- Department of Plant Science, McGill University, Montreal, QC, Canada
Plant growth often occurs under a range of stressful conditions, including soil acidity and alkalinity. Hydrogen ion concentration, which determines pH of the soil, regulates the entire chemistry of plant nutrient colloidal solutions. Beyond certain levels of pH, multiple stresses such as hydrogen ion toxicity, and nutrient imbalance, toxicities and deficiencies are induced in plants. Breeding for stress coupled with suitable agronomic practices has been a way to deal with this situation in agriculture. However, plant growth promoting microbes (PGPM) have shown potential as sustainable plant growth enhancers and have potential to help with a range of stresses in their environment. Considering the long-term evolutionary relationships between plants and microbes, it is probably that much remains unknown about potential benefits of microbes that could be harnessed from PGPM. This article reviews the current understanding of acidity and alkalinity stress effects on plants and various approaches have or could address these stresses. This review provides a detailed account of the current understanding regarding the role of PGPM in acidity and alkalinity stress management, including when agronomic practices and plant breeding are combined. Approaches already evaluated have shown limitations because acidity and alkalinity in soils are gradual and progressive conditions. Greater exploitation of PGPM in this regard, would be interesting to explore as they have the potential to address multiple stresses in a more sustainable fashion. Future crop production will require further breeding for pH stress resistance, but also implementation of microbial technologies that provide enhanced tolerance to pH stress.
Introduction
Abundant microscopic life resides in the soil including bacteria, algae, protozoa and fungi (Glick, 1995; Müller et al., 2016), together with below-ground plant parts. The vigor of the microbes in the soil depends on nutrient availability, temperature, water and pH, among others. Plants, partition significant amounts of photosynthetically synthesized carbon to their root systems (Zhalnina et al., 2018) for the important roles of root growth and maintenance. Additionally, this C partitioning, to a large extent, is released from the roots into the rhizosphere in the form of exudates and sloughed off cells, together called rhizodeposition (Paterson et al., 1997; Badri and Vivanco, 2009). Rhizodeposition serves as the main reduced C source for the microscopic life inhabiting in the soil and sustains diverse groups of microbes and microbial feeding life forms (Nguyen, 2009). The root exudates are organic compounds which include: organic acids, sugars, fatty acids and amino acids (Huang et al., 2014); all these have nutritional, preferential microbe selection and soil colloidal effects. Exudates therefore, control interactions between plants and microbes in part because they contain signal molecules that facilitate the interactions (Bulgarelli et al., 2013). Many studies have shown that plants expend this C to attract beneficial microbes such as rhizobia, involved in biological nitrogen fixation in legumes (Msimbira et al., 2016; Chagas et al., 2018) and mycorrhizal associations (Van Der Heijden et al., 2015). The legume-rhizobia symbiosis is a plant growth promoting mechanism acting through nitrogen fixation that is well described as compared to other plant-microbe associations (Oldroyd, 2013); it is reported to contribute to about 50% of the biologically fixed nitrogen on earth (Lindström et al., 2010). The second-most largely studied mechanism is that of a set of plant-fungal interactions, which involves about 90% of all plant species on planet Earth, by way of mycorrhizal symbioses (Gough and Cullimore, 2011; Zifcakova, 2020). Such interactions benefit plants by improving nutrient acquisition, water uptake and ability to survive various stresses. The usefulness of plant-microbe interactions has been the focus of intensive research, focused on unlocking key unanswered question, since their first description (Kloepper et al., 1989). Since then, beneficial interactions and better understanding of the mechanism(s) involved in microbial enhancement of plant growth have been demonstrated (Lira, 2015; Smith et al., 2015).
Beneficial symbiotic associations between early plants and mycorrhizal fungi are thought to have evolved to overcome limitations of terrestrial ecosystems, such as restricted water and nutrient availability (Kenrick and Crane, 1997; Kenrick and Strullu-Derrien, 2014). In soils, nutrient availability is related to hydrogen ion concentration (H+), which is the measure of soil pH. The pH variation in the environment has a direct impact on the availability of nutrients and plant growth; the critical and important effects of these conditions on microbial communities are not well understood. In soils, pH is an important driver for soil microbial community structures. Microbial survival and colonization in such conditions requires the capacity to sense, and adapt to, environmental changes (Biswas et al., 2007).
Recent years have witnessed considerable interest in unraveling the role and potential of microbes in the success of plants and animals. Of all, the human microbiome is the most studied, as reviewed by Gilbert et al. (2018); more recently, much attention has been focused on plants and their associated phytomicrobiome (Compant et al., 2019); terrestrial plants being the entry point of most energy into the terrestrial biosphere. Much has been done, mostly on symbiotic microbes and particularly under optimum conditions for plant growth. This review investigates the current understanding of one of the common, but complex and less explored abiotic factors, pH, as a determinant factor of the distribution and survival of microbes and plants. This review also wishes to understand how much is known regarding aspects of acidity and alkalinity stress alleviation related to the evolutionary understanding that microbes have co-evolved with plants, each benefiting the other. Microbes, having a large surface area to volume, are very exposed to environmental stressors, so that their mode(s) of adaptation is of great importance for survival and, potentially of high impact, if these could be translated to multicellular organisms. While optimum pH is a crucial factor for survival in an evolutionary context, challenging conditions improve fitness over the course of evolution. Other factors of great importance include temperature and nutrients, which will also be touched upon as related to pH.
Plant Microbe Interaction
Plants do not exist alone; always have complex interactions with microbes. Plants co-live with microorganisms (fungi, bacteria and archaea), allowing them to inhabit almost all of their tissues; and the resulting assemblage of microbes is collectively known as the plant-microbiome or phytomicrobiome (Knack et al., 2015; Smith et al., 2017). This perspective has helped, in recent years, to start answering some common evolutionary questions regarding how microbes have evolved, together with their host organisms, from their original ancestors. It is of critical importance to understand how plant adaptation has been influenced by their interactions with microbes, though much remains unknown. Plant-microbe interactions are a lifelong process for plants, as some microbes may be leaving the plant-associated community, while others will be entering the community (Baltrus, 2017). The ability of plants and microbes to communicate prior to physical contact being established is a very important (Chagas et al., 2018) as it helps the partners maximize the chance of benefiting from one another, without harm. There are a number of phytomicrobiome groupings, for instance depending on the plant part colonized by microbes: rhizomicrobiome – roots, caulomicrobiome – stem, phyllomicrobiome – leaves, anthromicrobiome – flowers, carpo microbiome – fruit, or degree of intimacy with the plant tissue which are termed as endophyte (interaction inside plant parts), epiphyte (on the surface of plant structures such as shoots, stems, leaves, flowers and fruits) (Laksmanan et al., 2014; Chagas et al., 2018). The rhizosphere phytomicrobiome richness, activities and diversity is far greater than the phyllosphere (Laksmanan et al., 2014). This is primarily because much of the root exudation and sloughed off cells contain nutrient rich compounds for microbes associated with roots (Meharg and Killham, 1990; Beneduzi et al., 2012; Daguerre et al., 2017). Even with many questions unanswered about their full potential role in each of their tissues of colonization, plant-associated microbes provide promising insights around some best ways to augment plant growth and productivity, and understanding continues to expand.
For agriculture, microbes hold great promise in promoting productivity through synergistic interactions with host plants. All agricultural production taking place under field conditions face a range of challenges. This means increasing production with a constant, or even decreasing, land resource, and the need for a breakthrough to find possible sustainable means for food production under field conditions. Plant growth promoting elements in the rhizomicrobiome provide promising potential for sustainable crop production and there has been increased interest in optimizing their use under a range of stresses, such as salinity and drought (Booth et al., 2002; Subramanian et al., 2016). As the world faces changing, and generally harsher, crop-growth conditions related to ongoing climate change, preparedness requires multiple options for sustainability. Apart from drought and salinity two other key growth stressors of the phytomicrobiome are increasing acidity and alkalinity of the soils.
Many recent studies have identified beneficial microbes that help plants, including crop plants, to survive the stresses they encounter, including nutrient imbalance (Mylona et al., 1995; Yazdani et al., 2009), salinity (Subramanian et al., 2016), and drought (Lim and Kim, 2013), with much less being reported regarding soil acidity and alkalinity. Thus, there is potential to understand better and move forward with a more sustainable agriculture based on knowledge at hand on each physical stress, and the role microbes can play in agricultural management of these stresses. A key factor in microbial proliferation in the wild is pH. The acidity and alkalinity of soils has been linked directly to soil and plant associated microbial population dynamics (Biswas et al., 2007; Zhalnina et al., 2015). Despite its obvious importance much seems unclear as to why microbes behave the way they do at varying pH levels.
Soil: a Center for Plant Microbe Interaction
Soil is a reservoir of basic natural resources, such as nutrients, for animals, plants and microbes. It is a life support system that provides a wide range of necessary ecosystem goods and services ranging from storage of carbon, to water purification, soil fertility and agricultural production (Rojas and Caon, 2016). Variation in soil characteristics throughout the world is affected by weather/climate and how it is geopositioned on the globe. Apart from nutrients, soil also contains plant-available water which plays a key role in creating an aqueous nutrient solution, the form taken up by plants (Sassenrath et al., 2018). The fact that all living biological cells are water-based systems makes a cell’s survival very dependent on aqueous equilibria. For any aqueous solution reaction to occur, presence of anions and cations is needed. The necessity of appropriate pH in a biological system is crucial as it helps maintaining biochemical equilibria, correct levels of proton dissociable groups and maintain the cell pH at near neutral all the time. Like any other living cells, microbes need an appropriate pH balance to maintain physiological functions.
Soil pH
The measure of soil reaction (alkalinity or acidity) is expressed as pH. It is mostly measured in water solutions and to lesser extent, for research purposes, 0.01 M calcium chloride is used (Blake et al., 1999). Soil pH is a key condition with substantial influence on soil biology, chemistry and physical processes which have direct impacts on plant growth and development. It is clear soil and crop productivity are linked to pH. The United States Department of Agricultural National Resources Conservation Service has categorized soil pH as follows: ultra-acidic (<3.5), extremely acidic (3.5–4.4), very strongly acid (4.5–5.0), strongly acidic (5.1–5.5), moderately acidic (5.6–6.0), slightly acidic (6.1–6.5), neutral (6.6–7.3), slightly alkaline (7.4–7.8), moderately alkaline (7.9–8.4), strongly alkaline (8.5–9.0) and very strongly alkaline (>9.0) (Burt, 2014).
Agricultural crop production is generally conducted within the range of slightly acidic to slightly alkaline, a window that is associated with optimal availability of soil nutrients. In all soils, solubility, mobility and bioavailability of trace elements is strongly affected by pH. However, soils which fall outside of the range of optimum nutrient availability are grouped as either acidic or alkaline and pose a range of challenges to plants. Though plants differ in their tolerance to extreme pH, most agricultural plants perform optimally at a pH near neutrality (Läuchli and Grattan, 2012). In the context of crop production, pH variation is associated with all the ways the soil is managed before, during and after crop production, which includes; soil tillage, planting of cover crops, fertilizer application and lime addition, as well as precipitation and other climate variables.
