- 1EPSRC Centre for Doctoral Training, Centre for Sustainable Chemical Technologies, University of Bath, Bath, United Kingdom
- 2Department of Chemical Engineering, University of Bath, Bath, United Kingdom
- 3Department of Pharmacy and Pharmacology, University of Bath, Bath, United Kingdom
Cultured meat, as a cellular agriculture product, utilizes tissue engineering techniques and consequently faces not only cell culture challenges but also scale-up limitations. To ensure cultured meat is financially viable, efficient bioprocess design for scale-up is required. In this mini-review we focus on the design of the expansion bioreactor, and put it in context of the entire bioprocess by providing an overview of the upstream and downstream process considerations. As a full-scale cultured meat bioprocess is still hypothetical we include a review of the key factors and fundamental cell biology parameters required as input data for the design of a process with a product that is not only viable but price competitive. This review highlights the vital aspects of a cultured meat bioreactor design that are often overlooked when parallels are drawn against fermentation processes such as brewing or recombinant protein production in the pharmaceutical industry. Practical application and awareness of the concepts presented here will enable more accurate estimation of the production expenses and raw material requirements. This will form a basis for both further academic research and the design of industrial-scale processes in the field of cultured meat and the wider field of tissue engineering-based cellular agriculture.
Introduction
The current status of the cultured meat field is still within the research and development phase: at the time of writing there is no commercially available cultured meat product. Cultured meat is also known as cell-based meat, clean meat and in vitro meat. The biological knowledge to produce cultured meat—tissue engineering of muscle and fat—is relatively well-understood and developed at lab scale, however cultured meat production technology is still early stage. There are a number of technical challenges facing large-scale production, including cell source, scaffold, culture media and bioprocessing (Edelman et al., 2005; Datar and Betti, 2010; Specht et al., 2018; Stephens et al., 2018). Whether this be on a small kilogram-scale, or a large ton-scale is yet to be determined; hypothetical options vary from small-scale, local factories capable of supplying villages to large-scale, industrial-sized, commercial production plants (van der Weele and Tramper, 2014). The technical landscape of the field is more prevalent within the start-up space than academia, with accessible intellectual property (IP) in the form of patents; publicly available patents that are currently available include: (Van Eelen et al., 1999; Vein, 2004; Van Eelen, 2007; Marga and Forgacs, 2014; Hasson et al., 2015; Forgacs et al., 2016, 2017; Genovese et al., 2016, 2017, 2018, 2019; Marga, 2016; Miller, 2017; Elfenbein and Kolbeck, 2018; Nahmias, 2018; Savir et al., 2018; Ben-Arye and Levenberg, 2019).
In conjunction with the production process, quality assurance (QA) will need to form an integral part of the process, pertaining to monitoring of cell-state, for example cell viability, phenotype, protein content, etc. also mentioned in Stephens et al. (2018). As no ISO standard exists for cultured meat to date, current practice is based on lab-scale techniques and Good Cell Culture Practice (GCCP) as outlined by Coecke et al. (2005). To make cultured meat production financially viable it will be necessary to utilize a less manual-labor intensive and more automated and efficient process of production than lab-scale tissue culture, in the form of a bioprocess. In this article, use of the term “large-scale” will be used to refer to any automated and scalable bioprocess irrespective of production volume. Aspects of this challenge have been previously reviewed (Moritz et al., 2015) while the purpose of this mini-review is to outline the design considerations necessary for large-scale production of cultured meat in the form of muscle cells alone, focused on the expansion bioreactor, with an overview of the other related key design considerations. While we focus on cultured meat grown on a scaffold, the general design considerations can be applied to all other cell types whether they are adherent or non-adherent.
The Bioreactor in Context of the Bioprocess
The starting point for bioprocess design is to specify the final product form e.g., processed vs. full cut meat, or a dry powder source of protein vs. a wet cell biomass. This decision impacts the type of bioprocess units required for both the upstream and downstream process. The purpose of a bioreactor is to generate a controlled environment suitable for the in vitro management of the mammalian cells. The two sequential cell culture phases of proliferation and differentiation form the foundation of the bioprocess design as the cultured cells are the desired end product. The design is iterative as choices must be made alongside the calculations of the mass balances, energy balances, and methods of heat supply/removal including heat integration to save energy. An example of upstream and downstream design iteration is the use of a recycle to save water and sizing of waste valorization units to utilize waste products will be dependent on the bioreactor effluent flow rate. Alongside the bioreactor(s) for proliferation and differentiation the upstream process is likely to include units such as media storage tanks, media heat exchangers, and a means of maintaining isothermal (constant temperature) conditions within the bioreactor(s). A summary of the key areas for consideration in the design and optimization of a cultured meat bioprocess is provided in Figure 1, with the bioreactor at the center of the system.
