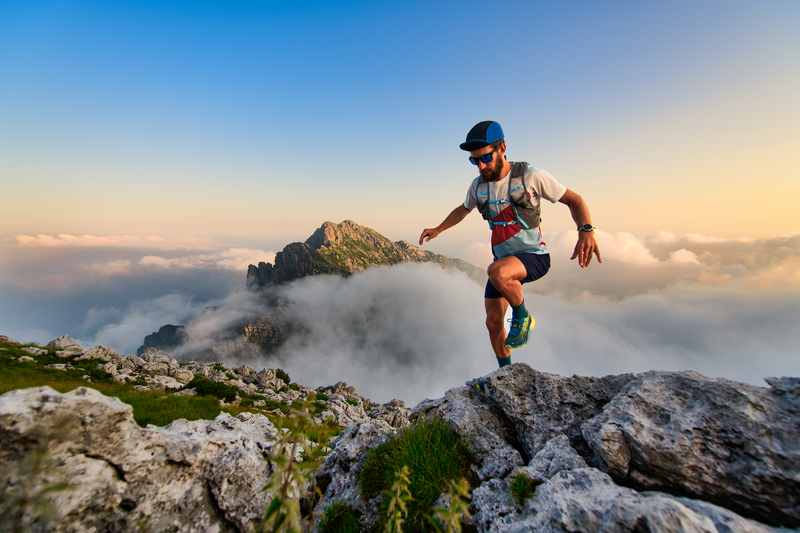
95% of researchers rate our articles as excellent or good
Learn more about the work of our research integrity team to safeguard the quality of each article we publish.
Find out more
REVIEW article
Front. Sustain. Food Syst. , 29 September 2020
Sec. Crop Biology and Sustainability
Volume 4 - 2020 | https://doi.org/10.3389/fsufs.2020.00136
This article is part of the Research Topic Plant Growth-Promoting Microorganisms for Sustainable Agricultural Production View all 50 articles
Free-living bacteria that actively colonize plant roots and provide positive effects on plant development are called plant-growth promoting. Plant growth-promoting bacteria can promote plant growth and use their own metabolism to solubilize phosphates, produce hormones and fix nitrogen, and they can directly affect plant metabolism. PGPR also increase plant absorption of water and nutrients, improving root development and increasing plant enzymatic activity; moreover, PGPR can promote other microorganisms as part of a synergistic effect to improve their effects on plants, promoting plant growth or suppressing pathogens. Many studies have shown several benefits of the use of PGPR in maize and sugarcane crops. These bacteria are an excellent alternative to farmers to reduce chemical fertilization and pesticide input without promoting the environment impact and yield-reducing. The present review is an effort to elucidate the concept of rhizobacteria in the current scenario and their underlying mechanisms of plant growth promotion with recent updates. The latest paradigms of a wide range of applications of these beneficial rhizobacteria in both crops maize and sugarcane have been presented explicitly to garner broad perspectives regarding their functioning and applicability. The results from several studies have shown that the utilization of PGPR in maize and sugarcane is the great alternative to farmers face the challenge the modern agriculture.
This review focuses on plant growth-promoting rhizobacteria that are beneficial for the plant. Some beneficials do so by promoting plant growth directly, i.e., in the absence of pathogens. Others do this indirectly by protecting the plant against soil- borne diseases, most of which are caused by fungi.
The use of PGPR is one potential way to decrease negative environmental impact resulting from continued use of chemical fertilizers, pesticides and herbicides. This term was first defined by Kloepper and Schroth (1978) to describe soil bacteria that colonize the rhizosphere of plants, growing in, on or around plant tissues that stimulate plant growth by several mechanisms. Since that time, research activities aimed at understanding how these bacteria perform their positive (or negative) effect have steadily increased and many studies have been published on these microorganisms (Vessey, 2003; Lugtenberg and Kamilova, 2009; Perez-Montano et al., 2014).
Increasing crop production to meet the demands of consumer markets and the growing world population relies on the use of a large amount of chemical fertilizers and pesticides, which are often overused in soil (Kumar et al., 2017). The use of chemical fertilizers in crop production provides an average yield increase of approximately 50% compared to production without their use; however, chemical fertilization practices ignore the biological potential of roots or the rhizosphere by increasing nutrient mobilization and acquisition and decreasing the interactions between plants and rhizospheric microorganisms (Meena et al., 2017). Many studies have demonstrated the abilities of plant growth-promoting microorganisms to increase plant nutritional status and reduce the use of pesticides (Aloo et al., 2019).
Plant growth-promoting rhizobacteria is an excellent alternative to farmers face the new challenges of modern agriculture as serious environmental and social problems emerged as a consequence of industrialization of agriculture provoked by necessity to increase a great amount of food to the general population. Currently, it is urgent to maintain high productivity impacting the environment as little as possible (Pérez-Montaño et al., 2013).
There are two mechanisms used by PGPR to promote plant growth. Each mechanism contains several parameters related to plant growth. The direct mechanism contains the parameters production of phytohormones Cassán et al. (2009) such as auxins (Khalid et al., 2004b); siderophores (Yu et al., 2019); phosphorous solubilization Krey et al. (2013), or nitrogen-fixing (Riggs et al., 2001). Indirect mechanism is related to biocontrol, by mean of antagonistic activity against phytopathogenic microorganisms inducing plant systemic resistance responses, interfering in the bacterial quorum sensing (QS) systems, etc. Some reports show PGPR may use more than one of these mechanisms for accomplishing plant growth enhancement (Bashan and Holguin, 1997; Ahmad et al., 2016).
In this review we begin with a description of how bacteria live on the root, which nutrients are available, and how the bacteria colonize the root. Competitive rhizosphere colonization is crucial for many mechanisms of action of plant- beneficial bacteria. Which bacterial traits are important for root colonization when bacteria compete with each other and with other organisms. We describe various mechanisms used by specialized beneficial rhizobacteria to positively influence plant growth. These mechanisms were classified as direct mechanisms and indirect mechanisms. The direct mechanisms approached in this review were phytohormone production, biological nitrogen fixation, phosphorus solubilization, potassium solubilization. The indirect mechanisms approached were production of antibiotics, induced systemic resistance, production of siderophores, rhizoremediation, and stress control and interference with the quorum sensing system. This review brings in Tables 1, 2 the most important studies related to the use of these bacteria in maize and sugarcane production, respectively. Finally, this review also brings in Figure 1 that shows the benefits to plants from host-PGPR interactions, Figure 2, direct mechanisms that benefit plant growth, and in Figure 3, indirect mechanisms that benefit plant growth.
Table 1. Bacteria specie, abilities, experiment condition, and results promoted by the application of many plant growth- promoting rhizobacteria in maize crop.
Table 2. Bacteria specie, abilities, experiment condition, and results promoted by the application of many plant growth- promoting rhizobacteria in sugarcane.
Figure 1. Benefits to plants from host-PGPR interactions. These benefits have been shown to include in sees germination rate, root growth, yield, leaf area, chlorophyll content, nutrient uptake, protein content, hydraulic activity, tolerance to abiotic stress, shoot and root weights and heights, bio-control, and delayed senescence.
Figure 2. Direct mechanisms that benefit plant growth from host-PGPR interactions. Biofertilization, nutrients solubilization, and phytostimulation.
Figure 3. Indirect mechanisms that benefit plant growth from host-PGPR interactions. Antibiosis, siderophores, interference with the quorum sensing (QS), induced systemic resistance (ISR).
For the plant growth-promoting rhizobacteria colonize the rhizosphere two-step selection process by which the bacterial microbiota of roots is differentiated from the surrounding soil biome are necessary. One potential molecular mechanism underlying the formation of a distinctive rhizosphere microbiota from soil biomes is rhizodeposition. This process refers to intertwined plant developmental and secretory activities in the root system. Rhizodermis cells secrete a wide range of compounds, including organic acid ions, inorganic ions, phytosiderophores, sugars, vitamins, amino acids, purines, and nucleosides, and the root cap produces polysaccharide mucilage (Dakora and Phillips, 2002). Rhizodeposition appears to fuel an initial substrate-driven community shift in the rhizosphere, which converges with host genotype–dependent finetuning of microbiota profiles in the selection of root endophyte assemblages (Bulgarelli et al., 2013). To exert their beneficial effects, bacteria usually must colonize the root surface efficiently (Lugtenberg and Kamilova, 2009). The substrate-driven selection also underlies the establishment of phyllosphere communities but takes place solely at the immediate leaf surface. Both the leaf and root microbiota contain bacteria that provide indirect pathogen protection, but root microbiota members appear to serve additional host functions through the acquisition of nutrients from the soil for plant growth. Thus, the plant microbiota emerges as a fundamental trait that includes mutualism enabled through diverse biochemical mechanisms, as revealed by studies on plant growth–promoting and plant health–promoting bacteria (Bulgarelli et al., 2013).
The direct action of plant growth-promoting microorganisms involves soil improvements and the production of substances needed for plant growth, which improves fertility by mobilizing soil minerals (Naik et al., 2019). These improvements include the supply of growth regulators and essential minerals such as potassium, phosphorous and (Tabassum et al., 2017).
Phytohormones are responsible for plant growth development and allow plants to tolerate different stress conditions (Shaterian et al., 2005). Some rhizobacteria are able to produce phytohormones, including cytokinins, auxins, gibberellins, ethylene, and abscisic acid (ABA), which play a role in different growth processes in plants, including cell multiplication, which results in increased cell and root expansion (Glick, 2014; Kaur et al., 2016). However, the production of ABA by rhizobacteria is considered an indirect way of promoting plant growth (Belimov et al., 2014).
Auxins influence many aspects of plant development (Halliday et al., 2009; Grossmann, 2010). The most essential (and well-known) is indole-3-acetic acid (IAA), which is produced by different microorganisms and this hormone, in plants, plays an essential role in cell division, fruit development and leaves senescence (McSteen, 2010). IAA stimulates the development of many parts of the plants such as roots, leaves, and flowers (Phillips et al., 2011). In dicotyledons, IAA induces the formation of lateral roots, while in monocotyledons, it induces the formation of adventitious roots (McSteen, 2010).
Plant development is affected by IAA in both favorable and harmful ways and many bacteria have the ability to synthesize IAA, including beneficial bacteria and phytopathogens (Duca et al., 2014). More than 80% of bacteria isolated from the rhizosphere are IAA producer (Patten and Glick, 1996; Khalid et al., 2004a). IAA is responsible by part of the communication and signaling system between plants and rhizospheric bacteria (Spaepen et al., 2007).
The tryptophan is the main precursor for the synthesis of IAA and the addition of this amino acid to culture media results in increased production in all cases (Spaepen et al., 2007). Tryptophan biosynthesis starts from metabolic nodes in a five-step reaction encoded by trp genes (Merino et al., 2008).
The pathways of tryptophan-dependent include indole-3-acetamide, indole-3-pyruvate, indole-3-acetonitrile pathways and tryptamine (Spaepen et al., 2007), although some intermediaries may differ most pathways show similarity to those described in plants (Patten and Glick, 1996; Woodward and Bartel, 2005). IAA synthesis pathways have been identified using several biochemical and genetic methods; however, a small set of genes and enzymes involved in these pathways have been characterized (Spaepen and Vanderleyden, 2011).
The interaction between IAA concentration and plant growth correlation is not linear, and plants should have optimal levels of endogenous IAA for optimal development (Duca et al., 2014). Excessive amounts of IAA can promote harmful effects on plants, decreasing root growth (Duca et al., 2014). Therefore, to stimulate plant growth using IAA it must be carefully regulated to avoid inhibitory effects caused by overdosing (Duca et al., 2014). In this context, plants have many mechanisms of neutralization to control excess IAA, such as amino acids production (Sitbon et al., 1992). However, plants are not always able to carry out neutralization and are sometimes harmed by excess IAA (Duca et al., 2014).
Aeromonas punctata (Iqbal and Hasnain, 2013), Azospirillum brasilense (Camilios-Neto et al., 2014), Bacillus subtilis (Tahir et al., 2017), and Burkholderia phytofirmans (Poupin et al., 2016) are some bacterial species that efficiently synthesize IAA.
Cytokinins are responsible for the formation of shoots, inhibition of root elongation, and the improvement of cell division and root development; cytokinins are also a type of growth regulator (Porcel et al., 2014; Jha and Saraf, 2015). In particular, they are mandatory for the progression of the cell cycle (Schaller et al., 2015). Furthermore, the balance between auxins and cytokinins determines the function of the meristem, the architecture of the root system, the formation of lateral organs of shoots and the development of reproductive organs (Schaller et al., 2015). Cytokinins can regulate chloroplast biogenesis and chlorophyll biosynthesis (Cortleven and Schmulling, 2015), and they are related to development of plant resistance to abiotic stressors and biotic (Grosskinsky et al., 2011; O'Brien and Benkova, 2013).