A full understanding of pH is necessary for optimizing nutrient cycling, soil remediation and plant nutrition, as it affects the entire interacting system. In order to establish ways to deal with various aspects that are affected by soil pH, one should initially understand what causes variation in the soil pH. One of the major causes of pH variation is the inherent mineral composition of the parent soil material. In this review, acidification and alkalization of soil are discussed to enlighten our understanding of causes of pH changes in the soil below and above neutrality.
Soil Acidification
Soil acidification is the result of various direct and indirect factors interacting with the soil; these include nutrient cycling and organic matter decomposition, high and acidic rainfall, fertilizer application, crop growth and weathering (Figure 1). Acidification is a gradual and progressive process which is influenced by agricultural practices and now by climate change (Bolan et al., 1991; Filipek, 1994; Hao et al., 2019). It is the result of increased H+ concentration with the H+ released from Carbon (C), Nitrogen (N) and Sulfur (S) during transformation and cycling. For example S and N oxides released from burning of fossil fuels react with rain water to form tetraoxosulfate (vi) acid and trioxonitrate (v) acid (Oshunsanya, 2018). Mineralization and oxidation of organic N and S release H+, thus lowering the soil pH. Organic matter decomposition causes release of CO2 into the soil air which, when dissolved in soil water, forms H2CO3 which causes a decline in pH (Bolan et al., 1991).
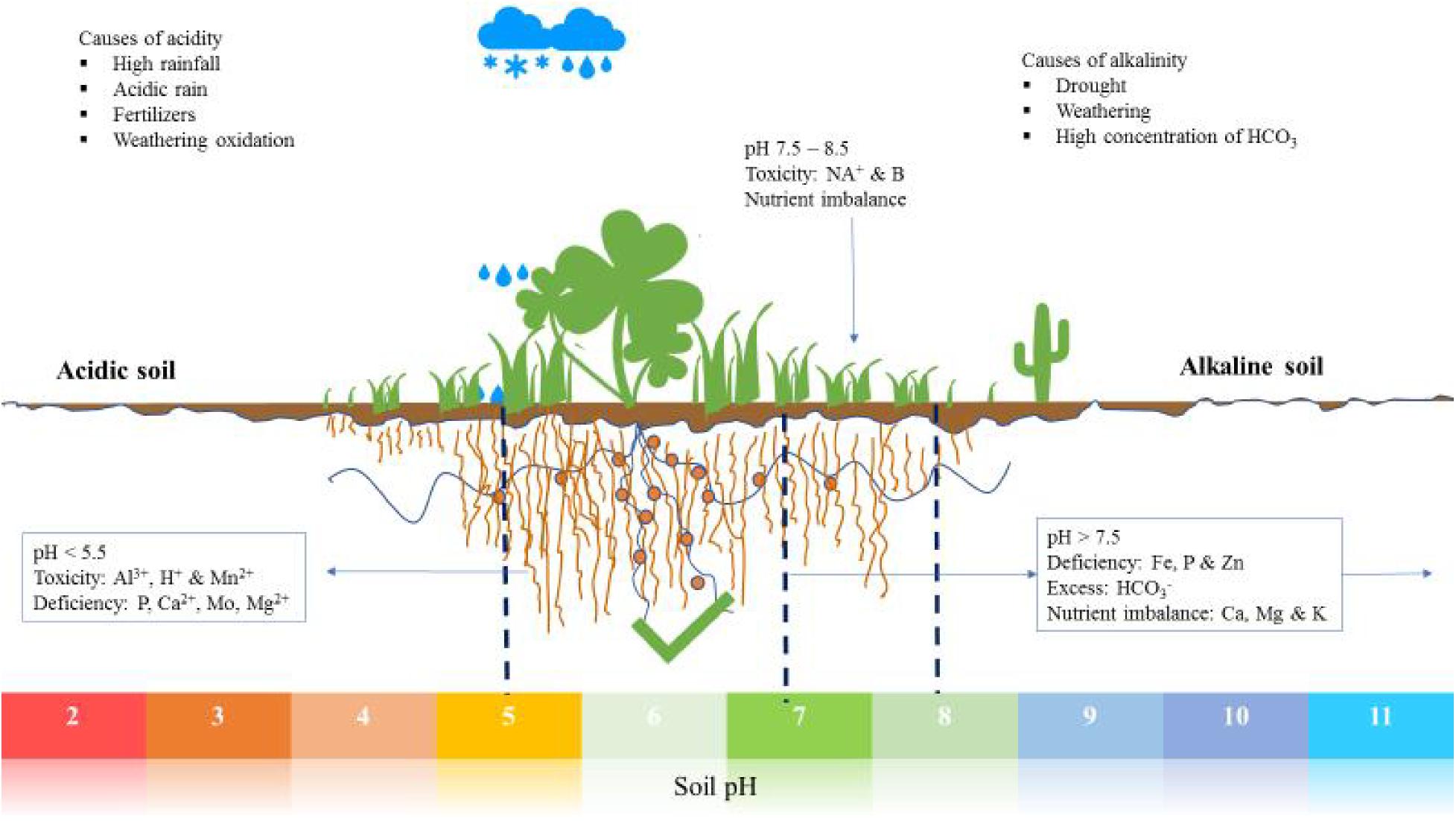
Figure 1. An illustration of various soils with respect to pH, nutrient availability, deficiencies, and imbalances.
High rainfall is also a cause of soil acidification because rainwater is slightly acidic (around pH 5.7), a result of reaction with atmospheric CO2 forming carbonic acid, hence reducing the pH of soil. In addition, water in the soil causes leaching of basic cations, such as bicarbonate, leaving more Al3+ and H+ relative to other cations in the soil (Oshunsanya, 2018).
In agricultural soils a major contributor to acidity is the application of ammonium-based fertilizers, urea, sulfur and legume cultivation. The salts from applied fertilizers have strong effects on acidification of the soil through nitrification. This happens only when NH4+ undergoes nitrification and/or NO3– is leached but not when the nitrate is taken up by plants (Marschner, 2011). The scope of the problem is becoming worrisome as the occurrence of acid rain and continued intensive use of synthetic fertilizers. In addition, N2 fixation also has impact on soil acidification. Comparatively, legumes are known to cause more soil acidification than non-legumes, due to excessive uptake of cations relative to anions during N2 fixation, and also leaching of nitrates eventually resulting from fixed N (Tang, 1998; Tang et al., 1999). However, variation in N2 fixation among legumes exists, which results in variation of the acid generated with a range of 0.2 to 1 mol H+ for each mol of fixed N (Bolan et al., 1991). Other factors which influence acidification by legumes are soil nutrients and nitrogen (Yan et al., 1996; Marschner, 2011).
Crop growth is another factor which causes localized soil acidification as a result of nutrient uptake. Plants take up nutrients from the soil solution in ionic form with a preference for cations over anions, which leads to cation reduction in the soil (Tang and Rengel, 2003). To counteract the effect of charge imbalance, plants release H+ from roots to the rhizosphere, hence lowering soil pH. In addition, roots naturally exude organic acids which cause acidification of the soil.
Soil Alkalization
Soil alkalinity can be a result of natural weathering processes or man-made conditions. Weathering of silicates, aluminosilicates and carbonate containing compounds such as Na+, Mg2+, K+, and Ca2+ is linked to silicates being hydrolyzed and subsequent OH– release, which increases soil pH. Irrigation is also associated with alkalinity of the soil, especially when the used water contain large quantities of bicarbonates (Oshunsanya, 2018). Drought is another natural cause of soil alkalinity due to insufficient water to leach soluble salts, allowing their accumulation in the upper soil profile. Alkaline soils are characterized by high concentrations of carbonates (CO32–) and bicarbonates (HCO3–) which have the ability to neutralize acids (Bailey, 1996). As a result, alkaline soils are associated with desertification in most parts of the world, and this is also closely associated with soil salinity. Recently, the demand for aluminum in the world has contributed to increased alkalinity in surrounding ecosystems because mining and disposing of the alkaline bauxite residue (Kong et al., 2017). Lastly, over liming also leads to alkalization of soil. Therefore, liming should carefully consider the knowledge of soil acidity so that required liming material can be calculated before it can result in soil alkalization.
Microbial Communities in Relation to Soil Ph
Environmental factors are the main drivers of the phytomicrobiome composition (Chu et al., 2016; Baltrus, 2017) with soil pH exerting a large effect in microbial community structure (Zhalnina et al., 2015). According to Graham et al. (1994), prokaryotic lifeforms are profoundly influenced by the pH of their environment. For all living cells there are optimum pH requirements for normal physiological functions. The pH range 5.5–6.5 is optimal for plant growth as the availability of nutrients is optimal. This is also so for most soil microbes, in part because in this range plants grow well and produce more root exudates as a carbon source available for survival and multiplication of microbes. Though, some microbes have the ability to alter soil pH by acidifying their surroundings, as a way to outcompete other microbes, most bacteria do best around neutral pH. Fungal activities on the other hand are favored by at least somewhat acidic pH conditions, which explains why they are dominant in forest acidic soils compared to range land soils and sub-humid and arid prairies which are mildly acidic and are dominated by bacteria (Zifcakova, 2020).
Bacteria are among the single celled organisms most able to adapt to and thrive under harsh environmental pH conditions. Acidic soils are dominated by Acidobacteria and Alphaproteobacteria (Shen et al., 2019) while Actinobacteria abundance increases toward alkalinity (Jeanbille et al., 2016). However, the most sensitive component of the cell to pH changes is its workhorse, the protein (Hyyryläinen et al., 2001). Slight changes in pH have been reported to interfere with amino acid functional group ionization and impair hydrogen bonding, as a result protein folding is changed, leading to denaturation and cessation of enzymatic or other activities (Booth et al., 2002).
Variation of pH in the environment has a direct impact on the availability of Al, Fe, Mn, Cu, and plant growth; the critical and important effects of these conditions on microbial communities are not well understood. Graham et al. (1994), reported that there were two pH related mechanisms influencing microbial communities, the direct and indirect, the latter being the spillover effects of pH.
Acidity Tolerance in PGPM
In general, organisms have developed mechanisms to survive environmental variation. Among other abiotic factors, most organisms need to sense and adapt to hydrogen ion concentration (pH) (Booth et al., 2002). In soils, pH is an important driver for soil microbial community structures. Microbial survival under such conditions requires the capacity to sense, and adapt to, environmental changes (Biswas et al., 2007).
However, little is known about optimal pH ranges and nutrient availabilities for many species of microorganisms (Ratzke and Gore, 2018). Plant–microbe interactions such as that of legumes and rhizobia are affected by Ca, P, Fe, and Mo; they influence rhizobia and their optimal growth, which is near pH 6. Biochemical properties and activities of microbes are partly affected by pH, leading to diversity effects in microbial community structure (Roe et al., 1998).