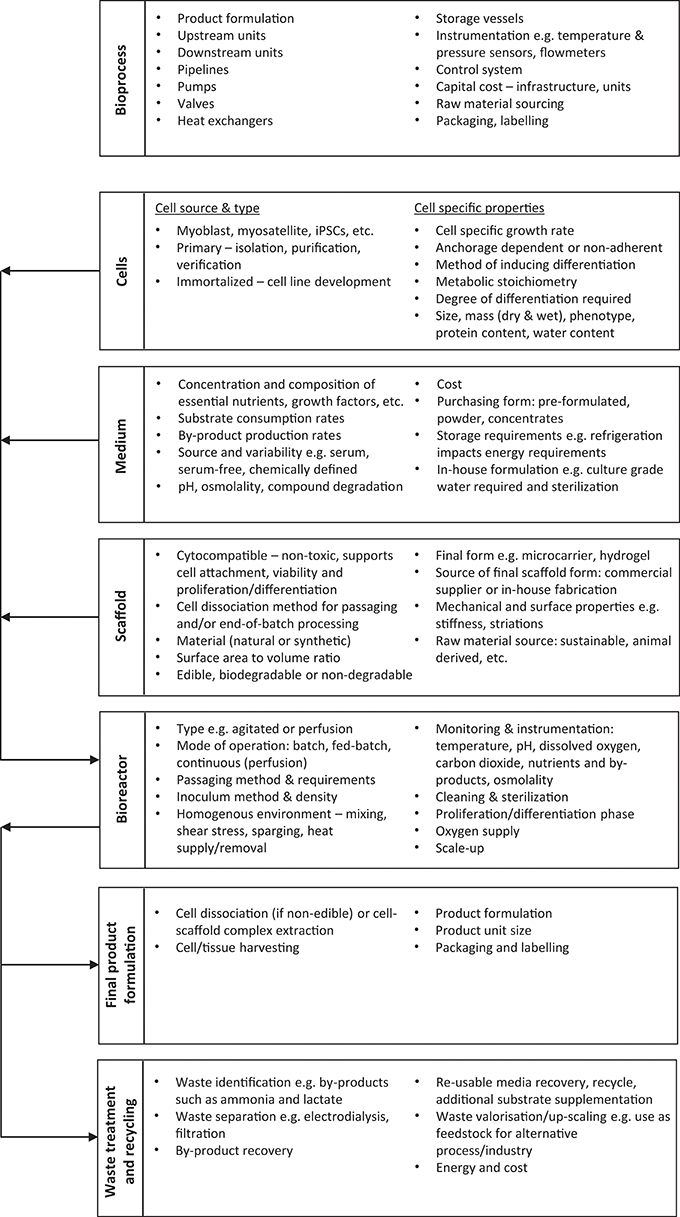
Figure 1. Summary of the key areas of consideration for cultured meat bioprocess design. The top block gives a whole-process overview, and the remaining boxes provide a summary of the inputs to and outputs from the bioreactor.
Cells, Media, and Scaffold
While not the subject of review here, a bioprocess design review cannot overlook the cells, media and scaffold entirely, so we highlight key considerations. The cell source can be primary cells isolated from tissue, via live or dead animal biopsies or immortalized cell lines (Datar and Betti, 2010; Specht et al., 2018; Stephens et al., 2018). Stem cells will likely be required for the biomass expansion phase due to their ease of proliferation and can be muscle progenitor cells such as myoblasts or myosatellite stem cells due to their directed lineage to myotubes or alternatives such as induced pluripotent stem cells (iPSCs) (Post, 2012; Kadim et al., 2015; Specht et al., 2018).
The media requirements can be evaluated in two ways; (i) the media required to satisfy the minimum working volume of a bioreactor based on the maximum achievable cell density (cells/mL) and (ii) the media required based on cell consumption. Ideally, the minimum media requirement should be based on cell consumption to prevent substrate limitations being imposed on cell viability, maintenance and growth. Use of an optimized and chemically-defined media could minimize waste and the associated cost of media as well as CAPEX.
In the expansion phase, the desire is to maximize biomass yields e.g., biomass per glucose and minimize by-product yields. The deciding factors in cell choice and media choice should also include total protein yield; ease of maintaining replicative ability for proliferation; ease of inducing differentiation; and “robustness” to the bioprocessing steps.
Scaffold design and form should be considered as an intrinsic part of the bioprocess design, as the surface area to volume ratio has a direct impact on the size of the bioreactor(s) required, and choice of scaffold affects the bioreactor seeding efficiency, passaging requirements of the system, downstream processing, bioreactor fluid dynamics and mass transfer, and cost. The scaffold can either be edible, or non-edible. One perceived benefit of using edible biomaterials is that the scaffold may contribute to the texture of the final product, which may be viewed upon favorably until the technical challenge of co-culturing (e.g., with fat cells), has been overcome (Edelman et al., 2005; Datar and Betti, 2010). Further considerations specific to cultured meat include the foreseeable benefit of aligning cells to assist differentiation into myotubes and maturation into myofibres, Schuster et al. (2017) either by stimulation (Edelman et al., 2005) or scaffolding surface properties such as fibrillar striations shown to align satellite cells (Yan et al., 2007).
If the cells need to be removed from the scaffold, for passaging or if the scaffold is not edible or biodegradable, several dissociation techniques exist. With lab-scale culture it is common practice to perform enzymatic dissociation using trypsin, however trypsin is animal-derived from cows or pigs and can be subject to batch-to-batch variation (Masters and Stacey, 2007). Other methods are available such as mechanical or shear-induced dissociation (Wang et al., 2018), and controllable biomaterials (Duffy et al., 2014; Miotto et al., 2017). Non-animal derived dissociation alternatives such as the recombinant enzyme TrypLE, derived from microbial sources, or an enzyme-free cell dissociation buffer such as Versene (EDTA) to promote aggregates could also be used (Masters and Stacey, 2007; Beers et al., 2012; Daniszewski et al., 2018). These alternatives may be preferable in serum-free applications as serum is not required to inhibit enzymatic activity.
