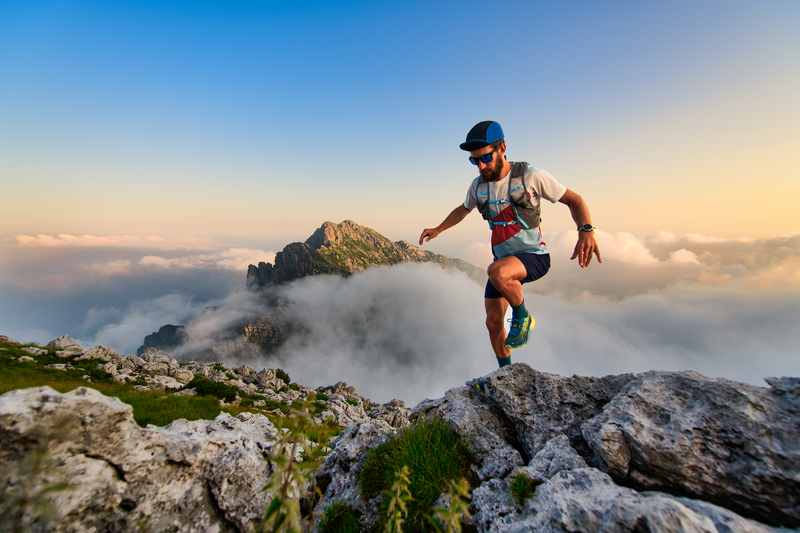
94% of researchers rate our articles as excellent or good
Learn more about the work of our research integrity team to safeguard the quality of each article we publish.
Find out more
ORIGINAL RESEARCH article
Front. Sustain. Food Syst. , 04 September 2020
Sec. Crop Biology and Sustainability
Volume 4 - 2020 | https://doi.org/10.3389/fsufs.2020.00131
This article is part of the Research Topic Plant Growth-Promoting Microorganisms for Sustainable Agricultural Production View all 50 articles
The date palm is a commercially important woody crop and is a good target plant for improving agricultural yields in extreme environments. However, salinity has been the primary abiotic stress complicating its cultivation and damaging its production worldwide. This study investigated the effect of alleviating salt stress on date palm growth and development by using arbuscular mycorrhizal fungi (AMF) and/or compost. The experiment was arranged in a completely randomized design with eight treatments. The treatments comprised control without inoculation or amendment and application of compost (made from green waste) and AMF (an autochthonous consortium) individually or in combination under non-saline (0 mM NaCl) or saline (240 mM NaCl) conditions. Growth, physiological characteristics, nutrient uptake, chlorophyll content, oxidative stress markers, and antioxidant enzyme activities were assessed. Salt stress increased sodium (Na+) and chlorine (Cl−) content, lipid peroxidation and proline, soluble sugar, and H2O2 content. However, it reduced growth parameters, AMF colonization, leaf water potential, nitrogen (N), phosphorus (P), potassium (K+), calcium (Ca2+), and chlorophyll content. The application of AMF and compost separately or in combination mitigated the deleterious effects induced by salinity. AMF inoculation contributed to plant salt tolerance through strategies such as increased nutrient uptake (particularly P and Ca2+), chlorophyll content, relative water content, stomatal conductance, antioxidant enzymatic activities (superoxide dismutase, ascorbate peroxidase, catalase) and by decreasing lipid peroxidation and H2O2 content. Plants grown in soil amended with compost under salt stress showed an improvement particularly in K+ and proline content and a decrease in H2O2 concentration compared to controls under the saline condition. In the presence of NaCl stress, the dual application of the compost and AMF consortium maximized plant growth, stomatal conductance, leaf water potential, all antioxidant enzyme activities and P, K+, N, and Ca2+ uptake as well as proline and soluble sugar content. However, it reduced Na+ and Cl− uptake and oxidative stress marker content. In conclusion, our study suggests that the application of AMF with compost has the potential to improve the tolerance of date palm seedlings to salt stress more than AMF or compost applied separately.
Climate change causes perturbations to the surrounding environment and significantly induces soil salinization. In arid and semi-arid regions, sporadic rainfall and high temperatures have resulted in increased evapotranspiration rates, which, when combined with the use of saline water for irrigation and excessive use of chemical products, has led to increasing soil salinization (Munns, 2005; Trenberth et al., 2014). It has been estimated that saline soils account for around 8% of the earth's surface land and are increasing worldwide (Hajiboland, 2013). Soil salinity is a global problem, damaging agricultural lands and causing a >20% reduction in agricultural yields (Porcel et al., 2012; Setia et al., 2013). The increase in soil salinity results in osmotic as well as specific ion effects, which induce secondary stress in plants known as oxidative stress (Evelin et al., 2019; Abdel Latef et al., 2020). Consequently, salt stress causes adverse effects on physiological and biochemical activities such as mineral homeostasis, seed germination, osmotic balance, photosynthesis, and respiration processes (Porcel et al., 2012; Ben Laouane et al., 2019). Oxidative salt-induced stress leads to the production of reactive oxygen species (ROS) that induce deleterious effects on normal metabolism and growth (Gill and Tuteja, 2010). However, plants can tolerate both osmotic and oxidative stress caused by soil salinity through several mechanisms (Evelin et al., 2019). Osmotic adjustment is one of the defense mechanisms that help plants to handle the osmotic and ion toxicity effects by the exclusion of the salts and compartmentation of Na+ ions in the vacuole or apoplast (Apse and Blumwald, 2007). Salinity tolerance can also occur via the production of osmolytes such as glycine-betaine, proline, and soluble sugars (Zhu, 2003). Furthermore, plants possess an efficient antioxidant defense system to scavenge ROS (Sharma et al., 2012). Enzymatic antioxidants, including superoxide dismutase (SOD), catalase (CAT), peroxidase (POD), ascorbate peroxidase (APX), and glutathione reductase (GR) have been previously reported as the main antioxidant enzymes under salinity stress (Abdel Latef and Chaoxing, 2014; Foyer, 2018; Evelin et al., 2019).
Arbuscular mycorrhizal fungi (AMF) have generated growing interest as an efficient alternative to promote plant growth and stress tolerance. They may also contribute to increased yield and nutrition of host plants for sustainable agriculture (Baslam et al., 2011a, 2012; Baslam and Goicoechea, 2012; Bouamri et al., 2014; Bowles et al., 2017; Rillig et al., 2019; Zhang et al., 2019). This symbiosis is known to mitigate the harmful effects of salt stress on plants although the fungi are adversely affected by salinity themselves (Abdel Latef and Chaoxing, 2011; Ait-El-Mokhtar et al., 2019). In the saline environment, AMF improve the rhizospheric condition of the soil and enhance the mineral nutrition and the water uptake of the host plant (Augé et al., 2014; Hodge and Storer, 2014; El Kinany et al., 2019). AMF colonization has been widely reported to reduce the influx of toxic ions (sodium and chlorine) into the root system (Daei et al., 2009), and stimulate the photosynthetic apparatus and enhance the effectiveness of antioxidant defense system (Hidri et al., 2016; Boutasknit et al., 2020). These features led to in recent years the commercial production of AMF as a “biofertilizer” for field use, to reduce the intensive use of agrochemicals with no loss in yield. However, the merits of such biofertilizers are currently under debate owing to the variable effects of AMF on plant biomass (Berruti et al., 2016; Ryan and Graham, 2018; Rillig et al., 2019) which are driven by intrinsic (host plant and AMF identities) and extrinsic/environmental (nutrient and water) factors.
Previous studies have reported the utilization of organic compost fertilizers for the restoration of salt-affected soils (Meena et al., 2016; Mbarki et al., 2018). When incorporated into the soil, compost is mineralized, thereby providing a sustained release of available nutrients to plants (D'Hose et al., 2014; Ngo and Cavagnaro, 2018; Ou Zin et al., 2020). Compost can alleviate salinity stress in plants by improving soil fertility (Meena et al., 2016; Raklami et al., 2019), promoting nutrient availability and plant growth (Duong et al., 2012; Trivedi et al., 2017) and stimulating respiration, photosynthesis, and chlorophyll content (Lakhdar et al., 2008).
The date palm (Phoenix dactylifera L.) is one of the most important fruit crops in the arid and semiarid areas of North Africa and the Middle East where it is cultivated for its nutritional, socio-economical, and environment value (Chao and Krueger, 2007). The fruits of the date palm are rich in essential nutrients such as sugars, proteins, fibers, minerals, antioxidants, and vitamins (Vayalil, 2012; Kamal-Eldin and Ghnimi, 2018). To date, several studies have analyzed date palm responses to salinity stress (Sperling et al., 2014; Yaish and Kumar, 2015; Meddich et al., 2018; Ait-El-Mokhtar et al., 2019; Al Kharusi et al., 2019) but few have addressed the effects of AMF inoculation in alleviating salt stress in date palms (Meddich et al., 2018; Ait-El-Mokhtar et al., 2019). Furthermore, no study has yet tested the role of the combined application of AMF and compost in the salt tolerance of date palms and the underlying mechanisms. Therefore, the present study was carried out to investigate the effects of both fertilizers alone and in combination on growth, physiological, and biochemical traits of date palm seedlings under saline conditions. This was accomplished by using native AMF and locally produced compost to improve plant tolerance, as well as a clear understanding of the mechanisms implicated in AM-compost-induced salt stress tolerance. The study paves the way for the use of biological fertilizers to strengthen plants' adaptability and to further studies on the defense mechanism and functioning of AMF and/or compost in the palm family of plants in response to changing environments.
Seeds of Phoenix dactylifera cv. Boufeggous obtained from Aoufous palm grove (Errachidia, Morocco) were surface-sterilized by immersion for 10 min in 10% (v/v) sodium hypochlorite solution followed by several washings with sterile distilled water. Seeds were germinated in plastic bowls containing a sterile sandy substrate collected from “Oued Lahjar” river limiting the Northeastern palm grove of Marrakesh, Morocco. The river sand has the following characteristics: pH: 9.31, EC: 0.29 dS cm−1, 0.001% P (Phosphorus), 0.001% K (Potassium), 0.006% Mg (Magnesium), 0.012% Fe (Iron), 0.01% Ca (Calcium), 0.002% Na (Sodium), and 0.01% Al (Aluminum) (Anli et al., 2020). The date palm seeds were incubated for 3 weeks at 38°C in the dark. Two months after germination, seedlings were transplanted into plastic pots containing 2.3 kg of river sand (same substrate as above) previously sterilized for 3 h at 180°C during three consecutive days.
The native mycorrhizal Aoufous consortium (MAC) had been isolated earlier from the rhizospheric soil of P. dactylifera from the Tafilalet palm grove (500 km southeast of Marrakesh) and it contained a mixture of native species: (i) Glomus sp. (15 spores/g soil), (ii) Sclerocystis sp. (9 spores/g soil), and (iii) Acaulospora sp. (one spore/g soil) (Meddich et al., 2015).
The trap culture protocol was used to propagate MAC under greenhouse-controlled conditions for 3 months using Zea mays L. as a host plant. The mycorrhizal fungi inoculum was a mixture of spores, soil, hyphae, and infected root fragments from the trap culture. The most probable number (MPN) test (Sieverding, 1991) was used to assess inoculum potential infectivity and to determine the doses to be applied. According to the MPN test, the trap culture inoculum contained 1056 infective propagules/100 g. The application of mycorrhizal inoculum was carried out when transplanting seedlings. The inoculated dosage, added to the corresponding pots, was 10 g of inoculum per pot containing approximately 110 spores with Ma = 78.8%. Non-inoculated pots received the same amount of autoclaved mycorrhizal fungi inoculum.
