- 1Independent Researcher, Helsinki, Finland
- 2Air Liquide, Paris Innovation Centre, Les Loges en Josas, Jouy en Josas, France
- 3Department of Food and Nutrition, University of Helsinki, Helsinki, Finland
Worldwide, tremendous amounts of food, including milk and dairy products, are wasted. Although refrigeration permits longer storage of raw milk, low temperatures still do not prevent the action of other abiotic and biotic factors that promote biochemical, chemical, microbial, sensorial and nutritional changes in raw milk, which also affect milk-derived dairy products. Treatment by N2 gas-flushing showed great potential in preserving the microbiological quality of raw milk during storage at low and milder temperatures. Here, we examined the impact of cold-storage (at 6°C up to 7 days), on the ascorbic acid content, extent of auto-oxidation, and on levels of lipolysis and proteolysis along with bacterial growth, in three raw-milk samples in the presence or absence of N2. When N2 gas was applied, lower levels of lipolysis and proteolysis were found to coincide with the detection of lower numbers of bacterial lipase and protease-producers in raw milk. Furthermore, lower auto-oxidation was detected in N2-treated samples than in simply cold stored controls. By demonstrating that key components of milk were better preserved during cold storage, the present study further highlights the advantages of the N2-flushing treatment in terms of preserving the quality and safety of raw milk and its derived dairy products.
Introduction
The Food and Agriculture Organization (FAO) estimates that globally one third of the food produced annually for human consumption is lost or wasted. In Europe, the annual dairy product wastage and loss amounts to 29 × 106 t (FAO, 2012). These enormous losses contribute to major resource squandering with a proportionately negative impact on the environment.
Despite the implementation of specific processing steps in existing high-tech food systems, spoilage due to microbial growth and concomitant biochemical activities remains significant as not all risks can be eradicated. This is also due to the fact that microbes, especially bacteria, have remarkable adaptation properties (Angelidis et al., 2002; Jay et al., 2005; Burgess et al., 2016). Bovine milk, which comprises 4.6% carbohydrates, 4.1% lipids, 3.3% proteins, vitamins and minerals, is an essential food for new-born mammals but also an ideal growth base for various microorganisms (Walstra et al., 2006).
Where cold-chain conditions exist, the microbiological quality of raw milk mainly relies on bacteriological criteria that are based on an estimation of “total bacterial counts,” enumerated under certain defined conditions: in the EU, bacterial levels in raw milk, at dairy farms and processing sites, should not exceed 105 and 3 × 105 cfu/mL, respectively (European Parliament Council, 2004).
Native enzymes in bovine raw milk originate from alveolar epithelium cells, somatic cells (mainly white blood cells), and the milk fat globule membrane (MFGM) or blood plasma (Fox and Kelly, 2006; Campbell and Drake, 2013). However, various types of enzymes are also synthesized by contaminating microorganisms. Under cold-chain conditions, raw-milk spoilage is mainly due to psychrotrophic bacteria that produce various proteases, lipases, and phospholipases, even at low temperatures. These enzymes are mainly synthesized in the late exponential and early stationary growth phases when cell densities are high (Cousin, 1982; Sorhaug and Stepaniak, 1997; Dunstall et al., 2005; Machado et al., 2017; Munsch-Alatossava et al., 2018). Raw milk is subjected to heat treatment in an effort to eliminate the major bacterial component, yet due to remarkable heat stability, a significant quantity of bacterial enzymes remain active and degrade the milk components in processed products. Excessive levels of proteolytic or lipolytic activity can impact the quality of fluid milk, dry whole milk and butter, can affect cheese yield, cause bitter flavors, or trigger gelation of UHT milk (Sorhaug and Stepaniak, 1997; Fox and Mc Sweeney, 1998; Machado et al., 2017). A link between bacterial levels in raw milk and UHT milk quality was previously established by Sorhaug and Stepaniak (1997), who demonstrated that a psychrotrophic bacterial load of 5.5 log-units in raw milk triggered gelation of UHT milk, after 20 weeks storage.
The pH of raw milk also constitutes an indicator of quality. Typical pH values for fresh, unspoiled bovine raw-milk, range between 6.5 and 6.7, with divergent values indicative of spoilage or signs of mastitis infection (Fox and Mc Sweeney, 1998).
Milk contains a native lipase (lipoprotein lipase, LPL) that could cause rancidity in a short period if the MFGM is ruptured, since the milk fat is then exposed to the serum phase (Deeth, 2006). The occurrence of undesirable lipolysis mostly implicates contaminating bacterial genera (e.g., Pseudomonas, Acinetobacter, Bacillus, etc…), which often synthesize heat-stable lipases that liberate fatty acids, leading to the alteration of flavor and functionality of milk and dairy products (Stead, 1986; Sorhaug and Stepaniak, 1997; Dogan and Boor, 2003; Deeth, 2006; Vithanage et al., 2016; Machado et al., 2017). The action of bacterial lipases is further facilitated by the simultaneous production of phospholipase C (PLC), as evidenced in both Gram-positive and Gram-negative bacteria, where PLC disrupts the MFGM thereby enabling the degradation of neutral lipids (mainly triacylglycerols) by lipases (Craven and Macauley, 1992; Sorhaug and Stepaniak, 1997; Deeth, 2006). The extent of lipolysis, commonly expressed as “Acid Degree Value (ADV),” is defined as the amount of 1N base required to neutralize free fatty acids in 100 g of milk fat (Fox and Mc Sweeney, 1998); (Hooi et al., 2004).