Downstream and Recycle Operation Units
Foreseeable downstream units required with recycle operation include cell debris removal, and medium refinement, cell harvesting and product formulation. Medium refinement will allow the retention of valuable components such as glucose, glutamine and proteins, plus removal of unwanted lactate and ammonia. Recovery of purified water may also be carried out using downstream separation units. Potential downstream units may include one or more of membrane filtration, (electro)dialysis, precipitation, solvent extraction, and adsorption. Relevant industrial examples include fermentation (Li et al., 2008; Lee et al., 2017; Seankham et al., 2017); water treatment (Bódalo et al., 2005); and iPSC culture (Nath et al., 2017). Further product formulation units capable of chopping, drying, flavoring, texturizing, packaging and labeling may be required (Park et al., 2014) depending on the product of interest.
Bioreactor Design
The first stage to consider, and one that remains a challenge for the industry, is cell expansion via proliferation at scale; this will form the focus of the bioreactor discussion here.
Lessons From Other Industries
Within the field of cultured meat, a popular analogy is to describe the large-scale process required for production as comparable to a brewery to help consumers and experts from different fields visualize a hypothetical cultured meat process. A brewery and the majority of other large-scale biotechnology processes are fermentation based using prokaryotes or simple eukaryotes such as yeast, while cultured meat requires complex eukaryotic cells such as mammalian or fish cells. Current commercial applications of mammalian cell culture are in the recombinant (therapeutic) protein industry as host-cells, and the cell therapy industry. All these biotechnology industries present opportunities to merge established technology to scale-up cultured meat production.
Therapeutic protein production is carried out in large-scale stirred tank bioreactors (STRs) up to 20,000 L (Eibl et al., 2009; Kunert and Reinhart, 2016). This presents a good parallel and source of technical expertise for cultured meat production, however the cell lines approved for the production of monoclonal antibodies (mAbs) for human therapy are CHO, NSO and Sp2/0 cells (Dumont et al., 2016; Kunert and Reinhart, 2016) all of which are cultured in suspension, without the need for a scaffold. Although mammalian cells are more shear-sensitive than prokaryotes, STRs with impeller-driven mixing and air sparging are used in the therapeutic protein industry with eukaryotes. This is possible due to the use of suspension cell-lines, however the myocyte precursors required for cultured meat are anchorage-dependent and have lower shear limits (Venkat et al., 1996; Hu et al., 2011). The limitation results from the hydrodynamic forces (shear and normal stresses) that detach the cell from the scaffold surface, resulting in eventual cell death, while suspension cells can withstand higher agitation in the form of both air sparging and impeller rotations (Cherry and Papoutsakis, 1986; Hu et al., 2011). This limitation could be overcome by utilizing scaffold-free cell aggregates or spheroids (Kumar and Starly, 2015; Merten, 2015). Possible advantages are the potential to achieve high cell densities and the in vivo-like culture environment; the disadvantages include difficulties in controlling diameters in bioreactors which may lead to necrotic centers and passaging limitations if adhesion proteins are damaged during aggregate dissociation to single-cell form (Kumar and Starly, 2015).
The relatively new industry of cell therapies, where the cell is the product of interest, is the most relevant comparison, with allogeneic (off-the-shelf) rather than autologous (patient-specific) cell therapies most pertinent due to the scale requirement. The industry is seeing a drive toward the need for fully automated bioprocesses, both upstream and downstream to reduce variations associated with manual culture and improve reproducibility, but is largely currently at the scale of 100–1000 well-plates and tissue culture flasks (Daniszewski et al., 2018). Applicable cell therapies include the culture of anchorage-dependent mesenchymal stem cells (MSCs) reviewed by Jossen et al. (2018). The allogeneic, cell therapy industry is experiencing a similar bottleneck to the cultured meat industry resulting from the difficulties of large-scale adherent cell culture. An industry shift from planar flask cultures to automated bioreactors is taking place seeing the use of single-use STRs ranging from working volumes of 35–50 L (Schirmaier et al., 2014; Lawson et al., 2017; Jossen et al., 2018), note the scale difference to suspension cell culture.
Bioreactor Configuration and Sizing
Bioreactor Configurations
The mode of operation, be it batch, fed-batch or continuous will impact the bioreactor size and media requirements. From a large-scale perspective, typically fed-batch or continuous supply of media is favored. Several configurations can operate in these modes. Agitated vessels are currently the most common in biotech industries. They provide a time-averaged homogenous, well-mixed environment through convective mixing initiated by mechanical, pneumatic or hydraulic agitation such as impeller driven stirred tank bioreactors (STRs), rotating wall bioreactors (RWBs), and rocking motions as seen with wave bioreactors. Other bioreactor configurations enable continuous, perfusion operation such as packed bed bioreactors (PBBs), fluidized bed bioreactors (FBBs) and membrane bioreactors such as hollow fiber bioreactors (HFBs). For non-perfusion reactors, such as STRs, continuous (perfusion) operation requires the coupling of the bioreactor with an internal or external cell retention device on a recycle line, by centrifugation, sedimentation, ultrasonic separation or microfiltration with spin-filters, alternating tangential flow (ATF) filtration or tangential flow filtration (TFF) (Woodside et al., 1998; Czermak et al., 2007; Clincke et al., 2013a,b). A summary of the bioreactors tested for animal myosatellite and myoblast cell culture to date is presented in Table 1.