Microbes have developed various means to tolerate extreme pH changes. Production of extracellular polysaccharides by rhizobia is one of the reported behaviors (Gopalakrishnan et al., 2015). Rhizobium tropici demonstrated an ability to tolerate acidic pH by producing glutathione, a tripeptide (Muglia et al., 2007; Wang et al., 2018). Some rhizobia are known for their ability to accumulate high levels of potassium and phosphorus as a means to tolerate low pH, as compared to acid sensitive strains (Watkin et al., 2003). However, the relationship between these microbial survival mechanisms and plant growth promotion is not well understood.
Cells’ major functions, such as nutrient acquisition, cytoplasmic pH homeostasis and protection of DNA and proteins are largely affected by low pH (Booth et al., 2002). Mechanisms involved in the induction of protective systems pose a considerable challenge. The advent of proteomics (Blankenhorn et al., 1999) has complemented the genome information in this area, for example, Lactobacillus spp., like many microorganisms, produces a thin biofilm composed of polysaccharides and proteins, which protects the cell against changes in the pH of the environment (Wang et al., 2018).
Alkalinity Tolerance in PGPM
High pH disrupts the bonds holding together the DNA helix strands, and lipid hydrolysis occurs more readily as the environment becomes more basic (Rousk et al., 2010; Shen et al., 2019). Most microbes adjust their surrounding medium to near neutrality as a way to survive high pH. Sodium is very important in intracellular pH maintenance for microbes because it allows exchange of H+/Na+ antiporters into the culture media (Satyanarayana et al., 2005). Furthermore, the H+ concentration gradients across the membranes plays an important role in producing ATP during cellular respiration, through proton motive force (Celiker and Gore, 2013). It is not very clear how PGPM are able to use sodium-dependent ATP synthases as an alkali tolerance mechanism.
Acidity and Alkalinity Stress in Plants
Acidity Stress
Acidification of the soil is currently a major limit for sustainable agricultural production in the world. Acid soil covers about 30–40% of the arable land worldwide, and about 70% of the world’s potential agricultural land (Von Uexküll and Mutert, 1995; Kochian et al., 2004). In the soil plant roots are in constantly adjusting to varying pH as a result of water status variability (Misra and Tyler, 1999). Soil pH has significant influence on plants because it affects almost every aspect of nutrient uptake by them. In acid soil plants face three major toxicities, Al3+, Mn2+ and H+, which inhibit plant growth. In any acidic soil Al toxicity is the major and often first limitation to plant growth. Effects of Al toxicity include; inhibition of root growth, inhibition of root cell division, modification of the cytoskeleton and inhibition of nutrient uptake (Bojórquez-Quintal et al., 2017; Kaur et al., 2019). In many cases direct Al toxicity effects are not obvious, instead they are manifest as P deficiency symptoms with overall stunting, dark green leaves, late maturity and purpling of stems, leaves and leaf veins. All these P deficiency symptoms occur because of delocalized P metabolism by Al. Also P ends up being fixed by Fe in most acidic soils, degrading conditions for crop production (Kaur et al., 2019).
The second prevalent toxic metal in acidic soil is Mn. In contrast with Al toxicity, Mn as an essential plant nutrient, toxic when plants absorb it in excess. Mn toxicity is prevalent at pHs as high as 5.6; this makes it more important as a constraint in crop production, in some acid mineral soils, than Al (Sumner et al., 1991).
Low pH stress has been associated with inhibition of root growth (Yang et al., 2005) by facilitating H+ influx into roots, which causes poor plant growth. High H+ influx causes depolarization of the plasma membrane, impacting the acidity of the cytoplasm (Babourina et al., 2001). Thus generally, low pH stress caused by H+ adversely affects root tissues, which leads to reduced growth and development of crops. There is a meaningful lack of knowledge regarding how various plants respond to low pH conditions; low pH tolerance would be a good trait to select for in plant breeding programs.
Alkalinity Stress
Most land desertification in the world is linked with soil alkalization and lower water availability and retention ability, soil erodibility and also reduced biodiversity. Alkaline soils are characterized by high concentrations of carbonates (CO32–) and bicarbonates (HCO3–) which have the ability to neutralize acids (Bailey, 1996; Rashid et al., 2019), high pH and poor amounts of organic carbon, leading ultimately to poor availability of nutrients. Other minor contributors to soil alkalinity are those which result from hydroxides (OH–), borates, organic bases, silicates, phosphates and ammonia. The problem of alkalinity in soils is prevalent, as a secondary effect of drought in many places. Most arid and semi-arid regions of the globe experience soil alkalinity since concentrations of salts decrease and levels of carbonates and bicarbonates increase, leading to alkalinity of the soils. Alkalinity stress effects on crop plants are remarkably similar to those of salt stress (Xu et al., 2013), though it has remained a less researched area. Most of the studies to date have dwelt on the relationship between salinity stress and alkalinity stress by showing a strong link between them (Bui et al., 2014). The stress caused by carbonate salts is sometimes higher than that of salinization by NaCl and NaSO4 (Shi and Sheng, 2005).
The higher pH of sodic soils results in nutrient imbalance stress in crop production by affecting bioavailability of phosphorus, iron, copper, boron and zinc (Chen et al., 2011). However, it is important to note that under high pH (more OH– than H+) the activity of the OH– ions comes into play, increasing alkalinity at pHs greater than 11, whereas below pH 11 forms of carbonates are responsible for alkalinity (Whipker et al., 1996). In alkaline soils with pH < 11 there are major effects of pH on plants that are largely due to carbonate ions, rather than hydroxide ions.
Nutrient availability for plant uptake is related to soil chemistry, which is predominantly influenced by pH. When addressing pH related stresses, many other associated stresses come into play; under alkalinity stress, apart from essential element stresses, there are also osmotic, ion-induced injury or high pH effects that are automatically problematic (Lynch and Clair, 2004).
Most plants under alkalinity stress manifest stunted growth due to poor nutrient uptake and leaf chlorosis due to high and low uptake of Na+ and Fe+, respectively (Zhang et al., 2012; Singh et al., 2018). High levels of Na+ interfere with stomatal closure, which worsens the problem of water loss for plants (Bernstein, 1975), a common phenomenon under saline conditions, which can be similar under alkalinity conditions. Bicarbonates reduce Fe absorption and sometimes increase internal precipitation of Fe (Norvell and Adams, 2006), all of which affects synthesis of chlorophyll, and hence leads to chlorosis. Chlorosis, which is linked to diminished photosynthesis, has an ultimate impact on plant growth. Similarly, in calcareous soils reports have shown Fe and lime-induced chlorosis are dominant factors leading to iron deficiency (Coulombe et al., 1984). Conversely, the concentration of bicarbonate ions in the soil is known to induce minor to severe stunting in plants. For instance, cucumber plants were reported to experience negative effects of HCO3– on their growth (Rouphael et al., 2010).
Alkalinity stress causes inhibition of root growth due to high concentrations of HCO3– in the soil solution, though this varies among crop species. The suppression of root growth by HCO3– is associated with inhibition of respiration by the roots (Alhendawi et al., 1997). The inhibition of root growth may also result from excessive accumulation of organic acids (OAs) in root cells. Of the OAs commonly reported to stop root elongation, malate is a problem when concentrated in the elongation zone, as a result of bicarbonate in calcifuge grass species (Lee and Woolhouse, 1969). Furthermore, plant hormones are known for variation in their activity under a range of stresses, leading to alleviation of negative impacts on plants, though their final mechanisms of action are often unclear. Under alkaline stress abscisic acid (ABA) is secreted by roots into the rhizosphere, which negatively impacts root growth as water becomes limiting (Slovik et al., 1995).
Management of Acidity and Alkalinity Stresses in Plants
Acid soil management is the application of indirect and direct means to ensure that production potential of a particular soil is regained or attained. Some of the direct acidic soil amendments include correction of acidity by liming and manipulation of agricultural practices for optimum crop yield (Yirga et al., 2019). Liming is one of the major known ways to manage acid soils. However, breeding for acidity tolerance and use of PGPM are also becoming established mechanisms to address soil acidity stress. The combination of two or more of these methods could be more helpful than single method strategies. The problem is recurrent for soils that are prone to acidification.
To ensure optimum plant growth, soil pH should be monitored and the soil amended to optimal pH levels, near neutrality. Generally, it has been established that soils with high organic matter, >5%, have pHs between 5.0 and 5.5. In contrast with soil acidity, which can be tolerated by some crops, very few crops survive in even moderately alkaline soils, due to restricted nutrient mobility and availability. Alkaline soil amendment for crop production involves the use of cultural practices (conservation tillage, crop covers and rotation, organic matter amendments, avoiding bare fallow), use of PGPM and production of alkali stress tolerant crop varieties.
PGPM Enhance Plant Resilience to Acidity and Alkalinity Stresses
Acidity
Sustainable agricultural innovations are not immune to the effects of acidic soils. Considerable effort has been made regarding the use of PGPM as a strategy for dealing with various environmental stresses of plants. Current understanding indicates that about 2–5% of culturable rhizobacteria are plant growth promotors, either directly and or indirectly (Dutta and Bora, 2019); the need to exploit this resource in agriculture is increasing. Legume symbioses with rhizobia, a well-studied beneficial plant microbe interaction, are constrained with regard to nodule formation and poor and/or failed bacterial survival (Correa and Barneix, 1997) by various stresses, including soil acidity. Many reports share a similar perspective, indicating that selecting for acid-soil tolerant symbiotic partners can improve the survival and productivity of crop plants (Zhang et al., 2020). With Sinorhizobium, for instance, the genetic control of acid tolerance is becoming increasingly understood (Draghi et al., 2016). In legume symbioses, as with other interactions, there is a requirement for specific recognition of signal molecules produced by both bacterial and plant partners. One of the factors affecting the signal molecule exchange and recognition process is pH, with effects on both plant and bacterial partners (Zhang et al., 2020). Though there have been few advances in understanding the direct effect of PGPM on acid stress, there is a substantial body of research literature indicating the potential for using microbes to address secondary effects of acidity in the soil, such as Al toxicity (Zerrouk et al., 2016) and P deficiency (de la Luz Mora et al., 2017; Delfim et al., 2018).
It has been established that, within the legume-rhizobia nitrogen fixing symbiosis, rhizobia isolated from acidic soils have more ability to colonize and improve plant growth under acidic conditions. Several genes contributing to rhizobial survival under acidic conditions have been identified. Some of them are those which code for stress tolerance proteins, including ActA (apolipoprotein N-acyl transferase) and ActR (response regulator) (Tiwari et al., 1996a, b). Despite advances regarding tolerance and ability of legumes to nodulate under low pH conditions, much remains unknown regarding signal molecules in this capacity.
