- 1Bio-Protection Research Center, Lincoln University, Lincoln, New Zealand
- 2Plataforma de Bioinsumos, Programa de Producción y Sustentabilidad Ambiental, Instituto Nacional de Investigación Agropecuaria (INIA), INIA - Las Brujas, Canelones, Uruguay
- 3Faculty of Agriculture and Life Sciences, Lincoln University, Lincoln, New Zealand
- 4Department Chemical Process and Pharmaceutical Development, Unit Formulation, Research Institute of Sweden (RISE), Borås, Sweden
- 5Saville Statistical Consulting Limited, Lincoln, New Zealand
- 6AgResearch, Lincoln Research Center, Lincoln, New Zealand
- 7United States Department of Agriculture (USDA), National Center for Agricultural Utilization, Peoria, IL, United States
The commercial use of the entomopathogenic fungi Metarhizium spp. in biopesticides has gained more interest since the discovery that several species of this genus are able to colonize roots. In general, commercial products with Metarhizium are formulated based on conidia for insect pest control. The process of mass production, harvesting, and formulation of infective conidia can be detrimental for conidial viability. Entomopathogenic fungi such as Metarhizium spp. are able to produce high concentrations of resistant structures, known as microsclerotia, when grown in liquid media. Microsclerotia are desiccation tolerant, with excellent storage stability, and are capable of producing high quantities of infective conidia after rehydration. The aim of this study was to evaluate microsclerotia production by different isolates of Metarhizium spp. and determine the effect of microsclerotia coated onto maize seeds on plant growth in the presence of soil-borne pathogen Fusarium graminearum. On average, ~1 × 105 microsclerotia/mL were produced by selected isolates of M. anisopliae (A1080 and F672) and Metarhizium robertsii (F447). Microsclerotia were formulated as granules with diatomaceous earth and used for seed coating, after which propagules produced around 5 × 106 CFU/g of seeds. In the presence of the plant pathogen, maize plants grown from untreated seeds had the lowest growth, while plants treated with the Metarhizium microsclerotia had significantly greater growth than the control plants. Hyphae were observed growing on and in root tissues in all the Metarhizium spp. treatments but not in samples from control plants. Metarhizium hyphal penetration points' on roots were observed 1 month after sowing, indicating the fungi were colonizing roots as endophytes. The results obtained indicate that microsclerotia can be coated onto seeds, providing plant protection against soil plant pathogens and a method to establish Metarhizium in the ecto- and endo-rhizosphere of maize roots, allowing the persistence of this biocontrol agent.
Introduction
The commercial use of entomopathogenic fungi such as Metarhizium (Metschnikoff) Sorokin, 1883 (Hypocreales: Clavicipitaceae) or Beauveria (Balsamo) Vuillemin, 1912 (Hypocreales: Clavicipitaceae) as “mycoinsecticides” or “biopesticides” is generally practiced using the inundative biocontrol approach, where the environment harboring the insect pest is treated with high concentrations of infective fungal propagules (Eilenberg et al., 2001; Jackson et al., 2010). Generally, fungal antagonists of plant pathogens or fungal biopesticides for biocontrol purposes are produced using solid substrate fermentations on grains which are subsequently formulated as powders or liquids for topical applications or as granules for soil applications (Jackson et al., 2010; Tripathi et al., 2010; Jaronski, 2014). However, the different steps involved in mass production of infective conidia as well as during harvesting and formulation can be detrimental for conidia viability and survival. Additionally, proper application with standard farming equipment and homogenous distribution through the soil to target phytopathogens or soil dwelling insect pests, can be difficult to achieve (Jaronski and Jackson, 2008). Granular formulations are a practical alternative to agrochemicals for the application of fungal antagonists for plant protection targeting soil plant pathogens and soil-dwelling insect pests (Burges, 1998; Jaronski and Jackson, 2008).
The development of mycoinsecticides as conidiogenic granules is preferred since fresh conidia are produced in situ where the target plant pathogens or insect pests dwell (Jackson and Jaronski, 2012). Using this propagule alternative, conidia avoid all the necessary steps from mass-production up to application, which can damage these fungal reproductive structures. When incorporated into soil, biocontrol agents formulated as conidiogenic granules have some additional advantages such as protection from the harmful effects of UV radiation or from unfavorable conditions such as high temperatures and low humidity to which microorganisms can be exposed after foliar applications. Another benefit is that in soil, moisture content and temperatures are generally within the optimal range for fungal survival and growth (Jackson and Jaronski, 2009).
Over the past decade, it has been discovered that entomopathogenic fungi belonging to M. anisopliae, M. brunneum, Beauveria bassiana, B. brongniartii, and B. pseudobassiana are able to produce high concentrations of microsclerotia (MS) when grown in liquid media (Jackson and Jaronski, 2009, 2012; Vega et al., 2009; Wang et al., 2011; Villamizar et al., 2018). These environmentally resistant structures are desiccation tolerant, have excellent storage stability, and are capable of producing high quantities of infective conidia suitable for management insect pests or as antagonists of phytopathogens (Jaronski and Jackson, 2008; Jackson and Jaronski, 2009; Vega et al., 2009; Song, 2018). Microsclerotia from M. anisopliae can be produced by culturing in liquid substrate fermentation for ~3 to 4 days in continuous agitation, while further fermentation up to 8 days allows the complete MS melanization. Subsequently, obtained MS are harvested and formulated in diatomaceous earth (DE) and air-dried to produce MS-DE granules (Jackson and Jaronski, 2009). MS-DE granules can survive a moderate drying process without significant viability losses and can be safely stored at 4° for up to 12 months. In appropriate conditions, MS germinate (hyphal development) and produce high quantities of conidia, in general above 1 × 108 conidia/g dried MS-DE (Jackson and Jaronski, 2009, 2012). As for conidiogenic granules, the soil environment is suitable for reactivation of MS, since humid conditions are favorable for the rehydration and germination process of these fungal resting structures. Consequently, MS obtained from entomopathogenic fungi have been recognized as a viable alternative for use in biocontrol programmes (Jaronski and Jackson, 2008).
Another interesting feature of some entomopathogenic fungi like Metarhizium and Beauveria is their natural ability to associate endophytically with plant roots and to colonize the rhizosphere (Hu and St. Leger, 2002; Vega et al., 2009; Rivas-Franco et al., 2019). This property not only allows entomopathogenic fungi to persist in the root system, but also fulfill additional ecological roles such as antagonism of plant pathogens, plant growth promotion, biofertilization, and promotion of the plant induced resistance (Goettel et al., 2008; Vega et al., 2009; Sasan and Bidochka, 2012; Rivas-Franco et al., 2020). In order to make use of these advantages, an alternative to MS formulated as granules (0.6–1.2 mm) and incorporated into soils at planting is to formulate the MS directly as a seed coating. Therefore, after sowing, MS coated onto seeds reactivated by soil humidity produce hyphae in a process known as MS germination. When nutrients in microsclerotia are scarce, hyphae develop phialides which, in turn, produce fresh conidia. These conidia are released in proximity to the nutrient-rich environment of the rhizosphere, where conidia soon germinate, and produce hyphae. Finally, either hyphae from microsclerotia or from conidia will interact and associate with the growing roots. Entomopathogenic fungal colonization of the rhizosphere allows fungal persistence, exerting biocontrol activity against the pests or plant pathogens threatening plant roots (Rivas-Franco et al., 2019).
Previous studies with seed treatments with different species of Metarhizium, resulted in 50% of larvae of Anomala cincta (Coleoptera: Rutelidae) or up to 60% of Costelytra giveni larvae (Coleoptera: Scarabaeidae) infected with the entomopathogenic fungi (Peña-Peña et al., 2015; Rivas-Franco et al., 2019). However, the biocontrol ability of Metarhizium is not limited only to insects but also against plant pathogens like Fusarium. Several studies have shown that Metarhizium spp. can reduce Fusarium root rot symptoms after seeds were coated with conidia obtained from this biocontrol agents (Sasan and Bidochka, 2013; Rivas-Franco et al., 2019). Additionally, maize roots provide support for Metarhizium rhizosphere colonization as well as increasing fungal persistence in the root system through endophytic growth (Keyser et al., 2014; Peña-Peña et al., 2015; Rivas-Franco et al., 2020). The ability of Metarhizium to colonize the rhizosphere and plant roots endophytically supports the bodyguard hypothesis (Elliot et al., 2000) where the host plant plays an important role driving this interaction in the presence of insect pests or phytopathogens threatening roots (Rivas-Franco et al., 2020). Previously, we found that several species of Metarhizium coated on maize seeds showed a significant decrease in their ability to colonize the rhizosphere in the presence of larvae of C. giveni, while endophytic colonization by Metarhizium increased in the presence of the pathogen Fusarium graminearum (Rivas-Franco et al., 2020). In those studies, root colonization by Metarhizium spp. altered maize physiology modifying the content of the phytohormones salicylic acid and jasmonic acid, possibly leading to induction of the systemic response in maize (Rivas-Franco et al., 2020).