Bioreactor Sizing, Passaging and the Importance of Cell Density
Decisions related to the type, size and number of bioreactors will be influenced by a number of factors including passaging. Passaging, in the form of sequential transference to reactors of increasing size, as seen in seed trains, is required to satisfy the minimum and maximum cell densities. Microcarrier culture and bead-to-bead transfer capability of a cell-line (Wang and Ouyang, 1999; Ferrari et al., 2012; Verbruggen et al., 2017) may enable passaging through the addition of microcarriers to increase surface area without increasing vessel size. Bioreactor comparisons should be made based on final cell density achievable and not on the volume, an arbitrary concept without context such as the seeding density and final cell number or density and passaging steps. The achievable cell density will differ for suspension systems that use microcarriers for anchorage-dependent cells vs. single-cell suspension. Jossen et al. (2018) presents a summary of the working volume and cell-density achieved in different studies and bioreactor types culturing adherent cells for cultured therapies:
• 1.90 ×105−2 ×106 cells/mL in production scale STRs (35–50 L),
• 1.90 ×105 cells/mL in wave bioreactors (0.5–1.5 L),
• 2.93 ×106 cells/mL in PBBs (1.0–5.0 L),
• 108−109 cells/mL in HFBs.
Back of the Envelope (BOTE) calculations enable a quantifiable comparison of the working volume required for different bioreactors. Assuming a wet cell mass of c.a. 3.5 ×10−12 kg/cell (Park et al., 2008; Hu, 2012; Mattick et al., 2015) the number of cells required to produce 1 kg of wet “meat” in the form of muscle cells is c.a. 2.9 x 1011 cells. Based on theoretical maximum cell density achievable reported by Ellis et al. (2005), which are in-line with the densities summarized by Jossen et al. (2018), for adherent cell culture the working volumes by bioreactor type are calculated to be:
• 2,900 L for tissue culture flasks (lab-scale culture),
• 570 L for STRs,
• 110 L for PBBs,
• 48 L for FBBs,
• 1.4 L for HFBs.
These numbers are provided to give an idea of the impact cell density can have on the size of a bioreactor required to achieve the same biomass target. However, this does not account for the number of seed-train reactors dictated by inoculum cell numbers, passaging requirements, and scaffold surface area to volume ratios. Reader attention is drawn to the fact that this is the ‘working volume' of the bioreactor and does not dictate the process media requirements, a common misconception within the field when making predictions of media requirements; which will be much greater than the bioreactor working volumes due to cell metabolic requirements over the duration of the culture period.
A recent development in these high-value product industries is the move toward single-use bioreactors (SUBs), with the associated benefits of reduced down-time between batches, minimal cleaning in place (CIP), reduced contamination risks and improved scale-up flexibility with bioreactor configurations available up to 2,000 L (Langer and Rader, 2014; Jacquemart et al., 2016; Schnitzler et al., 2016). For the recombinant protein industry, the increased operating expenses associated with replacing SUBs per batch are offset due to higher product throughput (Jacquemart et al., 2016). However, a cost of goods analysis specific to cultured meat should be carried out as the economics will differ for a high volume, low value commodity. The environmental impact of single-use items must also be considered since the plastic waste will end up in landfill or the sea due to mixed plastic content and contamination imposing recycling difficulties.
Other Design Considerations
Important Considerations for Upstream Bioprocess Design
The following are considered key factors to be considered in the design of an upstream bioprocess for mammalian cells:
• Cell starting number, N0. Essential for calculating the number of days required to expand to the desired final number, when used with the inoculation efficiency.
• Inoculation efficiency. How many of the starting cell number “take” to the bioreactor environment and are able to proliferate on the scaffold.
• Media requirements. Affects OPEX and CAPEX in terms of sizing of vessels, piping, and pumps. See section Cells, Media, and Scaffold.
• Cleaning/sterilization. Has financial and time implications plus must meet regulations.
• Scaffold source. Must be sustainable, always available, and have minimal batch to batch variation
• Passaging. May be needed during proliferation to maintain exponential growth. See section Bioreactor Sizing, Passaging and the Importance of Cell Density.
• Inoculation and cell removal methods. How the cells are added to and removed from the bioreactor will impact yield.
Availability of the following kinetic and fundamental cell biology parameters specific to muscle cells are required for the design of a process with a product that is not only viable but price competitive. These parameters are cell-type and species specific and also system specific and need quantifying for detailed bioprocess design.
• Cell specific mass (wet and dry) and volume. Impacts the final desired cell number, NF for product of interest.
• Cell specific growth rate and doubling efficiency. Combined with N0 and NF determines the proliferation batch time.
