- 1Department of Plant Sciences, University of California, Davis, Davis, CA, United States
- 2World Agroforestry (ICRAF), ICRAF Country Programme, Dar es Salaam, Tanzania
- 3Leibniz Centre for Agricultural Landscape Research (ZALF), Research Area “Land Use and Governance”, Müncheberg, Germany
- 4Land Health Decisions, World Agroforestry (ICRAF), Kinshasa, Democratic Republic of Congo
There is an urgent need to develop resilient agroecosystems capable of helping smallholder farmers adapt to climate change, particularly drought. In East Africa, diversification of maize-based cropping systems by intercropping with grain and tree legumes may foster productivity and resilience to adverse weather conditions. We tested whether intercropping enhances drought resistance and crop and whole-system yields by imposing drought in monocultures and additive intercrops along a crop diversity gradient—sole maize (Zea mays), sole pigeonpea (Cajanus cajan), maize-pigeonpea, maize-gliricidia (Gliricidia sepium, a woody perennial), and maize-pigeonpea-gliricidia—with and without fertilizer application. We developed and tested a novel low-cost, above-canopy rainout shelter design for drought experiments made with locally-sourced materials that successfully reduced soil moisture without creating sizeable artifacts for the crop microenvironment. Drought reduced maize grain yield under fertilized conditions in some cropping systems but did not impact pigeonpea grain yield. Whole-system grain yield and theoretical caloric and protein yields in two intercropping systems, maize-pigeonpea and maize-gliricidia, were similar to the standard sole maize system. Maize-pigeonepea performed most strongly compared to other systems in terms of protein yield. Maize-pigeonpea was the only intercrop that consistently required less land than its corresponding monocultures to produce the same yield (Land Equivalent Ratio >1), particularly under drought. Despite intercropping systems having greater planting density than sole maize and theoretically greater competition for water, they were not more prone to yield loss with drought. Our results show that maize-pigeonpea intercropping provides opportunities to produce the same food on less land under drought and non-drought conditions, without compromising drought resistance of low-input smallholder maize systems.
Introduction
Climate change and weather variability already affect farming conditions across sub-Saharan Africa, and the vulnerability of farmers, agricultural production, and food security will only increase in the future. A significant portion of smallholder farmer households in Tanzania (40%) have been negatively impacted by drought in the past 5 years (Reincke et al., 2018). In the future, seasonal temperature increases of 2°C for 2050 are predicted to cause yield losses of 13% for maize (Zea mays), with additional 4–7% reductions in yields due to higher intra-seasonal rainfall variability in this region (Rowhani et al., 2011). With maize contributing 21–57% of total daily calorie supply in East Africa (Krivanek et al., 2007), there is an urgent need for adoption of drought-resilient agricultural management practices in the maize-based cropping systems predominant across East Africa.
Diversification of maize cropping systems, both in time and space, provides opportunities to decrease vulnerability and improve drought resilience through ecological intensification and production of more diverse food products (Lin, 2011; Altieri et al., 2015; Bullock et al., 2017; Degani et al., 2019; Steward et al., 2019). A resilient agroecosystem shows greater interannual yield stability due to higher resistance to stress or faster recovery after stress (Urruty et al., 2016; Peterson et al., 2018). Intercropping can enhance land use efficiency (food production per unit area) (Rusinamhodzi et al., 2012, Yu et al., 2015) and foster resilience through a portfolio effect whereby different plant species in mixtures have differential drought responses (Doak et al., 1998; Tilman et al., 1998; Tilman, 1999) and altering multiple plant and soil interactions regulating crop performance under drought. In smallholder cropping systems, intercropping C4 cereals with grain and tree legumes has been shown to positively impact soil carbon, fertility, infiltration, and moisture (Jackson et al., 2000; Makumba et al., 2006; Chirwa et al., 2007; Rusinamhodzi et al., 2012; Muchane et al., 2020). Intercropping can also alter plant traits involved in water acquisition and status such as root distribution (Makumba et al., 2009), the depth of plant water sourcing (Sekiya and Yano, 2004), and leaf water potential (Harris and Natarajan, 1987). Crop diversity has been shown to increase the stability of food production at the national and district scales, including to rainfall deficits, but testing of such a portfolio effect at the field scale has been limited (Birthal and Hazrana, 2019; Renard and Tilman, 2019). Together, these mechanisms and evidence suggest that field-scale diversification represents an underexplored opportunity for building resilience in low-input smallholder systems.
Whether these shifts in soil and plant processes and plant mixture composition translate into greater yield stability under drought has seldom been empirically demonstrated in low-input smallholder cropping systems. One study showed that sorghum-groundnut intercropping with a replacement design (i.e., same planting density in monoculture and intercropping) becomes progressively superior to monocultures under increasing drought (Natarajan and Willey, 1986). However, the drought response of additive intercropping (i.e., greater planting in intercropping than monoculture) remains unclear. One study in Malawi found that maize yield loss due to drought in no-till additive maize-cowpea intercropping systems was similar to or greater than in sole maize (Steward et al., 2019). Fully additive designs (planting density in intercropping is the sum of densities of monocultures) are nearly always superior to monocultures in terms of their productivity whereas replacement designs are superior to monocultures only about half the time (Yu et al., 2015), making an evaluation of the drought response of additive designs necessary given their importance for increasing smallholder food production. Although additive intercropping of maize with leguminous trees can enhance maize yield under less favorable conditions (Sileshi et al., 2011), higher competition for water with higher planting densities such as additive intercropping could also increase risk of yield loss (Lobell et al., 2014). Smallholder systems are often co-limited by resources, such as low soil fertility in addition to drought, which can further alter the relative advantage of intercropping (Sileshi et al., 2011; Rusinamhodzi et al., 2012). Most evidence to date on intercropping and drought is solely focused on yield performance, with an emphasis on maize. How current evidence scales up from maize yield to whole-system caloric or protein production from all crops (Snapp et al., 2010; Smith et al., 2016) needs to be assessed to evaluate the potential of intercropping to boost drought resilience of smallholder subsistence farming systems.
We investigated how intercropping impacts whole-system vulnerability to drought, in terms of crop and whole-system grain and nutritional yields and drought-induced yield losses, by excluding rainfall using rainout shelters at a field trial in semi-arid Tanzania. Rain exclusion systems have proven useful in assessing how agroecosystem resilience is affected by management practices in different agroecosystems (Degani et al., 2019; Steward et al., 2019). Such rainout shelters are particularly useful for testing practices with impacts that may emerge over years to even decades, and, as such, remain difficult to test at several sites along a rainfall gradient. We focused on smallholder maize systems in East Africa, where there are relatively high adoption rates of intercropping and tree planting on-farm (27–88 and 14–23% of households surveyed, respectively) in the last 10 years (Kristjanson et al., 2012). Maize intercropping with grain legumes such as pigeonpea (Cajanus cajan) or shrub/tree legumes such as gliricidia (Gliricidia sepium) have been among the most widely studied and adopted diversified systems by East African smallholder farmers (Garrity et al., 2010; Snapp et al., 2010). Pigeonpea is a drought-tolerant grain crop with deep early-season taproot development and slow initial shoot growth (Snapp et al., 2003). Gliricidia trees have been shown in a long-term trial to increase soil organic matter, soil fertility, and soil moisture at the end of the rainy season (Makumba et al., 2006). We measured the impact of additive intercropping of maize with pigeonpea and gliricidia on crop and whole-system grain, calorie, and protein yields and drought resistance with and without fertilizer. We hypothesized that intercropping would outperform sole cropping in food production across rainfall levels but most strongly under drought, with greater land use efficiency and drought resistance, especially when fertilized.
Materials and Methods
Study Site
The experiment was conducted at an ongoing field trial established in 2015 in Manyusi village, Kongwa District, Dodoma, Tanzania (5° 33′ 56.16123" S, 36° 17′ 29.85319" E, elevation 1206.6 m, Supplementary Figure 1). Prior to establishment of the research trial, the site was under continuous maize cultivation by the landowner, a smallholder farmer. Like in other smallholder systems in the semiarid tropics, there was not fertilizer addition at this site prior to establishment of the trial. Tillage consisted of plowing with oxen for field preparation and weeding by hand hoe. The soil was an acidic (pH 5.16 ± 0.20) loamy sand with low organic carbon (0.54 ± 0.16%) (Supplementary Table 1) and minimal slope (1–2%) and classified as a chromic luvisol (Trans-SEC, 2017). This land management history and level of land degradation provide an opportunity to test the resilience potential of maize-legume intercropping. The trial was located in a semi-arid climate with a 30-year annual rainfall average of 635 mm (cropping season average of 425 mm) with a unimodal pattern with most rainfall between December and April and a dry period of 6–7 months (Shemsanga et al., 2016) (Figure 1A). Long-term historic (1988–2018) rainfall estimates for Manyusi village were downloaded from the Early Warning eXplorer (EWX) Lite time series database and web-based mapping tool (United States Geological Survey, 2020), and are similar to but slightly higher than average annual rainfall for the Dodoma region (589 mm) (Msongaleli et al., 2017).