Numerous species of the genus Fusarium are well-known plant pathogens affecting yield, nutritive value and hygienic quality of agricultural crops in most countries from Africa, Asia, Europe, North America, Oceania, and South America (van der Lee et al., 2015; Oldenburg et al., 2017; Kazan and Gardiner, 2018). In particular, the Fusarium graminearum Schwabe species complex cause Fusarium head blight in several plant cereals leading to yield losses above 50% in some cases and compromising grain quality (van der Lee et al., 2015; Garmendia et al., 2018). Infections of maize with Fusarium spp. can cause other serious plant diseases such as seedling blight, seed rot, root and stem rot, ear and kernel rot, and rudimentary ear rot (Oldenburg et al., 2017; Zhou et al., 2018). Fusarium graminearum also produces a class of mycotoxins known as trichothecenes (deoxynivalenol, nivalenol, and their derivatives) and zearalenone, with acute toxicity for animals and humans (van der Lee et al., 2015; Kazan and Gardiner, 2018; Zhou et al., 2018). Maize plants are susceptible to Fusarium spp. infections throughout the cultivation period and mycotoxins can be accumulated in the affected tissues and grains, which represents a risk for human and animal health (Oldenburg et al., 2017; Kazan and Gardiner, 2018). Management of F. graminearum can be difficult because standard seed fungicide treatments are not always sufficient, while aerial applications are costly. Additional difficulties are associated with correct application timing, environmental risk and the development of Fusarium resistance to fungicides (Kazan and Gardiner, 2018; Zhou et al., 2018).
Seed coating with conidia from entomopathogenic fungi has proved to be a strong tool for the delivery of these biocontrol agents, promoting fungal persistence in the system after entomopathogenic fungal colonization of the rhizosphere or even roots endophytically (Rivas-Franco et al., 2019). The aim of this study was to evaluate MS production by different isolates of entomopathogenic fungi and determine the effects of MS coated onto maize seeds on plant growth performance in the presence of F. graminearum. The ability of the entomopathogenic fungal isolates, coated on seeds as MS, to associate with roots was determined through fluorescent and laser confocal microscopy.
Materials and Methods
Plants
Maize seeds (Zea mays) of the hybrid 34H31 (US patent 6,897.360 B1) were used. This hybrid is characterized by a stable yield performance in different environments, early flowering and good drought tolerance.
Fungal Isolates
The isolates selected for this study (Table 1) are all held in the fungal collections of the Bio-Protection Research Center and AgResearch (both at Lincoln, New Zealand) and the Agricultural Research Service Collection of Entomopathogenic Fungal Cultures (ARSEF–USDA). The plant pathogen F. graminearum 13083 was obtained from a maize root rot sample and held in the Manaaki Whenau Landcare Research culture collection (Auckland, New Zealand). For liquid culture fermentation studies, monosporic cultures of each fungal isolate were used. Monosporic cultures were obtained by transferring a sole conidium from each fungal isolate to a Petri plate containing potato dextrose agar (PDA, Difco) and incubating at 25 ± 2°C in light:dark conditions (12:12). After 3 weeks, a conidial suspension was prepared for each fungal isolate from the monosporic fungal colony grown on PDA. The resulting monosporic conidial suspension was aliquoted in tubes with 10% glycerol and stored at −80°C (stock cultures) until use. Conidial inoculants for liquid culture experiments were obtained by inoculating PDA Petri plates with 100 μL from fungal stock cultures. Petri plates were then incubated at 25 ± 2°C in light:dark conditions (12:12) for 2 weeks until complete sporulation of colonies. Subsequently, a 250 mL flask containing 90 mL of liquid medium was inoculated with 10 mL of a conidial suspension of 5 × 106 conidia/mL. All conidial suspensions were obtained by rinsing the fungal colony grown on a PDA plate with 3–5 mL of 0.01% Triton X-100 and transferring the resulting conidial suspension to a 15 mL Falcon tube. Conidial suspensions were quantified using a haemocytometer (Neubauer Improved).
Fungal Growth and Microsclerotia Production in Liquid Substrate Fermentation
Media Composition and Fermentation Conditions
Media composition and fermentation conditions were as described by Jackson and Jaronski (2009, 2012) with slight modifications in carbon and nitrogen content. The liquid media used to produce hyphal inoculum and MS from the fungal isolates were composed of a basal salts solution with trace metals and vitamins (Jackson and Jaronski, 2009). The pre-culture medium [C:N ratio 33:1; (C) = 40 g/L] used for producing the hyphal inoculum of fungal isolates contained the basal medium supplemented with 80 g/L glucose and 15 g/L acid hydrolysed casein (Casamino acids, Difco). Production medium for MS contained the same composition but with 25 g/L of hydrolysed casein [C:N ratio 23:1, (C) = 45 g/L]. Carbon concentration and C:N ratio calculations were based on 40% carbon in glucose and 53% carbon and 8% nitrogen in acid hydrolysed casein (Jackson and Jaronski, 2009). Glucose solutions (20% w/v) were autoclaved separately and added to the basal salts solution with trace metals and vitamins prior to inoculation. Pre-cultures were obtained by inoculating 90 mL pre-culture medium in baffled Erlenmeyer flasks (250 mL) with 10 mL conidial suspension of 5 × 106 conidia/mL. Production cultures were obtained by inoculating 90 mL of production medium in baffled Erlenmeyer flasks (250 mL) with 10 mL of 4 day-old pre-culture broth. Production cultures were grown for 8 days at 28°C in an orbital shaker incubator (Cocono TU 4540) at 300 RPM. Total fermentation time for pre-cultures plus production cultures was 12 days. All pre-cultures and production cultures were inoculated in duplicate. All experiments were carried out at least three times.
Quantification Methods and Sample Processing During Liquid Fermentations
Samples for the determination of biomass, blastospore and MS were taken after inoculation for pre-cultures (days 3 and 4) and for production cultures (days 3, 4, 6, 7, and 8). At each sampling day, 5 mL replicates of culture broth were taken from each isolate and processed for quantification of biomass, blastospore, and MS content determination. Biomass was determined as dry weight after incubating 1 mL of culture broth in a 1.5 mL Eppendorf tube for 96 h at 65°C. Before drying biomass, the medium culture was removed by two consecutive washes with 1 ml of 0.01% Triton X-100 solution. Blastospore concentration was determined by transferring 1 mL of culture broth to a tube containing 9 mL of distilled water and quantifying microscopically using a haemocytometer (Neubauer Improved). MS production was determined using 70 μL of sample placed onto a glass slide and covered with a large (50 × 24 mm) coverslip. All MS on the slide were counted. Only discrete hyphal aggregates larger than 50 μm in diameter and melanized were counted as MS (Jaronski and Jackson, 2012). All quantifications were done in duplicate. Culture broth was diluted as appropriate for quantifications and all broth suspensions were vortexed to ensure homogeneity before quantification. Microscopic analysis was done using a Leica DM 2500 microscope and images were taken with the Celsens Standard software (Olympus). Quantifications (biomass, blastospores, and MS) from each sampling day and fungal isolate were averaged. Data were analyzed by a two-way analysis of variance (ANOVA).
Microsclerotia Production for Characterization and Seed Coating
Microsclerotia for maize seed coating were produced following the procedure indicated above using the isolates of M. anisopliae A1080 and F672 and M. robertsii F447. These isolates exhibited highest MS production among remaining Metarhizium isolates and were selected for the following studies. The pre-cultures for biomass and blastospore production were evaluated only on day 4 after inoculation, and production cultures were evaluated for biomass and MS production on harvest at day 6 after inoculation. MS production cultures were completed using two replicates per isolate. Experiments were repeated three times. Biomass and MS quantification from each fungal isolate at sampling day were averaged for data analysis.
Microsclerotia Harvest and Drying Process
After six fermentation days, total fungal biomass volume including MS was determined and 5% (W/V) of diatomaceous earth (DE, Hyflo Sigma-Aldrich) was added. The combined MS-DE were mixed, and vacuum filtered with a Büchner funnel using Whatman N°54 filter paper. The resulting filtered MS-DE solid block was broken up with a coffee blender by applying short pulses until small crumbs were obtained. The resulting crumbs were layered on glass Petri plates of 15 cm diameter and air-dried overnight in the air flow within a laminar-flow. The moisture content of the MS-DE granules was determined with an activity meter (Aqualab Lite V4). The granules were dried until the water content was between 3–5 % and then sealed in plastic bags and stored at 4°C until further processing. Moisture content (aw) determination from the MS of each fungal isolate was completed in duplicates. Each experiment was repeated three times. Data were analyzed by a two-way ANOVA.