Compost was prepared from green waste as described previously by Meddich et al. (2016). The compost had the following characteristics based on dry matter: pH (6.87), organic matter (527.2 g kg−1), organic C (306.5 g kg−1), total N (21.9 g kg−1), C/N ratio (14.0), ashes (490 g kg−1), available P (2.5 mg g−1), (0.03 10−3 mg g−1), (0.07 10−3 mg g−1), / ratio (0.44), bacterial population (2.12 105 CFU g−1), and fungal population (9.75 104 CFU g−1). The compost was added to the corresponding pots, when transplanting seedlings, in a proportion of 5% (w/w).
After transplantation, date palm seedlings were irrigated regularly with distilled water for 5 months after germination, and then transplants were subjected to two salt concentrations (0 and 240 mM NaCl). The NaCl concentration was gradually added (60, 120, 180, and 240 mM NaCl) to the pots on alternative days for 2 weeks to reach 240 mM NaCl without causing osmotic shock (Estrada et al., 2013). Excess water due to drainage was collected and added to the soil of the corresponding pots. Plants were irrigated with either distilled water (control) or saline solution (NaCl), with EC fixed of 24 dS/m until harvest. EC of the saline solution was monitored regularly and adjusted (and pH level) when necessary using NaCl and distilled water. The pH and EC were measured using a pH meter HI 9025 and a conductivity meter HI-9033 (Hanna Instruments, Padova, Italy), respectively. All plants were grown for 14 months without adding any fertilizer.
The experimental design consisted of eight treatments (untreated plants under normal condition, Control 0 mM; seedlings treated with compost alone under 0 mM, +compost 0 mM, seedlings inoculated with AMF alone under 0 mM, +AMF 0 mM, seedlings treated with compost and inoculated with AMF under 0 mM, +compost+AMF 0 mM, untreated plants under 240 mM NaCl, Control 240 mM; seedlings treated with compost alone under 240 mM, +compost 240 mM, seedlings inoculated with AMF alone under 240 mM, +AMF 240 mM, seedlings treated with compost and inoculated with AMF under 240 mM, +compost+AMF 240 mM) with a factorial combination of 2 × 2 × 2 CxMxS (CompostxAMFxSalinity). Pots were arranged in a completely randomized block design. Ten replications (one plant per pot) were sampled in each treatment.
Plants were grown for 14 months under semi-controlled environmental conditions in a greenhouse at the Faculty of Science Semlalia (Cadi Ayyad University, Marrakesh, Morocco) under natural light (photon flux density ranged from 500 to 750 μmol m−2 s−1). The average temperature was 25.5°C and a relative humidity average of 68%.
At harvest, plants were first rinsed with tap water and then with distilled water. The shoot and root system were separated and the growth parameters (plant height, number of leaves, leaf area, and shoot and root dry weights) were measured. The leaf area was determined using an LC 4800 scanner and Winfolia v. 2004 software.
To assess AMF colonization, roots fragments, from the lateral root system, were carefully washed, cleared with 10% of KOH and stained with Trypan blue (Phillips and Hayman, 1970). Thirty 1 cm long fine root segments, for each replicate sample, were observed under a Zeiss Axioskop 40 microscope at 40–100× magnification. The number of root segments colonized by different AMF structures was used to calculate AMF colonization (McGonigle et al., 1990). AMF infection frequency and intensity were determined using the equations below:
Where “n5” corresponds to the number of roots with infection level of 5 (infection rate 90–100%). “n4” corresponds to the number of roots at level 4 (infection rate 50–90%). “n3” corresponds to the number of roots at level 3 (infection rate at 10–50%). “n2” corresponds to the number of roots at infection level 2 (infection rate 1–10%). “n1” corresponds to the number of roots at level 1 (infection rate 0–1%).
Oven-dried shoots and roots were powdered to determine the mineral concentration. P content was estimated using the Olsen method (Olsen and Dean, 1965). Na+, K+, and Ca2+ content in the plant was estimated by flame photometry (JENWAY, PFP7) as described by Wolf (1982). The Nitrogen (N) content was determined by colorimetry after the Kjeldahl digestion and chlorine (Cl−) content was measured using the silver nitrate (AgNO3) titration method.
The third youngest, fully expanded and attached leaves from five plants for each treatment were used for measurements. An average of four records from different parts of each leaf was considered for each replicate. The efficiency of photosystem II was evaluated by measuring chlorophyll fluorescence using a portable fluorometer (Opti-sciences OSI 30p) for dark-adapted leaves. Leaves were acclimated to dark for 30 min using leaf clips before measurements were taken. Chlorophyll fluorescence variables were recorded: Initial (F0), maximum (Fm), variable (Fv = Fm- F0) fluorescence as well as Fv/Fm ratio (Baker, 2008).
Stomatal conductance (gs) was measured on five replications per treatment on a sunny day before harvest and by using a porometer system (Leaf Porometer LP1989, Decagon Device, Inc., Washington, USA) following the user manual instructions. The second youngest leaf from five different plants from each treatment was used for measurements.
Leaf portions were taken from two plants per replicate (the third leaf from the top) to determine the fresh weight (FW), dry weight (DW), and turgid weight (TW). Values of FW, TW, and DW were used to calculate leaf relative water content (LRWC) using the following equation (Barrs and Weatherly, 1962):
The leaf water potential (LWP) was measured on newly mature leaves from each plant (five repetitions per treatment) using a pressure chamber according to Scholander et al. (1965).
Photosynthetic pigments were extracted from leaf samples in 80% acetone according to Arnon (1949). The extracted material was centrifuged at 10,000 × g for 10 min. The optical density of the supernatants was recorded at 480, 645, and 663 nm using a UV–vis spectrophotometer (UV-3100PC spectrophotometer). Values of absorbance were used to calculate the content of photosynthetic pigments. A blank with 80% acetone served as the control.
Lipid peroxidation was measured in terms of malondialdehyde (MDA) content according to the method of Dhindsa et al. (1981). Fresh leaf and root material (0.25 g) was homogenized in 10 mL of 0.1% trichloroacetic acid (TCA) and centrifuged at 18,000 × g for 10 min. Two-milliliter aliquots of the supernatant were mixed with 2 mL of 20% TCA containing 0.5% thiobarbituric acid (TBA). The mixture was heated at 100°C for 30 min, quickly cooled and then centrifuged at 10,000 × g for 10 min for clarification. The absorbance of the supernatant was measured at 532 nm (A532). The unspecific turbidity was corrected by subtracting A600 from A532.
Hydrogen peroxide content in leaves and roots was determined as described by Velikova et al. (2000). 0.25 g of fresh material was homogenized in a cold mortar with 5 mL 10% (w/v) TCA and then centrifuged at 15,000 × g for 15 min at 4°C. The supernatant was then recovered to determine the content of H2O2. 0.5 mL of potassium phosphate buffer (10 mM, pH 7) and 1 mL of iodic potassium (1 M) was added to 0.5 mL of the supernatant. The absorbance at 390 nm was recorded after 1 h of incubation in the dark. The blanks were made by replacing the sample extract with 10% TCA.
Proline from the date palm leaves and roots was extracted following the method of Bates et al. (1973). Fresh plant material (0.1 g) was ground in a mortar with 5 mL of 3% (w/v) aqueous sulphosalicylic acid and then centrifuged at 12,000 × g for 15 min. The supernatant (2 mL) was mixed with 2 mL of glacial acetic acid and 2 mL of acid ninhydrin reagent and incubated at 100°C water bath for 1 h. The reaction was terminated by placing the mixture in an ice bath followed by extraction with toluene. The absorbance was read at 520 nm and the proline concentration was calculated from a calibration curve using proline as standard.
A sample of 0.1 g of frozen materiel from leaves and roots was homogenized with 4 mL of ethanol (80%) then the homogenate was centrifuged at 5,000 rpm for 10 min. The resulting supernatant was collected, while the rest was resuspended again by adding 2 mL of ethanol and recentrifuged. Both supernatants were used to quantify total soluble sugar according to the method described by Dubois et al. (1956). The supernatant (1 mL) was mixed with 1 mL of phenol solution (5%) and 5 mL of concentrated sulfuric acid. After 5 min, the absorbance was measured at 485 nm in a UV-3100PC spectrophotometer. Soluble sugar content was determined using glucose as a standard.
Enzyme extraction was performed according to optimized protocols described by Benhiba et al. (2015). Fresh leaf and root material (0.5 g) were frozen in liquid nitrogen and the powder was extracted at 4°C in a 5 mL solution containing 0.1 M potassium phosphate buffer (pH 7.0), 0.1 g polyvinylpolypyrrolidone (PVPP), and 0.1 mmol ethylenediaminetetraacetic acid (EDTA). The homogenate was centrifuged at 18,000 × g for 10 min at 4°C, and the supernatant was kept at −20°C for subsequent enzyme assays.
Superoxide dismutase (SOD, EC 1.15.1.1) activity estimation was based on the ability of SOD to inhibit the photochemical reduction of nitro blue tetrazolium (NBT) and was assayed by the method of Beyer and Fridovich (1987). One unit of SOD was defined as the amount of enzyme required to induce a 50% inhibition of NBT reduction at 25 °C. The activity of SOD was expressed at unit min−1 mg−1 protein. Catalase (CAT, EC 1.11.1.6) activity was determined by monitoring the decrease in absorbance at 240 nm for 3 min following the consumption of H2O2 (Aebi, 1984). The reaction mixture consisted of 0.1 M potassium phosphate buffer (pH 7.0), 0.1 mM EDTA, 20 mM H2O2 and 100 μL of enzyme extract in a 2 mL volume. The activity of ascorbate peroxidase (APX, EC 1.11.1.11) was measured according to the method of Amako et al. (1994). APX was assayed as a decrease in absorbance at 290 nm for 1 min. The assay mixture contained 50 mM potassium phosphate buffer (pH 7.0), 0.5 mM H2O2, 0.1 mM ascorbate, and 100 μL of enzyme extract. The reaction was started by adding the enzyme extract and the decrease in absorbance was recorded. Peroxidase (POD, EC 1.11.1.7) activity was measured using the guaiacol test by following the change of absorbance at 470 nm. The activity was assayed for 3 min in a reaction solution containing 100 mM potassium phosphate buffer (pH 7.0), 20 mM guaiacol, 10 mM H2O2, and 0.1 mL enzyme extract in a 3 mL volume (Polle et al., 1994). The protein concentration in the different extracts was determined following the protocol of Bradford (1976) by using bovine serum albumin (BSA) as a standard.
The experiment data presented are mean values based on five replicates ± standard error (SE) per treatment. Statistical analysis was carried out with the software package SPSS 10.0 for Windows. All results were subjected to a three-way multivariate analysis of variance (MANOVA) for main effects (Salinity, S; Compost, C; AMF inoculation, AMF) and their interactions. A principal component analysis (PCA) was performed using XLSTAT-software v. 2016, to explore the different interactions among variables and different applied treatments. The comparisons among means were assessed using Duncan's test calculated at P < 0.05.