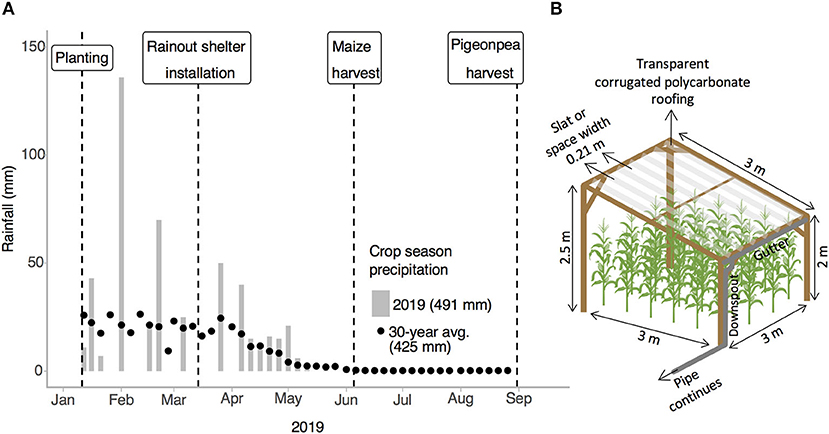
Figure 1. (A) Drought experiment timeline and cropping season rainfall for the experiment and 30-year average (1988–2018). Total cropping season rainfall is indicated in parentheses in the figure legend. (B) Diagram of rainout shelter designed to intercept 50% of incoming rainfall. The pipe drainage system carries water to the field border. Shelters were bordered by a short (40 cm) bund of field soil to prevent surface runoff into the drought plots during rainfall events. Post burial depth (not pictured) was 0.5 m. Plant density not to scale (2 plants per hill). Photographs of shelters are provided in Supplementary Figure 3.
Experimental Design
The field trial was established in a randomized complete block design (RCBD) with three replications in 2015. Because of the presence of gliricidia, a woody perennial, in some plots, tillage by plowing with oxen for field preparation and weeding by hand hoe—standard tillage practices in the study area—were used throughout the experimental period, except for the initial site preparation which was done by tractor. Five cropping systems, sole maize (M), sole pigeonpea (P), maize-pigeonpea (MP), maize-gliricidia (MG), and maize-gliricidia-pigeonpea (MGP), were randomly assigned to plots (16 × 16 m). Sole maize represents the standard farmer practice for growing maize in the study area. Intercropping maize with other annual crops like sunflower, groundnuts, and bambara groundnut is a traditional practice in the study area similar to other smallholder subsistence farming systems in East Africa. Gliricidia woodlots and gliricidia and pigeonpea integration into maize systems are a subject of ongoing research in the study area. Pigeonpea is a more common crop elsewhere in Tanzania. Gliricidia seedlings were transplanted at the establishment of the trial in 2015 at a spacing of 4 × 4 m (25 trees per plot or 625 trees per hectare). Beginning in 2016, gliricidia was pruned heavily to 50 cm height twice a year during the cropping season, once before seeding of maize and pigeonpea in January and once during maize vegetative growth. Green foliage was distributed evenly across its plot of origin as green manure and incorporated into the soil via cultivation by oxen at the first pruning and by hand hoeing at the second pruning. Each year, about 1 month after the onset of the rainy season and immediately following cultivation with oxen to prepare the land and incorporate gliricidia foliage, maize and pigeonpea were sown by hand at a spacing of 75 cm between rows and 60 cm within rows (Supplementary Figure 2). Three maize seeds or three pigeonpea seeds were sown per planting hill and thinned to two plants per hill during maize vegetative growth for a planting density of 44,444 plants per hectare in sole maize and sole pigeonpea. Intercropping was additive: pigeonpea rows with a row spacing of 75 cm were sown between maize rows also with a row spacing of 75 cm (Supplementary Figure 2). The planting density of each crop individually was the same in intercrop and sole crop plots. The total planting density in intercrop plots was thus double that of sole crop plots for an intercrop planting density of 88,889 plants per hectare. In 2019, when the study we report here took place, the maize cultivar was Staha, the pigeonpea cultivar was ICEAP 0040, and the average soil moisture at planting was 7.92 g water g−1 soil.
In 2017, the experimental design was modified to a split-plot. Each cropping system main plot was divided into sub-plots (8 × 16 m) and randomly assigned one of two levels of fertilization: unfertilized or fertilized. Not applying fertilizer represents the standard farmer practice in the study area. Fertilization consisted of 72 and 100% of recommended rates for maize monocrop of nitrogen (N) and phosphorus (P), respectively. Soil potassium levels at the study site are non-limiting to crop production (Supplementary Table 1) (Landon, 2014). Starter fertilizer was broadcast at seeding and 13.4 kg N ha−1 and 15.0 kg P ha−1 were applied as diammonium phosphate (18-46-0). Side dress fertilizer was banded at the soil surface during maize vegetative growth and 30 kg N ha−1 were applied as urea (46-0-0), for a season total of 43 kg N ha−1. Plots with gliricidia also received organic nutrients from gliricidia green foliage prunings incorporated into the soil at maize/pigeonpea seeding and during maize vegetative growth. The estimated gliricidia foliage production for the 2019 cropping season, when the study we report here took place, was 2.27 tons ha−1 in maize-gliricidia and 1.60 tons ha−1 in maize-pigeonpea-gliricidia (dry weight). Based on assumed N concentration of gliricidia foliage (Kimaro et al., 2008), crops received an additional 69.3 kg N ha−1 in maize-gliricidia and 48.8 kg N ha−1 in maize-pigeonpea-gliricidia. However, actual availability of N from gliricidia foliage incorporation and other pathways depends on multiple factors including soil moisture and decomposition rates that are beyond the scope of this experiment.
In 2019, the experimental design was modified to add water treatments. Each cropping system-fertilization combination was split and randomly assigned one of two levels of rainfall water inputs: ambient rainfall and drought (50% ambient rainfall), creating a split-split plot (3 × 3 m total area including borders). To simulate drought in the field, we designed a novel above-canopy partial rain exclusion system (Figure 1B, Supplementary Figure 3) adapted from previous designs. We combined the tall (≥2 m) stature of rainout shelters used to simulate drought in maize (Steward et al., 2019) with a slatted roof that intercepts rainfall and minimizes side effects on the crop microenvironment, as shown in longer term (>1 month) drought simulations in grassland and desert ecosystems (Yahdjian and Sala, 2002; Gherardi and Sala, 2013). Rainout shelters were 3 m wide × 2.96 m long × 2 and 2.5 m tall at their shortest and tallest heights, respectively. Roof slats were 3 m long × 0.21 m wide and cut from transparent corrugated polycarbonate roofing material. Slats were spaced every 0.42 m, such that the roof was designed to intercept 50% of incoming rainfall. Rainfall intercepted by rainout shelters was collected in gutters along the lower edge of the shelter roof and diverted by gravity flow to the edge of the field via a connected system of PVC pipes. Shelters were oriented with the tallest side to the northeast (i.e., the roof sloping down to the southwest) to maximize direct solar radiation from the north to crops beneath the rainout shelter and minimize indirect radiation passing through the roof to crops (Yahdjian and Sala, 2002). Rainout shelters were bordered by a short (40 cm) bund of field soil to prevent surface runoff into the drought plots during rainfall events. The shelters were installed at the onset of maize tasseling and maintained through the harvests of maize and pigeonpea (Figure 1A), in order to simulate drought during maize anthesis and grain filling, the growth stages most vulnerable to drought (Grant et al., 1989; Monneveux et al., 2006).
Weeds were removed with hand hoes, the standard farmer practice for weed management in the study area, twice, during maize vegetative and early reproductive growth. To manage fall armyworm in maize, an insecticide, Acetamiprid + Emamectin benzoate, was applied twice to all plots using a backpack sprayer, at late maize vegetative stage and at tasseling according to manufacturer rates.
Crop Environment Monitoring
Rainfall during the cropping season was measured using a rain gauge located in a border area between plots at 1 m height and recorded manually daily.
All data were collected in the center 2 × 2 m area of each plot. Temperature and relative humidity were measured and recorded at the height of the top of the crop canopy (slightly <2 m, the shortest height of the rainout shelter roof) with a Tramex DL-RHTA FeedBack Datalogger (Tramex Ltd, Orlando, FL, USA) on one date during maize grain filling at midday. The saturated partial pressure of water in the air was calculated from air temperature using the Buck equation (Buck, 1981) and used with relative humidity measurements to calculate actual partial pressure and vapor pressure deficit.
Photosynthetically Active Radiation (PAR) received by the top of the crop canopy was measured in all plots with and without shelters using an Accupar LP-80 ceptometer (Meter Group, Pullman, WA, USA/München, Germany) at one date during maize grain filling at midday with mixed cloudy light conditions typical of other data collection dates. The fraction of PAR transmitted (fTPAR) by the rainout shelter roofing was calculated as the ratio of PAR under a rainout shelter to PAR in the paired ambient rainfall plot.
Soil moisture during the drought imposition period was measured by sampling soil (0–20 cm) at four random points within each sub-sub-plot and compositing subsamples. A subsample taken from the mixed, composited subsamples was analyzed at Sokoine University of Agriculture Department of Ecosystems and Conservation laboratory (Morogoro, Tanzania) for gravimetric water content.
