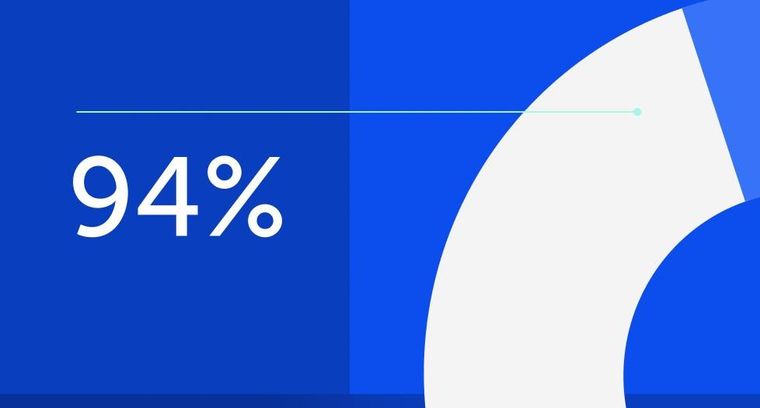
94% of researchers rate our articles as excellent or good
Learn more about the work of our research integrity team to safeguard the quality of each article we publish.
Find out more
MINI REVIEW article
Front. Sustain. Food Syst., 31 March 2020
Sec. Crop Biology and Sustainability
Volume 4 - 2020 | https://doi.org/10.3389/fsufs.2020.00021
In today's global market, some organic farmers must meet regulatory requirements to demonstrate that their plants and feedstocks are genetically modified organism (GMO)-free. Many GM plants are engineered to contain a promoter from the plant virus, Cauliflower mosaic virus (CaMV), in order to facilitate expression of an engineered target gene. The relative ubiquity of this CaMV 35S promoter (P35S) in GM constructs means that assays designed to detect GM plants often target the P35S DNA sequence, but these detection assays can yield false-positives from plants that are infected by naturally-occurring CaMV or its relatives within the Caulimoviridae. This review places CaMV infection and these ambiguous GM plant detection assays in context, serving as a resource for industry professionals, regulatory bodies, and researchers at the nexus of organic farming and global commerce. We first briefly introduce GM plants from a regulatory perspective, and then we describe CaMV biology, transmission, and management practices, highlighting the relatively widespread nature of CaMV infection in both GM and non-GM crops within the Brassicaceae and Solanaceae families. Finally, we discuss current knowledge of public food safety related to the consumption of CaMV-infected produce.
Organic agriculture, an ecological production management system that serves to promote and enhance biodiversity, biogeochemical cycles, and soil biological activity, has become increasingly popular, with global retail sales reaching more than $80 billion (Brantsæter et al., 2017; Mie et al., 2017). Organic agriculture relies on fertilizers of organic origin, such as compost, and encourages the use of biological pest control. It allows for the use of natural substances, such as pyrethrin and rotenone, while prohibiting most synthetic fertilizers and pesticides (some synthetic substances, such as copper sulfate and elemental sulfur, can be allowed). However, genetically modified organisms (GMOs) and plant growth regulators are prohibited in organic farming in regulated markets (Santhoshkumar et al., 2017).
A genetically modified organism (GMO) is any organism with genetic material that has been altered using genetic engineering techniques, and in the case of GM plants, a new trait is typically introduced to the plant. GM plants can confer advantages such as herbicide resistance, disease resistance, stress (e.g., drought) resistance, and/or insect tolerance (Oliver, 2014), yet GMO use in commercial crops and feedstocks is becoming regulated in some countries (Sharples, 1982; Maghari and Ardekani, 2011; Zhang et al., 2016; Tsatsakis et al., 2017). For example, many countries in the European Union (EU) have banned or imposed legislation to regulate GMOs (Davison, 2010; Lau, 2015; Wong and Chan, 2016). GMO regulation has impacts on international trade (Krueger and Le Buanec, 2008; Ramessar et al., 2008), and the popularity of organic foods, along with the tight regulations surrounding GMOs in some markets, have made GMO detection technologies essential for product labeling and traceability. Indeed, many countries have instituted labeling laws stating that products must be labeled when they contain GMOs above a certain threshold concentration (Wong and Chan, 2016). These thresholds can vary—for example, the threshold is 0.9% in the EU and 5% in Japan, whereas the U.S. has voluntary labeling, and China has “yes-or-no” labeling (Fu et al., 2015). In the U.S., a new law has just passed with mandatory labeling for some GMO products that will be effective in 2020.1
More than 80% of engineered genetic constructs in GM plants are built with the 35S promoter (P35S) from Cauliflower mosaic virus (CaMV) and/or the NOS terminator (TNOS) derived from the soil-borne bacterium, Agrobacterium tumefaciens (Figure 1A). Specifically, as of 2015, P35S and TNOS were used in 65.7 and 53.49%, respectively, of commercialized GMOs, with at least one of the two used in 81.4% of these constructs (Chaouachi et al., 2013; Wu et al., 2014; Fu et al., 2015). Thus, most GMO detection methods are based on marker sequences for P35S and TNOS detected through polymerase chain reaction (PCR) or quantitative PCR (qPCR) (Holden et al., 2010; Wu et al., 2014; Fraiture et al., 2015). However, it is known that CaMV infection of non-GM plants can yield false-positive results in some GMO detection assays, due to the presence of the P35S region in both the CaMV genome and many GMO constructs (Figures 1A,B; Wolf et al., 2000; Chaouachi et al., 2008; Becker and Ulrich, 2018). Although a detailed consideration of A. tumefaciens infections is beyond the scope of this mini-review, A. tumefaciens is naturally found in the soil and can infect plants, so assays designed to detect TNOS can yield false-positive GMO results too, as described elsewhere (Wolf et al., 2000; Escobar and Dandekar, 2003; Yang et al., 2013; Nabi et al., 2016; Becker and Ulrich, 2018).
Figure 1. Schematic representation of (A) a genetically modified (GM) plant construct and (B) the Cauliflower mosaic virus (CaMV) genome, highlighting the shared P35S promoter sequence. (A) In a GM plant, a gene conferring a specific trait (in blue) is inserted, and this transgene is expressed via the 35S promoter, P35S (in yellow) and the Nos terminator, TNOS (in orange). (B) The coding sequences of the seven CaMV genes (I-VII) encoding the seven proteins (Pl-P7) are represented with blue arrows. The CaMV 35S promoter (P35S) is shown in yellow and the 19S promoter (P19S) is shown in orange. P35S overlaps with the end of gene VI. Gene IV overlaps with the end of gene III and the beginning of gene V.
