- 1Department of Food Science, Center for Food Safety, University of Arkansas, Fayetteville, AR, United States
- 2Jones-Hamilton Co., Walbridge, OH, United States
As human populations increase in numbers, access to clean, fresh water is becoming increasingly difficult to balance between agricultural and municipal demands. Water scarcity is a limiting factor of food production in many countries, whether they are emerging or established economies. In conventional poultry processing systems, access to water is particularly critical for the maintenance and disinfection of processing areas, as well as in processing operations such as scalding, chilling, and carcass washing. Therefore, poultry processing plants use an excessive amount of water, limiting where facilities can operate, increasing overhead costs, and ultimately resulting in potential environmental concerns. The need for sustainable alternatives to single-use water supplies is becoming increasingly more urgent. As a result, the implementation of water reuse in poultry-processing plants has emerged as an attractive alternative means to meet water requirements during processing. Because the water is reused, it is essential to de-contaminate the water with chemicals, such as peracetic acid and chlorine, and improve water filtration strategies to kill and remove potential pathogens and contaminants. However, questions remain as to the efficacy of commonly used disinfectants to achieve that goal. Thus, novel strategies must be developed to improve the capabilities of poultry processing plants to counter water insecurity worldwide. These new stratagems must be economical and enable poultry processing plants to reduce their environmental footprint while meeting new food safety challenges. The current review will focus exclusively on water reuse in conventional poultry processing in the United States. The specific objectives of this review are to discuss the approaches for treating processing water in poultry processing systems, including reuse water systems, as well as investigate possible substitutes for maintaining food safety.
Introduction
Water scarcity, while a constant concern for developing countries, is rapidly emerging as a global concern (Beekman, 1998; Casani et al., 2005). Alcamo et al. (1997, 2000) estimates that by 2025, half the world' s population will be living in countries facing considerable water stress or scarcity issues (Rijsberman, 2006). Several factors, including climate change, population growth, dietary shifts toward animal protein, irrigated agriculture, seawater intrusion, and increasing demands for domestic and industrial water, all contribute to this emergent problem (Meneses et al., 2017). Globally, the average water footprint is 7.45 *1015 L per year (Hoekstra and Chapagain, 2005, 2006), while the estimated minimum basic water need is 50 L per capita per day (Gleick, 1996, 1998). However, in 2010, the United States alone used 1.1 trillion liters of potable fresh water per day, or over 3,000 L per capita per day (Maupin et al., 2014). This substantial demand for fresh water comes primarily from horticulture, livestock, and energy needs, which accounts for 80% of water usage (Shannon et al., 2008). With expected growth in the global human population, and only 0.007% of the world' s water supply clean and accessible, there is a critical and current need for water conservation practices and technologies, especially in the food industry (United States Census Bureau, 2011; United Nations Department of Economics and Social, Affairs (UNDESA), 2017).
From 1998 to 2008 water use in the food industry increased by ~40% and has continued to grow (Klemes et al., 2008; Meneses et al., 2017). These water requirements have become limiting factors for economic growth in China and India (Klemes et al., 2008). As an example of this extensive water consumption, the Australian food processing industry utilizes 30% of the water used in all industrial facilities in the nation (Department of Agriculture and Water Resources, 2007). In 2005, the Netherlands food industry was the third largest user of water at 247 billion L in the country behind only the chemical and refinery industry (Casani et al., 2005). In poultry processing, water usage is particularly critical as it facilitates the maintenance and disinfection of processing areas and aids in many basic operations such as scalding, chilling, and carcass washing (Luján-Rhenals et al., 2017). Water also helps with meeting regulations for pathogen reduction, Hazard Analysis and Critical Control Point (HACCP), and from other regulatory bodies such as the United States Department of Agriculture-Food Safety and Inspection Service (USDA-FSIS) (Northcutt and Jones, 2004; Sanitation Performance Standards Compliance Guide, 2016). However, the environmental and financial costs of this water use are notable. For example, in Wesley (1977) estimated that the poultry industry in Virginia would save $150,000 ($600,000+ adjusted for inflation) annually if they cut the water usage in one poultry processing facility in half from 1.9 to 0.95 L per bird (Lillard, 1979). However, current practices and regulations put water use per bird to range from 21 to 30 L revealing a dramatic increase in consumption for fresh water in processing operations (Kiepper, 2003; Northcutt and Jones, 2004; Walsh et al., 2018). Therefore, to continue to meet these food safety regulations, while reducing costs and environmental pressures, alternative production systems involving water reuse have been implemented (Andelman and Clise, 1977; Meneses et al., 2017). Water reuse in the overall food industry has recently been reviewed by Meneses et al. (2017). The current review will focus exclusively on water reuse in conventional poultry processing in the United States. The specific objectives of this review are to discuss the approaches for treating processing water in poultry processing systems, including reuse water systems, as well as investigate possible substitutes for maintaining food safety.
Water Use in Poultry Processing Systems
In 2017, over 41.6 billion pounds of chicken were processed and produced in the U.S. with projections of 42.5 billion pounds of chicken to be produced in 2018 (United States Department of Agriculture, 2018). To generate this quantity and meet demand, streamlined plants can process up to 140 birds/ minute per line (9 CFR 381.69) (Owens et al., 2000). Due to the rate of processing, numerous sanitization steps are needed which requires extensive water usage (Meneses et al., 2017). The conventional poultry processing system is outlined in Figure 1. To reduce cross-contamination water is used at nearly all points including killing, bleeding, scalding, defeathering, evisceration, washing, and chilling (Keener et al., 2004; Guerin et al., 2010; Park et al., 2015).
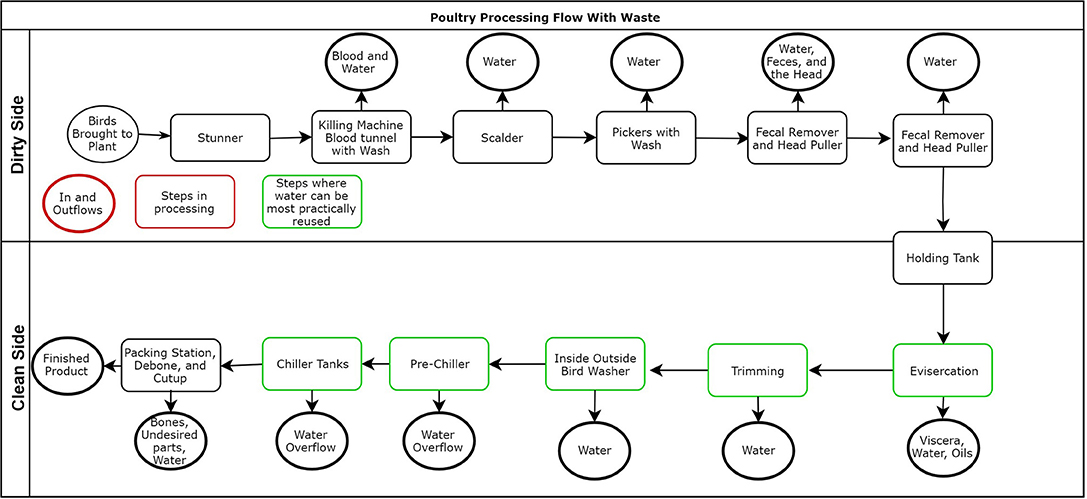
Figure 1. Depicts the layout of a typical poultry slaughter and processing facility. The outflow from each area is also detailed. The figure demonstrates that at almost every step water is a component of the waste (Keener et al., 2004; Avula et al., 2009). Steps in green highlight regions where physical and chemical treatment may be most practical for water reuse (Avula et al., 2009).
As defined by Avula et al. (2009), the average water consumption of a poultry processing plant is 26.5 L/2.3 kg bird. In comparing processing operational steps, they described the steps that utilized the most amount of water per bird as evisceration (7.57 L/Bird), the wash steps (4.25 L/Bird), the deboning and cut-up steps (3.03 L/Bird), and the chilling step (2.12 L/Bird). Water is also utilized in the defeathering and scalding steps, and variations in water usage are present depending on the plant and the finished products. For instance, plants that process whole chicken carcasses may not utilize substantial quantities of water during the deboning step if one is included at all. Processing waters can be used to lower the temperature of a bird, such as chiller water, as well as a lubricant for the machinery, but they are also utilized to disinfect the bird and remove unwanted components, such as the gastrointestinal tract (GIT) (Avula et al., 2009). These processing waters are often treated as wastewater after use due to their high concentration of proteins, fats, carbohydrates, as well as grit and other inorganic materials (Fonkwe et al., 2001; Avula et al., 2009).
