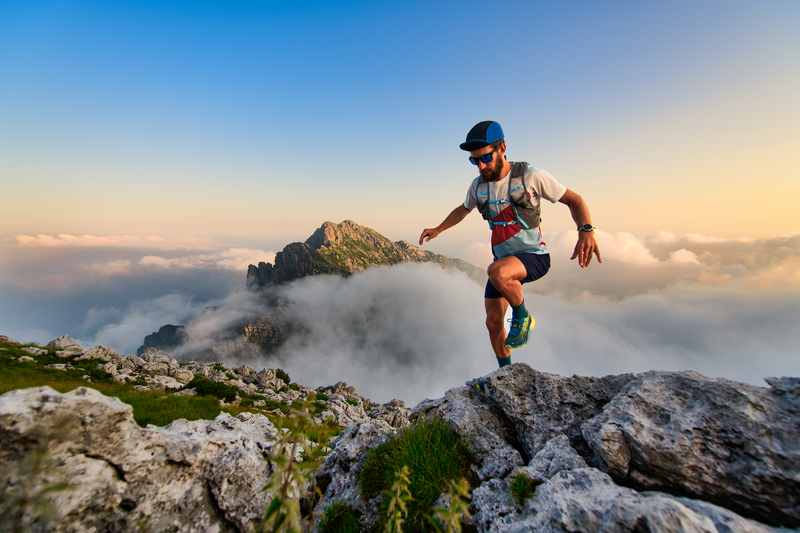
94% of researchers rate our articles as excellent or good
Learn more about the work of our research integrity team to safeguard the quality of each article we publish.
Find out more
REVIEW article
Front. Sustain. Food Syst. , 23 October 2020
Sec. Agroecology and Ecosystem Services
Volume 4 - 2020 | https://doi.org/10.3389/fsufs.2020.543403
This article is part of the Research Topic Grazing in Future Multi-scapes: From Thoughtscapes to Landscapes, Creating Health from the Ground Up View all 46 articles
The industrialization of agriculture based on inexpensive fossil fuels allowed for unprecedented levels of food production and population growth, but simultaneously contributed to a threat to food systems and population well-being: climate change. This paper analyses the impacts and adaptations available to the world's pastoral production systems including potential mitigation and adaptation strategies. The current food system is under pressure to satisfy the needs of the increasing population with already limited natural resources particularly land and water, and now increasingly under pressure due to climate change. Increasing incomes from greater number of people have increased demand for animal source foods. As a result, domestic livestock numbers are rising, particularly in low-income countries with greater dependence on pasture for animal feed. The carrying capacity of pastured land is limited; therefore, increasing animal numbers may cause environmental degradation and loss of productivity from pastoral industries. In some countries, the increasing demand has prompted some to burn forestlands and convert them into crop production, and after the land is degraded into pasture mainly for raising large ruminants. Changes in atmospheric carbon dioxide levels and ambient temperatures are predicted to make a number of changes to the growth of herbage for animals. Several strategies can be implemented to optimize the pasture-based food system, including choosing the right breed, improving reproductive efficiency, improving grazing management, and maintaining an animal feed base. The global challenge is to meet our food production needs while sustaining our environment. Producers in all income categories have a role to play in adapting to these challenges.
Pastoralism, herding of large animals as a means of livelihood, is one of the oldest viable and potentially sustainable systems, if properly managed [International Fund for Agricultural Development (IFAD), 2008; Dyer, 2016]. Pastoralism exists in about 100 countries across all continents except Antarctica [Food and Agriculture Organization of the United Nations (FAO, 2001)]. Pastoral livestock systems utilize 25% of terrestrial land on earth (mostly drylands where conventional farming is limited or not feasible) producing about 10% of meat consumed worldwide and supporting 200 million households (Blench, 2001). Pastoralism has a huge impact on the economies of several countries especially in Africa and Central Asia. For instance, in Africa pastoralism contributes 10 to 44% of gross domestic product (African Union, 2010). In addition to economic importance, pastoralism occurs in socio-cultural hotspots (e.g., world heritage sites), provides ecosystem services (e.g., maintaining and even enhancing rangeland biodiversity), and maintains ecological integrity (e.g., act as a carbon sink) (Stolton et al., 2019).
Pastoralism has considerable economic ecological, and socio-cultural importance (summarized in Figure 1). Pastoralists make their livelihoods in some of the harshest of conditions and unpredictable climatic conditions, however, they face challenges due to demographic, economic, political and climatic changes (Dong et al., 2016). Since the industrial revolution, the concentration of greenhouse gases (GHG) in the atmosphere have increased resulting in global land surface temperature rise, sea level rise, and drought in some areas (e.g., West Asia, North-Eastern Asia). On the other hand, climate change has increased precipitation, frequency and intensity of floods, storms and wildfires, and emergence of new diseases for crops, livestock and humans [Intergovernmental Panel on Climate Change (IPCC), 2019]. Climate change is negatively affecting food security particularly in pastoral systems of African drylands and mountainous regions of Asia and South America either directly or indirectly affecting both crop (e.g., increasing or decreasing yield of some crops depending on latitudes) and livestock (e.g., lower growth and production of pastoral livestock system) production (IPCC, 2019). Logically, pastoral livestock production is exacerbating climate change because agriculture (including forestry and land use) and livestock production represent 23 and 14.5% of total anthropogenic GHG emissions, respectively (Gerber et al., 2013; IPCC, 2019). Nevertheless, pastoral system can be a sink of GHG if it is well-managed without modifying landscape and natural ecosystems (e.g., clearing forests or modification of natural ecosystems). Additionally, global population is predicted to exceed 9.7 billion by 2050 (UN, 2019). Most of the population growth will occur in low-income countries primarily in Africa and Asia. This increment in global population along with increasing living standard and incomes is increasing global food demand. For instance, milk and meat demand will increased about 60% by 2050 (Brian, 2015). This will not only force intensification of crop and livestock production but will increase climate change risk due to land use changes and increasing total GHG emissions. Thus, it is of utmost importance to understand the two-way interactions between climate change and pastoral livestock production systems. The objective of this review is to (1) summarize and critically analyze the current literature focusing on the impact of food production and climate change on the pastoral livestock industry at a global scale, and (2) discuss the potential mitigation and adaptation strategies of climate change risks for pastoral industries.
Figure 1. Benefits of pastoralism (adapted from Blench, 2001; and Nyariki and Amwata, 2019).
Pastoralism, found mainly in low-income countries and sometimes in isolated less developed regions of high-income countries, is a form of livestock production where human activity and nature affect each other. Pastoralism is the caring and herding of large animals in dryland areas when looked at through a production perspective (Dong et al., 2016). From a livelihood perspective, pastoralism is the subsistence and successful living through herding livestock in less productive lands (IFAD, 2008). “Pastoral system,” an alternative term for pastoralism, is the livestock production system where grazing of animals is the major land use. There are at least five different forms of pastoralism depending on herd mobility pattern, resource use and income generated from livestock and livestock products (Table 1; Dong et al., 2016). Nomadic and transhumant pastoralism are the most common forms. Nomadism is practiced mainly in dry and high non-arable lands with low rainfall and harsh climatic conditions. There are about 30 to 40 million nomads living mainly in Mongolia, Russia, China, Eastern Europe and Central Asia. Nomads live in tents and move between 10 and 1,000 km annually. Transhumant pastoralists live in permanent homes in all pastoral regions across continents and move their animals between summer and winter pastures annually. Vertical transhumance is typically practiced in mountainous regions (e.g., Bavaria in Austria, Swiss Alps), while horizontal transhumances is practiced in plain land areas (e.g., Mongolia). Horizontal pastoralism is more vulnerable to climatic or socio-economic changes than vertical pastoralism (Dong et al., 2016). Agro-pastoralism is a form of pastoralism in which most of the family income is generated from cultivating crops compared to pastoralists that generate more than 50% of family income from livestock and livestock products (IFAD, 2008). Although the focus of this review is nomadic and transhumant pastoralism, the review briefly discusses agro-pastoralism and silvo-pastoralism due to its dominance and importance for certain regions such as South-Asia and Latin America. Currently, pastoralism predominantly exists in Sub-Saharan Africa, Central Asia (including Himalayas), and Australia, some part of Northern Europe, Central South America and Western North America. Although distribution of livestock species depends on geographic location, climatic condition and history of pastoralism, the most common species of pastoral livestock at the global scale are cattle, goats and sheep. Nevertheless, roughly 15 different livestock species are found in different pastoral forms and geographic regions (Table 2).