Salinity had a significant adverse (P < 0.001) (Table 1 and Figure S1) effect on the growth of date palms by reducing plant height, the number of leaves, leaf area and shoot and root dry matter (Table 1). The impact of salinity was more pronounced in roots than shoots. Root dry matter was reduced by 42% while shoot dry weight was reduced by 34% compared with non-stressed plants. Application of AMF and compost separately or in combination improved all the plant growth characters affected by salt. The dual application of AMF and compost significantly mitigated the effects of salt stress on all growth parameters followed by the AMF treatment, which in turn was better than the compost treatment. Under the saline condition, the maximum improvement was recorded for the shoot dry matter of plants grown in the presence of AMF and compost with an increase of 226% and the minimum improvement was recorded for the plant height of seedlings grown in the presence of compost with an enhancement of 12% in comparison to untreated stressed plants. Interactions among C × M × S treatments were significant (P < 0.05) for the number of leaves and leaf area (Table 1 and Figure S1).
Table 1. Growth traits and AMF infection of date palm plants under saline and non-saline conditions after application of compost and AMF alone or in combination.
As shown in Table 1, no root colonization was observed in non-inoculated plants. Mycorrhizal colonization was particularly high in the absence of salinity stress and compost (Fa = 100%, Ma = 79%). Under NaCl stress, the frequency and intensity of mycorrhization decreased by 33 and 57%, respectively (P < 0.001). In the same conditions, root colonization was further reduced by the application of compost (Fa by 53% and Ma by 63%). Significant interactions between C×M×S treatment were observed for these two parameters (P < 0.01) (Table 1 and Figure S2).
Results from Figure 1 indicate that the application of the different treatments had considerable effects on the different mineral uptakes of date palm plants under saline and non-saline conditions. P, N, K+, and Ca2+ content was significantly (P < 0.001) decreased with the application of 240 mM NaCl in comparison with the control plants. K+ content was the most affected with ca. 40% decrease and the least affected was P content with ca. 25% decrease. However, the uptakes of Na+ and Cl− were significantly (P < 0.001) higher in salt-affected plants. As a result, the K+/Na+ and Ca2+/Na+ ratios were significantly (P < 0.001) reduced by NaCl stress (Table 2). Under saline stress, the dual application of AMF and compost showed a greater increase in P, N, K+, and Ca2+ nutrients and a maximum decrease in Na+ and Cl− content. This was followed by the application of AMF alone, which recorded a greater increase in P and Ca2+ uptakes and maximum decrease in Na+ content. In contrast, the application of compost alone showed only a high increase in K+ content (Figure 1). Accordingly, and under the same conditions, plants grown in the presence of AMF+compost had greater values for K+/Na+ and Ca2+/Na+ in both shoots and roots compared to the untreated plants, whereas the mycorrhizal plants only showed greater values for Ca2+/Na+ (Table 2). The interaction between C × M × S application had a significant effect on Na+ and K+ uptakes and K+/Na+ ratio (P < 0.05) (Figure 1 and Table 2).
Figure 1. Mineral concentrations (A, sodium, Na+; B, chloride, Cl−; C, phosphorous, P; D, nitrogen, N; E, potassium, K+; and F, calcium, Ca2+) of date palm plants under saline and non-saline conditions after application of compost and AMF alone or in combination. The values of each parameter labeled by different letters indicate significant differences assessed by Duncan's test after performing a three-way MANOVA (P < 0.05). nsnon-significant; *significant at P < 0.05; **significant at P < 0.005; ***significant at P < 0.001.
Table 2. Shoot and root K/Na and Ca/Na ratios of date palm plants under saline and non-saline conditions after application of compost and AMF alone or in combination.
The effect of salt stress and non-saline treatment on leaf relative water content (LRWC), leaf water potential (LWP), stomatal conductance (gs), and photosynthetic efficiency variables is shown in Table 3. In general, the untreated date palm plants were more sensitive to salinity than treated plants. The parameter most affected by salinity was gs, which decreased 42% in non-treated stressed plants. Under salt stress, LRWC and photosynthetic efficiency recorded the maximum improvement (20 and 92%, respectively) with mycorrhizal plants and best leaf water potential and stomatal conductance enhancement (53 and 107%, respectively) were obtained with the AMF+compost application. Interactions among C×M×S treatment had a significant effect (P < 0.001) on stomatal conductance and photosynthetic efficiency (Table 3). Salt stress caused a severe decrease in the quantum efficiency of photosystem II (Fv/Fm) in non-treated plants. The presence of AMF and/or compost had significantly higher Fv/Fm as compared to untreated plants independently of the salinity conditions.
Table 3. Physiological traits and water status of date palm plants under saline and non-saline conditions after application of compost and AMF alone or in combination.
As shown in Table 4, salinity caused a significant (P < 0.001) decline in photosynthetic pigment concentrations. Indeed, the application of NaCl stress in untreated plants decreased chlorophyll a (Chl a) by 58%, chlorophyll b (Chl b) by 51%, carotenoids by 47%, and total chlorophyll by 56% in comparison with non-stressed plants. The application of compost and AMF separately or in combination significantly improved the content of different photosynthetic pigments under saline conditions. Date palm seedlings grown in the presence of salt stress and the application of AMF alone or in combination with compost yielded marked increases in photosynthetic pigment concentrations (207 and 190%, respectively for Chl a, 222, and 212% for Chl b, 172 and 167%, respectively for carotenoids, and 213 and 198% for total chlorophyll) compared with untreated salt-affected plants. Significant interactions among C × M × S treatment were observed for chlorophyll a and carotenoid content (P < 0.05) (Table 4).
Table 4. Photosynthetic pigment content of date palm plants under saline and non-saline conditions after application of compost and AMF alone or in combination.
There was an apparent significant (P < 0.001) increase in the content of hydrogen peroxide (H2O2) and malondialdehyde (MDA) concentrations (in shoots and roots) with the application of NaCl stress as compared with non-salinized plants (Figure 2). A maximum increase (ca. 40%) was recorded for root H2O2 content and a minimum increase (18%) was observed for root MDA content. In contrast, AMF and/or compost applications reduced those parameters in both stressed and non-stressed date palm seedlings as compared to untreated plants. Indeed, the lowest significant values were recorded by plants treated with AMF+compost followed by AMF-inoculated plants and finally plants treated with compost.
Figure 2. Hydrogen peroxide and MDA content in the shoot (A,C) and the root (B,D) of date palm plants under saline and non-saline conditions after application of compost and AMF alone or in combination. The values of each parameter labeled by different letters indicate significant differences assessed by Duncan's test after performing three-way ANOVA (P < 0.05). nsnon-significant; *significant at P < 0.05; **significant at P < 0.005; ***significant at P < 0.001.
The results related to the effect of salt stress on proline and soluble sugar content in date palm seedlings are presented in Figure 3. Plants exposed to salinity showed significantly (P < 0.001) greater proline and soluble sugar concentrations in comparison with non-exposed plants. In the presence of NaCl stress, application of AMF alone or in combination with compost led to the highest percentage improvement of soluble sugar in shoots (32 and 41%, respectively) and roots (ca. 90% in both), while there were no significant differences in root proline content between untreated and plants treated with the dual application.
Figure 3. Proline and soluble sugar content in the shoot (A,C) and the root (B,D) of date palm plants under saline and non-saline conditions after application of compost and AMF alone or in combination. The values of each parameter labeled by different letters indicate significant differences assessed by Duncan's test after performing three-way ANOVA (P < 0.05). nsnon-significant; *significant at P < 0.05; **significant at P < 0.005; ***significant at P < 0.001.
Exposure of date palm plants to salt stress led to a significant (P < 0.001) increase in antioxidant enzymes APX, CAT, POD, and SOD in the shoot (Table 5) and root (Table 6) activity. POD activity was the most affected by salinity and recorded a maximum increase of 62% in roots compared to non-salt affected plants. A further significant increase was observed in antioxidant enzyme activity in plants treated with AMF and compost separately or in combination under saline condition. The highest significant improvement was recorded with the dual application of AMF and compost followed by AMF inoculated plants and then compost amended plants. This improvement was more marked in the roots than in the shoots. CAT activity was the activity most sensitive to the treatments applied and showed an enhancement of 74% in AMF treated plant shoots in comparison to untreated plants under salinity stress. C×M×S treatment effects on antioxidant enzymes activity were significant (P < 0.001) for root POD activity (Tables 5, 6).
Table 5. Shoot antioxidant enzyme activity (APX, CAT, POD, and SOD) of date palm plants under saline and non-saline conditions after application of compost and AMF alone or in combination.
Table 6. Root antioxidant enzyme activity (APX, CAT, POD, and SOD) of date palm plants under saline and non-saline conditions after application of compost and AMF alone or in combination.
Principal component analysis (PCA) took into account all the analyzed parameters of the different treatments. Two main components accounted for 91% of the variability observed in the data with 60% for PC1 and 31% for PC2 (Figure 4 and Table S1). Under the non-saline condition, PCA analysis showed a positive correlation among the different biofertilizers (applied separately or combined) for growth, physiological and water parameters, AMF infection, nutrient (N, P, K, and Ca, and K/Na and Ca/Na ratios), photosynthetic pigments, and protein content, which were highly correlated to each other. The analysis also confirmed the negative impact of salt concentration on these parameters. The biplot revealed the positive correlation linking salt treatment and Na, Cl, MDA, and H2O2 content. Under saline conditions, PCA showed a clear positive correlation between the antioxidant system (antioxidant enzyme and osmolytes) and the AMF application alone or combined with compost.
Figure 4. Principal component analysis of the studied traits of different biofertilizer treatments in date palm plants growing under saline and non-saline conditions. S, Salinity; M, arbuscular mycorrhizal fungi; C, compost; PH, plant height; NL, number of leaves; LA, leaf area; SDW, shoot dry weight; RDW, root dry weight; Fa, AMF infection frequency; Ma, AMF infection intensity; Na, sodium uptake; Cl, chloride uptake; P, phosphorous uptake; N, nitrogen uptake; K, potassium uptake; Ca, calcium uptake; LRWC, leaf relative water content; LWP, leaf water potential; gs, stomatal conductance; Fv/Fm, photosynthetic efficiency. Chl a, chlorophyll a; Chl b, chlorophyll b; Chl T, total chlorophyll; Car T, carotenoids; S-K/Na, shoot K/Na ratio; R-K/Na, root K/Na ratio; S-Ca/Na, shoot Ca/Na ratio; R-Ca/Na, root Ca/Na ratio; S-H2O2, shoot H2O2 content; R-H2O2, root H2O2 content S-MDA, shoot MDA content; R-MDA, root MDA content; S-Proline, shoot proline content; R-Proline, root proline content; S-Sugar, shoot sugar content; R-Sugar, root sugar content; S-APX, shoot ascorbate peroxidase activity; R-APX, root ascorbate peroxidase activity; S-CAT, shoot catalase activity; R-CAT, root catalase activity; S-POD, shoot peroxidase activity; R-POD, root peroxidase activity; S-SOD, shoot superoxide dismutase activity; R-SOD, root superoxide dismutase activity; S-Protein, shoot protein content; R-Protein, root protein content.