Furthermore, wastewater can not only accumulate microbiological contaminants but be an environment that promotes the growth of bacteria including pathogens (Meneses et al., 2017). From 2009 to 2015, foodborne disease was caused by Salmonella, Campylobacter, and shiga toxin producing Escherichia coli (STEC) in 23,662, 2,395, and 2,378 confirmed U.S. cases (Dewey-Mattia et al., 2018). Respectively, of these cases, 3,168, 134, and 672 hospitalizations occurred due to these foodborne diseases (Dewey-Mattia et al., 2018). In 2013, the costs of these foodborne illnesses have been estimated to be $275 million (United States Department of Agriculture, 2017). These pathogens can contaminate poultry products and a CDC study has found that from 2009 to 2015, of the 1,281 outbreaks traced back to a single source, poultry was responsible for 10% of the outbreaks ranking it the highest single source contributor to these outbreaks (Gremillion, 2018). These contaminants can be introduced to the processing waters at many points in processing. During evisceration, this water may come in contact with the ceca, crop, and the remainder of the GIT, which harbor significant levels of bacterial populations. Cecal content, in particular, can contain up to 1011 cells/g of digesta and this digesta can spill out during evisceration as organs may be ruptured (Hargis et al., 1995; Gong et al., 2002; Stanley et al., 2014). To prevent contamination on the carcass, multiple wash steps are often employed (Keener et al., 2004). Sprays of low levels of chlorine and peracetic acid are utilized at various locations to help ensure a potentially pathogen-free product (Owens et al., 2000). Often washers, such as the inside-outside bird washer, use sanitizers, that include chlorine at 20 to 50 ppm, to reduce contamination from blood, tissue, or fecal matter, with varied success (Keener et al., 2004). For example, one poultry processing plant, despite using 9 L of water per bird for carcass washing, more than double the average, only achieved 0.5 log CFU reductions in Campylobacter (Bashor et al., 2004).
Furthermore, chiller water is also used to sanitize the poultry product. Often the last line of poultry processing before cut-up, packaging, and distribution, chillers are filled with cold water, ice, and typically a sanitizer such as peracetic acid or chlorine (Keener et al., 2004). While their purpose is to rapidly cool the bird and prevent bacterial growth (United States Department of Agriculture, 2003a), they also serve as a final step to sanitize the carcass, and up to 50 ppm of chlorine can be added to these waters (Keener et al., 2004). Chiller water often contains 600 to 800mg/L of total solids, and 30% of those are grease and fat (Avula et al., 2009). Because of the content of these waters and despite the previous wash steps, (Northcutt et al., 2008) recovered 2.6, 2.9, and 2.6 log CFU/mL of E. coli, coliforms, and Campylobacter respectively. Furthermore, 9 of 40 post-chill carcasses were found to be positive for Salmonella, and this was not affected by the reuse or reconditioning of the chiller water (Northcutt et al., 2008).
In 1999, a survey estimated a typical poultry plant spends $500,000 to $1 million on water for carcass washers alone despite their low reduction potential on pathogen concentrations such as Campylobacter (Jackson et al., 1999; Keener et al., 2004). This survey found water costs per year for a typical plant to be $1.2 million and for water and sewer costs to be an average $0.4 per L and $0.58 per L (Jackson et al., 1999). Almost equally important is the environmental costs that can occur should water be improperly disposed of. In 1995, a large poultry waste lagoon ruptured and spilled 32.6 million L of waste into a nearby creek which diluted to 1 mg/L over 90 km downstream. This spill introduced high nitrogen and phosphorous loads of 92.1 mg/L and 6 mg/L as well as caused dense phytoplankton blooms, and Clostridium perfringens was detected in the range of 40,000 CFU/mL (Mallin et al., 1997). This spill consisted of conventional poultry processing waste and wastewater (Pellow, 2004).
With growing environmental and economic pressures, conventional poultry processing facilities will need to seek alternatives to their water consumption such as water systems that incorporate reuse water. By reusing water, economic concerns may be abated, while cleaning the water on-site should mitigate environmental damage.
Reuse of Processing Water
Reuse of water is defined as recovery from a processing step, including from the food matrices, its reconditioning treatment, if applicable; and its subsequent use in a food manufacturing operation (Meneses et al., 2017). The reuse of water has been approved in poultry production systems provided that critical control points are identified, and the water be equivalent to potable, or drinkable, water from a safety standpoint (Codex Alimentarius, 2007; United States Environmental Protection Agency, 2012; Sanitation Performance Standards Compliance Guide, 2016). Typically including sensory, chemical, and microbiological characteristics, water reuse regulations are determined at the state level in the U.S. with guidelines provided by the USDA and the Environmental Protection Agency (EPA) (AWWA, 1996; Sanitation Performance Standards Compliance Guide, 2016; Meneses et al., 2017; United States Environmental Protection Agency, 2018). One specific guideline, 9 CFR 416.2 (g) (3), states that to use water more than once for the same purpose, measures must be taken to reduce physical, chemical, and microbiological contamination to prevent adulteration of the finished product (Sanitation Performance Standards Compliance Guide, 2016).
These contaminants vary depending on the source and use of the water and can be found in the Sanitation Performance Standards Compliance Guide. Typically, these adulterants include oils, proteins, macroparticles, organic compounds, and bacteria (Meneses et al., 2017). Sanitation standards of poultry reuse water and other regulated waters in the U.S. are detailed in Table 1. In poultry reuse waters, total bacterial counts are limited to < 500 colony forming units (CFU) /mL (Casani et al., 2005; Sanitation Performance Standards Compliance Guide, 2016). There is also a zero tolerance for fecal coliforms, Salmonella, and Staphylococcus aureus (Casani et al., 2005; Sanitation Performance Standards Compliance Guide, 2016). Furthermore, turbidity is a physical parameter that is considered when contamination is present and measured with a nephelometer that observes light scattering. In the reuse of processing water, it must not exceed 5 Nephelometric Turbidity Units (NTU's) which is the maximum turbidity suggested for drinking water as set by the World Health Organization (World Health Organization, 2011). Closely related to this physical parameter is the total solid load which impacts how much light scattering occurs. Chemical parameters that must be met include nitrogen and biological and chemical oxygen demand (BOD and COD) which must comply with state requirements (Casani et al., 2005; Sanitation Performance Standards Compliance Guide, 2016). An additional guideline provided in the Code of Federal Regulations, states that reconditioning equipment must be approved and a 60% reduction in total bacterial load must be observed. Furthermore, light transmission, which is indicative of physical contamination, must be at least 60% of potable water used in the same process (Sheldon and Brown, 1986; Saravia et al., 2005).
To follow these guidelines while maintaining quality and safety throughout processing, several techniques have been employed to reduce upstream contamination including physical and chemical treatments (Meneses et al., 2017). The use of these treatments within the food industry has been documented in Meneses et al. (2017) and Casani et al. (2005). Physical methods, for example, filtration systems are often utilized to reduce chemical and physical adulterants, while chemical treatments such as chlorine and peracetic acid are added primarily to reduce microbial and pathogen loads (Casani et al., 2005). Each treatment, however, possesses deleterious properties (Stampi et al., 2001; Casani et al., 2005). As a consequence, these conventional treatments will be discussed for potential use in reuse water systems.
Chemical Sanitation Methods
Chlorine
Even before it was discovered as an element in 1809, chlorine was utilized in the textile industry as a bleaching agent. Once its disinfection properties were identified in the 19th century, chlorine was utilized for treatment of water, and in 1881 hypochlorite was shown to be deleterious to bacteria (Wei et al., 1985). Hypochlorite, a liquid, was soon utilized to reduce exposure to chlorine gas but deaths and health problems persisted (Cameron, 1870; Winder, 2001). Globally, chlorine is the most commonly used chemical oxidant for municipal water disinfection (Deborde and Von Gunten, 2008). This is due to its capability to kill microorganisms as well as serve as a taste and odor control (Hoff and Geldreich, 1981; Wolfe et al., 1984). Despite U.S. EPA restrictions of only 4 ppm of chlorine in drinking water, its use as a sanitization method has been shown to be nearly 100% effective against planktonic bacteria when paired with a sand filtration system (United States Environmental Protection Agency, 1992; Dunlop et al., 2002). In municipal wastewater systems chlorine is also utilized as a sanitizer for the disinfection and removal of pathogenic and nonpathogenic bacteria. While this practice has been commonplace in many developed countries, the long-term effects of on the environment of discharging chlorinated waste have not been largely elucidated (NSW Environmental Protection Agency, 2002). It is known that chlorine residues are toxic to aquatic organisms and they tend to persist and bioaccumulate within marine life (NSW Environmental Protection Agency, 2002). Furthermore, all forms of chlorine-based sanitizers are highly corrosive and toxic, and therefore it is encouraged to use the minimum amount of chlorine sanitizer necessary to reduce bacterial loads for each application. One treatment plant in Geneva, New York was able to meet fecal coliform limits, 200 CFU/100 ml, without exceeding 0.25 ppm/L of chlorine in their effluent (NSW Environmental Protection Agency, 2002; Sanders et al., 2013). Other studies have shown 100-fold reductions of enterococci and fecal coliforms in sewage effluents (Tyrrell et al., 1995). The bactericidal effects of chlorine use in municipal water and wastewater are also noted in the food industry.
With the use of chlorine-based products in the food industry not only were pathogenic bacterial levels significantly reduced, but a decrease of microbial slime and spore counts were also observed throughout the processing facilities (Mercer and Somers, 1957; Wei et al., 1985). By neutralizing essential amino acids, and exhibiting other bactericidal mechanisms such as lowering pH, chlorine-based products were considered essential for mid-20th-century food safety (Friberg, 1957; Camper and McFeters, 1979; Foegeding, 1983; Wei et al., 1985). In the fresh produce industry chlorine, ozone, peracetic acid (PAA), and chlorine dioxide (ClO2) have all been utilized to reduce pathogenic bacteria (Banach et al., 2015). Chlorine dioxide (ClO2) in particular has been reported to be sevenfold more effective than chlorine or hypochlorite in poultry chiller water (Lillard, 1980; Tsai et al., 1995). It has also been shown to reduce Salmonella incidence in chicken with a concentration as low as 5 ppm, compared to hypochlorite recommended use at 50–100 ppm (Lillard, 1980; Tsai et al., 1995; Casani and Knøchel, 2002). Sodium hypochlorite and other chlorine-based sanitizers function by saponifying fatty acids and glycerol creating byproducts such as chloramines which in turn convert amino acids into forms that antagonize cellular metabolism (Estrela et al., 2002). Acidified sodium chlorite has also been shown to be an effective antimicrobial, but must be in an acidic environment (pH 2.5–3.2), which can be generated by using citric acid (Warf, 2001; Allende et al., 2009).
