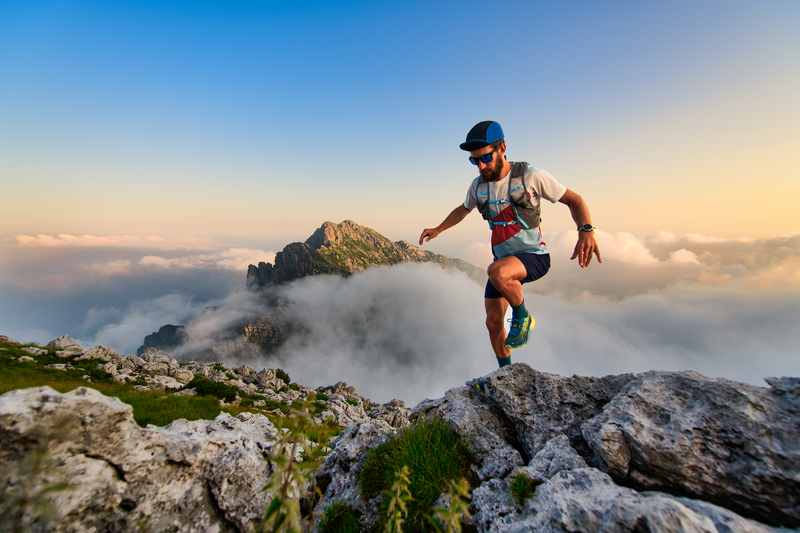
95% of researchers rate our articles as excellent or good
Learn more about the work of our research integrity team to safeguard the quality of each article we publish.
Find out more
ORIGINAL RESEARCH article
Front. Sustain. Food Syst. , 24 November 2020
Sec. Crop Biology and Sustainability
Volume 4 - 2020 | https://doi.org/10.3389/fsufs.2020.544996
This article is part of the Research Topic Intercropping Systems in Sustainable Agriculture View all 17 articles
In the context of sustainable tropical agriculture, an innovative corn (Zea mays L.) to silage-grass-legume intercropping system can promotes plant diversity, improves agronomic performance and land-use efficiency, and increases the yield of oversown black oat (Avena strigosa Schreb) and soybean [Glycine max (L.) Merr.] to silage in succession. Thus, during three growing seasons on a Typic Haplorthox in Botucatu, São Paulo State, Brazil, four treatments of a corn to silage production system were implemented in summer/autumn with black oat oversown in winter/spring: (1) corn intercropped with palisade grass (Urochloa brizantha “Marandu”) and black oat overseeded in lines; (2) corn intercropped with palisade grass and black oat overseeded in a broadcast system with superficial incorporation; (3) corn intercropped with palisade grass + pigeon pea [Cajanus cajan (L.) Millsp.] and black oat overseeded in lines; and (4) corn intercropped with palisade grass + pigeon pea and black oat overseeded in a broadcast system with superficial incorporation. During winter/spring, the black oat pastures were grazed by lambs, but results on forage allowance and nutritive value for animal grazing and on animal performance are not reported in the present manuscript. In the fourth growing season, the effect of soybean to silage intercropped with guinea grass (Panicum maximum “Aruana”), with only a residual effect of the four production systems from the previous three growing seasons, was evaluated. Despite greater interspecific competition of palisade grass and pigeon pea intercropped with corn, this more complex system produced better results. Thus, when analyzing this system as a whole, the triple intercrop (corn + pigeon pea + palisade grass) combined with oversown black oat in lines was the most effective option for silage production and for the improvement of other elements of system productivity, such higher surface mulch quantity, leaf nutrient concentrations, and yield of soybean to silage intercropped with guinea grass. This intercrop also generated better nutrient cycling because an increased quantity of nutrients was retained in standing plant residue and surface mulch, which resulted in better land- and nutrient-use efficiency, with an emphasis on nitrogen and potassium.
- Corn to silage is not affected by intercropping with palisade grass and pigeon pea.
- Pigeon pea increased the surface mulch quantity and soybean to silage in succession.
- The triple intercrop generated better nutrient cycling.
- The land equivalent ratio exceeded 1, showing the advantage of double and triple intercropping.
The adoption of the no-till system (NTS) is growing in different edaphoclimatic conditions around of the world. However, this system is highly dependent of crops for the production and maintenance of straw on the soil surface (Borghi et al., 2013a). Several cover crops have been researched and used in tropical conditions, being that forage grasses, intercropped or not, especially of the genus Urochloa (syn. Brachiaria) are standing out. In addition, the use of integrated crop-livestock system (ICLS) can provide grain production in the summer, pasture in the autumn/winter and straw in the spring for continuity of the NTS in tropical regions, such as in the Brazilian “Cerrado” and African “Savannah” (Mateus et al., 2012). It is also noteworthy that in Brazil, the problem of degraded pasture cause low forage yield and a low animal stocking rate (Pariz et al., 2011a). Thus, the ICLS is an option to recover these degraded areas, increasing animal production. Furthermore, ICLS can contribute to increased global food production in the future (Wirsenius et al., 2010; Franzluebbers and Stuedemann, 2014).
In this context, the intercropping of tropical forage grasses with grain crops could be a key strategy for enhancing the early establishment and successful production of a winter season (with low and irregular rainfall) forage for grazing (Costa et al., 2016; Crusciol et al., 2016; Pariz et al., 2016). Nevertheless, many of these studies did not utilize animal grazing. According Moraes et al. (2014a), approximately only 5% of studies about ICLS directly utilized animal grazing, alternating with cash crops. These authors also highlighted that a diversity of field studies is needed with ruminant livestock, including sheep (Ovis aries), to adequately characterize the impacts of ICLS on animal performance and crop yield because stocking rates and management approaches can alter crop residue and forage quantity and quality.
Furthermore, over the years, grass-only intercropping can compromise the sustainability of ICLS due to soil nitrogen deficiency (Costa et al., 2012; Garcia et al., 2016). Therefore, according Moraes et al. (2019), the “sustainable no-tillage silage production systems are needed, especially those combined with promising ICLS production strategies, to improve overall agricultural functionality.” Thus, corn intercropped with legumes (i.e., pigeon pea) is an alternative to improve the diversification of agricultural activities in tropical regions (Baldé et al., 2011). Pigeon pea is grown by smallholder farmers as a sole crop or intercropped with corn, sorghum [Sorghum bicolor (L.) Moench] or other crops, with cereal being the main crop that is common in Africa and Asia (Senkoro et al., 2017). When a tropical perennial grass is included in corn or sorghum-legume intercropping, there is the advantage of later pasture formation, and this intercropping system with legumes represents an alternative for the grower to implement nitrogen biological fixation in the soil because pigeon pea can fix up to 235 kg ha−1 of atmospheric N and can reduce the N fertilizer needed following cereal (Myaka et al., 2006) or pasture crops (Oliveira et al., 2011).
In general, results of Borghi et al. (2013a), Crusciol et al. (2014), Mateus et al. (2016), and Pariz et al. (2017c) demonstrated that in intercropping systems, the land equivalent ratio exceeded 1, showing the advantage in biomass production compared to sole crop systems. These results can be explained because companion crop stimulating the root growth of the grain crop (Hauggaard-Nielsen and Jensen, 2005). In addition, according Xiao et al. (2004), with the inclusion of legume intercropped with cereal crop (corn) and/or tropical forage grasses, it is also possible that the direct transfer of nitrogen from, e.g., a legume to a corn crop and grass. Results of Baldé et al. (2011) demonstrated that “high land equivalent ratio values provide evidence for the complementary and the high efficiency of use of available resources by the intercropped plants and thus the advantage of such systems to produce both corn grain and cover crop forage (pigeon pea and Urochloa).” The results presented by Ndungu-Magiroi et al. (2017) demonstrated that corn-bean (Phaseolus vulgaris) intercropping is more productive on a corn yield equivalent basis than monocropped corn, and the intercrop value is enhanced as bean to crop value ratios increase. Thus, despite several advances in research on intercropping systems, it is important to study the intensity of how each grain crop affects the growth of tropical perennial grasses and legumes in intercropping systems compared to monocropping systems (Brooker et al., 2015). The competition of crops in intercropping can be better explained by a land equivalent ratio (LER) (Mead and Willey, 1980), relative crowding coefficient (K) (Agegnehu et al., 2006), and aggressivity (A) (Takim, 2012).
Another good option in ICLS is the winter annual grazing grasses such as oat (Avena) (Moraes et al., 2014b). Results of Lopes et al. (2008) and Pariz et al. (2017b), demonstrated that in southern and central-southern Brazil, oversowing corn with oats is a viable alternative to increasing forage and meat production in winter/spring. However, the oversown of oat in the winter/spring with seeds planted in lines or seeds broadcast with superficial incorporation may alter the forage mass of pasture as well as nutrient cycling.
Thus, our objective was evaluated the effect of inclusion of pigeon pea in the intercrop of corn with palisade grass, as well as, the modalities of oversown of black oat on: corn and soybean leaf nutrient concentrations, agronomic characteristics and yields, intercropping competition factors, land-use efficiency, relative nutrient yields, mulching, straw decomposition, and nutrient release rates in an ICLS during four growing seasons in the Brazilian “Cerrado.”