• Cell specific protein content and mass change during differentiation as a function of differentiation time/stage. Dictates the differentiation batch time for product of interest.
• Cell stability, aging and differentiation potential as a function of time and passage number. Dictates the number of population doublings, passages, and ability to become mature muscle cells for a given starting cell population.
• Cell density per unit volume of bioreactor. Directly impacts seed train requirements, quantity and size of bioreactors used.
• Oxygen spatial and temporal variation, uptake rate (OUR) and CO2 production rate. Affects bioreactor size, oxygen supply method and influent media flow rate.
• Metabolic yield coefficients. Enable efficient supply of media to minimize waste and OPEX.
• Cell-scaffold dissociation method. Affects downstream units.
• Cell- and system-specific tolerances to lactate, ammonia, pH and osmolality. Impacts the design of the recycle, purge and downstream separation units.
• Heat released per mol of oxygen consumed, or other measure of metabolic pathway energy. Defines the energy requirements of the bioreactor for either heating or cooling to maintain isothermal conditions of 37°C.
Oxygen, Carbon Dioxide, and pH
Oxygen needs to be added to the media, either in the form of aeration through spargers within the bioreactor, or upstream to ensure the media is saturated with dissolved oxygen to meet the high oxygen requirements. Depending on the buffer used to maintain pH 7.2–7.4 within the bioreactor, provision and maintenance of carbon dioxide concentration may be required (Masters and Stacey, 2007). Bubble aeration, through sparging is traditionally used to supply oxygen in large scale bioreactors, however alternative bubble-free aeration methods exist such as use of gas permeable silicone tubing for feed piping, or an external media aeration device (Eibl et al., 2009).
Conclusions
This review has brought together literature from across the biochemical engineering and tissue engineering fields, with focus on the proliferation bioreactor, to highlight the design considerations that need to be met for a bioprocess capable of commercializing a cultured meat product. It acknowledges that while lessons can be learned from other biotech industries, there lacks key data required for bioprocess design. These have been identified and discussed in the hope readers will be able to address these and contribute to better design and progression of the nascent field.
Author Contributions
The review and manuscript was conducted and written by SA. ME supervised SA and revised and edited the manuscript. PD provided additional revision, support, and guidance. All authors contributed to manuscript revision, read and approved the submitted version.
Funding
The authors would like to thank New Harvest, a 501(c)(3) non-profit research institute and the EPSRC Centre for Doctoral Training in Sustainable Chemical Technologies (EP/L016354/1) for funding this review.
Conflict of Interest Statement
ME is a co-founder of Cellular Agriculture Ltd.
The remaining authors declare that the research was conducted in the absence of any commercial or financial relationships that could be construed as a potential conflict of interest.
Acknowledgments
The authors would like to thank the University of Bath for supporting this work.
References
Baba, K., and Sankai, Y. (2017). Development of biomimetic system for scale up of cell spheroids - Building blocks for cell transplantation. Proc. Annu. Int. Conf. IEEE Eng. Med. Biol. Soc. EMBS 2017, 1611–1616. doi: 10.1109/EMBC.2017.8037147
Beers, J., Gulbranson, D. R., George, N., Siniscalchi, L. I., Jones, J., Thomson, J. A., et al. (2012). Passaging and colony expansion of human pluripotent stem cells by enzyme-free dissociation in chemically defined culture conditions. Nat. Protoc. 7, 2029–40. doi: 10.1038/nprot.2012.130
Bettahalli, N. M. S., Steg, H., Wessling, M., and Stamatialis, D. (2011a). Development of poly(l-lactic acid) hollow fiber membranes for artificial vasculature in tissue engineering scaffolds. J. Memb. Sci. 371, 117–126. doi: 10.1016/j.memsci.2011.01.026
Bettahalli, N. M. S., Vicente, J., Moroni, L., Higuera, G. A., Van Blitterswijk, C. A., Wessling, M., et al. (2011b). Integration of hollow fiber membranes improves nutrient supply in three-dimensional tissue constructs. Acta Biomater. 7, 3312–3324. doi: 10.1016/j.actbio.2011.06.012
Bódalo, A., Gómez, J. L., Gómez, E., León, G., and Tejera, M. (2005). Ammonium removal from aqueous solutions by reverse osmosis using cellulose acetate membranes. Desalination 162, 149–155. doi: 10.1016/j.desal.2005.03.062
Breese, T. W., and Admassu, W. (1999). Feasibility of culturing C2C12 mouse myoblasts on glass microcarriers in a continuous stirred tank bioreactor. Bioprocess Eng. 20, 463–408. doi: 10.1007/s004490050616
Cherry, R. S., and Papoutsakis, E. T. (1986). Hydrodynamic effects on cells in agitated tissue culture reactors. Bioprocess Eng. 1, 29–41. doi: 10.1007/BF00369462
Clincke, M. F., Mölleryd, C., Samani, P. K., Lindskog, E., Fäldt, E., Walsh, K., et al. (2013a). Very high density of Chinese hamster ovary cells in perfusion by alternating tangential flow or tangential flow filtration in WAVE bioreactorTM-part II: Applications for antibody production and cryopreservation. Biotechnol. Prog. 29, 768–777. doi: 10.1002/btpr.1703
Clincke, M. F., Mölleryd, C., Zhang, Y., Lindskog, E., Walsh, K., and Chotteau, V. (2013b). Very high density of CHO cells in perfusion by ATF or TFF in WAVE bioreactorTM: Part I: Effect of the cell density on the process. Biotechnol. Prog. 29, 754–767. doi: 10.1002/btpr.1704
Coecke, S., Balls, M., Bowe, G., Davis, J., Gstraunthaler, G., Hartung, T., et al. (2005). Second ECVAM task force on good cell culture practice: guidance on good cell culture practice. a report of the second ECVAM task force on good cell culture practice. Altern Lab Anim 33, 261–287. doi: 10.1177/026119290503300313
Czermak, P., Nehring, D., and Wickramasinghe, R. (2007). “Membrane Filtration in Animal Cell Culture,” in Animal Cell Biotechnology, ed. R. Portner (Totowa, NJ: Human Press Inc., 397–420.