Several native proteases are present in raw milk: the most prominent of which is plasmin that can be also active during cold-storage (Fox and Mc Sweeney, 1998; Ismail and Nielsen, 2010). However, excessive proteolytic activity, which leads to casein degradation, usually implicates bacterial proteases. The production of proteases is a common trait of numerous bacterial genera, including Acinetobacter, Bacillus, and many Pseudomonas species in particular, which are well-represented in milk (Sorhaug and Stepaniak, 1997; Dogan and Boor, 2003; Munsch-Alatossava and Alatossava, 2006; Vithanage et al., 2016); (Machado et al., 2017).
During processing and storage, dairy materials and products are exposed to oxygen that is either dissolved or present within the head space of tanks or packages. In turn, the process of auto-oxidation deteriorates the flavor and nutritional properties of milk and dairy products (Fox and Mc Sweeney, 1998; Hedegaard et al., 2006). Polyunsaturated fatty acids (PUFA), which are mainly present in the MFGM of raw milk, are amongst the most sensitive compounds to auto-oxidation. PUFA oxidation results in the accumulation of flavorless and odorless hydroxyperoxides; however, the decomposition of hydroxyperoxides leads to compounds responsible for off-flavors (Fox and Mc Sweeney, 1998; O'Connor and O'Brien, 2006). The oxidation of lipids may have additional consequences, as lipid oxidation products may possibly interact with other essential nutrients (e.g., vitamins) present in raw milk (Wasowitz et al., 2004).
Thiobarbituric acid reactive substances (TBARS) are low molecular weight carbonyl compounds that are typically formed during the decomposition of lipid oxidation products (King, 1962). TBARS components, formed during lipid oxidation, can be spectrophotometrically quantified since the reaction of the oxidation products with thiobarbituric acid results in the formation of pink-colored products that present a strong absorbance peak at 532–535 nm.
Ascorbic acid (vitamin C), a natural antioxidant and primary redox system in bovine raw milk, also influences the extent of auto-oxidation. Ascorbic acid content ranges between 0.85 and 2.65 mg per 100 g raw milk (Fox and Mc Sweeney, 1998; Walstra et al., 2006).
Three studies previously used “closed systems,” to examine the potential of N2 gas, to reduce the impact of bacterial enzymes on milk spoilage. Skura et al. (1986) noticed that proteinase accumulation was effectively suppressed in UHT milk following inoculation with Pseudomonas fluorescens; also, under N2, a very moderate increase of the ADV suggested an inhibition of P. fluorescens lipase production. When raw milk was tested, no casein degradation was detected by Murray et al. (1983). Dechemi et al. (2005) observed minor changes in the pH of raw milk under 100% N2; however, the same study reported that the 100% N2-atmosphere showed poor inhibition of psychrotrophs, and their proteolytic and lipolytic activities.
N2 gas-flushing of raw milk, when applied to an “open system,” revealed the following advantages: bacterial development was significantly inhibited at laboratory and pilot-plant scales, while bacterial diversity was better preserved during cold-storage; the treatment was mostly of equal efficiency compared to the activated lactoperoxidase system (for treating raw milk stored at 15 or 25°C); the same treatment targeted Pseudomonas spp., triggered a bactericidal type of effect toward Bacillus weihenstephanensis, while showing a significant protective effect on antioxidant compounds, alleviation of phospholipolysis, and reduction in the risk of antibiotic resistance disseminating in raw milk (Munsch-Alatossava et al., 2010a,c, 2016, 2017, 2018; Munsch-Alatossava and Alatossava, 2014; Gschwendtner et al., 2016; Gursoy et al., 2017; Alatossava and Munsch-Alatossava, 2018).
In the EU, 6°C represents the maximum recommended storage temperature of milk, if the collection occurs daily (European Parliament Council, 2004). The present study aimed to determine whether raw-milk samples, that presented lower numbers of proteolytic and lipolytic bacteria under the N2-treatment combined to cold-storage (6°C for up to 7 days), also exhibited signs of lower levels of lipolysis and proteolysis. In addition, the effect of N2-treatment on ascorbic acid and auto-oxidation levels was also determined and compared to the conditions where raw-milk samples were only cold-stored.
Materials and Methods
N2 Gas-Based Treatment of Bovine Raw-Milk Samples
Three independent bovine raw-milk samples (M1, M2, and M3), representing pooled raw milk collected from local farms around the Helsinki region (Finland) and delivered to the Helsingin Meijeriliike Ltd. dairy plant, were considered for analysis. The N2 gas-flushing experimental settings were as described in Munsch-Alatossava et al. (2010a). The N2 gas, of 99.999% purity, was from AGA Ltd. (Riihimäki, Finland) and was adjusted to a continuous flow rate of 120 mL/min. For each condition examined, 135 mL raw milk (placed in a 250 mL vessel) was continuously mixed and stored at 6 ± 0.1°C in a water bath for up to 7 days, while being exposed to either constant N2 gas-flushing (N) or to air, which acted as the control (C). The description of the conditions (applied to each sample) was as follows: 0, initial condition; C3 and C7 represented the cold-stored control samples for 3 and 7 days, respectively; N3 and N7 represented the N2-flushed while cold-stored samples for the same periods.
Microbiological Analyses
At the sampling time points, “total bacterial counts” and psychrotrophs were enumerated using Plate Count Agar medium (PCA, LabM, Lancashire, UK), following incubation of the plates for 3 days at 30°C and 10 days at 7°C, respectively (Munsch-Alatossava et al., 2018). Protease and lipase-producers were, respectively, determined at each time point on PCA + 1% skim milk or tributyrin containing agar, as described by Dogan and Boor (2003), and Munsch-Alatossava and Alatossava (2006). The production of extracellular enzymes by bacterial colonies was evaluated on agar plates incubated at 30°C for 3 days. All bacterial colony counts were primarily determined from triplicate (if not, duplicate) platings, following aerobic incubation; the comparison of the conditions (controls or N2-treated milk) considered log-transformed bacterial counts.
pH Measurements
The pH of the raw-milk samples was determined with a pH meter (WTW Inolab R, Germany) at the initial (0) and final stages (day 7) of the experiments.