Viability and Conidiogenesis of MS-DE Granules
Microsclerotial viability and conidial production from MS were determined by sprinkling 25 mg of the air-dried MS–DE granules onto the surface of a water agar plate (Jackson and Jaronski, 2009). Two water agar plates were used for each of the MS-DE granules obtained from the corresponding Metarhizium isolate. After 24 hrs incubation at 28°C in light:dark conditions (12:12 h), a total of 100 MS–DE granules on each plate were examined with a stereo microscope (Olympus SZX 12) to determinate hyphal emergence (MS germination) as an indicator of viability. Conidial production was determined after further incubation of MS-DE granules on water agar plates in the same conditions for 8 days. Then, MS-DE water agar plates were flooded with 7 mL of a sterile 0.01% Triton X-100 and agitated at 60 RPM in a rotary shaker platform for 30 min at room temperature. After agitation, granules were dislodged from agar with the help of a hockey stick cell spreader and the resulting suspension containing granules and conidia was transferred to a 15 mL Falcon tube, and the recovered volume was recorded. The concentration of conidia was determined microscopically using a haemocytometer (Neubauer Improved) and total conidia per gram of MS was calculated from duplicate samples. Experiments were repeated three times. Data were analyzed by a two-way ANOVA.
Maize Seed Coating With Microsclerotia
After MS characterization, isolates M. anisopliae F672 and M. robertsii F447 were selected for maize seed coating based on their high MS production. Seed coating was prepared as described in Rivas-Franco et al. (2019) with slight modifications as follows. The conidial suspension in the polymer gel was substituted by an amount of MS-DE estimated to provide, after MS germination and conidiation, a final conidial concentration of ~1 × 108 conidia/mL per gram of seeds. The corresponding quantity of MS-DE was added to the polymer gel, mixed and then used for coating maize seeds with the same proportions of polymer with MS-DE, bentonite and talc as previously described (Rivas-Franco et al., 2019). Control seeds (CS) were coated with the polymer and the other corresponding ingredients without the microsclerotia. Germination of maize seeds with a MS-DE coating was determined using the between-paper method as described in Rivas-Franco et al. (2019). In this evaluation test four replicates, each consisting of 50 maize seeds with MS-DE coating or CS, were used. Experiments were repeated twice. Statistical design was a randomized block design with two blocks of the MS fungal seed coatings treatments. Data were analyzed with a one-way ANOVA.
MS-DE Coated Maize Seeds Propagule Viability
After maize seeds were coated with Metarhizium MS-DE granules, 10 g of coated seeds were placed in a Falcon centrifuge tube (50 mL) containing 30 mL of a solution of Triton x-100 (0.01%). The resulting mix composed of seeds and solution was agitated vigorously and incubated at 4°C overnight (mother suspension). After incubation, the mix was agitated vigorously and serial dilutions from mother suspension were prepared. From each dilution 0.1 mL was spread onto Petri plates containing half strength acidified PDA. To prepare half strength acidified PDA, two drops of Lactic acid were added to the sterilized medium before pouring in the Petri plates. This simple strategy of medium acidification reduces bacterial contamination with no effects on fungal development. Inoculated plates were incubated at 20°C with 12:12 h light:dark conditions. After 7 days, fungal colonies originated from MS were quantified as colony forming units (CFU). Data were analyzed by a one-way ANOVA.
Evaluation of Maize Plant Performance in the Presence of Fusarium graminearum After Seeds Were Coated With Microsclerotia of Metarhizium spp
Maize seeds coated with MS from M. anisopliae F672 and M. robertsii F447 were sown in 1 L pots containing 920 g of potting-mix with 0.5% (w/w) of F. graminearum 13083. Control seeds without MS treatment were grown in the absence (CS) or the presence of F. graminearum (CSfg). Plants were allowed to grow in the Biotron (controlled environment facility, Lincoln University), at 24.3 ± 2.5°C, (referred as 25°C) in light:dark conditions (16:8 h), 65 ± 10 RH% and watered every fourth day with 300 mL of tap water. Three weeks after sowing, plants were harvested and dry weight of shoots and roots determined (Rivas-Franco et al., 2019). Each maize plant trial consisted of two randomized blocks with five pots in each block with the corresponding MS seed coating treatments (M. anisopliae F672 and M. robertsii F447) and CS. All pots contained the fungal pathogen F. graminearum 13083 incorporated in the soil potting mix. Additionally, there were two randomized blocks, also with five pots in each block, with the corresponding CS without the fungal pathogen in the potting mix. Experiments were repeated four times. Data were analyzed by a two-way ANOVA.
Fusarium Graminearum 13083 Biomass Production for Maize Trials With MS-DE Coated Seeds
Fusarium graminearum isolate 13083 was maintained in slant tubes on PDA at 4°C and grown on PDA plates at 25°C with 12:12 h light:dark conditions for experiments. For maize trials, F. graminearum 13083 biomass was obtained as follows. From the edge of a Fusarium colony grew in PDA for 14 days, 10 plugs of ~0.5 cm diameter were taken and transferred to 1 L Erlenmeyer flasks containing 500 mL of malt extract broth (MEB). Flask were incubated in an orbital shaker at 180 rpm and 25 ± 2°C. After 7 days, biomass was harvested by centrifugation at 400 rpm for 5 min at 4°C. Following this procedure, up to 69.7 ± 3.4 g/L of F. graminearum 13083 biomass containing ~1.3 ± 0.5 (× 108) blastospores/mL was obtained. Then, the F. graminearum 13083 biomass was incorporated with the potting mix at 0.5% w/w and mixed by hand to homogenize. Immediately, the mix was used to fill pots and then CS and MS-DE coated maize seeds were sown. Filled pots were watered and maize plants let to grow for 3 weeks at 25°C before plant performance evaluation.
Determination of Fungal Endophytism by Confocal Fluorescent Microscopy After Maize Seed Coating With Metarhizium spp MS-DE Granules
Maize seeds were coated with MS from M. anisopliae F672 and A1080, and M. robertsii F447 as described above, while for CS the coating consisted of polymer gel without MS. After coating, maize seeds were sown in 1 L pots containing 600 g of vermiculite (fine grade 2). Pots were watered with 400 mL of tap water, and transferred to a growth chamber at 25°C with light:dark conditions (12:12 h). Plants were watered every fifth day with 400 mL of distilled water. After 1 month, maize plants were gently pulled out, and maize roots, stems and leaf sheath were cleared and fluorescent dyed as described by Rivas-Franco et al. (2019). Maize samples of roots, stems and leaves from seeds coated with MS, and control plants were analyzed for the presence of fungal structures on the surface or internally as endophytes using confocal microscopy (LSM 510 META–Zeiss, Germany). Confocal fluorescence images were recorded on a multichannel confocal microscope (LSM 510 META–Zeiss, Germany) using the program ZEN 2009.
Statistical Analysis
For each individual trial data were analyzed by the ANOVA test that was appropriate for the experimental design. For treatment factors with several levels (e.g., isolates, trial, day), an unrestricted LSD procedure was used to compare means (Saville, 2015). To combine data over several identical trials, an ANOVA test was used, as above, followed by an unrestricted LSD procedure, with data being treatment means from individual trials, and with “trial” being specified as a blocking factor.
Results
Fungal Growth and Microsclerotia Production in Liquid Substrate Fermentation
Biomass Production
All the fungal isolates had produced their highest biomass by day 3 (89.7 ± 16.5 mg/mL) and 4 (87.1 ± 15.1 mg/mL) after inoculation followed by a subsequent steady weight decrease (F28/78 = 5.1; p < 0.01; Figure 1A). By day 7, Trichoderma harzianum F327 and B. bassiana Bb21 had the lowest biomass among the isolates, 38.4 and 42.2 mg/mL, respectively, while M. anisopliae F672 and M. novozealandicum F99 had the highest 68.4 and 71.7 mg/mL also, respectively (LSD5% = 0.075; Figure 1A). At the end of the fermentation process on day 8, only T. harzianum F327 and B. bassiana Bb21 showed a significant increase in biomass compared to their biomass on day 7 (LSD5% = 0.075; Figure 1A).