Despite the efficacy of chlorine-based products as sanitizers, they are being phased out from many food production systems and are banned in poultry in the European Union since 1997 (Casani and Knøchel, 2002; Johnson, 2015). Several studies have identified chlorine resistance in Gram-positive bacteria and microorganisms in biofilms (Patterson, 1968; Bolton et al., 1988; Ryu and Beuchat, 2005). Biofilms are bacterial communities that live commensally and attach to surfaces and each other (Costerton, 1995). These biofilms increase the resistance of their residential bacterial cells to antibiotics and environmental stressors such as sanitizers (Kumar and Anand, 1998; Frank et al., 2003; Ryu and Beuchat, 2005). Furthermore, while microbial slimes can be removed in the presence of high concentrations of chlorine, it has relatively low activity on microorganisms harbored within the biofilms (Scher et al., 2005; Deborde and Von Gunten, 2008). Salmonella enterica serovar Typhimurium, when subjected to 50 ppm of sodium hypochlorite for 15 min, was completely reduced below the limits of detection (<10 CFU/mL) from an initial concentration of 1* 108 CFU/mL (Scher et al., 2005). However, when sodium hypochlorite was applied to a pellicle of Salmonella, an air-liquid biofilm, less than a 1 log CFU reduction was observed (Scher et al., 2005). At 250 ppm of sodium hypochlorite, only a 4 log CFU reduction was observed (Scher et al., 2005). This clear increase in resistance encourages higher chlorine use, but this can become dangerous to workers.
The threshold for chlorine gas that should never be exceeded is 1 ppm and is set by the Occupational Safety and Health Administrations (OSHA) Permissible Exposure Limit (PEL) (Occupational Safety and Health Administration, 2017a). If exceeded, chlorine gas can damage the lungs causing difficulty in breathing, coughing, throat irritation, impaired sense of smell, and in extreme cases chronic issues such as bronchitis, emphysema, and permanent pulmonary damage (Winder, 2001). Chlorine can also cause chemical burns and be an irritant in the case of skin or eye contact. An additional concern with chlorine use is that in the presence of high organic matter free chlorine forms trihalomethanes, such as chloroform, which pose a significant danger to plant workers and equipment (Tsai et al., 1992; Fawell, 2000; Casani et al., 2005). The corrosion rate of chlorine depends on a number of factors, mainly chlorine concentration and material (Tuthill et al., 1998; Nielsen et al., 2000). Cast iron, even at low concentrations of chlorine (< 2 mg/L) degrades by 0.1 mm/year, whereas stainless steel (SS) corrosion is insignificant at low concentrations except for localized buildup areas (Tuthill et al., 1998). However, at higher concentrations (5–20 mg/L) many types of SS alloys were not resistant to corrosion, and specific types of SS such as Types 304 and 316 are needed to withstand long exposures to high concentrations of chlorine (Tuthill et al., 1998). This can factor into the cost and be a detriment to poultry processing.
Furthermore, the by-product chloroform is considered carcinogenic, and its inhalation can impair the kidneys and cause liver necrosis (Occupational Safety and Health Administration, 2017a). Poultry processing water, in general, contains a high level of organic material that reacts with chlorine to produce these by-products and this is a concern in the use and reuse of processing water when sanitizing with chlorine or chlorine dioxide (Casani et al., 2005; Northcutt et al., 2008; White, 2010; Meneses et al., 2017). These health concerns have led to regulations limiting reused chiller water to have no more than 5 ppm of free available chlorine, which can be measured with a test kit or amperometric titration system (Tsai et al., 1992; United States Department of Agriculture, 2003b; Northcutt et al., 2008).
Chlorine-based sanitizers are also sensitive to temperature, pH, and residence time within the chiller water. As pH increases from pH 7.0 to 8.5 to 9.8 to 10.7, the killing power of chlorine against E. coli decreases, while raising temperature generally increases lethality (Butterfield et al., 1943; Nagel et al., 2013). However, this may result from an increase in temperature of the wash also reducing aerobic microbial populations independent of chlorine presence (Kelly et al., 1981). Due to the organic load within chillers, the bactericidal effects of chlorine sanitizers were found to be extremely low after 5 min of treatment (Tsai et al., 1992; Oyarzabal, 2005). It was determined that for a chiller tank with total dissolved solids of 3,500 ppm over 400 ppm of chlorine would be needed to saturate the demand from organic compounds which react with chlorine and produce by-products that are hazardous and ineffective as bactericidal agents (Tsai et al., 1992). Within this chiller tank, there was no available free chlorine, the agent of sanitation, after 30 min of chlorination with concentrations up to 300 ppm (Tsai et al., 1992). This was determined through a colorimetric assay. As a consequence, to utilize chlorine effectively as a sanitizer under these conditions, especially in the presence of high organic loads, it must be regulated and these physical and chemical characteristics can fluctuate in reuse water systems on a day to day basis (Mead and Thomas, 1973; Northcutt et al., 2008; White, 2010). Due to these issues, poultry processing water, increasingly being treated with peracetic acid as a sanitizer instead of chlorine.
Peracetic Acid and Hydrogen Peroxide
By breaking down into acetic acid, water, and oxygen, PAA has been considered a safer alternative to chlorine and is currently approved for poultry processing water use by the U.S. Food and Drug Administration (FDA) at up to 2,000 ppm (21 CFR 173.370) (FCN No. 1465) (Warburton, 2014; Kim et al., 2017). Studies show PAA is effective against Salmonella and Campylobacter at a concentration of 20 and 200 ppm, respectively, and this is likely achieved through membrane oxidation and acidifying the water (Oyarzabal, 2005; Bauermeister et al., 2008). While the mechanism of disinfection is debated, PAA is believed to function by disrupting enzymatic activity through the binding and reacting with sulfur and double bonds, which denatures proteins (Block, 1991; Lefevre et al., 1992; Liberti et al., 1999; Kitis, 2004). It has also been suggested PAA may disrupt intracellular solute concentrations and impair bacterial cell replication as well as inactivate catalase, which breaks down toxic hydroxyl radicals (Block, 1991; Lubello et al., 2002). Unlike chlorine, PAA is not significantly inhibited by a high organic load, and this has made it a popular alternative in poultry processing (Lillard, 1979; Casani et al., 2005; McKee, 2011). However, in the presence of highly concentrated organic matter the rate of disinfection may be impacted (Gehr and Cochrane, 2002; Gehr et al., 2003). At 200 ppm PAA in chiller water did not influence the sensory characteristics of poultry products including flavor appearance or product acceptability (Bauermeister et al., 2008). Hydrogen peroxide used in combination with peracetic acid has been shown to be beneficial as a synergistic sanitizer improving peracetic acid reductions of Escherichia coli, Staphylococcus aureus, and Pseudomonas aeruginosa (Alasri et al., 1992; McKee, 2011). Hydrogen peroxide (H2O2) is an intermediary in the degradation of peracetic acid into acetic acid, but H2O2 also has some antimicrobial capacity (Wagner et al., 2002; Bauermeister et al., 2008). However, in the municipal effluent, concentrations of 106 to 285 mg/L of H2O2 were required to sufficiently reduce fecal coliforms compared to 0.6–1.6 mg/L of PAA (Wagner et al., 2002). Peracetic acid functions similarly to other peroxides in that it releases active oxygen that oxidizes double bonds, sulfur bonds, and sulfhydryl groups of proteins and enzymes that are located intra- and intercellularly (Block, 1991; Kitis, 2004). The sanitation effect of H2O2 can be improved by the addition of PAA as the inactivation of catalase improves the persistence of H2O2 (Block, 1991; Kitis, 2004). This effect, along with the equilibrium reached between H2O2 and PAA in solution means that these chemicals are often paired to achieve synergistic effects.
The PAA-based sanitizers are used in poultry processing systems reuse water and can also be combined with diatomaceous earth filtration to improve safety (Casani et al., 2005; Lo et al., 2005). However, peracetic acid is corrosive to equipment and can be caustic to exposed skin, eyes and respiratory systems of personnel handling these materials (Casani et al., 2005; Peracetic acid. MSDS No, 2013). Furthermore, the potential for microrganisms to recover from the sanitization treatment and grow may exist due to the decomposition of PAA into acetic acid (Stampi et al., 2001; Kitis, 2004). This rapid decomposition can result in a short shelf life of a few days to weeks if not refrigerated or placed away from light (Deshpande et al., 2013, 2014; Walsh et al., 2018).