Rhizobacteria can produce several types of cytokines, of which zeatin and kinetin are the most abundant (O'Brien and Benkova, 2013). Rhizobacteria are able to synthesize zeatin in two different ways: directly and indirectly. The direct pathway involves the synthesis of dimethylalyl diphosphate and isopentenyl adenosine monophosphate, while the indirect pathway involves cis-zeatin that contains tRNA to release cytokinins (Tabassum et al., 2017).
Several Paenibacillus polymyxa, Azotobacter species., Rhizobium species., B. subtilis, Rhodospirillum rubrum, Pseudomonas fluorescens, Pantoea agglomerans, strains are capable of producing cytokines (de Salamone et al., 2001; Glick, 2012).
Gibberellins are another essential class of phytohormones released by rhizobacteria; these compounds are responsible for different processes in higher plants, such as seed germination, stem elongation and fruiting, the flowering process (Saleem et al., 2015). Gibberellins positively regulate cell division and elongation, stimulating the growth of the hypocotyl and stem, and they have a positive effect on root and leaf meristem size (Martínez et al., 2018). Gibberellin can promote xylem increase and shoot growth and can also decrease root growth (Guo et al., 2015; Wang et al., 2015).
Studies have shown that some plants with gibberellin-producing bacteria in their rhizospheres exhibit better growth rates (Poupin et al., 2013; Vacheron et al., 2013). Some gibberellin-producing bacterial species include Bacillus amyloliquefaciens (Shahzad et al., 2016), Enterococcus faecium (Wang et al., 2015), Sphingomonas spp. (Khan et al., 2014), and Bacillus pumilus (Joo et al., 2004).
At low concentrations, ethylene has been proven to be potentially active in fruit and leaf maturation, seed germination, leaf senescence, flower wilting, flower initiation, root elongation and branching, nodule formation, and leaf abscission (Reid, 1988). At relatively high concentrations, ethylene can be toxic to plants, causing defoliation, root growth inhibition and premature senescence (Vacheron et al., 2013). When plants experience various stresses, such as infection, flooding, drought, and even the presence of potentially toxic metals, they produce ethylene precursors, that is, 1-aminocyclopropane-1-carboxylate (ACC) (Reid, 1988; Li et al., 2005; Liu et al., 2013).
ACC deaminase (ACCD) is an enzyme synthesized by some rhizobacteria; this enzyme can benefit plants due to its action in reducing ACC levels, transform it into ammonia and α-ketobutyrate (Belimov et al., 2001). This breakage allows to regulate the adverse effects of excess ethylene, reducing its levels. Therefore, rhizobacteria capable of producing ACCD are beneficial for plants growing under stress conditions, such as high salinity (Mayak et al., 2004) drought (Sandhya et al., 2010), and the presence of potentially toxic metals (Belimov et al., 2001), regulating plant ACC levels and reducing the levels of ethylene to non-toxic levels.
Azotobacter spp. (Dubey et al., 2012; Farajzadeh et al., 2012), Bacillus spp. (Belimov et al., 2001), and Pseudomonas spp. (Sandhya et al., 2010; Kamran et al., 2016) are several species known for their production of ACCD.
Abscisic acid (ABA) is a phytohormone related in mediating stomatal opening (Mansfield et al., 1990; Maksimov et al., 2011) and also various plant aspects including development and growth in the absence of stress (Cheng et al., 2002). Abscisic acid is a molecule produced by plants, higher fungi, algae, and bacteria (Zeevaart, 1999).
Water stress stimulates high levels of ABA biosynthesis in plants, which causes partial stomatal closure as an adaptive response to conserve water (Dodd, 2007). ABA can also affect the inhibition of seed germination, the of induction of plant senescence and the abscission of fruits and leaves (Munemasa et al., 2015; Sah et al., 2016).
ABA has the abilities to reduce plant growth, even though a certain amount of ABA is required for growth, since this hormone regulates stomatal opening and, therefore, CO2 absorption and loss of water (Pospisilova, 2003). Some PGPR can reduce ABA levels in host plants and indirectly increase plant growth (Belimov et al., 2014); these positive effects depend on endogenous ABA levels in the host plant (Vacheron et al., 2015).
Many rhizobacteria produce ABA in culture media and mediate the ABA status of plants (Dodd et al., 2010). Achromobacter xylosoxidans (Forchetti et al., 2007; Sgroy et al., 2009), A. brasilense (Cohen et al., 2009), Bacillus licheniformis, B. subtilis, Brevibacterium halotolerans, and Pseudomonas putida (Sgroy et al., 2009) are some of these rhizobacteria.
Nitrogen (N) is the most limiting nutrient for plant development and can be assimilated from the soil in the form of ammonia, nitrate and nitrite (Gopalakrishnan et al., 2017). These N forms are not abundant in most soils, and chemical N fertilizer used in agriculture is often lost because of rain or by mineral leaching of nitrogen fertilizer (Perez-Montano et al., 2014).
Under these circumstances, bacteria play a fundamental role because some bacteria can perform biological nitrogen fixation (BNF) and N-fixing microorganisms are classified into two groups: symbiotic microorganisms and free-living microorganisms (Gopalakrishnan et al., 2017). BNF is carried out by a specific gene product called nif, which, together with other structural genes, participates in protein iron activation, electron transfer biosynthesis of the molybdenum iron cofactor and many other regulatory processes required for enzyme synthesis and activity (Reed et al., 2011).
Bacteria that perform symbiosis, such as Rhizobium and Bradyrhizobium, act by forming nodules on the roots of plant species such as soybean, pea, peanut, and alfalfa, converting N2 into ammonia, which can be used by the plant as a source of N (Murray, 2011).
Inside plant cells, rhizobia undergo a differentiation process that generates the specialized form for N fixation—the bacteroid (Olanrewaju et al., 2017). One or more bacteroids then become surrounded by parts of the plant cell membrane to form a so-called symbiosome (Madigan and Martinko, 2006). When the symbiosome is formed do the bacteroids convert atmospheric N through the enzyme nitrogenase into ammonia, in return, the plant provides organic acids (for bacteroids to produce energy) and provides an appropriate microenvironment for the action of nitrogenase, thus establishing a symbiotic relationship with bacteria (Madigan and Martinko, 2006; Olanrewaju et al., 2017).
Free-living bacteria are able to interact with roots; they live close to them so that the nitrogen fixed by these bacteria can be easily absorbed by plants and so that they the bacteria feed on root exudates (amino acids, peptides, proteins, enzymes, vitamins, and hormones) (Tabassum et al., 2017).
Some examples of free-living N-fixing bacteria include Azotobacter, Paenibacillus, Burkholderia, Bacillus and Azospirillum, Herbaspirillum (Huang et al., 2012; Anand et al., 2013; Angus et al., 2013; Habibi et al., 2014; Geddes et al., 2015; Goswami et al., 2016).
Phosphorus (P) is another essential nutrient for plants and plays an important role in nearly all major metabolic processes, including, signal transduction, energy transfer, respiration, photosynthesis, macromolecular biosynthesis (Anand et al., 2016). Although the P reserve in soils is large, it is present mainly in the form of insoluble compounds that cannot be absorbed by plants, limiting their growth. Plants absorb phosphate only in the form of monobasic (H2) and dibasic ions () (Perez-Montano et al., 2014).
Microorganisms play an important role in P transformation in the soil, including the P solubilization required for plant growth (Rodriguez and Fraga, 1999).
The ability to solubilize and mineralize P by phosphate-solubilizing bacteria is an important characteristic (Oteino et al., 2015). Several low-molecular-weight organic acids synthesized by various soil bacteria are capable of solubilizing inorganic P (Sharma et al., 2013). Members of the genera Arthrobacter, Bacillus, Beijerinckia, Burkholderia, Enterobacter, Microbacterium, Pseudomonas, Erwinia, Rhizobium, Mesorhizobium, Flavobacterium, Rhodococcus, and Serratia have the ability to solubilize phosphates (Oteino et al., 2015).
The phosphate solubilization is based on the secretion of organic acids by microorganisms due to sugar metabolism and organisms living in the rhizosphere use sugars from root exudates (Goswami et al., 2014). The acids released by microorganisms act as good chelators of divalent Ca2+ ions that follow the release of phosphates from insoluble compounds. Many phosphate-solubilizing microorganisms lower the pH of media by secreting organic acids such as acetic acid, lactic acid, malic acid, succinic acid, tartaric acid, gluconic acid, 2-ketogluconic acid, oxalic acid and citric acid (Rodriguez and Fraga, 1999; Patel et al., 2015).
Organic P solubilization is also called organic P mineralization and plays an essential role in the phosphorus cycling of an agricultural system (Khan et al., 2007). P can be released from organic compounds in the soil by enzymes such as non-specific acid phosphatases, phytases, phosphonatases and C-P lyases (Sharma et al., 2013).
Potassium (K) is the third most important macronutrient required for plant growth and this element plays a vital role in various plant physiological and metabolic processes (Zhao et al., 2001), including photosynthesis (Wang et al., 2012), plant growth, metabolism, assimilation, sugar accumulation, and plant growth and development (Sparks and Huang, 1985). Since more than 90% of K is present in the form of insoluble minerals of rock and silicate, the soluble K concentration is generally very low in soils (Parmar and Sindhu, 2013).
K-solubilizing bacteria, such as Acidothiobacillus spp., Bacillus edaphicus, Bacillus mucilaginosus, Pseudomonas spp., Burkholderia spp., and Paenibacillus spp., have been reported for their action of solubilizing K into assimilable forms from K minerals in the soil (Liu et al., 2012).
As in the case of P solubilization, the main mechanism of K solubilization is the production of organic acids, inorganic acids and protons (the acidolysis mechanism) (Sheng et al., 2008; Parmar and Sindhu, 2013; Maurya et al., 2014; Meena et al., 2015), which are capable of converting insoluble K (mica, muscovite, and feldspar biotite) into soluble forms of K that are easily absorbed by plants (Hu et al., 2006; Mo and Lian, 2011).
Several organic acids involved in the solubilization of insoluble K, tartaric acid, succinic acid, α-ketogluconic acid, citric acid, and oxalic acid are the most important ones released by K-solubilizing bacteria (Meena et al., 2014).
The indirect action of microorganisms to promote plant growth includes the production of biocontrol agents that inactivate or kill pathogens, providing a healthy environment for plants (Naik et al., 2019).
Antibiosis, competition, production of lytic enzymes (chitinases and glucanases) with the ability to hydrolyze fungal cell walls are considered indirect mechanisms of growth promotion (Bhattacharyya and Jha, 2012). Bacteria can also indirectly improve plant growth by suppressing pathogens and increasing plant innate immunity against pathogens (Tabassum et al., 2017).
Antibiotics are low-molecular-weight toxins produced by the bacterial community are able to eliminate or reduce the growth of other microorganisms (Bakker et al., 2013). Both antibiotics and toxins have been identified as being produced by certain rhizospheric bacteria (Nakkeeran et al., 2013). These include amphysines, phenazines, 2-4-diacetylfloroglucinol, pioluteorin, pyrrolnitrin, hydrogen cyanide (HCN), oomycins, polymyxin, circulin, colistin, tensin, tropolone, and cyclic lipopeptides (Maksimov et al., 2011; Pandya and Saraf, 2014; Wani and Khan, 2014; Sherathia et al., 2016).
Bacteria can produce only a single antibiotic substance, while others can secrete several substances (Reimer and Bode, 2014; Majed et al., 2016). The availability of nutrients and environmental stimuli within proximity strongly affect the synthesis of antibiotics (Choudhary et al., 2007).
Rhizobacteria of the genus Bacillus are the most important for the production of antibiotics (Jayaprakashvel and Mathivanan, 2011). B. amyloliquefaciens and B. subtilis are described as producers of a wide variety of antibacterial and antifungal antibiotics, including subtilin, bacillisin, and emicobacillin (Leclere et al., 2005; Chang et al., 2007).
ISR is described as an enhanced defensive capability of plants in response to various pathogens induced by beneficial microorganisms present in the rhizosphere (Conrath et al., 2015), a phenomenon in which the interaction of some microorganisms with roots results in plant resistance to some pathogenic bacteria, viruses, and fungi (Lugtenberg and Kamilova, 2009). ISR can also be triggered by specific environmental stimuli that lead to the upregulation of plants' innate defenses against biotic challenge and this heightened state of alert allows plants to respond faster and stronger against subsequent attack by pathogens (Van Loon, 1997).