The other highly studied plant microbe interaction is mycorrhiza associations, which are associated with about 90% of all terrestrial plants. With this degree of interaction, it is clear that for a large proportion of acid dominated soils where these plants occur there are mycorrhizal associations with the plants growing there. There are two main groups of these fungi: ectomycorrhiza and endomycorrhiza (Bonfante and Genre, 2010). Endomycorrhiza reside inside plant cells and form arbuscules within cortical root cells, which are directly involved in the symbiosis beneficial effects. This type of mycorrhizal association is as old as the evidence of first terrestrial plants on the earth (Chagas et al., 2018) and is the most widespread type. Endomycorrhiza are further divided into arbuscular mycorrhiza (AM), ericoid and orchidoid associations (Parniske, 2005). The promiscuity of AM make them associate with a wide range of hosts and assist most plant species with a range of stresses, and assist plants under nutrient imbalance situations, most notably P deficiencies (Zhu et al., 2007). Plants depend on AM particularly for P uptake from the nutrient stressed soils and the fungi involved depend on plants for their C requirements (Mishra et al., 2017). Acidic soils are also associated with metal toxicities, ultimately resulting in decreased root growth, which hinders overall plant growth and development. The major constraint to plant growth in acidic soil is the toxic effects of Al, Mn, and Fe, together with P deficiency. AM associations with plants is one of the most important plant-microbe associations, due to its ability to help plants with multiple stresses, compared to other association which may address only one stress. Many reports have shown that a wide variety of AM fungal species exist in acidic soils and help plants survive in such conditions; dealing with soil pH is always complex as it has numerous effects on both roots and mycorrhizal associations (Clark, 1997; Bloom et al., 2006). Even though more investigation is required to determine the best ways to exploit the potential benefits of both partners, some development has already been achieved. Studies of plants associations with AM fungi as a strategy to thrive in acid soils has revealed that these fungi provided benefit to plant growth through the ability to bind to toxic ions, secrete organic acids and glomalin (Thangavelu et al., 2014; Figure 2). Like plants AM fungal species also vary in their tolerance to acidic soils. AM fungal colonization of plant roots in soils is decreased at pH < 4 (Higo et al., 2011), which can explain differences in the ability of various AM fungal species to enhance plant growth under acidity stress and their variation in mechanisms to be employed (Figure 2).
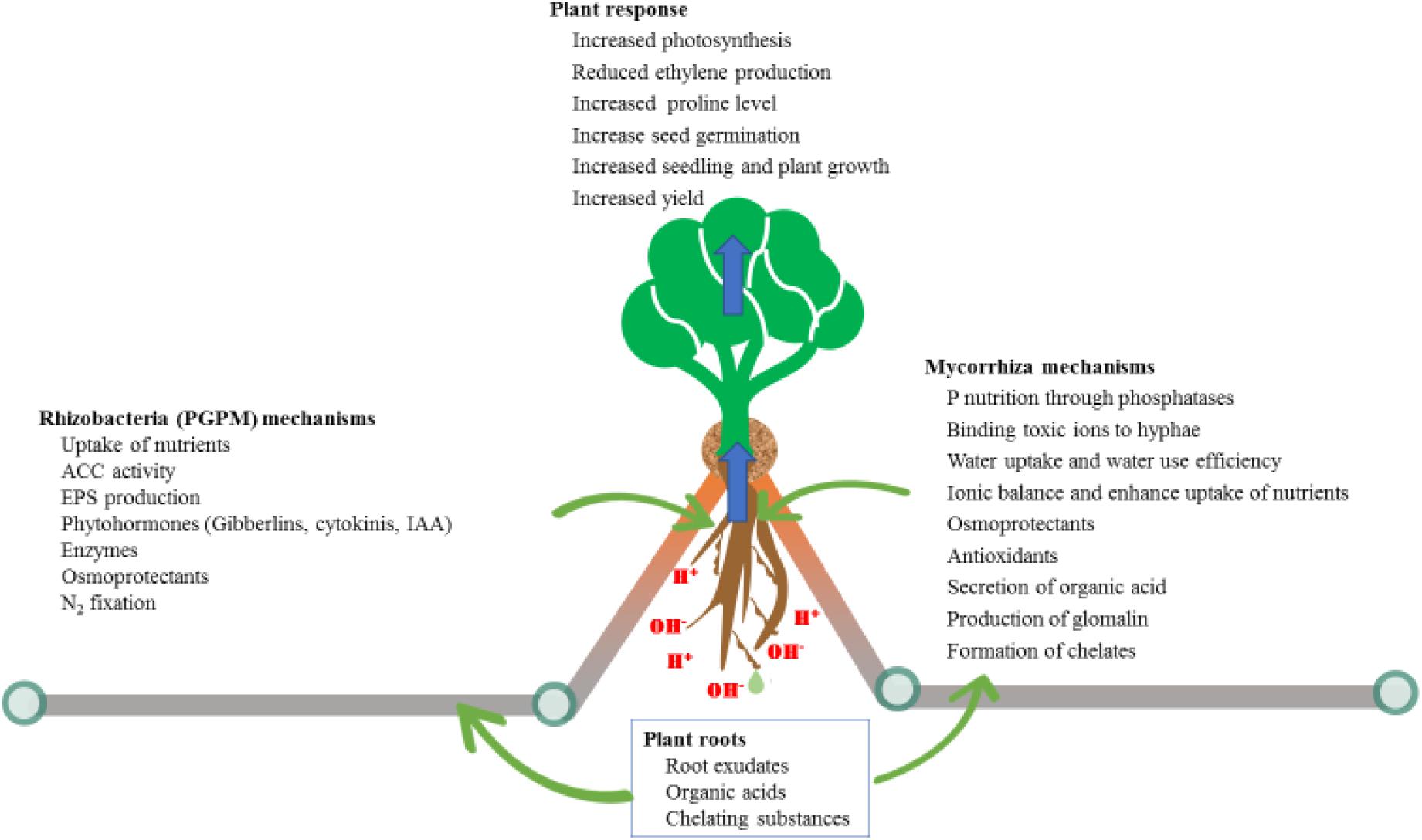
Figure 2. Differences in mechanisms used by microbes (fungal versus bacterial) to alleviate different environmental stressors.
Despite the advantages of AM to plants their beneficial impact on plants in acidic soils is less well documented than in non-acidic soils. The few studies that have examined the potential of AM fungi to assist plants in dealing with acidity stress (Table 1) have shown clearly that there are numerous benefits that can be acquired by plants. Clark (2002), revealed that use of various species of AM resulted in variable effects on switchgrass growth, but that all AM fungi caused greater yields than the control.
Alkalinity
Developing proper and economically beneficial techniques for managing major challenges in crop plants, such as alkalinity stress, requires intensive research. PGPM with dual (salinity and alkalinity) stress tolerance, termed as haloalkaliphilic, have the ability to alleviate both salinity and alkalinity stresses of plants and improve growth (Siddikee et al., 2011; Table 2). Bacteria with the ability to maintain their intrinsic pH below 9 when external pH is 9–11 are called alkaliphilic. This tolerance to alkalinity is achieved through a cytoplasmic membrane proton transfer system (Torbaghan et al., 2017). The Bacillaceae family of rhizobacteria is among the best described in this capacity, and has a wide range of host plants; the group of PGPM consists of 15 genera which include Alkalibacillus, Bacillus and Haloalkalibacillus, among others (Radhakrishnan et al., 2017; Torbaghan et al., 2017). The use of alkalinity tolerant rhizobacteria, with ability to produce indole acetic acid (IAA) and 1-aminocyclopropane-1-carboxylate (ACC), has the advantage of reducing ethylene production, and increasing K levels (Figure 2). This increases the relative humidity in plants and helps maintain ion homeostasis (Soleimani et al., 2018) which increased wheat growth. Several other mechanisms are also reported (Figure 2) by which PGPM alleviate abiotic stress in plants, such as by modulating hormones, enzymes, photosynthesis, secretion of organic acids and secondary metabolites (Bisht et al., 2019; Dixit et al., 2020). Moreover, rhizobacteria are involved in cycling of key nutrients such as N and C, which ensures long term reserves of nutrients in the soil.
The use of legumes is also regarded as a means of alkalinity management and has been applied in various parts of the world, as they have an inherent ability to acidify the soil. The combination of legumes and their symbionts to alleviate alkalinity problems is a sustainable way to redeem unproductive soils for agricultural use again. The use of AM fungi and rhizobia are among the oldest and best documented plant symbioses, and our understanding of them has shed light on helping plants withstand a range of stress conditions (Kumar et al., 2009). Abd-Alla et al. (2014) demonstrated that rhizobia and mycorrhizae can work together on faba bean to help the crop grow well under alkaline stress conditions; they found clear and synergistic contributions of inoculated symbionts to alkalinity stress resilience of faba bean, and that this resulted in increased nodulation, nitrogenase activity and yield.
Plant Breeding and PGPM to Improve Crop Yield Under Conditions of Acidity and Alkalinity Stresses
Use of Alkalizing Agents in Acidic Soils
Acidic soil causes negative effects on plant growth and development (Rengel, 2011) leading to poor or no crop yield. Liming is a practice that corrects soil pH, moving it toward neutrality by addition of alkalizing materials rich in Ca and Mg. This material can be in solid (limestone, chalk) or liquid (hydrated lime) forms. Liming is the most common amendment technique and uses CaCO3 or MgCO3 as they have substantial ability to neutralize acids, hence increasing soil pH. By increasing soil pH, Al toxicity, which inhibits root growth is alleviated; P, which is fixed by Fe, becomes available leading to improved crop productivity and yield. This is the reason for the popularity of liming (Fageria and Baligar, 2008) as an amendment for acidic soils. How much lime to be applied for soil acidity amendment depends mainly on the type of lime, fertility status, crop grown, management practices and cost benefit analysis (economic) considerations.
Use of Acid-Tolerant Crop Varieties
In acidic soils some plants are more tolerant than others, with variation even among genotypes of the same species; choosing the right crops based on the pH status of the soil is important (Hede et al., 2001). Though the soil may continue acidifying if no liming is done, it is advisable to include management practices, such as reducing the use of nitrogen fertilizers to reduce nitrate leaching, which increases soil acidity. Plowing crops or pasture into the soil will help reduce acidification of soil. In addition, rotation of legumes, which are rhizosphere acidifiers, with less acidifying crops would be of great importance in reducing soil acidity effects.
Use of Acidifying Agents
Lowering soil pH to reduce alkalinity can be achieved by adding organic carbon to the soil; minimum tillage also helps to improve water retention and soil structure improvement, as does the use of cover crops and legumes, all of which acidify the soil. Furthermore, the use of elemental sulfur acidifies the soil by neutralizing alkalinity (Goulding, 2016).
The use of manure and compost is a common practice for management alkaline soils, as it improves organic carbon pools and soil structure. Manure and compost decrease soil pH greatly, as a result of releasing NH4+, CO2 and organic acids during microbial decomposition (Walker et al., 2003). All these sum up to improvement of soil structure, water holding capacity and nutrient availability and, hence, soil health and productivity is regained.
