. doi: 10.1016/j.rhisph.2019.100146
Vazquez, P., Holguin, G., Puente, M. E., Lopez-Cortes, A., and Bashan, Y. (2000). Phosphate solubilizing microorganisms associated with the rhizosphere of mangroves in a semiarid coastal lagoon. Biol. Fertil. Soils 30, 460–468. doi: 10.1007/s003740050024
Vives-Peris, V., de Ollas, C., Gómez-Cadenas, A., and Pérez-Clemente, R. M. (2020). Root exudates: from plant to rhizosphere and beyond. Plant Cell. Rep. 39, 3–17. doi: 10.1007/s00299-019-02447-5
Wahid, F., Fahad, S., Danish, S., Adnan, M., Yue, Z., Saud, S., et al. (2020). Sustainable management with mycorrhizae and phosphate solubilizing bacteria for enhanced phosphorus uptake in calcareous soils. Agriculture 10:334. doi: 10.3390/agriculture10080334
Walpola, B. C., and Yoon, M. (2012). Isolation and characterization of phosphate solubilizing bacteria and their co-inoculation efficiency on tomato plant growth and phosphorous uptake. Afr. J. Microbiol. Res. 7, 266–275. doi: 10.5897/AJMR12.2282
Watanabe, F. S., and Olsen, S. R. (1965). Test of an ascorbic acid method for determining phosphorous in water and NaHCO3 extracts from soil. Soil Sci. Soc. Am. Proc. 29, 677–78. doi: 10.2136/sssaj1965.03615995002900060025x
Yazdani, M., Bahmanyar, M. A., Pirdashti, H., and Esmaili, M. A. (2009). Effect of phosphate solubilization microorganisms (PSM) and plant growth promoting rhizobacteria (PGPR) on yield and yield components of corn (Zea mays L.). Proc. World Acad. Sci. Eng. Technol. 49:90.
Yeates, C., Gillings, M. R., Davison, A. D., Altavilla, N., and Veal, D. A. (1998). Methods for microbial DNA extraction from soil for PCR amplification. Biol. Proced. Online 14, 40–47. doi: 10.1251/bpo6
Zhang, Z., Schwart, S., Wagner, L., and Miller, W. (2000). A greedy algorithm for aligning DNA sequences. J. Computat. Biol. 7, 203–214. doi: 10.1089/10665270050081478
Keywords: PGPR - plant growth-promoting rhizobacteria, nutrient uptake, Erwinia, biostimulant, sustainable agriculture, horticulture crops
Citation: Maldonado S, Rodríguez A, Ávila B, Morales P, González MP, Araya Angel JPA, Olalde V, Bravo J, Jana C, Sierra C and Stoll A (2020) Enhanced Crop Productivity and Sustainability by Using Native Phosphate Solubilizing Rhizobacteria in the Agriculture of Arid Zones. Front. Sustain. Food Syst. 4:607355. doi: 10.3389/fsufs.2020.607355
Received: 17 September 2020; Accepted: 23 November 2020;
Published: 23 December 2020.
Edited by:
Everlon Cid Rigobelo, Universidade Estadual Paulista, BrazilReviewed by:
Sumera Yasmin, National Institute for Biotechnology and Genetic Engineering, PakistanBikram Pratap Singh, Indian Council of Agricultural Research (ICAR), India
Copyright © 2020 Maldonado, Rodríguez, Ávila, Morales, González, Araya Angel, Olalde, Bravo, Jana, Sierra and Stoll. This is an open-access article distributed under the terms of the Creative Commons Attribution License (CC BY). The use, distribution or reproduction in other forums is permitted, provided the original author(s) and the copyright owner(s) are credited and that the original publication in this journal is cited, in accordance with accepted academic practice. No use, distribution or reproduction is permitted which does not comply with these terms.
*Correspondence: Alexandra Stoll, YWxleGFuZHJhLnN0b2xsJiN4MDAwNDA7Y2VhemEuY2w=