Ozone
While some chemical sanitizers are considered corrosive ozone can be utilized as a sanitizer without corrosive damage to equipment (Guzel-Seydim et al., 2004). Ozone (O3) is generated when oxygen molecules are subjected to high voltage (Horvath et al., 1985). It has been utilized in water disinfection of swimming pools, wastewater treatment plants, and in bottled water production (Rice et al., 1981; Legeron, 1982; Guzel-Seydim et al., 2004). Ozone solubility depends on pH, temperature, ozone bubble size, and water purity, which are difficult to regulate in reuse water systems (Rice et al., 1981). Typically, at 27°C, 270 mL of ozone can be dissolved into a liter of pure water (pH 7.00) (Rice et al., 1981). Water treated with ozone has also been used to chill poultry carcasses with no changes in sensory characteristics (Sheldon and Brown, 1986; Casani et al., 2005). The FDA has affirmed the GRAS status of ozone as a sanitizer but only if the provided applications and concentrations utilized are in agreement with good manufacturing practices (Graham, 1997; Food Drug Administration, 1999; Oyarzabal, 2005).
Ozone has been approved as a sanitizer to reuse poultry chiller water (Kim et al., 1999). Like chlorine, ozone oxidizes critical bacterial membrane proteins, but ozone possesses 1.5 times higher oxidizing effect compared to chlorine (Xu, 1999; Oyarzabal, 2005). This oxidative potential allows for the attack of bacterial membrane glycoproteins and glycolipids, which causes membrane permeability and lysis (Khadre and Yousef, 2001; Guzel-Seydim et al., 2004). Ozone can also decrease pH. For example, after 50 min of ozonation Sheldon and Chang (1987) observed a decreased chiller water pH from 6.9 to 5.6. To generate ozone on a commercial level the corona discharge method is utilized, which splits diametric oxygen into free radicals that, in turn, react with available diametric oxygen to form ozone (Rice et al., 1981). Depending on the system 8–16 kWh is required to generate 1 kg of ozone, and this is achieved by utilizing high and low-tension electrodes separated by a dielectric medium with a narrow discharge gap (Rice et al., 1981). When sufficient kinetic energy exists within the excited electrodes collision occurs through the narrow gap interacting with oxygen to form ozone and produces a 1–3% ozone mixture (Rice et al., 1981). Unlike chlorine, ozone's killing potential is not as drastically impacted by the presence of organic material, and ozone decomposes into nontoxic residues (Restaino et al., 1995; Graham, 1997; Xu, 1999). This effect has been utilized to reduce pathogenic Escherichia coli, Listeria monocytogenes, Pseudomonas spp., Bacillus spp., Salmonella spp., and yeasts (Restaino et al., 1995; Guzel-Seydim et al., 2004; Almeida and Gibson, 2016). By using a recirculating ozone reactor, Restaino et al. (1995) tested the efficacy of 0.188 mg/L of ozone (concentration determined at the outlet) against 106 CFU or spores/mL of several pathogens. The authors reported, at time zero, 5 log reductions of S. Typhimurium and E. coli along with more than a 4 log reduction of Listeria, and 3 log reductions of B. cereus and P. aeruginosa, but only a 1 log reduction in Aspergillus niger (CFU/mL) (Restaino et al., 1995). Using a dipper well ozone sanitation system, a 5 log reduction of E. coli and Listeria innocua was observed at 30 s after exposure to 0.45–0.55 ppm of residual ozone in Almeida and Gibson (2016). In Fabrizio et al. (2002), carcass surfaces were inoculated with Salmonella and allowed to attach, allowing for 3 log CFU/mL of rinsate (Fabrizio et al., 2002). These pathogen-inoculated carcasses were added to an immersion chiller at 4°C with ozone (10 mg/L) (Fabrizio et al., 2002). This led to 0.75 log CFU/mL reduction before storage and a 1 log CFU/mL reduction after storage but required pre-enrichment with tetrathionate to be detectable (Fabrizio et al., 2002). Ozone also reduced aerobic plate counts by 0.5 log CFU/mL which was statistically significant compared to all other treatments tested by Fabrizio et al. (2002). This was compared to 20 ppm of chlorine which was reported to be less effective with no immediate reduction and a 0.25 log CFU/mL reduction of Salmonella before and after storage in at 4°C, respectively (Fabrizio et al., 2002). These low reductions were likely due to the low level of Salmonella inoculation. Comparatively, a 1 h ozone treatment has been shown by Selma et al. (2008) to reduce the microbiota populations of vegetable wash water by 5.9 log CFU/mL.
Regardless of these bacterial reductions, there are several drawbacks to utilizing ozone as a sanitizer such as human health concerns, instability, and degradation (Hoof, 1982; Kim et al., 1999; Fabrizio et al., 2002; Casani et al., 2005). Ozone primarily affects the respiratory tract in humans and generates headaches, dizziness, and a burning sensation in the eyes and throat (Hoof, 1982; Guzel-Seydim et al., 2004). When exposed to chronic toxicity, memory can be affected, and an increased prevalence of bronchitis and muscular excitability can be observed (Hoof, 1982). Due to these effects, OSHA guidelines state that levels are not to exceed 0.1 ppm of air for 8 h of light work and no more than 0.05 ppm for heavy work, where the definitions of light and heavy workloads are defined by Occupational Safety and Health Administration (2017a,b). Additionally, while from a health perspective rapid degradation of ozone is beneficial, utilizing the product as a sanitizer can be difficult when considering its short half-life of 2–165 min (Wynn et al., 1973; Wickramanayake, 1984; Khadre et al., 2001). The degradation rate of aqueous ozone is dependent on alkalinity, mechanical stirring, water impurities, and temperature (Hill and Rice, 1982; Khadre et al., 2001). For instance, while ozone's half-life at 20°C is 20–30 min a shorter half-life of 2–4 min was observed at pH 7.0 and 25°C while stirring occurred (Wynn et al., 1973; Wickramanayake, 1984). At pH 9.0, no ozone was detected by Kim (1998) while lower pH levels appeared to be more favorable to ozone stability.
Independent of these factors, this degradation forces on-site generation of ozone, which may be a potential practical obstacle for some poultry processing operations (Pryor and Rice, 1999; Fabrizio et al., 2002. Furthermore, while organic loads found in chiller tanks have been shown not to impact ozone initial killing potential, 20 ppm of bovine serum albumin, a protein concentrate, significantly impacts the stability of ozone which reduces its killing potential over time (Horvath et al., 1985; Restaino et al., 1995). As a consequence of these negative effects, ozone has been investigated more as a synergistic sanitizer rather than a standalone application (Khadre et al., 2001). Since ozone disrupts membrane permeability, combining it with chlorine allows for deleterious effects on parasites such as Cryptosporidium parvum (Gyurek et al., 1996; Khadre et al., 2001). This occurs by denaturation of membrane proteins due to the oxidation potential of ozone, which eventually leads to leakage and cell death (Zhang et al., 2011). Ozone has been utilized along with filtration in poultry chiller water and reduced microbial counts by 99% and have been shown by Unal et al. (2001) to inactivate E. coli and L. monocytogenes when utilized with a pulse electrified field (Sheldon and Brown, 1986).
The potential for ozone as an independent sanitizer or synergistic sanitizer is promising. However, while significant log reductions of pathogens in poultry chiller water have been indicated, there are concerns with worker safety and the need for an on-site generation due to ozone's short half-life. As such, alternative sanitizers still need to be investigated to achieve further food safety improvements.
Sodium Bisulfate
The advantages of chlorine, peracetic acid, and ozone, are clear as they can greatly reduce microbial loads and do not alter product quality if used in low-dose quantities resulting in their widespread use in poultry processing (Wei et al., 1985; Gehr et al., 2003; Northcutt and Jones, 2004; Casani et al., 2005). However, these sanitizers can be corrosive, dangerous to workers, difficult to transfer in large quantities, and ineffective against biofilms in low doses. Sodium bisulfate may be an alternative solution as it is a dry acid which is soluble in water and is generally regarded as safe (GRAS, 21 CFR 582.1095) (United Stated Department of Agriculture., 2015; Jones-Hamilton., 2018). Recently, SBS has been listed as a safer choice as an antimicrobial and processing aid by the Environmental Protection Agency (United States Environmental Protection Agency, 2018). Sodium bisulfate can be transported as a granular solid. It dissociates into nontoxic sodium, hydrogen, and sulfate ions and has a pKa of 1.99 (Sun et al., 2008). It also possesses the ability to lower the pH of water without producing off flavors in finished products (Sun et al., 2008). Based on the U.S. National Fire Protection Agency (NFPA) code 704, sodium bisulfate is a class 2 health hazard where intense or continued exposure could cause injury, compared to PAA and hypochlorite, which are class 3 health hazards that could cause serious temporary or moderate residual injury on brief exposure (Sodium bisulfate. MSDS No., 2013; United Stated Department of Agriculture., 2015). Approved as a food ingredient and general purpose animal feed additive, SBS has wide range of applications including use in beverages, soups, dressings, ready-to-eat meals, vegetables, fruits, pet food, poultry feed, and in drinking water (Sun et al., 2008; Calvo et al., 2010; Kassem et al., 2012; Kim et al., 2018). Sodium bisulfate is especially attractive to alternative processing facilities as it is considered “natural” according to the FDA and the International Association of Natural Product Producers (IANPP) (Kim et al., 2018).