Table 1. Forms of pastoralism (adapted from Blench, 2001).
Table 2. Geographical distribution of pastoral livestock species across continents (adapted from Blench, 2001).
Anthropogenic GHG emissions have now reached the highest levels in recent history with clear evidence of human influence on climate change (IPCC, 2019). At current global food demand, about 25% anthropogenic GHG emissions arise from food production whereas agriculture accounts for 70% of freshwater use globally (IPCC, 2019). However, compared to intensive systems, pastoral livestock production systems utilize less water due to less reliance on cultivated feeds and fodders, resulting in less fossil fuel use (Steinfeld et al., 2010). Pastoral systems produce animal foods using forages and thus utilize much less human-edible protein compared to intensive production systems (Steinfeld, 2012). In 2050, the global demand for crop production is projected to increase by 100 to 110% (Tilman et al., 2011) and meat and milk demand will increase by 60% (Brian, 2015). Food demand will increase most in low-income countries due to increasing middle class and living standard (Figure 2). To meet increasing food demand, an additional 1 billion ha of land will be deforested with added agricultural GHG emissions of 3 Gt carbon dioxide equivalent (CO2-e) per annum by 2050 (Tilman et al., 2011). The increased GHG emissions resulting in extreme climate events will negatively affect the pastoral industry by directly or indirectly affecting both crops and livestock production (IPCC, 2019).
Figure 2. Changing per capita and total milk and meat consumption overtime in developing and developed countries (adapted from FAO, 2006).
Total anthropogenic GHG emissions are still increasing despite mitigation measures. In 2010, they reached 49 Gt CO2-e comprising 76% CO2, 16% methane (CH4), 6.2% nitrous oxide (N2O), and 2% fluorinated gases (IPCC, 2014). Combustion of fossil fuels and industrial processes are the main sources of CO2, whereas agriculture is one of the main contributors to non-CO2 GHG emissions. The IPCC (2019) special report outlines that increasing global GHG emissions increased land surface and ocean temperatures. The rise in land surface air temperature ranged from 1.38 to 1.68°C. Although rising temperatures could have stronger positive impact on biomass yields of C4 than C3 grass (Reeves et al., 2014), the impact of temperature on pastureland will depend on level of precipitation (Izaurralde et al., 2011). This warming climate has subsequently increased the frequency and intensity of drought in some regions and heavy precipitation in other regions. Increased air temperature along with decreased precipitation has amplified desertification in some areas such as Sub-Saharan Africa. Increased precipitation could increase productivity of pastureland, but drought could reduce both quantity and quality of pasture (Humphreys, 1991). Global warming will affect the distribution, abundance, and seasonality of plants and animal species. Warming climate has increased the likelihood of new disease outbreaks and infestations for plants, animals and humans. Several other consequences of global warming include flooding, melting ice, sea-level rise, wind and heat stress, which might have direct or indirect negative effects on plant and animal species. For instance, heat stress negatively effects the performance of pastoral livestock (Reyad et al., 2016), whereas flooding would make pastoral livestock systems more vulnerable mainly due to creating feed crisis and increasing morbidity and mortality of livestock (Hoffmann, 2010).
The pastoral industry is having both negative and positive impacts on climate change and ecosystems, whereas climate change is also affecting the pastoral industry. This two-way interaction between climate change and the pastoral industry is summarized in Figure 3.
Figure 3. Impact of food production and climate change on pastoralism and vice-versa (adapted from Thornton et al., 2009; Golub et al., 2013 and Sejian et al., 2018).
The livestock sector accounts for ~19, 15, and 1.35% of global anthropogenic CH4, N2O, and CO2 emissions (Rojas-Downing et al., 2017). Data about the impacts of the pastoral industry on climate change are not available. However, the intensity of the carbon footprint of livestock products is much greater for low input extensive systems than intensive production systems due to low productivity of animals in the former systems. For example, in 2015 the carbon footprint of milk production in Sub-Saharan Africa, South Asia, Western Europe and North America were 6.66, 4.10, 1.37, and 1.29 kg CO2-e per kg fat and-protein corrected milk, respectively (FAO, 2018a). Thus, producing the same amount of milk from extensive system of Sub-Saharan Africa and South Asia would have much greater impact on climate change compared to the intensive systems of Western Europe and North America.
Among common food protein sources beef cattle have the largest GHG emissions and land use intensity (Swain et al., 2018), though that varies widely across production systems. For instance, the carbon footprint for beef production ranges from 12 to 129 kg CO2-e per kg beef in extensive systems compared to 9 to 42 kg CO2-e per kg beef in intensive systems (Nijdam et al., 2012). Similarly, land required to produce a kilogram of beef ranges from 286 to 420 m2 in extensive systems and 15 to 29 m2 in intensive systems (Nijdam et al., 2012). Specifically, intensive (grain-fed) beef systems require 45% less land and emit 30% less GHG than grass-fed beef systems to produce a kilogram beef (Capper, 2012). However, pastoral systems have the potential to increase soil organic carbon (SOC) stock over time, which is often overlooked due to the lack of data. A meta-analysis based on 42 long-duration studies (3 to 83 years, with an average of 18 years) showed that manure applied to soil had significantly greater SOC compared to soil without added manure or soil with chemical fertilizer applied (Maillard and Angers, 2014). Additionally, in pastoral systems, manure is naturally recycled back to land rather than managed in lagoons which produce substantial GHG in intensive systems (Aguirre-Villegas and Larson, 2017).
Increased food demand has increased livestock populations globally. For example, buffalo, cattle and goat populations have increased ~2.2, 1.6, and 2.9 times between 1961 and 2016 (FAOSTAT, 2016). This increment in the livestock population has led to the expansion of pastureland by 20 and 33% in Asia and Latin America, respectively (FAOSTAT, 2017). Currently, 33.4 million km2 of land used as grazing land (including pasturelands, rangelands, grasslands, savannas, shrublands and steppes) which cover ~25% of the earth's surface (FAOSTAT, 2014). Grazing ruminant animals is one of the major drivers for pastureland expansion leading to deforestation particularly in the Brazilian Amazon as most of the deforested land ends up being used for cattle grazing (Barona et al., 2010; Schielein and Börner, 2018). Further increments in demand for animal source-food will reinforce pastureland expansion. Overgrazing by cattle can stimulate soil degradation and negatively affect freshwater and terrestrial ecosystems. Overgrazing resulting in reduction of vegetation cover is one of the major causes of soil erosion (Kairis et al., 2015). Overgrazing is a function of increasing stocking density. For example, stocking density increased from 1.3 to 1.7 animal units between 2003 and 2015 in the Brazilian Amazon region (Schielein and Börner, 2018). Blue water footprint for beef production in grain-fed system of United States is 2,034 L per kg carcass weight where more than 90% of the water is used for irrigation to produce feed (Rotz et al., 2019). However, properly managed grazing can enhance plant cover and soil health, which is discussed in the climate change adaptation section.
A decline in biomass yield and fluctuations in biomass availability of pastureland determine the extent and magnitude of climate change risk for pastoral livestock production (Godde et al., 2020). Global scale projection between 2000 and 2050 shows that 74% of rangeland might experience a decline in biomass yield whereas 64 and 54% rangelands might experience inter-annual (year to year) and intra-annual (month to month) fluctuations of biomass availability, respectively. These changes might pose a serious threat to about 174 million ruminants particularly in the tropical region of Sahel, Australia, Mongolia, China and Uzbekistan (Godde et al., 2020). Additionally, pastures in tropical Australia and Sub-Saharan Africa are poor in quality (e.g., low protein content and low digestibility), mainly during the dry season, due to poor soil nutrient profiles, which might be worsened with frequent and longer droughts (Humphreys, 1991).