The experiment was conducted in Botucatu in São Paulo, Brazil (48° 25′ 28″ W, 22° 51′ 01″ S; 777 m above sea level) over four consecutive growing seasons: 2013–2014, 2014–2015, 2015–2016, and 2016–2017. The soil was a clayey, kaolinitic, thermic Typic Haplorthox (FAO–Food Agriculture Organization of the United Nations, 2006) with 630, 90, and 280 g kg−1 of clay, silt, and sand, respectively. For 4 years, until October 2010, the field was fallow, with predominantly signal grass (Urochloa decumbens “Basilisk”) and annual broadleaf weeds (Bidens spp., Sonchus oleraceus, Raphanus raphanistrum, Commelina benghalensis, Ipomoea grandifolia, Chamaesyce hirta, Euphorbia heterophylla, Desmodium tortuosum, Leonotis nepetifolia, and Sida rhombifolia). In the growing seasons of 2010–2011 and 2011–2012, corn to silage was intercropped with palisade grass in the summer/autumn, and yellow oat (Avena byzantina “São Carlos”) was oversown, with grazing by lambs in the winter/spring (Pariz et al., 2017b). In the growing season of 2012–2013, soybean to silage was intercropped with guinea grass in the summer/autumn, and the pasture was cut in the winter/spring (Pariz et al., 2016, 2017a).
The climate of this area is Cwa, according to the Köppen climate classification system (Alvares et al., 2013). The long-term (1955–2015) mean annual maximum and minimum temperatures are 26.1 and 15.3°C, respectively, with a mean annual precipitation of 1,359 mm. The precipitation, temperature and incoming radiation were measured from 2013 to 2017 (Table 1).
Table 1. Rainfall, maximum and minimum temperatures, and radiation received at Botucatu, São Paulo, Brazil, during the study period and long-term averages.
The initial chemical characteristics of the soil [pH, organic matter, total acidity at pH 7.0 (H+, Al), exchangeable Al, available P and exchangeable Ca, Mg, and K] at depths of 0–0.20 and 0.20–0.40 m were determined (Table 2). The cation exchange capacity (CEC) was calculated based on the sum of the concentrations of H+, Al, K, Ca, and Mg cations. The base saturation (BS) was calculated by dividing the sum of K, Mg, and Ca (the bases) by the CEC and multiplying the result by 100% (van Raij et al., 2001).
Table 2. Soil chemical characteristics at two depths in the experimental area before the initiation of the experiment.
The experiment had a completely randomized design as a function of soil fertility homogeneity, consisting of four treatments of corn to silage production system in summer/autumn with black oat oversown in winter/spring and twelve replications: (1) corn intercropped with palisade grass and black oat overseeded in lines; (2) corn intercropped with palisade grass and black oat overseeded in a broadcast system with superficial incorporation; (3) corn intercropped with palisade grass + pigeon pea and black oat overseeded in lines; and (4) corn intercropped with palisade grass + pigeon pea and black oat overseeded in a broadcast system with superficial incorporation. The cultivars used were: Marandu, Embrapa 29 and BRS Mandarim (palisade grass, black oat, and pigeon pea, respectively). During winter/spring, the black oat pastures were grazed by lambs, but results regarding forage allowance and nutritive value for animal grazing, as well as results regarding animal performance, are not reported in the present manuscript. The experiment was repeated in the same location for four growing seasons (2013–2014, 2014–2015, 2015–2016, and 2016–2017). Corn was ensiled in 2013–2014, 2014–2015, and 2015–2016, and soybean was ensiled in 2016–2017. The fourth growing season accounted for the residual effect of the crop systems from the previous growing seasons, as the same soybean crop system was applied to all plots. Each plot consisted of twenty 25-m-long rows spaced 0.45 m apart, thus providing a total area of 225 m2. Monoculture plots of corn, palisade grass, or pigeon pea had the same size and number of replications as the other treatments. However, the corn, palisade grass, and pigeon pea monoculture plots were used only for determining the intercropping competition factors and land-use efficiency calculations.
On 20 November 2013, plants comprising the remaining forage grasses and weeds were sprayed with glyphosate (1.44 kg acid-equivalent ha−1) and 2,4-D amine (0.67 kg active ingredient ha−1). On 09 December 2013, any weed regrowth was sprayed with glyphosate (1.08 kg acid-equivalent ha−1). Both herbicide applications used a spray volume of 200 L ha−1.
Corn hybrid 2B587 PowerCore (Dow AgroSciences, Indianapolis, Indiana, USA) with early relative maturity was sown in all crop systems on 16 December 2013, 16 December 2014, and 14 December 2015 at a 4-cm depth, with a row spacing of 0.45 m and a density of 80,000 seeds ha−1, using no-till seeding (Semeato, model Personale Drill 13, Passo Fundo, Rio Grande do Sul, Brazil). When intercropped with corn, palisade grass was simultaneously sown at 12.0 kg ha−1 (pure live seed = 50%). Forage seeds were mixed with basic fertilizer (Pariz et al., 2016, 2017a) and sown at depths of 0.08 m below the soil surface, as described by Crusciol et al. (2012). Pigeon pea was first sown to a depth of 0.04 m using the same no-till seeding method (15 seeds per m) (~35 kg of seeds ha−1), as recommended by Oliveira et al. (2011); then, the corn + palisade grass combination was sown. Therefore, pigeon pea emerged between the rows of corn + palisade grass. For all crop systems and in both growing seasons, basic fertilizer applied in the sowing furrows was 36 kg ha−1 of N as urea, 126 kg ha−1 of P2O5 as triple superphosphate, and 72 kg ha−1 of K2O as potassium chloride, following the recommendation of Cantarella et al. (1997).
Corn seedling emergence occurred 11, 7, and 4 days after sowing (27 December 2013, 23 December 2014, and 18 December 2015, respectively). Differences were due to the absence of rain after sowing in the growing seasons (Table 1). Pigeon pea seedlings emerged 17, 12, and 9 days after sowing (02 January 2014, 28 December 2014, and 23 December 2015, respectively). Forage grass seedlings emerged 25, 22, and 21 days after sowing (10 January 2014, 07 January 2015, and 04 January 2016, respectively). In all growing seasons, due to the large amount of straw on the soil surface, there was no emergence of annual broadleaf weeds, and herbicide application during post-emergence of the corn crop was not necessary. Furthermore, it was not necessary to decrease the initial growth of palisade grass with a herbicide subdose due to the different emergence times of corn and grass seedlings.
On 15 January 2014, 13 January 2015, and 13 January 2016, when corn had four expanded leaves (V4 stage), mineral fertilizer was broadcast with no incorporation at 150 kg ha−1 of N as urea, 38 kg ha−1 of P2O5 as triple superphosphate, and 150 kg ha−1 of K2O as potassium chloride, following the recommendation of Cantarella et al. (1997).
The average corn growing season length from emergence to grain production under 35% moisture was 104, 106, and 108 days (10 April 2014, 08 April 2015, and 04 April 2016, respectively). Whole corn plants were harvested in each plot with a mechanical forage harvester (JF, model C-120, Itapira, SP, Brazil) at 0.45 m above the soil surface. Crops were chopped into particles with an average size of 1.0 cm, and knives were used to shred the corn silage grain. A two-row platform with reduced spacing (0.45–0.55 m between rows) was used to harvest the crops.
On 05 May 2014, 22 April 2015, and 13 April 2016, black oat was oversown in two modalities: (a) seeds were planted in lines to a depth of 3 cm at 65 kg ha−1 pure live seed density with 0.17 m row spacing, using no-till seeding (Semeato, model Personale Drill 13, Passo Fundo, Rio Grande do Sul, Brazil); (b) seeds were broadcast (manually) at 120 kg ha−1 pure live seed density, with superficial incorporation using a disk harrow (fully closed disks for minimum ground disturbance). Both oversown modalities followed the recommendation of Adami and Pitta (2012). In both oversown modalities, black oat seedlings emerged 24, 13, and 20 days after sowing (29 May 2014, 05 May 2015, and 03 May 2016, respectively). Differences in emergence were due to the absence of rain after oversowing during the growing seasons (Table 1).
In the first growing season, 48 (four crop systems × twelve lambs/crop system) uncastrated male crossbred Dorper, Texel and Ile de France lambs, with a mean age of 3 months and an initial body live weight of 27.0 ± 3.2 kg, were used. In the second growing season, 48 (four crop systems × 12 lamb/crop systems) uncastrated male crossbred Poll Dorset and Corriedale lambs with a mean age of 3 months and an initial body live weight of 24.4 ± 3.4 kg were used; 16 (four crop systems × four lambs/crop system) additional lambs were used to adjust the animal stocking rate. In the third growing season, 48 (four crop systems × 12 lambs/crop system) uncastrated male crossbred Dorper and Texel lambs with a mean age of 3 months and an initial body live weight of 26.4 ± 3.5 kg were used. The lambs were blocked based on weight variation and were randomly allocated to the crop systems. The management of lambs was conducted in accordance with the Ethics Committee on Animal Use (CEUA) of São Paulo State University (UNESP) at the College of Veterinary Medicine and Animal Science in Botucatu, São Paulo, Brazil, under protocol number 31/2014-CEUA.
The grazing method was rotational, with stocking at a fixed rate of 44, 55, and 44 lambs ha−1 in the first, second and third growing seasons, respectively, in a semi-feedlot scheme. The initial stocking rates were 1.2, 1.5, and 1.2 Mg ha−1 of body live weight, and the final stocking rates were 1.9, 2.2, and 1.9 Mg ha−1 of body live weight in the first, second, and third growing seasons, respectively. The grazing period in each paddock was 3 days, and the rest period was 33 days, totaling 12 paddocks per treatment. Two cycles of grazing were carried out, 36 and 33 days (first and second cycles, respectively), totaling 69 days. Lambs were on the pasture throughout the day (from 6:00 h. to 18:00 h.).
On 17 September 2016, 2.0 Mg ha−1 of dolomitic lime (CaCO3. MgCO3) with 28% CaO and 20% MgO was broadcast onto the soil surface, following the recommendation of Crusciol et al. (2016). On 02 December 2014, 16 November 2015, and 10 November 2016, plants composed of the remaining forage grasses and weeds were sprayed with glyphosate (1.44 kg acid-equivalent ha−1) and 2,4-D amine (1.34 kg active ingredient ha−1). On 17 December 2014, 04 December 2015, and 25 November 2016, any weed regrowth was sprayed with glyphosate (1.08 kg acid-equivalent ha−1) and on 02 December 2016, mulch was sprayed with paraquat (0.4 kg active ingredient ha−1). All herbicide applications used a spray volume of 200 L ha−1.