Planting of cover crops helps alkaline soils in many ways, including reduction of exposure of the soil to agents of erosion. The cover crops also help in dissolution of carbonates, through root exudates, improvement of the soil structure and addition of organic matter to soil. Best cover crops for alkaline soils are sorghum and legumes with high carbon sequestration by stabilizing soil organic matter and structure, hence carbon and nitrogen concentration in the soil is increased (Williams et al., 2016).
Ammonium sulfate and other sulfur containing fertilizers cause quick declines in soil pH, which makes them good fertilizer options for alkaline soils. When oxidized elemental S and SO2 from the atmosphere produce acids which decrease the pH of alkaline soils. Similarly, nitrogen fixation by legumes forms NH4+ inside the nodule, and excessive uptake of K+ causes charge imbalance, both of which leads to proton release by the roots to balance the charge (Marschner, 2011), resulting in rhizosphere pH decline.
Use of Alkalinity Tolerant Crop Varieties
Plants have developed various strategies in response to environmental stimuli, such as activation of various metabolic defense molecules. Among the metabolic molecules produced by plants to enhance defense capacity are salicylic acid, ethylene, calcium and jasmonic acid (Klessig and Malamy, 1994). Of the mentioned defense molecules salicylic acid has been confirmed to confer alkalinity tolerance to tomato plants, when applied exogenously, by reducing reactive oxygen species (ROS) generation and improving antioxidant defense against alkaline stress (Khan et al., 2019). Similarly, it was demonstrated that SA applied in combination with Si had positive effects on alkalinity tolerance in tomatoes (Khan et al., 2019). From such reports, it is clear that much is still to be understood regarding how different tolerance molecules and beneficial elements work together in helping plants grow under such alkaline stress conditions.
Plant breeding is one important approach to ensuring crop productivity in stress prone areas, including alkalinity of soil and water. A range of plants have shown various mechanisms of tolerance to alkaline stress, most of them showing early seed germination and seedling establishment. Cultivars of lentil tolerant to alkalinity stress are known to have shoots with a thicker epidermis than sensitive cultivars (Singh et al., 2018). Similarly, tolerant lentils (Singh et al., 2018), finger millet (Krishnamurthy et al., 2014) and Lotus tenuis (Paz et al., 2012) minimize Na+ uptake by having intact pericycle and stele regions. Despite the presence of tolerance mechanisms for alkalinity stress by plants much remains unknown in relation to other related stresses, such as salinity and drought. According to Bui et al. (2014), the success of breeding for salinity tolerance required that increased attention also be placed on alkalinity tolerance.
As previously indicated a major limiting nutrient under alkaline stress is Fe; plants have developed two strategies to deal with this problem. Firstly, most plants optimize Fe uptake via transporter by first reducing Fe+++ to Fe ++ in the root plasma membrane. Secondly, development of specific iron uptake by release of phytosiderophores for chelating iron (Curie et al., 2001). The iron chelators have high affinity to Fe+++ when released into the rhizosphere; the chelated iron is then taken up by the plant through yellow stripe-like (YSL) transporters a family of protein used by maize (Yordem et al., 2011).
Plants have also been shown to have mechanisms for alkaline stress management through acidification of the rhizosphere by H+ ATPase activity related to plasma membrane proton extrusion (Xu et al., 2013).
Future Perspectives
From various studies of tolerance to extreme pH there is a wider range of adaptation of microbes than plants. Our limited knowledge on the full information available in the genome of microbes that help them in adapting to such extremes will pave the way to understanding and broadening their application in biotechnology and crop production. The constant need to explore the unknown potential of microbes in helping enhance plant productivity under various unfavorable conditions of growth and is currently developing quickly and contributing to improvement of plant growth under stressful environmental conditions.
Currently we use synthetic fertilizers as part of our approach to feeding the growing global population. The uptake efficiency of these fertilizers by plants is generally 30–50%, leading to economic losses and large environmental impacts, due to large quantities of the fertilizer being lost to water bodies and the atmosphere (Adesemoye and Kloepper, 2009). PGPM have shown their ability to increase the efficiency of nutrient release from fertilizer and subsequent uptake by plants. Bearing in mind that soil acidification and alkalization are both gradual and progressive processes, preparing ahead of time is not optional. The future of crop production sustainability meaningfully depends on better understanding of PGPM in conferring stress alleviation and ways to effectively introducing them under field conditions, to provide the same results that are observed under controlled environment conditions.
Conclusion
The importance of pH in agriculture is well understood, and similar to a patient’s temperature in humans. Most of the literature has acknowledged pH is a “master” variable in productivity of agricultural soils as it controls soil chemistry. This review started with an evolutionary perspective regarding plants and microbes, an interaction that has always been present, to the benefit of both members of the partnership. It is becoming clearer that the interaction has always provided plants with a mechanism of survival even in harsh environmental stress conditions. With the ongoing development of climate change conditions and associated multiple stresses, potentially occurring simultaneously and impacting plant productivity. This is quite similar to pH stress which is accompanied by other stresses in its effects. The reviewed literature has shown that most of the acid and alkaline soil remediation measures are focused on cultural practices and breeding for tolerance. Little has been established regarding utility of PGPM as a mechanism of dealing with acidity and alkalinity stresses. Our future will require further breeding for pH stress and the help of microbes that provide enhanced tolerance to pH stress. With this consideration in mind the potential for alleviation of extreme pH stress by PGPM has become clearer and there is a need to now focus more research effort specifically on acid and alkaline stresses in this regard.
Author Contributions
LM structured and prepared manuscript initially. DS provided the conceptual framework for the manuscript as well as feedback and guidance during manuscript development. Both authors contributed to the article and approved the submitted version.
Funding
Funding was provided by the Consortium de Recherche et Innovations en Bioprocédés Industriels au Québec (CRIBIQ), grant number 2017-034-C30.
Conflict of Interest
The authors declare that the research was conducted in the absence of any commercial or financial relationships that could be construed as a potential conflict of interest.
References
Abd-Alla, M. H., El-Enany, A.-W. E., Nafady, N. A., Khalaf, D. M., and Morsy, F. M. (2014). Synergistic interaction of Rhizobium leguminosarum bv. viciae and arbuscular mycorrhizal fungi as a plant growth promoting biofertilizers for faba bean (Vicia faba L.) in alkaline soil. Microbiol. Res. 169, 49–58. doi: 10.1016/j.micres.2013.07.007
Adesemoye, A. O., and Kloepper, J. W. (2009). Plant–microbes interactions in enhanced fertilizer-use efficiency. Appl. Microbiol. Biotechnol. 85, 1–12. doi: 10.1007/s00253-009-2196-0
Alhendawi, R. A., Römheld, V., Kirkby, E. A., and Marschner, H. (1997). Influence of increasing bicarbonate concentrations on plant growth, organic acid accumulation in roots and iron uptake by barley, sorghum, and maize. J. Plant Nutr. 20, 1731–1753. doi: 10.1080/01904169709365371
Alloush, G., Zeto, S., and Clark, R. (2000). Phosphorus source, organic matter, and arbuscular mycorrhiza effects on growth and mineral acquisition of chickpea grown in acidic soil. J. Plant Nutr. 23, 1351–1369. doi: 10.1080/01904160009382105
Babourina, O., Hawkins, B., Lew, R. R., Newman, I., and Shabala, S. (2001). K+ transport by Arabidopsis root hairs at low pH. Funct. Plant Biol. 28, 637–643.
Badri, D. V., and Vivanco, J. M. (2009). Regulation and function of root exudates. Plant Cell Environ. 32, 666–681. doi: 10.1111/j.1365-3040.2009.01926.x
Bailey, D. (1996). Alkalinity, pH, and Acidification. Water, Media, and Nutrition for Greenhouse Crops. Batavia, IL: Ball Publishing, 69–91.
Baltrus, D. A. (2017). Adaptation, specialization, and coevolution within phytobiomes. Curr. Opin. Plant Biol. 38, 109–116. doi: 10.1016/j.pbi.2017.04.023
Beneduzi, A., Ambrosini, A., and Passaglia, L. M. (2012). Plant growth-promoting rhizobacteria (PGPR): their potential as antagonists and biocontrol agents. Genet. Mol. Biol. 35, 1044–1051. doi: 10.1590/s1415-47572012000600020
Bernstein, L. (1975). Effects of salinity and sodicity on plant growth. Annu. Rev. Phytopathol. 13, 295–312. doi: 10.1146/annurev.py.13.090175.001455
Bisht, N., Tiwari, S., Singh, P. C., Niranjan, A., and Chauhan, P. S. (2019). A multifaceted rhizobacterium Paenibacillus lentimorbus alleviates nutrient deficiency-induced stress in Cicer arietinum L. Microbiol. Res. 223, 110–119. doi: 10.1016/j.micres.2019.04.007
Biswas, A., Dasgupta, S., Das, S., and Abraham, A. (2007). A synergy of differential evolution and bacterial foraging optimization for global optimization. Neural Netw. World 17, 607.
Blake, L., Goulding, K., Mott, C., and Johnston, A. (1999). Changes in soil chemistry accompanying acidification over more than 100 years under woodland and grass at Rothamsted Experimental Station, UK. Eur. J. Soil Sci. 50, 401–412. doi: 10.1046/j.1365-2389.1999.00253.x
Blankenhorn, D., Phillips, J., and Slonczewski, J. L. (1999). Acid-and base-induced proteins during aerobic and anaerobic growth of Escherichia coli revealed by two-dimensional gel electrophoresis. J. Bacteriol. 181, 2209–2216. doi: 10.1128/jb.181.7.2209-2216.1999
Bloom, A. J., Frensch, J., and Taylor, A. R. (2006). Influence of inorganic nitrogen and pH on the elongation of maize seminal roots. Ann. Bot. 97, 867–873. doi: 10.1093/aob/mcj605
Bojórquez-Quintal, E., Escalante-Magaña, C., Echevarría-Machado, I., and Martínez-Estévez, M. (2017). Aluminum, a friend or foe of higher plants in acid soils. Front. Plant Sci. 8:1767. doi: 10.3389/fpls.2017.01767
Bolan, N., Hedley, M., and White, R. (1991). Processes of soil acidification during nitrogen cycling with emphasis on legume based pastures. Plant Soil 134, 53–63. doi: 10.1007/bf00010717
Bonfante, P., and Genre, A. (2010). Mechanisms underlying beneficial plant–fungus interactions in mycorrhizal symbiosis. Nat. Commun. 1:48.
Booth, I. R., Cash, P., and O’Byrne, C. (2002). Sensing and adapting to acid stress. Antonie Van Leeuwenhoek 81, 33–42.
Bui, E. N., Thornhill, A., and Miller, J. T. (2014). Salt-and alkaline-tolerance are linked in Acacia. Biol. Lett. 10:20140278. doi: 10.1098/rsbl.2014.0278
Bulgarelli, D., Schlaeppi, K., Spaepen, S., Van Themaat, E. V. L., and Schulze-Lefert, P. (2013). Structure and functions of the bacterial microbiota of plants. Annu. Rev. Plant Biol. 64, 807–838. doi: 10.1146/annurev-arplant-050312-120106
Burt, R. (2014). Soil Survey Staff: Soil Survey Field and Laboratory Methods Manual-Soil Survey Investigations Report, Vol. 51. Washington, DC: US Department of Agriculture, 227–234.