Crop and Whole-System Yields
At maize physiological maturity, maize grain was harvested from the inner 1.5 × 1.8 m (2.7 m2) plot areas. Total fresh grain weight was recorded, and subsamples were analyzed at Tanzania Coffee Research Institute (Moshi, Tanzania) for moisture content and dry matter, which were used to extrapolate fresh weight per plot area to dry yield per ha. Maize dry grain yield is reported at 12% moisture content. At pigeonpea physiological maturity, pigeonpea grain yield was harvested, recorded, subsampled, and analyzed for moisture content and dry matter which were used to calculate dry yield per ha. Pigeonpea grain yield is reported as dry grain yield (0% moisture content). Whole-system grain yield is reported as the sum of dry grain yields (0% moisture content) of maize and pigeonpea.
The land equivalent ratio (LER), a relative measure of the sole cropping area compared to the intercropping area required to achieve the same total crop production, was calculated as:
where I1 and I2 are the yields of species 1 and 2, respectively, in intercropping, and S1 and S2 are the yields of the species in sole cropping. The LER was calculated for maize and pigeonpea, the two cropping system outputs used directly for human consumption in the study area. For maize-pigeonpea and maize-pigeonpea-gliricidia, the LER was calculated relative to both sole maize and sole pigeonpea (i.e., I1/S1, I2/S2). For maize-gliricidia, LER was calculated relative to sole maize (i.e., I1/S1). We focused our LER calculation on cropping system outputs used directly for human consumption because (1) biomass for use as animal forage was not measured consistently for all crops, and (2) the field trial does not include a sole gliricidia treatment and therefore precludes including gliricidia fuelwood impacts on LER.
For all cropping systems, maize and pigeonpea grain yields per hectare were converted to theoretical calories and protein produced per hectare using published constant conversion factors of dry grain weight to calories or protein specific to Tanzania: 362 kcal 100 g−1 maize, 8.1 g protein 100 g−1 maize, 343 kcal 100 g−1 pigeonpea, and 21.7 g protein 100 g−1 pigeonpea (Lukmanji et al., 2008). For a given cropping system, calories or protein from both maize and pigeonpea were then summed to calculate total theoretical calorie or protein yield per hectare for the cropping system.
We focused on resistance to drought (smaller fluctuation from non-stress levels) as one aspect of system resilience (Peterson et al., 2018). Drought resistance was calculated as absolute (drought—ambient rainfall) change due to drought in yields of maize grain, pigeonpea grain, whole-system grain, calories, and protein for each cropping system-fertilization treatment replicate. Greater drought resistance indicates less change due to drought.
Statistical Analysis
All statistical analyses were conducted using R version 3.6.2. Linear mixed-effects models with cropping system, fertilization, and water as fixed effects, and block, main plot, sub-plot, and their interactions with treatments as random effects were used to test the effect of treatments on all response variables (lmer() command in lmerTest package (version 3.1-1) (Kuznetsova et al., 2017) in R), except for fTPAR (see below). In the case of multiple sampling events (gravimetric water content), a date fixed effect, a sub-subplot random effect, and respective interaction terms were added to the model. For each response variable, the full model based on experimental design was fit and then reduced by eliminating random effects that accounted for zero variance to avoid overfitting warnings. The fullest model representative of experimental design that did not result in overfitting was used for analysis of variance (ANOVA). In cases where all random effects accounted for zero variance and mixed-effects models resulted in overfitting, random effects were removed from the model, and linear fixed-effects models were fit [lm() command in stats package (version 3.6.2)]. The assumptions of homogeneity of variance and normality of residuals were assessed visually using diagnostic plots and quantitatively [shapiro.test() command in stats package (version 3.6.2); leveneTest() command in car package (version 3.0-6) Fox and Weisberg, 2019]. Response variables were transformed as necessary to meet assumptions. ANOVA [anova() command in stats package (version 3.6.2)] and means comparisons (CLD() command and contrasts [emmeans() command) in emmeans package (version 1.4.3.01) Lenth, 2019] were conducted using Satterthwaite's method to approximate the degrees of freedom. In cases of multiple comparisons, the Tukey method of p-value adjustment was used to compare families of multiple (three or more) estimates with a significance level of alpha = 0.05. For contrasts testing the effect of the water treatment within each cropping system-fertilization(-date) combination, the Bonferroni method of p-value adjustment was used to simultaneously conduct multiple tests. To test whether the fraction of PAR transmitted (fTPAR) through the rainout shelter roof slats was <1 (i.e., a null hypothesis that shelters transmit 100% of PAR), a one-sided t-test was conducted with a confidence level of 0.95 [t.test() command in stats package (version 3.6.2)]. Observations from one sub-plot where an underground termite nest significantly and visibly affected soil structure and plant growth were excluded from analyses for all crop response variables.
Results
Soil Moisture and Crop Microenvironment
No effects of the rainout shelters were detected at the top of the crop canopy on air temperature (p = 0.460), relative humidity (p = 0.141), or vapor pressure deficit (p = 0.658) (Figures 2B–D). The mean fraction of transmitted PAR through the rainout shelter roof (fTPAR) was 84.2% (15.8% reduction in PAR by rainout shelter) (p < 0.001) (Figure 2E). On a cloudy day typical of light conditions during data collection dates (maize anthesis through harvest), average light transmission through rainout shelters was 850 vs. 1,012 μmol m−2 s−1 without rainout shelters (Supplementary Figure 4).
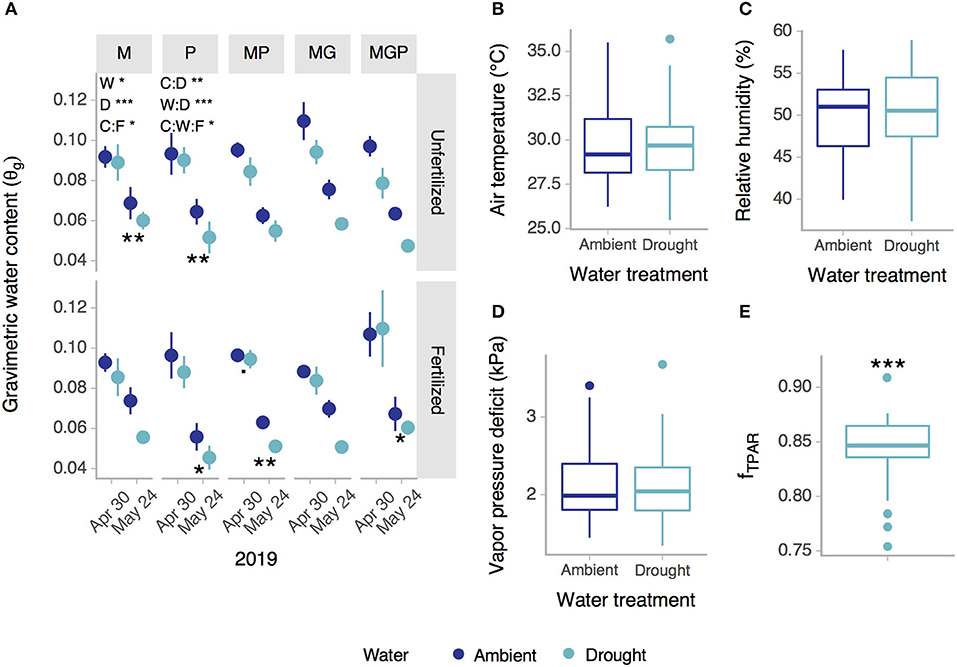
Figure 2. Effects of rainout shelters on (A) gravimetric water content (0–20 cm), (B) air temperature, (C) relative humidity, (D) vapor pressure deficit, and (E) the fraction of transmitted photosynthetically active radiation (fTPAR), (A) within or (B–E) across cropping system-fertilization combinations. Cropping system abbreviations: M, Maize; P, Pigeonpea; MP, Maize-pigeonpea; MG, Maize-gliricidia; MGP, Maize-gliricidia-pigeonpea. Treatment factor abbreviations: W, Water; C, Cropping system; F, Fertilization; D, Date. Asterisks indicate significant differences between ambient and drought (***p < 0.001, **p < 0.01, *p < 0.05). Error bars = standard error; (A) n = 3 or (B–E) n = 30.
The drought treatment using rainout shelters reduced gravimetric soil moisture by 12.5% on average (p = 0.015) with the magnitude of the water effect depending on cropping system, fertilization (cropping system:fertilization p = 0.011, cropping system:fertilization:water p = 0.034), and date (water:date p < 0.001) and varying in whether it was significant (Figure 2A). Reductions in soil moisture by rainout shelters was more often significant at the second sampling date after drought imposition. Gravimetric soil moisture was significantly affected by cropping system and fertilization with variation across dates (cropping system:date p = 0.002) (Figure 2A), but there was no evidence of higher soil moisture due to intercropping (Figure 2A).