Furthermore, a shift from herbaceous pasture to shrubs and trees is projected to happen on 51% of rangeland areas globally (Godde et al., 2020). This shift from herbs to shrubs and trees has important implications for pastoral production systems because herders need to couple livestock species with vegetation changes. For example, woody pastureland would be suitable for goats but not as suitable for cattle or sheep.
Elevated CO2, rising temperature and reduced precipitation could affect growth of forage species (Table 3). Increased CO2 and precipitation will increase net primary production of rangeland and pastureland species, but the impact of temperature rise on net primary production is uncertain due mainly to the uncertainty of predicting precipitation (Izaurralde et al., 2011). Impacts of climate change also depend on the metabolic pathways (e.g., C3 vs. C4) of pastureland grass species. For example, rising temperatures would have the greatest positive effect on the growth of warm season C4 grass whereas rise in CO2 concentrations would mainly affect cool season C3 grass (Reeves et al., 2014). In some regions (e.g., East and West Africa), increased population and land competition led people to covert pastureland into cropland, which reduced communal pastureland (Godde et al., 2020). Feeding crop residues could be a way to cope with this land competition.
Weed species showed a relatively strong response to elevated CO2 which could enhance growth and make them herbicide resistant (Ziska, 2003). This will increase competition between weed and crops resulting in greater loss of crop production. Competition will be exacerbated by rising temperatures because growth of most weeds (mostly C4 metabolic pathway) will be stimulated (Ramesh et al., 2017). Patterson et al. (1979) showed that a 3°C increase in temperature could potentially increase the biomass yield of itchgrass by 80%. Some weed species such as cheatgrass and yellow star thistle can grow faster with reduced precipitation due to their adaptability to drought (Hatfield et al., 2011). Weed infestation and growth also depend on crop species, therefore, all combinations of weed and crops (or forage) interactions (e.g., C3 weed and crop, C4 weed and crop, C4 weed and C3 crop and any other combinations) need to be examined (Ramesh et al., 2017).
Plant pests (insect and disease) cause major losses in crop yields, which could be aggravated by climate change. Foodtank (2019) summarized the potential impact of pests on crop yield losses under changing climate. Pests could reduce crop yields annually by 20 to 40% (Flood, 2010). Global crop yield losses will further increase between 10 and 25% for each degree of global surface temperature increase. Rising temperatures may affect distribution, severity of infestation and life cycles of cold-blooded pest species or they may reduce resistance of crop or forage species to disease. Climate change could create extremely severe food insecurity. For example, wheat blast caused by a fungus (Magnaporthe oryzae), a recently emerged wheat disease, poses a threat to tropical South America and South Asia. The disease affected approximately 3 million hectares of wheat field in 1990 [International Maize and Wheat Improvement Center (CIMMYT), 2019]. Recently, the disease has spread in South Asia causing 51% yield reduction in infected areas (CIMMYT, 2019). This fungus has the ability to infect other grass species such as barley and rice. Furthermore, climate change might increase the vulnerability of crops susceptible to the disease or newly emerged diseases due to reduced disease resistance of host crop species.
In addition to forage growth and distribution, climate change will also affect forage quality. A meta-analysis by Dumont et al. (2015) showed that elevated CO2 increased non-structural carbohydrates by 25% but decreased nitrogen content by 8%. However, the increased abundance of legume species with increasing CO2 might compensate for the reduction in nitrogen. The impact of temperature rise on forage chemical composition or digestibility was inconsistent, and while drought increased digestibility, variability among studies was high.
Pastoral systems utilize and maintain livestock diversity to cope with climatic variability and keep production costs as low as possible (Kaufmann et al., 2016). Locally adapted livestock breeds are reared in pastoral systems because local breeds have greater fit with the local climatic conditions than exotic breeds (Provenza, 2008). Pastoralists use various livestock species determined mainly by their geographic location and climatic conditions as discussed earlier (Table 2). However, Hoffmann (2010) concluded that climate change will affect diversity of pastoral livestock in two ways. First, direct effect of rising temperature (e.g., heat stress) or extreme weather events (e.g., flood or storm) will increase the risk of morbidity and mortality of locally adapted breeds compared to exotic breeds because exotic breeds in the high-output intensive systems are well-protected from climatic adversaries due to better housing and management than local breeds in the grazing systems. Second, increasing food demand and climate change will push low input and extensive production systems to improve efficiency and reduce their environmental footprints. This intensification will remove most locally adapted livestock species or breed, which will not be cost-effective in high input systems.
The impacts of climate change will not be limited to crop production, but will also affect efficiency, product quality, production, and reproduction of livestock species. Heat stress, for example could cause huge economic losses in the dairy and beef industries. Severity of heat stress is lower in beef cattle than dairy cattle due to lower metabolic rate and heat production resulting in higher temperature-humidity index in beef cattle than dairy cattle (St-Pierre et al., 2003). Heat stress can reduce up to 25% of daily milk yield along with reduced feed intake when dairy cows are exposed to high temperature and humidity (Cowley et al., 2015; Summer et al., 2019). Reductions in feed intake can be used as an indication of heat stress. Intake reductions start at 25°C with 20 to 40% intake reductions when temperatures exceed 40°C (Hahn, 1999). Heat stress could reduce milk production by 19% under extensive tropical production systems, which have comparable levels of milk production to pastoral systems (Reyad et al., 2016). In terms of milk quality, Cowley et al. (2015) reported a decrease in milk protein content due to heat stress. The effect of heat stress on milk lactose content is not yet clear as some studies report a decrease, but others report no change (Summer et al., 2019). Heat stress in beef cattle causes high mortality and affects animal behavior such as increased respiration rate, decreased rumination and feed intake, and increased frequency of drinking water (Summer et al., 2019). Morignat et al. (2015) reported that each degree temperature increase above a certain threshold is associated with a 5% increase in mortality risk. Heat stress adversely affects follicle development and reduces pregnancy rate in cattle (Liu et al., 2019). An increase in temperature-humidity index from 72 to 78 reduced pregnancy rate from 39.4 to 31.6% in dairy cows (Domínguez et al., 2005). Nonetheless, the impact of heat stress depends on livestock species or breed or type. For example, tropical cattle breeds are more adaptive to heat stress than temperate breeds. At a similar level of heat stress exposure, tropical cattle had relatively less reduction of feed intake (25 vs. 30%), average daily gain (12 vs. 18%) and daily milk yield (20 vs. 28%) than temperate cattle (Johnson, 2018). This difference in heat stress tolerance between cattle breeds is paramount to select breed with greater adaptive capacity, which will be further discussed in adaptation section.
Overall, the agriculture sector consumes about 70% of total freshwater making this sector the largest user of freshwater (Thornton et al., 2009; IPCC, 2019). Although the livestock sector accounts for 8% of human water use globally (Nardone et al., 2010), pastoral systems mostly depends on rainfall with minimal consumption of ground and surface water compared with intensive livestock production systems. Water is essential for livestock production because water is used for drinking, and to produce feed and process products. Approximately 90% of water consumption in the livestock sector is associated with feed production (FAO, 2018b). Thus, increasing livestock populations to meet global food demand will increase water consumption significantly. In general, ruminants drink 3.5 to 5.5 L of water/kg dry matter intake depending on physiological status of the animal, feed water content and local climatic conditions. Increasing temperature will further increase drinking water consumption by 2- to 3-fold (Nardone et al., 2010). Water unavailability or contamination can affect livestock production and reproduction (Rojas-Downing et al., 2017). For example, contamination of freshwater with saline water due to sea level rise could affect metabolism and fertility of animals (Nardone et al., 2010). Dehydration or water deprivation increases body and rectal temperature but reduces respiration rate in small ruminants. However, respiration rate might be increased due to dehydration with rising environmental temperature. Water deprivation leads to body weight loss due mainly to reduced feed intake, which can be up to 60% in small ruminants. Between 25 and 50% water restriction reduced milk yields by 18 to 20% in small ruminants (Akinmoladun et al., 2019).