Biochemical Analyses
Raw milk samples, representing the different experimental conditions, were collected at the considered time points and stored at −80°C until being subjected to the following analyses.
Lipolysis
The extent of lipolysis was quantified at the initial (0) and final (day 7) stages of the experiments. Determination of ADV used 50 mL raw milk for each sample (M1–M3), with the experimental procedure completed according to the method described by Hooi et al. (2004); 1% phenolphtalein was used as the pH indicator.
Proteolysis
For each sample (M1–M3), 600 μL of raw milk was treated with trichloroacetic acid (TCA) (final concentration of 6.7% w/v). Following centrifugation at 10,000 × g for 5 min, the level of proteolysis (represented by the amount of 6.7% TCA-soluble peptides) was determined in 100 μL supernatant fractions using the Bio-Rad Protein assay (Quick startTM Bradford Protein Assay, Bio-Rad, USA), according to the manufacturer's guidelines. The absorbance values were read at 595 nm for the initial (0), intermediate (day 3) and final (day 7) stages of the experiments.
Ascorbic Acid (Vitamin C)
For each sample (M1–M3), at each sampling time point, 1 mL raw milk was treated with chymosin (0.5% w/v final concentration). Following incubation for 1 h at 37°C, the samples were centrifuged for 5 min at 10,000 × g and the ascorbic acid content was determined in 100 μL supernatant fractions using the Ascorbic Acid Assay kit II (MAK075, Sigma-Aldrich, USA), according to the manufacturer's guidelines.
Thiobarbituric Acid Reactive Substances (TBARS)
For each sample (M1–M3), at each sampling point, 1 mL raw milk was treated with TCA (final concentration of 6.7% w/v). Following centrifugation for 5 min at 10 000 × g, 300 μL supernatant fractions were collected and tested using the QuantiChromTM TBARS assay kit (DTBA-100, Bioassay Systems USA), according to the manufacture's guidelines.
Statistical Analyses
The data (depicted in Table 1 and Figures 1–3) are presented as means of the three raw-milk samples (M1–M3) ± standard deviation (SD). The data comparison was performed using a two-tailed Student's t-test, assuming uniform variances. Graphpad (available at www.graphpad.com) served to convert t into p-values. In the graphs, significant differences were marked by asterisks (***) when p < 0.001. The Pearson's coefficient (r) was used for correlation analysis.
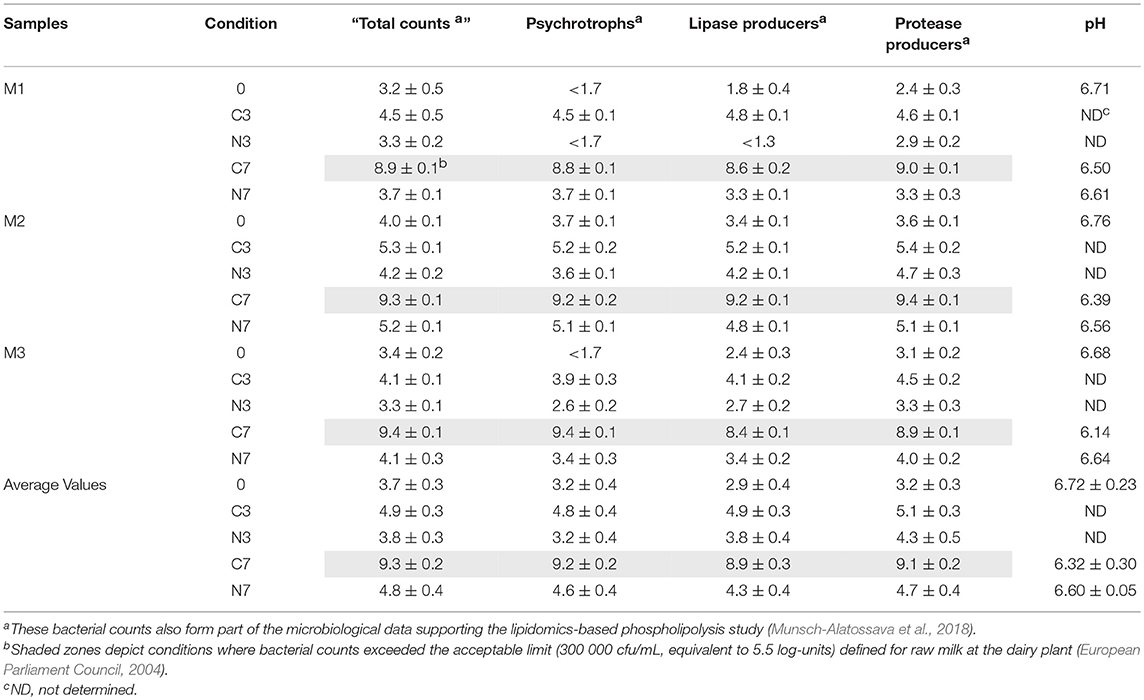
Table 1. Bacterial counts (expressed in log cfu/mL) and pH values recorded for the raw- milk samples (M1–M3), at initial stage (0) and after 3 and 7 days under cold-storage (C3, C7), and under N2-flushing combined to cold-storage (N3, N7).