Here, we synthesize relevant information for navigating issues related to CaMV infection and false-positive GM plant detection due to CaMV infection, with particular relevance to sustainable organic farming and international trade of organic products in GMO-regulated markets. We outline CaMV biology and the impacts of CaMV infection on plant health and yield, recommended management practices for reducing CaMV infection of crop plants, available detection assays for GM plants with relevance to CaMV infection, and current knowledge of the safety of human consumption of CaMV in whole or in part.
Cauliflower mosaic virus (CaMV) belongs to the Caulimoviridae family of circular, double-stranded DNA viruses. It predominantly infects members of the Brassicaceae family, including radish, turnip, canola, mustard, cauliflower, broccoli, and cabbage. Some CaMV strains (D4 and W260) are also able to infect Solanaceae species, such as devil's trumpets (genus Datura) and tobacco plants (genus Nicotiana) (Scholelz and Shepherd, 1988). CaMV genetic variants have been described in different host species with different symptoms, virulence, and transmission rates (Covey et al., 1990; Al-Kaff and Covey, 1994; Doumayrou et al., 2013; Yasaka et al., 2014), and recent studies have identified a high diversity of CaMV genomic sequences (Farzadfar et al., 2014; Gong and Han, 2017; Becker and Ulrich, 2018; Sukal et al., 2018).
The CaMV genome consists of approximately 8,000 base-pairs of circular, double-stranded DNA. The genome encodes seven genes (gene I to gene VII), also called P1 to P7 for encoded proteins 1-7. CaMV replicates by reverse transcription (Haas et al., 2002), and its genes are transcribed from two promoters, the 19S and 35S promoters (Figure 1B), which are DNA sequences that define where transcription of a gene (or group of genes) begins. After entry into a plant host cell, the CaMV virus particle migrates to the nucleus, where the viral genome is separated from the viral particle (called decapsidation). The viral DNA is transcribed via the two promoters (P19S and P35S) into two long messenger RNAs (mRNAs), the 19S mRNA that encodes protein P6 and the 35S mRNA that encodes the other six proteins. Translation of the 19S mRNA results in the production of protein P6, which aggregates in numerous cytoplasmic virus factories (Harries et al., 2009; Angel et al., 2013; Rodriguez et al., 2014; Schoelz et al., 2016; Schoelz and Leisner, 2017), where the translation of other viral proteins will take place: the movement protein P1 (required for cell-to-cell movement), the helper component P2 (required for aphid transmission), the virus-associated protein P3, the coat protein P4, and the reverse transcriptase P5. Protein P7 has never been detected in planta and can be deleted by mutagenesis without any effect on virus infection or transmission (Dixon et al., 1986; Wurch et al., 1990).
CaMV is transmitted between host plants by more than 27 aphid species in a non-persistent and non-circulative manner (Kennedy et al., 1962), meaning that after an aphid acquires the virus from an infected plant, the virus does not circulate or replicate within the insect. The virus is retained for a short period (a few hours) in the aphid stylets (mouthparts), where CaMV receptor candidates have recently been identified (Bak et al., 2012, 2013a,b; Martinière et al., 2013; Webster et al., 2018). The virus can then be released to initiate a new infection during aphid feeding on healthy plants. There are no known cases of CaMV transmission via seeds.
CaMV is a widespread virus in temperate regions and can cause significant loss in Brassicaceae crops, especially in cases of co-infection with other viruses (Shepherd, 1981; Sutic et al., 1999; Spence et al., 2007; Li et al., 2019). CaMV incidence can easily exceed 70%, and subsequent yields may be reduced up to 20–50% (Shepherd, 1981; Sutic et al., 1999). CaMV can affect plant development, especially in early infections, and the production of flowers can be blocked. Low seed yields have also been reported from plants with CaMV infection (Sutic et al., 1999). The virus can induce a range of systemic symptoms, such as chlorosis (loss of green leaf color), mosaic (patches of light and dark green on leaves), vein clearing (abnormal clear or translucent color of veins), and/or stunting (Figure 2). CaMV survives in Brassicaceae crop and weed hosts, including wild radish, turnip weed, canola, mustard, cauliflower, broccoli and cabbage, and weed hosts are known reservoirs for the virus outside the growing season (Farzadfar et al., 2005). Although global GM crop regulations vary widely (see discussion above), canola is a good example of a common GM crop that can also be infected by CaMV. It has recently been shown that water stress can influence CaMV virulence and transmission: under well-watered conditions, viral load, virulence, and transmission rate increased, whereas under water deficit, transmission rate, and virulence decreased (Bergès et al., 2018).
Figure 2. CaMV symptoms on leaves. For each plant type, the left panel shows an uninfected leaf and the right panel shows a leaf from a CaMV-infected plant with typical symptoms, such as mosaic (mottling, e.g., lighter and/or darker green patches, puckered or curled leaves) or vein clearing (yellow or white veins). Top left: turnip (Brassica rapa), bottom left: watercress (Nasturtium officinale), right: canola (Brassica napus).
The best way to minimize CaMV infection is to inhibit aphid contact with seedlings, which are very susceptible to virus infection (Jenkinson and Glynne Jones, 1951; Farzadfar et al., 2005; Shah et al., 2015). Seedbeds can be isolated from aphids with a barrier of cereals or by growing the seedlings under insect-proof mesh (Jenkinson and Glynne Jones, 1951; Broadbent, 1957; Shah et al., 2015). A barrier of cereals may act as a sink for the viruses and/or as a physical barrier, such that aphids will be more likely to land on the tall cereals first and lose their virus contents while probing (Simons, 1957; Toba et al., 1977; Alegbejo and Uvah, 1986; Difonzo et al., 1996; Fereres, 2000). Still, non-persistently transmitted viruses are difficult to control, since the insects only need to feed on the plant briefly to release viruses (Fereres, 2000; Bak et al., 2019), and pesticides are usually not an effective solution because aphids can transmit viruses before the pesticide has an effect (Simons, 1957; Jayasena and Randles, 1985).
CaMV can also be transmitted mechanically by sap inoculation using contaminated hands and pruning tools (Yasaka et al., 2014). It has been shown that CaMV can stay for hours on surfaces such as doors, phones, and gloves, and can be exchanged by hand-shaking (Jiang et al., 1998; Dancer, 2014), so disinfecting tools, equipment, and anything that contacts plants will reduce infection. Though it did not include CaMV, a study that tested how to prevent propagation of plant viruses, such as Pepino mosaic virus (PepMV), Potato spindle tuber viroid (PSTVd), Tomato mosaic virus (ToMV), and Tobacco mosaic virus (TMV), examined sixteen commercially available disinfectants and found that 10% bleach is the most effective solution for preventing viral infection of plants in greenhouse facilities (Li et al., 2015).