Crop Yields and Drought Resistance
Mean maize grain yield by treatment combination ranged from 1.39 to 7.08 t ha−1 (12% moisture content) and was interactively affected by water level (p = 0.049) and fertilization (p < 0.001) across cropping systems (cropping system:water:fertilization p = 0.006) (Figure 3A, Supplementary Table 2). Under fertilized conditions, maize grain yield was significantly reduced by the drought treatment in sole maize and maize-pigeonpea intercropping but not in maize-gliricidia or maize-gliricidia-pigeonpea intercropping. Under unfertilized conditions, maize grain yield was not significantly by drought in any cropping system. Maize drought resistance (yield loss to drought) varied with cropping system and fertilization (fertilization p = 0.015, cropping system:fertilization = 0.003) (Figure 3B). Under fertilized conditions, maize drought resistance was significantly lower in sole maize and maize-pigeonpea intercropping than in maize-gliricidia-pigeonpea intercropping (Figure 3B). Under unfertilized conditions, maize drought resistance did not vary by cropping system.
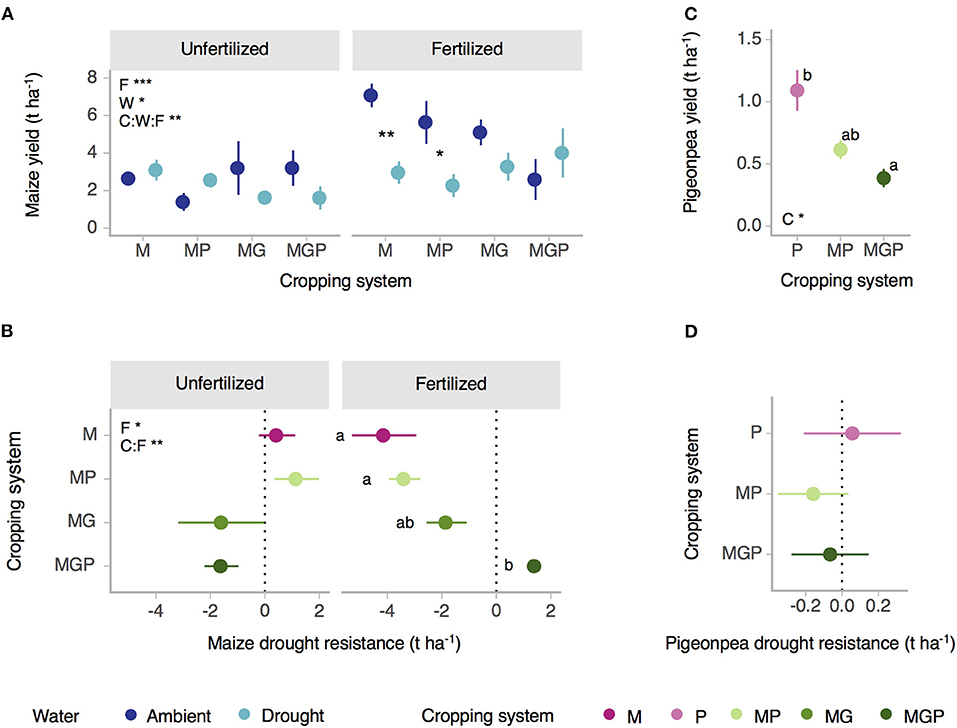
Figure 3. Effects of water, cropping system, and fertilization on (A) maize grain yield at 12% moisture and (C) pigeonpea dry grain yield. Effects of cropping system and fertilization on yield drought resistance of (B) maize and (D) pigeonpea. Dotted lines indicate high drought resistance (zero change due to drought). Cropping system abbreviations: M, Maize; P, Pigeonpea; MP, Maize-pigeonpea; MG, Maize-gliricidia; MGP, Maize-gliricidia-pigeonpea. Treatment factor abbreviations: W, Water; C, Cropping system; F, Fertilization. Asterisks indicate significance of treatment effects or significant differences between ambient and drought (***p < 0.001, **p < 0.01, *p < 0.05). Means sharing the same letter are not significantly different within a panel of a graph (alpha = 0.05). Error bars = standard error; (A,B) n = 3 (fertilized MGP n = 2) or (C,D) n = 12 (MGP = 10).
Mean pigeonpea dry grain yield varied by cropping system and ranged from 0.39 to 1.09 t ha−1 (Figure 3C). It decreased significantly from sole pigeonpea to maize-pigeonpea-gliricidia (cropping system p = 0.028) but was not impacted by drought or whether or not fertilizer was applied (Figure 3C, Supplementary Table 2). Pigeonpea drought resistance was not significantly affected by cropping system and fertilization treatments (cropping system p = 0.713; fertilization p = 0.737; cropping system:fertilization p = 0.196) (Figure 3D).
Whole-System Grain and Nutritional Yields and Drought Resistance
Whole-system dry grain yields ranged from 0.91 to 6.23 t ha−1 and were interactively affected by water (p = 0.003), cropping system (p = 0.001), and fertilization (p = 0.002, water:fertilization p = 0.047, water:cropping system:fertilization p = 0.002) (Figure 4A, Supplementary Table 2). Under fertilized ambient rainfall conditions, whole-system yield was significantly higher in sole maize and maize-pigeonpea intercropping than sole pigeonpea and maize-gliricidia-pigeonpea intercropping. Under fertilized drought conditions, whole-system yield did not vary significantly between cropping systems except for being greater in maize-gliricidia-pigeonpea intercropping than sole pigeonpea. Under unfertilized conditions, there were no differences in whole-system yields between cropping systems under either drought or ambient rainfall conditions. Whole-system grain yield drought resistance varied by cropping system (cropping system:fertilization p = 0.002) and fertilization (p = 0.023) (Figure 4C). Sole pigeonpea was the cropping system most consistently resistant to drought across fertilization levels. Under fertilized conditions, the whole-system drought resistance of sole maize and maize-pigeonpea intercropping was lower than that of sole pigeonpea and maize-gliricidia-pigeonpea intercropping. Under unfertilized conditions, cropping systems did not vary significantly in their whole-system drought resistance.
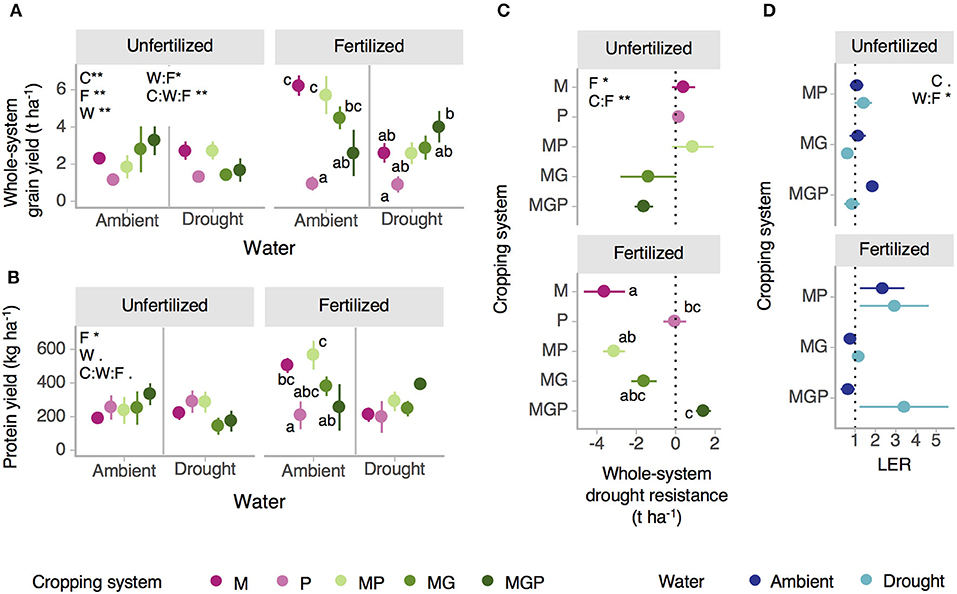
Figure 4. Effects of water, cropping system, and fertilization on (A) whole-system system dry grain yield and (B) whole-system protein yield (see Supplementary Figure 5 for whole-system caloric yield). (C) Effects of cropping system and fertilization on whole-system yield drought resistance; dotted lines indicate high drought resistance (zero change due to drought) (see Supplementary Figure 5 for drought resistance of caloric and protein yield). (D) Land equivalent ratio (LER) for grain crops (maize, pigeonpea) in all intercropping systems under drought and ambient rainfall conditions with and without fertilizer. Dotted line indicates LER = 1, above which intercropping is more efficient than its corresponding monocultures in crop production per unit area. Cropping system abbreviations: M, Maize; P, Pigeonpea; MP, Maize-pigeonpea; MG, Maize-gliricidia; MGP, Maize-gliricidia-pigeonpea. Treatment factor abbreviations: W, Water; C, Cropping system; F, Fertilization. Asterisks indicate significance of treatment effects (**p < 0.01, *p < 0.05. < 0.1). Means sharing the same letter are not significantly different within a panel of a graph (alpha = 0.05). Error bars = standard error; n = 3 (fertilized MGP n = 2).