ISR is stimulated by non-pathogenic microorganisms; ISR begins in the roots and extends to the shoots (Solano et al., 2008), initiates the defense mechanisms of plants and protects unexposed parts of plants against future pathogenic attacks by microorganisms and insects. This defense response depends on the signaling of ethylene and jasmonic acid in the plant (van Loon, 2007; Pieterse et al., 2012).
ISR has been reported to be one of the mechanisms by which PGPR can reduce the occurrence of some plant diseases, modulating the physical and biochemical properties of host plants and consequently promoting plant growth (Pieterse et al., 2014).
Some of the defense mechanisms produced via ISR in plants include reinforcement of cell walls (Ahn et al., 2007), production of secondary metabolites (Choudhary et al., 2007) and accumulation of defense-related enzymes, such as chitinases, glucanases, peroxidase, phenylalanine ammonia-lyase and polyphenol oxidase (Bhattacharyya and Jha, 2012).
P. fluorescens (Pieterse et al., 1996), Burkholderia phytofirmans (Compant et al., 2005), B. pumilus (Benhamou et al., 1996), Bacillus cereus (Conn et al., 2008), Rhizobium leguminosarum, P. putida and Serratia marcescens (Bhattacharyya and Jha, 2012) are examples of rhizobacteria that undergo ISR.
Iron, which is one of the most abundant elements on Earth, is not available for direct assimilation by plants and microorganisms because, in nature, it occurs mainly as Fe3+ and is generally present in the form of insoluble hydroxides and oxy-hydroxides (Rajkumar et al., 2010).
To obtain iron for their growth and development, some bacteria synthesize low-molecular-weight iron-chelating molecules called siderophores (Shaikh and Sayyed, 2015; Mhlongo et al., 2018). Siderophore-producing bacteria can stimulate plant growth directly, improving plant Fe nutrition, or indirectly, inhibiting the activity of plant pathogens in the rhizosphere, which in turn limits their Fe availability (Ma et al., 2011).
Pathogen suppression caused by the production of siderophores occurs by restricting the survival of pathogens by inhibiting iron nutrition by chelating available iron (Chaiharn et al., 2009). In other words, solubilization and the competitive acquisition of iron under limiting conditions reduces the availability of iron to other soil inhabitants, subsequently limiting their growth (Haas and Defago, 2005).
In addition to iron, there is evidence indicating that siderophores form stable compounds with other potentially toxic metals, such as Al, Cd, Cu, Pb, and Zn (Gururani et al., 2013). This phenomenon is advantageous for alleviating plant stress caused by potentially toxic metals present in polluted soils Ahemad and Kibret (2014) and is not solely due to the increased availability of mineral nutrients for plants (Babu et al., 2013).
Bacterial siderophores can be classified into four main classes based on the type of ligand and basic characteristics of the functional groups associated with iron. The main classes include catecholates, carboxylate, and hydroxamates (Crowley, 2006).
Several bacterial species are capable of producing siderophores, including, Azospirillum (Banik et al., 2016), Dickeya (Sandy and Butler, 2011), Klebsiella (Zhang et al., 2017; Bailey et al., 2018), Nocardia (Hoshino et al., 2011; Soutar and Stavrinides, 2018), Pantoea (Burbank et al., 2015), Pseudomonas (Baune et al., 2017; Deori et al., 2018; Pourbabaee et al., 2018), Azotobacter (Romero-Perdomo et al., 2017), Paenibacillus (Liu et al., 2017), Bacillus (Kesaulya et al., 2018; Pourbabaee et al., 2018), Serratia (Lee et al., 2017) and Streptomyces (Schutze et al., 2015; Gáll et al., 2016; Goudjal et al., 2016).
Plants are often exposed to various environmental stresses, and plant growth is sometimes inhibited by a large number of biotic (insects, bacteria, fungi, and viruses) and abiotic (radiation, salinity, temperature, flood, drought, and contaminants,) stresses, resulting in highly negative impacts on survival and plant biomass (Islam et al., 2016).
Phytoremediation uses plants and microorganisms to remove, destroy or scavenge toxic metals from contaminated environments in an efficient and economical way (Ma et al., 2011).
Most potential metals are toxic to plants, but bacteria are capable of neutralizing metal toxicity, linking it to negatively charged functional groups throughout the cell wall, which provides interactions with positive ions, in particular metal ions—a phenomenon called metal biosorption (Syed and Chinthala, 2015). Microbial species with considerable resistance to metals showed immobilization of toxic metals or reducing its concentration when added to contaminated soils by reducing their toxicity to the plant or crop (Wani and Khan, 2014).
Many bacteria rely on chemical communication to recognize the environment and retrieve information about population density. Consequently, multiple molecules are released, which synchronize the expression of genes, coordinate behavior through a process called quorum sensing (QS) and determine the relationships with eukaryotic species (Ortiz-Castro and Lopez-Bucio, 2019). QS is considered a social trait of bacteria (Whiteley et al., 2017).
Communication between cells is mediated by small molecules of diffusible signals called self-inducers (Fuqua et al., 1994). Generally, signaling mediated by self-inducers occurs at high population densities because microorganisms act in communities where there are advantages for the entire population of cells, simulating a multicellular organism (Ganin et al., 2009; Bai and Rai, 2011).
While N-acyl-hemoserine-lactones (AHLs) and occasionally 4-hydroxy-2-alkylquinolones (HAQs) are often found in gram-negative bacteria, gram-positive bacteria mainly use cyclic oligopeptides. AHLs are the most common self-inducing molecules that regulate the expression of genes involved in the production of virulence factors or biofilm formation in various plant pathogens (Quiñones et al., 2005). Many plants are capable of producing molecules that specifically interfere with QS systems of bacteria associated with plants, and in any case, depending on whether the bacterium is detected as a pathogen or as a beneficial microorganism, the molecule improves or inhibits QS-regulated phenotypes (Pérez-Montaño et al., 2013).
Maize (Zea mays L.), which is a member of the Poaceae family, is one of the most important cereal crop species in the world and serves as a staple food for many populations (Rouf Shah et al., 2016). According to the International Grains Council (2019), global maize consumption is expected to reach new peaks in the coming years (projection until 2024), and the use of maize for animal feed is expected to increase during the same period.
Maize is among the three most important crop species in the world, providing almost half of the daily energy to organisms in Africa and the Americas (FAOSTAT Food Balance Sheets, 2020). The demand for maize in response to growing populations will require marked increases in the production, sustainability, and resilience of maize-based agricultural systems (Shiferaw et al., 2011).
To maintain increases in maize productivity, an increase in the amount of fertilizers will be necessary, resulting in both an increase in production costs and a greater negative impact on the environment. Many beneficial effects of plant growth-promoting rhizobacteria on crop growth and yield have been well-documented. Breedt et al. (2017) found an increase yield ranging from 24 to 34% using Paenibacillus alvei, B. safensis, B. pumilus, and Brevundimonas vesicularis. Cassán et al. (2009) assessed the effect of the mixture of A. brasilense with Bradyrhizobium japonicum and verified the increase of seeds germination rate, and early development. Kuan et al. (2016) reported that plant growth-promoting bacteria may provide a biological alternative to fix atmospheric N2 and delay N remobilization in maize plant to increase crop yield based on an understanding that plant-N remobilization is directly correlated to its plant senescence promoting high ear up to 30.9% with reduced fertilizer-N input. Di Salvo et al. (2018) reported that PGPR used as inoculants of cereal crops including maize can improve their growth and grain yield. The crops responses to inoculation are complex because are defined by plant-microorganisms interactions, many of them still unknown. Thus, it is necessary to improve the knowledge about the microbial ecology of the rhizosphere of crops under different agricultural practices.
Several bacteria that have the ability to produce IAA and have positive effects on shoot and root weight and nutrient uptake on maize plants. Besides, activities like phosphorus solubilization, or even other non-evaluated PGPR traits that stimulate plant growth (Lobo et al., 2019).
The bioprotective role of PGPR on maize crops has also been studied. The toxigenic fungus Fusarium is one of the significant genera associated with maize. Some PGPR such as Bacillus amyloliquefaciens and Microbacterium oleovorans were able to protect maize against Fusarium verticillioides when applied in the form of seed coatings (Pereira et al., 2011). Interestingly, some PGPR species have appeared to promote plant growth by acting as both biofertilizers and as biocontrol agents. For instance, strains of B. cepacia have been observed with biocontrol characteristics against Fusarium spp. Simultaneously, they can also stimulate the growth of maize under iron-poor conditions via siderophore production (Bevivino et al., 1998).
Sugarcane (a hybrid of Saccharum species) is one of the oldest and most valuable crop species in the world due to the vast benefits associated with its industrial use. Sugarcane is grown in tropical and subtropical regions (Chhabra et al., 2016) and is mainly used to supply raw materials to the sugar industry for the production of sugar (Zhao and Li, 2015). In addition, sugarcane also has global importance due to the benefits associated with the production of biofuel and biogas (Hoang et al., 2015).
New technologies developed in all production sectors have allowed extending the period of sugarcane planting and, consequently, more efficient use of labor and inputs, thus increasing the sustainability and competitiveness of the sugar and alcohol industries (Oliveira et al., 2012).
Among the various techniques available, PGPR stand out for their environmental and economic gains, which can reduce the amount of fertilizers needed, optimizing sugarcane production.
One of the most limitations for sugarcane production is the poor and inadequate fertile soil which fail to meet the nutritional and growth requirements and hence unable to achieve high production (Caione et al., 2015). Phosphorus (P) is the most critical element with high interaction with soil Raj and Antil (2011) required in a small amount by sugarcane in comparison of N and K, but still plays an essential role in the development of tillering and root system and greatly influence the longevity (Kingston, 2013).
The low availability of phosphorus is due to low P in the source material, clay absorption and its precipitation with oxides and hydroxides of iron and aluminum (Caione et al., 2015). As consequence of it, a great amount of P fertilizers is applied in sugarcane production promoting high production cost. Furthermore, phosphate fertilizers are produced by rock phosphate and the phosphate source worldwide are not renewable (Sattari et al., 2012).
Around 10–30% of the phosphorus fertilizer applied in the first year is absorbed by the roots of cane crop whereas an intensive amount accumulates in the soil as fixed P, not available to plants (Syers et al., 2008). Therefore, is urgent to find alternatives to reduce the use of phosphate fertilizers. Since every day looking to a more sustainable agriculture combined with increase in yield and economically practicable.
The use of plant growth-promoting rhizobacteria (PGPR) is a promising alternative in sugarcane production with low environmental impact to increase the efficiency of the use of mineral fertilizers including phosphate, providing high cost-effective yields (Spolaor et al., 2016).
Several field and greenhouse studies with phosphate fertilizer in sugarcane (Calheiros et al., 2012; Caione et al., 2015; Albuquerque et al., 2016; Borges et al., 2019).
Rosa et al. (2020) evaluated the effect of inoculation with three PGPR species and five P doses in sugarcane and reported that the inoculation can play a fundamental role in cultivation, generating great benefits to the crop and saving fertilizers cost for the producers. These results revealed a combination of Azospirillum brasilense and Bacillus subtilis allied to the low cost of P2O5 was the best fertilizers management in the sugarcane which is meaningful production practice of sugarcane.
Santos et al. (2018) reported that the use of B. subtilis together with by-products can improve soil fertility parameters and decrease adverse effects associated with vinasse fertilization, in addition to providing shoot and root growth and providing collective synergy for high yield of sugarcane production with environmental benefits. Moura et al. (2018) have shown that the use of Azospirillum in sugarcane crop improved root system leading to better water and nutrient uptake that in turn may influence yield positively. This report showed that the significant interaction of cultivar x water regime x Azospirillum inoculation suggests a complex interplay of these factors, likewise, involving indigenous plant auxin pool. Li et al. (2017) isolated Pseudomonas sp associated with sugarcane rhizosphere and verified useful activity the isolate such as phosphate solubilization, siderophore production, ACC deaminase activity, and IAA production, as well as N2 fixing activity and diseases management. These features are measured as important PGP traits and have been found to be effective in improving the growth and nitrogen content of sugarcane plants. The association of PGPR in sugarcane production may be an eminent development biofertilizer application, for sustainable crops production, in reducing environmental pollution and in biological agri-business. (Muthukumarasamy et al., 2017) verified that the association between diazotrophic P and K solubilizing Rosneateles terrae and Burkholderia gladioli with sugarcane were able to increase the leaf chlorophyll, N content and total biomass and encourage the farmers to use PGPR to improve N, K, and K availability in the soil.