Climate change poses a threat to human and animal health due to either increasing severity or frequency of outbreaks for known diseases or emergence of new diseases. Rising temperature or increasing severity and frequency of extreme events could also affect animal health either directly or indirectly (Nardone et al., 2010; Bett et al., 2017). Rising temperature increases disease susceptibility and mortality of animals by suppressing immunity of host animals or favoring pathogens. Indirect effects of climate change on disease are associated with ecosystem changes or socio-cultural changes such as changes in life-style, which might increase vector-pathogen-host contact. Rising temperature has already changed parasitic disease occurrence by helminth (seasonality, abundance and spatial variation of spread) (Van Dijk et al., 2010). Climate change could affect animal health by affecting either vector or pathogens or host. Pathogens for anthrax, black quarter and helminths, which complete part of their life cycle outside a host, could be stimulated with temperature rise (Bett et al., 2017). For instance, growth of Bacillus anthracis spores (pathogen for anthrax) depend on temperature, humidity and nutrient availability of the environment (WHO, 2008). There is a lack of data on indirect effects of climate change on diseases (Bett et al., 2017). Furthermore, the association between climate change and disease occurrence is complex and further studies are required to unravel confounding between climate change parameters and other factors causing a disease. The potential and currently available climate change mitigation and adaptation strategies are discussed in the following two sections (Figure 4).
Mitigation of climate change aims to either reduce sources of GHG emissions or increase sinks of GHG (IPCC, 2014). Mitigation of emissions from the agricultural sector can be achieved either through reducing emissions per unit of food product or per unit of land (supply-side approach) or through reducing food demand, switching food choice, reducing avoidable food waste and improving access to food through proper distribution (demand-side approach) (IPCC, 2014).
Major emission sources for livestock products are enteric CH4, N2O, and CH4 from manure management during storage and after cropland-application, and CO2 and N2O emissions from feed production and transportation. Enteric emissions from ruminants could be reduced through: (1) nutritional manipulation (e.g., improving feed digestibility, replacing structural carbohydrates with non-structural carbohydrates in the diet) (Hristov et al., 2015); (2) supplementation of feed additives (e.g., feeding 3-nitrooxypropanol and red algae have great potential to reduce enteric emissions) (Roque et al., 2019); (3) genetic improvement (e.g., direct or indirect genetic selection for enteric CH4 reduction using advance technique such as genomic selection), and (4) improved herd management and fertility (Knapp et al., 2014). The enteric emission mitigation strategies might not be equally applicable and relevant for all pastoral production systems. For example, supplementation of concentrate feeds (i.e., source of non-structural carbohydrates) has great potential to reduce enteric CH4 either through improving production performance (i.e., dilution effect) or through acting as a sink of hydrogen in the rumen (Wattiaux et al., 2019). This strategy could be implemented in agro-pastoral systems. However, improving digestibility of natural pasture is challenging, but can be achieved to some degree through grazing management that helps to prevent maturation of plant species (see climate change adaptation strategies section below). Feed additives might be a challenge if they need to be given to animals on a daily basis. However, technologies can be developed in which feed additives can be given in a slow release form covering several days or weeks. Breeding programs focusing on improving productivity, fertility and adaptability of cattle in the pastoral system could also be implemented.
Reducing duration of manure storage, solid-liquid separation and anaerobic digestion are potential strategies to reduce emissions from manure storage, whereas feed production related emissions could be reduced by altering the amount and improving the timing and application of chemical fertilizer and manure to crop-fields (Aguirre-Villegas and Larson, 2017; Wattiaux et al., 2019). Most of these techniques are not necessary for pastoral systems because manure is recycled naturally.
A recent study showed that environmental impact of producing the same product can vary by 50-fold among producers (Poore and Nemecek, 2018). This variation among producers provides the potential to reduce environmental impact of food products by improving efficiency (e.g., feed efficiency for livestock production, crop yield per unit of N fertilizer) and by adopting best management practices (Clark and Tilman, 2017). Based on life cycle analysis, the carbon footprint for feedlot beef was much lower compared to grass-fed beef (6.0 vs. 9.6 kg CO2-e per kg carcass weight) due to greater productivity of feedlot beef production (Stanley et al., 2018). However, this comparison did not take into account SOC change. When SOC change was taken into account the carbon footprint for feedlot beef was greater than grass-fed beef (6.1 vs. −6.6 kg CO2-e per kg carcass weight) due to carbon sequestration in grass-fed beef systems (Stanley et al., 2018). Although pastoral management systems cannot be fully compared with grass-fed beef system it is important to evaluate these systems by taking into account all components within the system including accounting for SOC change and carbon sequestration.
Dietary choices can impact GHG emissions due to wide differences in emission intensity among food sources. For instance, the carbon footprint of ruminant meat is 3 to 10 times greater than other animal-source foods such as milk, dairy products, chicken, and 20 to 200 times greater than plant-based foods such as cereals and pulses (Clark and Tilman, 2017). Thus, diets composed of plant-based food could potentially reduce food production related GHG emissions by up to 50% (Poore and Nemecek, 2018). When the impacts of global population growth are combined with income-dependent dietary changes, the net effect would be 80% increase in food production related GHG emissions by 2050 compared to dietary trend of 2009, however, food production emissions would show no net increase if by 2050 the global diet became an average of the Vegetarian (combination of fruits, vegetables, grains, sugars, oils, eggs, and dairy with a single monthly serving of meat or seafood), Pescetarian (vegetarian plus seafood) and Mediterranean (Pescetarian plus modest amount of poultry, pork, lamb and beef) diets (Tilman and Clark, 2014). While diets composed of processed foods containing high sugars, fats and carbohydrates might help to reduce GHG emissions, they will not improve human health (Tilman and Clark, 2014). Myriad of factors, including acquired cultural preferences, accessibility, price, and nutritional needs and knowledge of consumers, influence people's dietary choices (Tilman and Clark, 2014). Therefore, dietary solution should consider the cultural and physical environments in tandem with human health. Any comparisons between animal- and plant-source foods also needs to consider the nutritional value of the products relative to the needs of the consumer. For instance, the environmental footprint of most animal- and plant-source foods are similar when comparisons account for the higher biological value of animal proteins (Tessari et al., 2016). Furthermore, livestock products produced under managed grazing systems have the potential to improve soil health, increase carbon sequestration and reduce water pollution (Derner and Schuman, 2007; Eisler et al., 2014; Teague et al., 2016; Stanley et al., 2018).
Supply chain (harvest to consumption) food loss including food waste accounts for roughly 30 to 40% of food loss (Godfray et al., 2010). The extent of food losses is almost the same between high, mid and low-income countries. However, in low-income countries most losses happen on-farm due to poor storage, distribution, conservation and processing whereas in high-income countries most food losses happen at the consumer level due to food waste (Godfray et al., 2010). Most food losses are avoidable, however, some losses cannot be avoided, such as those from peeling some fruits and vegetables. Avoiding food loss can have profound impact on GHG reductions from food production. Life cycle analysis conducted in the United States showed that the carbon footprint of milk can be reduced by 23% through avoiding 12% milk loss at retail or an additional 20% loss at consumer levels due to cooking loss, spoilage and waste (Thoma et al., 2013).
Although water scarcity is a regional issue, it needs to be addressed to meet the future water demand, which is expected to increase by 50% (UNEP, 2008). Increasing human populations along with increased water use by the industrial and agricultural sectors will further expand water scarcity even in places with high rainfall (Doreau et al., 2012). Although water footprint for the same livestock product varies widely across production systems, livestock-induced water scarcity can be avoided by reducing blue water use (surface plus ground water) (Doreau et al., 2012). Crop-livestock integration (i.e., feeding crop by-products to livestock) has been proposed as a potential management practice to reduce water use for livestock production through water recycling (van Breugel et al., 2010; Lal, 2020). Improved irrigation management (e.g., timing and optimizing irrigation through advanced precision technology), using crops which require less water (e.g., triticale and sorghum require less irrigation compared to alfalfa and corn), purchasing feed ingredients from areas where irrigation is not required, and breeding crops to increase either biomass yield or drought adaptability are some of the strategies to reduce water consumption for livestock products.