Sodium bisulfate has several potential properties that would make it an ideal alternative sanitizer, and while limited, there is information regarding its use as a sanitizer in the food industry. Campylobacter levels were reduced by 2 to 3 log over a 6 week period when 1.13 or 1.81 kg SBS was applied to 4.6 m2 poultry litter (Line, 2002). In SBS was effective in reused poultry processing water by reducing 1.5e8 CFU/100 mL of Salmonella to below the limit of detection, which was not accomplished by 200 ppm PAA. Dittoe et al. (2018) also demonstrated a 2 log reduction of Salmonella on poultry carcass rinses. To reduce S. Typhimurium on chicken carcasses 10% SBS solution was sprayed reducing concentrations by 2.4 logs (Yabin et al., 1997). Total aerobic microbial populations were decreased by 1.61 logs, and Salmonella counts were reduced by 2 logs when 5% SBS was utilized in the inside-outside bird washer (Yang et al., 1998). However, at this concentration slight discoloration was observed on the chicken carcass (Yang et al., 1998). The antimicrobial efficacy of SBS has also been tested on apples. When washed with a 1% solution of SBS and 60 ppm PAA reduced Listeria innocua by 5 logs after seven days storage compared to 150 ppm of chlorine which had only a 3.5 log reduction (Kim et al., 2018). When the treatment was increased to a 3% SBS solution with 60 ppm PAA, the 5 log reduction was also observed at day 14 (Kim et al., 2018). This bactericidal activity on apples corroborates findings in Fan et al. (2009) which demonstrated reduced total aerobic plate counts by SBS on apple slices. Further investigations into SBS efficacy as sanitizers must be performed, but due to its ability to be transported as a solid, and its relative safe use, there is noted interest on its use in poultry processing reuse water (Micciche et al., 2018).
Physical Treatment—Filtration
Despite the efficacy in reducing microbial populations chemical sanitizers alone are not sufficient in treating processing water. This is because there is a need to remove debris, dissolved solids, blood, fat, tissue, fecal matter, and other particulates that collect in the water (Sanitation Performance Standards Compliance Guide, 2016). In order to remove these dissolved solids, filtration systems may be utilized, and these could also assist in reducing microbial loads.
Filtration systems can be broken into three types, macro-, micro-, and ultrafiltration (Saravia et al., 2005). Unlike chemical treatments, filtration systems can concentrate and remove oils, macrosolutes, and organic compounds (Meneses et al., 2017). Macrofiltration systems collect particles 5 μm in size and are not suitable for bacterial concentrates, where microfiltration typically picks up particles up to a tenth of a micron in size (Saravia et al., 2005). Ultrafiltration can concentrate particles with a molecular weight >1,000 leaving only low molecular weight organic solutes and salts (Lo et al., 2005; Saravia et al., 2005). This is accomplished by utilizing pressures up to 145 psi and selective fractionation (Lo et al., 2005; Saravia et al., 2005). Ultrafiltration has been found to reduce 85% of total solids and 95% of chemical oxygen demand of poultry processing wastewater (Shih and Kozink, 1980; Avula et al., 2009). This conditioning has been utilized on chiller overflow and produced chemical compositions similar to tap or potable water (Mannapperuma and Santos, 2004; Avula et al., 2009). Mannapperuma and Santos (2004) designed a spiral membrane ultrafiltration system to recondition poultry overflow water. It was found that 480 L/min could be processed with an 80% reuse of water in this system. This would allow for 346 L/min of freshwater to be replaced and it was determined with an initial capital cost of $300,000 a full-scale processing facility would see a 2.4 year simple payback period for their investment. In a pilot processing plant, it was estimated $60,000 of savings for a typical processing plant per year could be obtained if chiller reuse water system with ultrafiltration would be implemented. Savaria et al. (2005) detailed the economic feasibility of ultrafiltration reuse water systems and found them feasible for large-scale poultry production plants even when factoring in the 5-year and 20-year life expectancy for the membrane and the unit, respectively (Saravia et al., 2005). They also estimated over 600,000 L of water per day could be recycled through this process when only considering reusing chiller water (Savaria et al., 2005; Avula et al., 2009).
Despite these potential advantages several hurdles remain before filtration and reuse water systems become commonplace in poultry processing plants. For example, due to the high startup cost, filtration units may not be applicable to smaller processing facilities or pilot plants. An additional concern lies with the potential for organisms to survive on filtration concentrates and form biofilms which may be a worker safety concern when removing the filtrate buildup (Drozd and Schwartzbrod, 1997; Casani et al., 2005). To remedy this concern, some of the previously discussed chemical sanitizers are often used with filtration to ensure reduction of microorganisms and chemical contamination (Chang et al., 1989; Northcutt and Jones, 2004; Casani et al., 2005). For instance, Lo et al. (2005) utilized chlorine resistant (up to 50 ppm) filters and were able to reduce COD below 200 mg/L while recovering crude protein by-products. Lillard (1979) investigated broiler necks submerged in chlorinated chiller water which had been passed through a diatomaceous earth filter. The conclusion was drawn that, by using these two sanitization methods, water did not have to be potable to maintain quality (Lillard, 1979). Regarding microbial load, neither total aerobic counts, fecal coliforms, nor Salmonella populations were significantly different between reused chlorinated, filtered chiller water and potable water (Lillard, 1979). However, with the aforementioned issues with traditional chemical sanitizers, alternative treatments should also be investigated for independent use or in combination with conventional sanitization methods in reuse water.
Conclusions
With environmental and financial pressures growing, the commercial poultry industry like the rest of the food industry must consider alternative measures in the near future to conserve one of the earth's most precious resource (Meneses et al., 2017). Reuse water systems have been demonstrated to be effective in traditional processing plants (Andelman and Clise, 1977; Casani et al., 2005). With food safety in mind, proper sanitation is required to avoid cross-contamination. These sanitizers may result in the necessity of using caustic, corrosive, and potentially dangerous chemicals which may not be cost-effective or viable for alternative production systems (Casani et al., 2005). As such, additional sanitizer sources must be developed and further investigated to improve food and operator safety. The organic poultry processing industry may have insights into these sources. Bacteriocins, essential oils, and bacteriophages, have been investigated in this alternative poultry processing market and with further experimentation may be found useful for conventional poultry processing (Sirsat et al., 2009; Calo et al., 2015). Sanitizer stability and interaction with other components in the poultry processing water would be of particular interest in reuse systems to determine their long-term viability. Once viable, alternative sanitizers implemented with reuse water systems in conventional poultry processing may be a part of the solution to water scarcity.
Author Contributions
All authors listed have made a substantial, direct and intellectual contribution to the work, and approved it for publication.
Conflict of Interest Statement
CK is employed by the company Jones-Hamilton. The remaining authors declare that the research was conducted in the absence of any commercial or financial relationships that could be construed as a potential conflict of interest.
Acknowledgments
AM is supported by a Distinguished Doctoral Fellowship and support from the Department of Food Science at the University of Arkansas. Jones-Hamilton Co. is acknowledged for its support and assistance.
References
Alasri, A., Roques, C., Michel, G., Cabassud, C., and Aptel, P. (1992). Bactericidal properties of peracetic acid and hydrogen peroxide, alone and in combination, and chlorine and formaldehyde against bacterial water strains. Can. J. Microbiol. 38, 635–642. doi: 10.1139/m92-104
Alcamo, J., Döll, P., Kaspar, F., and Siebert, S. (1997). Global change and global scenarios of water use and availability: an application of Water GAP 1.0. Center for Environmental Systems Research (CESR), University of Kassel.
Alcamo, J., Henrichs, T., and Rösch, T. (2000). World Water in 2025: Global Modeling and Scenario Analysis for the World Commission on Water for the 21st Century. Center for Environmental Systems Research (CESR), University of Kassel.
Allende, A., McEvoy, J., Tao, Y., and Luo, Y. (2009). Antimicrobial effect of acidified sodium chlorite, sodium chlorite, sodium hypochlorite, and citric acid on Escherichia coli O157: H7 and natural microflora of fresh-cut cilantro. Food Control. 20, 230–234. doi: 10.1016/j.foodcont.2008.05.009
Almeida, G., and Gibson, K. E. (2016). Evaluation of a recirculating dipper well combined with ozone sanitizer for control of foodborne pathogens in food service operations. J. Food Prot. 79, 1537–1548. doi: 10.4315/0362-028X.JFP-16-055
Andelman, J. B., and Clise, J. D. (1977). “Water reuse of wastewater from a poultry processing plant,” in Proceedings of the 8th National Symposium on Food Processing Wastes, EPA-600/2-77-184 (US Environmental Protection Agency), 389.
Avula, R. Y., Nelson, H. M., and Singh, R. K. (2009). Recycling of poultry process wastewater by ultrafiltration. Innov. Food Sci. Emerg. Tech. 10, 1–8. doi: 10.1016/j.ifset.2008.08.005
AWWA (1996). Water Reuse. American Water Works Association (AWWA) Government Affairs. AWWA MainStream. Available online at: http://www.awwa.org.
Banach, J., Sampers, I., Van Haute, S., and Van der Fels-Klerx, H. J. (2015). Effect of disinfectants on preventing the cross-contamination of pathogens in fresh produce washing water. Int. J. Environ. Res. Public Health 12, 8658–8677. doi: 10.3390/ijerph120808658
Bashor, M. P., Curtis, P. A., Keener, K. M., Sheldon, B. W., Kathariou, S., and Osborne, J. A. (2004). Effects of carcass washers on Campylobacter contamination in large broiler processing plants. Poult. Sci., 83, 1232–1239. doi: 10.1093/ps/83.7.1232
Bauermeister, L. J., Bowers, J. W. J., Townsend, J. C., and McKee, S. R. (2008). The microbial and quality properties of poultry carcasses treated with peracetic acid as an antimicrobial treatment. Poult. Sci., 87, 2390–2398.