Many GM plant detection assays target the 35S promoter (P35S) and/or the terminator NOS (TNOS). Polymerase chain reaction (PCR) was the first technique applied to GM plant detection, and it was mainly used as a fast (~2 h) and low-cost method (Gachet et al., 1998; Iloh et al., 2018; Grohmann et al., 2019). Real-time PCR, also known as qPCR, was developed later and became the preferred technique, providing both qualitative and quantitative results by measuring both the presence and concentration of gene sequences in a given sample (Akiyama et al., 2009; Holden et al., 2010; Wu et al., 2014). Both PCR and qPCR have been reviewed extensively elsewhere (e.g., VanGuilder et al., 2008; Emerson et al., 2017). In the context of GM plant detection, both are used to identify specific genetic regions of interest, based on the hybridization of primers to conserved DNA (or, in some cases, RNA) regions flanking the genetic sequence to be identified, followed by the amplification of the sequence of interest by a polymerase enzyme. In the case of PCR, the result is typically binary (detection or non-detection) and can be visualized by gel electrophoresis. More quantitative results can be attained by qPCR, including the concentration and/or number of copies of a specific genetic region, which can be useful if a threshold allowable concentration has been set by a regulatory agency.
Multiplex qPCR can allow for amplification of several DNA targets at the same time, e.g., for the simultaneous detection of multiple GMO markers in a single reaction (Akiyama et al., 2009; Singh et al., 2016; Cottenet et al., 2019). For example, in Cottenet et al. (2019), the authors developed a new GMO screening method based on two multiplex real-time PCR reactions, targeting six major GM markers in one reaction and six other GM events in another reaction. The method showed a broad screening capacity, due to the large number of targets, and the limit of detection ranged from 0.005 and 0.02% (Cottenet et al., 2019). However, the same fluorophore was used for all markers, so detection was based on the presence or absence of signal with no ability to distinguish among the markers. To distinguish among markers in the same reaction, a high level of multiplexing can be achieved using qPCR with different fluorophores for each target; depending on the instrument, up to five distinguishable targets have been successfully amplified in a single multiplexed qPCR reaction (Reller et al., 2013; Datukishvili et al., 2015). For example, we recently developed a multiplex qPCR assay that can distinguish CaMV infection from GM plants containing P35S in a single reaction, based on detection of four different targets: P35S, CaMV gene III, TNOS, and actin (a universal plant gene used as a positive control for the assay) (Bak and Emerson, 2019).
Given the increasing number and diversity of GMOs developed and the number of molecular biology technologies available in addition to PCR and qPCR, many different GMO detection methods have been developed (Broeders et al., 2012; Fraiture et al., 2015; Demeke and Dobnik, 2018) (for more information, see Supplementary Table 1 and references therein). Databases of GMO sequences and detection assays have also been compiled, facilitating the identification of an appropriate detection method or the development of a new technique for a given need (Dong et al., 2008; Petrillo et al., 2015; Wilkes et al., 2017). To date, there is no international standardized GMO or CaMV detection method, but PCR and qPCR (most commonly targeting P35S and/or TNOS) are still the most commonly used (Grohmann et al., 2019). From a regulatory standpoint, detection thresholds are typically evoked for various requirements. For example, in the European Union, detection of >0.9% GMO content requires a GMO label, but for specific GMOs that are not allowed in the EU under any circumstances, GMO contents must be 0%, which, practically speaking, means below the limit of detection of the assay (Davison, 2010). Thus, for regulatory purposes, the chosen GMO detection method must be sensitive enough to detect a GMO at the required threshold concentration(s), and qPCR is the most widely used method that provides this quantitative information.
Public concerns exist regarding whether plant viruses can infect humans, and a number of studies have attempted to answer this question (Bawa and Anilakumar, 2012; Rastogi Verma, 2013; Wunderlich and Gatto, 2015). Although a definitive, universal answer is not possible, here we point to existing literature for insights. Numerous viruses infect plants and are consumed through various types of fresh food and food products (Balique et al., 2015), and two widespread plant viruses (Pepper mild mottle virus, PMMoV, and tobacco mosaic virus, TMV) are the most comprehensively studied, in terms of their effects on human consumption. To the extent that human consumption of CaMV has been studied, we will first present results specific to CaMV, and then we will consider human consumption of PMMoV and TMV.
CaMV commonly and widely infects crucifers, also known as cole crops (cabbage, kale, cauliflower, broccoli, and mustard), and it has been reported that 10% of the cabbages and cauliflowers on sale in supermarkets are infected with CaMV (Raafat El–Gewely, 2001). In an epidemiological study of cauliflower in England, it was shown that 50% of the plants were infected with CaMV (Gong and Han, 2017), yet no ill effects or evidence of human pathogenicity have been found resulting from CaMV-infected plants (Hull et al., 2000). In terms of consumption of parts of CaMV, e.g., the P35S promoter in GM plants, some concerns have been raised, but to our knowledge, there are no scientific publications that have demonstrated a safety risk of eating CaMV, P35S, or any CaMV genetic sequences. In fact, many plants already contain sequences from members of the Caulimoviridae in their genomes as endogenous viral elements (EVEs), including commonly consumed crops, such as Vitis vinifera (grape), Oryza sativa (rice), and a variety of Citrus species (e.g., Citrus clementina, clementines) (Bertsch et al., 2009; Geering et al., 2014), meaning that we have been consuming parts of viruses in our food for centuries.
Still, we review some of the CaMV- and 35S promoter-specific concerns here for context. Sequence overlap exists between P35S and the coding sequences of one CaMV gene, gene VI, also known as P6 (Figure 1), indicating the potential for virus protein expression in humans (Podevin and Du Jardin, 2012). Another concern is the horizontal gene transfer risk with DNA recombination that can potentially occur between P35S and human genes, along with the potential for P35S to facilitate expression of human genes (Chiter et al., 2000; Morel and Tepfer, 2000; Nielsen and Townsend, 2004; Paparini and Romano-Spica, 2006; Bawa and Anilakumar, 2012). In two studies that have attempted to address these concerns, no evidence was observed for the activity of the P35S promoter in mammalian cells (Vlasák et al., 2003; Paparini and Romano-Spica, 2006). In terms of the potential for viruses to recombine with and acquire genes from transgenic plants (i.e., horizontal gene transfer from transgenic plants to a virus), earlier studies of CaMV have demonstrated that this is possible (Schoelz and Wintermantel, 1993; Wintermantel and Schoelz, 1996), but only under very specific conditions, such as strong selection pressure in the laboratory (Wintermantel and Schoelz, 1996).