The soybean cultivar “AS 3610 IPRO—INTACTA RR2 PRO” (super early cycle, maturity group 6.1, and indeterminate growth rate) was sown on 06 December 2016 at a 4-cm depth at a density of 350,000 seeds ha−1 and a row spacing of 0.45 m using no-till seeding (Semeato, model Personale Drill 13, Passo Fundo, Rio Grande do Sul, Brazil). The fungicide carboxin + thiram and the insecticide thiamethoxam were applied to soybean seeds at doses of 60 and 120 g of active ingredient (a.i.) to 100 kg of seeds, respectively. The soybean seeds were inoculated with Bradyrhizobium japonicum (SEMIA 5079—CPAC 15 and SEMIA 5080—CPAC 7) at 1,200 cells seed−1. All soybean crop systems were fertilized in furrows, with 7 kg ha−1 of N as urea, 70 kg ha−1 of P2O5 as triple superphosphate and 70 kg ha−1 of K2O as potassium chloride. In all crop systems, guinea grass cv. Aruana was intercropped with soybean and planted at 15 kg ha−1 (pure live seed = 32%). Forage seeds were mixed with basic fertilizer (Pariz et al., 2016, 2017a) and sown at depths of 0.06 m below the soil surface, as described by Crusciol et al. (2012). Soybean and guinea grass seedlings emerged 6 and 15 days after sowing, respectively (12 December 2017 and 21 December 2017, respectively). The herbicide glyphosate (0.54 kg acid-equivalent ha−1) was applied 7 days after the emergence of the soybean seedlings. All crop systems were side-dressed 23 days after soybean emergence with 90 kg ha−1 K2O as potassium chloride, with incorporation using a row crop cultivator for NTS (Tatu Marchesan, model CPD, Matão, São Paulo, Brazil).
Soybeans were cultivated according to crop needs (Embrapa—Empresa Brasileira de Pesquisa Agropecuária, 2006). The application of phytosanitary products was as follows: insecticide thiamethoxam + lambda-cyhalothrin (21 and 16 g of a.i. ha−1, respectively) during the V4 stage; the fungicide trifloxystrobin + prothioconazole (30 and 35 g of a.i. ha−1, respectively) and insecticide thiamethoxam + lambda-cyhalothrin (28 and 21 g of a.i. ha−1, respectively) during the R1 stage; the fungicide trifloxystrobin + prothioconazole (38 and 44 g of a.i. ha−1, respectively) and insecticide acephate (600 g of a.i. ha−1) during the R4 stage; and the fungicide azoxystrobin + benzovindiflupyr (60 and 30 g of a.i. ha−1, respectively) and insecticide thiamethoxam + lambda-cyhalothrin (28 and 21 g of a.i. ha−1, respectively) during the R6 stage. All fungicide and insecticide applications used a spray volume of 200 L ha−1 and adjuvant mineral oil (65 g ha−1).
The length of the soybean season (from emergence to growth stage R7—beginning of bean maturity and 50% yellow leaves), according to Fehr and Caviness (1977), was 95 days (16 March 2017). In this stage, whole soybeans and guinea grass plants were harvested according to the recommendation of Leonel et al. (2008) with a mechanical forage harvester (Model JF C-120 with 12 knives and total platform area of 1.30 m, Itapira, São Paulo, Brazil). The crops (soybean and guinea grass) were harvested at 0.15 m above the soil surface.
Corn leaf samples were collected for nutrient analysis when 50% of corn plants were in the full flowering stage. Selection was randomized, with 30 plants chosen per plot. The fourth leaf with a visible sheath from the apex was collected following the methods of Cantarella et al. (1997). Soybean leaf samples were collected, i.e., the upper third trifoliate leaf from top to bottom at the R2 growth stage during full bloom (Fehr and Caviness, 1977). Petioles from 30 plants per plot were collected as proposed by Ambrosano et al. (1997). Leaf samples were washed, dried by forced-air circulation at 65°C for 72 h, grinding was carried out in a Willey mill (0.85 mm sieve) and analysis were carried out to determine the chemical composition. The concentrations of N, P, K, Ca, Mg, S, B, Cu, Fe, Mn, and Zn were determined using the methods described by Malavolta et al. (1997). Nitrogen was extracted with H2SO4, and the other nutrients were extracted with a nitro-perchloric solution. The nitrogen concentration in the digested solution was determined by Kjeldahl analysis. The concentrations of the other nutrients were determined using atomic absorption spectrophotometry.
The corn plant population and number of ears (NE) (i.e., number of plants and ears in the four central rows, excluding 1 m from the end of each side of the row of each plot, extrapolated to plants, and ears per hectare) were evaluated. The plant height (PH), main ear insertion height (MEIH), and basal stalk diameter (BSD) were also evaluated. From harvested whole corn to silage, palisade grass, and pigeon pea plants, a representative sample was dried by forced-air circulation at 65°C for 72 h to determine the forage mass (Mg ha−1) and the proportions (%) of corn grains, palisade grass, and pigeon pea in the forage mass. Using the same plant evaluation methodology, current-year corn, palisade grass, and pigeon pea mulch were collected from the soil surface to determine the remaining straw (Mg ha−1). Concentrations of macro and micronutrients were determined (same methods described for corn leaf nutrient). Nutrient concentrations were multiplied by the quantity of forage mass and mulch to determine the nutrient contents (kg ha−1).
The soybean plant population (SPP) (calculated from the number of plants in the four central rows, excluding 1 m from the end of each side of the row of each plot, extrapolated to plants per hectare) was evaluated. The PH, height of the first pod insertion (HFPI), number of pods per plant (NPP), number of seeds per pod (NSP), dry weight of 100 seeds (W100), and dry weight of seeds per hectare were also evaluated. Soybean and guinea grass were harvested at 0.15 m above the soil surface. A representative sample was dried by forced-air circulation at 65°C for 72 h to determine the forage mass (Mg ha−1). Using the same plant evaluation methodology, current-year soybean, and guinea grass mulch were collected from the soil surface to determine the remaining straw (Mg ha−1).
The relative nutrient yield was calculated as the N, P, K, Ca, Mg, S, B, Cu, Fe, Mn, and Zn yield of the species in the mixture divided by the nutrient yield of the species in the monoculture based on the harvested nutrient yields (crop yield multiplied by the % nutrient concentration of the aboveground biomass) according to Lüscher and Aeschlimann (2006). The land equivalent ratio (LER) was calculated according to Mead and Willey (1980). The relative crowding coefficient (K) and aggressivity (A) were calculated according Agegnehu et al. (2006).
After grazing by lambs, following pasture and weed desiccation with glyphosate herbicide, estimates were obtained for the plant material killed (i.e., mulch quantity). Along two diagonal transects in each plot, three evaluators placed metal grid squares (1 m2) on the ground in three areas per plot and cut all plant material to the ground level. The collected material was dried by forced-air circulation at 65°C for 72 h, weighed, ground, and reported in Mg ha−1. Concentrations of macro and micronutrients were determined (same methods described for corn leaf nutrient). Nutrient concentrations were multiplied by the quantity of mulch to determine the nutrient contents (kg ha−1). The lignin content was also determined according to the method described by Silva and Queiroz (2002) and was used to calculate the total lignin/N ratio.
To determine the rate of decomposition, fresh forage from each plot was placed in nylon bags (litter bags of 0.06 m2, 0.3 × 0.2 m), proportionate to the standing mass (Pariz et al., 2011b). Litter bags were distributed and left on the soil for 15, 30, 60, 90, and 120 days. One litter bag per plot was removed at each sampling time as a function of days after desiccation (DAD) of pasture and weeds due to glyphosate herbicide following grazing. The contents of each litter bag were collected, purified by sieving, and rinsing with distilled water, and dried at 65°C to a constant weight to determine the dry weight. Subsequently, the concentrations of N, P, K, Ca, Mg, S, B, Cu, Fe, Mn, and Zn of the remaining forage residue per litter bag were determined according to the method proposed by Malavolta et al. (1997). The nutrient concentrations were multiplied by the amount of remaining residues, calculating the respective release rates for a period of 120 days (Pereira et al., 2016).
All data were initially tested for normality with the Shapiro and Wilk (1965) test using the UNIVARIATE procedure of SAS Institute (2015, SAS Institute Inc., Cary, NC). All data were distributed normally (W ≥ 0.90). The data were analyzed using the PROC MIXED procedure of SAS and Satterthwaite approximation to determine the denominator degrees of freedom for the test of fixed effects. The crop systems were considered fixed effects. A repeated statement was used with the growing season specified as the repeated variable. The covariance structure used in the analyses was autoregressive, which provided the best fit according to the Akaike information criterion. The results were reported as least square means and were separated by preplanned pairwise comparisons (PDIFF). The mean separations were conducted using an LSD test. The effects were considered statistically significant at p ≤ 0.05. Straw decomposition and nutrient release rates were analyzed, as suggested by Wider and Lang (1982), with the litter bag method, using the PROC REG procedure of SAS, and the best adjustments chosen had the highest coefficients of determination (r2) at p ≤ 0.05. Error bars represent standard errors (SEs), and the means were determined using the PROC MEANS procedure of SAS.