Beekman, G. B. (1998). Water conservation, recycling and reuse. Water Res. Dev. 14, 353–364. doi: 10.1080/07900629849268
Block, S. S. (1991). Disinfection, Sterilization and Preservation. 4th ed. Philadelphia, PA: Lea & Febiger.
Bolton, K. J., Dodd, C. E. R., Mead, G. C., and Waites, W. M. (1988). Chlorine resistance of strains of Staphylococcus aureus isolated from poultry processing plants. Lett. Appl. Microbiol. 6, 31–34.
Butterfield, C. T., Wattie, E., Megregian, S., and Chambers, C. W. (1943). Influence of pH and temperature on the survival of coliforms and enteric pathogens when exposed to free chlorine. Public Health Reports 58, 1837–1866.
Calo, J. R., Crandall, P. G., O'Bryan, C. A., and Ricke, S. C. (2015). Essential oils as antimicrobials in food systems–a review. Food Control, 54, 111–119. doi: 10.1016/j.foodcont.2014.12.040
Calvo, M. S., Gerry, A. C., McGarvey, J. A., Armitage, T. L., and Mitloehner, F. M. (2010). Acidification of calf bedding reduces fly development and bacterial abundance. J. Dairy Sci.93, 1059–1064. doi: 10.3168/jds.2009-2797
Camper, A. K., and McFeters, G. A. (1979). Chlorine injury and the enumeration of waterborne coliform bacteria. Appl. Environ. Microbiol. 37, 633–641.
Casani, S., and Knøchel, S. (2002). Application of HACCP to water reuse in the food industry. Food Control. 13, 315–327. doi: 10.1016/S0956-7135(02)00037-3
Casani, S., Rouhany, M., and Knøchel, S. (2005). A discussion paper on challenges and limitations to water reuse and hygiene in the food industry. Water Res., 39, 1134–1146. doi: 10.1016/j.watres.2004.12.015
Chang, S. Y., Toledo, R. T., and Lillard, H. S. (1989). Clarification and decontamination of poultry chiller water for recycling. Poult. Sci. 68, 1100–1108. doi: 10.3382/ps.0681100
Codex Alimentarius (2007). Code of Hygienic Practice for Eggs and Egg Products CAC/ RCP 15 e 1976. Available online at: http://www.fao.org/docrep/012/i1111e/i1111e01.pdf (Accessed April 23, 2018).
Costerton, J. W. (1995). Overview of microbial biofilms. J. Ind. Microbiol. 15, 137–140. doi: 10.1007/BF01569816
Deborde, M., and Von Gunten, U. R. S. (2008). Reactions of chlorine with inorganic and organic compounds during water treatment—kinetics and mechanisms: a critical review. Water Res. 42, 13–51. doi: 10.1016/j.watres.2007.07.025
Department of Agriculture Water Resources (2007). Australian Food Statistics 2007.Available online at: http://www.agriculture.gov.au/ag-farm-food/food/publications/afs/australian_food_statistics_2007 (Accessed April 23, 2018).
Deshpande, A., Mana, T. S., Cadnum, J. L., Jencson, A. C., Sitzlar, B., Fertelli, D., et al. (2014). Evaluation of a sporicidal peracetic acid/hydrogen peroxide–based daily disinfectant cleaner. Infect. Cont. Hosp. Epidemiol. 35, 1414–1416. doi: 10.1086/678416
Deshpande, A., Mana, T. S. C., Cadnum, J. L., Sitzlar, B., Fertelli, D., Kundrapu, S., et al. (2013). “Effectiveness of spray application of a novel sporicidal disinfectant cleaner,” in Paper Presented at ID Week 2013 (Philadelphia, PA).
Dewey-Mattia, D., Manikonda, K., Hall, A. J., Wise, M. E., and Crowe, S. J. (2018). Surveillance for Foodborne Disease Outbreaks—United States, 2009–2015. MMWR Surveill. Summ. 67:1. doi: 10.15585/mmwr.ss6710a1
Dittoe, D. K., Atchley, J. A., Feye, K. M., Knueven, C. J., and Ricke, S. C. (2018). “Effect of sodium bisulfate salt on mitigating the presence of an antibiotic resistant strain of Salmonella Enteritidis on chicken drug sticks,” in Poultry Science Association Annual Meeting. San Antonio, TX.
Drozd, C., and Schwartzbrod, J. (1997). Removal of Cryptosporidium from river water by crossflow microfiltration: a pilot-scale study. Water Sci. Technol. 35, 391–395.
Dunlop, P. S. M., Byrne, J. A., Manga, N., and Eggins, B. R. (2002). The photocatalytic removal of bacterial pollutants from drinking water. J. Photochem. Photobiol. 148, 355–363. doi: 10.1016/S1010-6030(02)00063-1
Edzwald, J. K., and Tobiason, J. E. (1999). Enhanced coagulation: US requirements and a broader view. Water Sci. Technol. 40, 63–70. doi: 10.2166/wst.1999.0444
Estrela, C., Estrela, C. R., Barbin, E. L., Spanó, J. C. E., Marchesan, M. A., and Pécora, J. D. (2002). Mechanism of action of sodium hypochlorite. Braz. Dent. J. 13, 113–117. doi: 10.1590/S0103-64402002000200007
Fabrizio, K. A., Sharma, R. R., Demirci, A., and Cutter, C. N. (2002). Comparison of electrolyzed oxidizing water with various antimicrobial interventions to reduce Salmonella species on poultry. Poult. Sci. 81, 1598–1605. doi: 10.1093/ps/81.10.1598
Fan, X., Sokorai, K. J., Liao, C. H., Cooke, P., and Zhang, H. Q. (2009). Antibrowning and antimicrobial properties of sodium acid sulfate in apple slices. J. Food Sci. 74:9. doi: 10.1111/j.1750-3841.2009.01362.x
Fawell, J. (2000). Risk assessment case study—chloroform and related substances. Food Chem. Toxicol. 38, S91–S95. doi: 10.1016/S0278-6915(99)00129-5
Fonkwe, L. G., Singh, R. K., and Lee, J. H. (2001). Effect of recycling on the protein recovery process using ultrafiltration. Korean Soc. Food Eng. 253–257.
Food and Drug Administration (1999). Ozone. Code of Federal Regulations 21, section 184.1563. US Government Printing Office, Washington, DC.
Frank, J. F., Ehlers, J., and Wicker, L. (2003). Removal of Listeria monocytogenes and poultry soil-containing biofilms using chemical cleaning and sanitizing agents under static conditions. Food Prot. Trends 23:8.
Friberg, L. (1957). Further quantitative studies on the reaction of chlorine with bacteria in water disinfection. 2. experimental investigations with CI36 and P32. Acta Pathol. et Microbiol. Scand. 40, 67–80.
Gehr, R., and Cochrane, D. (2002). Peracetic acid (PAA) as a disinfectant for municipal wastewaters: encouraging performance results from physicochemical as well as biological effluents. Proc. Water Environ. Feder. 2002, 182–198. doi: 10.2175/193864702785033527
Gehr, R., Wagner, M., Veerasubramanian, P., and Payment, P. (2003). Disinfection efficiency of peracetic acid, UV and ozone after enhanced primary treatment of municipal wastewater. Water Res. 37, 4573–4586. doi: 10.1016/S0043-1354(03)00394-4
Gleick, P. H. (1996). Basic water requirements for human activities: Meeting basic needs. Water Int. 21, 83–92.
Gong, J., Forster, R. J., Yu, H., Chambers, J. R., Sabour, P. M., Wheatcroft, R., et al. (2002). Diversity and phylogenetic analysis of bacteria in the mucosa of chicken ceca and comparison with bacteria in the cecal lumen. FEMS Microbiol. Lett. 208, 1–7. doi: 10.1111/j.1574-6968.2002.tb11051.x
Gremillion (2018). New CDC Report Highlights Role of Chicken in Foodborne Illness. Consumer Federation of America. Available online at: https://consumerfed.org/press_release/new-cdc-report-highlights-role-of-chicken-in-foodborne-illness/ (Accessed September 22, 2018).
Guerin, M., C., Sir, J., Sargeant, L., Waddell, A., O'Connor, R., Wills, R., Bailey, and J., Byrd (2010). The change in prevalence of Campylobacter on chicken carcasses during processing: a systematic review. Poult. Sci. 89, 1070–1084. doi: 10.3382/ps.2009-00213
Guzel-Seydim, Z. B., Greene, A. K., and Seydim, A. C. (2004). Use of ozone in the food industry. LWT-Food Sci. Technol. 37, 453–460. doi: 10.1016/j.lwt.2003.10.014
Gyurek, L. L., Finch, G. R., and Belosevic, M. (1996). “Inactivation of cryptosporidium using ozone and chlorine,” in Proceedings of the Annual Conference on West Canadian Water Wastewater Association, 48th (Regina), 62–69.
Hargis, B. M., Caldwell, D. J., Brewer, R. L., Corrier, D. E., and DeLoach, J. R. (1995). Evaluation of the chicken crop as a source of Salmonella contamination for broiler carcasses. Poult. Sci. 74, 1548–1552.