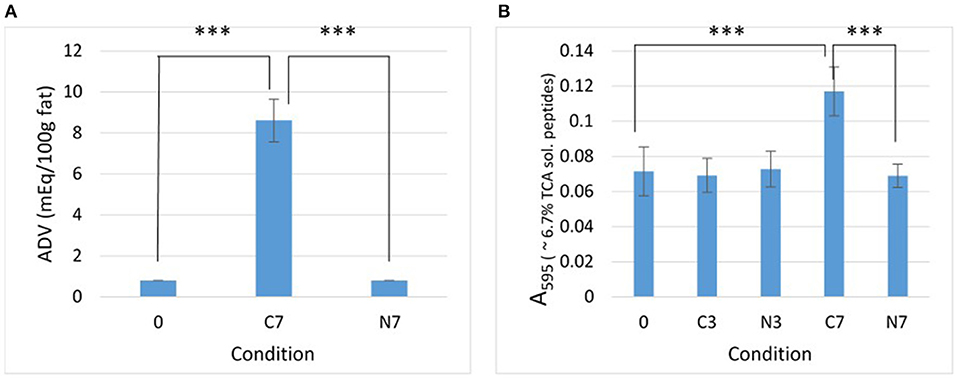
Figure 1. Lipolysis and proteolysis. Effect of the N2 gas-flushing on (A) lipolysis and (B) proteolysis, in the three raw-milk samples (M1–M3) at initial stage (0) and after cold-storage for 3 and 7 days at 6°C in the presence (N3, N7) or absence (C3, C7) of N2 gas-flushing. In (A), each data point represents the mean ADV value obtained from the three samples. In (B), data points correspond to the mean values obtained from three replicates for each raw-milk sample.
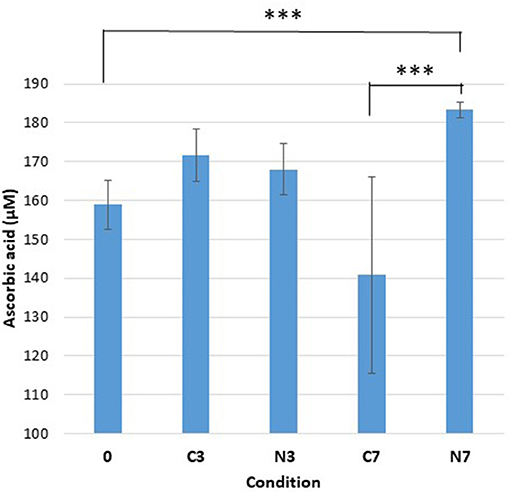
Figure 2. Ascorbic acid content. Ascorbic acid concentration (μM) in the three raw-milk samples (M1–M3) at initial stage (0), after cold-storage for 3 and 7 days at 6°C in the controls (C3, C7), and in the cold-stored while N2-treated conditions (N3, N7). Each data point represents the mean value obtained from the three samples.
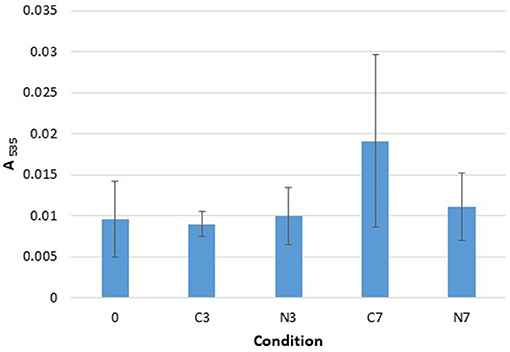
Figure 3. TBARS. Absorbance, at 535 nm, recorded with the Quanti ChromTM TBARS Assay kit for the three raw milk-samples (M1–M3) at initial stage (0) and after cold-storage for 3 and 7 days at 6°C in the controls (C3, C7), and in the cold-stored while N2-treated conditions (N3, N7). The data are presented as mean absorbance values recorded at 535 nm and obtained from three replicates for each sample.
Results
Bacterial Growth Trends
During cold-storage, “total bacterial counts” and psychrotrophs increased in the controls (C3 and C7) for all samples (M1–M3). After 7 days, “total counts” and psychrotrophs had increased on average by 5.6 and 6.0 log-units, respectively (Table 1). Treatment by N2-flushing considerably hindered bacterial growth, with “total counts” and psychrotrophs, respectively, rising on average by 1.1 and 1.4 log-units over 7 days (N7).
Initial protease and lipase-producers were at the highest and most similar levels in sample M2 (Table 1), which also showed the highest initial “total counts” and psychrotrophs. At the beginning of the experiments (condition 0), the detected colonies were on average 12% lipase-positive in all three samples. Thereafter, an increase of over 7-fold was recorded for the control conditions (C3), with up to 88% of the colonies exhibiting lipase activity. After 7 days, lipase-producers had further increased, but represented only 44% of the enumerated colonies in the evaluated samples. In the controls (C3), protease-producers increased on average by 1.8 log-units over 3 days (Table 1). After 7 days, practically all bacterial colonies were protease-positive in C7. Treatment by N2-flushing similarly impacted lipase and protease-producers. Following 7 days cold-storage, the average increase of lipase and protease-producers in N7 was only 1.4 and 1.5 log-units, respectively (Table 1).
pH of Milk Samples
At the end of the storage period, the pH values had dropped in a sample-dependent manner for the controls (C7), and were significantly lower (p = 0.0113) compared to the initial pH levels (0) (Table 1). In the presence of N2, over the same cold-storage period, minor changes occurred but pH values were also significantly different (p = 0.0110) between the N7 and (0) conditions. The pH values were also significantly higher (p = 0.0359) in N7, compared to C7.
Lipolysis and Proteolysis
After 7 days of cold-storage, the controls (C7) showed considerably increased ADV compared to initial condition (0) (p = 0.0002) (Figure 1A). In contrast, the ADV remained unchanged for the N2-flushed samples (N7).
After 3 and 7 days of cold-storage, the absorbance (A595) values recorded from the 6.7% TCA-soluble peptide fractions were quite similar for the C3, N3, and N7 conditions and were not very different from the initial absorbance (0) (Figure 1B). This indicated that the levels of proteolysis had not increased in cold-stored while N2-flushed raw milk samples over 7 days. Conversely, the cold-stored control samples (C7) exhibited significantly higher absorbance values compared to the initial value (0) (p < 0.0001). Proteolysis levels were also significantly higher in C7 than in N7 (p < 0.0001) (Figure 1B).