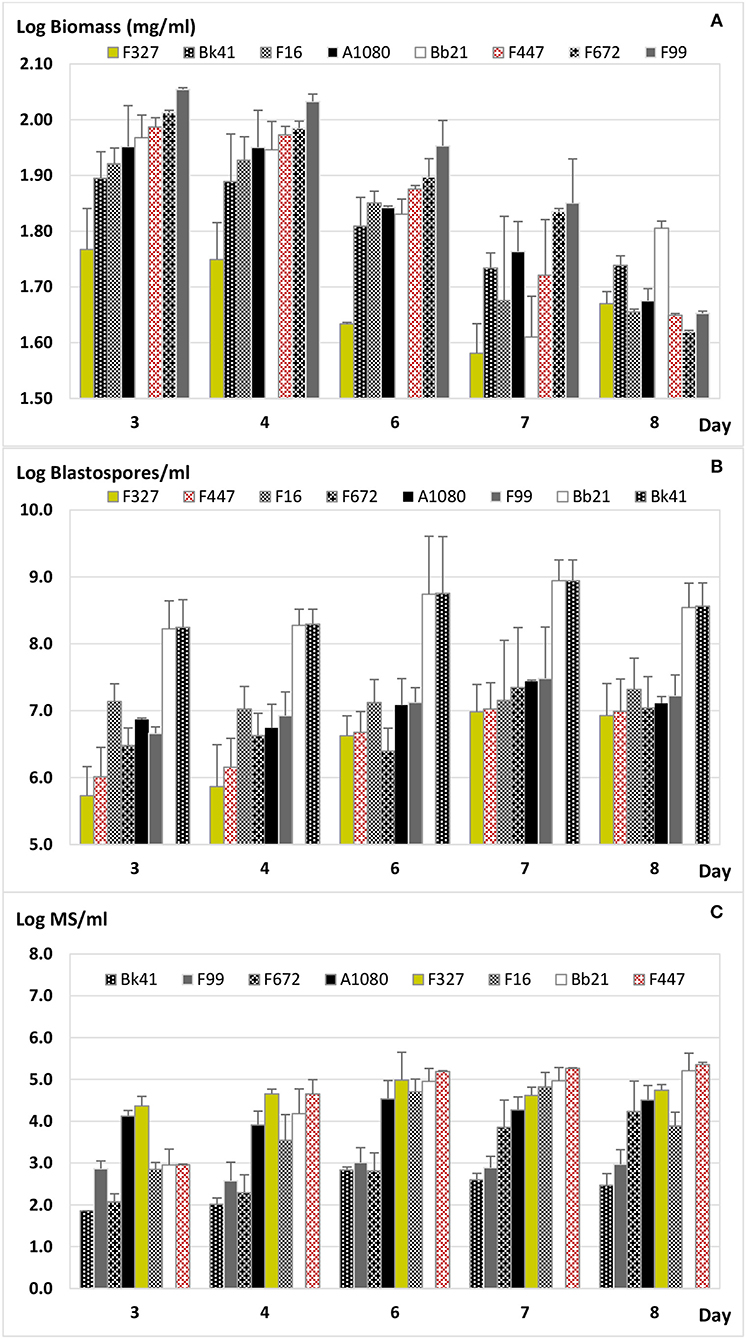
Figure 1. Fungal growth in liquid fermentation in basal salts solution with trace metals and vitamins (C:N ratio 23:1). (A) Fungal biomass (dry weight Log10 mg/mL); LSD5% = 0.075, p < 0.01. (B) Blastospore production (Log10 number/mL); LSD5% = 0.557, p = 0.288. (C) Microsclerotia production (Log10 number/mL); LSD5% = 0.498, p < 0.01. Error bars = standard error.
Blastospore Production
Contrary to the development of fungal biomass, blastospore production of each isolate increased over the fermentation period but with almost no significant variation during consecutive sampling days (F28/78 = 1.2; p = 0.288; Figure 1B). Only T. harzianum F327 and M. robertsii F447 had a significant increase in the number of blastopores per mL by day 6 of the experiment, 1.4 × 107 and 1.9 × 106 blastospores/mL, respectively (LSD5% = 0.557). In contrast, the remaining isolates had the highest number of blastospores per mL by day 7. The highest production of blastospores was by B. bassiana Bb21 and M. guizhouense Bk41, both with 9.1 × 108 blastopores/mL, while the lowest was found in T. harzianum F327 with 9.6 × 106 blastospores/mL (LSD5% = 0.557; Figure 1B).
Microsclerotia Production
As well as the trend observed with blastospore production, the amount of MS increased with fermentation time for most of the isolates. The highest concentration, 7.3 ± 7.9 (× 104) MS/mL, was found around the sixth day after inoculation and the level remained stable until the last sampling day (F28/78 = 7.0; p < 0.01). The exception was M. novozealandicum F99 which showed little variation in MS content over the 8 days of fermentation (LSD5%=0.498; Figure 1C). This isolate, together with M. guizhouense Bk41, produced, on average, the lowest number of MS, 9.9 ± 4.3 (× 102) MS/mL (Figure 1C). At the end of the fermentation process, most MS were melanised heterogenous round structures of differing sizes (Figure 2). The highest MS production was determined in M. robertsii F447 at day 8 with 2.3 × 105 MS/mL followed closely by B. bassiana Bb21 with 1.6 × 105 MS/mL (LSD5% = 0.498). The lowest production of MS was found in M. guizhouense Bk41 with 684 MS/mL at day 6, while the plant growth promotor T. harzianum showed its highest production with 9.7 × 104 MS/mL, also at 6 days after inoculation (Figure 1C). Microsclerotia formation during fermentation were first recorded on day 3, undergoing on the following days different developmental stages until complete melanization was achieved (Figure 2). At days 3 and 4 after inoculation, MS were still immature as aggregated hyphae were not completely melanized. By day 6, all isolates had slightly melanized compact hyphal structures, conforming to previous descriptions of MS structures. The stages in MS development differed among the isolates in rate of development, size and melanization. For example, MS from T. harzianum F327 were relatively small when compared to those from Metarhizium isolates. The MS in M. guizhouense melanized faster than those in M. novozealandicum F99 (data not shown). The longer the fermentation time, the greater melanization of the MS. Complete melanization of MS occurred after 7 days of fermentation culture (Figure 2).
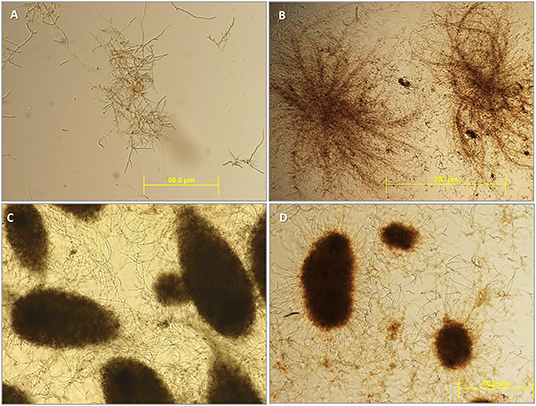
Figure 2. Microsclerotia development during liquid fermentation in basal salts solution with trace metals and vitamins (C:N ratio 23:1). The initial process for the formation of microsclerotia (MS) started early in the fermentation under liquid conditions with the formation of hyphal aggregates [(A,B), 4 days after inoculation]. After 7 days of inoculation, the MS formed as round structures with compact pseudoparenchymatous cells layers highly melanised (C,D). Images from: M. anisopliae A1080 (A); M. robertsii F447 (B); M. anisopliae F672 (C); and from M. novozelandicum F99 (D).
Microsclerotia Production for Seed Coating and Characterization
The isolates M. anisopliae A1080 and F672, and M. robertsii F447 were selected for MS production and incorporation into a coating for maize seeds. These isolates produced the highest concentration of MS among the Metarhizium isolates. Interestingly, they were obtained from different sources, M. anisopliae A1080 from an insect and the other two isolates from plant samples as endophytes (Table 1). Following the procedure for MS production described above, cultures yielded on average around 80 mg/mL of biomass and ~1 × 105 MS/mL (Figure 3).
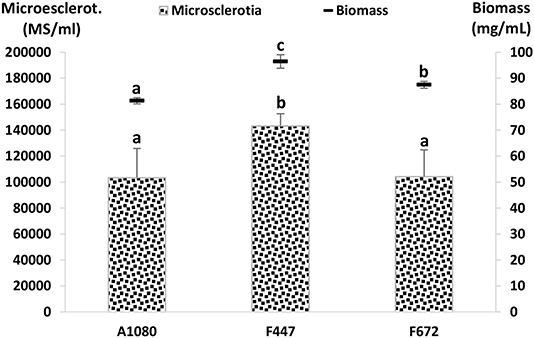
Figure 3. Biomass and MS production by entomopathogenic fungi in basal salts solution with trace metals and vitamins (C:N ratio 23:1). Biomass (mg/mL); LSD5% = 4.9, p < 0.01. Microsclerotia (MS/mL); LSD5% = 32,542.3, p < 0.05. Error bars = standard error.
All MS-DE granules obtained from the three isolates had germinations rates above 90% with no significant differences among isolates (F2/13 = 1.59; p = 0.242; Table 2). However, isolate-specific differences were found in the water content of MS-DE after drying. The lowest water content was found in M. anisopliae F672 granules (Table 2). Conidia production by the different MS-DE granules was always above 1 × 109 conidia/g, with the highest production achieved by M. anisopliae F672 (p < 0.01; Table 2).