Hill, A. G., and Rice, R. G. (1982). “Historical background, properties and applications,” in Ozone Treatment of Water for Cooling Application eds R. G. Rice (Ann Arbor, MI: Ann Arbor Science Publishers), 1–37.
Hoekstra, A. Y., and Chapagain, A. K. (2005). “The water footprint of cotton consumption,” in Globalization of Water: Sharing the Planet's Freshwater Resources (Delf: UNESCO-IHE Institute for Water Education), 103–130.
Hoekstra, A. Y., and Chapagain, A. K. (2006). “Water footprints of nations: water use by people as a function of their consumption pattern,” in Integrated Assessment of Water Resources and Global Change (Dordrecht: Springer), 35–48.
Hoff, J. C., and Geldreich, E. E. (1981). Comparison of the biocidal efficiency of alternative disinfectants. J. Am. Water Works Assoc. 73, 40–44. doi: 10.1002/j.1551-8833.1981.tb04636.x
Hoof, F. V. (1982). “Professional risks associated with ozone,” in Ozonation Manual for Water and Waste Water Treatment, eds, W. J. Masschelein (New York, NY: Wiley-Interscience), 200–201.
Horvath, M., Bilitzky, L., and Huttner, J. (1985). Fields of Utilization of Ozone. Ozone. New York, NY: Elsevier Science Publishing Co. Inc.
Jackson, W. C., Curtis, P. A., Carawan, R. E., Keener, K. M., and Taylor M. C. (1999). “Survey shows that poultry processors can save money by conserving water. No. CD-23,” in North Carolina Cooperative Extension Service, Raleigh, NC: NC State University.
Johnson, R. (2015). US-EU Poultry Dispute on the Use of Pathogen Reduction Treatments (PRTs). Washington, DC: Congressional Research Service.
Jones-Hamilton. (2018). Specialty Products of Jones Hamilton. Available online at: http://www.jones-hamilton.com/spd/specialty-products-of-jones-hamilton.html (Accessed May 26, 2018).
Kassem, I. I., Sanad, Y., Stonerock, R., and Rajashekara, G. (2012). An evaluation of the effect of sodium bisulfate as a feed additive on Salmonella enterica serotype Enteritidis in experimentally infected broilers. Poult. Sci., 91:1032e1037. doi: 10.3382/ps.2011-01935
Keener, K. M., Bashor, M. P., Curtis, P. A., Sheldon, B. W., and Kathariou, S. (2004). Comprehensive review of Campylobacter and poultry processing. Compr. Rev. Food Sci. Food Safety 3, 105–116. doi: 10.1111/j.1541-4337.2004.tb00060.x
Kelly, C. A., Dempster, J. F., and McLoughlin, A. J. (1981). The effect of temperature, pressure and chlorine concentration of spray washing water on number of bacteria on lamb carcasses. J. App. Bacteriol. 51, 415–424. doi: 10.1111/j.1365-2672.1981.tb01261.x
Khadre, M. A., and Yousef, A. E. (2001). Sporicidal action of ozone and hydrogen peroxide: A comparative study. Int. J. Food Microbiol. 71, 131–138. doi: 10.1016/S0168-1605(01)00561-X
Khadre, M. A., Yousef, A. E., and Kim, J. G. (2001). Microbiological aspects of ozone applications in food: a review. J. Food Sci. 66, 1242–1252. doi: 10.1111/j.1365-2621.2001.tb15196.x
Kiepper, B. (2003). Characterization of Poultry Processing Operations, Wastewater Generation and Wastewater Treatment Using Mail Survey and Nutrient Discharge Monitoring Methods. MS Thesis, University of Georgia, Athens, GA.
Kim, J. G. (1998). Ozone, as an Antimicrobial Agent in Minimally Processed Foods. Doctoral Dissertation, The Ohio State University
Kim, J. G., Yousef, A. E., and Dave, S. (1999). Application of ozone for enhancing the microbiological safety and quality of foods: a review. J. Food Prot. 62, 1071–1087.
Kim, S. A., Park, S. H., Knueven, C., Basel, R., and Ricke, S. C. (2018). A decontamination approach using a combination of bisulfate of soda and peracetic acid against Listeria innocua inoculated on whole apples. Food Control 84, 106–110. doi: 10.1016/j.foodcont.2017.07.036
Kim, S. A., Park, S. H., Lee, S. I., Owens, C. M., and Ricke, S. C. (2017). Assessment of chicken carcass microbiome responses during processing in the presence of commercial antimicrobials using a next generation sequencing approach. Sci. Rep. 7, 43354. doi: 10.1038/srep43354
Kitis, M. (2004). Disinfection of wastewater with peracetic acid: a review. Environ. Int. 30, 47–55. doi: 10.1016/S0160-4120(03)00147-8
Klemes, J., Smith, R., and Kim, J. K. (Eds.). (2008). Handbook of Water and Energy Management in Food Processing. Elsevier.
Kumar, C. G., and Anand, S. K. (1998). Significance of microbial biofilms in food industry: a review. Int. J. Food Microbiol. 42, 9–27.
Lefevre, F., Audic, J. M., and Ferrand, F. (1992). Peracetic acid disinfection of secondary effluents discharged off coastal seawater. Water Sci. Technol. 25, 155–164.
Legeron, J. P. (1982). “Utilization of ozone in swimming pools,” In Ozonization Manual for Water and Wastewater Treatment, ed, W. J. Masschelein (New York, NY: Wiley-Interscience), 243–247.
Liberti, L., Lopez, A., and Notarnicola, M. (1999). Disinfection with peracetic acid for domestic sewage re-use in agriculture. Water Environ. J. 13, 262–269.
Lillard, H. S. (1979). Levels of chlorine and chlorine dioxide of equivalent bactericidal effect in poultry processing water. J. Food Sci. 44, 1594–1597.
Lillard, H. S. (1980). Effect on broiler carcasses and water of treating chiller water with chlorine or chlorine dioxide. Poult. Sci. 59, 1761–1766.
Line, J. E. (2002). Campylobacter and Salmonella populations associated with chickens raised on acidified litter. Poult. Sci. 81, 1473–1477. doi: 10.1093/ps/81.10.1473
Lo, Y. M., Cao, D., Argin-Soysal, S., Wang, J., and Hahm, T. S. (2005). Recovery of protein from poultry processing wastewater using membrane ultrafiltration. Bioresour. Technol., 96, 687–698. doi: 10.1016/j.biortech.2004.06.026
Lubello, C., Caretti, C., and Gori, R. (2002). Comparison between PAA/UV and H2O2/UV disinfection for wastewater reuse. Water Sci. Technol. 2, 205–212. doi: 10.2166/ws.2002.0025
Luján-Rhenals, D., Morawicki, R., Van Loo, E. J., and Ricke, S. C. (2017). “Energy and water use in poultry processing,” in Achieving Sustainable Production of Poultry Meat. Safety, Quality and Sustainability, Vol. 1, ed S. C. Ricke (Cambridge: Burleigh Dodd Publishing), 389–409, Chapter 19.
Mallin, M. A., Burkholder, J. M., McIver, M. R., Shank, G. C., Glasgow, H. B., Touchette, B. W., et al. (1997). Comparative effects of poultry and swine waste lagoon spills on the quality of receiving streamwaters. J. Environ. Qual. 26, 1622–1631. doi: 10.2134/jeq1997.00472425002600060023x
Mannapperuma, J. D., and Santos, M. R. (2004). Reconditioning of poultry chiller overflow by ultrafiltration. J. Food Process Eng. 27, 497–516. doi: 10.1111/j.1745-4530.2004.00474.x
Maupin, M. A., Kenny, J. F., Hutson, S. S., Lovelace, J. K., Barber, N. L., and Linsey, K. S. (2014). Estimated Use of Water in the United States in 2010 (No. 1405). US Geological Survey.
McKee, S. R. (2011). Salmonella and Campylobacter Control During Poultry Processing. International Poultry Scientific Forum, Atlanta, GA.
Mead, G. G., and Thomas, N. L. (1973). Factors affecting the use of chlorine in the spin-chilling of eviscerated poultry. Br. Poult. Sci. 14, 99–117.
Meneses, Y. E., Stratton, J., and Flores, R. A. (2017). Water reconditioning and reuse in the food processing industry: current situation and challenges. Trends Food Sci. Technol. 61, 72–79. doi: 10.1016/j.tifs.2016.12.008
Mercer, W. A., and Somers, I. I. (1957). “Chlorine in food plant sanitation,” in Advances in Food Research Vol. 7. (Academic Press).
Micciche, A. C., Feye, K. M., Wages, J. A., Knueven, C. J., and, Ricke, S. C., (2018). Impact of Acid Treatments on the Microbial Populations of Commercial Poultry Processing Re-use Water Microcosms. Poultry Science Association Annual Meeting, San Antonio, TX.
Nagel, G. M., Bauermeister, L. J., Bratcher, C. L., Singh, M., and McKee, S. R. (2013). Salmonella and Campylobacter reduction and quality characteristics of poultry carcasses treated with various antimicrobials in a post-chill immersion tank. Int. J. Food Microbiol. 165, 281–286. doi: 10.1016/j.ijfoodmicro.2013.05.016
Nielsen, H. P., Frandsen, F. J., Dam-Johansen, K., and Baxter, L. L. (2000). The implications of chlorine-associated corrosion on the operation of biomass-fired boilers. Progr. Energy Combust. Sci. 26, 283–298. doi: 10.1016/S0360-1285(00)00003-4
Northcutt, J. K., and Jones, D. R. (2004). A survey of water use and common industry practices in commercial broiler processing facilities. J. Appl. Poultry Res. 13, 48–54. doi: 10.1093/japr/13.1.48
Northcutt, J. K., Smith, D., Huezo, R. I., and Ingram, K. D. (2008). Microbiology of broiler carcasses and chemistry of chiller water as affected by water reuse. Poult. Sci., 87, 1458–1463. doi: 10.3382/ps.2007-00480
NSW Environmental Protection Agency, Disinfection of Treated Wastewater (2002). EPA Victoria. Southbank, Victoria.