The Pearson's correlation coefficient (r), calculated for the differences in protease-positive bacterial counts (Table 1) and the differences in absorbance of the 6.7% TCA-soluble peptide fractions (Figure 1B) between controls and N2-treated samples, was 0.92 (p = 0.0013).
Ascorbic Acid
Initially (condition 0), the average concentration of ascorbic acid was approximately 160 μM (Figure 2). After 3 days, both control (C3) and N2-treated samples (N3) showed only minor increases. After 7 days, the ascorbic acid content had moderately increased in N7 and was significantly higher compared to the initial conditions (0) (p = 0.0084). The ascorbic acid levels for N7 were also established as significantly higher compared to the levels in C7 (p < 0.0001).
In contrast, the control conditions (C7) showed moderately lower levels, that were however not significantly different from the initial content (0). The C7 conditions also revealed significant sample variation as the M1, M2, and M3 samples exhibited levels of 172.9, 108.9, and 134.5 μM of ascorbic acid, respectively. For the conditions 0, C3, N3, C7, and N7, the recorded average amounts corresponded to 1.6, 1.7, 1.7, 1.4, and 1.8 mg of ascorbic acid/100 mL raw milk, respectively.
TBARS
The absorbance recorded for the C3, N3, and N7 conditions were quite similar to the initial absorbance (condition 0) (Figure 3). After 7 days of cold-storage, only the control conditions (C7) showed an increase in absorbance that was not significantly different from the initial (0) and N7 conditions (p = 0.2080 and p = 0.1161, respectively). However, the C7 conditions were also characterized by significant sample variations (with a higher value for the M2 compared to that for M1 and M3 samples).
Discussion
When considering initial “total bacterial counts,” the raw-milk samples (M1, M2, and M3) were of very good “bacteriological” quality. Cold-storage alone (C7) promoted a considerable increase of all bacterial types, including those associated with spoilage (Table 1). When N2-flushing was combined with cold-storage (N7), it either inhibited or permitted only a moderate increase of “total bacterial counts,” psychrotrophs, lipolytic, and proteolytic colonies, as well as prevented “total bacterial counts” to exceed the recommended limit (European Parliament Council, 2004). The efficiency of N2-treatment was highest for the M1 and M3 samples, which presented the lowest initial “total counts” and psychrotrophs below the enumeration limit. These samples presumably originated from raw milk with a shorter cold-storage history. The fact that the impact of N2-treatment was sample-dependent, was also previously observed (Munsch-Alatossava et al., 2013, 2017; Gschwendtner et al., 2016).
The evaluated raw-milk samples exhibited initial pH values (Table 1) in line with the literature (Fox and Mc Sweeney, 1998; Walstra et al., 2006). Cold-storage promoted a drop of pH values for the controls (C7), whereas the pH of the N2-treated (N7) raw milk showed a smaller decrease during cold-storage (Table 1). These observations are in agreement with previous reports (Skura et al., 1986; Dechemi et al., 2005); (Munsch-Alatossava et al., 2010a).
ADV determination demonstrated that the initial raw-milk samples had an average ADV value of 0.8, which was slightly above the range of 0.25–0.4 for “normal” raw milk, as defined by Hooi et al. (2004). A previous report showed that bacterial phospholipase (presumably PLC) producers present in raw milk, were particularly targeted or even “excluded” by N2-flushing treatment (Munsch-Alatossava et al., 2010b). A mass spectrometry-based lipidomics study revealed that, compared to a single cold-storage step, N2-based treatment combined with cold-storage preserved the phospholipid composition of raw milk during cold-storage (Munsch-Alatossava et al., 2018).
If N2-treatment better preserves the MFGM during cold-storage, lipolysis should be prevented since bacterial lipases (even if produced) cannot degrade triacylglycerols stored inside the milk fat globule. This assumption was corroborated by the observations made herein, as after 7 days, the cold-stored controls (C7) were extensively hydrolyzed, whereas N2-treatment prevented lipolysis in N7, during the same cold-storage period (Figure 1A).
The N2-treatment also kept proteolysis levels in the raw-milk samples at their initial levels (Figure 1B). In terms of the extent of lipolysis and proteolysis (Figures 1A,B), the observations recorded in this study with an “open system” are in agreement with those of the reports of Skura et al. (1986) and Murray et al. (1983), when considering ADV evaluation and casein degradation, respectively, but diverge from the observations of Dechemi et al. (2005).
Given that higher vs. lower bacterial loads coincided with higher vs. lower lipolysis and proteolysis levels detected in controls and N2-treated raw-milk samples, respectively (Table 1 and Figure 1), it is tempting to consider that the lower recorded hydrolytic activities simply result from lower levels of bacteria and enzymes in N2-flushed milk. Moreover, the differences in proteolytic activities were strongly correlated (r = 0.92) with differences in bacterial counts between controls and N2-treated samples. Interestingly, a strong correlation (r = 0.88, p = 0.0044) was also observed for the recorded phospholipolytic activities and the levels of phospholipase-producers in raw-milk during cold-storage, in the presence and absence of N2-flushing (Munsch-Alatossava et al., 2018).
Even though a deleterious effect of N2 on bacterial cells was noticed (Munsch-Alatossava and Alatossava 2014), other types of effects can at that stage not be excluded: presumably, N2 could modulate enzyme activity as observed by Zhang et al. (2017), or also affect gene expression. Further studies are requested to unravel direct and indirect modes of action by N2.