This review has focused on a heterogeneous group of microorganisms found in the rhizosphere. These microorganisms live in association with roots, can stimulate plant growth and can reduce the incidence of diseases. Among the large number of PGPR, the most studied genera include Azospirillum, Bacillus, and Pseudomonas. The important role that PGPR play in agriculture is proven by the large number of publications on this topic to date. The exact mechanisms used by PGPR are not yet fully known, although some characteristics of these bacteria can be used to promote plant development. In addition, for these growth-promoting characteristics to have an effect on plants, bacteria need to be rhizosphere competent and must be able to survive in rhizospheric soil, where communities can be affected by a large number of factors, such as soil characteristics, plant genotype, and agricultural practices, that together determine the presence and predominance of certain microbial groups. Growth promotion of maize and sugarcane could be optimized with appropriate combinations of PGPR, environmental conditions and plant genotypes. In this sense, additional efforts must be made in the development of good inoculants and production systems that allow reducing the amount of chemical fertilizers and insecticides used to increase soil fertility and crop productivity.
All authors listed have made a substantial, direct and intellectual contribution to the work, and approved it for publication.
The authors declare that the research was conducted in the absence of any commercial or financial relationships that could be construed as a potential conflict of interest.
Ahemad, M., and Kibret, M. (2014). Mechanisms and applications of plant growth promoting rhizobacteria: current perspective. J. King Saud Univ. 26, 1–20. doi: 10.1016/j.jksus.2013.05.001
Ahmad, F., Ahmad, I., Aqil, F., Ahmed Wani, A., and Sousche, Y. S. (2016). Plant growth promoting potential of free-living diazotrophs and other rhizobacteria isolated from Northern Indian soil. Biotechnol. J. 1, 1112–1123. doi: 10.1002/biot.200600132
Ahn, I. P., Kim, S., Lee, Y. H., and Suh, S. C. (2007). Vitamin B-1-induced priming is dependent on hydrogen peroxide and the NPR1 gene in Arabidopsis. Plant Physiol. 143, 838–848. doi: 10.1104/pp.106.092627
Albuquerque, A. W. D., Sá, L. D. A., Rodrigues, W. A., Moura, A. B., and Oliveira Filho, M. D. S. (2016). Growth and yield of sugarcane as a function of phosphorus doses and forms of application. Rev. Bras. Eng. Agr. Amb. 20, 29–35. doi: 10.1590/1807-1929/agriambi.v20n1p29-35
Aloo, B. N., Makumba, B. A., and Mbega, E. R. (2019). The potential of Bacilli rhizobacteria for sustainable crop production and environmental sustainability. Microbiol. Res. 219, 26–39. doi: 10.1016/j.micres.2018.10.011
Alori, E. T., Babalola, O. O., and Prigent-Combaret, C. (2019). Impacts of microbial inoculants on the growth and yield of maize plant. Open Agric. J. 13, 1–8. doi: 10.2174/1874331501913010001
Anand, K., Kumari, B., and Mallick, M. A. (2016). Phosphate solubilizing microbes: an effective and alternative approach as biofertilizers. J. Pharm. Pharm. Sci. 8, 37–40.
Anand, R., Grayston, S., and Chanway, C. (2013). N-2-fixation and seedling growth promotion of lodgepole pine by endophytic Paenibacillus polymyxa. Microb. Ecol. 66, 369–374. doi: 10.1007/s00248-013-0196-1
Angus, A. A., Lee, A., Lum, M. R., Shehayeb, M., Hessabi, R., Fujishige, N. A., et al. (2013). Nodulation and effective nitrogen fixation of Macroptilium atropurpureum (siratro) by Burkholderia tuberum, a nodulating and plant growth promoting beta-proteobacterium, are influenced by environmental factors. Plant Soil 369, 543–562. doi: 10.1007/s11104-013-1590-7
Babu, A. G., Kim, J. D., and Oh, B. T. (2013). Enhancement of heavy metal phytoremediation by Alnus firma with endophytic Bacillus thuringiensis GDB-1. J. Hazard. Mater. 250–251, 477–483. doi: 10.1016/j.jhazmat.2013.02.014
Bai, A. J., and Rai, V. R. (2011). Bacterial quorum sensing and food industry. Compr. Rev. Food Sci. Food Saf. 10, 184–194. doi: 10.1111/j.1541-4337.2011.00150.x
Bailey, D. C., Alexander, E., Rice, M. R., Drake, E. J., Mydy, L. S., Aldrich, C. C., et al. (2018). Structural and functional delineation of aerobactin biosynthesis in hypervirulent Klebsiella pneumoniae. J. Biol. Chem. 293, 7841–7852. doi: 10.1074/jbc.RA118.002798
Bakker, P., Berendsen, R. L., Doornbos, R. F., Wintermans, P. C. A., and Pieterse, C. M. J. (2013). The rhizosphere revisited: root microbiomics. Front. Plant Sci. 4:7. doi: 10.3389/fpls.2013.00165
Banik, A., Mukhopadhaya, S. K., and Dangar, T. K. (2016). Characterization of N-2-fixing plant growth promoting endophytic and epiphytic bacterial community of Indian cultivated and wild rice (Oryza spp.) genotypes. Planta 243, 799–812. doi: 10.1007/s00425-015-2444-8
Bashan, Y., and Holguin, G. (1997). Azospirillum-plant relationships: environmental and physiological advances (1990-1996). Can. J. Microbiol. 43, 103–121. doi: 10.1139/m97-015
Baune, M., Qi, Y. L., Scholz, K., Volmer, D. A., and Hayen, H. (2017). Structural characterization of pyoverdines produced by Pseudomonas putida KT2440 and Pseudomonas taiwanensis VLB120. Biometals 30, 589–597. doi: 10.1007/s10534-017-0029-7
Belimov, A. A., Dodd, I. C., Safronova, V. I., Dumova, V. A., Shaposhnikov, A. I., Ladatko, A. G., et al. (2014). Abscisic acid metabolizing rhizobacteria decrease ABA concentrations in planta and alter plant growth. Plant Physiol. Biochem. 74, 84–91. doi: 10.1016/j.plaphy.2013.10.032
Belimov, A. A., Safronova, V. I., Sergeyeva, T. A., Egorova, T. N., Matveyeva, V. A., Tsyganov, V. E., et al. (2001). Characterization of plant growth promoting rhizobacteria isolated from polluted soils and containing 1-aminocyclopropane-1-carboxylate deaminase. Can. J. Microbiol. 47, 642–652. doi: 10.1139/w01-062
Benhamou, N., Kloepper, J. W., Hallman, A., and Tuzun, S. (1996). Induction of defense-related ultrastructural modifications in pea root tissues inoculated with endophytic bacteria. Plant Physiol. 112, 919–929. doi: 10.1104/pp.112.3.919
Bevivino, A., Sarrocco, S., Dalmastri, C., Tabacchioni, S., Cantale, C., and Chiarini, L. (1998). Characterization of a free-living maize-rhizosphere population of Burkholderia cepacia: Effect of seed treatment on disease suppression and growth promotion of maize. FEMS Microbiol. Ecol. 27, 225–237. doi: 10.1016/S0168-6496(98)00069-5
Bhattacharyya, P. N., and Jha, D. K. (2012). Plant growth-promoting rhizobacteria (PGPR): emergence in agriculture. World J. Microbiol. Biotechnol. 28, 1327–1350. doi: 10.1007/s11274-011-0979-9
Borges, B. M. M. N., Abdala, D. B., de Souza, M. F., Viglio, L. M., Coelho, M. J. A., Pavinato, P. S., et al. (2019). Organomineral phosphate fertilizer from sugarcane byproduct and its effects on soil phosphorus availability and sugarcane yield. Geoderma. 339, 20–30. doi: 10.1016/j.geoderma.2018.12.036
Breedt, G., Labuschagne, N., and Coutinho, T. A. (2017). Seed treatment with selected plant growth-promoting rhizobacteria increases maize yield in the field. Ann. Appl. Biol. 171, 229–236. doi: 10.1111/aab.12366
Bulgarelli, D., Schlaeppi, K., Spaepen, S., van Themaat, E. V. L., and Schulze-Lefert, P. (2013). Structure and functions of the bacterial microbiota of plants. Annu. Rev. Plant Biol. 64, 807–838. doi: 10.1146/annurev-arplant-050312-120106
Burbank, L., Mohammadi, M., and Roper, M. C. (2015). Siderophore-mediated iron acquisition influences motility and is required for full virulence of the xylem-dwelling bacterial Phytopathogen Pantoea stewartii subsp. stewartii. Appl. Environ. Microbiol. 81, 139–148. doi: 10.1128/AEM.02503-14
Caione, G., Prado, R. D. M., Campos, C. N. S., Rosatto Moda, L., de Lima Vasconcelos, R., and Pizauro Júnior, J. M. (2015). Response of sugarcane in a red ultisol to phosphorus rates, phosphorus sources, and filter cake. Sci. World J. 2015, 1–10. doi: 10.1155/2015/405970
Calheiros, A. S., de Oliveira, M. W., Ferreira, V. M., de Souza Barbosa, G. V., Santiago, A. D., and dos Santos Aristides, E. V. (2012). Production of biomass, from sugar and protein in function of sugarcane varieties and phosphorous fertilization. Semina Ciênc Agrár. 33, 809–818. doi: 10.5433/1679-0359.2012v33n2p809
Camilios-Neto, D., Bonato, P., Wassem, R., Tadra-Sfeir, M. Z., Brusamarello-Santos, L. C. C., Valdameri, G., et al. (2014). Dual RNA-seq transcriptional analysis of wheat roots colonized by Azospirillum brasilense reveals up-regulation of nutrient acquisition and cell cycle genes. BMC Genomics 15:13. doi: 10.1186/1471-2164-15-378
Cassán, F., Perrig, D., Sgroy, V., Masciarelli, O., Penna, C., and Luna, V. (2009). Azospirillum brasilense Az39 and Bradyrhizobium japonicum E109, inoculated singly or in combination, promote seed germination and early seedling growth in corn (Zea mays L.) and soybean (Glycine max L.). Eur. J. Soil Biol. 45, 28–35. doi: 10.1016/j.ejsobi.2008.08.005
Chaiharn, M., Chunhaleuchanon, S., and Lumyong, S. (2009). Screening siderophore producing bacteria as potential biological control agent for fungal rice pathogens in Thailand. World J. Microbiol. Biotechnol. 25, 1919–1928. doi: 10.1007/s11274-009-0090-7
Chandra, P., Tripathi, P., and Chandra, A. (2018). Isolation and molecular characterization of plant growth-promoting Bacillus spp. and their impact on sugarcane (Saccharum spp. hybrids) growth and tolerance towards drought stress. Acta Physiol. Plant. 40:199. doi: 10.1007/s11738-018-2770-0
Chang, W. T., Chen, Y. C., and Jao, C. L. (2007). Antifungal activity and enhancement of plant growth by Bacillus cereus grown on shellfish chitin wastes. Bioresour. Technol. 98, 1224–1230. doi: 10.1016/j.biortech.2006.05.005
Cheng, W. H., Endo, A., Zhou, L., Penney, J., Chen, H. C., Arroyo, A., et al. (2002). A unique short-chain dehydrogenase/reductase in Arabidopsis glucose signaling and abscisic acid biosynthesis and functions. Plant Cell 14, 2723–2743. doi: 10.1105/tpc.006494
Chhabra, M. L., Parameswari, B., and Viswanathan, R. (2016). Pathogenic behaviour pattern of Colletotrichum falcatum isolates of sugarcane in sub-tropical India. Vegetos 29:76. doi: 10.5958/2229-4473.2016.00103.8
Choudhary, D. K., Prakash, A., and Johr, B. N. (2007). Induced systemic resistance (ISR) in plants: mechanism of action. Indian J. Microbiol. 47, 289–297. doi: 10.1007/s12088-007-0054-2
Cohen, A. C., Travaglia, C. N., Bottini, R., and Piccoli, P. N. (2009). Participation of abscisic acid and gibberellins produced by endophytic Azospirillum in the alleviation of drought effects in maize. Botany 87, 455–462. doi: 10.1139/B09-023
Compant, S., Duffy, B., Nowak, J., Clement, C., and Barka, E. A. (2005). Use of plant growth-promoting bacteria for biocontrol of plant diseases: Principles, mechanisms of action, and future prospects. Appl. Environ. Microbiol. 71, 4951–4959. doi: 10.1128/AEM.71.9.4951-4959.2005
Conn, V. M., Walker, A. R., and Franco, C. M. M. (2008). Endophytic actinobacteria induce defense pathways in Arabidopsis thaliana. Mol. Plant Microbe Interact. 21, 208–218. doi: 10.1094/MPMI-21-2-0208
Conrath, U., Beckers, G. J. M., Langenbach, C. J. G., and Jaskiewicz, M. R. (2015). “Priming for Enhanced Defense,” in Annual Review of Phytopathology, Vol. 53, ed. N. K. VanAlfen (Palo Alto: Annual Reviews), 97–119. doi: 10.1146/annurev-phyto-080614-120132
Cortleven, A., and Schmulling, T. (2015). Regulation of chloroplast development and function by cytokinin. J. Exp. Bot. 66, 4999–5013. doi: 10.1093/jxb/erv132
Council, I. G. (2019). Five-year baseline projections of supply and demand for wheat, maize (corn), rice and soyabeans to 2023/24. London: International Grains Council.