PMMoV is a widespread plant virus that infects pepper and is found in numerous products containing chilis and peppers (sauce, spicy powder, etc.). For example, PMMoV was found in 57% of 28 pepper-based foods found in supermarkets (Colson et al., 2010). Colson et al. found that humans who consumed PMMoV presented a specific immune response to the virus, including fever and abdominal pain symptoms (Colson et al., 2010). This observation may not be the infection of human cells by the virus itself, but rather viral RNA interfering with the function of human RNA. Tobacco mosaic virus (TMV) is often present in smoked tobacco and, therefore, it is resistant to manufacturing processes (Smith, 1957; Wetter, 1975; Wahyuni et al., 2008). A study found that TMV was viable in 53% of the cigarettes of six different brands (Balique et al., 2012). In addition, 45% of the saliva from 12 smokers, compared to 0% of the saliva from 15 non-smokers, tested positive for TMV RNA (Liu et al., 2013). Another study found that exposure to TMV-infected tobacco products can induce an immune response to TMV in humans; specifically, using an ELISA assay, the authors found anti-TMV antibodies in tobacco smokers (Kamthan et al., 2016). Nevertheless, there was no direct evidence of viral infection in any of these studies, and the symptoms described seem to be an indirect response to the viruses. The extent to which these results for PMMoV and TMV are relevant to CaMV is largely unknown (for example, both PMMoV and TMV have RNA genomes, whereas CaMV is a DNA virus), but these cases demonstrate the potential for some minor symptoms associated with an autoimmune response to plant viruses in some cases. In general, plant viruses are not considered to present pathogenicity to humans (Balique et al., 2015).
In parallel with the development of new GMOs in agriculture, organic farming is increasing (Shi-ming and Sauerborn, 2006; Kamthan et al., 2016). As a result of these farming trends and, in some countries, regulation of GMOs, international trade can necessitate quantification of the GMO content of a given product. Thus, numerous GMO detection methods are emerging (Kamle et al., 2017; Salisu et al., 2017; Umesha and Manukumar, 2018), but the potential for crop infection with CaMV can lead to false-positive results in the most commonly used GMO detection assays, due to the use of a CaMV-derived P35S promoter in many GM constructs (Lipp et al., 1999; Becker and Ulrich, 2018; Lübeck, 2019). This potential for false-positive GMO detection is particularly relevant for organic farmers trying to meet regulatory requirements for non-GM plants in a sustainable international market. Here we have provided information about GMO detection assays and how to disambiguate GMO detection from CaMV infection (e.g., by considering detection targets in the CaMV genome outside the P35S region, such as gene III), management practices to minimize CaMV infection (e.g., planting tall cereals around Brassicaceae crops and adding protective aphid-proof netting around seedlings), and our current understanding of the food safety risk associated with CaMV infection (CaMV is not currently known to pose a risk to humans, but specific studies related to CaMV impacts on human health are limited).
JE conceived of the article. AB performed the literature research and prepared the figures. AB and JE wrote and edited the article. All authors have approved of the final version of the manuscript.
We gratefully acknowledge Amway for funding this work in the form of salary support for AB from a grant awarded to JE.
We have no conflicts of interest to report. In the interest of full transparency, this work was funded by Nutrilite by Amway from a grant awarded to JE. This synthesis article was developed solely by JE and AB, who provided periodic progress reports to Nutrilite by Amway via conference calls. The funding body played no part in directing the research.
The Supplementary Material for this article can be found online at: https://www.frontiersin.org/articles/10.3389/fsufs.2020.00021/full#supplementary-material
Akiyama, H., Nakamura, F., Yamada, C., Nakamura, K., Nakajima, O., Kawakami, H., et al. (2009). A screening method for the detection of the 35S promoter and the nopaline synthase terminator in genetically modified organisms in a real-time multiplex polymerase chain reaction using high-resolution melting-curve analysis. Biol. Pharm. Bull. 32, 1824–1829. doi: 10.1248/bpb.32.1824
Alegbejo, M. D., and Uvah, I. (1986). Effect of intercropping pepper with tall crops on the incidence of pepper veinal mottle virus disease on pepper. Nigerian J. Entomol. 7, 82–87.
Al-Kaff, N., and Covey, S. N. (1994). Variation in biological properties of Cauliflower mosaic virus clones. J. Virol. 75, 3137–3145. doi: 10.1099/0022-1317-75-11-3137
Angel, C. A., Lutz, L., Yang, X., Rodriguez, A., Adair, A., Zhang, Y., et al. (2013). The P6 protein of Cauliflower mosaic virus interacts with CHUP1, a plant protein which moves chloroplasts on actin microfilaments. Virology 443, 363–374. doi: 10.1016/j.virol.2013.05.028
Bak, A., and Emerson, J. B. (2019). Multiplex quantitative PCR for single-reaction genetically modified (GM) plant detection and identification of false-positive GM plants linked to Cauliflower mosaic virus (CaMV) infection. BMC Biotechnol. 19:73. doi: 10.1186/s12896-019-0571-1
Bak, A., Gargani, D., Macia, J. L., Malouvet, E., Vernerey, M. S., Blanc, S., et al. (2013a). Virus factories of Cauliflower mosaic virus are virion reservoirs that engage actively in vector transmission. J. Virol. 87, 12207–12215. doi: 10.1128/JVI.01883-13
Bak, A., Irons, S. L., Martinière, A., Blanc, S., and Drucker, M. (2012). Host cell processes to accomplish mechanical and non-circulative virus transmission. Protoplasma 249, 529–539. doi: 10.1007/s00709-011-0328-8