The temperatures during the second (2014–2015) and third (2015–2016) growing seasons were relatively similar (Table 1) and appropriate for corn cultivation (Borghi et al., 2013b; Crusciol et al., 2013). According to Bergamaschi et al. (2004) and Araujo et al. (20111), the amount of precipitation would have allowed corn and intercropped forages to develop without water stress only during the second and third growing seasons (between 500 and 800 mm). In the fourth growing season (2016–2017), the weather conditions were also appropriate for soybean development (Crusciol et al., 2012, 2014). According to Embrapa—Empresa Brasileira de Pesquisa Agropecuária (2006), the amount of precipitation would have caused minimal water stress of soybean and intercropped guinea grass (between 450 and 850 mm).
In the first growing season (2013–2014), the rainfall (754 mm) was 45% lower than the historical average (1,360 mm), with low rainfall occurring in the summer (mainly from 17 January 2014 to 15 February 2014), which was associated with a greater mean maximum temperature than the historical average and caused severe water stress mainly during corn vegetative development, with precipitation around 300 mm between corn seedlings emergence until harvest (December 2013 to March 2014; Table 1). In the second and third growing seasons (2014–2015 and 2015–2016, respectively), rainfall amounts of 1,776 and 2,026 mm were 23 and 49% higher, respectively, than the historical averages, and the mean maximum and minimum temperatures were similar to the historical average. In the fourth growing season, the rainfall amount of 940 mm from November 2016 to March 2017 was 15% higher than the historical average during this period (820 mm), and the mean maximum and minimum temperatures were similar to the historical averages. The radiation received by corn between seedling emergence and harvest was similar in all growing seasons (1,986, 2,142, and 2,065 MJ m−2 in the first, second and third growing seasons, respectively), and in all months, the values were similar to the historical average. The radiation received by soybean between seedling emergence and harvest was 1,919 MJ m−2 in the fourth growing season.
Between the harvesting of corn and stocking of lambs on pastures, rainfall amounts of 151, 232, and 302 mm occurred in the first, second and third growing seasons, respectively, and after the oversowing of black oat, rainfall amounts of 85, 189, and 302 mm occurred in the first, second and third growing seasons, respectively. The radiation received by pasture between the corn harvest and stocking of lambs was similar for all growing seasons (1,243, 1,231, and 1,050 MJ m−2 in the first, second and third growing seasons, respectively).
The crop system affected the N, Cu, and Mn concentrations and the growing season affected Ca, Mg, and B concentrations of corn leaf tissue (Table 3). The crop system affected the standing plant residues, forage mass, and concentration of corn grains, and the growing season affected the corn plant population, NE per hectare, PH (corn, palisade grass, and pigeon pea), MEIH, bulk stalk diameter, standing plant residues, forage mass, proportions of corn grains, palisade grass, and pigeon pea (Table 4). Forage mass was also affected by the interaction between crop system × growing season.
Table 3. Leaf nutrient concentrations at the full flowering stage in corn to silage intercropped with palisade grass (C + PG) and with palisade grass and pigeon pea (C + PG + PP) with black oat (BO) oversown in lines and broadcast in three growing seasons.
Table 4. Corn plant population (CPP), number of ears per hectare (NE), plant height (PH), main ear insertion height (MEIH), basal stalk diameter (BSD), standing plant residue (SPR), forage mass (FM), and proportions of corn grains (CG), palisade grass and pigeon pea in corn to silage intercropped with palisade grass (C + PG) and with palisade grass and pigeon pea (C + PG + PP) with black oat (BO) oversown in lines and broadcast in three growing seasons.
Corn intercropped with palisade grass and pigeon pea had higher N, K, and Fe contents in the forage mass, and growing season affected only the N content in the forage mass (Table 6). Corn intercropped with palisade grass and pigeon pea had higher nutrient contents in standing residue than other crop systems, and growing season also affected all nutrient contents in standing residue.
Intercropping corn with palisade grass and pigeon pea increased the total LER in the three growing seasons compared to intercropping corn with palisade grass alone (Table 7). Intercropping corn with palisade grass and pigeon pea affected the K-value of corn and palisade grass in the three growing seasons compared to intercropping corn only with palisade grass and affected the A-value of corn in the three growing seasons as well as the A value of palisade grass only in the first growing season compared to intercropping corn only with palisade grass.
The inclusion of pigeon pea in the production system with intercropped corn and palisade grass provided an increase in the surface mulch, mulch cover and nutrient contents and caused a decrease in the lignin/N ratio, mainly with black oat oversown in lines (Table 9). The growing season also affected all these attributes except the lignin/N ratio.
With a lower lignin/N ratio than other treatments, surface mulch in the palisade grass plots generated during triple intercropping had greater initial decomposition [logarithmic (log 10) decomposition]; furthermore, with a higher lignin/N ratio than other treatments, surface mulch in the palisade grass plots generated during double intercropping had lower initial decomposition (Figure 1). The release rate of N was similar to that of surface mulch decomposition in all crop systems. The rate of release of P, K, Mg, and B contained in the plant residues was logarithmic over 120 DAD; however, there was no crop system effect (Figures 1–3). The release of Ca, S, Cu, Fe, Mn, and Zn from the plant residues was exponential at 120 DAD; however, without a crop system effect, and in the first 30 days, the release of these nutrients was slower than that of the other nutrients (Figures 2–4).
Figure 1. Release rate of remaining straw, nitrogen, and phosphorus in palisade grass (PG) intercropped with corn (C) and with corn and pigeon pea (PP) to silage with black oat (BO) oversown in lines and broadcast after grazing by lambs as a function of days after desiccation (mean of three growing seasons). Values are the mean of 12 replicates, and the associated error bar is ± 1 SE. Days after desiccation (DAD): days after pasture and weed desiccation with glyphosate herbicide, after grazing by lambs.
Figure 2. Release rate of potassium, calcium, and magnesium in palisade grass (PG) intercropped with corn (C) and with corn and pigeon pea (PP) to silage with black oat (BO) oversown in lines and broadcast after grazing by lambs as a function of days after desiccation (mean of three growing seasons). Values are the mean of 12 replicates, and the associated error bar is ± 1 SE. Days after desiccation (DAD): days after pasture and weed desiccation with glyphosate herbicide, after grazing by lambs.
Figure 3. Release rates of sulfur, boron, and copper in palisade grass (PG) intercropped with corn (C) and with corn and pigeon pea (PP) to silage with black oat (BO) oversown in lines and broadcast after grazing by lambs as a function of days after desiccation (mean of three growing seasons). Values are the mean of 12 replicates, and the associated error bar is ± 1 SE. Days after desiccation (DAD): days after pasture and weed desiccation with glyphosate herbicide, after grazing by lambs.
Figure 4. Release rates of iron, manganese, and zinc in palisade grass (PG) intercropped with corn (C) and with corn and pigeon pea (PP) to silage with black oat (BO) oversown in lines and broadcast after grazing by lambs as a function of days after desiccation (mean of three growing seasons). Values are the mean of 12 replicates, and the associated error bar is ± 1 SE. Days after desiccation (DAD): days after pasture and weed desiccation with glyphosate herbicide, after grazing by lambs.
The residual effect of corn intercropped with palisade grass and pigeon pea, mainly with black oat oversown in lines, resulted in higher N and K concentrations in soybean leaves (Table 10). Residual crop system effects were significant for the SPP, PH, HFPI, NPP, dry weight of 100 seeds, dry weight of seeds per hectare, forage mass, and standing plant residue, with higher values after triple intercropping than after double intercropping (Table 11).
In all crop systems, corn leaf nutrient concentrations were optimal under sufficient ranges (Cantarella et al., 1997), despite following a recommended seeding and side-dressing fertilization programme (Table 3). Previous studies have shown higher cash-crop leaf nutrient concentrations when these crops are intercropped with palisade grass (Crusciol et al., 2012; Borghi et al., 2013b). However, the inclusion of pigeon pea in intercropping increased corn leaf N, Cu, and Mn concentrations (increase of 9, 18, and 36%, respectively), demonstrating a direct transfer of these nutrients to the cereal crop (Xiao et al., 2004).
A reduction in corn leaf Ca and Mg concentrations over the three growing seasons suggested the absorption of these nutrients that depended on the effectiveness of the root systems and nutrient availability (Table 3). Monteiro et al. (1995) found that “the omission of Mg in nutrient solutions for cultivating palisade grass reduced the production of root dry matter by 70% compared to a treatment with adequate concentrations of Mg.” Although the levels of Ca and Mg in the soil were adequate before implementation of the experiment (Table 2), a short- to medium-term ICLS began in the growing season of 2010–2011 with dolomitic lime and agricultural gypsum application (Pariz et al., 2016, 2017a,b). In this context, the results of Crusciol et al. (2016) indicated that reapplication of lime to the surface of no-till soil can improve plant nutrition, dry matter production, crop yield, revenue and the long-term sustainability of tropical agriculture in the Brazilian “Cerrado.” Therefore, since this agricultural system has high nutrient exports, the availability of Ca and Mg in the soil should be monitored annually, with surface lime reapplications when necessary to prevent deficiency in plants. Accordingly, prior to soybean sowing, dolomitic lime was broadcast onto the soil surface. Another nutrient that supported this necessary annual monitoring is B because the concentration of this nutrient in corn leaves was lower in the third growing season than in the first and second growing seasons.
Greater standing plant residue with the incorporation of pigeon pea as an intercrop was due to the stems of this crop growing closer to the ground (Table 4). Corn intercropped with palisade grass had a lower forage mass than that of other crop systems during the first growing season (Table 5). This was a function of low rainfall in the summer, which was associated with higher mean maximum temperature than the historical average and caused severe water stress during corn vegetative development. Thus, the inclusion of pigeon pea in the production system intercropped with corn and palisade grass generated an increase of 16% in the forage mass during the first growing season, probably due to the development of the pivotal root system of pigeon pea (up to 1 m deep in relation to the soil surface), which resulted in greater water absorption, causing the crop to be more resistant to the water deficit. Therefore, the mass that was produced by the pigeon pea intercropped with corn and palisade grass allowed for the forage mass (~15 Mg ha−1) to be similar to that produced during the other growing seasons without water deficiency. The inclusion of pigeon pea accounted for ~5.8–6.4% in the forage mass (Table 4), reducing the proportion of corn grains in the forage mass from 50.0–50.5% to 47.5–46.7%.