The present study confirms, for the first time, the synergistic effect of AMF inoculation and the addition of green waste compost on improving date palm tolerance to salinity. Organic amendments (Tartoura et al., 2014; Mbarki et al., 2018) and AMF inoculation (Ait-El-Mokhtar et al., 2019; Ben Laouane et al., 2019) have been reported to confer certain complex beneficial changes on plant growth and physiological and biochemical characters under salt stress. Adverse effects of salinity on date palm growth and development have been reported by many authors (Sperling et al., 2014; Yaish and Kumar, 2015; Ait-El-Mokhtar et al., 2019; Al Kharusi et al., 2019). In the present investigation, salinity stress significantly reduced date palm growth parameters, with the impact of salinity being more pronounced in roots than shoots. Previous studies showed that salt stress adversely affects the establishment, growth, and development of plants due to osmotic stress and disrupts many metabolic processes (Porcel et al., 2012; Abdel Latef et al., 2019a,b; Evelin et al., 2019). Roots are in direct contact with the soil solution from which they absorb minerals and water. Thus, they are the first to encounter the saline medium and play a key role in transporting these elements to the leaves. In the context of salt tolerance, roots are the first site of defense against NaCl and may directly limit or exclude sodium uptake (Munns, 2005; Liu et al., 2015; Rana et al., 2019). Another important aspect of root systems compared to shoots is their anatomy. The stele tissues in roots are internally surrounded by cortex and epidermis, while they are distributed throughout parenchyma cells in the shoots. Evidence has emerged that the root cortex must do the heavy lifting of excluding Na+ from shoots, which requires an energy cost (ATP) used by antiporters as part of a tissue tolerance mechanism (Munns et al., 2020). Further, salt typically affects root elongation and architecture by reducing cell size and cell division and altering differentiation patterns (Potters et al., 2007; Bernstein, 2013). AMF inoculation and compost amendment significantly mitigated the inhibitory effects of salinity on growth which may be because AMF can acquire nutrients released from the composted waste (Hodge et al., 2001; Cavagnaro, 2014). The application of AMF and/or compost might improve the physicochemical and biological characteristics of the soil (Rillig and Mummey, 2006; Weber et al., 2014), and enhance the release of nutrients in the soil, which makes them more available to the plant (Astiko et al., 2013; Baslam et al., 2014). Furthermore, the spreading of AMF hyphae, beyond the root zone, provides P and other immobile nutrients to plants and thereby promotes biomass production (Porcel et al., 2012).
Under saline stress, date palm growth parameters were mostly enhanced by the dual application of AMF and compost although salinity and compost amendment hampered AMF root colonization. Zhang et al. (2017) reported that mycorrhizal colonization of trifoliate orange (Citrus trifoliate L.) root was negatively affected by salinity stress which was attributed to the direct effect of NaCl on the fungi. Several authors have also shown that salinity reduces mycorrhizal colonization through inhibition of spore germination and hyphal growth or reduction of the spread of mycorrhizal colonization and the number of arbuscules (Hajiboland et al., 2010; Evelin et al., 2019). Likewise, a reduced percentage of colonization of roots by AMF in the substrate amended with compost has been reported in other research (Graceson et al., 2014; Watts-Williams and Cavagnaro, 2014). This could be explained by the release of larger amounts of mineralized P into the soil, which can result in a reduced colonization (Baslam et al., 2011b; Lehmann et al., 2011). Besides, composted organic material comprises products of decomposition, which can induce inhibition of mycorrhizal fungi (Gryndler et al., 2008).
The adverse effects of salinity stress on plant growth could be related to nutrient imbalances (Evelin et al., 2019). In this study, the uptake of P, N, K+, and Ca2+ and K+/Na+ and Ca2+/Na+ ratios in date palm seedlings was lower under salinity stress. On the other hand, Na+ and Cl− content was higher in plants irrigated with saline water—factors which induce the ionic imbalance and disrupt photosynthesis and other metabolic functions of the cell (Ramoliya et al., 2004). AMF and compost applications have been shown to affect plant physiological processes, especially nutrient absorption, and therefore plant tolerance to salt stress (Abdel Latef et al., 2016; Chaichi et al., 2017; Enebe and Babalola, 2018; Mbarki et al., 2018). In this investigation, the improvements in nutrient uptake (K+, P, N, and Ca2+) under saline conditions were greatest with the dual application of AMF and compost. Improvements were less marked with AMF inoculation and even fewer with just compost treatments. Chaichi et al. (2017) demonstrated that tomato plants grown in the presence of compost tea enriched with AMF showed enhanced cation acquisition, including K+, Ca2+, and Mg2+, under salt stress. In the same vein, Sallaku et al. (2019) reported an obvious increase in the uptake of P, K+, and Ca2+ in AM cucumber seedlings, while AMF inoculation reduced the uptake of Na+. Furthermore, it has been reported that organic matter amendments improve N and P concentrations in plants grown in saline soils (Mbarki et al., 2018). The improvement of date palm tolerance to salinity by increasing P might be attributable to the role of P uptake in preserving the integrity of cell membranes and in facilitating the compartmentalization of toxic ions in vacuoles (Bothe, 2012). Moreover, the inhibition of Na+ absorption through roots in mycorrhizal plants and/or the decrease of Na+ mobility in compost-amended soil might be considered other strategies that improve salt adaptation in plants (Enebe and Babalola, 2018). AMF and compost application can alleviate Na+ and Cl− toxicity. For example, vacuoles of AMF vesicles can store different ions such as sodium and chloride under salinity constraints (Miransari, 2010). Also, the organic amendment is directly related to mechanisms for Na+ removal from soil such as Na+ leaching and the Na+ adsorption ratio ratio (Chaganti and Crohn, 2015; Drake et al., 2016). Our study demonstrated that the K+/Na+ and Ca2+/Na+ ratios in mycorrhizal date palm seedlings, especially in the presence of the compost application, were increased under saline conditions. The higher K+/Na+ and Ca2+/Na+ ratios were found to be a key parameter to alleviate salt stress in plants, since Na+ ions may compete with K+ and Ca2+ ions for membrane transport sites (Evelin et al., 2019). Maintenance of a higher K+/Na+ and Ca2+/Na+ ratios in the cytosol is a crucial aspect for the protecting physiological cellular functioning from detrimental salinity changes (Ahanger et al., 2015). Greater values of these ratios protect photosynthetic tissues by blocking Na+ entry (Cuin et al., 2011) and improve hydraulic conductivity and Ca2+ signaling pathways (Lovelock and Ball, 2006).
Salt stress affects the photosynthesis apparatus in many ways. The osmotic stress induced by salinity reduces stomatal conductance, which decreases the availability and assimilation of CO2 (Chaves et al., 2009). Salinity disrupts the photochemical reactions of photosynthesis, especially PSII (Hidri et al., 2016). Our study showed a decline in photosynthetic pigment content (Chl a, Chl b, carotenoids, and total chlorophyll) coupled with a decrease in photosynthetic efficiency (Fv/Fm) under saline conditions. This adverse effect is linked to the destruction of chloroplast as a direct effect of salt stress (Zörb et al., 2009) and the decrease in the activity of photosynthetic pigment synthesizing enzymes (Murkute et al., 2006). The reduced chlorophyll content may be attributed to the low uptake of minerals, especially magnesium, in the presence of salinity (Giri and Mukerji, 2004). Application of AMF and compost resulted in a significant increase in photosynthetic attributes such as chlorophyll pigment content and stomatal conductance, reflecting a significant improvement in photosynthetic efficiency. The enhancement of N uptake owing to the AMF and compost treatment might improve photosynthetic attributes because N is considered a key component of the Rubisco enzyme (Ahanger et al., 2014). Rao and Chaitanya (2016) reported that the presence of Mg2+ due to organic amendment and subsequent increase in its uptake due to AMF application could be possible reasons for greater photosynthetic capacity. This might also be attributed to the accumulation of proline and glycine betaine in mycorrhizal plants which protects PSII pigment-protein complexes as well as CO2-fixing enzymes such as RuBisCO and rubisco activase (Talaat and Shawky, 2014).
Salinization not only affected photosynthetic capacity, but also the water status of the seedlings. Plants grown under saline conditions showed a significant decrease in LRWC and leaf water potential. This decline in water status is related to the water stress generated by salinity since salt immobilizes water and makes it unavailable for the plant roots (Füzy et al., 2008). However, AMF and compost application significantly improved the water status of the plant under salt stress, as indicated by the greater LRWC and less negative leaf water potential values. This may be explained by improved hydraulic conductivity and water absorption capacity mediated by the mycorrhizae even under saline conditions (Kapoor et al., 2008). Medina and Azcón (2010) suggested that organic amendment and AMF symbiosis were able to change soil structure by inducing aggregate formation which improves water availability in soil.
The decline in date palm biomass under saline condition was accompanied by increased levels of MDA and H2O2. Our results are supported by earlier studies on two cultivars of P. dactylifera L. cv. Umsila and cv. Zabad (Al Kharusi et al., 2019), Cicer arietinum L. (Rasool et al., 2013), Capsicum annuum L. (Abdel Latef and Chaoxing, 2014), and Ephedra aphylla (Alqarawi et al., 2014). Root tissues exhibited higher ROS accumulation and oxidative damage than shoot tissues. Plants treated with NaCl in combination with AMF and compost application showed a smaller increase in MDA and H2O2 concentrations. The same result was reported by Rasool et al. (2013) and Tartoura et al. (2014) in the presence of salt stress. This effect can be linked to improvements in the antioxidant enzyme system which scavenges the ROS before they react with membrane lipids thus reducing lipid peroxidation. Proline and sugars figure among the most commonly used metabolites to assess the degree of response to salt stress (Evelin et al., 2019). In the present study, under stress conditions, date palm plants showed enhanced proline and soluble sugar content, which was further enhanced by the application of AMF and compost. This may be a defensive reaction which improves the resistance of date palm seedlings to salt stress by maintaining osmotic balance and mitigating the free radical damage caused by osmotic stress (Ahanger et al., 2014). In the presence of NaCl, AMF colonization could set in motion mechanisms that launch improved proline synthesis and therefore a better tolerance of the plant to salt stress (Hashem et al., 2016). Our findings of an increase in proline and soluble sugar concentrations following the AMF and compost application under saline stress confirm the findings of Chinsamy et al. (2013) and Talaat and Shawky (2014). Proline has been shown to act as a chemical protein chaperone and to prevent protein aggregation under salt stress (Ignatova and Gierasch, 2006). The enhancement of proline in plants treated with AMF and compost helped to protect against oxidative stress given its involvement in the stabilization of redox enzymes. Hoque et al. (2008) reported that the exogenous application of proline to cell cultures was positively correlated with the increased activity of different antioxidant enzymes (i.e SOD, CAT, and (ASC)-GSH cycle-related enzymes) under saline conditions.
Salinity tolerance in plants has been associated with the induction of the antioxidant enzyme system and the reduction of oxidative damage. Here, we show that NaCl stress significantly increased the antioxidant enzyme activity (SOD, POD, APX, and CAT) of the date palm, and this activity was further increased in treated plants, particularly those grown with the dual application of AMF and compost. Similar results were reported previously in other species (Hashem et al., 2015; Hidri et al., 2016; Ramzani et al., 2017; Ben Laouane et al., 2019). SOD is considered one of the first enzymes of defense and enables the scavenging of superoxide radicals into hydrogen peroxide which is further detoxified to water (Mittler, 2002). The conversion of H2O2 to H2O can be catalyzed by the action of enzymes, such as CAT, APX, and POD (Mittler, 2002). In our study, the increase in SOD activity was consistent with the improvement in the activity of hydrogen peroxide- scavenging enzymes (CAT, POD, and APX). Furthermore, the induction of the activity of antioxidant enzymes in plants treated with AMF and compost compared with untreated plants was associated with lower H2O2 content and lipid peroxidation, thereby indicating an efficient ROS scavenging system in the date palm through a reduction in oxidative stress and less membrane damage in the treated plants.