The initial ascorbic acid content (Figure 2) was in line with that reported previously (Fox and Mc Sweeney, 1998; Walstra et al., 2006). Despite not being significantly different from the initial conditions (0), a moderate drop in ascorbic acid content was recorded in the controls (C7) (Figure 2), which was in agreement with that reported by Gursoy et al. (2017). However, following N2 gas-flushing, the ascorbic acid content was maintained and even moderately increased under N7 conditions (Figure 2), which was contrary to previous observations (Gursoy et al., 2017). Different experimental approaches together with the fact that various factors like cow feeding rations or milk storage conditions can impact the ascorbic acid levels in milk (Lindmark-Mansson and Akesson, 2000), may explain the observed differences.
In terms of TBARS detection, minor changes were observed overall: however, the occurrence of lipid oxidation was suggested under C7 conditions, which was not observed under N7 conditions (Figure 3). The method employed herein, together with the fact that other milk components may interfere with the reaction between thiobarbituric acid and oxidation products, indicate that lipid oxidation quantification should be performed using a more specific and sensitive experimental approach. However, a connection between an N2-based atmosphere and TBARS levels was highlighted in the report by Lund et al. (2007), wherein it was demonstrated that packaging meat with 100% N2 gas yielded lower TBARS levels.
The absolute need to further develop sustainable food production systems also requires the implementation of environmentally friendly strategies that tackle food waste and losses. In industrialized countries, at consumption levels, milk wastage remains high. For example, the UK Waste and Resources Action Program (WRAP) estimated that milk waste in British households amounted 290 000 t in 2012, with a corresponding loss of 290 million pounds sterling (WRAP, 2015): if ~70% (by weight) of the wasted food arose in households, 18% of the wastage occurred at the food manufacturing stage. It was also estimated that for every $1 invested in food loss and waste reduction, a median company site achieved a $14 return (WRAP, 2017).
Indeed, the development of improved storage technologies would also contribute to improved preservation of food materials before and after processing. Ultimately, when regarding both quality and economical aspects, consumers as well as industries would benefit substantially from food products that present lower spoilage risks.
Conclusion
The single cold-storage step at 6°C for 7 days promoted a significant increase in lipolysis and proteolysis levels in raw milk. In contrast, the additional N2-flushing treatment hindered milk protein and lipid degradation. Compared to the initial analysis of raw-milk samples, lipolysis and proteolysis levels remained constant under N2 and coincided with little growth of lipolytic and proteolytic bacteria. By simultaneously maintaining the oxidative stability of raw milk over the same time period, the potential of the N2-based treatment to preserve the quality of raw milk was herein further expanded.
The outcome of our investigations support the view that N2-flushing seems “not so much to improve” but rather “to preserve” an existing situation. Both the biochemical and microbiological-based observations claim in favor of the earliest possible application of N2, ideally at the dairy farm level.
Contribution to the Field Statement
The FAO recommends that the quality and safety of raw milk should be ensured either by the activation of the lactoperoxidase system (LPS) or by cold-storage; however, both options present disadvantages. The latter selects for psychrotrophic bacteria, that produce various heat-stable enzymes (for which the effects are not fully known) and which simultaneously carry features of antibiotic resistance (AR). Conversely, the LPS, which has a time-limited effect and seems also to favor AR dissemination under certain circumstances, is in reality also often replaced by numerous adulterants (including antibiotics).
As an additional or alternative treatment, we evaluated an N2 gas-based flushing technology. The treatment inhibits bacterial growth at low and milder temperatures, hinders AR dissemination, hampers auto-oxidation as well as prevents both lipolysis and proteolysis in raw milk.
N2 gas, which constitutes 78% of our atmosphere, is an unlimited resource. The flushing technology, built for an “open system” (and successfully tested at pilot plant scale) is simply “borrowing” the gas from the environment and could therefore be applied to all types of dairies around the world.
Addressing food spoilage, offers opportunities to reduce poverty, improve food security and nutrition, and ensure better health, while promoting sustainable economic growth.
Data Availability
Requests to access the datasets should be directed to dGFwYW5pLmFsYXRvc3NhdmEmI3gwMDA0MDtoZWxzaW5raS5maQ==.
Author Contributions
PM-A, DI, MY-I, and TA contributed to the conception and design of the study. PM-A and TA carried out the experiments and analyzed the datasets. PM-A wrote the 1st draft of the MS. TA contributed to the revision of the MS. All authors read and approved the submitted version.
Funding
We gratefully acknowledge the support of these studies by a specific research grant (received in 2016) from M. Leprince-Ringuet, Director of the Paris Saclay Research Center of Air Liquide (France).
Conflict of Interest Statement
DI and MY-I are employed by Air Liquide (France).
The remaining authors declare that the research was conducted in the absence of any commercial or financial relationships that could be construed as a potential conflict of interest.
Acknowledgments
We also express gratitude to CEO Antti Alavuotunki (Helsingin Meijeriliike Ltd.) for the gift of the raw milk samples. We are very grateful to the reviewers for all comments and suggestions to improve our MS: we especially acknowledge Reviewer 2 for the outstanding contribution.
References
Alatossava, T., and Munsch-Alatossava, P. (2018). European Patent 2162021, Munich: European Patent Office.
Angelidis, A. S., Tombras Smith, L., and Smith, G. M. (2002). Elevated carnitine accumulation by Listeria monocytogenes impaired in glycine betaine transport is insufficient to restore wild-type cryotolerance in milk whey. Int. J. Food Microbiol. 75, 1–9. doi: 10.1016/S0168-1605(02)00005-3
Burgess, C. M., Gianotti, A., Gruzdev, N., Holah, J., Knochel, S., Lehner, A., et al. (2016). The response of foodborne pathogens to osmotic and desiccation stresses in the food chain. Int. J. Food Microbiol. 221, 37–53. doi: 10.1016/i.jfoodmicro.2015.12014
Campbell, R. E., and Drake, M. A. (2013). The effect of native and non-native enzymes on the flavour of dried dairy ingredients. J. Dairy Sci. 96, 4773–4783. doi: 10.3168/jds.2013-6598
Cousin, M. A. (1982). Presence and activity of psychrotrophic microorganisms in milk and dairy products: a review. J. Food Prot. 45, 172–207.