The pastoral industry has been using indigenous plants and livestock breed/variety/species, which are known to be most adapted to specific regional climatic conditions and exposure of other challengers such as disease outbreak, heat stress, parasite or pest infestation (Provenza, 2008). Local zebu cattle, for example, have greater heat tolerance and disease resistance in tropical climatic conditions than exotic temperate cattle (Johnson, 2018). However, livestock with greater disease resistance and heat tolerance only may not sustain livelihoods due to their low productivity and poor fertility. Thus, breeding strategies have focused on multi-trait selection aiming to create a climate-smart or climate-adapted livestock breed or variety along with improved reproductive and productive efficiency resulting in lower emission intensity. Similarly, plant breeding needs to improve forage and crop varieties that are drought tolerant, resistant to pest infestations and plant diseases, with greater yield and ability to compete with weed infestations. Traditional breeding strategies that utilize natural variation might not always be useful or might take longer to create climate smart livestock and crops. Modern breeding and selection techniques such as genomic selection could make the improvement quicker by reducing generation intervals and allowing traits with low heritability (e.g., fertility traits in cattle) to be included in the breeding scheme. New techniques such as CRISPR genome editing could make much faster progress in livestock and crop varieties that are adapted to ever changing climate to ensure the sustenance of the pastoral industry (Derazmahalleh et al., 2019). However, choice of breeding approaches will depend on multiple factors such as phenotypic and genotypic information, type of traits (simple or polygenic) and regulations in the regions or countries.
Managed and adaptive multi-paddock grazing based beef system can be a potential sink for carbon rather than a source of carbon emissions due to sequestration (Stanley et al., 2018). Although grazing can stimulate soil erosion, lightly grazed grassland can potentially reduce soil erosion by about 60% compared to cropland (Yu et al., 2019). The impacts of grazing on soil erosion and SOC stock depend on regional climatic condition (e.g., dry warm vs. dry cool, moist warm vs. moist cool), grassland vegetation type (e.g., C3 or C4 or mixed), and how grazing is managed (Abdalla et al., 2018). For instance, cover crops can reduce soil erosion substantially (Teague et al., 2016). High grazing intensity has greater impact on SOC increment in C4- than C3-grassland (Abdalla et al., 2018). As an additional benefit, winter grazing can reduce risk and intensity of wildfire in shrub-grassland areas relative to un-grazed areas through reducing fuel load for wildfire (Davies et al., 2016).
Several other forms of regenerative production systems namely silvopastoral, intercropping and conservation agricultural systems also have great potential to sequester carbon (Provenza et al., 2019). Silvopastoral systems, for example, can enhance soil carbon restoration five times greater than managed grazing and are resilient against abrupt climatic events (e.g., rising temperature, drought) due to presence of grasses, shrubs, and trees (Dass et al., 2018). Saponins and tannins commonly found in forbs, shrubs and tree leaves can reduce CH4 and N2O emissions from ruminant production systems which is an additional benefit of silvopastoral system (Hristov et al., 2013). In addition to GHG mitigations, co-benefits of managed grazing and regenerative agriculture include enhancement of soil health, biodiversity, water holding capacity of soil and ecosystem services (Teague et al., 2016; Provenza et al., 2019).
Provenza (2008) mentioned the following strategies to improve grazing: (1) coupling animal needs with forage availability, (2) selecting animals that are adapted to local conditions and landscapes, and (3) creating grazing systems that simultaneously benefit soils, plants, herbivores and human beings. Grazing management should also focus drought and weeds, two serious threats to pastures. Drought can be managed by carefully managing grazing, reducing carrying capacity of pasture to 75% less than in normal years, and slowing grazing rotations (Rinehart, 2008). Weeds can be managed by multispecies and high-density rotational grazing (Rinehart, 2008). Increased grazing duration can be more damaging than higher stocking density which can help to avoid palatability issue and weed problem in pastures (Rinehart, 2008).
Finally, integrating scientific knowledge gathered by researchers with the experiences acquired by the herders would help to manage grazing in better ways through: (1) improving animal training to utilize pasture properly, (2) maintaining grazing boundaries, (3) altering temporary forage palatability scoring made by grazing animals, and (4) stimulating animal appetite and intake by manipulating meal sequence (Meuret and Provenza, 2015; Molnár et al., 2020). For example, skilled herders in France design grazing circuits at a meal scale to increase appetite and intake, to create synergies among meal phases, and to increase intake of abundant but less palatable forages (Meuret and Provenza, 2015). To do so, they partition landscapes into grazing sectors that are carefully sequenced within daily circuits. Meals are based on complementarity blends of terrain and plant communities within and among sectors, not on particular plants. To do so, herders identify and ration various sectors into phases of a meal: appetite stimulator or moderator, first course, booster, second course, and dessert sectors.
Climate change and increased food demand pose a serious threat to the pastoral industry, which has considerable economic, ecological, and socio-cultural importance. Further population growth will aggravate the impact of climate change on the pastoral industry. Climate change is affecting the pastoral industry through feed and fodder production, productivity and fertility of livestock, pest and disease outbreak for crops and livestock, water scarcity for livestock and feed production. The pastoral industry also impacts climate change negatively through emissions of GHG, expansion of pastureland through deforestation, erosion and degradation of soil, and air and water quality. Both supply-side (e.g., reducing emission intensity and improving resource-use efficiency) and demand-side (e.g., reducing food demand and food waste, and improving food access through proper distribution) mitigation strategies are needed to reduce emissions. In addition to properly managing animals and pastures, modern breeding and selection techniques, such as genomic selection, CRISPR genome-editing tools, should also be used to aid crop and livestock adaptation to ever changing climate, thus ensuring sustainability of the pastoral industry.
MEU participated in all components of the review, conception and design, and major contribution in writing the manuscript. EK participated in drafting and writing the manuscript. All authors read and approved the final manuscript. All authors contributed to the article and approved the submitted version.
The authors declare that the research was conducted in the absence of any commercial or financial relationships that could be construed as a potential conflict of interest.
Abdalla, M., Hastings, A., Chadwick, D. R., Jones, D. L., Evans, C. D., Jones, M. B., et al. (2018). Critical review of the impacts of grazing intensity on soil organic carbon storage and other soil quality indicators in extensively managed grasslands. Agric. Ecosyst. Environ. 253, 62–81. doi: 10.1016/j.agee.2017.10.023
African Union (2010). Policy Framework for Pastoralism in Africa: Securing, Protecting and Improving the Lives, Livelihoods and Rights of pastoRal Communities, Addis Ababa. Available online at: https://au.int/sites/default/files/documents/30240-doc-policy_framework_for_pastoralism.pdf (accessed February 28, 2020).
Aguirre-Villegas, H. A., and Larson, R. A. (2017). Evaluating greenhouse gas emissions from dairy manure management practices using survey data and lifecycle tools. J. Clean. Prod. 143, 169–179. doi: 10.1016/j.jclepro.2016.12.133
Akinmoladun, O. F., Muchenje, V., Fon, F. N., and Mpendulo, C. T. (2019). Small ruminants: farmers' hope in a world threatened by water scarcity. Animals 9, 1–20. doi: 10.3390/ani9070456
Barona, E., Ramankutty, N., Hyman, G., and Coomes, O. T. (2010). The role of pasture and soybean in deforestation of the Brazilian Amazon. Environ. Res. Lett. 5:24002. doi: 10.1088/1748-9326/5/2/024002
Bett, B., Kiunga, P., Gachohi, J., Sindato, C., Mbotha, D., Robinson, T., et al. (2017). Effects of climate change on the occurrence and distribution of livestock diseases. Prev. Vet. Med. 137, 119–129. doi: 10.1016/j.prevetmed.2016.11.019
Blench, R. (2001). ‘You Can't Go Home Again': Pastoralism in the New Millennium. Overseas Development Institute, London. Available online at: https://www.odi.org/sites/odi.org.uk/files/odi-assets/publications-opinion-files/6329.pdf (accessed February 16, 2020).
Brian, R. (2015). Meat and milk consumption 2050: the potential for demand-side solutions to greenhouse gas emissions reduction. Agric. Policy Environ. 14, 4–11. doi: 10.1111/1746-692X.12103
Capper, J. L. (2012). Is the grass always greener? Comparing the environmental impact of conventional, natural and grass-fed beef production systems. Animals 2, 127–143. doi: 10.3390/ani2020127
CIMMYT (2019). What is Wheat Blast? Available online at: https://www.cimmyt.org/news/what-is-wheat-blast/ (accessed February 25, 2020).