Bak, A., Martinière, A., Blanc, S., and Drucker, M. (2013b). Early interactions during the encounter of plants, aphids and arboviruses. Plant Signal. Behav. 8:e24225. doi: 10.4161/psb.24225
Bak, A., Patton, M. F., Perilla-Henao, L. M., Aegerter, B. J., and Casteel, C. L. (2019). Ethylene signaling mediates Potyvirus spread by aphid vector in potato. Oecologia 190, 139–148. doi: 10.1007/s00442-019-04405-0
Balique, F., Colson, P., and Raoult, D. (2012). Tobacco mosaic virus in cigarettes and saliva of smokers. J. Clin. Virol. 55, 374–376. doi: 10.1016/j.jcv.2012.08.012
Balique, F., Lecoq, H., Raoult, D., and Colson, P. (2015). Can plant viruses cross the kingdom border and be pathogenic to humans? Viruses 7, 2074–2098. doi: 10.3390/v7042074
Bawa, A. S., and Anilakumar, K. R. (2012). Genetically modified foods: safety, risks and public concerns–a review. J. Food Sci. Technol. 50, 1035–1046. doi: 10.1007/s13197-012-0899-1
Becker, R., and Ulrich, A. (2018). Improved detection and quantification of Cauliflower mosaic virus in food crops: assessing false positives in GMO screening based on the 35S promoter. Eur. Food Res. Technol. 244, 1861–1871. doi: 10.1007/s00217-018-3099-z
Bergès, S. E., Vile, D., Vazquez-Rovere, C., Blanc, S., Yvon, M., Bédiée, A., et al. (2018). Interactions between drought and plant genotype change epidemiological traits of Cauliflower mosaic virus. Front Plant Sci. 9:703. doi: 10.3389/fpls.2018.00703
Bertsch, C., Beuve, M., Dolja, V. V., Wirth, M., Pelsy, F., Herrbach, E., et al. (2009). Retention of the virus-derived sequences in the nuclear genome of grapevine as a potential pathway to virus resistance. Biol. Direct. 4:21. doi: 10.1186/1745-6150-4-21
Brantsæter, A. L., Ydersbond, T. A., Hoppin, J. A., Haugen, M., and Meltzer, H. M. (2017). Organic food in the diet: exposure and health implications. Annu. Rev. Public Health 38, 295–313. doi: 10.1146/annurev-publhealth-031816-044437
Broadbent, L. (1957). Investigation of Virus Diseases of Brassica Crops Cambridge. Cambridge: Cambridge University Press. doi: 10.2307/1292322
Broeders, S., Papazova, N., Van den Eede, G., and Roosens, N. (2012). “Development of a molecular platform for GMO detection in food and feed on the basis of “combinatory qPCR” technology,” in Polymerase Chain Reaction ed P. Hernandez-Rodriguez (Rijeka: IntechOpen) 377–381. doi: 10.5772/37898
Chaouachi, M., Fortabat, M., Geldreich, A., Yot, P., Kerlan, C., Kebdani, N., et al. (2008). An accurate real-time PCR test for the detection and quantification of Cauliflower mosaic virus (CaMV): applicable in GMO screening. Eur. Food Res. Technol. 227, 789–798. doi: 10.1007/s00217-007-0787-5
Chaouachi, M., Nabi, N., Hafsa, A. B., Zellama, M. S., Skhiri, F., and Saïd, K. (2013). Monitoring of genetically modified food and feed in the Tunisian market using qualitative and quantitative real-time PCR. Food Sci. Biotechnol. 22, 1161–1170. doi: 10.1007/s10068-013-0198-2
Chiter, A., Forbes, J. M., and Blair, G. E. (2000). DNA stability in plant tissues: implications for the possible transfer of genes from genetically modified food. FEBS Lett. 481, 164–168. doi: 10.1016/S0014-5793(00)01986-4
Colson, P., Richet, H., Desnues, C., Balique, F., Moal, V., Grob, J.-J., et al. (2010). Pepper mild mottle virus, a plant virus associated with specific immune responses, fever, abdominal pains, and pruritus in humans. PLoS ONE 5:e10041. doi: 10.1371/journal.pone.0010041
Cottenet, G., Blancpain, C., Sonnard, V., and Chuah, P. F. (2019). Two FAST multiplex real-time PCR reactions to assess the presence of genetically modified organisms in food. Food Chem. 274, 760–765. doi: 10.1016/j.foodchem.2018.09.050
Covey, S. N., Turner, D. S., Lucy, A. P., and Saunders, K. (1990). Host regulation of the Cauliflower mosaic virus multiplication cycle. Proc. Natl. Acad. Sci. U.S.A. 87, 1633–1637. doi: 10.1073/pnas.87.5.1633
Dancer, S. J. (2014). “Cleaning and decontamination of the healthcare environment,” in Decontamination in Hospitals and Healthcare, ed J. T. Walker (Lanarkshire: Woodhead Publishing), 370–397. doi: 10.1533/9780857096692.2.370
Datukishvili, N., Kutateladze, T., Gabriadze, I., Bitskinashvili, K., and Vishnepolsky, B. (2015). New multiplex PCR methods for rapid screening of genetically modified organisms in foods. Front. Microbiol. 6:757. doi: 10.3389/fmicb.2015.00757
Davison, J. (2010). GM plants: science, politics and EC regulations. Plant Sci. 178, 94–98. doi: 10.1016/j.plantsci.2009.12.005
Demeke, T., and Dobnik, D. (2018). Critical assessment of digital PCR for the detection and quantification of genetically modified organisms. Anal. Bioanal. Chem. 410, 4039–4050. doi: 10.1007/s00216-018-1010-1
Difonzo, C. D., Ragsdale, D. W., Radcliffe, E. B., Gudmestad, N. C., and Secor, G. A. (1996). Crop borders reduce potato virus Y incidence in seed potato. Ann. Appl. Biol. 129, 289–302. doi: 10.1111/j.1744-7348.1996.tb05752.x
Dixon, L., Jiricny, J., and Hohn, T. (1986). Oligonucleotide directed mutagenesis of Cauliflower mosaic virus DNA using a repair-resistant nucleoside analogue: identification of an agnogene initiation codon. Gene 41, 225–231. doi: 10.1016/0378-1119(86)90102-2
Dong, W., Yang, L., Shen, K., Kim, B., Kleter, G. A., Marvin, H. J., et al. (2008). GMDD: a database of GMO detection methods. BMC Bioinformatics 9:260. doi: 10.1186/1471-2105-9-260
Doumayrou, J., Avellan, A., Froissart, R., and Michalakis, Y. (2013). An experimental test of the transmission-virulence trade-off hypothesis in a plant virus. Evolution 67, 477–486. doi: 10.1111/j.1558-5646.2012.01780.x
Emerson, J. B., Adams, R. I., Román, C. M. B., Brooks, B., Coil, D. A., Dahlhausen, K., et al. (2017). Schrödinger's microbes: tools for distinguishing the living from the dead in microbial ecosystems. Microbiome 5:86. doi: 10.1186/s40168-017-0285-3
Escobar, M. A., and Dandekar, A. M. (2003). Agrobacterium tumefaciens as an agent of disease. Trends Plant Sci. 8, 380–386. doi: 10.1016/S1360-1385(03)00162-6