Theoretical whole-system protein yield ranged from 143 to 566 kg protein ha−1 and was affected by fertilization (p = 0.042) with near significant interactions with water (water p = 0.064) and cropping system (water:cropping system:fertilization p = 0.065) (Figure 4B). Under fertilized ambient rainfall conditions, protein yield was significantly higher for maize-pigeonpea intercropping and sole maize than for sole pigeonpea. Under all drought and all unfertilized conditions, protein yield did not vary between cropping systems. The drought resistance of protein yield was similar to that of whole system grain yield (Supplementary Figure 5). Patterns of theoretical whole-system caloric yield and drought resistance were similar to those of whole-system grain yield (Supplementary Figure 5). Caloric yield ranged from 3,126 to 22,549 thousand kcal ha−1 and was interactively affected by cropping system (p < 0.001), water (p = 0.021), and fertilization (p = 0.007; cropping system:water:fertilization p = 0.010). Variation in theoretical nutritional yield reported here is due to treatment effects on measured crop yield not on grain nutritional content, with nutrient content within a crop assumed to be constant for all treatments (see Methods).
Land Use Efficiency
Maize-pigeonpea was the only intercropping system (cropping system p = 0.078) with a mean Land Equivalent Ratio (LER) >1 across water and fertilization levels, indicating a consistent advantage of over monocultures in that less land was required in maize-pigeonpea intercropping than in maize and pigeonpea monocultures to achieve the same grain production (Figure 4D). LER was interactively impacted by water and fertilization (water:fertilization p = 0.031). For maize-gliricidia and maize-pigeonpea-gliricidia intercropping, whether or not intercropping was superior to monocropping depended on the water level and whether or not fertilizer was applied. In these two intercrops, LER was above one in fertilized drought and unfertilized ambient conditions but was below one in unfertilized drought and fertilized ambient conditions.
Discussion
In this experiment, we empirically tested whether intercropping enhances drought resistance and yield under drought at the single crop and whole-system scales, with and without fertilizer addition. We show that maize grain yield was negatively affected by drought in some cropping systems under fertilized conditions, whereas pigeonpea grain yield was not impacted by drought (Figures 3A,C). Whole-system grain yield and theoretical caloric and protein yields in two intercropping systems, maize-pigeonpea and maize-gliricidia, were as high as in sole maize across all water levels with and without fertilizer (Figures 4A,B, Supplementary Figure 5). Maize-pigeonpea performed most strongly compared to other systems in terms of protein yield (Figure 4B). Maize-pigeonpea was the only intercropping system that consistently required less land than its corresponding monocultures to produce the same yield under a broader range of inputs (mean Land Equivalent Ratio >1) (Figure 4D). All intercrops maintained or increased whole-system drought resistance compared to the standard sole maize across fertilization levels (Figure 4C). We also report a novel rainout shelter design for drought experiments made with locally sourced materials that successfully reduces soil moisture without creating sizable artifacts for the crop microenvironment (Figure 2).
Impact of Drought Differs Between Crops
Drought had no significant detrimental impact on pigeonpea yield but did significantly reduce maize yield under fertilized conditions in two cropping systems, sole maize and maize-pigeonpea (Figures 3A,C). When nutrients were limiting (a common scenario in low-input smallholder cropping systems), drought did not limit yields of maize, the staple crop, in any cropping system (Figure 3A). This superior drought response of pigeonpea was observed despite the drought imposition period spanning two-thirds of the growing season of pigeonpea compared to only half of the growing season of maize (Figure 1) and is consistent with the deep early-season taproot development and slow initial shoot growth of pigeonpea (Snapp et al., 2003).
Maize-Pigeonpea Intercropping Is Consistently Superior to Monocultures Across Rainfall and Fertilization Conditions
Additive maize-pigeonpea intercropping was the only intercropping system that consistently outperformed its corresponding monocultures: it used a smaller land area to produce the same amount of food, particularly under drought, regardless of whether or not fertilizer was added (mean Land Equivalent Ratio >1) (Figure 4D). Maize-pigeonpea was also one of two intercrops with whole-system grain, protein, and caloric yields that were as high as in sole maize, a finding consistent across all water levels with and without fertilizer (Figures 4A,B, Supplementary Figure 5). The high productivity of maize-pigeonpea intercropping is consistent with meta-analysis evidence showing that intercropping C4 cereals with C3 legumes, particularly additive intercropping, increases efficiency of land use for crop production compared to sole cropping (Yu et al., 2015). That maize-pigeonpea LER does not significantly increase under drought compared to ambient rainfall is consistent with meta-analysis results that that LER did not vary with irrigation level or aridity (Martin-Guay et al., 2018), but contrasts with one study that found that the LER of C4 cereal/C3 legume replacement intercropping systems increased with drought (Natarajan and Willey, 1986). Superior maize-pigeonpea performance across growing conditions could be due to limited niche overlap in rooting over time and space, more efficient use of existing of water, nutrient, and light, competition for soil water and nutrients below thresholds for negative impacts on whole-system yield, and/or facilitation of maize by legumes through decomposition of legume residue and maize uptake of mineralized nitrogen.
Under drought, the superior performance of maize-pigeonpea intercropping compared to monocultures could also be due to pigeonpea being the less drought-sensitive crop in the mixture (Figure 3) and/or long term shifts in soil properties due to intercropping. Trends toward greater drought resistance and LER under drought for maize-pigeonpea compared to sole maize (Figure 4) could indicate a portfolio effect whereby pigeonpea responds less negatively than maize to drought, similar to the stabilizing effect of crop diversification that has been observed at larger spatial scales (Doak et al., 1998; Tilman et al., 1998; Tilman, 1999; Birthal and Hazrana, 2019; Renard and Tilman, 2019). The advantage of maize-pigeonpea intercropping over monocultures under drought could also be driven by impacts of maize-legume intercropping on soil hydrology and fertility as shown in longer term studies. Smallholder intercropping systems similar to those tested here have been shown to impact soil and plant mechanisms by mediating facilitative interactions and plant nutrient and water acquisition. Intercropping maize with grain and tree legumes increases soil carbon and water infiltration with measurable gains in soil moisture during periods of peak rainfall especially near trees and particularly in sandy soils (Jackson et al., 2000; Makumba et al., 2006; Chirwa et al., 2007; Rusinamhodzi et al., 2012; Muchane et al., 2020). We did not find a significant benefit of intercropping for soil moisture per se (Figure 2A) and measuring water fluxes through soil and plants and plant water status under drought could provide more robust insight into drivers of greater food production in diversified systems in water-limited scenarios (Nyadzi et al., 2003; Kimaro et al., 2016). Improved leaf water potential in intercrops and hydraulic lift by pigeonpea to maize have been reported (Harris and Natarajan, 1987; Sekiya and Yano, 2004), but their importance for increasing crop yield rather than merely facilitating plant survival under drought is unclear and likely minimal.
Productivity Outcomes of Intercrops With Gliricidia Are Inconsistent
In contrast to maize-pigeonpea, maize-gliricidia and maize-pigeonpea-gliricidia intercropping were inferior or superior to monocultures depending on input combination (i.e., water level and whether or not fertilizer was added) (Figure 4D). Our results highlight the importance of empirically considering multiple potentially interacting resource limitations in the field when testing the resilience of diversified cropping systems. Linear trends in LER observed along broad global fertility and aridity resource gradients (Yu et al., 2015; Martin-Guay et al., 2018) may not reflect the response of low fertility, high aridity marginal smallholder systems to water, and nutrient limitations. The absence of reliable advantages in land-use efficiency of intercropping systems with gliricidia over monocultures could be due to competitive for resources between annual crops and gliricidia (Jackson et al., 2000; Chirwa et al., 2007; Makumba et al., 2009; Muthuri et al., 2009) that were not measured here, such as for light under more productive conditions (i.e., with fertilizer, without drought). Assessing the competitive ability of each crop in mixture through indices such as the competitive ratio and aggressivity (McGilchrist, 1965; Willey and Rao, 1980) would have allowed a fuller evaluation of intercropping but was precluded by the lack of a sole gliricidia control or measurement of gliricidia productivity, a limitation of this study.
Our study focuses only on grain yields of maize and pigeonpea because these are the main benefits determining the adoption of intercropping by smallholder farmers, and excludes other products of gliricidia. The gliricidia fuelwood yield (Kimaro et al., 2007) is a bonus product in intercropping, and its inclusion in LER calculations would make gliricidia intercropping systems more likely to be advantageous compared to monocultures. On-farm wood production also provides benefits that cannot directly be evaluated based on its contribution to food security. Studies showed that, depending on the location, people in rural areas in Tanzania—often women and children—spend a substantial amount of time collecting firewood. Firewood collection trips take up to several hours in Tanzania (Kegode et al., 2017), promoting gender-based violence against those most responsible for firewood collection (Levison et al., 2018).
No Downside of Additive Intercropping for Drought Resistance
This study offers evidence that additively intercropping maize with pigeonpea and/or gliricidia does not compromise drought resistance (i.e., avoids increasing the risk of yield loss) (Figure 4C), despite its higher plant density. At the individual crop scale, intercropping maintained, or increased maize drought resistance and did not impact pigeonpea drought resistance (Figures 3B,D). Similarly, at the whole-system level, all intercropping systems maintained or increased the drought resistance of whole-system grain, protein, and caloric yields compared to the standard sole maize system (Figure 4C, Supplementary Figure 5). This finding weakly supports the conclusion that intercropping increases crop yield stability, particularly in the tropics (Raseduzzaman and Jensen, 2017), in that we did not observe greater yield losses in intercropping than in sole cropping. The lack of a strong interaction between water input level and intercropping vs. monocropping that we found is similar to a study showing that maize yield response to maize-cowpea intercropping is not consistently affected by drought (Steward et al., 2019).