Clark, M., and Tilman, D. (2017). Comparative analysis of environmental impacts of agricultural production systems, agricultural input efficiency, and food choice comparative analysis of environmental impacts of agricultural production systems, agricultural input efficiency, and food. Environ. Res. Lett. 12:064016. doi: 10.1088/1748-9326/aa6cd5
Cowley, F. C., Barber, D. G., Houlihan, A. V., and Poppi, D. P. (2015). Immediate and residual effects of heat stress and restricted intake on milk protein and casein composition and energy metabolism. J. Dairy Sci. 98, 2356–2368. doi: 10.3168/jds.2014-8442
Dass, P., Houlton, B. Z., Wang, Y., and Warlind., D. (2018). Grasslands may be more reliable carbon sinks than forests in California. Environ. Res. Lett. 13:074027. doi: 10.1088/1748-9326/aacb39
Davies, K. W., Boyd, C. S., Bates, J. D., and Hulet, A. (2016). Winter grazing can reduce wildfire size, intensity and behaviour in a shrub-grassland. Int. J. Wildland Fire 25, 191–199. doi: 10.1071/WF15055
Derazmahalleh, M. M., Erskine, W., Hane, J. K., Nelson, M. N., Nguyen, H. T., Varshney, R. K., et al. (2019). Adapting legume crops to climate change using genomic approaches. Plant Cell Environ. 42, 6–19. doi: 10.1111/pce.13203
Derner, J. D., and Schuman, G. E. (2007). Carbon sequestration and rangelands: a synthesis of land management and precipitation effects. J. Soil. Water Conserv. 62, 77–85. Available online at: https://www.jswconline.org/content/jswc/62/2/77.full.pdf
Domínguez, L. R. R., Vásquez, P. C. G., and Gonzalez, P. E. (2005). Effect of heat stress and its interaction with other management and productive variables on pregnancy rate in dairy cows in Aguas- calientes, Mexico. Vet. Mexico 36, 245–260. Available online at: https://www.medigraphic.com/pdfs/vetmex/vm-2005/vm053a.pdf
Dong, S., Liu, S., and Wen, L. (2016). “Vulnerability and resilience of human-natural systems of pastoralism worldwide,” in Building Resilience of Human-Natural Systems of Pastoralism in the Developing World. eds S. Dong, K. A. Kassam, J. Tourrand, and R. Boone (Cham: Springer). Available online at: https://www.springer.com/gp/book/9783319307305 (accessed February 18, 2020).
Doreau, M., Corson, M. S., and Wiedemann, S. G. (2012). Water use by livestock: a global perspective for a regional issue? Anim. Front. 2, 9–16. doi: 10.2527/af.2012-0036
Dumont, B., Andueza, D., Niderkorn, V., Lüscher, A., Porqueddu, C., and Picon-Cochard, C. (2015). A meta-analysis of climate change effects on forage quality in grasslands: specificities of mountain and Mediterranean areas. J. Brit. Grassland Soc. 70, 239–254. doi: 10.1111/gfs.12169
Dyer, C. (2016). Approaches to education provision for mobile pastoralists. Rev. Sci. Tech. 35, 631–638. doi: 10.20506/rst.35.2.2525
Eisler, M. C., Lee, M. R. F., Tarlton, J. F., Martin, G. B., Beddington, J., and Dungait, J. A. J., et al. (2014). Steps to sustainable livestock. Nature 507:32–34. doi: 10.1038/507032a
FAO (2001). “Pastoralism in the new millennium,” Animal Production and Health Paper no 150 (Rome: Food and Agriculture Organization of the United Nations). Available online at: http://www.fao.org/3/y2647e/y2647e00.htm (accessed February 13, 2020).
FAO (2006). World Agriculture: Towards 2030/2050-Interim Report. Rome. Available online at: http://www.fao.org/fileadmin/user_upload/esag/docs/Interim_report_AT2050web.pdf (accessed February 15, 2020).
FAO (2018a). Climate change and the global dairy cattle sector-The role of the dairy sector in a low-carbon future. Rome, Italy. 36 pp. Licence: CC BY-NC-SA-3.0 IGO. Available online at: http://www.fao.org/3/CA2929EN/ca2929en.pdf (accessed January 8, 2020).
FAO (2018b). Water Use of Livestock Production Systems and Supply Chains – Guidelines for Assessment. Livestock Environmental Assessment and Performance Partnership. FAO, Rome (accessed January 8, 2020).
FAOSTAT (2014). FAO. Available online at: http://www.fao.org/faostat/en/?#data/RL (accessed March 1, 2020).
FAOSTAT (2016). FAO. Available online at: http://www.fao.org/faostat/en/#home (accessed March 4, 2020).
FAOSTAT (2017). FAO. Available online at: http://www.fao.org/faostat/en/#home (accessed March 6, 2020).
Flood, J. (2010). The importance of plant health to food security. Food Sec. 2, 215–231. doi: 10.1007/s12571-010-0072-5
Foodtank (2019). Farmers Look to FAO to Help Resolve the Worsening Pest Outbreak Issue. Available online at: https://foodtank.com/news/2019/03/climate-change-exacerbates-the-challenge-of-plant-pests/ (accessed 25 February 2020).
Gerber, P., Steinfeld, H., Henderson., B., Mottet, A., Opio, C., et al. (2013). Tackling Climate Change through Livestock. A Global Assessment of Emissions and Mitigation Opportunities. FAO, Rome. Available online at: http://www.fao.org/3/a-i3437e.pdf (accessed February 20, 2020).
Godde, C. M., Boone, R. B., Ash, A. J., Waha, K., Sloat, L. L., Thornton, P. K., et al. (2020). Global rangeland production systems and livelihoods at threat under climate change and variability. Environ. Res. Lett. 15:044021. doi: 10.1088/1748-9326/ab7395
Godfray, H. C. J., Beddington, J. R., Crute, I. R., Haddad, L., Lawrence, D., Muir, J. F., et al. (2010). Food security: the challenge of feeding 9 billion people. Science 327, 812–818. doi: 10.1126/science.1185383
Golub, A. A., Henderson, B. B., Hertel, T. W., Gerber, P. J., Rose, S. K., and Sohngen, B. (2013). Global climate policy impacts on livestock, land use, livelihoods, and food security. Proc. Nat. Acad. Sci. U.S.A. 110, 20894–20899. doi: 10.1073/pnas.1108772109
Greer, D. H., Laing, W. A., and Campbell, B. D. (1995). Photosynthetic responses of thirteen pasture species to elevated CO2 and temperature. Aust. J. Plant Phys. 22, 713–722. doi: 10.1071/PP9950713
Hahn, G. L. (1999). Dynamic responses of cattle to thermal heat loads dynamic responses of cattle to thermal heat loads. J. Anim. Sci. 77, 10–20. doi: 10.2527/1997.77suppl_210x
Hatfield, J. L., Boote, K. J., Kimball, B. A., Ziska, L. H., Izaurralde, R. C., Ort, D., et al. (2011). Climate impacts on agriculture: implications for crop production. Agron. J. 103, 351–370. doi: 10.2134/agronj2010.0303
Hoffmann, I. (2010). “Impacts of climate change on livestock genetic diversity and selection,” in Proceedings of the World Conference on Genetics applied to Livestock Production (Leipzig). Available online at: http://www.wcgalp.org/system/files/proceedings/2010/impacts-climate-change-livestock-genetic-selection-and-diversity.pdf (accessed March 9, 2020).
Hristov, A. N., Oh, J., Firkins, J. L., Dijkstra, J., Kebreab, E., and Waghorn, G., et al. (2013). Mitigation of methane and nitrous oxide emissions from animal operations: I. A review of enteric methane mitigation options. J. Anim. Sci. 91:5045–5069. doi: 10.2527/jas.2013-6583
Hristov, A. N., Oh, J., Giallongo, F., Frederick, T. W., Harper, M. T., Weeks, H. L., et al. (2015). An inhibitor persistently decreased enteric methane emission from dairy cows with no negative effect on milk production. Proc. Nat. Acad. Sci. U.S.A. 112, 10663–10668. doi: 10.1073/pnas.1504124112
Humphreys, L. R. (1991). The Response of Grazing Animals to Tropical Pastures Tropical Pasture Utilisation. Cambridge: Cambridge University Press, 88–106.