Crowley, D. E. (2006). “Microbial siderophores in the plant rhizosphere,” in Iron Nutrition in Plants and Rhizospheric Microorganisms, eds. L. L. Barton and J. Abadía (Dordrecht: Springer), 169–198. doi: 10.1007/1-4020-4743-6_8
Dakora, F. D., and Phillips, D. A. (2002). “Root exudates as mediators of mineral acquisition in low-nutrient environments,” in Food Security in Nutrient-Stressed Environments: Exploiting Plants' Genetic Capabilities (Netherlands: Springer), 201–213. doi: 10.1007/978-94-017-1570-6_23
Danish, S., Zafar-ul-Hye, M., Mohsin, F., and Hussain, M. (2020). ACC-deaminase producing plant growth promoting rhizobacteria and biochar mitigate adverse effects of drought stress on maize growth. PLoS ONE 15:e0230615. doi: 10.1371/journal.pone.0230615
de Salamone, I. E. G., Hynes, R. K., and Nelson, L. M. (2001). Cytokinin production by plant growth promoting rhizobacteria and selected mutants. Can. J. Microbiol. 47, 404–411. doi: 10.1139/w01-029
Deori, M., Jayamohan, N. S., and Kumudini, B. S. (2018). Production, characterization and iron binding affinity of hydroxamate siderophores from rhizosphere associated fluorescent Pseudomonas. J. Plant Protect. Res. 58, 36–43. doi: 10.24425/119116
Di Salvo, L. P., Cellucci, G. C., Carlino, M. E., and de Salamone, I. E. G. (2018). Plant growth-promoting rhizobacteria inoculation and nitrogen fertilization increase maize (Zea mays L.) grain yield and modified rhizosphere microbial communities. Appl. Soil Ecol. 126, 113–120. doi: 10.1016/j.apsoil.2018.02.010
Dodd, I. C. (2007). Soil moisture heterogeneity during deficit irrigation alters root-to-shoot signalling of abscisic acid. Funct. Plant Biol. 34, 439–448. doi: 10.1071/FP07009
Dodd, I. C., Zinovkina, N. Y., Safronova, V. I., and Belimov, A. A. (2010). Rhizobacterial mediation of plant hormone status. Ann. Appl. Biol. 157, 361–379. doi: 10.1111/j.1744-7348.2010.00439.x
Dubey, R. C., Maheshwari, D. K., Kumar, V., and Pandey, R. R. (2012). Growth enhancement of Sesamum indicum L. by rhizosphere-competent Azotobacter chroococcum AZO2 and its antagonistic activity against Macrophomina phaseolina. Arch. Phytopathol. Plant Protect. 45, 437–454. doi: 10.1080/03235408.2011.587979
Duca, D., Lorv, J., Patten, C. L., Rose, D., and Glick, B. R. (2014). Indole-3-acetic acid in plant-microbe interactions. Antonie Van Leeuwenhoek 106, 85–125. doi: 10.1007/s10482-013-0095-y
FAOSTAT Food Balance Sheets (2020). Available online at: http://www.fao.org/faostat/en/#data/FBS (accessed April 24,2020).
Farajzadeh, D., Yakhchali, B., Aliasgharzad, N., Sokhandan-Bashir, N., and Farajzadeh, M. (2012). Plant growth promoting characterization of indigenous azotobacteria isolated from soils in Iran. Curr. Microbiol. 64, 397–403. doi: 10.1007/s00284-012-0083-x
Forchetti, G., Masciarelli, O., Alemano, S., Alvarez, D., and Abdala, G. (2007). Endophytic bacteria in sunflower (Helianthus annuus L.): isolation, characterization, and production of jasmonates and abscisic acid in culture medium. Appl. Microbiol. Biotechnol. 76, 1145–1152. doi: 10.1007/s00253-007-1077-7
Fuqua, W. C., Winans, S. C., and Greenberg, E. P. (1994). Quorum sensing in bacteria - the luxr-luxi family of cell density-responsive transcriptional regulators. J. Bacteriol. 176, 269–275. doi: 10.1128/JB.176.2.269-275.1994
Gáll, T., Lehoczki, G., Gyemant, G., Emri, T., Szigeti, Z. M., Balla, G., et al. (2016). Optimization of desferrioxamine e production by Streptomyces parvulus. Acta Microbiol. Immunol. Hung. 63, 475–489. doi: 10.1556/030.63.2016.029
Ganin, H., Tang, X., and Meijler, M. M. (2009). Inhibition of Pseudomonas aeruginosa quorum sensing by AI-2 analogs. Bioorg. Med. Chem. Lett. 19, 3941–3944. doi: 10.1016/j.bmcl.2009.03.163
Geddes, B. A., Ryu, M. H., Mus, F., Costas, A. G., Peters, J. W., Voigt, C. A., et al. (2015). Use of plant colonizing bacteria as chassis for transfer of N-2-fixation to cereals. Curr. Opin. Biotechnol. 32, 216–222. doi: 10.1016/j.copbio.2015.01.004
Glick, B. R. (2012). Plant growth-promoting bacteria: mechanisms and applications. Scientifica 2012:963401. doi: 10.6064/2012/963401
Glick, B. R. (2014). Bacteria with ACC deaminase can promote plant growth and help to feed the world. Microbiol. Res. 169, 30–39. doi: 10.1016/j.micres.2013.09.009
Gopalakrishnan, S., Srinivas, V., and Samineni, S. (2017). Nitrogen fixation, plant growth and yield enhancements by diazotrophic growth-promoting bacteria in two cultivars of chickpea (Cicer arietinum L.). Biocatal. Agric. Biotechnol. 11, 116–123. doi: 10.1016/j.bcab.2017.06.012
Goswami, D., Dhandhukia, P., Patel, P., and Thakker, J. N. (2014). Screening of PGPR from saline desert of Kutch: growth promotion in Arachis hypogea by Bacillus licheniformis A2. Microbiol. Res. 169, 66–75. doi: 10.1016/j.micres.2013.07.004
Goswami, D., Thakker, J. N., and Dhandhukia, P. C. (2016). Portraying mechanics of plant growth promoting rhizobacteria (PGPR): a review. Cogent Food Agric. 2:1127500. doi: 10.1080/23311932.2015.1127500
Goudjal, Y., Zamoum, M., Meklat, A., Sabaou, N., Mathieu, F., and Zitouni, A. (2016). Plant-growth-promoting potential of endosymbiotic actinobacteria isolated from sand truffles (Terfezia leonis Tul.) of the Algerian Sahara. Ann. Microbiol. 66, 91–100. doi: 10.1007/s13213-015-1085-2
Grosskinsky, D. K., Naseem, M., Abdelmohsen, U. R., Plickert, N., Engelke, T., Griebel, T., et al. (2011). Cytokinins mediate resistance against pseudomonas syringae in tobacco through increased antimicrobial phytoalexin synthesis independent of salicylic acid signaling. Plant Physiol. 157, 815–830. doi: 10.1104/pp.111.182931
Grossmann, K. (2010). Auxin herbicides: current status of mechanism and mode of action. Pest Manag. Sci. 66, 113–120. doi: 10.1002/ps.1860
Guo, H. Y., Wang, Y. C., Liu, H. Z., Hu, P., Jia, Y. Y., Zhang, C. R., et al. (2015). Exogenous GA(3) application enhances xylem development and induces the expression of secondary wall biosynthesis related genes in Betula platyphylla. Int. J. Mol. Sci. 16, 22960–22975. doi: 10.3390/ijms160922960
Gururani, M. A., Upadhyaya, C. P., Baskar, V., Venkatesh, J., Nookaraju, A., and Park, S. W. (2013). Plant growth-promoting rhizobacteria enhance abiotic stress tolerance in solanum tuberosum through inducing changes in the expression of ROS-scavenging enzymes and improved photosynthetic performance. J. Plant Growth Regul. 32, 245–258. doi: 10.1007/s00344-012-9292-6
Haas, D., and Defago, G. (2005). Biological control of soil-borne pathogens by fluorescent pseudomonads. Nat. Rev. Microbiol. 3, 307–319. doi: 10.1038/nrmicro1129
Habibi, S., Djedidi, S., Prongjunthuek, K., Mortuza, M. F., Ohkama-Ohtsu, N., Sekimoto, H., et al. (2014). Physiological and genetic characterization of rice nitrogen fixer PGPR isolated from rhizosphere soils of different crops. Plant Soil 379, 51–66. doi: 10.1007/s11104-014-2035-7
Halliday, K. J., Martinez-Garcia, J. F., and Josse, E. M. (2009). Integration of light and auxin signaling. Cold Spring Harb. Perspect. Biol. 1:a001586. doi: 10.1101/cshperspect.a001586
Hassan, M. N., Afghan, S., and Hafeez, F. Y. (2010). Suppression of red rot caused by Colletotrichum falcatum on sugarcane plants using plant growth-promoting rhizobacteria. Biocontrol 55, 695. doi: 10.1007/s10526-010-9298-6
Hoang, N. V., Furtado, A., Botha, F. C., Simmons, B. A., and Henry, R. J. (2015). Potential for genetic improvement of sugarcane as a source of biomass for biofuels. Front. Bioeng. Biotechnol. 3:182. doi: 10.3389/fbioe.2015.00182
Hoshino, Y., Chiba, K., Ishino, K., Fukai, T., Igarashi, Y., Yazawa, K., et al. (2011). Identification of nocobactin NA biosynthetic gene clusters in Nocardia farcinica. J. Bacteriol. 193, 441–448. doi: 10.1128/JB.00897-10
Hu, X. F., Chen, J. S., and Guo, J. F. (2006). Two phosphate- and potassium-solubilizing bacteria isolated from Tianmu Mountain, Zhejiang, China. World J. Microbiol. Biotechnol. 22, 983–990. doi: 10.1007/s11274-006-9144-2
Huang, C. J., Tsay, J. F., Chang, S. Y., Yang, H. P., Wu, W. S., and Chen, C. Y. (2012). Dimethyl disulfide is an induced systemic resistance elicitor produced by Bacillus cereus C1L. Pest Manag. Sci. 68, 1306–1310. doi: 10.1002/ps.3301
Iqbal, A., and Hasnain, S. (2013). Auxin producing Pseudomonas strains: biological candidates to modulate the growth of Triticum aestivum beneficially. Am. J. Plant Sci. 4, 1693–1700. doi: 10.4236/ajps.2013.49206
Islam, F., Yasmeen, T., Ali, Q., Mubin, M., Ali, S., Arif, M. S., et al. (2016). Copper-resistant bacteria reduces oxidative stress and uptake of copper in lentil plants: potential for bacterial bioremediation. Environ. Sci. Pollut. Res. 23, 220–233. doi: 10.1007/s11356-015-5354-1
Jayaprakashvel, M., and Mathivanan, N. (2011). “Management of plant diseases by microbial metabolites,” in Bacteria in Agrobiology: Plant Nutrient Management, ed. D. K. Maheshwari (Berlin: Springer), 237–265. doi: 10.1007/978-3-642-21061-7_10
Jha, C. K., and Saraf, M. (2015). Plant growth promoting rhizobacteria (PGPR): a review. J. Agric. Res. Dev. 2015, 108–119. doi: 10.13140/RG.2.1.5171.2164
Joo, G. J., Kim, Y. M., Lee, I. J., Song, K. S., and Rhee, I. K. (2004). Growth promotion of red pepper plug seedlings and the production of gibberellins by Bacillus cereus, Bacillus macroides and Bacillus pumilus. Biotechnol. Lett. 26, 487–491. doi: 10.1023/B:BILE.0000019555.87121.34
Kamran, M. A., Eqani, S. A. M. A. S., Bibi, S., Xu, R. K., Monis, M. F. H., Katsoyiannis, A., et al. (2016). Bioaccumulation of nickel by E. sativa and role of plant growth promoting rhizobacteria (PGPRs) under nickel stress. Ecotoxicol. Environ. Saf. 126, 256–263. doi: 10.1016/j.ecoenv.2016.01.002
Kaur, H., Kaur, J., and Gera, R. (2016). Plant growth promoting rhizobacteria: a boon to agriculture. Int. J. Cell Sci. Biotechnol. 5, 17–22. doi: 10.1007/978-981-13-6040-4_6
Kesaulya, H., Hasinu, J. V., and Tuhumury, G. N. (2018). “Potential of Bacillus spp produces siderophores insuppressing thewilt disease of banana plants,” in IOP Conference Series: Earth and Environmental Science (Semarang: IOP Publishing), 1–6. doi: 10.1088/1755-1315/102/1/012016
Khalid, A., Arshad, M., and Zahir, Z. A. (2004a). Screening plant growth-promoting rhizobacteria for improving growth and yield of wheat. J. Appl. Microbiol. 96, 473–480. doi: 10.1046/j.1365-2672.2003.02161.x
Khalid, A., Tahir, S., Arshad, M., and Zahir, Z. A. (2004b). Relative efficiency of rhizobacteria for auxin biosynthesis in rhizosphere and non-rhizosphere soils. Aust. J. Soil Res. 42, 921–926. doi: 10.1071/SR04019
Khan, A. L., Waqas, M., Kang, S. M., Al-Harrasi, A., Hussain, J., Al-Rawahi, A., et al. (2014). Bacterial endophyte Sphingomonas sp LK11 produces gibberellins and IAA and promotes tomato plant growth. J. Microbiol. 52, 689–695. doi: 10.1007/s12275-014-4002-7
Khan, M. S., Zaidi, A., and Wani, P. A. (eds.). (2007). “Role of phosphate-solubilizing microorganisms in sustainable agriculture—a review,” in Agronomy for sustainable development (Springer), 29–43. doi: 10.1051/agro:2006011
Kingston, G. (2013). Mineral nutrition of sugarcane. Sugarcane 85–120. doi: 10.1002/9781118771280.ch5
Kloepper, J. W., and Schroth, M. N. (1978). “Plant growth-promoting rhizobacteria on radishes,” in Proceedings of the 4th International Conference on Plant Pathogenic Bacteria, Vol. 2. (Angers: Station de Pathologie Végétale et de Phytobactériologie; INRA).