Celiker, H., and Gore, J. (2013). Cellular cooperation: insights from microbes. Trends Cell Biol. 23, 9–15. doi: 10.1016/j.tcb.2012.08.010
Chagas, F. O., De Cassia Pessotti, R., Caraballo-Rodríguez, A. M., and Pupo, M. T. (2018). Chemical signaling involved in plant–microbe interactions. Chem. Soc. Rev. 47, 1652–1704. doi: 10.1039/c7cs00343a
Chen, L., Yin, H., Xu, J., and Liu, X. (2011). Enhanced antioxidative responses of a salt-resistant wheat cultivar facilitate its adaptation to salt stress. Afr. J. Biotechnol. 10, 16884–16886.
Chu, H., Sun, H., Tripathi, B. M., Adams, J. M., Huang, R., Zhang, Y., et al. (2016). Bacterial community dissimilarity between the surface and subsurface soils equals horizontal differences over several kilometers in the western Tibetan Plateau. Environ. Microbiol. 18, 1523–1533. doi: 10.1111/1462-2920.13236
Clark, R. (1997). Arbuscular mycorrhizal adaptation, spore germination, root colonization, and host plant growth and mineral acquisition at low pH. Plant Soil 192, 15–22.
Clark, R. (2002). Differences among mycorrhizal fungi for mineral uptake per root length of switchgrass grown in acidic soil. J. Plant Nutr. 25, 1753–1772. doi: 10.1081/pln-120006056
Compant, S., Samad, A., Faist, H., and Sessitsch, A. (2019). A review on the plant microbiome: ecology, functions and emerging trends in microbial application. J. Adv. Res. 19, 29–37. doi: 10.1016/j.jare.2019.03.004
Correa, O., and Barneix, A. (1997). Cellular mechanisms of pH tolerance in Rhizobium loti. World J. Microbiol. Biotechnol. 13, 153–157.
Coulombe, B., Chaney, R., and Wiebold, W. (1984). Use of bicarbonate in screening soybeans for resistance to iron chlorosis. J. Plant Nutr. 7, 411–425. doi: 10.1080/01904168409363208
Curie, C., Panaviene, Z., Loulergue, C., Dellaporta, S. L., Briat, J.-F., and Walker, E. L. (2001). Maize yellow stripe1 encodes a membrane protein directly involved in Fe (III) uptake. Nature 409, 346–349. doi: 10.1038/35053080
Daguerre, Y., Plett, J. M., and Veneault-Fourrey, C. (2017). “Signaling pathways driving the development of ectomycorrhizal symbiosis,” in Molecular Mycorrhizal Symbiosis, ed. F. Martin (Hoboken, NJ: John Wiley & Sons, Inc.), 141–157. doi: 10.1002/9781118951446.ch9
Damodaran, T., Mishra, V., Jha, S., Pankaj, U., Gupta, G., and Gopal, R. (2019). Identification of rhizosphere bacterial diversity with promising salt tolerance, PGP traits and their exploitation for seed germination enhancement in sodic soil. Agric. Res. 8, 36–43. doi: 10.1007/s40003-018-0343-5
de la Luz Mora, M., Demanet, R., Acuña, J. J., Viscardi, S., Jorquera, M., Rengel, Z., et al. (2017). Aluminum-tolerant bacteria improve the plant growth and phosphorus content in ryegrass grown in a volcanic soil amended with cattle dung manure. Appl. Soil Ecol. 115, 19–26. doi: 10.1016/j.apsoil.2017.03.013
Delfim, J., Schoebitz, M., Paulino, L., Hirzel, J., and Zagal, E. (2018). Phosphorus availability in wheat, in volcanic soils inoculated with phosphate-solubilizing Bacillus thuringiensis. Sustainability 10:144. doi: 10.3390/su10010144
Dixit, V. K., Misra, S., Mishra, S. K., Tewari, S. K., Joshi, N., and Chauhan, P. S. (2020). Characterization of plant growth-promoting alkalotolerant Alcaligenes and Bacillus strains for mitigating the alkaline stress in Zea mays. Antonie Van Leeuwenhoek 113, 889–905. doi: 10.1007/s10482-020-01399-1
Draghi, W. O., Del Papa, M. F., Hellweg, C., Watt, S., Watt, T., Barsch, A., et al. (2016). A consolidated analysis of the physiologic and molecular responses induced under acid stress in the legume-symbiont model-soil bacterium Sinorhizobium meliloti. Sci. Rep. 6:29278.
Dutta, J., and Bora, U. (2019). Role of PGPR for Alleviating Aluminum Toxicity in Acidic Soil. Plant Growth Promoting Rhizobacteria for Sustainable Stress Management. Berlin: Springer.
Fageria, N., and Baligar, V. (2008). Ameliorating soil acidity of tropical Oxisols by liming for sustainable crop production. Adv. Agron. 99, 345–399. doi: 10.1016/s0065-2113(08)00407-0
Fernández, L. A., Zalba, P., Gómez, M. A., and Sagardoy, M. A. (2007). Phosphate-solubilization activity of bacterial strains in soil and their effect on soybean growth under greenhouse conditions. Biol. Fertil. Soils 43, 805–809. doi: 10.1007/s00374-007-0172-3
Filipek, T. (1994). Natural and anthropogenic causes and effects of soil acidification. Zeszyty Problemowe Postêpów Nauk Rolniczych 413, 1–7.
Gilbert, J. A., Blaser, M. J., Caporaso, J. G., Jansson, J. K., Lynch, S. V., and Knight, R. (2018). Current understanding of the human microbiome. Nat. Med. 24:392. doi: 10.1038/nm.4517
Glick, B. R. (1995). The enhancement of plant growth by free-living bacteria. Can. J. Microbiol. 41, 109–117. doi: 10.1139/m95-015
Gopalakrishnan, S., Sathya, A., Vijayabharathi, R., Varshney, R. K., Gowda, C. L., and Krishnamurthy, L. (2015). Plant growth promoting rhizobia: challenges and opportunities. 3 Biotech 5, 355–377. doi: 10.1007/s13205-014-0241-x
Gough, C., and Cullimore, J. (2011). Lipo-chitooligosaccharide signaling in endosymbiotic plant-microbe interactions. Mol. Plant Microb. Interact. 24, 867–878. doi: 10.1094/mpmi-01-11-0019
Goulding, K. (2016). Soil acidification and the importance of liming agricultural soils with particular reference to the United Kingdom. Soil Use Manage. 32, 390–399. doi: 10.1111/sum.12270
Graham, P. H., Draeger, K. J., Ferrey, M. L., Conroy, M. J., Hammer, B. E., Martinez, E., et al. (1994). Acid pH tolerance in strains of Rhizobium and Bradyrhizobium, and initial studies on the basis for acid tolerance of Rhizobium tropici UMR1899. Can. J. Microbiol. 40, 198–207. doi: 10.1139/m94-033
Hansen, V., Bonnichsen, L., Nunes, I., Sexlinger, K., Lopez, S., Van Der Bom, F., et al. (2020). Seed inoculation with Penicillium bilaiae and Bacillus simplex affects the nutrient status of winter wheat. Biol. Fertil. Soils 56, 97–109.
Hao, T., Zhu, Q., Zeng, M., Shen, J., Shi, X., Liu, X., et al. (2019). Quantification of the contribution of nitrogen fertilization and crop harvesting to soil acidification in a wheat-maize double cropping system. Plant Soil 434, 167–184. doi: 10.1007/s11104-018-3760-0
Hede, A., Skovmand, B., and Lopez Cesati, J. (2001). “Acid soils and aluminum toxicity,” in Application of Physiology in Wheat Breeding, eds M. P. Reynolds, J. I. Ortiz-Monasterio, and A. McNa (Mexico City, MX: CIMMYT).
Higo, M., Isobe, K., Kang, D.-J., Maekawa, T., and Ishii, R. (2011). Molecular diversity and spore density of indigenous arbuscular mycorrhizal fungi in acid sulfate soil in Thailand. Ann. Microbiol. 61, 383–389. doi: 10.1007/s13213-010-0142-0
Huang, X.-F., Chaparro, J. M., Reardon, K. F., Zhang, R., Shen, Q., and Vivanco, J. M. (2014). Rhizosphere interactions: root exudates, microbes, and microbial communities. Botany 92, 267–275. doi: 10.1139/cjb-2013-0225
Hyyryläinen, H. L., Bolhuis, A., Darmon, E., Muukkonen, L., Koski, P., Vitikainen, M., et al. (2001). A novel two−component regulatory system in Bacillus subtilis for the survival of severe secretion stress. Mol. Microbiol. 41, 1159–1172. doi: 10.1046/j.1365-2958.2001.02576.x
Jeanbille, M., Buée, M., Bach, C., Cébron, A., Frey-Klett, P., Turpault, M. P., et al. (2016). Soil parameters drive the structure, diversity and metabolic potentials of the bacterial communities across temperate beech forest soil sequences. Microb. Ecol. 71, 482–493. doi: 10.1007/s00248-015-0669-5
Kaur, C., Selvakumar, G., and Ganeshamurthy, A. (2019). Acid Tolerant Microbial Inoculants: A Requisite for Successful Crop Production in Acidic Soils. Phyto and Rhizo Remediation. Berlin: Springer.
Kenrick, P., and Crane, P. R. (1997). The origin and early evolution of plants on land. Nature 389, 33–39. doi: 10.1038/37918
Kenrick, P., and Strullu-Derrien, C. (2014). The origin and early evolution of roots. Plant Physiol. 166, 570–580. doi: 10.1104/pp.114.244517
Khan, A., Kamran, M., Imran, M., Al-Harrasi, A., Al-Rawahi, A., Al-Amri, I., et al. (2019). Silicon and salicylic acid confer high-pH stress tolerance in tomato seedlings. Sci. Rep. 9, 1–16.
Klessig, D. F., and Malamy, J. (1994). The salicylic acid signal in plants. Plant Mol. Biol. 26, 1439–1458. doi: 10.1007/bf00016484
Kloepper, J. W., Lifshitz, R., and Zablotowicz, R. M. (1989). Free-living bacterial inocula for enhancing crop productivity. Trends Biotechnol. 7, 39–44. doi: 10.1016/0167-7799(89)90057-7
Knack, J., Wilcox, L., Delaux, P.-M., Ané, J.-M., Piotrowski, M., Cook, M., et al. (2015). Microbiomes of streptophyte algae and bryophytes suggest that a functional suite of microbiota fostered plant colonization of land. Int. J. Plant Sci. 176, 405–420. doi: 10.1086/681161
Kochian, L. V., Hoekenga, O. A., and Pineros, M. A. (2004). How do crop plants tolerate acid soils? Mechanisms of aluminum tolerance and phosphorous efficiency. Annu. Rev. Plant Biol. 55, 459–493. doi: 10.1146/annurev.arplant.55.031903.141655
Kong, X., Guo, Y., Xue, S., Hartley, W., Wu, C., Ye, Y., et al. (2017). Natural evolution of alkaline characteristics in bauxite residue. J. Clean. Prod. 143, 224–230. doi: 10.1016/j.jclepro.2016.12.125
Krishnamurthy, L., Upadhyaya, H. D., Purushothaman, R., Gowda, C. L. L., Kashiwagi, J., Dwivedi, S. L., et al. (2014). The extent of variation in salinity tolerance of the minicore collection of finger millet (Eleusine coracana L. Gaertn.) germplasm. Plant Sci. 227, 51–59. doi: 10.1016/j.plantsci.2014.07.001
Kumar, A., Sharma, S., and Mishra, S. (2009). Effect of alkalinity on growth performance of Jatropha curcas inoculated with PGPR and AM fungi. J. Phytol. 1, 177–184.