Taken together, the results indicate that the application of compost and AMF alleviated salt stress in date palm crops in a synergistic manner and through physiological and biochemical processes (Figure 4). Even if the organic amendment decreased AMF root colonization, the combined effect of compost and AMF seems to be the most efficient way to boost the tolerance of date palm seedlings to salinity. Indeed, AMF and compost alone or in combination (right side of PC1) appear to confer multiple forms of protection to the date palm, as these application are positively correlated with growth-related parameters, plant photosynthetic traits, and nutrients homeostasis. AMF and/or compost were negatively associated with MDA and H2O2. Under stress, the combination AMF+compost was positively associated (PC2 axis) with antioxidant enzyme (SOD, POD, and CAT) activity and proline biosynthesis. These associations suggest that the application of microbial inocula and compost co-occurred with enhancement in leaf gas exchange, induction in the photosynthetic apparatus and increased accumulation of pigments associated with light harvest, and alleviation of stress damages by regulating the antioxidant enzymes under high-salinity. This finding is supported by the fact that at high-salinity (240 mM NaCl) concomitant protein degradation occurred in untreated plants (as a single group, negatively correlated with agro-physiological and growth parameters) that lost antioxidant enzyme activity, while the high salt-treated plants still had a strong scavenging system consisting of antioxidative molecules. As a result, AMF and compost improved water relations without penalizing plant growth under salt stress. Figure 5 gives an overview of the various mechanisms commonly elicited by compost and AMF which systemically counter the deleterious effects of salt stress and rectifies them, thereby ultimately improving plant growth and productivity. Date palm plants growing under saline conditions showed an ionic imbalance, physiological drought, and oxidative stress, which leads to reduced biomass. Although there are similarities in the mechanisms induced by the application of compost and AMF to alleviate all the negative effects of salinity, there are, however, slight variations underlying the basic mechanisms involved when both biofertilizers are used. AMF can improve the water and nutrient status of host plants via the boosting of nutrient and water uptake by the hyphal structure effect and the activity of ion and water transporters. Similarly, compost also raises nutrient concentrations in plants, since it is a source of mineral elements (N, P, and K). Furthermore, compost may improve water uptake by increasing soil water holding capacity. The improvement of nutrient and hydraulic conductance alleviates the salinity-induced growth effects in plants. AMF and compost can also mitigate the toxicity of Na+ and Cl− by preventing these ions from interfering with the plant root system. Also, AMF symbiosis and compost amendment can alleviate osmotic and oxidative stress by stimulating osmolyte synthesis and antioxidant enzymes and by reducing oxidative stress marker levels (H2O2 and MDA). Furthermore, increased stomatal conductance along with chlorophyll content enhancement and PSII efficiency contribute to boost the photosynthesis machinery necessary for plant performance under saline conditions. The symbiotic association of AMF together with compost (5% W/W with respect to culture soil) might have influenced positive plant growth by an increase in nutrient supply. The addition of compost alters the soil pH and in turn, affects nutrient availability to plants. The positive effects of the compost on AMF derived from altering the physicochemical properties of soil, changes in nutrient dynamics—mainly phosphorous availability—, the release of organic compounds, increased water content and plant available water, root hydraulic conductivity, and changes in the composition of root exudates might all have contributed to a significant increase in the plants' performance under normal and saline conditions as compared to untreated plants or those containing AMF or compost alone (Akhter et al., 2015; Kohler et al., 2015; Kranz et al., 2020). Mimmo et al. (2011) have shown the substrate-dependent variations in plants root exudation patterns. The altered response could be due to the accumulation of new compounds in root exudates which may have a stimulatory effect on mycelial growth and development (Scheffknecht et al., 2006). The growth advantage conferred by the mycorrhizal consortium in the amended soil might be related to the capacity of the fungi to acquire nutrients slow-released from the composted residue (Cavagnaro, 2014). El Kinany et al. (2019) found that the mixture of compost and AMF contributed to uptake of mobile micronutrient boron, which has a crucial role in cell wall formation and stability, maintenance of structural and functional integrity of biological membranes, movement of sugar or energy into growing parts of plants (Takano et al., 2002; Shireen et al., 2018).
Figure 5. Schematic representation of various mechanisms induced by AMF and compost application in date palm plants under salt stress.
Our results provide an experimental basis for the application of compost and AMF fertilizers as a sustainable method for the remediation of salt-affected soils in arid and semi-arid areas, especially in fragile ecosystems such as palm grove oases.
Salinity stress reduced growth, physiological, and biochemical traits of date palm seedlings considerably. AMF-inoculated plants grown in compost-amended soil exhibited large improvements in these parameters under NaCl stress. The application of AMF and compost separately or in combination increased water status parameters, photosynthetic efficiency, and the concentration of photosynthetic pigments under saline conditions by enhancing N, P, K+, and Ca2+ uptake and reducing Na+ and Cl− content. The synergy between AMF and compost in salinity tolerance was manifested in the improvement in growth, soil nutrient status, and physio-biochemical traits together with the decrease in MDA and H2O2 as compared to no-mycorhizal and compost-free plants. This dual application alleviated the oxidative stress induced by salinity by stimulating antioxidant enzyme activity and therefore the reduction of hydrogen peroxide and lipid peroxidation. All these beneficial effects protected membrane integrity and the different cell components from the adverse effects of salinity. The combined treatment of AMF and compost yielded the greatest improvement in the parameters measured in date palm seedlings under salt stress, with the application of AMF alone in second place and then finally that application of compost alone. Our findings highlight the importance of considering the dual application of AMF and compost to alleviate the detrimental effects of salinity and promote plant growth in salinized soils in arid and semiarid areas.
All datasets generated for this study are included in the article/Supplementary Material.
AM, SW, and MB: conceptualization, validation, supervision. MA-E-M, AM, SW, and MB: methodology. MA-E-M, RB-L, MA, and AB: formal analysis. MA-E-M and MB: writing original draft preparation. AM, SW, MB, and TM: project administration. MB and TM: funding acquisition. All authors have read and agreed to the published version of the manuscript.
This research was partially supported by EIG CONCERT- Japan 3rd Joint Call on Food Crops and Biomass Production Technologies under the Strategic International Research Cooperative Program of the Japan Science and Technology Agency (JST), and by a Grant for Promotion of KAAB Projects (Niigata University) from the Ministry of Education, Culture, Sports, Science, and Technology, Japan. The funders had no role in the data collection, decision to publish, or preparation of the manuscript.
The authors declare that the research was conducted in the absence of any commercial or financial relationships that could be construed as a potential conflict of interest.
The Supplementary Material for this article can be found online at: https://www.frontiersin.org/articles/10.3389/fsufs.2020.00131/full#supplementary-material
Abdel Latef, A. A. H., Abu Alhmad, M. F., Kordrostami, M., Abo–Baker, A. B. A. E., and Zakir, A. (2020). Inoculation with Azospirillum lipoferum or Azotobacter chroococcum reinforces maize growth by improving physiological activities under saline conditions. J. Plant Growth Regul. 1–14. doi: 10.1007/s00344-020-10065-9
Abdel Latef, A. A. H., and Chaoxing, H. (2011). Effect of arbuscular mycorrhizal fungi on growth, mineral nutrition, antioxidant enzymes activity and fruit yield of tomato grown under salinity stress. Sci. Hortic. 127, 228–233. doi: 10.1016/j.scienta.2010.09.020
Abdel Latef, A. A. H., and Chaoxing, H. (2014). Does inoculation with Glomus mosseae improve salt tolerance in pepper plants? J. Plant Growth Regul. 33, 644–653. doi: 10.1007/s00344-014-9414-4
Abdel Latef, A. A. H., Hashem, A., Rasool, S., Abd-Allah, E. F., Alqarawi, A. A., Egamberdieva, D., et al. (2016). Arbuscular mycorrhizal symbiosis and abiotic stress in plants: a review. J. Plant Biol. 59, 407–426. doi: 10.1007/s12374-016-0237-7
Abdel Latef, A. A. H., Kordrostami, M., Zakir, A., Zaki, H., and Saleh, O. M. (2019a). Eustress with H2O2 facilitates plant growth by improving tolerance to salt stress in two wheat cultivars. Plants 8:303. doi: 10.3390/plants8090303
Abdel Latef, A. A. H., Mostofa, M. G., Rahman, M. M., Abdel-Farid, I. B., and Tran, L. S. P. (2019b). Extracts from yeast and carrot roots enhance maize performance under seawater-induced salt stress by altering physio-biochemical characteristics of stressed plants. J. Plant Growth Regul. 38, 966–979. doi: 10.1007/s00344-018-9906-8
Aebi, H. (1984). Catalase in vitro. Methods Enzymol. 105, 121–126. doi: 10.1016/S0076-6879(84)05016-3
Ahanger, M. A., Agarwal, R. M., Tomar, N. S., and Shrivastava, M. (2015). Potassium induces positive changes in nitrogen metabolism and antioxidant system of oat (Avena sativa L cultivar Kent). J. Plant Interact. 10, 211–223. doi: 10.1080/17429145.2015.1056260
Ahanger, M. A., Hashem, A., Abd-Allah, E. F., and Ahmad, P. (2014). “Arbuscular mycorrhiza in crop improvement under environmental stress,” in Emerging Technologies and Management of Crop Stress Tolerance, Vol. 2, ed P. Ahmad (San Diego, CA: Elsevier Inc.), 69–95. doi: 10.1016/B978-0-12-800875-1.00003-X
Ait-El-Mokhtar, M., Ben Laouane, R., Anli, M., Boutasknit, A., Wahbi, S., and Meddich, A. (2019). Use of mycorrhizal fungi in improving tolerance of the date palm (Phoenix dactylifera L.) seedlings to salt stress. Sci. Hortic. 253, 429–438. doi: 10.1016/j.scienta.2019.04.066
Akhter, A., Hage-Ahmed, K., Soja, G., and Steinkellner, S. (2015). Compost and biochar alter mycorrhization, tomato root exudation, and development of Fusarium oxysporum f. sp. lycopersici. Front. Plant Sci. 6:529. doi: 10.3389/fpls.2015.00529
Al Kharusi, L., Al Yahyai, R., and Yaish, M. (2019). Antioxidant response to salinity in salt-tolerant and salt-susceptible cultivars of date palm. Agriculture 9:8. doi: 10.3390/agriculture9010008
Alqarawi, A. A., Abd Allah, E. F., and Hashem, A. (2014). Alleviation of salt-induced adverse impact via mycorrhizal fungi in Ephedra aphylla Forssk. J. Plant Interact. 9, 802–810. doi: 10.1080/17429145.2014.949886
Amako, K., Chen, G.-X., and Asada, K. (1994). Separate assays specific for ascorbate peroxidase and guaiacol peroxidase and for the chloroplastic and cytosolic isozymes of ascorbate peroxidase in plants. Plant Cell Physiol. 35, 497–504.