Krey, T., Vassilev, N., Baum, C., and Eichler-Löbermann, B. (2013). Effects of long-term phosphorus application and plant-growth promoting rhizobacteria on maize phosphorus nutrition under field conditions. Eur. J. Soil Biol. 55, 124–130. doi: 10.1016/j.ejsobi.2012.12.007
Kuan, K. B., Othman, R., Rahim, K. A., and Shamsuddin, Z. H. (2016). Plant growth-promoting rhizobacteria inoculation to enhance vegetative growth, nitrogen fixation and nitrogen remobilisation of maize under greenhouse conditions. PLoS ONE 11:19. doi: 10.1371/journal.pone.0152478
Kumar, A., Maurya, B. R., Raghuwanshi, R., Meena, V. S., and Tofazzal Islam, M. (2017). Co-inoculation with enterobacter and rhizobacteria on yield and nutrient uptake by wheat (Triticum aestivum L.) in the alluvial soil under Indo-Gangetic Plain of India. J. Plant Growth Regul. 36, 608–617. doi: 10.1007/s00344-016-9663-5
Leclere, V., Bechet, M., Adam, A., Guez, J. S., Wathelet, B., Ongena, M., et al. (2005). Mycosubtilin overproduction by Bacillus subtilis BBG100 enhances the organism's antagonistic and biocontrol activities. Appl. Environ. Microbiol. 71, 4577–4584. doi: 10.1128/AEM.71.8.4577-4584.2005
Lee, C. M., Monson, R. E., Adams, R. M., and Salmond, G. P. (2017). The LacI-family transcription factor, RbsR, is a pleiotropic regulator of motility, virulence, siderophore and antibiotic production, gas vesicle morphogenesis and flotation in Serratia. Front. Microbiol. 8:1678. doi: 10.3389/fmicb.2017.01678
Li, H. B., Singh, R. K., Singh, P., Song, Q. Q., Xing, Y. X., Yang, L. T., et al. (2017). Genetic diversity of nitrogen-fixing and plant growth promoting pseudomonas species isolated from sugarcane rhizosphere. Front. Microbiol. 8:20. doi: 10.3389/fmicb.2017.01268
Li, Q., Saleh-Lakha, S., and Glick, B. R. (2005). The effect of native and ACC deaminase-containing Azospirillum brasilense Cd1843 on the rooting of carnation cuttings. Can. J. Microbiol. 51, 511–514. doi: 10.1139/w05-027
Liu, D., Yang, Q. Q., Ge, K., Hu, X. N., Qi, G. Z., Du, B. H., et al. (2017). Promotion of iron nutrition and growth on peanut by Paenibacillus illinoisensis and Bacillus sp strains in calcareous soil. Braz. J. Microbiol. 48, 656–670. doi: 10.1016/j.bjm.2017.02.006
Liu, D. F., Lian, B., and Dong, H. L. (2012). Isolation of Paenibacillus sp and assessment of its potential for enhancing mineral weathering. Geomicrobiol. J. 29, 413–421. doi: 10.1080/01490451.2011.576602
Liu, F. C., Xing, S. J., Ma, H. L., Du, Z. Y., and Ma, B. Y. (2013). Cytokinin-producing, plant growth-promoting rhizobacteria that confer resistance to drought stress in Platycladus orientalis container seedlings. Appl. Microbiol. Biotechnol. 97, 9155–9164. doi: 10.1007/s00253-013-5193-2
Liu, K., McInroy, J. A., Hu, C. H., and Kloepper, J. W. (2018). Mixtures of plant-growth-promoting rhizobacteria enhance biological control of multiple plant diseases and plant-growth promotion in the presence of pathogens. Plant Dis. 102, 67–72. doi: 10.1094/PDIS-04-17-0478-RE
Lobo, L. L. B., dos Santos, R. M., and Rigobelo, E. C. (2019). Promotion of maize growth using endophytic bacteria under greenhouse and field condition. Aust. J. Crop Sci. 13, 2067–2074. doi: 10.21475/ajcs.19.13.12.p2077
Lopes, V. R., Bespalhok-Filho, J. C., Araujo, L. M., Rodrigues, F. V., Daros, E., and Oliveira, R. A. (2012). The selection of sugarcane families that display better associations with plant growth promoting rhizobacteria. J. Agron. 11, 43–52. doi: 10.3923/ja.2012.43.52
Lugtenberg, B., and Kamilova, F. (2009). “Plant-growth-promoting rhizobacteria,” in Annual Review of Microbiology (Palo Alto: Annual Reviews), 541–556. doi: 10.1146/annurev.micro.62.081307.162918
Ma, Y., Prasad, M. N. V., Rajkumar, M., and Freitas, H. (2011). Plant growth promoting rhizobacteria and endophytes accelerate phytoremediation of metalliferous soils. Biotechnol. Adv. 29, 248–258. doi: 10.1016/j.biotechadv.2010.12.001
Majed, R., Faille, C., Kallassy, M., and Gohar, M. (2016). Bacillus cereus biofilms-same, only different. Front. Microbiol. 7:16. doi: 10.3389/fmicb.2016.01054
Maksimov, I. V., Abizgil'dina, R. R., and Pusenkova, L. I. (2011). Plant growth promoting rhizobacteria as alternative to chemical crop protectors from pathogens (review). Appl. Biochem. Microbiol. 47, 333–345. doi: 10.1134/S0003683811040090
Mansfield, T. A., Hetherington, A. M., and Atkinson, C. J. (1990). Some current aspects of stomatal physiology. Annu. Rev. Plant Physiol. Plant Mol. Biol. 41, 55–75. doi: 10.1146/annurev.pp.41.060190.000415
Martínez, C., Espinosa-Ruiz, A., and Prat, S. (2018). Gibberellins and plant vegetative growth. Annu Plant Rev Online. 49, 285–322. doi: 10.1002/9781119312994.apr0539
Maurya, B. R., Meena, V. S., and Meena, O. P. (2014). Influence of Inceptisol and Alfisol's potassium solubilizing bacteria (KSB) isolates on release of K from waste mica. Vegetos 27, 181–187. doi: 10.5958/j.2229-4473.27.1.028
Mayak, S., Tirosh, T., and Glick, B. R. (2004). Plant growth-promoting bacteria confer resistance in tomato plants to salt stress. Plant Physiol. Biochem. 42, 565–572. doi: 10.1016/j.plaphy.2004.05.009
McSteen, P. (2010). Auxin and monocot development. Cold Spring Harb. Perspect. Biol. 2:a001479. doi: 10.1101/cshperspect.a001479
Meena, K. K., Sorty, A. M., Bitla, U. M., Choudhary, K., Gupta, P., Pareek, A., et al. (2017). Abiotic stress responses and microbe-mediated mitigation in plants: the omics strategies. Front. Plant Sci. 8:172. doi: 10.3389/fpls.2017.00172
Meena, V. S., Maurya, B. R., and Verma, J. P. (2014). Does a rhizospheric microorganism enhance K+ availability in agricultural soils? Microbiol. Res. 169, 337–347. doi: 10.1016/j.micres.2013.09.003
Meena, V. S., Maurya, B. R., Verma, J. P., Aeron, A., Kumar, A., Kim, K., et al. (2015). Potassium solubilizing rhizobacteria (KSR): Isolation, identification, and K-release dynamics from waste mica. Ecol. Eng. 81, 340–347. doi: 10.1016/j.ecoleng.2015.04.065
Merino, E., Jensen, R. A., and Yanofsky, C. (2008). Evolution of bacterial trp operons and their regulation. Curr. Opin. Microbiol. 11, 78–86. doi: 10.1016/j.mib.2008.02.005
Mhlongo, M. I., Piater, L. A., Madala, N. E., Labuschagne, N., and Dubery, I. A. (2018). The chemistry of plant-microbe interactions in the rhizosphere and the potential for metabolomics to reveal signaling related to defense priming and induced systemic resistance. Front. Plant Sci. 9:17. doi: 10.3389/fpls.2018.00112
Mo, B., and Lian, B. (2011). Interactions between Bacillus mucilaginosus and silicate minerals (weathered adamellite and feldspar): Weathering rate, products, and reaction mechanisms. Chin. J. Geochem. 30:187. doi: 10.1007/s11631-011-0500-z
Moreira, H., Pereira, S. I. A., Marques, A., Rangel, A., and Castro, P. M. L. (2019). Effects of soil sterilization and metal spiking in plant growth promoting rhizobacteria selection for phytotechnology purposes. Geoderma 334, 72–81. doi: 10.1016/j.geoderma.2018.07.025
Moura, R. T. D. A., Garrido, M. D. S., Sousa, C. D. S., Menezes, R. S. C., and Sampaio, E. V. D. S. B. (2018). Comparison of methods to quantify soil microbial biomass carbon. Acta Sci. Agron. 40:39451. doi: 10.4025/actasciagron.v40i1.39451
Moutia, J. F. Y., Saumtally, S., Spaepen, S., and Vanderleyden, J. (2010). Plant growth promotion by Azospirillum sp. in sugarcane is influenced by genotype and drought stress. Plant Soil 337, 233–242. doi: 10.1007/s11104-010-0519-7
Munemasa, S., Hauser, F., Park, J., Waadt, R., Brandt, B., and Schroeder, J. I. (2015). Mechanisms of abscisic acid-mediated control of stomata! aperture. Curr. Opin. Plant Biol. 28, 154–162. doi: 10.1016/j.pbi.2015.10.010
Murray, J. D. (2011). Invasion by invitation: rhizobial infection in legumes. Mol. Plant Microbe Interact. 24, 631–639. doi: 10.1094/MPMI-08-10-0181
Muthukumarasamy, R., Revathi, G., Vadivelu, M., and Aruri, K. (2017). Isolation of bacterial strains possessing nitrogen-fixation, phosphate and potassium-solubilization and their inoculation effects on sugarcane. Indian J. Exp. Biol. 55, 161–170.
Naik, K., Mishra, S., Srichandan, H., Singh, P. K., and Sarangi, P. K. (2019). Plant growth promoting microbes: potential link to sustainable agriculture and environment. Biocatal. Agric. Biotechnol. 21:101326. doi: 10.1016/j.bcab.2019.101326
Nakkeeran, S., Marimuthu, T., and Raguchander, T. E. (2013). Exploring, D. A. P. G., and phenazine producing PGPR strains and fungal antagonists for the management of diseases of Noni., Morinda citrifolia, L. (2013).