Laksmanan, V., Selvaraj, G., and Bais, H. (2014). Functional soil microbiome: belowground solutions to an aboveground problem. Plant Physiol. 66, 689–700. doi: 10.1104/pp.114.245811
Läuchli, A., and Grattan, S. R. (2012). “Soil pH extremes,” in Plant Stress Physiology, ed. S. Shabala (Wallingford: Centre for Agriculture and Bioscience International), 194. doi: 10.1079/9781845939953.0194
Lee, J., and Woolhouse, H. (1969). A comparative study of bicarbonate inhibition of root growth in calcicole and calcifuge grasses. New Phytol. 68, 1–11. doi: 10.1111/j.1469-8137.1969.tb06413.x
Lim, J.-H., and Kim, S.-D. (2013). Induction of drought stress resistance by multi-functional PGPR Bacillus licheniformis K11 in pepper. Plant Pathol. J. 29:201. doi: 10.5423/ppj.si.02.2013.0021
Lin, X., Wang, S., and Shi, Y. (2001). Tolerance of VA mycorrhizal fungi to soil acidity. Pedosphere 11, 105–113.
Lindström, K., Murwira, M., Willems, A., and Altier, N. (2010). The biodiversity of beneficial microbe-host mutualism: the case of rhizobia. Res. Microbiol. 161, 453–463. doi: 10.1016/j.resmic.2010.05.005
Lira, M. A. Jr. (2015). Legume-rhizobia signal exchange: promiscuity and environmental effects. Front. Microbiol. 6:945. doi: 10.3389/fmicb.2015.00945
Liu, W., Wang, Q., Hou, J., Tu, C., Luo, Y., and Christie, P. (2016). Whole genome analysis of halotolerant and alkalotolerant plant growth-promoting rhizobacterium Klebsiella sp, D5A. Sci. Rep. 6, 1–10.
Lynch, J. P., and Clair, S. B. S. (2004). Mineral stress: the missing link in understanding how global climate change will affect plants in real world soils. Field Crops Res. 90, 101–115. doi: 10.1016/j.fcr.2004.07.008
Marschner, H. (2011). Marschner’s Mineral Nutrition of Higher Plants. Cambridge, MA: Academic press.
Meharg, A. A., and Killham, K. (1990). Carbon distribution within the plant and rhizosphere in laboratory and field-grown Lolium perenne at different stages of development. Soil Biol. Biochem. 22, 471–477. doi: 10.1016/0038-0717(90)90180-8
Mishra, S., Upadhyay, S., and Shukla, R. K. (2017). The role of strigolactones and their potential cross-talk under hostile ecological conditions in plants. Front. Physiol. 7:691. doi: 10.3389/fphys.2016.00691
Misra, A., and Tyler, G. (1999). Influence of soil moisture on soil solution chemistry and concentrations of minerals in the calcicoles Phleum phleoides and Veronica spicata grown on a limestone soil. Ann. Bot. 84, 401–410. doi: 10.1006/anbo.1999.0941
Msimbira, L. A., Jaiswal, S. K., and Dakora, F. D. (2016). Identification and characterization of phages parasitic on bradyrhizobia nodulating groundnut (Arachis hypogaea L.) in South Africa. Appl. Soil Ecol. 108, 334–340. doi: 10.1016/j.apsoil.2016.09.010
Muglia, C. I., Grasso, D. H., and Aguilar, O. M. (2007). Rhizobium tropici response to acidity involves activation of glutathione synthesis. Microbiology 153, 1286–1296. doi: 10.1099/mic.0.2006/003483-0
Müller, D. B., Vogel, C., Bai, Y., and Vorholt, J. A. (2016). The plant microbiota: systems-level insights and perspectives. Annu. Rev. Genet. 50, 211–234. doi: 10.1146/annurev-genet-120215-034952
Nguyen, C. (2009). Rhizodeposition of Organic C by Plant: Mechanisms and Controls. Sustainable Agriculture. Berlin: Springer.
Norvell, W., and Adams, M. (2006). Screening soybean cultivars for resistance to iron-deficiency chlorosis in culture solutions containing magnesium or sodium bicarbonate. J. Plant Nutr. 29, 1855–1867. doi: 10.1080/01904160600899402
Oldroyd, G. E. (2013). Speak, friend, and enter: signalling systems that promote beneficial symbiotic associations in plants. Nat. Rev. Microbiol. 11, 252–263. doi: 10.1038/nrmicro2990
Oshunsanya, S. O. (2018). “Introductory chapter: relevance of soil pH to agriculture,” in Soil pH for Nutrient Availability and Crop Performance, ed. S. Oshunsanya (London: IntechOpen).
Panhwar, Q. A., Naher, U. A., Jusop, S., Othman, R., Latif, M. A., and Ismail, M. R. (2014). Biochemical and molecular characterization of potential phosphate-solubilizing bacteria in acid sulfate soils and their beneficial effects on rice growth. PLoS One 9:e97241. doi: 10.1371/journal.pone.0097241
Parniske, M. (2005). Plant–fungal associations: cue for the branching connection. Nature 435:750. doi: 10.1038/435750a
Paterson, E., Hall, J., Rattray, E., Griffiths, B., Ritz, K., and Killham, K. (1997). Effect of elevated CO2 on rhizosphere carbon flow and soil microbial processes. Glob. Chang. Biol. 3, 363–377. doi: 10.1046/j.1365-2486.1997.t01-1-00088.x
Paz, R. C., Rocco, R. A., Reinoso, H., Menéndez, A. B., Pieckenstain, F. L., and Ruiz, O. A. (2012). Comparative study of alkaline, saline, and mixed saline–alkaline stresses with regard to their effects on growth, nutrient accumulation, and root morphology of Lotus tenuis. J. Plant Growth Regul. 31, 448–459. doi: 10.1007/s00344-011-9254-4
Radhakrishnan, R., Hashem, A., and Abd_Allah, E. F. (2017). Bacillus: a biological tool for crop improvement through bio-molecular changes in adverse environments. Front. Physiol. 8:667. doi: 10.3389/fphys.2017.00667
Rashid, M., Hussain, Q., Khan, K. S., Alwabel, M. I., Ahmad, M., Alvi, S., et al. (2019). Carbon Sequestration in Alkaline Soils. Sustainable Agriculture Reviews 38. Berlin: Springer.
Ratzke, C., and Gore, J. (2018). Modifying and reacting to the environmental pH can drive bacterial interactions. PLoS Biol. 16:e2004248. doi: 10.1371/journal.pbio.2004248
Rengel, Z. (2011). Soil pH, Soil Health and Climate Change. Soil Health and Climate Change. Berlin: Springer.
Roe, A. J., Mclaggan, D., Davidson, I., O’Byrne, C., and Booth, I. R. (1998). Perturbation of anion balance during inhibition of growth of Escherichia coli by weak acids. J. Bacteriol. 180, 767–772. doi: 10.1128/jb.180.4.767-772.1998
Rohyadi, A. (2008). Growth responses of external hyphae of arbuscular mycorrhizal fungi to acidic soil conditions and their effects on cowpea growth. Microbiol. Indonesia 2, 5–5.
Rojas, R. V., and Caon, L. (2016). The international year of soils revisited: promoting sustainable soil management beyond 2015. Environ. Earth Sci. 75:1184.
Rouphael, Y., Cardarelli, M., Di Mattia, E., Tullio, M., Rea, E., and Colla, G. (2010). Enhancement of alkalinity tolerance in two cucumber genotypes inoculated with an arbuscular mycorrhizal biofertilizer containing Glomus intraradices. Biol. Fertil. Soils 46, 499–509. doi: 10.1007/s00374-010-0457-9
Rousk, J., Bååth, E., Brookes, P. C., Lauber, C. L., Lozupone, C., Caporaso, J. G., et al. (2010). Soil bacterial and fungal communities across a pH gradient in an arable soil. ISME J. 4:1340. doi: 10.1038/ismej.2010.58
Sassenrath, G., Davis, K., Sassenrath-Cole, A., and Riding, N. (2018). Exploring the physical, chemical and biological components of soil: improving soil health for better productive capacity. Kansas Agric. Exp. Stat. Res. Rep. 4:16.
Satyanarayana, T., Raghukumar, C., and Shivaji, S. (2005). Extremophilic microbes: diversity and perspectives. Curr. Sci. 89, 78–90.
Segundo, E., Martinez-Abarca, F., Dillewijn, P. V., Fernández-López, M., Lagares, A., Martinez-Drets, G., et al. (1999). Characterisation of symbiotically efficient alfalfa-nodulating rhizobia isolated from acid soils of Argentina and Uruguay. FEMS Microbiol. Ecol. 28, 169–176. doi: 10.1111/j.1574-6941.1999.tb00572.x
Shen, C., Shi, Y., Fan, K., He, J.-S., Adams, J. M., Ge, Y., et al. (2019). Soil pH dominates elevational diversity pattern for bacteria in high elevation alkaline soils on the Tibetan Plateau. FEMS Microbiol. Ecol. 95:fiz003.
Shi, D., and Sheng, Y. (2005). Effect of various salt–alkaline mixed stress conditions on sunflower seedlings and analysis of their stress factors. Environ. Exp. Bot. 54, 8–21.