Craven, H. M., and Macauley, B. (1992). Microorganisms in pasteurised milk after refrigerated storage, 1: identification of types. Aust. J. Dairy Technol. 47, 38–45.
Dechemi, S., Benjelloun, H., and Lebeault, J. M. (2005). Effect of modified atmospheres on the growth and extracellular enzymes of psychrotrophs in raw milk. Eng. Life Sci. 5, 350–356. doi: 10.1002/elsc.200520082
Deeth, H. C. (2006). Lipoprotein lipase and lipolysis in milk. Int. Dairy J. 16, 555–562. doi: 10.1016/j.idairyj.2005.08.011
Dogan, B., and Boor, K. J. (2003). Genetic diversity and spoilage potentials among Pseudomonas spp. isolated from fluid milk products and dairy processing plants. Appl. Environ. Sci. 69, 130–138. doi: 10.1128/AEM.69.1.130-138.2003
Dunstall, G., Rowe, M. T., Wisdom, G. B., and Kilpatrick, D. (2005). Effect of quorum sensing agents on the growth kinetics of Pseudomonas spp. of raw milk origin. J. Dairy Res. 72, 276–280. doi: 10.1017/S0022029905000713
European Parliament Council (2004). Regulation (EC) No 853/2004 of the European Parliament and of the Council of 29 April 2004 Laying Down Specific Hygiene Rules for on the Hygiene of Foodstuffs. European Parliament Council.
FAO (2012). Available online at: www.fao.org/save-food/resources/keyfindings/infographics/dairy/en (accessed February 24, 2019).
Fox, P. F., and Kelly, A. L. (2006). Indigenous enzymes in milk: overview and historical aspects. Part 2. Int. Dairy J. 16, 517-532. doi: 10.1016/j.idairyj.2005.09.017
Fox, P. F., and Mc Sweeney, P. L. H. (1998). Dairy Chemistry and Biochemistry. London: Blackie Academic & Professionnal.
Gschwendtner, S., Alatossava, T., Kublik, S., Mrkonjic'Fuka, M., Schloter, M., and Munsch-Alatossava, P. (2016). N2 gas flushing alleviates the loss of bacterial diversity and inhibits psychrotrophic Pseudomonas during the cold storage of bovine raw milk. PLoS ONE 11:e0146015. doi: 10.1371/journalpone.0146015
Gursoy, O., Munsch-Alatossava, P., Ertan, K., Yilmaz, Y., and Alatossava, T. (2017). Effect of nitrogen gas flushing treatments on total antioxidant capacity and ascorbic acid content in raw bovine milk during cold storage. Mljekarstvo 67, 155–164. doi: 10.15567/mljekarstvo.2017.0208
Hedegaard, R. V., Kristensen, D., Nielsen, J. H., Frost, M. B., Ostdal, H., Hermansen, J. E., et al. (2006). Comparison of descriptive sensory analysis and chemical analysis for oxidative changes in milk. J. Dairy Sci. 89, 495–504. doi: 10.3168/jds.S0022-0302(06)72112-9
Hooi, R., Barbano, D. M., Bradley, R. L., Budde, D., Bulthaus, M., Chettiar, M., et al. (2004). “Chemical and physical methods”, in Standard Methods for the Examination of Dairy Products, eds H. M. Wehr and J. F. Frank (Washington, DC: American Public Health Association), 363–536.
Ismail, B., and Nielsen, S. S. (2010). Plasmin protease in milk: current knowledge and relevance to dairy industry. J. Dairy Sci. 93, 4999–5009. doi: 10.3168/jds.2010-3122
Jay, J., Loessner, M. J., and Golden, D. A. (2005). “Protection of foods with low-temperatures, and characteristics of psychrotrophic microorganisms,” in Modern Food Microbiology, ed D. R Heldman (San Marcos, CA: Springer), 395–413.
King, R. L. (1962). Oxidation of milk fat globule membrane material. Thiobarbituric acid reactions as a measure of oxidized flavor in milk and model systems. J. Dairy Sci. 45, 1165–1171. doi: 10.3168/jds.S0022-0302(62)89590-3
Lindmark-Mansson, H., and Akesson, B. (2000). Antioxidative factors in milk. Br. J. Nutr. 84, 103–110. doi: 10.1017/s0007114500002324
Lund, M. N., Hviid, M. S., and Skibsted, L. H. (2007). The combined effect of antioxydants and modified atmosphere packaging on protein and lipid oxidation in beef patties during chill storage. Meat Sci. 76, 226–233. doi: 10.1016/j.meatsci.2006.11.003
Machado, S. G., Baglinière, F., Marchand, S., Van Coillie, E., Vanetti, M. C. D., De Block, J., et al. (2017). The biodiversity of the microbiota producing heat-resistant enzymes responsible for spoilage in processed bovine milk and dairy products. Front. Microbiol. 8, 302. doi: 10.3389/fmicb.2017.00302
Munsch-Alatossava, P., and Alatossava, T. (2006). Phenotypic characterization of raw-milk associated psychrotrophic bacteria. Microbiol. Res. 161, 334–346. doi: 10.1016/j.micres.2005.12.004
Munsch-Alatossava, P., and Alatossava, T. (2014). Nitrogen gas flushing can be bactericidal: the temperature-dependent destiny of Bacillus weihenstephanensis KBAB4 under a pure N2 atmosphere. Front. Microbiol. 5:619. doi: 10.33889/fmicb.2014.00619
Munsch-Alatossava, P., Ghafar, A., and Alatossava, T. (2013). Potential of nitrogen gas (N2) flushing to extend the shelf life of cold stored pasteurised milk. Int. J. Mol. Sci. 14, 5668–5685. doi: 10.3390/ijms14035668