O'Brien, J. A., and Benkova, E. (2013). Cytokinin cross-talking during biotic and abiotic stress responses. Front. Plant Sci. 4:451. doi: 10.3389/fpls.2013.00451
Olanrewaju, O. S., Glick, B. R., and Babalola, O. O. (2017). Mechanisms of action of plant growth promoting bacteria. World J. Microbiol. Biotechnol. 33:16. doi: 10.1007/s11274-017-2364-9
Oliveira, T. B. A., Selig, P. M., Barbosa, V. M., de Souza Campos, L. M., Bornia, A. C., and de Oliveira, M. W. (2012). Tecnologia e custos de produção de cana-de-açúcar: um estudo de caso em uma propriedade agrícola. Lat. Am. J. Bus. Manag. 3, 151–172.
Ortiz-Castro, R., and Lopez-Bucio, J. (2019). Review: Phytostimulation and root architectural responses to quorum-sensing signals and related molecules from rhizobacteria. Plant Sci. 284, 135–142. doi: 10.1016/j.plantsci.2019.04.010
Oteino, N., Lally, R. D., Kiwanuka, S., Lloyd, A., Ryan, D., Germaine, K. J., et al. (2015). Plant growth promotion induced by phosphate solubilizing endophytic Pseudomonas isolates. Front. Microbiol. 6:745. doi: 10.3389/fmicb.2015.00745
Pandya, U., and Saraf, M. (2014). “In vitro evaluation of PGPR strains for their biocontrol potential against fungal pathogens,” in Microbial Diversity and Biotechnology in Food Security, eds. R. N. Kharwar et al. (New Delhi: Springer), 293–305. doi: 10.1007/978-81-322-1801-2_26
Parmar, P., and Sindhu, S. S. (2013). Potassium solubilization by rhizosphere bacteria: influence of nutritional and environmental conditions. J. Microbiol. Res. 3, 25–31. doi: 10.5923/j.microbiology.20130301.04
Patel, K., Goswami, D., Dhandhukia, P., and Thakker, J. (2015). “Techniques to study microbial phytohormones,” in Bacterial Metabolites in Sustainable Agroecosystem, ed. D. K. Maheshwari (Dordrecht: Springer), 1–27. doi: 10.1007/978-3-319-24654-3_1
Patel, P., Shah, R., Joshi, B., Ramar, K., and Natarajan, A. (2019). Molecular identification and biocontrol activity of sugarcane rhizosphere bacteria against red rot pathogen Colletotrichum falcatum. Biotechnol. Rep. 21:e00317. doi: 10.1016/j.btre.2019.e00317
Patten, C. L., and Glick, B. R. (1996). Bacterial biosynthesis on indole-3-acetic acid. Can. J. Microbiol. 42, 207–220. doi: 10.1139/m96-032
Pereira, N. C. M., Galindo, F. S., Gazola, R. P. D., Dupas, E., Rosa, P. A. L., Mortinho, E. S., et al. (2020). Corn yield and phosphorus use efficiency response to phosphorus rates associated with plant growth promoting bacteria. Front. Environ. Sci. 8:40. doi: 10.3389/fenvs.2020.00040
Pereira, P., Ibàñez, F., Rosenblueth, M., Etcheverry, M., and Martínez-Romero, E. (2011). Analysis of the bacterial diversity associated with the roots of maize (Zea mays L.) through culture-dependent and culture-independent methods. ISRN Ecol. 10. doi: 10.5402/2011/938546
Pérez-Montaño, F., Jiménez-Guerrero, I., Contreras Sánchez-Matamoros, R., López-Baena, F. J., Ollero, F. J., Rodríguez-Carvajal, M. A., et al. (2013). Rice and bean AHL-mimic quorum-sensing signals specifically interfere with the capacity to form biofilms by plant-associated bacteria. Res. Microbiol. 164, 749–760. doi: 10.1016/j.resmic.2013.04.001
Perez-Montano, F., Alias-Villegas, C., Bellogin, R. A., del Cerro, P., Espuny, M. R., Jimenez-Guerrero, I., et al. (2014). Plant growth promotion in cereal and leguminous agricultural important plants: from microorganism capacities to crop production. Microbiol. Res. 169, 325–336. doi: 10.1016/j.micres.2013.09.011
Phillips, K. A., Skirpan, A. L., Liu, X., Christensen, A., Slewinski, T. L., Hudson, C., et al. (2011). vanishing tassel2 encodes a grass-specific tryptophan aminotransferase required for vegetative and reproductive development in maize. Plant Cell 23, 550–566. doi: 10.1105/tpc.110.075267
Pieterse, C. M. J., Van der Does, D., Zamioudis, C., Leon-Reyes, A., and Van Wees, S. C. M. (2012). “Hormonal modulation of plant immunity,” in Annual Review of Cell and Developmental Biology, Vol. 28, ed. R. Schekman (Palo Alto: Annual Reviews), 489–521. doi: 10.1146/annurev-cellbio-092910-154055
Pieterse, C. M. J., vanWees, S. C. M., Hoffland, E., vanPelt, J. A., and vanLoon, L. C. (1996). Systemic resistance in Arabidopsis induced by biocontrol bacteria is independent of salicylic acid accumulation and pathogenesis-related gene expression. Plant Cell 8, 1225–1237. doi: 10.1105/tpc.8.8.1225
Pieterse, C. M. J., Zamioudis, C., Berendsen, R. L., Weller, D. M., Van Wees, S. C. M., and Bakker, P. (2014). “Induced systemic resistance by beneficial microbes,” in Annual Review of Phytopathology, Vol. 52, ed. N. K. VanAlfen (Palo Alto: Annual Reviews), 347–375. doi: 10.1146/annurev-phyto-082712-102340
Porcel, R., Zamarreno, A. M., Garcia-Mina, J. M., and Aroca, R. (2014). Involvement of plant endogenous ABA in Bacillus megaterium PGPR activity in tomato plants. BMC Plant Biol. 14:36. doi: 10.1186/1471-2229-14-36
Pospisilova, J. (2003). Participation of phytohormones in the stomatal regulation of gas exchange during water stress. Biol. Plant. 46, 491–506. doi: 10.1023/A:1024894923865
Poupin, M. J., Greve, M., Carmona, V., and Pinedo, I. (2016). A complex molecular interplay of auxin and ethylene signaling pathways is involved in arabidopsis growth promotion by Burkholderia phytofirmans PsJN. Front. Plant Sci. 7:16. doi: 10.3389/fpls.2016.00492
Poupin, M. J., Timmermann, T., Vega, A., Zuniga, A., and Gonzalez, B. (2013). Effects of the plant growth-promoting bacterium Burkholderia phytofirmans PsJN throughout the life cycle of Arabidopsis thaliana. PLoS ONE 8:e69435. doi: 10.1371/journal.pone.0069435
Pourbabaee, A. A., Shoaibi, F., Emami, S., and Alikhani, H. A. (2018). The potential contribution of siderophore producing bacteria on growth and Fe ion concentration of sunflower (Helianthus annuus L.) under water stress. J. Plant Nutr. 41, 619–626. doi: 10.1080/01904167.2017.1406112
Quiñones, B., Dulla, G., and Lindow, S. E. (2005). Quorum sensing regulates exopolysaccharide production, motility, and virulence in Pseudomonas syringae. Mol. Plant-Microbe Interact. 18, 682–693. doi: 10.1094/MPMI-18-0682
Raj, D., and Antil, R. S. (2011). Evaluation of maturity and stability parameters of composts prepared from agro-industrial wastes. Bioresour. Technol. 102, 2868–2873. doi: 10.1016/j.biortech.2010.10.077
Rajkumar, M., Ae, N., Prasad, M. N. V., and Freitas, H. (2010). Potential of siderophore-producing bacteria for improving heavy metal phytoextraction. Trends Biotechnol. 28, 142–149. doi: 10.1016/j.tibtech.2009.12.002
Reed, S. C., Cleveland, C. C., and Townsend, A. R. (2011). “Functional ecology of free-living nitrogen fixation: a contemporary perspective,” in Annual Review of Ecology, Evolution, and Systematics, Vol. 42, eds. D. J. Futuyma, H. B. Shaffer, and D. Simberloff (Palo Alto: Annual Reviews), 489–512. doi: 10.1146/annurev-ecolsys-102710-145034
Reid, M. S. (1988). “The role of ethylene in flower senescence,” in: IV International Symposium on Postharvest Physiology of Ornamental Plants, 261.
Reimer, D., and Bode, H. B. (2014). A natural prodrug activation mechanism in the biosynthesis of nonribosomal peptides. Nat. Prod. Rep. 31, 154–159. doi: 10.1039/C3NP70081J
Riggs, P. J., Chelius, M. K., Iniguez, A. L., Kaeppler, S. M., and Triplett, E. W. (2001). Enhanced maize productivity by inoculation with diazotrophic bacteria. Funct. Plant Biol. 28, 829–836. doi: 10.1071/PP01045
Rocha, I., Ma, Y., Carvalho, M. F., Magalhaes, C., Janouskova, M., Vosatka, M., et al. (2019). Seed coating with inocula of arbuscular mycorrhizal fungi and plant growth promoting rhizobacteria for nutritional enhancement of maize under different fertilisation regimes. Arch. Agron. Soil Sci. 65, 31–43. doi: 10.1080/03650340.2018.1479061
Rodriguez, H., and Fraga, R. (1999). Phosphate solubilizing bacteria and their role in plant growth promotion. Biotechnol. Adv. 17, 319–339. doi: 10.1016/S0734-9750(99)00014-2
Romero-Perdomo, F., Abril, J., Camelo, M., Moreno-Galvan, A., Pastrana, I., Rojas-Tapias, D., et al. (2017). Azotobacter chroococcum as a potentially useful bacterial biofertilizer for cotton (Gossypium hirsutum): effect in reducing N fertilization. Rev. Argentina Microbiol. 49, 377–383. doi: 10.1016/j.ram.2017.04.006
Rosa, P. A. L., Mortinho, E. S., Jalal, A., Galindo, F. S., Buzetti, S., Fernandes, G. C., et al. (2020). Inoculation with growth-promoting bacteria associated with the reduction of phosphate fertilization in sugarcane. Front. Environ. Sci. 8:32. doi: 10.3389/fenvs.2020.00032
Rosas, S. B., Avanzini, G., Carlier, E., Pasluosta, C., Pastor, N., and Rovera, M. (2009). Root colonization and growth promotion of wheat and maize by Pseudomonas aurantiaca SR1. Soil Biol. Biochem. 41, 1802–1806. doi: 10.1016/j.soilbio.2008.10.009
Rouf Shah, T., Prasad, K., and Kumar, P. (2016). Maize-A potential source of human nutrition and health: a review. Cogent Food Agric. 54:1166995. doi: 10.1080/23311932.2016.1166995
Sah, S. K., Reddy, K. R., and Li, J. X. (2016). Abscisic acid and abiotic stress tolerance in crop plants. Front. Plant Sci. 7:571. doi: 10.3389/fpls.2016.00571
Saleem, M., Zamir, M. S. I., Haq, I., Irshad, M. Z., Khan, M. K., Asim, M., et al. (2015). Yield and quality of forage oat (Avena sativa L.) cultivars as affected by seed inoculation with nitrogenous strains. Am. J. Plant Sci. 6:3251. doi: 10.4236/ajps.2015.619316
Sandhya, V., Ali, S. Z., Grover, M., Reddy, G., and Venkateswarlu, B. (2010). Effect of plant growth promoting Pseudomonas spp. on compatible solutes, antioxidant status and plant growth of maize under drought stress. Plant Growth Regul. 62, 21–30. doi: 10.1007/s10725-010-9479-4
Sandy, M., and Butler, A. (2011). Chrysobactin siderophores produced by Dickeya chrysanthemi EC16. J. Nat. Prod. 74, 1207–1212. doi: 10.1021/np200126z
Santos, R. M., Kandasamy, S., and Rigobelo, E. C. (2018). Sugarcane growth and nutrition levels are differentially affected by the application of PGPR and cane waste. Microbiologyopen 7:e00617. doi: 10.1002/mbo3.617
Sattari, S. Z., Bouwman, A. F., Giller, K. E., and van Ittersum, M. K. (2012). Residual soil phosphorus as the missing piece in the global phosphorus crisis puzzle. Proc. Natl Acad. Sci. U.S.A. 109, 6348–6353. doi: 10.1073/pnas.1113675109
Schaller, G. E., Bishopp, A., and Kieber, J. J. (2015). The Yin-Yang of hormones: cytokinin and auxin interactions in plant development. Plant Cell 27, 44–63. doi: 10.1105/tpc.114.133595
Schutze, E., Ahmed, E., Voit, A., Klose, M., Greyer, M., Svatos, A., et al. (2015). Siderophore production by streptomycetes-stability and alteration of ferrihydroxamates in heavy metal-contaminated soil. Environ. Sci. Pollut. Res. 22, 19376–19383. doi: 10.1007/s11356-014-3842-3
Sgroy, V., Cassán, F., Masciarelli, O., Del Papa, M. F., Lagares, A., and Luna, V. (2009). Isolation and characterization of endophytic plant growth-promoting (PGPB) or stress homeostasis-regulating (PSHB) bacteria associated to the halophyte Prosopis strombulifera. Appl. Microbiol. Biotechnol. 85, 371–381. doi: 10.1007/s00253-009-2116-3
Shahzad, R., Waqas, M., Khan, A. L., Asaf, S., Khan, M. A., Kang, S. M., et al. (2016). Seed-borne endophytic Bacillus amyloliquefaciens RWL-1 produces gibberellins and regulates endogenous phytohormones of Oryza sativa. Plant Physiol. Biochem. 106, 236–243. doi: 10.1016/j.plaphy.2016.05.006
Shaikh, S. S., and Sayyed, R. Z. (2015). “Role of plant growth-promoting rhizobacteria and their formulation in biocontrol of plant diseases,” in Plant Microbes Symbiosis: Applied Facets, ed. D. K. Maheshwari (New Delhi: Springer), 337–351. doi: 10.1007/978-81-322-2068-8_18
Sharma, S. B., Sayyed, R. Z., Trivedi, M. H., and Gobi, T. A. (2013). Phosphate solubilizing microbes: sustainable approach for managing phosphorus deficiency in agricultural soils. Springerplus 2:587. doi: 10.1186/2193-1801-2-587
Shaterian, J., Waterer, D., De Jong, H., and Tanino, K. K. (2005). Differential stress responses to NaCl salt application in early- and late-maturing diploid potato (Solanum sp.) clones. Environ. Exp. Bot. 54, 202–212. doi: 10.1016/j.envexpbot.2004.07.005
Sheng, X. F., Zhao, F., He, L. Y., Qiu, G., and Chen, L. (2008). Isolation and characterization of silicate mineral-solubilizing Bacillus globisporus Q12 from the surfaces of weathered feldspar. Can. J. Microbiol. 54, 1064–1068. doi: 10.1139/W08-089
Sherathia, D., Dey, R., Thomas, M., Dalsania, T., Savsani, K., and Pal, K. K. (2016). Biochemical and molecular characterization of DAPG-producing plant growth promoting rhizobacteria (PGPR) of groundnut (Arachis hypogaea L.). Legume Res. 39, 614–622. doi: 10.18805/lr.v0iOF.9389
Shiferaw, B., Prasanna, B. M., Hellin, J., and Banziger, M. (2011). Crops that feed the world 6. Past successes and future challenges to the role played by maize in global food security. Food Secur. 3, 307–327. doi: 10.1007/s12571-011-0140-5
Sitbon, F., Hennion, S., Sundberg, B., Little, C. H. A., Olsson, O., and Sandberg, G. (1992). Transgenic tobacco plants coexpressing the Agrobacterium-tumefaciens-iaam and iaah genes display altered growth and indoleacetic-acid metabolism. Plant Physiol. 99, 1062–1069. doi: 10.1104/pp.99.3.1062
Solano, B. R., Maicas, J. B., and Mañero, F. G. (2008). “Physiological and molecular mechanisms of plant growth promoting rhizobacteria (PGPR),” in Plant-Bacteria Interactions: Strategies and Techniques to Promote Plant Growth, eds. I. Ahmad, J. Pichtel, and S. Hayat (Weinheim: Wiley), 41–52.