Siddikee, M. A., Glick, B. R., Chauhan, P. S., Jong Yim, W., and Sa, T. (2011). Enhancement of growth and salt tolerance of red pepper seedlings (Capsicum annuum L.) by regulating stress ethylene synthesis with halotolerant bacteria containing 1-aminocyclopropane-1-carboxylic acid deaminase activity. Plant Physiol. Biochem. 49, 427–434. doi: 10.1016/j.plaphy.2011.01.015
Singh, D., Singh, C. K., Singh, Y. P., Singh, V., Singh, R., Tomar, R. S. S., et al. (2018). Evaluation of cultivated and wild genotypes of Lens species under alkalinity stress and their molecular collocation using microsatellite markers. PLoS One 13:e0199933. doi: 10.1371/journal.pone.0199933
Slovik, S., Daeter, W., and Hartung, W. (1995). Compartmental redistribution and long-distance transport of abscisic acid (ABA) in plants as influenced by environmental changes in the rhizosphere–a biomathematical model. J. Exp. Bot. 46, 881–894. doi: 10.1093/jxb/46.8.881
Smith, D. L., Gravel, V., and Yergeau, E. (2017). Editorial: signaling in the phytomicrobiome. Front. Plant Sci. 8:611. doi: 10.3389/fpls.2017.00611
Smith, D. L., Praslickova, D., and Ilangumaran, G. (2015). Inter-organismal signaling and management of the phytomicrobiome. Front. Plant Sci. 6:722. doi: 10.3389/fpls.2015.00722
Soleimani, R., Alikhani, H. A., Towfighi, H., Pourbabaei, A. A., and Khavazi, K. (2018). Indole-3-acetic acid and 1-aminocyclopropane-1-carboxylate deaminase-producing bacteria alleviate sodium stress and promote wheat growth. Iran. J. Sci. Technol. Trans. A Sci. 42, 1037–1048. doi: 10.1007/s40995-016-0070-3
Subramanian, S., Souleimanov, A., and Smith, D. L. (2016). Proteomic studies on the effects of lipo-chitooligosaccharide and thuricin 17 under unstressed and salt stressed conditions in Arabidopsis thaliana. Front. Plant Sci. 7:1314. doi: 10.3389/fpls.2016.01314
Sumner, M., Fey, M., and Noble, A. (1991). Nutrient Status and Toxicity Problems in Acid Soils. Soil Acidity. Berlin: Springer.
Suri, V., Choudhary, A. K., Chander, G., and Verma, T. (2011). Influence of vesicular arbuscular mycorrhizal fungi and applied phosphorus on root colonization in wheat and plant nutrient dynamics in a phosphorus-deficient acid Alfisol of western Himalayas. Commun. Soil Sci. Plant Anal. 42, 1177–1186. doi: 10.1080/00103624.2011.566962
Tang, C. (1998). Factors affecting soil acidification under legumes I. Effect of potassium supply. Plant Soil 199, 275–282.
Tang, C., and Rengel, Z. (2003). “Role of plant cation/anion uptake ratio in soil acidification,” in Handbook of Soil Acidity, ed. Z. Rengel (New York, NY: Marcel Dekker), 57–81.
Tang, C., Unkovich, M., and Bowden, J. (1999). Factors affecting soil acidification under legumes. III. Acid production by N 2-fixing legumes as influenced by nitrate supply. New Phytol. 143, 513–521. doi: 10.1046/j.1469-8137.1999.00475.x
Thangavelu, M., Priyadharsini, P., Eswaranpillai, U., Jaison, S., and Pandey, R. (2014). “Role of arbuscular mycorrhizal fungi in alleviation of acidity stress on plant growth,” in Use of Microbes for the Alleviation of Soil Stresses, Vol. 1, ed. M. Miransari (New York, NY: Springer).
Tiwari, R. P., Reeve, W. G., Dilworth, M. J., and Glenn, A. R. (1996a). Acid tolerance in Rhizobium meliloti strain WSM419 involves a two-component sensor-regulator system. Microbiology 142, 1693–1704. doi: 10.1099/13500872-142-7-1693
Tiwari, R. P., Reeve, W. G., Dilworthan, M. J., and Glenn, A. R. (1996b). An essential role for actA in acid tolerance of Rhizobium melilotix. Microbiology 142, 601–610. doi: 10.1099/13500872-142-3-601
Torbaghan, M. E., Lakzian, A., Astaraei, A. R., Fotovat, A., and Besharati, H. (2017). Salt and alkali stresses reduction in wheat by plant growth promoting haloalkaliphilic bacteria. J. Soil Sci. Plant Nutr. 17, 1058–1087. doi: 10.4067/s0718-95162017000400016
Van Der Heijden, M. G., Martin, F. M., Selosse, M. A., and Sanders, I. R. (2015). Mycorrhizal ecology and evolution: the past, the present, and the future. New Phytol. 205, 1406–1423. doi: 10.1111/nph.13288
Von Uexküll, H., and Mutert, E. (1995). Global extent, development and economic impact of acid soils. Plant Soil 171, 1–15. doi: 10.1007/bf00009558
Walker, D. J., Clemente, R., Roig, A., and Bernal, M. P. (2003). The effects of soil amendments on heavy metal bioavailability in two contaminated Mediterranean soils. Environ. Pollut. 122, 303–312. doi: 10.1016/s0269-7491(02)00287-7
Wang, C., Cui, Y., and Qu, X. (2018). Mechanisms and improvement of acid resistance in lactic acid bacteria. Arch. Microbiol. 200, 195–201. doi: 10.1007/s00203-017-1446-2
Watkin, E. L., O’Hara, G. W., and Glenn, A. R. (2003). Physiological responses to acid stress of an acid-soil tolerant and an acid-soil sensitive strain of Rhizobium leguminosarum biovar trifolii. Soil Biol. Biochem. 35, 621–624. doi: 10.1016/s0038-0717(03)00012-9
Whipker, B. E., Bailey, D. A., Nelson, P. V., Fonteno, W. C., and Hammer, P. A. (1996). A novel approach to calculate acid additions for alkalinity control in greenhouse irrigation water. Commun. Soil Sci. Plant Anal. 27, 959–976. doi: 10.1080/00103629609369610
Williams, A., Kane, D. A., Ewing, P. M., Atwood, L. W., Jilling, A., Li, M., et al. (2016). Soil functional zone management: a vehicle for enhancing production and soil ecosystem services in row-crop agroecosystems. Front. Plant Sci. 7:65. doi: 10.3389/fpls.2016.00065
Xu, W., Jia, L., Shi, W., Baluška, F., Kronzucker, H. J., Liang, J., et al. (2013). The tomato 14-3-3 protein TFT4 modulates H+ efflux, basipetal auxin transport, and the PKS5-J3 pathway in the root growth response to alkaline stress. Plant Physiol. 163, 1817–1828. doi: 10.1104/pp.113.224758
Yan, F., Schubert, S., and Mengel, K. (1996). Soil pH changes during legume growth and application of plant material. Biol. Fertil. Soils 23, 236–242. doi: 10.1007/bf00335950
Yang, J. L., Zheng, S. J., He, Y. F., and Matsumoto, H. (2005). Aluminium resistance requires resistance to acid stress: a case study with spinach that exudes oxalate rapidly when exposed to Al stress. J. Exp. Bot. 56, 1197–1203. doi: 10.1093/jxb/eri113
Yano, K., and Takaki, M. (2005). Mycorrhizal alleviation of acid soil stress in the sweet potato (Ipomoea batatas). Soil Biol. Biochem. 37, 1569–1572. doi: 10.1016/j.soilbio.2005.01.010
Yazdani, M., Bahmanyar, M. A., Pirdashti, H., and Esmaili, M. A. (2009). Effect of phosphate solubilization microorganisms (PSM) and plant growth promoting rhizobacteria (PGPR) on yield and yield components of corn (Zea mays L.). World Acad. Sci. Eng. Technol. 49, 90–92.
Yirga, C., Erkossa, T., and Agegnehu, G. (2019). Soil Acidity Management. Addis Ababa: Ethiopian Institute of Agricultural Research.
Yordem, B. K., Conte, S. S., Ma, J. F., Yokosho, K., Vasques, K. A., Gopalsamy, S. N., et al. (2011). Brachypodium distachyon as a new model system for understanding iron homeostasis in grasses: phylogenetic and expression analysis of Yellow Stripe-Like (YSL) transporters. Ann. Bot. 108, 821–833. doi: 10.1093/aob/mcr200
Yousef, N. M. (2018). Capability of plant growth-promoting rhizobacteria (PGPR) for producing indole acetic acid (IAA) under extreme conditions. Eur. J. Biol. Res. 8, 174–182.
Zerrouk, I. Z., Benchabane, M., Khelifi, L., Yokawa, K., Ludwig-Müller, J., and Baluska, F. (2016). A Pseudomonas strain isolated from date-palm rhizospheres improves root growth and promotes root formation in maize exposed to salt and aluminum stress. J. Plant Physiol. 191, 111–119. doi: 10.1016/j.jplph.2015.12.009
Zhalnina, K., Dias, R., De Quadros, P. D., Davis-Richardson, A., Camargo, F. A., Clark, I. M., et al. (2015). Soil pH determines microbial diversity and composition in the park grass experiment. Microb. Ecol. 69, 395–406. doi: 10.1007/s00248-014-0530-2
Zhalnina, K., Louie, K. B., Hao, Z., Mansoori, N., Da Rocha, U. N., Shi, S., et al. (2018). Dynamic root exudate chemistry and microbial substrate preferences drive patterns in rhizosphere microbial community assembly. Nat. Microbiol. 3, 470–480. doi: 10.1038/s41564-018-0129-3
Zhang, J., Singh, D., Guo, C., Shang, Y., and Peng, S. (2020). Rhizobia at Extremes of Acidity, Alkalinity, Salinity, and Temperature. Microbial Versatility in Varied Environments. Berlin: Springer.
Zhang, P., Fu, J., and Hu, L. (2012). Effects of alkali stress on growth, free amino acids and carbohydrates metabolism in Kentucky bluegrass (Poa pratensis). Ecotoxicology 21, 1911–1918. doi: 10.1007/s10646-012-0924-1
Zhou, C., Zhu, L., Xie, Y., Li, F., Xiao, X., Ma, Z., et al. (2017). Bacillus licheniformis SA03 confers increased saline–alkaline tolerance in chrysanthemum plants by induction of abscisic acid accumulation. Front. Plant Sci. 8:1143. doi: 10.3389/fpls.2017.01143
Zhu, H.-H., Yao, Q., Sun, X.-T., and Hu, Y.-L. (2007). Colonization, ALP activity and plant growth promotion of native and exotic arbuscular mycorrhizal fungi at low pH. Soil Biol. Biochem. 39, 942–950.
Keywords: acidity, alkalinity, PGPM, soil pH, plant stress
Citation: Msimbira LA and Smith DL (2020) The Roles of Plant Growth Promoting Microbes in Enhancing Plant Tolerance to Acidity and Alkalinity Stresses. Front. Sustain. Food Syst. 4:106. doi: 10.3389/fsufs.2020.00106
Received: 20 May 2020; Accepted: 16 June 2020;
Published: 10 July 2020.
Edited by:
Everlon Cid Rigobelo, São Paulo State University, BrazilReviewed by:
Noemi Carla Baron Cozentino, São Paulo State University, BrazilSaddam Hussain, University of Agriculture, Faisalabad, Pakistan
Copyright © 2020 Msimbira and Smith. This is an open-access article distributed under the terms of the Creative Commons Attribution License (CC BY). The use, distribution or reproduction in other forums is permitted, provided the original author(s) and the copyright owner(s) are credited and that the original publication in this journal is cited, in accordance with accepted academic practice. No use, distribution or reproduction is permitted which does not comply with these terms.
*Correspondence: Donald L. Smith, donald.smith@mcgill.ca