Table 5. Forage mass of corn to silage intercropped with palisade grass (C + PG) and with palisade grass and pigeon pea (C + PG + PP) with black oat (BO) oversown in lines and broadcast in three growing seasons.
The severe water stress during corn vegetative development during the first growing season also reduced the corn plant population, NE, PH (corn and palisade grass), MEIH, BSD, standing plant residue, and forage mass compared to the two other growing seasons (Table 4). However, as a function of the best resistance to the water deficit of pigeon pea previously discussed, associated with lower corn PH (greater incidence of light between the crop rows), the PH and proportion of pigeon pea in the forage mass were highest in the first growing season. Furthermore, it is noteworthy that after the flowering and pollination of the corn crop, the rainfall normalized, promoting adequate grain filling. Thus, as the corn plants had lower mass of leaves and stems, higher proportions of grains, and palisade grass were verified in the forage mass.
Considering that the proportion of pigeon pea in the forage mass was 9.4% in the first growing season and ~4.5% in the second and third growing seasons (Table 4) and that this legume had a higher N concentration than corn and palisade grass, this result demonstrates how the inclusion of legumes in crop systems promotes the accumulation of plant nitrogen via the biological fixation of atmospheric nitrogen (N2) (Oliveira et al., 2011), which increases the content of this nutrient in the forage mass and in standing residue (Table 6).
Table 6. Nutrient content in forage mass and in standing plant residue of corn to silage intercropped with palisade grass (C + PG) and with palisade grass and pigeon pea (C + PG + PP) with black oat (BO) oversown in lines and broadcast compared to corn, palisade grass, and pigeon pea monoculture in three growing seasons.
Furthermore, silage with more protein, as is the case in a corn-legume intercrop system, can reduce the use of protein concentrates, which increase the cost of an animal's diet. Also over the growing seasons [first (2013–2014) to third (2015–2016)], a greater accumulation of N occurred in the forage mass and in standing residue (Table 6), which can be considered an effect of the transition phase of NTS (between 5 and 10 years, considering that the experimental area has been managed since 2010). At this stage, the accumulation of straw on the soil surface begins, and N immobilization is similar to mineralization (Sá et al., 2009). A carryover effect of legumes may also occur in the crop system over the three growing seasons. The same carryover effect was also verified for all nutrients in standing residue, with higher contents in the second and third growing seasons than in the first growing season.
The higher N content in the forage mass and in standing residue from crop systems with oversown black oat in lines during the winter/spring compared to broadcast oversown black oat (Table 6) was due to the lower loss of soil nitrogen (emission of N2O) in the line treatment because of the minimum ground disturbance under no-till seeding. In broadcast treatments, the superficial incorporation of black oat seeds using a disk harrow increased the pore space. High N2O emission rates occur when the soil has a large proportion of water-filled pores, above 60%, which makes it difficult for O2 to diffuse into the soil and favors the formation of anaerobic environments (Bateman and Baggs, 2005).
The higher K content in standing residue in the corn to silage systems likely provided significant K recycling and contributed to exchangeable forms in the soil (Garcia et al., 2008). Corn intercropped with palisade grass and pigeon pea also had a higher content of all nutrients in standing residue than other crop systems (Table 6). Thus, as a function mainly of stems that remained on the soil surface after harvest, pigeon pea had ~0.7–0.9 Mg ha−1 more standing plant residue than that measured when there was no inclusion of this species in the cropping system (Table 4), which contributed to the greater accumulation of all nutrients in standing residue. Furthermore, considering that the soil of the experimental area is a Typic Haplorthox rich in magnetite (iron oxide), pigeon pea exhibited a strong capacity for Fe absorption and accumulation.
As previously reported, the low rainfall in the summer of the first growing season (Table 1) caused severe water stress during corn vegetative development. Thus, in the first growing season, as a function of the best resistance to water deficit and of increased competition with corn and palisade grass, pigeon pea resulted in higher individual (0.40–0.44 × 0.19–0.23) and total (1.63–1.59 × 1.28–1.31) LER than in the two other growing seasons (Table 7). During the second and third growing seasons, corn may have competed with pigeon pea, slightly reducing the individual LER (~0.10). However, the LER of pigeon pea was ~0.20, offsetting this reduction and resulting in a greater total LER.
Table 7. Land equivalent ratio (LER) of corn, palisade grass, and pigeon pea monoculture, relative crowding coefficient (K) and aggressivity (A) of corn to silage intercropped with palisade grass (C + PG) and with palisade grass and pigeon pea (C + PG + PP) with black oat (BO) oversown in lines and broadcast in three growing seasons.
In all growing seasons, palisade grass, and pigeon pea exhibited weak interspecific competition, but corn was strongly competitive in interspecific interactions (Table 7) because of the corn value (–K) and the palisade grass and pigeon pea value (+K) (Zarochentseva, 2012). However, the values of palisade grass and pigeon pea were extremely low, and the value of corn was closer to zero when intercropped only with palisade grass. In the triple intercrop (corn + palisade grass + pigeon pea), the K-value of corn and palisade grass was far from zero, demonstrating that these crops had to be much more competitive in the presence of pigeon pea.
Over three growing seasons, all of the crop system A-values for corn were negative, whereas such values for palisade grass and pigeon pea were always positive (Table 7), indicating that these crops presented higher aggression to compete with corn as a function of the high rates of corn dry matter accumulation (Pariz et al., 2017c). In the three growing seasons, the intercropping of pigeon pea reduced the aggressivity of corn, and in the first growing season, as a function of the less favorable weather conditions already discussed, the aggressivity of palisade grass was also lower. However, in all cases, the A-values were extremely low, indicating that this aggressivity was minimal.
Concerning the individual relative nutrient yields, only the N of corn was affected by the cropping system (Table 8). In the same way as N in corn leaves (Table 3) and the N content in the forage mass and in standing residue (Table 6), the relative N yield was higher in crop systems with triple intercropping (corn + palisade grass + pigeon pea) than in systems with double intercropping (corn + palisade grass), mainly with black oat oversown in lines in winter/spring compared to oversown black oat that was broadcast, also reflecting a higher total relative N yield.
Table 8. Relative nutrient yield (%) of corn to silage intercropped with palisade grass (C + PG) and with palisade grass and pigeon pea (C + PG + PP) with black oat (BO) oversown in lines and broadcast compared to corn, palisade grass, and pigeon pea monoculture (mean of three growing seasons).
It should be noted that of all the nutrients, other than N, only P and Zn presented relative yields in corn plants >100% in all cropping systems (Table 8). Therefore, the results show that intercropping with palisade grass resulted in an ~20% higher accumulation of these nutrients in the corn plants than monocropping. Tropical perennial grasses introduced into a cropping system resulted in a higher soil labile P content than fallow systems, probably because tropical perennial grasses can take up moderately labile soil P fractions that are recycled in the system, regardless of the P fertilization strategy (Almeida and Rosolem, 2016).
The relative yield of the other nutrients (K, Ca, Mg, S, B, Cu, Fe, and Mn) in the corn plants was <100% in all crop systems (Table 8). Among these nutrients, what surprised us was K, of which the accumulation reached approximately only 60% in all crop systems compared to monocropped corn. This is very good from the standpoint of extracting this nutrient in corn to silage production areas because the replacement management of K in these areas is a problem since the harvesting of corn to silage plants extracts large amounts of K from the soil (Pariz et al., 2016, 2017a). Thus, assuming that plants in all crop systems were adequately supplied with K (Table 3), we can hypothesize that monocropped corn presented extensive absorption of this nutrient, mainly from exchangeable forms in soil, which may exhaust this more available form of K in the soil over the medium to long term.
According to Rosolem and Steiner (2017) “regarding recommendations on sustainable fertilizer practices, sampling the 0–0.2-m layer seems to be insufficient, and increased rates of fertilizer K intensify K leaching below 1 m in tropical sandy clay loam soil.” In this context, the results of Garcia et al. (2008) demonstrated that “K recycling in intercropping systems is very important for plant growth to avoid losses in the soil profile. However, it must be emphasized that if K rates are underestimated in a fertilization programme, mainly corn to silage, soil K reserves may be depleted, as corn intercropped with palisade grass is very effective in recycling K, leading to increased exchangeable K contents in the surface soil layers; further, palisade grass is able to take up non-exchangeable forms of K in the soil. Ultimately, this K, after cover crop desiccation, is washed out of plant residues, and eventually, more K is available for crops in succession.” Furthermore, corn to silage intercropped with palisade grass at greater heights, as performed in the present study (0.45 m above the soil surface), can contribute to K cycling from K contained in the lower internodes of plants (Pariz et al., 2016, 2017a). Therefore, the intercropping of palisade grass with corn becomes an excellent alternative in the cycling and maintenance of exchangeable K content in the soil, not allowing the extensive absorption of this nutrient by corn plants. The same analogy can be considered true for the other nutrients (Ca, Mg, S, B, Cu, Fe, and Mn) because the relative yield in corn plants was <100%, and the corn plants were well-fed (Table 8). Thus, we can also hypothesize that the contact of different intercropped crop roots can inhibit this expansive absorption of some nutrients by corn plants (Hauggaard-Nielsen and Jensen, 2005).