Anli, M., Symanczik, S., El Abbassi, A., Ait-El-Mokhtar, M., Boutasknit, A., Ben-Laouane, R., et al. (2020). Use of arbuscular mycorrhizal fungus Rhizoglomus irregulare and compost to improve growth and physiological responses of Phoenix dactylifera “Boufgouss.” Plant Biosyst. 1–14. doi: 10.1080/11263504.2020.1779848
Apse, M. P., and Blumwald, E. (2007). Na+ transport in plants. FEBS Lett. 581, 2247–2254. doi: 10.1016/j.febslet.2007.04.014
Arnon, D. I. (1949). Copper enzymes in isolated chloroplasts. polyphenoloxidase in Beta vulgaris. Plant Physiol. 24, 1–15. doi: 10.1104/pp.24.1.1
Astiko, W., Sastrahidayat, I. R., Djauhari, S., and Muhibuddin, A. (2013). The role of indigenous mycorrhiza in combination with cattle manure in improving maize yield (Zea mays L) on sandy loam of Northern Lombok, Eastern of Indonesia. J. Trop. Soils 18, 53–58. doi: 10.5400/jts.2013.18.1.53
Augé, R. M., Toler, H. D., and Saxton, A. M. (2014). Arbuscular mycorrhizal symbiosis alters stomatal conductance of host plants more under drought than under amply watered conditions: a meta-analysis. Mycorrhiza 25, 13–24. doi: 10.1007/s00572-014-0585-4
Baker, N. R. (2008). Chlorophyll fluorescence: a probe of photosynthesis in vivo. Annu. Rev. Plant Biol. 59, 89–113. doi: 10.1146/annurev.arplant.59.032607.092759
Barrs, H. D., and Weatherly, P. E. (1962). A re-examination of the relative turgidity technique for estimating water deficits in leaves. Aust. J. Biol. Sci. 15, 413–428. doi: 10.1071/BI9620413
Baslam, M., Garmendia, I., and Goicoechea, N. (2011a). Arbuscular mycorrhizal fungi (AMF) improved growth and nutritional quality of greenhouse-grown Lettuce. J. Agric. Food Chem. 59, 5504–5515. doi: 10.1021/jf200501c
Baslam, M., Garmendia, I., and Goicoechea, N. (2012). Elevated CO2 may impair the beneficial effect of arbuscular mycorrhizal fungi on the mineral and phytochemical quality of lettuce. Ann. Appl. Biol. 161, 180–191. doi: 10.1111/j.1744-7348.2012.00563.x
Baslam, M., and Goicoechea, N. (2012). Water deficit improved the capacity of arbuscular mycorrhizal fungi (AMF) for inducing the accumulation of antioxidant compounds in lettuce leaves. Mycorrhiza 22, 347–359. doi: 10.1007/s00572-011-0408-9
Baslam, M., Pascual, I., Sánchez-Díaz, M., Erro, J., García-Mina, J. M., and Goicoechea, N. (2011b). Improvement of nutritional quality of greenhouse-grown lettuce by arbuscular mycorrhizal fungi is conditioned by the source of phosphorus nutrition. J. Agric. Food Chem. 59, 11129–11140. doi: 10.1021/jf202445y
Baslam, M., Qaddoury, A., and Goicoechea, N. (2014). Role of native and exotic mycorrhizal symbiosis to develop morphological, physiological and biochemical responses coping with water drought of date palm, Phoenix dactylifera. Trees Struct. Funct. 28, 161–172. doi: 10.1007/s00468-013-0939-0
Bates, L. S., Aldren, R. P., and Teare, L. D. (1973). Rapid determination of free proline for water-stress studies. Plant Soil 207:2104.
Ben Laouane, R., Meddich, A., Bechtaoui, N., Oufdou, K., and Wahbi, S. (2019). Effects of arbuscular mycorrhizal fungi and rhizobia symbiosis on the tolerance of Medicago sativa to salt stress. Gesunde Pflanz. 71, 135–146. doi: 10.1007/s10343-019-00461-x
Benhiba, L., Fouad, M. O., Essahibi, A., Ghoulam, C., and Qaddoury, A. (2015). Arbuscular mycorrhizal symbiosis enhanced growth and antioxidant metabolism in date palm subjected to long-term drought. Trees 29, 1725–1733. doi: 10.1007/s00468-015-1253-9
Bernstein, N. (2013). “Effects of salinity on root growth plant roots,” in Plant Roots: The Hidden Half, 4th Edn., Vol. 36, eds A. Eshel and T. Beeckman (Boca Raton, FL: CRC Press), 1–18. doi: 10.1201/b14550-42
Berruti, A., Lumini, E., Balestrini, R., and Bianciotto, V. (2016). Arbuscular mycorrhizal fungi as natural biofertilizers: Let's benefit from past successes. Front. Microbiol. 6, 1–13. doi: 10.3389/fmicb.2015.01559
Beyer, W. F., and Fridovich, I. (1987). Assaying for superoxide dismutase activity: some large consequences of minor changes in conditions. Anal. Biochem. 161, 559–566. doi: 10.1016/0003-2697(87)90489-1
Bothe, H. (2012). Arbuscular mycorrhiza and salt tolerance of plants. Symbiosis 58, 7–16. doi: 10.1007/s13199-012-0196-9
Bouamri, R., Dalpé, Y., and Serrhini, M. N. (2014). Effect of seasonal variation on arbuscular mycorrhizal fungi associated with date palm. Emirates J. Food Agric. 26, 977–986. doi: 10.9755/ejfa.v26i11.18985
Boutasknit, A., Baslam, M., Ait-El-mokhtar, M., Anli, M., Ben-Laouane, R., Douira, A., et al. (2020). Arbuscular mycorrhizal fungi mediate drought tolerance and recovery in two contrasting carob (Ceratonia siliqua l.) ecotypes by regulating stomatal, water relations, and (in)organic adjustments. Plants 9:80. doi: 10.3390/plants9010080
Bowles, T. M., Jackson, L. E., Loeher, M., and Cavagnaro, T. R. (2017). Ecological intensification and arbuscular mycorrhizas: a meta-analysis of tillage and cover crop effects. J. Appl. Ecol. 54, 1785–1793. doi: 10.1111/1365-2664.12815
Bradford, M. M. (1976). A rapid and sensitive method for the quantitation of microgram quantities of protein utilizing the principle of protein-dye binding. Anal. Biochem. 72, 248–254. doi: 10.1016/0003-2697(76)90527-3
Cavagnaro, T. R. (2014). Impacts of compost application on the formation and functioning of arbuscular mycorrhizas. Soil Biol. Biochem. 78, 38–44. doi: 10.1016/j.soilbio.2014.07.007
Chaganti, V. N., and Crohn, D. M. (2015). Evaluating the relative contribution of physiochemical and biological factors in ameliorating a saline–sodic soil amended with composts and biochar and leached with reclaimed water. Geoderma 259–260, 45–55. doi: 10.1016/j.geoderma.2015.05.005
Chaichi, M. R., Keshavarz-Afshar, R., Lu, B., and Rostamza, M. (2017). Growth and nutrient uptake of tomato in response to application of saline water, biological fertilizer, and surfactant. J. Plant Nutr. 40, 457–466. doi: 10.1080/01904167.2016.1246567
Chao, C. T., and Krueger, R. R. (2007). The date palm (Phoenix dactylifera L.): Overview of biology, uses, and cultivation. HortScience 42, 1077–1082. doi: 10.21273/HORTSCI.42.5.1077
Chaves, M. M., Flexas, J., and Pinheiro, C. (2009). Photosynthesis under drought and salt stress: Regulation mechanisms from whole plant to cell. Ann. Bot. 103, 551–560. doi: 10.1093/aob/mcn125
Chinsamy, M., Kulkarni, M. G., and Van Staden, J. (2013). Garden-waste-vermicompost leachate alleviates salinity stress in tomato seedlings by mobilizing salt tolerance mechanisms. Plant Growth Regul. 71, 41–47. doi: 10.1007/s10725-013-9807-6
Cuin, T. A., Zhou, M., Parsons, D., and Shabala, S. (2011). Genetic behaviour of physiological traits conferring cytosolic K+/Na+ homeostasis in wheat. Plant Biol. 14, 438–446. doi: 10.1111/j.1438-8677.2011.00526.x
Daei, G., Ardekani, M. R., Rejali, F., Teimuri, S., and Miransari, M. (2009). Alleviation of salinity stress on wheat yield, yield components, and nutrient uptake using arbuscular mycorrhizal fungi under field conditions. J. Plant Physiol. 166, 617–625. doi: 10.1016/j.jplph.2008.09.013
Dhindsa, R. S., Plumbdhindsa, P., and Thorpe, T. A. (1981). Leaf senescence : Correlated with increased levels of membrane-permeability and lipid peroxidation, and decreased levels of superoxide dismutase and catalase. J. Exp. Bot. 32, 93–101. doi: 10.1093/jxb/32.1.93
D'Hose, T., Cougnon, M., De Vliegher, A., Vandecasteele, B., Viaene, N., Cornelis, W., et al. (2014). The positive relationship between soil quality and crop production: A case study on the effect of farm compost application. Appl. Soil Ecol. 75, 189–198. doi: 10.1016/j.apsoil.2013.11.013
Drake, J. A., Cavagnaro, T. R., Cunningham, S. C., Jackson, W. R., and Patti, A. F. (2016). Does biochar improve establishment of tree seedlings in saline sodic soils? L. Degrad. Dev. 27, 52–59. doi: 10.1002/ldr.2374
Dubois, M., Gilles, K. A., Hamilton, J. K., Rebers, P. A., and Smith, F. (1956). Colorimetric method for determination of sugars and related substances. Anal. Chem. 28, 350–356. doi: 10.1021/ac60111a017
Duong, T. T. T., Penfold, C., and Marschner, P. (2012). Differential effects of composts on properties of soils with different textures. Biol. Fertil. Soils 48, 699–707. doi: 10.1007/s00374-012-0667-4
El Kinany, S., Achbani, E., Faggroud, M., Ouahmane, L., El Hilali, R., Haggoud, A., et al. (2019). Effect of organic fertilizer and commercial arbuscular mycorrhizal fungi on the growth of micropropagated date palm cv. Feggouss. J. Saudi Soc. Agric. Sci. 18, 411–417. doi: 10.1016/j.jssas.2018.01.004
Enebe, M. C., and Babalola, O. O. (2018). The influence of plant growth-promoting rhizobacteria in plant tolerance to abiotic stress: a survival strategy. Appl. Microbiol. Biotechnol. 102, 7821–7835. doi: 10.1007/s00253-018-9214-z
Estrada, B., Aroca, R., Barea, J. M., and Ruiz-Lozano, J. M. (2013). Native arbuscular mycorrhizal fungi isolated from a saline habitat improved maize antioxidant systems and plant tolerance to salinity. Plant Sci. 201–202, 42–51. doi: 10.1016/j.plantsci.2012.11.009
Evelin, H., Devi, T. S., Gupta, S., and Kapoor, R. (2019). Mitigation of salinity stress in plants by arbuscular mycorrhizal symbiosis: current understanding and new challenges. Front. Plant Sci. 10:470. doi: 10.3389/fpls.2019.00470
Foyer, C. H. (2018). Reactive oxygen species, oxidative signaling and the regulation of photosynthesis. Environ. Exp. Bot. 154, 134–142. doi: 10.1016/j.envexpbot.2018.05.003