Daniszewski, M., Crombie, D. E., Henderson, R., Liang, H. H., Wong, R. C. B., Hewitt, A. W., et al. (2018). Automated cell culture systems and their applications to human pluripotent stem cell studies. SLAS Technol. 23, 315–325. doi: 10.1177/2472630317712220
Datar, I., and Betti, M. (2010). Possibilities for an in vitro meat production system. Innov. Food Sci. Emerg. Technol. 11, 13–22. doi: 10.1016/j.ifset.2009.10.007
Duffy, C. R. E., Zhang, R., How, S. E., Lilienkampf, A., De Sousa, P. A., and Bradley, M. (2014). Long term mesenchymal stem cell culture on a defined synthetic substrate with enzyme free passaging. Biomaterials 35, 5998–6005. doi: 10.1016/j.biomaterials.2014.04.013
Dumont, J., Euwart, D., Mei, B., Estes, S., and Kshirsagar, R. (2016). Human cell lines for biopharmaceutical manufacturing: history, status, and future perspectives. Crit. Rev. Biotechnol. 36, 1110–1122. doi: 10.3109/07388551.2015.1084266
Edelman, P., McFarland, D., Mironov, V., and Matheny, J. (2005). In vitro cultured meat production. Tissue Eng. 11, 659–662. doi: 10.1089/ten.2005.11.659
Eibl, R., Eibl, D., Pörtner, R., Catapano, G., and Czermak, P. (2009). Cell and Tissue Reaction Engineering. Heidelberg: Springer-Verlag. doi: 10.1007/978-3-540-68182-3
Ellis, M., Jarman-Smith, M., and Chaudhuri, J. (2005). “Bioreactor Systems for Tissue Engineering: A Four-Dimensional Challenge,” in Bioreactors for Tissue Engineering, eds. J. Chaudhuri and M. Al-Rubeai (Dordrecht: Springer), 1–18.
Ferrari, C., Balandras, F., Guedon, E., Olmos, E., Chevalot, I., and Marc, A. (2012). Limiting cell aggregation during mesenchymal stem cell expansion on microcarriers. Biotechnol. Prog. 28, 780–787. doi: 10.1002/btpr.1527
Forgacs, G., Jakab, K., Neagu, A., and Mironov, V. (2017). Self-Assembling Cell Aggregates and Methods of Making Engineering Tisue Using the Same. Patent. US 2017/0145386 A1.
Forgacs, G., Marga, F., and Jakab, K. R. (2016). Engineered Comestible Meat. Patent. US 8,703,216 B2. 2.
Genovese, N., Desmet, D. N., and Schulze, E. (2017). Methods for extending the replicative capacity of somatic cells during an ex vivo cultivation process. Patent. WO 2017124100 A1.
Genovese, N. J., Firpo, M. T., and Dambournet, D. (2018). Compositions and Methods for Increasing the Culture Density of a Cellular Biomass Within a Cultivation Infrastructure. Patent. WO 2018/208628 A1.
Genovese, N. J., Roberts, R. M., and, V. L., and Telugu, B. P. (2016). Method for Scalable Skeletal Muscle Lineage Specification and Cultivation. Patent. US 2016//0227830 A1.
Genovese, N. J., Schulze, E. N., and Desmet, D. N. (2019). Compositions and Method for Increasing the Efficiency of Cell Cultures Used for Food Production. Patent. WO 2019/014652 A1.
Hasson, A., Yirme, G., Lavon, N., Feffen-Shalev, C., and Molakandov, K. (2015). Methods for Large Scale Generation of Stem Cells. Patent. WO 2015/008275 A1.