Maize seeds were coated with an amount of MS-DE granules which provided an estimated final concentration of 1 × 108 conidia per gram of seeds. Control seeds (CS) were coated with the same amount of DE and the rest of the coating ingredients, but without fungal MS or conidia. Seeds coated with MS-DE from M. anisopliae F672 had a viable conidia production of 7.8 × 106 CFU per gram of maize seeds. This did not differ from M. anisopliae A1080 but was greater than for M. robertsii, which produced only 2.0 × 106 CFU per gram of maize seeds (Figure 4). These CFU values were expected since previous results showed that CFU decreased an estimated two orders in relation to the initial conidial concentration coated onto maize seeds. From CS no fungal colonies were obtained.
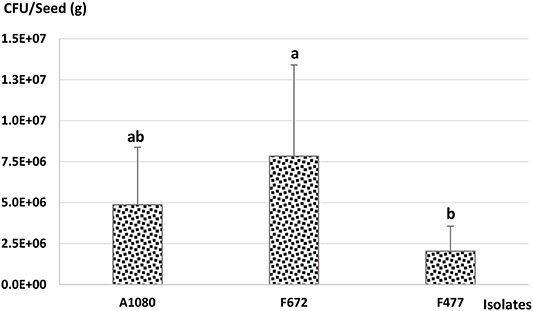
Figure 4. Quantification of viable conidia produced from MS-DE coated on maize seeds. The final entomopathogenic fungal loading on maize seeds after seed coating with MS granules was determined as colony forming units (CFU) per gram of coated seeds; LSD5% = 4.6 × 106, p < 0.05. Error bars = standard error.
Maize Plant Performance in the Presence of Fusarium graminearum After Seeds Were Coated With Microsclerotia of Metarhizium spp
Maize Plant Dry Weight
Maize growth, determined as total dry weight of shoots and roots, differed significantly among treatments (F3/153 = 145.6; p < 0.01). Maize dry weight was highest in CS in the absence of F. graminearum, while CS in the presence of this phytopathogen (CSfg) had a significantly lower dry weight (Figure 5; LSD5% = 0.029). In the presence of F. graminearum, maize plants with the Metarhizium spp. MS-DE coated seed treatments had a significantly greater dry weight than CSfg (LSD5% = 0.029). In the presence of F. graminearum, plants coated with the M. anisopliae F672 MS-DE treatment had, on average, a dry weight gain of 32% compared to the maize plants without the coating, while the dry weight of plants was almost 26% lower when compared to CS in the absence of F. graminearum (Figure 5; F3/153 = 145.6; p < 0.01).
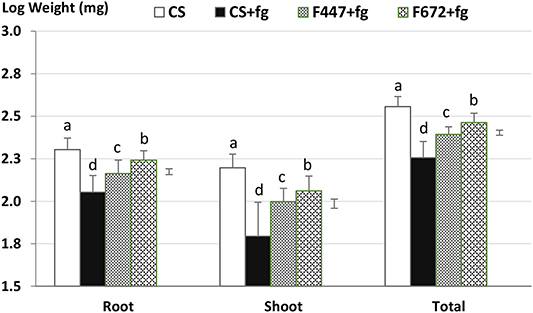
Figure 5. Maize dry weight of three-week old potted plants from seeds coated with Metarhizium microsclerotia or coated without fungus (control seeds; CS) and grown in the absence or in the presence of Fusarium graminearum. Maize seeds were coated with microsclerotia from Metarhizium robertsii F447 or M. anisopliae F672 and sown in potting mix containing Fusarium graminearum. After 3 weeks, plants were harvested for the determination of dry weight. CS, control plants in absence of F. graminearum; CSfg, control plants in presence of F. graminearum; F447 and F672, maize plants from seeds coated with microsclerotia from M. robertsii F447 or M. anisopliae F672, respectively. Error bars = standard deviation. Bars = LSD5% (Roots = 0.034; Shoots = 0.054; Total = 0.029); p < 0.01.
The negative effect of F. graminearum on total plant dry weight was due to the phytopathogen's impact on both shoot and root dry weight, which was significantly lower in the CSfg compared to the CS treatment (Figure 5; p < 0.01). The Metarhizium spp. seed coating with MS-DE partially compensated this reduction in shoot dry weight caused by the plant pathogen. Compared to CS grew in the absence of F. graminearum, the dry weight of shoots from plants treated with M. anisopliae F672 was reduced by 27% (F3/153 = 73.5; p < 0.01). In plants treated with M. robertsii F447 a 37% shoot dry weight reduction occurred when plants were grown in the presence of F. graminearum (Figure 5; LSD5% = 0.054). In the presence of the phytopathogen maize plants with MS-DE treatments had a greater root dry weight when compared to plants without a fungal treatment (Figure 5; LSD5% = 0.034). However, in the presence of F. graminearum, dry weight of roots from plants with the MS-DE treatments with M. anisopliae F672 and M. robertsii F447 were 14 and 28%, respectively, lower than root dry weight in CS in the absence of F. graminearum (Figure 5; F3/153 = 80.5; p < 0.01).
Determination of Fungal Endophytism by Confocal Fluorescent Microscopy After Maize Seed Coating With Metarhizium spp. MS-DE Granules
The presence of hyphae on and in root tissues was observed in all the Metarhizium MS-DE treatments but not in samples from CS without fungal treatment. Fungal hyphae growing on the surface of roots were observed growing in proximity to the root crown, in seeds remains and all along the length of the root, but not in root apices. The protrusion zone, where secondary roots emerge, seemed to be one of the access points into the roots. In this area, extensive colonization by M. anisopliae A1080 was observed (Figures 6A–D). The presence of hyphae growing inside the cortical root cells was observed 1-month after sowing the MS-DE coated seeds (Figure 7). The lectin, CoA-AF688, binds to glycoproteins, α-mannopyranosyl, and/or α- glucopyranosyl residues allowing the identification of hypha penetration sites through the root cell wall which are visualized as red dots (Figure 7A, arrow heads). The constriction points in the hypha also confirmed these penetration zones (Figure 7B, arrow heads). At this plant stage, the roots showed extensive fungal colonization on the surface of the roots but also endophytic colonization (Figure 7D).
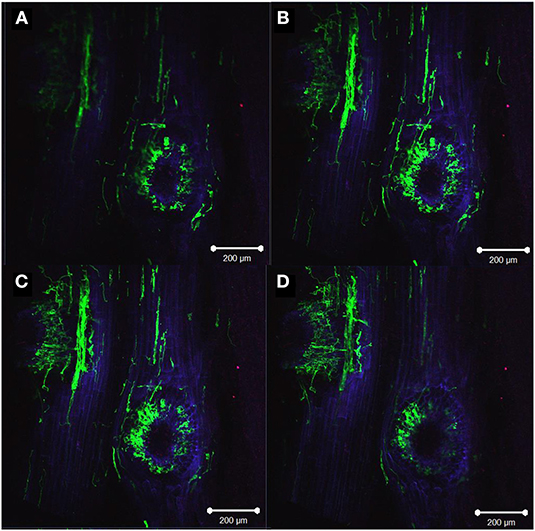
Figure 6. Metarhizium anisopliae A1080 colonization of roots from two weeks old maize plants, showing the protrusion zone of secondary root emergence with fungi growing on the surface and inside the tissues. Overlay of images: plant membranes were stained with propidium iodide (blue) and Congo red (red), while fungal structures were stained with WGA-AF488 (green). Z-Stack image (A–D): upper left image (A) distal focus, bottom right panel (D) closest focus (total distance 51.7 μm). Images were taken with a confocal microscope (LSM 510 META–Zeiss) using the program ZEN 2009. The bar represents 200 μm.
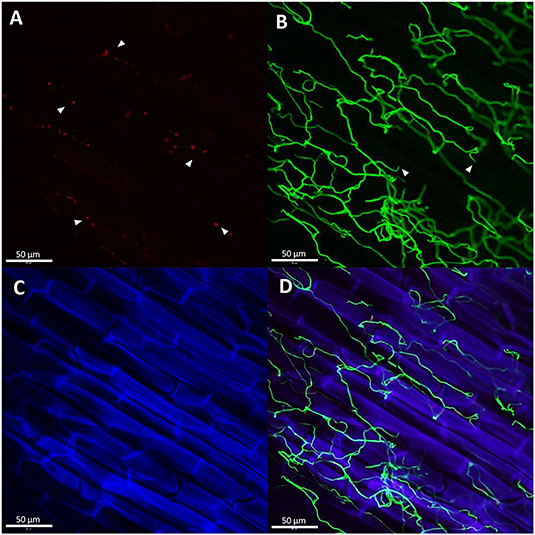
Figure 7. Metarhizium anisopliae F672 hyphae growing on 1-month old maize roots. The presence of glycoproteins, α-mannopyranosyl, and/or α- glucopyranosyl residues, around hyphal adhesion sites were stained with ConA-AF633. These sites indicate the hyphal penetration points through the root cell wall visualized as red dots [(A), arrowheads). The net of hyphae growing around and inside the root cell stained with WGA-AF488 are visualized in green (B). The constriction zones in the hypha also confirm the vegetal cell wall penetration points [(B), arrowheads]. The root cells were stained with propidium iodide visualized in blue (C). Overlay of the three previous images (D). Images were taken with a confocal microscope (LSM 510 META–Zeiss) using the program ZEN 2009. The bar represents 50 μm.