The lack of downside risk of additive intercropping for drought resistance suggests that water use patterns between species were sufficiently complementary or that increased competition for soil moisture was not enough to make intercrops more susceptible to drought, at least for the planting densities typical of the study area and within the ranges of rainfall and soil moisture in our study. Additive intercropping outcomes for whole-system yield under drought may be negative in intensive cropping systems with higher planting density.
Novel Tall Slatted Rainout Shelter With Few Crop Microenvironment Artifacts
We report a novel rainout shelter design for drought experiments that combines the slatted design used in lower stature systems such as grassland, deserts (Yahdjian and Sala, 2002; Gherardi and Sala, 2013), and wheat (Kundel et al., 2018) with the taller (≥2 m) height of fully covered rainout shelters used previously in maize (Steward et al., 2019) and wheat (Degani et al., 2019). Our rainout shelter design successfully reduced soil moisture without creating crop microenvironment artifacts such as higher air temperature, relative humidity, or vapor pressure deficit (Figures 2B–D). Rainout shelters reduced photosynthetically active radiation (PAR) at midday compared to without shelters by an average of 16% (Figure 2E), lower than the maximum midday difference previously reported with rainout shelters in the field (25%) (Yahdjian and Sala, 2002). On a cloudy day typical of light conditions during data collection dates (maize anthesis through harvest), average light transmission through rainout shelters was 850 vs. 1,012 μmol m−2 s−1 without rainout shelters (Supplementary Figure 4). This difference corresponds to a 13% reduction in maize photosynthesis based on maize photosynthetic light response curves (Leakey et al., 2006). This gap in photosynthesis due to light interception by rainout shelters would theoretically become progressively smaller under sunny conditions as photosynthetic light response curves reach a light-saturated rate, although the canopy light response might be less saturating for maize as a C4 plant than for pigeonpea and gliricidia. We observed no negative effect of rainout shelters on maize yields in multiple cropping system-fertilization treatment combinations nor on pigeonpea yields (Figures 3A,C), indicating that light was not yield-limiting. We acknowledge the limitations of above-canopy rainout shelters in terms of the confounding of rainfall interception and light interception and potential impacts on yield [unless controlled for with additional rainout shelters without rainfall interception (Kundel et al., 2018)]. However, we note that rainout shelter impacts on light are often not reported (Degani et al., 2019; Steward et al., 2019) or are reported as roof material manufacturer specifications (Kundel et al., 2018) but not measured in the field. Also, despite our study being conducted over one season, we note the value of rainout shelter experiments for controlled manipulation of rainfall, effectively isolating the impact of drought in terms of rainfall amount while holding rainfall timing and other weather and sources of variation constant. We conducted our study in a season with rainfall slightly above average (Figure 1), and our water treatments thus span reasonable ambient rainfall and drought rainfall levels for this region.
Rainout shelter designs with slatted roofs allow varying amounts of incoming rainfall to be intercepted based on slat spacing and minimize side effects on the crop microenvironment by allowing greater air flow and some direct radiation. These qualities make them apt for longer term (>1 month) drought simulations (Yahdjian and Sala, 2002; Kundel et al., 2018). However, roof slats are often made of relatively expensive transparent acrylic bands (Yahdjian and Sala, 2002; Kundel et al., 2018). Other studies use more economical but less durable greenhouse plastic fully covered rainout shelter roofs with impacts on air temperature and crop microenvironment (Degani et al., 2019; Steward et al., 2019). We highlight our use of transparent corrugated polycarbonate roofing material as more economical than acrylic and sturdier than greenhouse plastic. This roofing material enables use of slatted roof rainout shelter designs in locations where corrugated polycarbonate roofing material is more widely available and affordable.
Significance for Socio-Economics and Drought Adaptation of Maize-Based Farming Systems
Superior performance of maize-pigeonpea intercropping compared to monocultures across rainfall and fertilization levels should be considered in its broader socio-economic context. Sole maize and maize-pigeonpea intercropping achieved similar whole-system grain, caloric, and protein yields, but maize-pigeonpea performed most strongly compared to other systems in terms of protein yield (Figures 4A,B, Supplementary Figure 5). Thus maize monoculture and maize-pigeonpea intercropping may be comparable in their importance for addressing food insecurity based on grain and calorie yields but maize-pigeonpea intercropping produces diverse nutrients (e.g., protein) and may therefore be better suited to address malnutrition. Despite this potential benefits and wider adoption and consumption of pigeonpea in other regions of Tanzania, the adoption of pigeonpea in semi-arid central Tanzania is currently limited by low access to pigeonpea seed via local supply chains (Shiferaw et al., 2008). Costs and benefits of intercropping in terms of labor and economics also influence use of intercropping. The change in crop output per unit labor input in intercropping is highly variable and generally around one, indicating that labor demand increases with intercropping but so does yield to a similar degree (Dahlin and Rusinamhodzi, 2019). On average across Africa, intercropping generally increases gross incomes but with significant variability depending on other management practices (Himmelstein et al., 2017). These differences underscore the importance of matching systems to both risks and individual community needs (Sinclair and Coe, 2019).
Our results have implications for efforts to identify smallholder cropping systems with not only greater productivity but also greater long-term stability (i.e., lower year-to-year fluctuations in yield) (Urruty et al., 2016; Peterson et al., 2018). We found that intercropping maintained or increased whole-system drought resistance (Figure 4C). This suggests that intercropping in most cases may avoid compromising long-term interannual stability and in one case may benefit it. We found greater differences between cropping systems in their drought resistance when fertilizer was applied, which suggests that fertilizers could compromise long-term yield stability in some cropping systems despite boosting productivity. This finding is consistent with adoption of diversification practices of intercropping and rotations by smallholder farmers in East Africa to adapt to change (Kristjanson et al., 2012) and modeling evidence that integrating legumes into maize systems maintains or increases the modeled chance of meeting smallholder household calorie and protein needs without increasing fertilizer inputs (Smith et al., 2016). The potential of intercropping and agroforestry practices that build soil carbon (Muchane et al., 2020) to boost cropping system drought resistance (Iizumi and Wagai, 2019) should be tested in long-term trials beyond the length of our study. Such testing would generate better understanding of how to mitigate greater drought limitation of crop yield as fertilizer promotion and adoption across semi-arid Tanzania reduce nutrient limitation of crop yield.
Conclusions
Our study presents one of the few assessments of additive maize-legume intercropping impacts on yield vulnerability to drought in resource-poor smallholder maize systems. For the environment tested here, we conclude that diversifying maize-systems through maize-pigeonpea intercropping lowers the land area needed to produce the same food, including under drought, avoids compromising—but does not build—drought resistance, and can help supply protein in maize-dominated landscapes. Outcomes of intercropping will likely vary with edaphic conditions, climate, and drought stress timing and intensity. We provide direct evidence that diversification of maize-based cropping systems via intercropping constitutes a tool adapted to low-input smallholder systems to build productivity across drought and non-drought conditions in the face of a changing climate.
Data Availability Statement
Datasets are available on request. The raw data supporting the conclusions of this article will be made available by the authors, without undue reservation. Datasets will be made publicly available following publication at the World Agroforestry Research Data Repository: https://data.worldagroforestry.org/.
Author Contributions
AK conceived, designed, and established the intercropping field trial at which the drought experiment was conducted. LR, AG, AK, and TR conceived and designed the drought experiment. LR and JH established the drought experiment and collected data with support from AK. LR processed the data with contributions from JH and AK, and analyzed the data. LR drafted the manuscript, which was critically revised by AG, AK, JH, and TR. All authors contributed to the article and approved the submitted version.
Funding
The experimental site was established and managed with support from the United States Agency for International Development's Feed the Future initiative through the Africa RISING program. The drought resistance experiment was implemented as part of the United States Department of Agriculture-Foreign Agriculture Service-funded project on building capacity for resilient food security in Tanzania (FX19TA-10960C012) and under the Partnerships for Scaling Climate-Smart Agriculture (P4S) project of the CGIAR Research Program on Climate Change, Agriculture and Food Security (CCAFS), which is carried out with support from the CGIAR Trust Fund and through bilateral funding agreements. For details please visit https://ccafs.cgiar.org/donors. LR's work on this project and materials for the drought experiment were supported by fellowships and small grants to LR from the United States National Science Foundation Graduate Research Fellowship Program (Grant No. 1650042), the University of California Research and Innovation Fellowship for Agriculture (RIFA), the UC Davis Blum Center for Developing Economies Poverty Alleviation through Sustainable Solutions (PASS) project grants program, and a UC Davis College of Agriculture and Environmental Sciences International Agricultural Development Graduate Group Henry A. Jastro Graduate Research Award. Any opinions, findings, and conclusions or recommendations expressed in this material are those of the authors and do not necessarily reflect the views of the National Science Foundation or other funding organizations.
Conflict of Interest
The authors declare that the research was conducted in the absence of any commercial or financial relationships that could be construed as a potential conflict of interest.