Hu, W., Berdugo, C., and Chalmers, J. J. (2011). The potential of hydrodynamic damage to animal cells of industrial relevance: current understanding. Cytotechnology 63, 445–460. doi: 10.1007/s10616-011-9368-3
Jacquemart, R., Vandersluis, M., Zhao, M., Sukhija, K., Sidhu, N., and Stout, J. (2016). A single-use strategy to enable manufacturing of affordable biologics. Comput. Struct. Biotechnol. J. 14, 309–318. doi: 10.1016/j.csbj.2016.06.007
Jossen, V., van den Bos, C., Eibl, R., and Eibl, D. (2018). Manufacturing human mesenchymal stem cells at clinical scale: process and regulatory challenges. Appl. Microbiol. Biotechnol. 102, 3981–3994. doi: 10.1007/s00253-018-8912-x
Kadim, I. T., Mahgoub, O., Baqir, S., Faye, B., and Purchas, R. (2015). Cultured meat from muscle stem cells: A review of challenges and prospects. J. Integr. Agric. 14, 222–233. doi: 10.1016/S2095-3119(14)60881-9
Kumar, A., and Starly, B. (2015). Large scale industrialized cell expansion: Producing the critical raw material for biofabrication processes. Biofabrication 7:044103. doi: 10.1088/1758-5090/7/4/044103
Kunert, R., and Reinhart, D. (2016). Advances in recombinant antibody manufacturing. Appl. Microbiol. Biotechnol. 100, 3451–3461. doi: 10.1007/s00253-016-7388-9
Langer, E. S., and Rader, R. A. (2014). Single-use technologies in biopharmaceutical manufacturing: A 10-year review of trends and the future. Eng. Life Sci. 14, 238–243. doi: 10.1002/elsc.201300090
Lawson, T., Kehoe, D. E., Schnitzler, A. C., Rapiejko, P. J., Der, K. A., Philbrick, K., et al. (2017). Process development for expansion of human mesenchymal stromal cells in a 50 L single-use stirred tank bioreactor. Biochem. Eng. J. 120, 49–62. doi: 10.1016/j.bej.2016.11.020
Lee, H. D., Lee, M. Y., Hwang, Y. S., Cho, Y. H., Kim, H. W., and Park, H. B. (2017). Separation and purification of lactic acid from fermentation broth using membrane-integrated separation processes. Ind. Eng. Chem. Res. 56, 8301–8310. doi: 10.1021/acs.iecr.7b02011
Li, Y., Shahbazi, A., Williams, K., and Wan, C. (2008). Separate and concentrate lactic acid using combination of nanofiltration and reverse osmosis membranes. Appl. Biochem. Biotechnol. 147, 1–9. doi: 10.1007/s12010-007-8047-5
Marga, F. S. (2016). Dried Food Products Formed From Cultured Muscle Cells. Patent. US 2016/0227831 A1.
Marga, F. S., and Forgacs, G. (2014). Spherical Multicellular Aggregates with Endogenous Extracellular Matrix. Patent. WO 2014/039938 A1.
Masters, J. R., and Stacey, G. N. (2007). Changing medium and passaging cell lines. Nat. Protoc. 2, 2276–2284. doi: 10.1038/nprot.2007.319
Mattick, C. S., Landis, A. E., Allenby, B. R., and Genovese, N. J. (2015). Anticipatory life cycle analysis of in vitro biomass cultivation for cultured meat production in the United States. Environ. Sci. Technol. 49, 11941–11949. doi: 10.1021/acs.est.5b01614
Merten, O. W. (2015). Advances in cell culture: anchorage dependence. Philos. Trans. R. Soc. B Biol. Sci. 370, 20140040–20140040. doi: 10.1098/rstb.2014.0040
Miotto, M., Gouveia, R., Abidin, F. Z., Figueiredo, F., and Connon, C. J. (2017). Developing a continuous bioprocessing approach to stromal cell manufacture. ACS Appl. Mater. Interfaces 9, 41131–41142. doi: 10.1021/acsami.7b09809
Molnar, G., Schroedl, N. A., Gonda, S. R., and Hartzell, C. R. (1997). Skeletal muscle satellite cells cultured in simulated microgravity. Vitr. Cell. Dev. Biol. Anim. 33, 386–391. doi: 10.1007/s11626-997-0010-9
Moritz, M. S. M., Verbruggen, S. E. L., and Post, M. J. (2015). Alternatives for large-scale production of cultured beef: a review. J. Integr. Agric. 14, 208–216. doi: 10.1016/S2095-3119(14)60889-3
Nath, S. C., Nagamori, E., Horie, M., and Kino-oka, M. (2017). Culture medium refinement by dialysis for the expansion of human induced pluripotent stem cells in suspension culture. Bioprocess Biosyst. Eng. 40, 123–131. doi: 10.1007/s00449-016-1680-z
Park, K., Jang, J., Irimia, D., Sturgis, J., Lee, J., Robinson, J. P., et al. (2008). “Living cantilever arrays” for characterization of mass of single live cells in fluids. Lab Chip 8, 1034–1041. doi: 10.1039/b803601b
Park, S. H., Lamsal, B. P., and Balasubramaniam, V. M. (2014). “Principles of Food Processing,” in Food Processing: Principles and Applications, eds. S. Clark, S. Jung, and B. Lamsal (Chichester: John Wiley & Sons), 1–15.