Samples of stems and leaves were also analyzed in all the MS-DE treatments by fluorescent and confocal microscopy, but no endophytic colonization was observed. At least 1 month after sowing Metarhizium MS-DE coated seeds, fungal colonization seemed to be limited only to roots.
Discussion
In this study the production and formulation of microsclerotia (MS) by isolates of M. anisopliae, M. guizhouense, M. novozealandicum, M. robertsii, B. bassiana, and T. harzianum was determined. All isolates produced MS, compact hyphal aggregates that become pigmented with culture age, in addition to more typical blastospores and mycelia. Previous reports have reported the production of these resistant structures in M. anisopliae, M. brunneum, M. humberi, M. rileyi, M. robertsii, B. bassiana, B. brogniartii, B. pseudobassiana, and T. harzianum (Jaronski and Jackson, 2008; Jackson and Jaronski, 2009; Behle et al., 2013; Kobori et al., 2015; Villamizar et al., 2018; Lira et al., 2020). However, this study also reports on the production of MS in several Metarhizium species, T. harzianum, and B. bassiana simultaneously, and it is the first report of MS obtained from isolates of M. guizhouense and M. novozealandicum.
The biomass obtained by the New Zealand Metarhizium spp. isolates (42–55 mg/mL) was higher than values previously reported which vary between 7.0 and 33.0 mg/mL (Jackson and Jaronski, 2009; Mascarin et al., 2014). In a previous study with M. rileyi, with a biomass production of 40.7 mg/mL (Song et al., 2016), similar values were obtained as in the present study. The highest biomass production was in B. bassiana Bb21 with 64 mg/mL. This yield was higher than in previous work under similar conditions where biomass weight obtained was between 15.3 and 20.5 mg/mL (Bidochka et al., 1987; Lohse et al., 2014). The variances in biomass yield might be due to differences in growth conditions and media [C:N ratio 23:1, (C) = 45 g/L], or to the different isolates used. The reasons behind the biomass increase observed in Trichoderma and Beauveria isolates compared to Metarhizium could be to their well-known fast growth and the ability to produce different types of fungal propagules such as submerged conidia, blastospores, blastoconidia, and budding hyphal cells from the developing hyphae (microcycle conidiation).
The production of blastospores by the M. anisopliae isolates varied between 6.5 × 105 and 1.3 × 107 blastospores/mL after six fermentation days. Blastospore concentration obtained was slightly lower than that reported by Jackson and Jaronski (2009) for Metarhizium spp., which was around 2.0–1.6 × 108 blastospores/mL after eight fermentation days in similar conditions. The differences in blastospore production might be based on the slight differences in C:N ratios but is more likely to be due to the intrinsic properties of the isolates used. Beauveria bassiana Bb21 blastospore production after 3 days of fermentation at 2.7 × 108 blastospore/mL was close to the values reported by Mascarin et al. (2015) of between 0.95 and 7.9 × 108 blastospores/mL in a similar study. However, in the present work, B. bassiana Bb21 maximum production, 4.3 × 109 blastospore/mL, was obtained after six fermentation days.
The quantity of MS obtained from both M. anisopliae isolates (3.5–4.0 × 104 MS/mL) was close to those reported previously by Jackson and Jaronski (2009) for M. anisopliae (1.8 × 104-6.4 × 104 MS/mL) using identical fermentation conditions and similar C:N ratios. Although in their study the highest production was by M. brunneum F52 (1.2 × 105 MS/mL), in this study the highest MS production was found in M. robertsii F447 (2.3 × 105 MS/mL). Mascarin et al. (2015) reported yields of around 6.1–7.3 × 106 MS/L for M. anisopliae, M. acridum, and M. robertsii isolates after growth in liquid fermentation for 3 days, with a maximum yield of 0.7–1.1 × 104 MS/mL after 5 days in liquid fermentation. In a different study with M. rileyi MS production of up to 9.7 × 104 MS/mL was reported (Behle et al., 2013). Lira et al. (2020) screened 48 isolates of different species of Metarhizium using only 3-day liquid fermentation obtaining a range from 1.3 × 102 to 3.6 × 103 MS/mL.
Evidently, all Metarhizium species tested are capable of producing MS although, as expected, the production of the resting structures varied between species and isolates, as reported previously (Lira et al., 2020). In this study, the lowest MS production was by M. guizhouense Bk41 with 3.3 × 102 MS/mL, while for M. guizhouense F16, 9.5 × 103 MS/mL was achieved. However, M. guizhouense Bk41 produced higher amounts of blastospores (2.6 × 107 blastospores/mL) than M. guizhouense F16 (6.1 × 105 blastospores/mL). This fact highlights the importance of isolate characterization to determine potential use and applicability. The results obtained in the current study confirm that growth conditions required for formation of MS can vary among fungal species and even among isolates of a particular species as stated also by Wang et al. (2013).
Another factor to consider during MS production is maturation. Although the production of these resistant structures can be obtained at an early stage of fermentation, a complete melanization of MS did not occur until after at least 6 days of liquid fermentation. The longer the fermentation process, the higher the melanization of the MS, not only in entomopathogenic fungi but also in T. harzianum (Jackson and Jaronski, 2009; Mascarin et al., 2014; Kobori et al., 2015). On average, MS can be observed after 4 days of fermentation, but this period might not be sufficient for complete melanization. Further studies are needed to determine the relationship between melanization and subsequent performance of MS. If these resistant structures are not fully mature, an early harvest, and following downstream processing (drying and formulation) could result in MS viability losses. Melanization has been associated with prolonged persistence in soil and resistance to desiccation (stress tolerance) in various filamentous fungi (Jackson and Jaronski, 2009; Kobori et al., 2015). Melanin has also been reported as a natural barrier against the harmful effects of UV-B radiation as well as resistance to toxic substances (Eisenman and Casadevall, 2012).
The rehydration and incubation of air-dried MS-DE granules from the isolates M. anisopliae A1080 and F672, and M. robertsii F447, on water agar plates resulted in hyphal formation after just 24 h. The development of sporogenic structures able to produce high numbers of conidia was obtained in less than a week. This was also reported in previous studies for M. brunneum and M. anisopliae (Jackson and Jaronski, 2009; Mascarin et al., 2014), Lecanicillium lecanii (Wang et al., 2013), T. harzianum (Kobori et al., 2015), M. robertsii, and M. acridum (Mascarin et al., 2014). In the current study, the concentration of conidia/g of dried MS-DE reached were one log higher than those reported by Jackson and Jaronski (2009), but similar to those values reported by Mascarin et al. (2014).
Lira et al. (2020), showed that coating maize seeds with MS is a feasible method for delivery of entomopathogenic fungi for biocontrol and plant growth promotion purposes. In our study, we demonstrated that after coating, MS were viable, and provided protection to maize plants by counteracting the negative effects of F. graminearum. As stated by Partida-Martínez and Heil (2011) the plant gain from any beneficial microorganism is mainly noticed when challenged by plant pathogens. Thus, the potential benefits of MS coated to maize seeds on maize growth was observed in the presence of the plant pathogen F. graminearum. Control plants grown in the absence of F. graminearum (CS) had significantly greater dry weight than plants treated with M. robertsii F447 or M. anisopliae F672. Conversely, in the presence of this phytopathogen, shoot development in plants grown from seeds coated with MS from M. robertsii and M. anisopliae was significantly better, when compared to maize plants from seeds without the MS coating.
Previous work found that bean or maize plants colonized by Metarhizium spp., and then exposed to F. solani or F. graminearum, had greater plant growth and lower disease indices compared with uncolonized plants (Sasan and Bidochka, 2013; Rivas-Franco et al., 2019). The mechanisms used by Metarhizium to counteract the negative effects of the presence of the plant pathogen could be due to multiple factors including competence for rhizosphere colonization, promotion of the plant induced response or production of Metarhizium secondary metabolites that inhibit Fusarium growth (Sasan and Bidochka, 2013; Ravindran et al., 2014). Thus, the overall results suggest that species of Metarhizium may be used as a control for plant pathogens as well as insect pests. The mechanisms behind the entomopathogenic fungi Metarhizium spp. ability to reduce the impact of this plant root pathogen represents an area that warrants further research.