Farzadfar, S., Pourrahim, R., and Ebrahimi, H. (2014). A phylogeographical study of the Cauliflower mosaic virus population in mid-Eurasia Iran using complete genome analysis. Arch. Virol. 159, 1329–1340. doi: 10.1007/s00705-013-1910-5
Farzadfar, S., Pourrahim, R., Golnaraghi, A., and Ahoonmanesh, A. (2005). Occurrence of Cauliflower mosaic virus in different cruciferous plants in Iran. Plant Pathol. 54, 810–810. doi: 10.1111/j.1365-3059.2005.01224.x
Fereres, A. (2000). Barrier crops as a cultural control measure of non-persistently transmitted aphid-borne viruses. Virus Res. 71, 221–231. doi: 10.1016/S0168-1702(00)00200-8
Fraiture, M. A., Herman, P., Taverniers, I., De Loose, M., Deforce, D., and Roosens, N. H. (2015). Current and new approaches in GMO detection: challenges and solutions. Biomed Res. Int. 2015:392872. doi: 10.1155/2015/392872
Fu, W., Zhu, P., Wang, C., Huang, K., Du, Z., Tian, W., et al. (2015). A highly sensitive and specific method for the screening detection of genetically modified organisms based on digital PCR without pretreatment. Sci. Rep. 5:12715. doi: 10.1038/srep12715
Gachet, E., Martin, G. G., Vigneau, F., and Meyer, G. (1998). Detection of genetically modified organisms (GMOs) by PCR: a brief review of methodologies available. Trends Food Sci. Technol. 9, 380–388. doi: 10.1016/S0924-2244(99)00002-3
Geering, A. D. W., Maumus, F., Copetti, D., Choisne, N., Zwickl, D. J., Zytnicki, M., et al. (2014). Endogenous florendoviruses are major components of plant genomes and hallmarks of virus evolution. Nat. Commun. 5:5269. doi: 10.1038/ncomms6269
Gong, Z., and Han, G.-Z. (2017). Hidden diversity and macroevolutionary mode of Caulimoviridae uncovered by euphyllophyte paleoviruses. bioRxiv 170415. doi: 10.1101/170415
Grohmann, L., Keilwagen, J., Duensing, N., Dagand, E., Hartung, F., Wilhelm, R., et al. (2019). Detection and identification of genome editing in plants: challenges and opportunities. Front. Plant Sci. 10:236. doi: 10.3389/fpls.2019.00236
Haas, M., Bureau, M., Geldreich, A., Yot, P., and Keller, M. (2002). Cauliflower mosaic virus: still in the news. Mol. Plant Pathol. 3, 419–429. doi: 10.1046/j.1364-3703.2002.00136.x
Harries, P. A., Palanichelvam, K., Yu, W., Schoelz, J. E., and Nelson, R. S. (2009). The Cauliflower mosaic virus protein P6 forms motile inclusions that traffic along actin microfilaments and stabilize microtubules. Plant Physiol. 149, 1005–1016. doi: 10.1104/pp.108.131755
Holden, M. J., Levine, M., Scholdberg, T., Haynes, R. J., and Jenkins, G. R. (2010). The use of 35S and Tnos expression elements in the measurement of genetically engineered plant materials. Anal. Bioanal. Chem. 396, 2175–2187. doi: 10.1007/s00216-009-3186-x
Hull, R., Covey, S. N., and Dale, P. (2000). Genetically modified plants and the 35S promoter: assessing the risk and enhancing the debate. Microb. Ecol. Health Dis. 12, 1–5. doi: 10.3402/mehd.v12i1.8034
Iloh, A., Onyenekwe, P., and Ojo, O. (2018). Detection of genetically modified DNA in processed maize and soybean products in Nigeria. Afr. J. Biotechnol. 17, 1091–1094. doi: 10.5897/AJB2018.16479
Jayasena, K. W., and Randles, J. W. (1985). The effect of insecticides and a plant barrier row on aphid populations and the spread of bean yellow mosaic potyvirus and subterranean clover red leaf luteovirus in Vicia faba in Australia. Ann. Appl. Biol. 107, 355–364. doi: 10.1111/j.1744-7348.1985.tb03152.x
Jenkinson, J. G., and Glynne Jones, G. D. (1951). Control of Cauliflower mosaic virus. Nature 168, 336–337. doi: 10.1038/168336b0
Jiang, X., Dai, X., Goldblatt, S., Buescher, C., Cusack, T. M., Matson, D. O., et al. (1998). Pathogen transmission in child care settings studied by using a cauliflower virus DNA as a surrogate marker. J. Infect. Dis. 177, 881–888. doi: 10.1086/515253
Kamle, M., Kumar, P., Patra, J. K., and Bajpai, V. K. (2017). Current perspectives on genetically modified crops and detection methods. Biotech 7:219. doi: 10.1007/s13205-017-0809-3
Kamthan, A., Chaudhuri, A., Kamthan, M., and Datta, A. (2016). Genetically modified (GM) crops: milestones and new advances in crop improvement. Theor. Appl. Genet. 129, 1639–1655. doi: 10.1007/s00122-016-2747-6
Kennedy, J. S., Day, M. F., and Eastop, V. F. (1962). A Conspectus of Aphids as Vectors of Plant Viruses. London: Commonwealth Institute of Entomology.
Krueger, R., and Le Buanec, B. (2008). Action needed to harmonize regulation of low–level presence of biotech traits. Nat. Biotechnol. 26, 161–162. doi: 10.1038/nbt0208-161
Lau, J. (2015). Same Science, Different Policies: Regulating Genetically Modified Foods in the US and Europe. Science in the News Harvard University. Available online at: http://sitn.hms.harvard.edu/flash/2015/same-science-different-policies (accessed May 15, 2019).
Li, G., Lv, H., Zhang, S., Zhang, S., Li, F., Zhang, H., et al. (2019). TuMV management for brassica crops through host resistance: retrospect and prospects. Plant Pathol. 68, 1035–1044. doi: 10.1111/ppa.13016
Li, R., Baysal-Gurel, F., Abdo, Z., Miller, S. A., and Ling, K. S. (2015). Evaluation of disinfectants to prevent mechanical transmission of viruses and a viroid in greenhouse tomato production. Virol. J 12:5. doi: 10.1186/s12985-014-0237-5
Lipp, M., Brodmann, P., Pietsch, K., Pauwels, J., Anklam, E., Börchers, T., et al. (1999). IUPAC collaborative trial study of a method to detect genetically modified soy beans and maize in dried powder. JAOAC Int. 82, 923–928. doi: 10.1093/jaoac/82.4.923
Liu, R., Vaishnav, R. A., Roberts, A. M., and Friedland, R. P. (2013). Humans have antibodies against a plant virus: evidence from tobacco mosaic virus. PLoS ONE 8:e60621. doi: 10.1371/journal.pone.0060621
Lübeck, M. (2019). Detection of genetically modified plants - methods to sample and analyse GMO content in plants and plant products. Available online at: https://naturstyrelsen.dk/media/nst/Attachments/REPORT_rev_maj.pdf (accessed May 30, 2019).