Soutar, C. D., and Stavrinides, J. (2018). The evolution of three siderophore biosynthetic clusters in environmental and host-associating strains of Pantoea. Mol. Genet. Genomics 293, 1453–1467. doi: 10.1007/s00438-018-1477-7
Spaepen, S., and Vanderleyden, J. (2011). Auxin and plant-microbe interactions. Cold Spring Harb. Perspect. Biol. 3, 1–13. doi: 10.1101/cshperspect.a001438
Spaepen, S., Vanderleyden, J., and Remans, R. (2007). Indole-3-acetic acid in microbial and microorganism-plant signaling. FEMS Microbiol. Rev. 31, 425–448. doi: 10.1111/j.1574-6976.2007.00072.x
Sparks, D. L., and Huang, P. M. (1985). “Physical chemistry of soil potassium,” in Potassium in Agriculture, ed. R. D. Munson, 201–276. doi: 10.2134/1985.potassium.c9
Spolaor, L. T., Gonçalves, L. S. A., Santos, O. J. A. P. D., Oliveira, A. L. M. D., Scapim, C. A., Bertagna, F. A. B., et al. (2016). Plant growth-promoting bacteria associated with nitrogen fertilization at topdressing in popcorn agronomic performance. Bragantia 75, 33–40. doi: 10.1590/1678-4499.330
Syed, S., and Chinthala, P. (2015). Heavy metal detoxification by different Bacillus species isolated from solar salterns. Scientifica 2015:319760. doi: 10.1155/2015/319760
Syers, J., Johnston, A., and Curtin, D. (2008). Efficiency of Soil and Fertilizer Phosphorus Use. Roma: FAO Fertilizer and plant nutrition bulletin, 18.
Tabassum, B., Khan, A., Tariq, M., Ramzan, M., Khan, M. S. I., Shahid, N., et al. (2017). Bottlenecks in commercialisation and future prospects of PGPR. Appl. Soil Ecol. 121, 102–117. doi: 10.1016/j.apsoil.2017.09.030
Tahir, H. A. S., Gu, Q., Wu, H. J., Raza, W., Hanif, A., Wu, L. M., et al. (2017). Plant growth promotion by volatile organic compounds produced by Bacillus subtilis SYST2. Front. Microbiol. 8:11. doi: 10.3389/fmicb.2017.00171
Vacheron, J., Desbrosses, G., Bouffaud, M. L., Touraine, B., Moenne-Loccoz, Y., Muller, D., et al. (2013). Plant growth-promoting rhizobacteria and root system functioning. Front. Plant Sci. 4:19. doi: 10.3389/fpls.2013.00356
Vacheron, J., Renoud, S., Muller, D., Babalola, O. O., and Prigent-Combaret, C. (2015). “Alleviation of abiotic and biotic stresses in plants by Azospirillum,” in Handbook for Azospirillum, ed. B. Rewald (Villeurbanne: Springer), 333–365. doi: 10.1007/978-3-319-06542-7_19
Van Loon, L. C. (1997). Induced resistance in plants and the role of pathogenesis-related proteins. Eur. J. Plant Pathol. 103, 753–765. doi: 10.1023/A:1008638109140
van Loon, L. C. (2007). Plant responses to plant growth-promoting rhizobacteria. Eur. J. Plant Pathol. 119, 243–254. doi: 10.1007/s10658-007-9165-1
Verma, P., Agrawal, N., and Shahi, S. K. (2018). Enterobacter cloacae strain PGLO9: Potential source of maize growth promoting rhizobacteria. Int. J. Bot. Stud. 3, 172–175.
Vessey, J. K. (2003). Plant growth promoting rhizobacteria as biofertilizers. Plant Soil 255, 571–586. doi: 10.1023/A:1026037216893
Viruel, E., Erazzu, L. E., Calsina, L. M., Ferrero, M. A., Lucca, M. E., and Sineriz, F. (2014). Inoculation of maize with phosphate solubilizing bacteria: effect on plant growth and yield. J. Soil Sci. Plant Nutr. 14, 819–831. doi: 10.4067/S0718-95162014005000065
Viswanathan, R., and Samiyappan, R. (2002). Induced systemic resistance by fluorescent pseudomonads against red rot disease of sugarcane caused by Colletotrichum falcatum. Crop Protect. 21, 1–10. doi: 10.1016/S0261-2194(01)00050-3
Wang, G. L., Que, F., Xu, Z. S., Wang, F., and Xiong, A. S. (2015). Exogenous gibberellin altered morphology, anatomic and transcriptional regulatory networks of hormones in carrot root and shoot. BMC Plant Biol. 15:290. doi: 10.1186/s12870-015-0679-y
Wang, N., Hua, H. B., Eneji, A. E., Li, Z. H., Duan, L. S., and Tian, X. L. (2012). Genotypic variations in photosynthetic and physiological adjustment to potassium deficiency in cotton (Gossypium hirsutum). J. Photochem. Photobiol B Biol. 110, 1–8. doi: 10.1016/j.jphotobiol.2012.02.002
Wani, P. A., and Khan, M.S. (2014). Screening of multiple metal and antibiotic resistant isolates and their plant growth promoting activity. Pak. J. Biol. Sci. 17, 206–212. doi: 10.3923/pjbs.2014.206.212
Whiteley, M., Diggle, S. P., and Greenberg, E. P. (2017). Progress in and promise of bacterial quorum sensing research. Nature 551, 313–320. doi: 10.1038/nature24624
Woodward, A. W., and Bartel, B. (2005). Auxin: Regulation, action, and interaction. Ann. Bot. 95, 707–735. doi: 10.1093/aob/mci083
Xia, Y., Farooq, M. A., Javed, M. T., Kamran, M. A., Mukhtar, T., Ali, J., et al. (2020). Multi-stress tolerant PGPR Bacillus xiamenensis PM14 activating sugarcane (Saccharum officinarum L.) red rot disease resistance. Plant Physiol. Biochem. 151, 640–649. doi: 10.1016/j.plaphy.2020.04.016
Youseif, S. H. (2018). Genetic diversity of plant growth promoting rhizobacteria and their effects on the growth of maize plants under greenhouse conditions. Ann. Agric. Sci. 63, 25–35. doi: 10.1016/j.aoas.2018.04.002
Yu, H., Ling, N., Wang, T., Zhu, C., Wang, Y., Wang, S., et al. (2019). Responses of soil biological traits and bacterial communities to nitrogen fertilization mediate maize yields across three soil types. Soil Till. Res. 185, 61–69. doi: 10.1016/j.still.2018.08.017
Zeevaart, J. (1999). “Abscisic acid metabolism and its regulation,” in Biochemistry and Molecular Biology of Plant Hormones, eds P. Hooykaas, M. Hall, and K. Libbenga (Amsterdam: Elsevier), 189–207.
Zhang, W. L., Zhang, Y., Wang, X. X., Ding, F. S., Fu, Y. M., Zhao, J. Z., et al. (2017). Siderophores in clinical isolates of Klebsiella pneumoniae promote ciprofloxacin resistance by inhibiting the oxidative stress. Biochem. Biophys. Res. Commun. 491, 855–861. doi: 10.1016/j.bbrc.2017.04.108
Zhao, D. L., and Li, Y. R. (2015). Climate change and sugarcane production: potential impact and mitigation strategies. Int. J. Agron. 2015, 1–10. doi: 10.1155/2015/547386
Zhao, D. L., Oosterhuis, D. M., and Bednarz, C. W. (2001). Influence of potassium deficiency on photosynthesis, chlorophyll content, and chloroplast ultrastructure of cotton plants. Photosynthetica 39, 103–109. doi: 10.1023/A:1012404204910
Keywords: plant-growth-promoting, Saccharum spp., Zea mays, rhizosphere, yield
Citation: dos Santos RM, Diaz PAE, Lobo LLB and Rigobelo EC (2020) Use of Plant Growth-Promoting Rhizobacteria in Maize and Sugarcane: Characteristics and Applications. Front. Sustain. Food Syst. 4:136. doi: 10.3389/fsufs.2020.00136
Received: 25 May 2020; Accepted: 29 July 2020;
Published: 29 September 2020.
Edited by:
Matteo Balderacchi, Independent Researcher, Piacenza, ItalyReviewed by:
John A. McInroy, Auburn University, United StatesCopyright © 2020 dos Santos, Diaz, Lobo and Rigobelo. This is an open-access article distributed under the terms of the Creative Commons Attribution License (CC BY). The use, distribution or reproduction in other forums is permitted, provided the original author(s) and the copyright owner(s) are credited and that the original publication in this journal is cited, in accordance with accepted academic practice. No use, distribution or reproduction is permitted which does not comply with these terms.
*Correspondence: Everlon Cid Rigobelo, ZXZlcmxvbi5jaWRAdW5lc3AuYnI=
†ORCID: Everlon Cid Rigobelo orcid.org/0000-0002-9734-3338
Disclaimer: All claims expressed in this article are solely those of the authors and do not necessarily represent those of their affiliated organizations, or those of the publisher, the editors and the reviewers. Any product that may be evaluated in this article or claim that may be made by its manufacturer is not guaranteed or endorsed by the publisher.
Research integrity at Frontiers
Learn more about the work of our research integrity team to safeguard the quality of each article we publish.