IFAD (2008). Women and Pastoralists. Available online at: https://www.ifad.org/documents/38714170/39148759/Women$+$and$+$pastoralism.pdf/bc1ac853-bfd4-420e-aeae-1d63dd7ea3e1 (accessed February 12, 2020).
IPCC (2014). Climate Change 2014: Mitigation of Climate Change. Contribution of Working Group III to the Fifth Assessment Report of the Intergovernmental Panel on Climate Change, eds O. Edenhofer, R. Pichs-Madruga, Y. Sokona, E. Farahani, S. Kadner, K. Seyboth, et al. (Cambridge; New York, NY: Cambridge University Press). Available online at: https://www.ipcc.ch/site/assets/uploads/2018/02/ipcc_wg3_ar5_frontmatter.pdf (accessed February 10, 2020).
IPCC (2019). “Summary for Policymakers',” in Climate Change and Land: An IPCC Special Report on Climate Change, Desertification, Land Degradation, Sustainable Land Management, Food Security, and Greenhouse Gas Fluxes in Terrestrial Ecosystems, eds P. R. Shukla, J. Skea, E. Calvo Buendia, V. Masson-Delmotte, H.- O. Pörtner, D. C. Roberts, et al. Available online at: https://www.ipcc.ch/site/assets/uploads/2019/11/02_Summary-for-Policymakers_SPM.pdf (accessed February 10, 2020).
Izaurralde, R. C., Thomson, A. M., Morgan, J. A., Fay, P. A., Polley, H. W., and Hatfield, J. L. (2011). Climate impacts on agriculture: implications for forage and rangeland production. Agron. J. 103, 371–381. doi: 10.2134/agronj2010.0304
Johnson, J. S. (2018). Heat stress: impact on livestock well-being and productivity and mitigation strategies to alleviate the negative effects. Anim. Prod. Sci. 58, 1404–1413. doi: 10.1071/AN17725
Kairis, O., Karavitis, C., Salvati, L., Kounalaki, A., and Kosmas, K. (2015). Exploring the impact of overgrazing on soil erosion and land degradation in a dry mediterranean agro-forest landscape (Crete, Greece). Arid Land Res. Manage. 29, 360–374. doi: 10.1080/15324982.2014.968691
Kaufmann, B. A., Lelea, M. A., and Hulsebusch, C. G. (2016). Diversity in livestock resources in pastoral systems in Africa. Rev. Sci. Tech. 35, 445–459. doi: 10.20506/rst.35.2.2535
Knapp, J. R., Laur, G. L., Vadas, P. A., Weiss, W. P., and Tricarico, J. M. (2014). Invited review: enteric methane in dairy cattle production: Quantifying the opportunities and impact of reducing emissions. J. Dairy Sci. 97, 3231–3261. doi: 10.3168/jds.2013-7234
Lal, R. (2020). Integrating animal husbandry with crops and trees. Front. Sustain. Food Syst. 4:113. doi: 10.3389/fsufs.2020.00113
Liu, J., Li, L., Chen, X., Lu, Y., and Wang, D. (2019). Effects of heat stress on body temperature, milk production, and reproduction in dairy cows: a novel idea for monitoring and evaluation of heat stress-a review. Asian Aust. J. Anim. Sci. 32, 1332–1339. doi: 10.5713/ajas.18.0743
Maillard, É., and Angers, D. A. (2014). Animal manure application and soil organic carbon stocks: a meta-analysis. Glob. Chang. Biol. 20:666–679. doi: 10.1111/gcb.12438
Meuret, M., and Provenza, F. D. (2015). When art and science meet: integrating knowledge of French herders with science of foraging behavior. Rangel. Ecol. Manag. 68, 1–17. doi: 10.1016/j.rama.2014.12.007
Molnár, Z., Kelemen, A., Kun, R., Máté, J., Sáfián, L., Provenza, F., et al. (2020). Knowledge co-production with traditional herders on cattle grazing behaviour for better management of species-rich grasslands. J. Appl. Ecol. 57, 1677–1687. doi: 10.1111/1365-2664.13664
Morignat, E., Gay, E., Vinard, J. L., Calavas, D., and Hénaux, V. (2015). Quantifying the influence of ambient temperature on dairy and beef cattle mortality in France from a time-series analysis. Environ. Res. 140:524–534. doi: 10.1016/j.envres.2015.05.001
Nardone, A., Ronchi, B., Lacetera, N., Ranieri, M. S., and Bernabucci, U. (2010). Effects of climate change on animal production and sustainability of livestock systems. Livest. Sci. 130, 57–69. doi: 10.1016/j.livsci.2010.02.011
Newman, Y. C., Sollenberger, L. E., Boote, K. J., Allen, L. H. Jr., and Littell, R. C. (2001). Carbon dioxide and temperature effects on forage dry matter production. Crop Sci. 41, 399–406. doi: 10.2135/cropsci2001.412399x
Nijdam, D., Rood, T., and Westhoek, H. (2012). The price of protein: review of land use and carbon footprints from life cycle assessments of animal food products and their substitutes. Food Policy 37, 760–770. doi: 10.1016/j.foodpol.2012.08.002
Nyariki, D. M., and Amwata, D. A. (2019). The value of pastoralism in kenya: application of total economic value approach. Pastoralism 9, 1–13. doi: 10.1186/s13570-019-0144-x
Patterson, D. T., Meyer, C. R., Flint, E. P., and Quimby, P. C. Jr. (1979). Temperature responses and potential distribution of itchgrass (Rottboellia exaltata) in the United States. Weed Sci. 27, 77–82. doi: 10.1017/S0043174500043538
Poore, J., and Nemecek, T. (2018). Reducing food's environmental impacts through producers and consumers. Science 360, 987–992. doi: 10.1126/science.aaq0216
Provenza, F. D. (2008). What does it mean to be locally adapted and who cares anyway? J. Anim. Sci. 86. doi: 10.2527/jas.2007-0468
Provenza, F. D., Kronberg, S. L., and Gregorini, P. (2019). Is grassfed meat and dairy better for human and environmental health? Front. Nutr. 6, 1–13. doi: 10.3389/fnut.2019.00026
Ramesh, K., Matloob, A., Aslam, F., Florentine, S. K., and Chauhan, B. S. (2017). Weeds in a changing climate: vulnerabilities, consequences, and implications for future weed management. Front. Plant Sci. 8:95. doi: 10.3389/fpls.2017.00095
Reeves, M. C., Moreno, A. L., Bagne, K. E., and Running, S. W. (2014). Estimating climate change effects on net primary production of rangelands in the United States. Clim. Change 126, 429–442. doi: 10.1007/s10584-014-1235-8
Reyad, M., Sarker, M. A., Uddin, M. E., Habib, R., and Rashid, M. H. (2016). Effect of heat stress on milk production and its composition of holstein friesian crossbred dairy cows. Asian J. Med. Biol. Res. 2, 190–195. doi: 10.3329/ajmbr.v2i2.29060
Rinehart, L. (2008). Pasture, Rangeland and Grazing Management. National Sustainable Agriculture Information Service. Available online at: https://extension.usu.edu/rangelands/ou-files/Pasture_Range_Grazing_Management.pdf (accessed September 10, 2020).