Using laser confocal microscopy, we showed that the hyphae were closely associated with the ecto and endorhizosphere of maize roots. In the present work, two isolates, M. anisopliae F672, and M. robertsii F447, were obtained from plant material, Pinus radiata and Actinidia deliciosa, respectively, while M. anisopliae A1080 was isolated from a lepidopteran larva, Trichoplusia ni. The ability to produce MS as well as the capability to associate with maize roots seemed to be conserved in Metarhizium independent of origin. This reinforces the fact that Metarhizium, as soil dwelling fungi, have the capability to form resting structures to survive unsuitable conditions, and the ability to associate with roots which guarantees long term persistence.
Vega et al. (2009) stated “the ability of Metarhizium anisopliae to form sclerotia may be important for rhizosphere competence following a pattern seen in phytopathogenic fungi.” In this study, M. anisopliae and M. robertsii strongly colonized the differentiation and the root hair zones, inter- and intracellularly, and were infrequently detected in the elongation and meristematic zones. This colonization pattern was also observed in the plant pathogen Piriformospora indica (Zuccaro et al., 2011). However, the endophytic ability of Metarhizium and the ability to colonize cortical cell roots, places this genus closer to ecto- and arbuscular mycorrhizal fungi, which either grow intercellularly or predominantly colonize the deeper cortex layers of younger parts of the root (Zuccaro et al., 2011). This suggests that entomopathogenic fungi might also follow a pattern similar to that observed in P. indica and mycorrhiza. In P. inidica, the failure of WGA-AF488 to stain the hyphae inside living cells strongly suggested that the fungus remained enveloped in an intact plant-derived membrane throughout intracellular growth (Zuccaro et al., 2011). Metarhizium anisopliae and M. robertsii were observed inside the cells stained with the WGA-AF488 which may indicate that entomopathogenic fungi growing inside the plant cell were not enveloped by an endomembrane as in P. indica. Whether the association pattern between hyphae of entomopathogenic fungi and roots resembles a similar process to that of phytopathogenic fungi or mycorrhizae, or even represents a novel process, requires further studies.
In summary, the results obtained provide insights into liquid culture production of MS from different species of Metarhizium and demonstrates the potential use of these structures when coated onto seeds for the biocontrol of plant pathogens and plant growth promotion. Additionally, this MS coating strategy places the fungus close to the roots which can be colonized both superficially and endophytically. This fungus-root association allows persistence of the biocontrol agent, and places it close to harmful plant challengers such us plant pathogens and soil-dwelling insect pests. Innovation in the development of Metarhizium as a biocontrol agent needs to consider all its capabilities including insect pathogenicity, antagonism of fungal phytopathogens, root colonization, and stimulation of the plant induced response, making Metarhizium a multifunctional bioinput for plant health.
Data Availability Statement
The raw data supporting the conclusions of this article will be made available by the authors, without undue reservation.
Author Contributions
FR-F: conceptualization, methodology, investigation, formal analysis, and writing–original draft. JH, MR, TJ, MJ, and TG: conceptualization, resources, writing–review & editing, and supervision. NA: resources, writing–review & editing, and supervision. JS: conceptualization and methodology. PW: conceptualization, resources, and supervision. DS: validation, formal analysis, and writing–review & editing. All authors contributed to the article and approved the submitted version.
Funding
Operational funding was supplied by the Bio-Protection Research Center's Next Generation Biopesticide programme (CX10X1310 NZ MBIE) and Smarts Seeds Programme (LINX0702 NZ MBIE). The National Agency for Research and Innovation (ANNI—Uruguay) funded F. Rivas-Franco's PhD studies (POS_EXT_2014_1_105884).
Conflict of Interest
DS was employed by the institute Bio-Protection Research Centre, as a consultant to provide assistant in experimental design, statistics analysis and data processing involved in the different research lines carry on by the institute.
The remaining authors declare that the research was conducted in the absence of any commercial or financial relationships that could be construed as a potential conflict of interest.
Acknowledgments
We thank to the National Agency for Research and Innovation (ANNI–Uruguay) for funding FR-F Ph.D., studies (POS_EXT_2014_1_105884). We especially thank Dr. Artemio Mendoza for his invaluable advice for the Metarhizium transformation with the GFP protein as well as assistance with laser confocal microscopy observations. We also thanks to Dr. Verónica Ciganda (PPSA - INIA) for her support.
References
Behle, R. W., Jackson, M. A., and Flor-Weiler, L. B. (2013). Efficacy of a granular formulation containing Metarhizium brunneum F52 (Hypocreales: Clavicipitaceae) microsclerotia against nymphs of Ixodes scapularis (Acari: Ixoididae). J. Econ. Entomol. 106, 57–63. doi: 10.1603/EC12226
Bidochka, M. J., Pfeifer, T. A., and Khachatourians, G. G. (1987). Development of the entomopathogenic fungus Beauveria bassiana in liquid cultures. Mycopathologia 99, 77–83. doi: 10.1007/BF00436909
Burges, H. D. (1998). “Formulation of microbial biopesticides,” in Formulation of Microbial Biopesticides (Dordrecht: Springer).
Eilenberg, J., Hajek, A. E., and Lomer, C. (2001). Suggestions for unifying the terminology in biolgical control. BioControl 46, 387–400. doi: 10.1023/A:1014193329979
Eisenman, H. C., and Casadevall, A. (2012). Synthesis and assembly of melanin. Appl. Microbiol. Biotechnol. 93, 931–940. doi: 10.1007/s00253-011-3777-2
Elliot, S. L., Sabelis, M. W., Janssen, A., Van der Geest, L. P. S., Beerling, E. A. M., and Fransen, J. (2000). Can plants use entomopathogens as bodyguards? Ecol. Lett. 3, 228–235. doi: 10.1046/j.1461-0248.2000.00137.x
Garmendia, G., Pattarino, L., Negrín, C., Martínez-Silveira, A., Pereyra, S., Ward, T. J., et al. (2018). Species composition, toxigenic potential and aggressiveness of Fusarium isolates causing head blight of barley in Uruguay. Food Microbiol. 76, 426–433. doi: 10.1016/j.fm.2018.07.005
Goettel, M. S., Koike, M., Kim, J. J., Aiuchi, D., Shinya, R., and Brodeur, J. (2008). Potential of Lecanicillium spp. for management of insects, nematodes and plant diseases. J. Invertebr. Pathol. 98, 256–261. doi: 10.1016/j.jip.2008.01.009
Hu, G., and St. Leger, R. J. (2002). Field studies using a recombinant mycoinsecticide (Metarhizium anisopliae) reveal that it is rhizosphere competent. Appl. Environ. Microbiol. 68, 6383–6387. doi: 10.1128/AEM.68.12.6383-6387.2002
Jackson, M. A., Dunlap, C. A., and Jaronski, S. T. (2010). Ecological considerations in producing and formulating fungal entomopathogens for use in insect biocontrol. BioControl 55, 129–145. doi: 10.1007/s10526-009-9240-y
Jackson, M. A., and Jaronski, S. T. (2009). Production of microsclerotia of the fungal entomopathogen Metarhizium anisopliae and their potential for use as a biocontrol agent for soil-inhabiting insects. Mycol. Res. 113, 842–850. doi: 10.1016/j.mycres.2009.03.004
Jackson, M. A., and Jaronski, S. T. (2012). Development of pilot-scale fermentation and stabilisation processes for the production of microsclerotia of the entomopathogenic fungus Metarhizium brunneum strain F52. Biocontrol Sci. Technol. 22, 915–930. doi: 10.1080/09583157.2012.696578
Jaronski, S. T. (2014). “Chapter 11 - mass production of entomopathogenic fungi: state of the art,” in Mass Production of Beneficial Organisms, editors J. A. Morales-Ramos, M. G. Rojas, & B. O. Shapiro-Ilan (Academic Press), 357–413.
Jaronski, S. T., and Jackson, M. A. (2008). Efficacy of Metarhizium anisopliae microsclerotial granules. Biocontrol Sci. Technol. 18, 849–863. doi: 10.1080/09583150802381144
Jaronski, S. T., and Jackson, M. A. (2012). “Mass production of entomopathogenic hypocreales,” in Manual of Techniques in Invertebrate Pathology, 2nd Edn., ed L. A. Lacey (Yakima, WA: IP Consulting International).