Post, M. J. (2012). Cultured meat from stem cells: challenges and prospects. Meat Sci. 92, 297–301. doi: 10.1016/j.meatsci.2012.04.008
Savir, I., Friedman, S., and Barak, K. (2018). Cultured Meat-Containing Hybrid Food. WO 2018/189738 A1. doi: 10.1007/978-94-007-6167-4_106-2
Schirmaier, C., Jossen, V., Kaiser, S. C., Jüngerkes, F., Brill, S., Safavi-Nab, A., et al. (2014). Scale-up of adipose tissue-derived mesenchymal stem cell production in stirred single-use bioreactors under low-serum conditions. Eng. Life Sci. 14, 292–303. doi: 10.1002/elsc.201300134
Schnitzler, A. C., Verma, A., Kehoe, D. E., Jing, D., Murrell, J. R., Der, K. A., et al. (2016). Bioprocessing of human mesenchymal stem/stromal cells for therapeutic use: Current technologies and challenges. Biochem. Eng. J. 108, 3–13. doi: 10.1016/j.bej.2015.08.014
Schuster, E., Wallin, P., Klose, F. P., Gold, J., and Ström, A. (2017). Correlating network structure with functional properties of capillary alginate gels for muscle fiber formation. Food Hydrocoll. 72, 210–218. doi: 10.1016/j.foodhyd.2017.05.036
Seankham, S., Novalin, S., and Pruksasri, S. (2017). Kinetics and adsorption isotherm of lactic acid from fermentation broth onto activated charcoal. Chem. Ind. Chem. Eng. Q. 23, 515–521. doi: 10.2298/CICEQ160511004S
Specht, E. A., Welch, D. R., Rees Clayton, E. M., and Lagally, C. D. (2018). Opportunities for applying biomedical production and manufacturing methods to the development of the clean meat industry. Biochem. Eng. J. 132, 161–168. doi: 10.1016/j.bej.2018.01.015
Stephens, N., Di, L., Dunsford, I., Ellis, M., Glencross, A., and Sexton, A. (2018). Bringing cultured meat to market: technical, socio-political, and regulatory challenges in cellular agriculture. Trends Food Sci. Technol. 78, 155–166. doi: 10.1016/j.tifs.2018.04.010
van der Weele, C., and Tramper, J. (2014). Cultured meat: every village its own factory? Trends Biotechnol. 32, 294–296. doi: 10.1016/j.tibtech.2014.04.009
Van Eelen, W. F. (2007). Industrial Production of Meat Using Cell Culture Methods. Patent. US 7270829 B2.2.
Van Eelen, W. F., Van Kooten, W. J., and Westerhof, W. (1999). Industrial Scale Production of Meat from In vitro Cell Cultures. Patent. WO 99/31222.
Vein, J. (2004). Method for Producing Tissue Engineered Meat for Consumption. Patent. US 6835390 B1.
Venkat, R. V., Stock, L. R., and Chalmers, J. J. (1996). Study of hydrodynamics in microcarrier culture spinner vessels: A particle tracking velocimetry approach. Biotechnol. Bioeng. 49, 456–466.
Verbruggen, S., Luining, D., van Essen, A., and Post, M. J. (2017). Bovine myoblast cell production in a microcarriers-based system. Cytotechnology, 1–10. doi: 10.1007/s10616-017-0101-8
Wang, H., Xia, J., Zheng, Z., Zhuang, Y. P., Yi, X., Zhang, D., et al. (2018). Hydrodynamic investigation of a novel shear-generating device for the measurement of anchorage-dependent cell adhesion intensity. Bioprocess Biosyst. Eng. 41, 1371–1382. doi: 10.1007/s00449-018-1964-6
Wang, Y., and Ouyang, F. (1999). Bead-to-bead-transfer of vero cells in microcarrier culture. Bioprocess Eng. 21, 211–213. doi: 10.1007/s004490050665
Woodside, S. M., Bowen, B. D., and Piret, J. M. (1998). Mammalian cell retention devices for stirred perfusion bioreactors. 163–175. doi: 10.1007/978-94-011-4786-6_18
Yamamoto, Y., Ito, A., Jitsunobu, H., Yamaguchi, K., Kawabe, Y., Mizumoto, H., et al. (2012). Hollow fiber bioreactorperfusion culture system for magnetic force-based skeletal muscle tissue engineering. J. Chem. Eng. Japan 45, 348–354. doi: 10.1252/jcej.11we237
Keywords: bioprocessing, bioreactor, cellular agriculture, cultured meat, in vitro meat, muscle cell, scale-up, tissue engineering
Citation: Allan SJ, De Bank PA and Ellis MJ (2019) Bioprocess Design Considerations for Cultured Meat Production With a Focus on the Expansion Bioreactor. Front. Sustain. Food Syst. 3:44. doi: 10.3389/fsufs.2019.00044
Received: 26 October 2018; Accepted: 22 May 2019;
Published: 12 June 2019.
Edited by:
Lorenzo Pastrana, Laboratório Ibérico Internacional de Nanotecnologia (INL), PortugalReviewed by:
Azila Abdul-Aziz, University of Technology Malaysia, MalaysiaSara M. Oliveira, Laboratório Ibérico Internacional de Nanotecnologia (INL), Portugal
Copyright © 2019 Allan, De Bank and Ellis. This is an open-access article distributed under the terms of the Creative Commons Attribution License (CC BY). The use, distribution or reproduction in other forums is permitted, provided the original author(s) and the copyright owner(s) are credited and that the original publication in this journal is cited, in accordance with accepted academic practice. No use, distribution or reproduction is permitted which does not comply with these terms.
*Correspondence: Scott J. Allan, cy5qLmFsbGFuJiN4MDAwNDA7YmF0aC5hYy51aw==