Acknowledgments
We thank Jimmy Sianga for his technical assistance and leadership in logistics and translation in the field and Yuda Kirway and other farmers from Manyusi village for their work supporting the establishment of the research trial, the construction and installation of rainout shelters, and data collection.
Supplementary Material
The Supplementary Material for this article can be found online at: https://www.frontiersin.org/articles/10.3389/fsufs.2020.562663/full#supplementary-material
References
Altieri, M. A., Nicholls, C. I., Henao, A., and Lana, M. A. (2015). Agroecology and the design of climate change-resilient farming systems. Agron. Sustain. Dev. 35, 869–890. doi: 10.1007/s13593-015-0285-2
Birthal, P. S., and Hazrana, J. (2019). Crop diversification and resilience of agriculture to climatic shocks: evidence from India. Agric. Syst. 173, 345–354. doi: 10.1016/j.agsy.2019.03.005
Buck, A. L. (1981). New equations for computing vapour pressure and enhancement factor. J. Appl. Meteorol. 20, 1527–1532. doi: 10.1175/1520-0450(1981)020<1527:NEFCVP>2.0.CO;2
Bullock, J. M., Dhanjal-Adams, K. L., Milne, A., Oliver, T. H., Todman, L. C., Whitmore, A. P., et al. (2017). Resilience and food security: rethinking an ecological concept. J. Ecol. 105, 880–884. doi: 10.1111/1365-2745.12791
Chirwa, P. W., Ong, C. K., Maghembe, J., and Black, C. R. (2007). Soil water dynamics in cropping systems containing gliricidia sepium, pigeonpea and maize in southern Malawi. Agrofor. Syst. 69, 29–43. doi: 10.1007/s10457-006-9016-7
Dahlin, A. S., and Rusinamhodzi, L. (2019). Yield and labor relations of sustainable intensification options for smallholder farmers in sub-Saharan Africa. a meta-analysis. Agron. Sustain. Dev. 39:32. doi: 10.1007/s13593-019-0575-1
Degani, E., Leigh, S. G., Barber, H. M., Jones, H. E., Lukac, M., Sutton, P., et al. (2019). Crop rotations in a climate change scenario: short-term effects of crop diversity on resilience and ecosystem service provision under drought. Agric. Ecosyst. Environ. 285:106625. doi: 10.1016/j.agee.2019.106625
Doak, D. F., Bigger, D., Harding, E. K., Marvier, M. A., O'Malley, R. E., and Thomson, D. (1998). The statistical inevitability of stability-diversity relationships in community ecology. Am. Nat. 151, 264–276. doi: 10.1086/286117
Fox, J., and Weisberg, S. (2019). An R Companion to Applied Regression, 3rd Edn, Thousand Oaks, CA: Sage.
Garrity, D. P., Akinnifesi, F. K., Ajayi, O. C., Weldesemayat, S. G., Mowo, J. G., Kalinganire, A., et al. (2010). Evergreen agriculture: a robust approach to sustainable food security in Africa. Food Secur. 2, 197–214. doi: 10.1007/s12571-010-0070-7
Gherardi, L. A., and Sala, O. E. (2013). Automated rainfall manipulation system: a reliable and inexpensive tool for ecologists. Ecosphere 4, 1–10. doi: 10.1890/ES12-00371.1
Grant, R. F., Jackson, B. S., Kiniry, J. R., and Arkin, G. F. (1989). Water deficit timing effects on yield components in maize. Agron. J. 81, 61–65. doi: 10.2134/agronj1989.00021962008100010011x
Harris, D., and Natarajan, M. (1987). Physiological basis for yield advantage in a sorghum/groundnut intercrop exposed to drought. 2. plant temperature, water status, and components of yield. F. Crop. Res. 17, 273–288. doi: 10.1016/0378-4290(87)90040-2
Himmelstein, J., Ares, A., Gallagher, D., and Myers, J. (2017). A meta-analysis of intercropping in Africa: impacts on crop yield, farmer income, and integrated pest management effects. Int. J. Agric. Sustain. 15, 1–10. doi: 10.1080/14735903.2016.1242332
Iizumi, T., and Wagai, R. (2019). Leveraging drought risk reduction for sustainable food, soil and climate via soil organic carbon sequestration. Sci. Rep. 9:19744. doi: 10.1038/s41598-019-55835-y
Jackson, N. A., Wallace, J. S., and Ong, C. K. (2000). Tree pruning as a means of controlling water use in an agroforestry system in Kenya. For. Ecol. Manage. 126, 133–148. doi: 10.1016/S0378-1127(99)00096-1
Kegode, H. J. S., Oduol, J., Wario, A. R., Muriuki, J., Mpanda, M., and Mowo, J. (2017). Households' choices of fuelwood sources: implications for agroforestry interventions in the southern Highlands of Tanzania. Small Scale For. 16, 535–551. doi: 10.1007/s11842-017-9369-y
Kimaro, A. A., Mpanda, M., Rioux, J., Aynekulu, E., Shaba, S., Thiong'o, M., et al. (2016). Is conservation agriculture climate-smart for maize farmers in the highlands of Tanzania? Nutr. Cycl. Agroecosyst. 105, 217–228. doi: 10.1007/s10705-015-9711-8
Kimaro, A. A., Timmer, V. R., Chamshama, S. A. O., Mugasha, A. G., and Kimaro, D. A. (2008). Differential response to tree fallows in rotational woodlot systems in semi-arid tanzania: post-fallow maize yield, nutrient uptake, and soil nutrients. Agric. Ecosyst. Environ. 125, 73–83. doi: 10.1016/j.agee.2007.11.007
Kimaro, A. A., Timmer, V. R., Mugasha, A. G., Chamshama, S. A. O., and Kimaro, D. A. (2007). Nutrient use efficiency and biomass production of tree species for rotational woodlot systems in semi-arid morogoro, Tanzania. Agrofor. Syst. 71, 175–184. doi: 10.1007/s10457-007-9061-x
Kristjanson, P., Neufeldt, H., Gassner, A., Mango, J., Kyazze, F. B., Desta, S., et al. (2012). Are food insecure smallholder households making changes in their farming practices? Evidence from East Africa. Food Secur. 4, 381–397. doi: 10.1007/s12571-012-0194-z
Krivanek, A. F., De Groote, H., Gunaratna, N. S., Diallo, A. O., and Friesen, D. (2007). Breeding and disseminating quality protein maize (QPM) for Africa. African J. Biotechnol. 6, 312–324. doi: 10.5897/AJB2007.000-2007
Kundel, D., Meyer, S., Birkhofer, H., Fliessbach, A., Mäder, P., Scheu, S., et al. (2018). Design and manual to construct rainout-shelters for climate change experiments in agroecosystems. Front. Environ. Sci. 6:14. doi: 10.3389/fenvs.2018.00014
Kuznetsova, A., Brockhoff, P. B., and Christensen, R. H. B. (2017). lmertest package: tests in linear mixed effects models. J. Stat. Softw. 82, 1–26. doi: 10.18637/jss.v082.i13
Landon, J. (2014). Booker Tropical Soil Manual: A Handbook for Soil Survey and Agricultural Land Evaluation in the Tropics and Subtropics. New York, NY: Routledge. doi: 10.4324/9781315846842
Leakey, A. D. B., Uribelarreà, M., Ainsworth, E. A., Naidu, S. L., Rogers, A., Ort, D. R., et al. (2006). Photosynthesis, productivity, and yield of maize are not affected by open-air elevation of CO2 concentration in the absence of drought. Plant Physiol. 140, 779–790. doi: 10.1104/pp.105.073957
Levison, D., Degraff, D. S., and Dungumaro, E. W. (2018). Implications of environmental chores for schooling: Children's time fetching water and firewood in Tanzania. Eur. J. Dev. Res. 30, 217–234. doi: 10.1057/s41287-017-0079-2
Lin, B. B. (2011). Resilience in agriculture through crop diversification: adaptive management for environmental change. Bioscience 61, 183–193. doi: 10.1525/bio.2011.61.3.4
Lobell, D. B., Roberts, M. J., Schlenker, W., Braun, N., Little, B. B., Rejesus, R. M., et al. (2014). Greater sensitivity to drought accompanies maize yield increase in the U.S. Midwest. Science 344, 516–519. doi: 10.1126/science.1251423
Lukmanji, Z., Hertzmark, E., Mlingi, N., Assey, V. G. N., et al. (2008). Tanzania Food Composition Tables, 1st Edn. Dar es Salaam: Muhimbili University of Health and Allied Sciences, Tanzania Food and Nutrition Centre, and Harvard School of Public Health.