Maghari, B. M., and Ardekani, A. M. (2011). Genetically modified foods and social concerns. Avicenna J. Med. Biotechnol. 3, 109–117.
Martinière, A., Bak, A., Macia, J. L., Lautredou, N., Gargani, D., Doumayrou, J., et al. (2013). A virus responds instantly to the presence of the vector on the host and forms transmission morphs. eLife 2:e00183. doi: 10.7554/eLife.00183
Mie, A., Andersen, H. R., Gunnarsson, S., Kahl, J., Kesse-Guyot, E., Rembiałkowska, E., et al. (2017). Human health implications of organic food and organic agriculture: a comprehensive review. Environ. Health 16:111. doi: 10.1186/s12940-017-0315-4
Morel, J. B., and Tepfer, M. (2000). Are there potential risks associated with use of the Cauliflower mosaic virus 35S promoter in transgenic plants? Biofutur 201, 32–35. doi: 10.1016/S0294-3506(00)80076-9
Nabi, N., Chaouachi, M., Zellama, M. S., Ben Hafsa, A., Mrabet, B., Saïd, K., et al. (2016). A new QRT-PCR assay designed for the differentiation between elements provided from Agrobacterium sp. in GMOs plant events and natural Agrobacterium sp. bacteria. Food Chem. 196, 58–65. doi: 10.1016/j.foodchem.2015.09.015
Nielsen, K. M., and Townsend, J. P. (2004). Monitoring and modeling horizontal gene transfer. Nat. Biotechnol. 22, 1110–1114. doi: 10.1038/nbt1006
Paparini, A., and Romano-Spica, V. (2006). Gene transfer and Cauliflower mosaic virus promoter 35S activity in mammalian cells. J. Environ. Sci. Health. B 41, 437–449. doi: 10.1080/03601230600616957
Petrillo, M., Angers-Loustau, A., Henriksson, P., Bonfini, L., Patak, A., and Kreysa, J. (2015). JRC GMO-Amplicons: a collection of nucleic acid sequences related to genetically modified organisms. Database 2015:bav101. doi: 10.1093/database/bav101
Podevin, N., and Du Jardin, P. (2012). Possible consequences of the overlap between the CaMV 35S promoter regions in plant transformation vectors used and the viral gene VI in transgenic plants. GM Crops Food 3, 296–300. doi: 10.4161/gmcr.21406
Ramessar, K., Capell, T., Twyman, R. M., Quemada, H., and Christou, P. (2008). Trace and traceability - a call for regulatory harmony. Nat. Biotechnol. 26, 975–978. doi: 10.1038/nbt0908-975
Rastogi Verma, S. (2013). Genetically modified plants: public and scientific perceptions. ISRN Biotechnol. 2013:820671. doi: 10.5402/2013/820671
Reller, M. E., Chen, W. H., Dalton, J., Lichay, M. A., and Dumler, J. S. (2013). Multiplex 5′ nuclease quantitative Real–time PCR for clinical diagnosis of malaria and species-level identification and epidemiologic evaluation of malaria-causing parasites, including Plasmodium knowlesi. J. Clin. Microbiol. 51, 2931–2938. doi: 10.1128/JCM.00958-13
Rodriguez, A., Angel, C. A., Lutz, L., Leisner, S. M., Nelson, R. S., and Schoelz, J. E. (2014). Association of the P6 protein of Cauliflower mosaic virus with plasmodesmata and plasmodesmal proteins. Plant Physiol. 166, 1345–1358. doi: 10.1104/pp.114.249250
Salisu, I. B., Shahid, A. A., Yaqoob, A., Ali, Q., Bajwa, K. S., Rao, A. Q., et al. (2017). Molecular approaches for high throughput detection and quantification of genetically modified crops: a review. Front. Plant Sci. 8:1670. doi: 10.3389/fpls.2017.01670
Santhoshkumar, M., Reddy, G. C., and Sangwan, P. S. (2017). A review on organic farming - Sustainable agriculture development. Int. J. Pure App. Biosci. 5, 1277–1282. doi: 10.18782/2320-7051.5649
Schoelz, J. E., Angel, C. A., Nelson, R. S., and Leisner, S. M. (2016). A model for intracellular movement of Cauliflower mosaic virus: the concept of the mobile virion factory. J. Exp. Bot. 67, 2039–2048. doi: 10.1093/jxb/erv520
Schoelz, J. E., and Leisner, S. (2017). Setting up shop: the formation and function of the viral factories of Cauliflower mosaic virus. Front. Plant Sci. 30:1832. doi: 10.3389/fpls.2017.01832
Schoelz, J. E., and Wintermantel, W. M. (1993). Expansion of viral host range through complementation and recombination in transgenic plants. Plant Cell 5, 1669–1679. doi: 10.2307/3869748
Scholelz, J. E., and Shepherd, R. J. (1988). Host range control of Cauliflower mosaic virus. Virology 162, 30–37. doi: 10.1016/0042-6822(88)90391-1
Shah, M. A., Khan, A. A., Junaid, J. M., Somina, M., and Mohi-ud-din, S. (2015). “Chapter 27: Aphid vectored viral diseases and their management,” Insect Pests Management of Fruit Crops, eds A. K. Pandey and P. Mall (Darya Ganj: Biotech Books®), 511–554.
Sharples, F. E. (1982). Spread of organisms with novel genotypes: thoughts from an ecological perspective. Recomb. DNA Tech. Bull. 6, 43–56.
Shepherd, R. J. (1981). Cauliflower mosaic virus AAB Descriptions of Plant Viruses No 243. Wellesbourne: Association of Applied Biologists.
Shi-ming, M. A., and Sauerborn, J. (2006). Review of history and recent development of organic farming worldwide. Agric. Sci. China 5, 169–178. doi: 10.1016/S1671-2927(06)60035-7
Simons, J. (1957). Effects of insecticides and physical barriers on field spread of pepper veinbanding mosaic virus. Phytopathology 47, 139–145.