Rojas-Downing, M. M., Nejadhashemi, A. P., Harrigan, T., and Woznicki, S. A. (2017). Climate change and livestock: impacts, adaptation, and mitigation. Clim. Risk Manage. 16, 145–163. doi: 10.1016/j.crm.2017.02.001
Roque, B. M., Salwen, J. K., Kinley, R., and Kebreab, E. (2019). Inclusion of Asparagopsis armata in lactating dairy cows' diet reduces enteric methane emission by over 50 percent. J. Cleaner Prod. 234, 132–138. doi: 10.1016/j.jclepro.2019.06.193
Rotz, C. A., Asem-Hiablie, S., Place, S., and Thoma, G. (2019). Environmental footprints of beef cattle production in the United States. Agric. Syst. 169, 1–13. doi: 10.1016/j.agsy.2018.11.005
Schielein, J., and Börner, J. (2018). Recent transformations of land-use and land-cover dynamics across different deforestation frontiers in the Brazilian Amazon. Land Use Policy 76, 81–94. doi: 10.1016/j.landusepol.2018.04.052
Sejian, V., Bhatta, R., Gaughan, J. B., Dunshea, F. R., and Lacetera, N. (2018). Review: adaptation of animals to heat stress. Animals 12, S431–S444. doi: 10.1017/S1751731118001945
Stanley, P. L., Rowntree, J. E., Beede, D. K., DeLonge, M. S., and Hamm, M. W. (2018). Impacts of soil carbon sequestration on life cycle greenhouse gas emissions in Midwestern USA beef finishing systems. Agric. Syst. 162, 249–258. doi: 10.1016/j.agsy.2018.02.003
Steinfeld, H. (2012). “Global environmental challenges,” in Presentation for the ILRI World Bank Consultation on the Global Livestock Agenda by 2020, Nairobi March 2012 (Rome: FAO). Available online at: http://www.slideshare.net/ILRI/global-environmental-challenges (Accessed August 25, 2020).
Steinfeld, H., Mooney, H. A., Schneider, F., and Neville, L. E. (2010). Livestock in a Changing Landscape: Drivers, Consequences, and Responses, Vol. 1. Scientific Committee on Problems of the Environment, Island Press, Washington, DC. Rome. Available online at: https://islandpress.org/books/livestock-changing-landscape-volume-1 (accessed August 25, 2020).
Stolton, S., Dudley, N., and Zogib, L. (2019). Mobile Pastoralism and the World Heritage Convention. A DiversEarth publication (Switzerland). Available online at: https://roads-less-travelled.org/wp-content/uploads/2019/08/Mobile-Pastoralism-and-the-World-Heritage-Convention-_-For-Web.pdf (accessed February 13, 2020).
St-Pierre, N. R., Cobanov, B., and Schnitkey, G. (2003). Economic losses from heat stress by U.S. livestock industries. J. Dairy Sci. 86, E52–E77. doi: 10.3168/jds.S0022-0302(03)74040-5
Summer, A., Lora, I., Formaggioni, P., and Gottardo, F. (2019). Impact of heat stress on milk and meat production. Anim. Front. 9, 39–46. doi: 10.1093/af/vfy026
Suter, D., Nösberger, J., and Lüscher, A. (2001). Response of perennial ryegrass to free-air CO2 enrichment (FACE) is related to the dynamics of sward structure during regrowth. Crop Sci. 41, 810–817. doi: 10.2135/cropsci2001.413810x
Swain, M., Blomqvist, L., McNamara, J., and Ripple, W. J. (2018). Reducing the environmental impact of global diets. Sci. Total Environ. 610–611, 1207–1209. doi: 10.1016/j.scitotenv.2017.08.125
Teague, W. R., Apfelbaum, S., Lal, R., Kreuter, U. P., Rowntree, J., Davies, C. A., et al. (2016). The role of ruminants in reducing agriculture's carbon footprint in North America. J. Soil Water Conserv. 71, 156–164. doi: 10.2489/jswc.71.2.156
Tessari, P., Lante, A., and Mosca, G. (2016). Essential amino acids: master regulators of nutrition and environmental footprint? Sci. Rep. 6:26074. doi: 10.1038/srep26074
Thoma, G., Popp, J., Nutter, D., Shonnard, D., Ulrich, R., Matlock, M., et al. (2013). Greenhouse gas emissions from milk production and consumption in the United States: a cradle-to-grave life cycle assessment circa 2008. Int. Dairy J. 31, S3–S14. doi: 10.1016/j.idairyj.2012.08.013
Thomson, A. M., Brown, R. A., Rosenberg, N. J., Izaurralde, R. C., and Benson, V. W. (2005). Climate change impacts for the conterminous USA: an integrated assessment part 3. dryland production of grain and forage crops. Clim. Change 69, 43–65. doi: 10.1007/s10584-005-3612-9
Thornton, P. K., van de Steeg, J., Notenbaert, A., and Herrero, M. (2009). The impacts of climate change on livestock and livestock systems in developing countries: a review of what we know and what we need to know. Agric. Syst. 101, 113–127. doi: 10.1016/j.agsy.2009.05.002
Tilman, D., Balzer, C., Hill, J., and Befort, B. L. (2011). Global food demand and the sustainable intensification of agriculture. Proc. Nat. Acad. Sci.U.S.A. 108, 20260–20264. doi: 10.1073/pnas.1116437108
Tilman, D., and Clark, M. (2014). Global diets link environmental sustainability and human health. Nature 515, 518–522. doi: 10.1038/nature13959
UN (2019). World Population Prospects 2019: Highlights (ST/ESA/SER.A/423). UN Department of Economic and Social Affairs, Population Division. Available online at: https://population.un.org/wpp/Publications/Files/WPP2019_Highlights.pdf (accessed February 18, 2020).
UNEP (2008). Vital Water Graphics - An Overview of the State of the World's Fresh and Marine Waters, 2nd ed. UNEP, Nairobi. Available online at: http://www.grida.no/publications/vg/water2/ (accessed February 16, 2020).
van Breugel, P., Herrero, M., van de Steeg, J., and Peden, D. (2010). Livestock water use and productivity in the Nile Basin. Ecosystem 13, 205–221. doi: 10.1007/s10021-009-9311-z
Van Dijk, J., Sargison, N. D., Kenyon, F., and Skuce, P. J. (2010). Climate change and infectious disease: helminthological challenges to farmed ruminants in temperate regions. Animals 4, 377–392. doi: 10.1017/S1751731109990991
Wattiaux, M. A., Uddin, M. E., Letelier, P., Jackson, R. D., and Larson, R. A. (2019). Invited review : emission and mitigation of greenhouse gases from dairy farms : the cow, the manure, and the field. Appl. Anim. Sci. 35, 238–254. doi: 10.15232/aas.2018-01803
WHO (2008). Anthrax in Humans and Animals, 4th Edn. World Health Organization, Geneva. Available online at: https://apps.who.int/iris/bitstream/handle/10665/97503/9789241547536_eng.pdf; jsessionid$=$FE2B7AD71CA58BCA4337F1628773248E?sequence$=$1 (accessed Feb. 22, 2020).
Yu, H., Li, Y., Oshunsanya, S. O., Are, K. S., Geng, Y., et al. (2019). Re-introduction of light grazing reduces soil erosion and soil respiration in a converted grassland on the Loess Plateau, China. Agric. Ecosyst. Environ. 280, 43–52. doi: 10.1016/j.agee.2019.04.020
Keywords: adaptation, environment, greenhouse gas, livestock, pastoralism
Citation: Uddin ME and Kebreab E (2020) Review: Impact of Food and Climate Change on Pastoral Industries. Front. Sustain. Food Syst. 4:543403. doi: 10.3389/fsufs.2020.543403
Received: 16 March 2020; Accepted: 28 September 2020;
Published: 23 October 2020.
Edited by:
Fred Provenza, Utah State University, United StatesReviewed by:
Dean Revell, University of Western Australia, AustraliaCopyright © 2020 Uddin and Kebreab. This is an open-access article distributed under the terms of the Creative Commons Attribution License (CC BY). The use, distribution or reproduction in other forums is permitted, provided the original author(s) and the copyright owner(s) are credited and that the original publication in this journal is cited, in accordance with accepted academic practice. No use, distribution or reproduction is permitted which does not comply with these terms.
*Correspondence: Ermias Kebreab, ZWtlYnJlYWJAdWNkYXZpcy5lZHU=
Disclaimer: All claims expressed in this article are solely those of the authors and do not necessarily represent those of their affiliated organizations, or those of the publisher, the editors and the reviewers. Any product that may be evaluated in this article or claim that may be made by its manufacturer is not guaranteed or endorsed by the publisher.
Research integrity at Frontiers
Learn more about the work of our research integrity team to safeguard the quality of each article we publish.