Makumba, W., Akinnifesi, F. K., and Janssen, B. H. (2009). Spatial rooting patterns of gliricidia, pigeon pea and maize intercrops and effect on profile soil N and P distribution in southern Malawi. African J. Agric. Res. 4, 278–288. doi: 10.5897/AJAR.9000652
Makumba, W., Janssen, B., Akinnifesi, F. K., Mweta, D., and Kwesiga, F. (2006). The long-term effects of a gliricidia–maize intercropping system in Southern Malawi, on gliricidia and maize yields, and soil properties. Agric. Ecosyst. Environ. 116, 85–92. doi: 10.1016/j.agee.2006.03.012
Martin-Guay, M. O., Paquette, A., Dupras, J., and Rivest, D. (2018). The new green revolution: sustainable intensification of agriculture by intercropping. Sci. Total Environ. 615, 767–772. doi: 10.1016/j.scitotenv.2017.10.024
McGilchrist, C. A. (1965). Analysis of competition experiments. Biometrics 21, 975–985. doi: 10.2307/2528258
Monneveux, P., Sánchez, C., Beck, D., and Edmeades, G. O. (2006). Drought tolerance improvement in tropical maize source populations: evidence of progress. Crop Sci. 46, 180–191. doi: 10.2135/cropsci2005.04-0034
Msongaleli, B. M., Tumbo, S. D., Kihupi, N. I., and Rwehumbiza, F. B. (2017). Performance of Sorghum Varieties under Variable Rainfall in Central Tanzania. Int. Sch. Res. Not. 2017:2506946. doi: 10.1155/2017/2506946
Muchane, M. N., Sileshi, G. W., Gripenberg, S., Jonsson, M., Pumariño, L., and Barrios, E. (2020). Agroforestry boosts soil health in the humid and sub-humid tropics: a meta-analysis. Agric. Ecosyst. Environ. 295:106899. doi: 10.1016/j.agee.2020.106899
Muthuri, C. W., Ong, C. K., Craigon, J., Mati, B. M., Ngumi, V. W., and Black, C. R. (2009). Gas exchange and water use efficiency of trees and maize in agroforestry systems in semi-arid Kenya. Agric. Ecosyst. Environ. 129, 497–507. doi: 10.1016/j.agee.2008.11.001
Natarajan, M., and Willey, R. W. (1986). The effects of water stress on yield advantages of intercropping systems. F. Crop. Res. 13, 117–131. doi: 10.1016/0378-4290(86)90015-8
Nyadzi, G. I., Janssen, B. H., Otsyina, R. M., Booltink, H. W. G., Ong, C. K., and Oenema, O. (2003). Water and nitrogen dynamics in rotational woodlots of five tree species in western Tanzania. Agrofor. Syst. 59, 215–229. doi: 10.1023/B:AGFO.0000005223.27670.7f
Peterson, C. A., Eviner, V. T., and Gaudin, A. C. M. (2018). Ways forward for resilience research in agroecosystems. Agric. Syst. 162, 19–27. doi: 10.1016/j.agsy.2018.01.011
Raseduzzaman, M., and Jensen, E. S. (2017). Does intercropping enhance yield stability in arable crop production? A meta-analysis. Eur. J. Agron. 91, 25–33. doi: 10.1016/j.eja.2017.09.009
Reincke, K., Vilvert, E., Fasse, A., Graef, F., Sieber, S., and Lana, M. A. (2018). Key factors influencing food security of smallholder farmers in Tanzania and the role of cassava as a strategic crop. Food Secur. 10, 911–924. doi: 10.1007/s12571-018-0814-3
Renard, D., and Tilman, D. (2019). National food production stabilized by crop diversity. Nature 571, 257–260. doi: 10.1038/s41586-019-1316-y
Rowhani, P., Lobell, D. B., Linderman, M., and Ramankutty, N. (2011). Climate variability and crop production in Tanzania. Agric. For. Meteorol. 151, 449–460. doi: 10.1016/j.agrformet.2010.12.002
Rusinamhodzi, L., Corbeels, M., Nyamangara, J., and Giller, K. E. (2012). Maize–grain legume intercropping is an attractive option for ecological intensification that reduces climatic risk for smallholder farmers in central Mozambique. F. Crop. Res. 136, 12–22. doi: 10.1016/j.fcr.2012.07.014
Sekiya, N., and Yano, K. (2004). Do pigeon pea and sesbania supply groundwater to intercropped maize through hydraulic lift? - hydrogen stable isotope investigation of xylem waters. F. Crop. Res. 86, 167–173. doi: 10.1016/j.fcr.2003.08.007
Shemsanga, C., Muzuka, A. N. N., Martz, L., Komakech, H., and Omambia, A. N. (2016). “Statistics in climate variability, dry spells, and implications for local livelihoods in semiarid regions of Tanzania: The way forward,” in Handbook of Climate Change Mitigation and Adaptation, 2nd Edn. eds W. Y. Chen, T. Suzuki, M. Lackner (Springer), 801–848. doi: 10.1007/978-3-319-14409-2_66. Available online at: https://link.springer.com/referenceworkentry/10.1007%2F978-3-319-14409-2_66#citeas
Shiferaw, B. A., Kebede, T. A., and You, L. (2008). Technology adoption under seed access constraints and the economic impacts of improved pigeonpea varieties in Tanzania. Agric. Econ. 39, 309–323. doi: 10.1111/j.1574-0862.2008.00335.x
Sileshi, G. W., Akinnifesi, F. K., Ajayi, O. C., and Muys, B. (2011). Integration of legume trees in maize-based cropping systems improves rain use efficiency and yield stability under rain-fed agriculture. Agric. Water Manag. 98, 1364–1372. doi: 10.1016/j.agwat.2011.04.002
Sinclair, F., and Coe, R. I. C. (2019). The options by context approach: a paradigm shift in agronomy. Exp. Agric. 55, 1–13. doi: 10.1017/S0014479719000139
Smith, A., Snapp, S., Dimes, J., Gwenambira, C., and Chikowo, R. (2016). Doubled-up legume rotations improve soil fertility and maintain productivity under variable conditions in maize-based cropping systems in Malawi. Agric. Syst. 145, 139–149. doi: 10.1016/j.agsy.2016.03.008
Snapp, S. S., Blackie, M. J., Gilbert, R. A., Bezner-Kerr, R., and Kanyama-Phiri, G. Y. (2010). Biodiversity can support a greener revolution in Africa. Proc. Natl. Acad. Sci. U.S.A. 107, 20840–20845. doi: 10.1073/pnas.1007199107
Snapp, S. S., Jones, R. B., Minja, E. M., Rusike, J., and Silim, S. N. (2003). Pigeon pea for africa: a versatile vegetable—and more. HortScience 38, 1073–1079. doi: 10.21273/HORTSCI.38.6.1073
Steward, P. R., Thierfelder, C., Dougill, A. J., and Ligowe, I. (2019). Conservation agriculture enhances resistance of maize to climate stress in a Malawian medium-term trial. Agric. Ecosyst. Environ. 277, 95–104. doi: 10.1016/j.agee.2018.07.009
Tilman, D. (1999). The ecological consequences of changes in biodiversity: a search for general principles. Ecology 80, 1455–1474. doi: 10.1890/0012-9658(1999)080[1455:TECOCI]2.0.CO;2
Tilman, D., Lehman, C. L., and Bristow, C. E. (1998). Diversity-stability relationships: statistical inevitability or ecological consequence? Am. Nat. 151, 277–282. doi: 10.1086/286118
Trans-SEC (2017). Tanzania Land Evaluation Tool, Tanzania Food and Land Productivity Information System.
United States Geological Survey (2020). Early Warning eXplorer (EWX) Lite, United States Geological Survey Famine Early Warning System Network (USGS FEWS NET) Data Portal.
Urruty, N., Tailliez-Lefebvre, D., and Huyghe, C. (2016). Stability, robustness, vulnerability and resilience of agricultural systems. a review. Agron. Sustain. Dev. 36, 1–15. doi: 10.1007/s13593-015-0347-5
Willey, R. W., and Rao, M. R. (1980). A competitive ratio for quantifying competition between intercrops. Exp. Agric. 16, 117–125. doi: 10.1017/S0014479700010802
Yahdjian, L., and Sala, O. E. (2002). A rainout shelter design for intercepting different amounts of rainfall. Oecologia 133, 95–101. doi: 10.1007/s00442-002-1024-3
Keywords: resilience, land equivalent ratio, drought, pigeonpea, gliricidia, maize, agroforestry, diversification
Citation: Renwick LLR, Kimaro AA, Hafner JM, Rosenstock TS and Gaudin ACM (2020) Maize-Pigeonpea Intercropping Outperforms Monocultures Under Drought. Front. Sustain. Food Syst. 4:562663. doi: 10.3389/fsufs.2020.562663
Received: 15 May 2020; Accepted: 11 November 2020;
Published: 07 December 2020.
Edited by:
Selena Ahmed, Montana State University, United StatesReviewed by:
Guzel Kudoyarova, Institute of Biology of Karelian Research Centre (RAS), RussiaLeonard Rusinamhodzi, International Institute of Tropical Agriculture (IITA), Nigeria
Copyright © 2020 Renwick, Kimaro, Hafner, Rosenstock and Gaudin. This is an open-access article distributed under the terms of the Creative Commons Attribution License (CC BY). The use, distribution or reproduction in other forums is permitted, provided the original author(s) and the copyright owner(s) are credited and that the original publication in this journal is cited, in accordance with accepted academic practice. No use, distribution or reproduction is permitted which does not comply with these terms.
*Correspondence: Anthony A. Kimaro, QS5LaW1hcm9AY2dpYXIub3Jn