Singh, M., Bhoge, R. K., and Randhawa, G. (2016). Crop-specific GMO matrix-multiplex PCR: a cost-efficient screening strategy for genetically modified maize and cotton events approved globally. Food Control 70, 271–280. doi: 10.1016/j.foodcont.2016.05.032
Spence, N. J., Phiri, N. A., Hughes, S. L., Mwaniki, A., Simons, S., Oduor, G., et al. (2007). Economic impact of Turnip mosaic virus, Cauliflower mosaic virus and Beet mosaic virus in three Kenyan vegetables. Plant Pathol. 56, 317–323. doi: 10.1111/j.1365-3059.2006.01498.x
Sukal, A. C., Kidanemariam, D. B., Dale, J. L., Harding, R. M., and James, A. P. (2018). Characterization of a novel member of the family Caulimoviridae infecting Dioscorea nummularia in the Pacific, which may represent a new genus of dsDNA plant viruses. PLoS ONE 13:e0203038. doi: 10.1371/journal.pone.0203038
Sutic, D. D., Ford, R. E., and Tosic, M. T. (1999). Handbook of Plant Virus Diseases. Boca Raton, FL: CRC Press.
Toba, H. H., Kishaba, A. N., Bohn, G. W., and Hield, H. (1977). Protecting muskmelons against aphid-borne viruses. Phytopathology 67, 1418–1423. doi: 10.1094/Phyto-67-1418
Tsatsakis, A. M., Nawaz, M. A., Kouretas, D., Balias, G., Savolainen, K., Tutelyan, V. A., et al. (2017). Environmental impacts of genetically modified plants: a review. Environ. Res. 156, 818–833. doi: 10.1016/j.envres.2017.03.011
Umesha, S., and Manukumar, H. M. (2018). Advanced molecular diagnostic techniques for detection of food-borne pathogens: current applications and future challenges. Crit. Rev. Food Sci. Nutr. 58, 84–104. doi: 10.1080/10408398.2015.1126701
VanGuilder, H. D., Vrana, K. E., and Freeman, W. M. (2008). Twenty-five years of quantitative PCR for gene expression analysis. BioTechniques 44, 619–626. doi: 10.2144/000112776
Vlasák, J., Smahel, M., Pavlík, A., Pavingerová, D., and Bríza, J. (2003). Comparison of hCMV immediate early and CaMV 35S promoters in both plant and human cells. J. Biotechnol. 103, 197–202. doi: 10.1016/S0168-1656(03)00124-X
Wahyuni, W. S., Hanapi, M., and Hartana, I. (2008). The presence of tobacco mosaic virus in the compost extract of cigar tobacco debris. J. Biosci. 15, 118–122. doi: 10.4308/hjb.15.3.118
Webster, C. G., Pichon, E., van Munster, M., Monsion, B., Deshoux, M., Gargani, D., et al. (2018). Identification of plant virus receptor candidates in the stylets of their aphid vectors. J. Virol. 92:e00432–18. doi: 10.1128/JVI.00432-18
Wetter, C. (1975). Tobacco mosaic virus and para–tobacco mosaic virus in cigarettes. Naturwissenschaften 62:533.
Wilkes, T., Hall, L., and Burns, M. (2017). A brief review of current bioinformatics decision support system (DSS) tools for screening for GMOs in EU using PCR-based approaches. J. Assoc. Publ. Analysts 45, 023–040.
Wintermantel, W. M., and Schoelz, J. E. (1996). Isolation of recombinant viruses between cauliflower mosaic virus and a viral gene in transgenic plants under conditions of moderate selection pressure. Virology 223, 156–164. doi: 10.1006/viro.1996.0464
Wolf, C., Scherzinger, M., Wurz, A., Pauli, U., Hübner, P., and Lüthy, J. (2000). Detection of Cauliflower mosaic virus by the polymerase chain reaction: testing of food components for false-positive 35S-promoter screening results. Eur. Food Res. Technol. 210, 367–372. doi: 10.1007/s002170050565
Wong, A. Y. T., and Chan, A. W. K. (2016). Genetically modified foods in China and the United States: a primer of regulation and intellectual property protection. Food Sci. Hum. Wellness 5, 124–140. doi: 10.1016/j.fshw.2016.03.002
Wu, Y., Wang, Y., Li, J., Li, W., Zhang, L., Li, Y., et al. (2014). Development of a general method for detection and quantification of the P35S promoter based on assessment of existing methods. Sci. Rep. 4:7358. doi: 10.1038/srep07358
Wunderlich, S., and Gatto, K. A. (2015). Consumer perception of genetically modified organisms and sources of information. Adv. Nutr. 6, 842–851. doi: 10.3945/an.115.008870
Wurch, T., Kirchherr, D., Mesnard, J. M., and Lebeurier, G. (1990). The Cauliflower mosaic virus open reading frame VII product can be expressed in Saccharomyces cerevisiae but is not detected in infected plants. J. Virol. 64, 2594–2598. doi: 10.1128/JVI.64.6.2594-2598.1990
Yang, L., Wang, C., Wang, L., Xu, C., and Chen, K. (2013). An efficient multiplex PCR assay for early detection of Agrobacterium tumefaciens in transgenic plant materials. Turk. J. Agric. For. 37, 157–162. doi: 10.3906/tar-1009-1265
Yasaka, R., Nguyen, H. D., Ho, S. Y. W., Duchêne, S., Korkmaz, S., Katis, N., et al. (2014). The temporal evolution and global spread of Cauliflower mosaic virus, a plant pararetrovirus. PLoS ONE 9:e856410. doi: 10.1371/journal.pone.0085641
Keywords: Cauliflower mosaic virus, CaMV, organic agriculture, qPCR, GMO
Citation: Bak A and Emerson JB (2020) Cauliflower mosaic virus (CaMV) Biology, Management, and Relevance to GM Plant Detection for Sustainable Organic Agriculture. Front. Sustain. Food Syst. 4:21. doi: 10.3389/fsufs.2020.00021
Received: 29 November 2019; Accepted: 17 February 2020;
Published: 31 March 2020.
Edited by:
Avtar Krishan Handa, Purdue University, United StatesReviewed by:
James Schoelz, University of Missouri, United StatesCopyright © 2020 Bak and Emerson. This is an open-access article distributed under the terms of the Creative Commons Attribution License (CC BY). The use, distribution or reproduction in other forums is permitted, provided the original author(s) and the copyright owner(s) are credited and that the original publication in this journal is cited, in accordance with accepted academic practice. No use, distribution or reproduction is permitted which does not comply with these terms.
*Correspondence: Joanne B. Emerson, amJlbWVyc29uQHVjZGF2aXMuZWR1
Disclaimer: All claims expressed in this article are solely those of the authors and do not necessarily represent those of their affiliated organizations, or those of the publisher, the editors and the reviewers. Any product that may be evaluated in this article or claim that may be made by its manufacturer is not guaranteed or endorsed by the publisher.
Research integrity at Frontiers
Learn more about the work of our research integrity team to safeguard the quality of each article we publish.