The relative yield of all nutrients in palisade grass and pigeon pea plants was <100% and was not influenced by crop system (Table 8). However, the incorporation of pigeon pea in corn intercropped with palisade grass increased the total relative yield of all nutrients. Therefore, considering that the presence of pigeon pea in the triple intercrop did not interfere with the relative nutrient yields of corn and palisade grass, it can be inferred that this legume possibly absorbed and accumulated nutrients that might not be absorbed by corn and palisade grass plants, especially in deeper soil depths, as a function of the pivotal root system of pigeon pea (up to 1 m deep in relation to the soil surface).
The higher surface mulch quantity, mulch cover, and N and K contents, mainly in the triple intercrop with black oat oversown in lines compared to double intercrop with black oat oversown in broadcast (increase of 75, 50, 72, and 71%, respectively), despite subsequent grazing by lambs (Table 9), was probably due to the nitrogen dynamics and soil decompaction under the development of the pivotal root system of pigeon pea. In addition, results of Costa et al. (2021) demonstrated that the inclusion of pigeon pea in the intercrop of corn with palisade grass efficiently increased the use of N fertilizer by corn, promoted greater N recovery in the soil, and increased total N in the crop system, thus decreasing unrecovered N from fertilizer in the plant-soil system, what according to Sprent (2007) can be explained because occurs symbiosis between plants and bacteria found in root nodules. In the case of oversown oats, the in-row modality provides better distribution, seed burial, and uniformity of the plant population than the broadcast modality. Furthermore, superficial incorporation into the soil using a disk harrow (fully closed disks for minimal ground disturbance) is necessary for better germination and to reduce the number of seeds vulnerable to consumption by birds. However, this mechanical operation can reduce the soil nitrogen concentration because it increases the porous space and breaks the stability of the soil organic matter, favoring greater N2O emission.
Table 9. Surface mulch quantity, mulch cover, nutrient contents, and lignin/N ratio (Lig/N) in surface mulch of palisade grass intercropped with corn (PG + C) and with corn and pigeon pea (PG + C + PP) to silage with black oat (BO) oversown in lines and broadcast in three growing seasons after grazing by lambs.
However, in all crop systems, the mulch remaining 120 DAD was ~50–60% (Figure 1). Other nutrient (P, Ca, Mg, S, B, Cu, Fe, Mn, and Zn) contents were also higher when palisade grass was intercropped with corn and pigeon pea than when intercropped with other treatments; however, there was no effect of oversown black oat. The surface mulch quantity, mulch cover, and all nutrient contents increased in the third growing season compared with the second and first growing seasons, possibly due to a carryover effect and more time between the end of grazing by lambs and desiccation of the remaining plants.
The crop system affected the lignin/N ratio of the surface mulch of palisade grass (Table 9), which was lower in crop systems with triple intercropping than in those with double intercropping, because of the highest N content in plant surface mulch. Potassium was the nutrient with the highest accumulation in the mulch of palisade grass and exceeded 100 kg ha−1 in all crop systems and nitrogen was the nutrient with the second highest accumulation in the mulch of palisade grass (Table 9); in general, the residues released ~50–60% of this nutrient at 120 DAD. The peak release of P, K, Mg, and B occurred in the first 30 days and in general, the residues released more than 60% of this nutrient prior to 120 DAD and at ~90 DAD, K had been completely released from the palisade grass residues (Figure 2). The remaining Mg (30–40%) was subsequently and gradually released as part of the structural plant compounds. In this way, at 30 DAD, ~50% of the Mg contained in the plant residues had been released.
Concentrations of N in soybean leaves (Table 10) were slightly below a sufficiency level when previous crop systems were corn intercropped only with palisade grass (Ambrosano et al., 1997; Embrapa—Empresa Brasileira de Pesquisa Agropecuária, 2006). This result demonstrated that crop systems without the inclusion of legumes in intercropping or crop rotation over time may reduce the availability of N and consequently uptake by crops. The triple intercrop with black oat oversown in lines increased 23% the N content in soybean leaves compared to double intercrop with both black oat oversown systems. The differences in K concentrations in soybean leaves as a function of previous crop systems were possibly a function of the K content in the surface mulch (Table 9), demonstrating good nutrient cycling and a synchronized K release rate from mulch with absorption by soybeans during their growth cycle. The triple intercrop with black oat oversown in lines increased 13% the K content in soybean leaves compared to double intercrop with both black oat oversown systems. Despite no differences among crop systems with respect to other nutrients (P, Ca, Mg, S, B, Cu, Fe, Mn, and Zn), soybean leaf concentrations were within a range considered appropriate for soybean crops (Ambrosano et al., 1997; Embrapa—Empresa Brasileira de Pesquisa Agropecuária, 2006).
Table 10. Soybean leaf nutrient concentrations at the R2 growth stage intercropped with guinea grass to silage in the fourth growing season in succession with corn to silage intercropped with palisade grass (C + PG) and with palisade grass and pigeon pea (C + PG + PP) with black oat (BO) oversown in lines and broadcast in the first three growing seasons.
The higher values of SPP after triple intercropping (corn + palisade grass + pigeon pea) (Table 11) were a function of a higher quantity of surface mulch and the best mulch cover (Table 9), which reduced the bird attacks (mainly doves) on soybean seedlings. According to Yokomizo et al. (2000), the HFPI should be >0.12 m to enable proper mechanical harvesting of soybean. In our study, the HFPI of soybean was 0.13–0.15 m; therefore, mechanized harvesting was not a problem. The higher values of NPP, dry weight of 100 seeds (W100), dry weight of seeds per hectare, forage mass (FM), and standing plant residue (SPR) after triple intercropping can be explained by better mulch cover, N and K plant nutrition (Table 10) and possibly soil physical characteristics as a function of the root system of the pigeon pea and palisade grass discussed above, which favored the vegetative and reproductive growth of soybean. Thus, the combination of higher SPP, NPP, and W100 was reflected in ~1.0, 1.5, and 0.4 Mg ha−1 more seeds, FM and SPR, respectively, than in crop systems with double intercropping (corn + palisade grass). Despite a lack of data on the N derived from the soil, pigeon pea may have provided some N (Baldé et al., 2011) to soybeans and guinea grass to enhance the seeds and FM (Table 11). The triple intercrop with both black oat oversown increased 24 and 16% the seeds and FM of soybean compared to double intercrop with both black oat oversown systems.
Table 11. Soybean plant population (SPP), plant height (PH), height of the first pod insertion (HFPI), number of pods per plant (NPP), number of seeds per pod (NSP), dry weight of 100 seeds (W100), dry weight of seeds per hectare, forage mass (FM), and standing plant residue (SPR) on the soil surface of soybean to silage intercropped with guinea grass in the fourth growing season in succession with corn to silage intercropped with palisade grass (C + PG) and with palisade grass and pigeon pea (C + PG + PP) with black oat (BO) oversown in lines and broadcast in the first three growing seasons.
Our study revealed the potential for intercropping palisade grass and pigeon pea with corn in summer/autumn in the Brazilian “Cerrado” for produce forage mass and, subsequently, mulching in NTS. In addition, oversown black oat with palisade grass as a cover crop is also an good option for mulch production in the spring. Thus, with the inclusion of pigeon pea, maintaining long-term productivity would require fewer external N inputs. However, more constant monitoring of soil fertility, with an emphasis on the application of limestone, gypsum, phosphorus, and micronutrients, is necessary, especially because a majority of the biomass produced in these ICLS throughout the year is exported via the cutting of corn plants for ensilage.
Despite greater interspecific competition of palisade grass and pigeon pea intercropped with corn, this more complex system had better results. Thus, when analyzing this ICLS as a whole during the four growing seasons, the triple intercrop (corn + pigeon pea + palisade grass) combined with oversown black oats (mainly in lines) in the first three growing seasons was the most effective option for silage production and for the improvement of other elements of system productivity, such as higher surface mulch quantity (75%), leaf nutrient concentrations, mainly nitrogen and potassium (23 and 13%, respectively), and yield of soybean forage mass (16%) to silage intercropped with guinea grass in the fourth growing season. This type of intercropping also produced better nutrient cycling because it increased the quantity of nutrients retained in SPRs and surface mulch and provided better land- and nutrient-use efficiency, with an emphasis on nitrogen and potassium.
The original contributions presented in the study are included in the article/supplementary materials, further inquiries can be directed to the corresponding author/s.
The animal study was reviewed and approved by this study was conducted in accordance with the Ethics Committee on Animal Use (CEUA) of São Paulo State University (UNESP) at the College of Veterinary Medicine and Animal Science in Botucatu, São Paulo, Brazil, under protocol number 31/2014-CEUA.
CP, NC, and FA: experimental work, data analysis, results interpretation, and writing. CC, CACC, PM, JC, MA, and AF: results interpretation and writing. AC: data analysis, results interpretation, and writing. DS, IC, and VL: experimental work and writing. VP, JS, MP, VM, RS, DF, and LS: experimental work. All authors contributed to the article and approved the submitted version.
The authors declare that the research was conducted in the absence of any commercial or financial relationships that could be construed as a potential conflict of interest.
The authors thank the São Paulo Research Foundation (FAPESP) (grant #2013/13702-3, #2013/23853-9, #2014/12950-6, #2014/14935-4, #2014/21772-4, #2015/25413-1, #2015/25718-7, and #2017/05044-7), the National Council for Scientific and Technological Development (CNPq) (grant #458225/2014-2), and the Fundação Agrisus (Projects 1378/14 and 1387/14) for financial support. The authors also thank UNESP (Recém-Doutor) and CNPq for the grant awarded to CP and for an award for excellence in research awarded to CC, CACC, JC, and MA.
Adami, P. F., and Pitta, C. S. R. (2012). Pastagem E Bovinocultura De Leite. Curitiba: Instituto Federal do Paraná 80 (In Portuguese).