Füzy, A., Biró, B., Tóth, T., Hildebrandt, U., and Bothe, H. (2008). Drought, but not salinity, determines the apparent effectiveness of halophytes colonized by arbuscular mycorrhizal fungi. J. Plant Physiol. 165, 1181–1192. doi: 10.1016/j.jplph.2007.08.010
Gill, S. S., and Tuteja, N. (2010). Reactive oxygen species and antioxidant machinery in abiotic stress tolerance in crop plants. Plant Physiol. Biochem. 48, 909–930. doi: 10.1016/j.plaphy.2010.08.016
Giri, B., and Mukerji, K. G. (2004). Mycorrhizal inoculant alleviates salt stress in Sesbania aegyptiaca and Sesbania grandiflora under field conditions: Evidence for reduced sodium and improved magnesium uptake. Mycorrhiza 14, 307–312. doi: 10.1007/s00572-003-0274-1
Graceson, A., Hare, M., Hall, N., and Monaghan, J. (2014). Use of inorganic substrates and composted green waste in growing media for green roofs. Biosyst. Eng. 124, 1–7. doi: 10.1016/j.biosystemseng.2014.05.007
Gryndler, M., Sudová, R., Püschel, D., Rydlová, J., Janoušková, M., and Vosátka, M. (2008). Cultivation of high-biomass crops on coal mine spoil banks: can microbial inoculation compensate for high doses of organic matter? Bioresour. Technol. 99, 6391–6399. doi: 10.1016/j.biortech.2007.11.059
Hajiboland, R. (2013). “Role of arbuscular mycorrhiza in amelioration of salinity,” in Salt Stress in Plants: Signalling, Omics and Adaptations, eds P. Ahmad, M. M. Azooz, and M. N. V. Prasad (New York: Springer Science+Business Media), 301–354. doi: 10.1007/978-1-4614-6108-1_13
Hajiboland, R., Aliasgharzadeh, N., Laiegh, S. F., and Poschenrieder, C. (2010). Colonization with arbuscular mycorrhizal fungi improves salinity tolerance of tomato (Solanum lycopersicum L.) plants. Plant Soil 331, 313–327. doi: 10.1007/s11104-009-0255-z
Hashem, A., Abd Allah, E. F., Alqarawi, A. A., Aldubise, A., and Egamberdieva, D. (2015). Arbuscular mycorrhizal fungi enhances salinity tolerance of Panicum turgidum forssk by altering photosynthetic and antioxidant pathways. J. Plant Interact. 10, 230–242. doi: 10.1080/17429145.2015.1052025
Hashem, A., Abd-Allah, E. F., Alqarawi, A. A., Al-Huqail, A. A., Wirth, S., and Egamberdieva, D. (2016). The interaction between arbuscular mycorrhizal fungi and endophytic bacteria enhances plant growth of Acacia gerrardii under salt stress. Front. Microbiol. 7, 1–15. doi: 10.3389/fmicb.2016.01089
Hidri, R., Barea, J. M., Ben Mahmoud, O. M., Abdelly, C., and Azcon, R. (2016). Impact of microbial inoculation on biomass accumulation by Sulla carnosa provenances, and in regulating nutrition, physiological and antioxidant activities of this species under non-saline and saline conditions. J. Plant Physiol. 201, 28–41. doi: 10.1016/j.jplph.2016.06.013
Hodge, A., Campbell, C. D., and Fitter, A. H. (2001). An arbuscular mycorrhizal fungus accelerates decomposition and acquires nitrogen directly from organic material. Nature 413, 297–299. doi: 10.1038/35095041
Hodge, A., and Storer, K. (2014). Arbuscular mycorrhiza and nitrogen: Implications for individual plants through to ecosystems. Plant Soil 386, 1–19. doi: 10.1007/s11104-014-2162-1
Hoque, M. A., Banu, M. N. A., Nakamura, Y., Shimoishi, Y., and Murata, Y. (2008). Proline and glycinebetaine enhance antioxidant defense and methylglyoxal detoxification systems and reduce NaCl-induced damage in cultured tobacco cells. J. Plant Physiol. 165, 813–824. doi: 10.1016/j.jplph.2007.07.013
Ignatova, Z., and Gierasch, L. M. (2006). Inhibition of protein aggregation in vitro and in vivo by a natural osmoprotectant. Proc. Natl. Acad. Sci. U.S.A. 103, 13357–13361. doi: 10.1073/pnas.0603772103
Kamal-Eldin, A., and Ghnimi, S. (2018). Classification of date fruit (Phoenix dactylifera, L.) based on chemometric analysis with multivariate approach. J. Food Meas. Charact. 12, 1020–1027. doi: 10.1007/s11694-018-9717-4
Kapoor, R., Sharma, D., and Bhatnagar, A. K. (2008). Arbuscular mycorrhizae in micropropagation systems and their potential applications. Sci. Hortic. 116, 227–239. doi: 10.1016/j.scienta.2008.02.002
Kohler, J., Caravaca, F., Azcón, R., Díaz, G., and Roldán, A. (2015). The combination of compost addition and arbuscular mycorrhizal inoculation produced positive and synergistic effects on the phytomanagement of a semiarid mine tailing. Sci Total Environ. 514:42–48. doi: 10.1016/j.scitotenv.2015.01.085
Kranz, C. N., McLaughlin, R. A., Johnson, A., and Miller, G. (2020). The effects of compost incorporation on soil physical properties in urban soils - a concise review J. Environ. Manage. 261, 10. doi: 10.1016/j.jenvman.2020.110209
Lakhdar, A., Hafsi, C., Rabhi, M., Ouerghi, Z., Abdelly, C., Montemurro, F., et al. (2008). Application of municipal solid waste compost reduces the negative effects of saline water in Hordeum maritimum L. Bioresour. Technol. 99, 7160–7167. doi: 10.1016/j.biortech.2007.12.071
Lehmann, J., Rillig, M. C., Thies, J., Masiello, C. A., Hockaday, W. C., and Crowley, D. (2011). Biochar effects on soil biota - a review. Soil Biol. Biochem. 43, 1812–1836. doi: 10.1016/j.soilbio.2011.04.022
Liu, W., Li, R. J., Han, T. T., Cai, W., Fu, Z. W., and Lu, Y. T. (2015). Salt stress reduces root meristem size by nitric oxide-mediated modulation of auxin accumulation and signaling in Arabidopsis. Plant Physiol. 168, 343–356. doi: 10.1104/pp.15.00030
Lovelock, C. E., and Ball, M. C. (2006). “Influence of salinity on photosynthesis of halophytes,” in Salinity: Environment - Plants - Molecules, eds A. Läuchli and U. Lüttge (Netherlands: Kluwer Academic Publishers), 315–339. doi: 10.1007/0-306-48155-3_15
Mbarki, S., Cerdà, A., Zivcak, M., Brestic, M., Rabhi, M., Mezni, M., et al. (2018). Alfalfa crops amended with MSW compost can compensate the effect of salty water irrigation depending on the soil texture. Process Saf. Environ. Prot. 115, 8–16. doi: 10.1016/j.psep.2017.09.001
McGonigle, T. P., Miller, M. H., Evans, D. G., Fairchild, G. L., and Swan, J. A. (1990). A new method which gives an objective measure of colonization of roots by vesicular- arbuscular mycorrhizal fungi. New Phytol. 115, 495–501. doi: 10.1111/j.1469-8137.1990.tb00476.x
Meddich, A., Ait El Mokhtar, M., Bourzik, W., Mitsui, T., Baslam, M., and Hafidi, M. (2018). “Optimizing growth and tolerance of date palm (Phoenix dactylifera L.) to drought, salinity, and vascular fusarium-induced wilt (Fusarium oxysporum) by application of arbuscular mycorrhizal fungi (AMF),” in Root Biology, eds B. Giri, R. Parsad, and A. Varma (Cham: Springer), 239–250. doi: 10.1007/978-3-319-75910-4_9
Meddich, A., Elouaqoudi, F. Z., Khadra, A., and Bourzik, W. (2016). Valorization of green and industrial wastes by composting process. Rev. des Compos. des Mater. Av. 26, 451–469. doi: 10.3166/rcma.26.451-469
Meddich, A., Jaiti, F., Bourzik, W., Asli, A., and El Hafidi, M. (2015). Use of mycorrhizal fungi as a strategy for improving the drought tolerance in date palm (Phoenix dactylifera). Sci. Hortic. 192, 468–474. doi: 10.1016/j.scienta.2015.06.024
Medina, A., and Azcón, R. (2010). Effectiveness of the application of arbuscular mycorrhiza fungi and organic amendments to improve soil quality and plant performance under stress conditions. J. Soil Sci. Plant Nutr. 10, 354–372. doi: 10.4067/S0718-95162010000100009
Meena, M. D., Joshi, P. K., Jat, H. S., Chinchmalatpure, A. R., Narjary, B., Sheoran, P., et al. (2016). Changes in biological and chemical properties of saline soil amended with municipal solid waste compost and chemical fertilizers in a mustard-pearl millet cropping system. Catena 140, 1–8. doi: 10.1016/j.catena.2016.01.009
Mimmo, T., Hann, S., Jaitz, L., Cesco, S., Gessa, C. E., and Puschenreiter, M. (2011). Time and substrate dependent exudation of carboxylates by Lupinus albus L. and Brassica napus L. Plant Phys. Biochem. 49, 1272–1278. doi: 10.1016/j.plaphy.2011.08.012
Miransari, M. (2010). Contribution of arbuscular mycorrhizal symbiosis to plant growth under different types of soil stress. Plant Biol. 12, 563–569. doi: 10.1111/j.1438-8677.2009.00308.x
Mittler, R. (2002). Oxidative stress, antioxidants and stress tolerance. Trends Plant Sci. 7, 405–410. doi: 10.1016/S1360-1385(02)02312-9
Munns, R. (2005). Genes and salt tolerance. New Phytol. 167, 645–663. doi: 10.1111/j.1469-8137.2005.01487.x
Munns, R., Passioura, J. B., Colmer, T. D., and Byrt, C. S. (2020). Osmotic adjustment and energy limitations to plant growth in saline soil. New Phytol. 225, 1091–1096. doi: 10.1111/nph.15862
Murkute, A. A., Sharma, S., and Singh, S. K. (2006). Studies on salt stress tolerance of citrus rootstock genotypes with arbuscular mycorrhizal fungi. Hortic. Sci. 33, 70–76. doi: 10.17221/3742-HORTSCI
Ngo, H. T. T., and Cavagnaro, T. R. (2018). Interactive effects of compost and pre-planting soil moisture on plant biomass, nutrition and formation of mycorrhizas: a context dependent response. Sci. Rep. 8, 1–9. doi: 10.1038/s41598-017-18780-2
Olsen, S. R., and Dean, L. A. (1965). “Phosphorus,” in Methods of Soil Chemical Analysis, Part 2, eds C. A. Black, D. D. Evans, J. L. White, L. E. Ensminger, F. E. Clark, and R. C. Dinauer (Madison: American Society of Agronomy), 1035–1049. doi: 10.2134/agronmonogr9.2.c22
Ou Zin, M., Bouhlal, Y., El Hilali, R., Achbani, E. H., Haggoud, A., and Rachid, B. (2020). Evaluation of compost quality and bioprotection potential against Fusarium wilt of date palm. Waste Manag. 113, 12–19. doi: 10.1016/j.wasman.2020.05.035
Phillips, J. M., and Hayman, D. S. (1970). Improved procedures for clearing roots and staining parasitic and vesicular-arbuscular mycorrhizal fungi for rapid assessment of infection. Trans. Br. Mycol. Soc. 55, 158-IN18. doi: 10.1016/S0007-1536(70)80110-3