Munsch-Alatossava, P., Gursoy, O., and Alatossava, T. (2010a). Potential of nitrogen gas (N2) to control psychrotrophs and mesophiles in raw milk. Microbiol. Res. 165, 122–132. doi: 10.1016/j.micres.2009.02.002
Munsch-Alatossava, P., Gursoy, O., and Alatossava, T. (2010b). Exclusion of phospholipases (PLs)-producing bacteria in raw milk flushed with nitrogen gas (N2). Microbiol. Res. 165, 61–65. doi: 10.1016/j.micres.2008.07.001
Munsch-Alatossava, P., Gursoy, O., and Alatossava, T. (2010c). Improved storage of cold raw milk by continuous flushing of N2 gas separated from compressed air: a pilot scale study. J. Food Process. Technol. 1, 1–4. doi: 10.4172/2157-7110.1000101
Munsch-Alatossava, P., Jääskeläinen, S., Alatossava, T., and Gauchi, J. P. (2017). N2 gas flushing limits the rise of antibiotic-resistant bacteria in bovine raw milk during cold storage. Front. Microbiol. 8:655. doi: 10.3389/fmicb.2017.00655
Munsch-Alatossava, P., Käkel,ä, R., Ibarra, D., Youbi-Idrissi, M., and Alatossava, T. (2018). Phospholipolysis caused by different types of bacterial phospholipases during cold storage of bovine raw milk is prevented by N2 gas flushing. Front. Microbiol. 9:1307. doi: 10.3389/fmicb.2018.01307
Munsch-Alatossava, P., Quintyn, R., De Man, I., Alatossava, T., and Gauchi, J. P. (2016). Efficiency of N2 gas flushing compared to the lactoperoxidase system at controlling bacterial growth in bovine raw milk stored at mild temperatures. Front. Microbiol. 7:839. doi: 10.3389/fmicb.2016.00839
Murray, S. K., Kwan, K. K. H., Skura, B. J., and Mc Kellar, R. C. (1983). Effect of nitrogen flushing on the production of proteinase by psychrotrophic bacteria in raw milk. J. Food Sci. 48, 1166–1169. doi: 10.1111/j.1365-2621.1983.tb09183.x
O'Connor, T. P., and O'Brien, N. M. (2006). “Lipid oxidation,” in Advanced Dairy Chemistry, eds P. F. Fox and P. L. H. Mc Sweeney (New York, NY: Springer), 557–600.
Skura, B. J., Craig, C., and McKellar, R. C. (1986). Effect of disruption of an N2-overlay on growth and proteinase production in milk by Pseudomonas fluorescens. Can. Inst. Food Sci. Technol. J. 19, 104–106. doi: 10.1016/S0315-5463(86)71462-4
Sorhaug, T., and Stepaniak, L. (1997). Psychrotrophs and their enzymes in milk and dairy products: quality aspects. Trends Food Sci. Technol. 8, 35–41. doi: 10.1016/S0924-2244(97)01006-6
Stead, D. (1986). Microbial lipases: their characteristics, role in food spoilage and industrial uses. J. Dairy Res. 53, 481–505.
Vithanage, N. R., Dissanayake, M., Bolge, G., Palombo, E. A., Yeager, T. R., and Datta, N. (2016). Biodiversity of culturable psychrotrophic microbiota in raw milk attributable to refrigeration conditions, seasonality and their spoilage potential. Int. Dairy J. 57, 80–90. doi: 10.1016/j.idairyj.2016.02.042
Walstra, P., Wouters, J. T. M., and Geurts, T. J. (2006). Dairy Science and Technology. Boca Raton, FL: Taylor and Francis Group (CRC Press).
Wasowitz, E., Gramoza, A., Hes, M., Jelen, H. M., Korczak, J., Malecka, M., et al. (2004). Oxidation of lipids in food. Pol. J. Food Nut. Sci. 13, 87–100.
WRAP (2015). Household Food and Drink Waste in the United Kingdom 2012. Available online at: www.wrap.org.uk (accessed February 27, 2019).
WRAP (2017). The business case for reducing food loss and waste. A report on behalf of Champions 12.3. Available online at: www.wrap.org.uk (accessed April 29, 2019).
Keywords: cold-storage, food spoilage, raw milk, psychrotrophs, lipolysis, proteolysis, auto-oxidation, N2 gas
Citation: Munsch-Alatossava P, Ibarra D, Youbi-Idrissi M and Alatossava T (2019) N2 Gas-Flushing Prevents Bacteria-Promoted Lipolysis and Proteolysis and Alleviates Auto-Oxidation in Bovine Raw Milk During Cold-Storage. Front. Sustain. Food Syst. 3:41. doi: 10.3389/fsufs.2019.00041
Received: 10 April 2019; Accepted: 02 May 2019;
Published: 24 May 2019.
Edited by:
Joshua B. Gurtler, Agricultural Research Service, United States Department of Agriculture, United StatesReviewed by:
Steve Flint, College of Sciences, Massey University, New ZealandApostolos S. Angelidis, School of Veterinary Medicine, Faculty of Health Sciences, Aristotle University of Thessaloniki, Greece
Copyright © 2019 Munsch-Alatossava, Ibarra, Youbi-Idrissi and Alatossava. This is an open-access article distributed under the terms of the Creative Commons Attribution License (CC BY). The use, distribution or reproduction in other forums is permitted, provided the original author(s) and the copyright owner(s) are credited and that the original publication in this journal is cited, in accordance with accepted academic practice. No use, distribution or reproduction is permitted which does not comply with these terms.
*Correspondence: Tapani Alatossava, dGFwYW5pLmFsYXRvc3NhdmEmI3gwMDA0MDtoZWxzaW5raS5maQ==