Agegnehu, G., Ghizaw, A., and Sinebo, W. (2006). Yield performance and land-use efficiency of barley and faba bean mixed cropping in Ethiopian highlands. Eur. J. Agron. 25, 202–207. doi: 10.1016/j.eja.2006.05.002
Almeida, D. S., and Rosolem, C. A. (2016). Ruzigrass grown in rotation with soybean increases soil labile phosphorus. Agron. J. 108, 2444–2452. doi: 10.2134/agronj2015.0478
Alvares, C. A., Stape, J. L., Serntelhas, P. C., Gonçalves, J. L. M., and Sparovek, G. (2013). Köppen's climate classification map for Brazil. Meteorol. Zeitschrift 22, 711–728. doi: 10.1127/0941-2948/2013/0507
Ambrosano, E. J., Tanaka, R. T., Mascarenhas, H. A. A., van Raij, B., Quaggio, J. A., and Cantarella, H. (1997). “Leguminosas e oleaginosas,” in Recomendações de adubação e calagem para o Estado de São Paulo. 2nd ed. eds B. van Raij, J. A. Quaggio, and H. Cantarella (Campinas. Agronômico (IAC)), 187–202
Araujo, L. C., Santos, P. M., Mendonça, F. C., and Lima, N. R. C. B. (2011). Development of maize and palisadegrass plants cultivated in intercrop under water deficit. Rev. Bras. Zootecn. 40, 1397–1404. doi: 10.1590/S1516-35982011000700001
Baldé, A. B., Scopel, E., Affholder, F., Corbeels, M. Da Silva, F. A. M., Xavier, J. H. V., et al. (2011). Agronomic performance of no-tillage relay intercropping with maize under smallholder conditions in Central Brazil. Field Crops Res. 124, 240–251. doi: 10.1016/j.fcr.2011.06.017
Bateman, E. J., and Baggs, E. M. (2005). Contributions of nitrification and denitrification to N2O emissions from soils at different water-filled pore space. Biol. Fert. Soils 41, 379–388. doi: 10.1007/s00374-005-0858-3
Bergamaschi, H., Dalmago, G. A., Bergonci, J. I., Bianchi, C. A. M., Müller, A. G., Comiran, F., et al. (2004). Water supply in the critical period of maize and the grain production. Pesqui. Agropecu. Bras. 39, 831–839. doi: 10.1590/S0100-204X2004000900001
Borghi, E., Crusciol, C. A. C., Mateus, G. P., Nascente, A. S., and Martins, P. O. (2013b). Intercropping time of corn and palisadegrass or guineagrass affecting grain yield and forage production. Crop Sci. 53, 629–636. doi: 10.2135/cropsci2012.08.0469
Borghi, E., Crusciol, C. A. C., Nascente, A. S., Souza, V. V., Martins, P. O., Mateus, G. P., et al. (2013a). Sorghum grain yield, forage biomass production and revenue as affected by intercropping time. Eur. J. Agron. 51, 130–139. doi: 10.1016/j.eja.2013.08.006
Brooker, R. W., Bennett, A. E., Cong, W. F., Daniell, T. J., George, T. S., Hallett, P. D., et al. (2015). Improving intercropping: a synthesis of research in agronomy, plant physiology and ecology. New Phytol. 206, 107–117. doi: 10.1111/nph.13132
Cantarella, H., van Raij, B., and Camargo, C. E. O. (1997). “Cereais,” in Boletim Técnico 100, Recomendação de Adubação e Calagem para o Estado de São Paulo, 2nd Edn, eds B. van Raij, H. Cantarella, J. A. Quaggio, and A. M. C. Furlani (Instituto Agronômico Campinas), 43–71. (In Portuguese)
Costa, N. R., Andreotti, M., Crusciol, C. A. C., Pariz, C. M., Lopes, K. S. M., Yokobatake, K. L. A., et al. (2016). Effect of intercropped tropical perennial grasses on the production of sorghum-based silage. Agron. J. 108, 2379–2390. doi: 10.2134/agronj2016.07.0385
Costa, N. R., Andreotti, M., Gameiro, R. A., Pariz, C. M., Buzetti, S., and Lopes, K. S. M. (2012). Nitrogen fertilization in the intercropping of corn with two Brachiaria species in a no-tillage system. Pesqui. Agropecu. Bras. 47, 1038–1047. doi: 10.1590/S0100-204X2012000800003
Costa, N. R., Crusciol, C. A. C., Trivelin, P. C. O., Pariz, C. M., Costa, C., Castilhos, A. M., et al. (2021). Recovery of 15N fertilizer in intercropped maize, grass and legume and residual effect in black oat under tropical conditions. Agric. Ecosyst. Environ.
Crusciol, C. A. C., Marques, R. R., Carmeis Filho, A. C. A., Soratto, R. P., Costa, C. H. M., Ferrari Neto, J., et al. (2016). Annual crop rotation of tropical pastures with no-till soil as affected by lime surface application. Eur. J. Agron. 80, 88–104. doi: 10.1016/j.eja.2016.07.002
Crusciol, C. A. C., Mateus, G. P., Nascente, A. S., Martins, P. O., Borghi, E., and Pariz, C. M. (2012). An innovative crop-forage intercrop system: early cycle soybean cultivars and palisadegrass. Agron. J. 104, 1085–1095. doi: 10.2134/agronj2012.0002
Crusciol, C. A. C., Nascente, A. S., Mateus, G. P., Borghi, E., Leles, E. P., and Santos, N. C. B. (2013). Effect of intercropping on yields of corn with different relative maturities and palisadegrass. Agron. J. 105, 599–606. doi: 10.2134/agronj2012.0426
Crusciol, C. A. C., Nascente, A. S., Mateus, G. P., Pariz, C. M., Martins, P. O., and Borghi, E. (2014). Intercropping soybean and palisadegrass for enhanced land use efficiency and revenue in a no till system. Eur. J. Agron. 58, 53–62. doi: 10.1016/j.eja.2014.05.001
Embrapa—Empresa Brasileira de Pesquisa Agropecuária (2006). Centro Nacional de Pesquisa de soja. Londrina: Tecnologias de produção de soja (In Portuguese,)
FAO–Food and Agriculture Organization of the United Nations (2006). World Reference Base for Soil Resources 2006: A Framework for International Classification, Correlation and Communication. Rome: WorldSoil Resources Reports No. 103 Available at: http://www.fao.org/3/a-a0510e.pdf (accessed November 01, 2017).
Fehr, W. R., and Caviness, C. E. (1977). Stages of Soybean Development. Ames, IA: Iowa State University.
Franzluebbers, A. J., and Stuedemann, J. A. (2014). Crop and cattle production responses to tillage and cover crop management in an integrated crop–livestock system in the southeastern USA. Eur. J. Agron. 57, 62–70. doi: 10.1016/j.eja.2013.05.009
Garcia, C. M. P., Costa, C., Andreotti, M., Meirelles, P. R. L., Pariz, C. M., Freitas, L. A., et al. (2016). Wet and dry corn yield under intercrop culivation with marandu grass and/or dwarf pigeon pea and nutritional value of the marandu grass in succession. Austral. J. Crop Sci. 10, 1564–1571. doi: 10.21475/ajcs.2016.10.11.PNE183
Garcia, R. A., Crusciol, C. A. C., Calonego, J. C., and Rosolem, C. A. (2008). Potassium cycling in a corn-brachiaria cropping system. Eur. J. Agron. 28, 579–585. doi: 10.1016/j.eja.2008.01.002
Hauggaard-Nielsen, H., and Jensen, E. S. (2005). Facilitative root interactions in intercrops. Plant Soil 274, 237–250. doi: 10.1007/1-4020-4099-7_13
Leonel, F. P., Pereira, J. C., Costa, M. G., De Marco, P., Júnior Lara, L. A., Sousa, D. P., et al. (2008). Intercropping of signal grass and soybean: productive performance of the crops and nutritional characteristics and quality of silage. Rev. Bras. Zootecnia 37, 2031–2040. doi: 10.1590/S1516-35982008001100020
Lopes, M. L. T., Carvalho, P. C. F., Anghinoni, I., Santos, D. T., Kuss, F., Freitas, F. K., et al. (2008). Crop-livestock integration system: performance and carcass quality of superprecoce beef steers finished in oat and ryegrass pasture managed under different heights. Cienc. Rural 38, 178–184. doi: 10.1590/S0103-84782008000100029
Lüscher, A., and Aeschlimann, U. (2006). “Effects of elevated [CO2] and N fertilization on interspecific interactions in termperate grassland model ecosystems,” in Managed ecosystems and CO2 case studies, processes and perspectives. eds J. Nösberger, S. P. Long, R. J. Norby, M. Stitt, G. R. Hendrey, and H. Blum (Berlin: Springer), 337–350. doi: 10.1007/3-540-31237-4_19
Malavolta, E., Vitti, G. C., and Oliveira, S. A. (1997). Avaliação do estado nutricional das plantas: princípios e aplicações. 2nd Edn. Piracicaba: POTAFOS.
Mateus, G. P., Crusciol, C. A. C., and Borghi, E. (2012). Integrated crop-livestock: the new green revolution in the tropics. Pesqui. Tecnol. 4, 1–5. (In Portuguese).