Polle, A., Otter, T., and Seifert, F. (1994). Apoplastic peroxidases and lignification in needles of norway spruce (Picea abies L.). Plant Physiol. 106, 53–60. doi: 10.1104/pp.106.1.53
Porcel, R., Aroca, R., and Ruiz-Lozano, J. M. (2012). Salinity stress alleviation using arbuscular mycorrhizal fungi. A review. Agron. Sustain. Dev. 32, 181–200. doi: 10.1007/s13593-011-0029-x
Potters, G., Pasternak, T. P., Guisez, Y., Palme, K. J., and Jansen, M. A. (2007). Stress-induced morphogenic responses: growing out of trouble? Trends Plant Sci. 12, 98–105. doi: 10.1016/j.tplants.2007.01.004
Raklami, A., Bechtaoui, N., Tahiri, A. I., Anli, M., Meddich, A., and Oufdou, K. (2019). Use of rhizobacteria and mycorrhizae consortium in the open field as a strategy for improving crop nutrition, productivity and soil fertility. Front. Microbiol. 10:1106. doi: 10.3389/fmicb.2019.01106
Ramoliya, P. J., Patel, H. M., and Pandey, A. N. (2004). Effect of salinization of soil on growth and macro- and micro-nutrient accumulation in seedlings of Salvadora persica (Salvadoraceae). For. Ecol. Manage. 202, 181–193. doi: 10.1016/j.foreco.2004.07.020
Ramzani, P. M. A., Shan, L., Anjum, S., Khan, W. U.-D., Ronggui, H., Iqbal, M., et al. (2017). Improved quinoa growth, physiological response, and seed nutritional quality in three soils having different stresses by the application of acidified biochar and compost. Plant Physiol. Biochem. 116, 127–138. doi: 10.1016/j.plaphy.2017.05.003
Rana, M. M., Takamatsu, T., Baslam, M., Kaneko, K., Itoh, K., Harada, N., et al. (2019). Salt tolerance improvement in rice through efficient SNP and marker-assisted selection coupled with speed-breeding. Int. J. Mol. Sci. 20:2585. doi: 10.3390/ijms20102585
Rao, D. E., and Chaitanya, K. V. (2016). Photosynthesis and antioxidative defense mechanisms in deciphering drought stress tolerance of crop plants. Biol. Plant. 60, 201–218. doi: 10.1007/s10535-016-0584-8
Rasool, S., Ahmad, A., Siddiqi, T. O., and Ahmad, P. (2013). Changes in growth, lipid peroxidation and some key antioxidant enzymes in chickpea genotypes under salt stress. Acta Physiol. Plant 35, 1039–1050. doi: 10.1007/s11738-012-1142-4
Rillig, M. C., Aguilar-Trigueros, C. A., Camenzind, T., Cavagnaro, T. R., Degrune, F., Hohmann, P., et al. (2019). Why farmers should manage the arbuscular mycorrhizal symbiosis. New Phytol. 222, 1171–1175. doi: 10.1111/nph.15602
Rillig, M. C., and Mummey, D. L. (2006). Mycorrhizas and soil structure. New Phytol. 171, 41–53. doi: 10.1111/j.1469-8137.2006.01750.x
Ryan, M. H., and Graham, J. H. (2018). Little evidence that farmers should consider abundance or diversity of arbuscular mycorrhizal fungi when managing crops. New Phytol. 220, 1092–1107. doi: 10.1111/nph.15308
Sallaku, G., Sandén, H., Babaj, I., Kaciu, S., Balliu, A., and Rewald, B. (2019). Specific nutrient absorption rates of transplanted cucumber seedlings are highly related to RGR and influenced by grafting method, AMF inoculation and salinity. Sci. Hortic. 243, 177–188. doi: 10.1016/j.scienta.2018.08.027
Scheffknecht, S., Mammerler, R., Steinkellner, S., and Vierheilig, H. (2006). Root exudates of mycorrhizal tomato plants exhibit a different effect on microconidia germination of Fusarium oxysporum f.sp. lycopersici than root exudates from non-mycorrhizal tomato plants. Mycorrhiza 16, 365–370. doi: 10.1007/s00572-006-0048-7
Scholander, P. F., Hammel, H. T., Bradstreet, E. D., and Hemmingsen, E. A. (1965). Sap pressure in vascular plants. Science. 148, 339–346. doi: 10.1126/science.148.3668.339
Setia, R., Gottschalk, P., Smith, P., Marschner, P., Baldock, J., Setia, D., et al. (2013). Soil salinity decreases global soil organic carbon stocks. Sci. Total Environ. 465, 267–272. doi: 10.1016/j.scitotenv.2012.08.028
Sharma, P., Jha, A. B., Dubey, R. S., and Pessarakli, M. (2012). Reactive oxygen species, oxidative damage, and antioxidative defense mechanism in plants under stressful conditions. J. Bot. 2012, 1–26. doi: 10.1155/2012/217037
Shireen, F., Nawaz, M. A., Chen, C., Zhang, Q., Zheng, Z., Sohail, H., et al. (2018). Boron: functions and approaches to enhance its availability in plants for sustainable agriculture. Int. J. Mol. Sci. 19, 1856. doi: 10.3390/ijms19071856
Sieverding, E. (1991). Vesicular-Arbuscular Mycorrhiza Management in Tropical Agrosystems. Eschbor: Sonderpublikation der GTZ.
Sperling, O., Lazarovitch, N., Schwartz, A., and Shapira, O. (2014). Effects of high salinity irrigation on growth, gas-exchange, and photoprotection in date palms (Phoenix dactylifera L., cv. Medjool). Environ. Exp. Bot. 99, 100–109. doi: 10.1016/j.envexpbot.2013.10.014
Takano, J., Noguchi, K., Yasumori, M., Kobayashi, M., Gajdos, Z., Miwa, K., et al. (2002). Arabidopsis boron transporter for xylem loading. Nature 420, 337–340. doi: 10.1038/nature01139
Talaat, N. B., and Shawky, B. T. (2014). Protective effects of arbuscular mycorrhizal fungi on wheat (Triticum aestivum L .) plants exposed to salinity. Environ. Exp. Bot. 98, 20–31. doi: 10.1016/j.envexpbot.2013.10.005
Tartoura, K. A. H., Youssef, S. A., and Tartoura, E. S. A. A. (2014). Compost alleviates the negative effects of salinity via up-regulation of antioxidants in Solanum lycopersicum L. plants. Plant Growth Raegulation 74, 299–310. doi: 10.1007/s10725-014-9923-y
Trenberth, K. E., Dai, A., Van Der Schrier, G., Jones, P. D., Barichivich, J., Briffa, K. R., et al. (2014). Global warming and changes in drought. Nat. Clim. Chang. 4, 17–22. doi: 10.1038/nclimate2067
Trivedi, P., Singh, K., Pankaj, U., Verma, S. K., Verma, R. K., and Patra, D. D. (2017). Effect of organic amendments and microbial application on sodic soil properties and growth of an aromatic crop. Ecol. Eng. 102, 127–136. doi: 10.1016/j.ecoleng.2017.01.046
Vayalil, P. K. (2012). Date fruits (Phoenix dactylifera Linn): an emerging medicinal food. Crit. Rev. Food Sci. Nutr. 52, 249–271. doi: 10.1080/10408398.2010.499824
Velikova, V., Yordanov, I., and Edreva, A. (2000). Oxidative stress and some antioxidant systems in acid rain-treated bean plants. Plant Sci. 151, 59–66. doi: 10.1016/S0168-9452(99)00197-1
Watts-Williams, S. J., and Cavagnaro, T. R. (2014). Nutrient interactions and arbuscular mycorrhizas: a meta-analysis of a mycorrhiza-defective mutant and wild-type tomato genotype pair. Plant Soil 384, 79–92. doi: 10.1007/s11104-014-2140-7
Weber, J., Kocowicz, A., Bekier, J., Jamroz, E., Tyszka, R., Debicka, M., et al. (2014). The effect of a sandy soil amendment with municipal solid waste (MSW) compost on nitrogen uptake efficiency by plants. Eur. J. Agron. 54, 54–60. doi: 10.1016/j.eja.2013.11.014
Wolf, B. (1982). A comprehensive system of leaf analyses and its use for diagnosing crop nutrient status. Commun. Soil Sci. Plant Anal. 13, 1035–1059. doi: 10.1080/00103628209367332
Yaish, M. W., and Kumar, P. P. (2015). Salt tolerance research in date palm tree (Phoenix dactylifera L.), past, present, and future perspectives. Front. Plant Sci. 6, 1–5. doi: 10.3389/fpls.2015.00348
Zhang, S., Lehmann, A., Zheng, W., You, Z., and Rillig, M. C. (2019). Arbuscular mycorrhizal fungi increase grain yields: a meta-analysis. New Phytol. 222, 543–555. doi: 10.1111/nph.15570
Zhang, Y. C., Wang, P., Wu, Q. H., Zou, Y. N., Bao, Q., and Wu, Q. S. (2017). Arbuscular mycorrhizas improve plant growth and soil structure in trifoliate orange under salt stress. Arch. Agron. Soil Sci. 63, 491–500. doi: 10.1080/03650340.2016.1222609
Zhu, J. K. (2003). Regulation of ion homeostasis under salt stress. Curr. Opin. Plant Biol. 6, 441–445. doi: 10.1016/S1369-5266(03)00085-2
Keywords: biofertilizers, mycorrhiza, nutrient uptake, oxidative stress, salinity, sustainable agriculture
Citation: Ait-El-Mokhtar M, Baslam M, Ben-Laouane R, Anli M, Boutasknit A, Mitsui T, Wahbi S and Meddich A (2020) Alleviation of Detrimental Effects of Salt Stress on Date Palm (Phoenix dactylifera L.) by the Application of Arbuscular Mycorrhizal Fungi and/or Compost. Front. Sustain. Food Syst. 4:131. doi: 10.3389/fsufs.2020.00131
Received: 04 June 2020; Accepted: 24 July 2020;
Published: 04 September 2020.
Edited by:
Everlon Cid Rigobelo, São Paulo State University, BrazilReviewed by:
Arafat Abdel Hamed Abdel Latef, South Valley University, EgyptCopyright © 2020 Ait-El-Mokhtar, Baslam, Ben-Laouane, Anli, Boutasknit, Mitsui, Wahbi and Meddich. This is an open-access article distributed under the terms of the Creative Commons Attribution License (CC BY). The use, distribution or reproduction in other forums is permitted, provided the original author(s) and the copyright owner(s) are credited and that the original publication in this journal is cited, in accordance with accepted academic practice. No use, distribution or reproduction is permitted which does not comply with these terms.
*Correspondence: Marouane Baslam, bWJhc2xhbUBncy5uaWlnYXRhLXUuYWMuanA=; Abdelilah Meddich, YS5tZWRkaWNoQHVjYS5tYQ==
Disclaimer: All claims expressed in this article are solely those of the authors and do not necessarily represent those of their affiliated organizations, or those of the publisher, the editors and the reviewers. Any product that may be evaluated in this article or claim that may be made by its manufacturer is not guaranteed or endorsed by the publisher.
Research integrity at Frontiers
Learn more about the work of our research integrity team to safeguard the quality of each article we publish.