Kazan, K., and Gardiner, D. M. (2018). Transcriptomics of cereal–Fusarium graminearum interactions: what we have learned so far. Mol. Plant Pathol. 19, 764–778. doi: 10.1111/mpp.12561
Keyser, C. A., Thorup-Kristensen, K., and Meyling, N. V. (2014). Metarhizium seed treatment mediates fungal dispersal via roots and induces infections in insects. Fungal Ecol. 11, 122–131. doi: 10.1016/j.funeco.2014.05.005
Kobori, N. N., Mascarin, G. M., Jackson, M. A., and Schisler, D. A. (2015). Liquid culture production of microsclerotia and submerged conidia by Trichoderma harzianum active against damping-off disease caused by Rhizoctonia solani. Fungal Biol. 119, 179–190. doi: 10.1016/j.funbio.2014.12.005
Lira, A. C., de, Mascarin, G. M., and Delalibera Júnior, Í. (2020). Microsclerotia production of Metarhizium spp. for dual role as plant biostimulant and control of Spodoptera frugiperda through corn seed coating. Fungal Biol. 124, 689–699. doi: 10.1016/j.funbio.2020.03.011
Lohse, R., Jakobs-Schönwandt, D., and Patel, A. V. (2014). Screening of liquid media and fermentation of an endophytic Beauveria bassiana strain in a bioreactor. AMB Express 4:47. doi: 10.1186/s13568-014-0047-6
Mascarin, G. M., Jackson, M. A., Kobori, N. N., Behle, R. W., and Delalibera Júnior, Í. (2015). Liquid culture fermentation for rapid production of desiccation tolerant blastospores of Beauveria bassiana and Isaria fumosorosea strains. J. Invertebr. Pathol. 127, 11–20. doi: 10.1016/j.jip.2014.12.001
Mascarin, G. M., Kobori, N. N., de Jesus Vital, R. C., Jackson, M. A., and Quintela, E. D. (2014). Production of microsclerotia by Brazilian strains of Metarhizium spp. using submerged liquid culture fermentation. World J. Microbiol. Biotechnol. 30, 1583–1590. doi: 10.1007/s11274-013-1581-0
Oldenburg, E., Höppner, F., Ellner, F., and Weinert, J. (2017). Fusarium diseases of maize associated with mycotoxin contamination of agricultural products intended to be used for food and feed. Mycotoxin Res. 33, 167–182. doi: 10.1007/s12550-017-0277-y
Partida-Martínez, L. P., and Heil, M. (2011). The microbe-free plant: fact or artifact? Front. Plant Sci. 2:100. doi: 10.3389/fpls.2011.00100
Peña-Peña, A. J., Santillán-Galicia, M. T., Hernández-López, J., and Guzmán-Franco, A. W. (2015). Metarhizium pingshaense applied as a seed treatment induces fungal infection in larvae of the white grub Anomala cincta. J. Invertebr. Pathol. 130, 9–12. doi: 10.1016/j.jip.2015.06.010
Ravindran, K., Chitra, S., Wilson, A., and Sivaramakrishnan, S. (2014). “Evaluation of antifungal activity of Metarhizium anisopliae against plant phytopathogenic fungi,” in Microbial Diversity and Biotechnology in Food Security (New Delhi: Springer), 251–255.
Rivas-Franco, F., Hampton, J. G., Morán-Diez, M. E., Narciso, J., Rostás, M., Wessman, P., et al. (2019). Effect of coating maize seed with entomopathogenic fungi on plant growth and resistance against Fusarium graminearum and Costelytra giveni. Biocontrol Sci. Technol. 29, 877–900. doi: 10.1080/09583157.2019.1611736
Rivas-Franco, F., Hampton, J. G., Narciso, J., Rostás, M., Wessman, P., Saville, D. J., et al. (2020). Effects of a maize root pest and fungal pathogen on entomopathogenic fungal rhizosphere colonization, endophytism and induction of plant hormones. Biol. Control 150:104347. doi: 10.1016/j.biocontrol.2020.104347
Sasan, R. K., and Bidochka, M. J. (2012). The insect-pathogenic fungus Metarhizium robertsii (Clavicipitaceae) is also an endophyte that stimulates plant root development. Am. J. Bot. 99, 101–107. doi: 10.3732/ajb.1100136
Sasan, R. K., and Bidochka, M. J. (2013). Antagonism of the endophytic insect pathogenic fungus Metarhizium robertsii against the bean plant pathogen Fusarium solani f. sp. phaseoli. Can. J. Plant Pathol. 35, 288–293. doi: 10.1080/07060661.2013.823114
Saville, D. J. (2015). Multiple comparison procedures—cutting the gordian knot. Agron. J. 107, 730–735. doi: 10.2134/agronj2012.0394
Song, Z. (2018). Fungal microsclerotia development: essential prerequisites, influencing factors, and molecular mechanism. Appl. Microbiol. Biotechnol. 102, 9873–9880. doi: 10.1007/s00253-018-9400-z
Song, Z., Zhong, Q., Yin, Y., Shen, L., Li, Y., and Wang, Z. (2016). The high osmotic response and cell wall integrity pathways cooperate to regulate morphology, microsclerotia development, and virulence in Metarhizium rileyi. Sci. Rep. 6:38765. doi: 10.1038/srep38765
Tripathi, A., Sharma, N., and Tripathi, N. (2010). “Chapter 10: Biological control of plant diseases: an overview and the Trichoderma system as biocontrol agents,” in Management of fungal plant pathogens, eds A. Arya, A. E. Perelló, 121–137. doi: 10.1079/9781845936037.0000
van der Lee, T., Zhang, H., van Diepeningen, A., and Waalwijk, C. (2015). Biogeography of Fusarium graminearum species complex and chemotypes: a review. Food Addit. Contam. Part A Chem. Anal. Control Expo. Risk Assess. 32, 453–460. doi: 10.1080/19440049.2014.984244
Vega, F. E., Goettel, M. S., Blackwell, M., Chandler, D., Jackson, M. A., Keller, S., et al. (2009). Fungal entomopathogens: new insights on their ecology. Fungal Ecol. 2, 149–159. doi: 10.1016/j.funeco.2009.05.001
Villamizar, L. F., Nelson, T. L., Jones, S. A., Jackson, T. A., Hurst, M. R. H., and Marshall, S. D. G. (2018). Formation of microsclerotia in three species of Beauveria and storage stability of a prototype granular formulation. Biocontrol Sci. Technol. 28, 1097–1113. doi: 10.1080/09583157.2018.1514584
Wang, H., Lei, Z., Reitz, S., Li, Y., and Xu, X. (2013). Production of microsclerotia of the fungal entomopathogen Lecanicillium lecanii (Hypocreales: Cordycipitaceae) as a biological control agent against soil-dwelling stages of Frankliniella occidentalis (Thysanoptera: Thripidae). Biocontrol Sci. Technol. 23, 234–238. doi: 10.1080/09583157.2012.745482
Wang, H.-H., Wang, J.-L., Li, Y.-P., Liu, X., Wen, J.-Z., and Lei, Z.-R. (2011). Liquid culturing of microsclerotia of Beauveria bassiana, an entomopathgeonic fungus to control western flower thrip, Frankliniella occidentalis. Chin. J. Appl. Entomol. 48, 588–595.
Zhou, S., Bae, J. S., Bergstrom, G. C., and Jander, G. (2018). Fusarium graminearum-induced shoot elongation and root reduction in maize seedlings correlate with later seedling blight severity. Plant Direct 2:e00075. doi: 10.1002/pld3.75
Keywords: plant growth promotion, microsclerotia, plant protection, Metarhizium spp, Fusarium graminearum, seed coating, endophytic entomopathogenic fungi, biocontrol
Citation: Rivas-Franco F, Hampton JG, Altier NA, Swaminathan J, Rostás M, Wessman P, Saville DJ, Jackson TA, Jackson MA and Glare TR (2020) Production of Microsclerotia From Entomopathogenic Fungi and Use in Maize Seed Coating as Delivery for Biocontrol Against Fusarium graminearum. Front. Sustain. Food Syst. 4:606828. doi: 10.3389/fsufs.2020.606828
Received: 15 September 2020; Accepted: 30 October 2020;
Published: 10 December 2020.
Edited by:
Everlon Cid Rigobelo, São Paulo State University, BrazilReviewed by:
Inmaculada Garrido Jurado, University of Cordoba, SpainJürg Enkerli, Agroscope, Switzerland
Robert Behle, National Center for Agricultural Utilization Research (NCAUR), United States
Copyright © 2020 Rivas-Franco, Hampton, Altier, Swaminathan, Rostás, Wessman, Saville, Jackson, Jackson and Glare. This is an open-access article distributed under the terms of the Creative Commons Attribution License (CC BY). The use, distribution or reproduction in other forums is permitted, provided the original author(s) and the copyright owner(s) are credited and that the original publication in this journal is cited, in accordance with accepted academic practice. No use, distribution or reproduction is permitted which does not comply with these terms.
*Correspondence: Federico Rivas-Franco, ZnJpdmFzQGluaWEub3JnLnV5