Mateus, G. P., Crusciol, C. A. C., Pariz, C. M., Borghi, E., Costa, C., Martello, J. M., et al. (2016). Sidedress nitrogen application rates to sorghum intercropped with tropical perennial grasses. Agron J. 108, 433–447. doi: 10.2134/agronj2015.0236
Mead, R., and Willey, R. W. (1980). The concept of ‘Land Equivalent Ratio' and advantages in yields from intercropping. Exp. Agric. 16, 217–228. doi: 10.1017/S0014479700010978
Monteiro, F. A., Ramos, A. K. B., Carvalho, D. D., Abreu, J. B. R., Daiub, J. A. S., Silva, J. E. P., et al. (1995). Growth of Brachiaria brizantha Stapf. cv. Marandu in nutrient solution with macronutrient omissions. Sci. Agr. 52, 135–141. doi: 10.1590/S0103-90161995000100022
Moraes, A., Carvalho, P. C. F., Anghinoni, I., Lustosa, S. B. C., Costa, S. E. V. G. A., and Kunrath, T. R. (2014b). Integrated crop-livestock systems in the Brazilian subtropics. Eur. J. Agron. 57, 4–9. doi: 10.1016/j.eja.2013.10.004
Moraes, A., Carvalho, P. C. F., Crusciol, C. A. C., Lang, C. R., Pariz, C. M., Deiss, L., et al. (2019). Integrated crop-livestock systems as a solution facing the destruction of Pampa and Cerrado biomes in South America by intensive monoculture systems. Agroecosyst. Div. 1, 257–273. doi: 10.1016/B978-0-12-811050-8.00016-9
Moraes, A., Carvalho, P. C. F., Lustosa, S. B. C., Lang, C. R., and Deiss, L. (2014a). Research on integrated crop-livestock systems in Brazil. Rev. Ciênc. Agron. 45, 1024–1031. doi: 10.1590/S1806-66902014000500018
Myaka, F. M., Sakala, W. D., Adu-Gyamfi, J. J., Kamalongo, D., Ngwira, N., Odgaard, R., et al. (2006). Yields and accumulation of N and P in farmer-managed intercrops of maize–pigeonpea in semi-arid Africa. Plant Soil 285, 207–220. doi: 10.1007/s11104-006-9006-6
Ndungu-Magiroi, K. W., Wortmann, C. S., Kibunja, C., Senkoro, C., Mwangi, T. J. K., Wamae, D., et al. (2017). Maize-bean intercrop response to nutrient application relative to maize sole crop response. Nutr. Cycl. Agroecosyst. 109, 17–27. doi: 10.1007/s10705-017-9862-x
Oliveira, P., Kluthcouski, J., Favarin, J. L., and Santos, D. C. (2011). Maize intercropped with palisadegrass and pigeon pea in partial desiccation system. Pesqu. Agropecu. Bras. 46, 1184–1192. doi: 10.1590/S0100-204X2011001000010
Pariz, C. M., Andreotti, M., Buzetti, S., Bergamaschine, A. F., Ulian, N. A., Furlan, L. C., et al. (2011b). Straw decomposition of nitrogen-fertilized grasses after intercropping with corn crop in irrigated integrated crop-livestock system. R. Bras. Ci. Solo. 35, 2029–2037. doi: 10.1590/S0100-06832011000600019
Pariz, C. M., Carvalho, M. P., Chioderoli, C. A., Nakayama, F. T., Andreotti, M., and Montanari, R. (2011a). Spatial variability of forage yield and soil physical attributes of a Brachiaria decumbens pasture in the Brazilian Cerrado. Rev. Bras. Zootec. 40, 2111–2120. doi: 10.1590/S1516-35982011001000007
Pariz, C. M., Costa, C., Crusciol, C. A. C., Castilhos, A. M., Meirelles, P. R. L., Roça, R. O., et al. (2017b). Lamb production responses to grass grazing in a companion crop system with corn silage and oversowing of yellow oat in a tropical region. Agric. Syst. 151, 1–11. doi: 10.1016/j.agsy.2016.11.004
Pariz, C. M., Costa, C., Crusciol, C. A. C., Meirelles, P. R. L., Castilhos, A. M., Andreotti, M., et al. (2016). Production and soil responses to intercropping of forage grasses with corn and soybean silage. Agron. J. 108, 2541–2553. doi: 10.2134/agronj2016.02.0082
Pariz, C. M., Costa, C., Crusciol, C. A. C., Meirelles, P. R. L., Castilhos, A. M., Andreotti, M., et al. (2017a). Production, nutrient cycling and soil compaction to grazing of grass companion cropping with corn and soybean. Nutr. Cycl. Agroecosyst. 108, 35–54. doi: 10.1007/s10705-016-9821-y
Pariz, C. M., Costa, C., Crusciol, C. A. C., Meirelles, P. R. L., Castilhos, A. M., Andreotti, M., et al. (2017c). Silage production of corn intercropped with tropical forages in an integrated crop-livestock system with lambs. Pesqu. Agropecu. Bras. 52, 54–62. doi: 10.1590/s0100-204x2017000100007
Pereira, F. C. B. L., Mello, L. M. M., Pariz, C. M., Mendonça, V. Z., Yano, E. H., Miranda, E. E. V., et al. (2016). Autumn maize intercropped with tropical forages: crop residues, nutrient cycling, subsequent soybean and soil quality. R. Bras. Ci. Solo. 40:e0150003. doi: 10.1590/18069657rbcs20150003
Rosolem, C. A., and Steiner, F. (2017). Effects of soil texture and rates of K input on potassium balance in tropical soil. Eur. J. Soil Sci. 68, 658–666. doi: 10.1111/ejss.12460
Sá, J. C. M., Cerri, C. C., Lal, R., Dick, W. A., Piccolo, M. C., and Feigl, B. E. (2009). Soil organic carbon and fertility interactions affected by a tillage chronosequence in a Brazilian Oxisol. Soil Till. Res. 104, 56–64. doi: 10.1016/j.still.2008.11.007
Senkoro, C. J., Marandu, A. E., Ley, G. J., and Wortmann, C. S. (2017). Maize and pigeon pea sole crop and intercrop nutrient response functions for Tanzania. Nutr. Cycl. Agroecosyst. 109, 303–314. doi: 10.1007/s10705-017-9889-z
Shapiro, S. S., and Wilk, M. B. (1965). An analysis of variance test for normality (complete samples). Biometrika 52, 591–611. doi: 10.1093/biomet/52.3-4.591
Silva, D. J., and Queiroz, A. C. (2002). Análise de Alimentos: Métodos Químicos e Biológicos. 3rd Edn. Viçosa: UFV, 235. (In Portuguese).
Sprent, J. I. (2007). Evolving ideas of legume evolution and diversity: a taxonomic perspective on the occurrence of nodulation. New Phytol. 174, 11–25. doi: 10.1111/j.1469-8137.2007.02015.x
Takim, F. O. (2012). Advantages of maize-cowpea intercropping over sole cropping through competition indices. J. Agr. Biod. Res. 1, 53–59.
van Raij, B., Andrade, J. C., Cantarella, H., and Quaggio, J. A. (2001). Análise química para avaliação da fertilidade de solos tropicais. Campinas: IAC Campinas 284. (In Portuguese).
Wider, R. K., and Lang, G. E. (1982). A critique of the analytical methods used in examining decomposition data obtained from litter bags. Ecology 63, 1636–1642. doi: 10.2307/1940104
Wirsenius, S., Azar, C., and Berndes, G. (2010). How much land is needed for global food production under scenarios of dietary chances and livestock productivity increases in 2030? Agric. Syst. 103, 621–638. doi: 10.1016/j.agsy.2010.07.005
Xiao, Y., Li, L., and Zhang, F. (2004). Effect of root contact on interspecific competition and N transfer between wheat and faba bean using direct and indirect 15N techniques. Plant Soil 262, 45–54. doi: 10.1023/B:PLSO.0000037019.34719.0d
Yokomizo, G. K., Duarte, J. B., and Vello, N. A. (2000). Phenotypic correlation between seed size and other characteristics in top crosses of vegetable soybean with grain type. Pesqu. Agropecu. Bras. 35, 2235–2241. doi: 10.1590/S0100-204X2000001100016
Keywords: Cajanus cajan, integrated crop-livestock systems, Urochloa brizantha, Zea mays, land use efficiency
Citation: Pariz CM, Costa NR, Costa C, Crusciol CAC, de Castilhos AM, Meirelles PRdL, Calonego JC, Andreotti M, Souza DMd, Cruz IV, Longhini VZ, Protes VM, Sarto JRW, Piza MLSdT, Melo VFdP, Sereia RC, Fachiolli DF, Almeida FAd, Souza LGMd and Franzluebbers AJ (2020) An Innovative Corn to Silage-Grass-Legume Intercropping System With Oversown Black Oat and Soybean to Silage in Succession for the Improvement of Nutrient Cycling. Front. Sustain. Food Syst. 4:544996. doi: 10.3389/fsufs.2020.544996
Received: 23 March 2020; Accepted: 28 October 2020;
Published: 24 November 2020.
Edited by:
Paulo Mazzafera, Campinas State University, BrazilReviewed by:
Agnieszka Barbara Najda, University of Life Sciences of Lublin, PolandCopyright © 2020 Pariz, Costa, Costa, Crusciol, de Castilhos, Meirelles, Calonego, Andreotti, Souza, Cruz, Longhini, Protes, Sarto, Piza, Melo, Sereia, Fachiolli, Almeida, Souza and Franzluebbers. This is an open-access article distributed under the terms of the Creative Commons Attribution License (CC BY). The use, distribution or reproduction in other forums is permitted, provided the original author(s) and the copyright owner(s) are credited and that the original publication in this journal is cited, in accordance with accepted academic practice. No use, distribution or reproduction is permitted which does not comply with these terms.
*Correspondence: Cristiano Magalhães Pariz, Y21wem9vQGdtYWlsLmNvbQ==
Disclaimer: All claims expressed in this article are solely those of the authors and do not necessarily represent those of their affiliated organizations, or those of the publisher, the editors and the reviewers. Any product that may be evaluated in this article or claim that may be made by its manufacturer is not guaranteed or endorsed by the publisher.
Research integrity at Frontiers
Learn more about the work of our research integrity team to safeguard the quality of each article we publish.