Occupational Safety and Health Administration (2017a). United States Department of Labor. Available online at: https://www.osha.gov/dts/chemicalsampling/data/CH_226500.html (Accessed May 12, 2018).
Occupational Safety and Health Administration (2017b). United States Department of Labor. Available online at: https://www.osha.gov/SLTC/heatillness/heat_index/work_rates_loads.html (Accessed June 22, 2018).
Owens, C. M., Sams, A. R., and Alvarado, C. (2000). Introduction to Poultry Meat Processing. Poultry Meat Processing. Boca Raton, FL: CRC Press.
Oyarzabal, O. A. (2005). Reduction of Campylobacter spp. by commercial antimicrobials applied during the processing of broiler chickens: a review from the United States perspective. J. Food Protect. 68, 1752–1760. doi: 10.4315/0362-028X-68.8.1752
Park, H. J., Chon, J. W., Lim, J. S., Seo, K. H., Kim, Y. J., Heo, E. J., Wee, S. H., et al. (2015). Prevalence analysis and molecular characterization of Salmonella at different processing steps in broiler slaughter plants in South Korea. J. Food Sci. 80, 2822–2826. doi: 10.1111/1750-3841.13106
Patterson, J. T. (1968). Chlorination of water used for poultry processing. Br. Poult. Sci. 9, 129–133.
Pellow, D. N. (2004). The politics of illegal dumping: An environmental justice framework. Qual. Sociol. 27, 511–525. doi: 10.1023/B:QUAS.0000049245.55208.4b
Peracetic acid. MSDS No (2013). SLP5503; Sciencelab.com, Inc. 14025. Houston, TX. Available online at: http://www.sciencelab.com/msds.php?msdsId=9926439 (Accessed May 5, 2018).
Pryor, A., and Rice R. G. (1999) “Introduction to the use of ozone in food processing applications,” in 14th Ozone World Congress. Dearborn, MI, 26–36.
Restaino, L., Frampton, E. W., Hemphill, J. B., and Palnikar, P. (1995). Efficacy of ozonated water against various food-related microorganisms. Appl. Environ. Microbiol. 61, 3471–3475.
Rice, R. G., Robson, C. M., Miller, G. W., and Hill, A. G. (1981). Uses of ozone in drinking water treatment. J. Am. Water Works Assoc. 73, 44–57. doi: 10.1002/j.1551-8833.1981.tb04637.x
Rijsberman, F. R. (2006). Water scarcity: fact or fiction? Agricult. Water Manag. 80, 5–22. doi: 10.1016/j.agwat.2005.07.001
Ryu, J. H., and Beuchat, L. R. (2005). Biofilm formation by Escherichia coli O157: H7 on stainless steel: effect of exopolysaccharide and curli production on its resistance to chlorine. Appl. Environ. Microbiol., 71, 247–254. doi: 10.1128/AEM.71.1.247-254.2005
Sanders, E. C., Yuan, Y., and Pitchford, A. (2013). Fecal coliform and E. coli concentrations in effluent-dominated streams of the Upper Santa Cruz watershed. Water 5, 243–261. doi: 10.3390/w5010243
Sanitation Performance Standards Compliance Guide (2016). Available online at: https://www.fsis.usda.gov/wps/portal/fsis/topics/regulatory-compliance/compliance-guides-index/sanitation-performance-standards/sanitation-compliance-guid
Saravia, H., Houston, J. E., Toledo, R., and Nelson, H. M. (2005). Economic Feasibility of Recycling Chiller Water in Poultry Processing Plants by Ultrafiltration. Georgia Institute of Technology.
Scher, K., Romling, U., and Yaron, S. (2005). Effect of heat, acidification, and chlorination on Salmonella enterica serovar Typhimurium cells in a biofilm formed at the air-liquid interface. Appl. Environ. Microbiol., 71, 1163–1168. doi: 10.1128/AEM.71.3.1163-1168.2005
Selma, M. V., Allende, A., Lopez-Galvez, F., Conesa, M. A., and Gil, M. I. (2008). Disinfection potential of ozone, ultraviolet-C and their combination in wash water for the fresh-cut vegetable industry. Food Microbiol. 25, 809–814. doi: 10.1016/j.fm.2008.04.005
Shannon, M. A., Bohn, P. W., Elimelech, M., Georgiadis, J. G., Marinas, B. J., and Mayes, A. M. (2008). Science and technology for water purification in the coming decades. Nature 452, 301–310. doi: 10.1038/nature06599
Sheldon, B. W., and Brown, A. L. (1986). Efficacy of ozone as a disinfectant for poultry carcasses and chill water. J. Food Sci. 51, 305–309. doi: 10.1111/j.1365-2621.1986.tb11116.x
Sheldon, B. W., and Chang, Y. H. (1987). “The application of ozone and other physical processes for treating spent poultry chiller water,” in Proceedings of the Food Processing Waste Conference. Atlanta, GA.
Shih, J., and Kozink, M. B. (1980). Ultrafiltration treatment of poultry processing wastewater and recovery of a nutritional by-product. Poult. Sci. 59, 247–252. doi: 10.3382/ps.0590247
Sirsat, S. A., Muthaiyan, A., and Ricke, S. C. (2009). Antimicrobials for foodborne pathogen reduction in organic and natural poultry production. J. Appl. Poultry Res. 18, 379–388. doi: 10.3382/japr.2008-00140
Sodium bisulfate. MSDS No. (2013). SLS2104; Sciencelab.com, Inc. Houston, TX. Available online at: http://www.sciencelab.com/msds.php?msdsId=9926439 (Accessed May 5, 2018).
Stampi, S., De Luca, G., and Zanetti, F. (2001). Evaluation of the efficiency of peracetic acid in the disinfection of sewage effluents. J. Appl. Microbiol. 91, 833–838. doi: 10.1046/j.1365-2672.2001.01451.x
Stanley, D., Hughes, R. J., and Moore, R. J. (2014). Microbiota of the chicken gastrointestinal tract: influence on health, productivity and disease. Appl. Microbiol. Biotechnol., 98, 4301–4310. doi: 10.1007/s00253-014-5646-2
Sun, H., Pan, Y., Zhao, Y., Jackson, W. A., Nuckles, L. M., Malkina, I. L., et al. (2008). Effects of sodium bisulfate on alcohol, amine, and ammonia emissions from dairy slurry. J. Environ. Qual. 37:608e614. doi: 10.2134/jeq2006.0446
Tsai, L. S., Higby, R., and Schade, J. (1995). Disinfection of poultry chiller water with chlorine dioxide: consumption and byproduct formation. J. Agric. Food Chem. 43, 2768–2773.
Tsai, L. S., Schade, J. E., and Molyneux, B. T. (1992). Chlorination of poultry chiller water: chlorine demand and disinfection efficiency. Poult. Sci. 71, 188–196.
Tuthill, A., Avery, R., Lamb, S., and Kobrin, G. (1998). Effect of chlorine on common materials in fresh water. Mater. Perform. 37, 52–56.
Tyrrell, S. A., Rippey, S. R., and Watkins, W. D. (1995). Inactivation of bacterial and viral indicators in secondary sewage effluents, using chlorine and ozone. Water Res. 29, 2483–2490. doi: 10.1016/0043-1354(95)00103-R
Unal, R., Kim, J. G., and Yousef, A. E. (2001). Inactivation of Escherichia coli O157: H7, Listeria monocytogenes, and Lactobacillus leichmannii by combinations of ozone and pulsed electric field. J. Food Prot. 64, 777–782. doi: 10.4315/0362-028X-64.6.777
United Nations Department of Economics Social, Affairs (UNDESA) (2017) World Population Projected to Reach 9.8 Billion in 2050, and 11.2 Billion in 2100. Available online at: https://www.un.org/development/desa/en/news/population/world-population-prospects-2017.html
United Stated Department of Agriculture. (2015). Sodium Bisulfate - Agricultural Marketing Service. Available online at: https://www.ams.usda.gov/sites/default/files/media/Sodium%20Bi%20report%202015.pdf (Accessed May 26, 2018.)
United States Census Bureau (2011). International Data Base World Population: 1950-2050. Available online at: https://www.census.gov/population/international/data/idb/worldpopgraph.php
United States Department of Agriculture (2003a). Temperatures and Chilling and Freezing Procedures. 9 CFR, part 381.66b. Washington, DC: US Government Printing Office.
United States Department of Agriculture (2017). Cost Estimates of Foodborne Illnesses. Availble online at: https://www.ers.usda.gov/data-products/cost-estimates-of-foodborne-illnesses/ (Accessed September 22, 2018).
United States Department of Agriculture (2018). World Agricultural Supply and Demand Estimates. Avialble online at: https://www.usda.gov/oce/commodity/wasde/latest.pdf (Accessed June 25, 2018).
United States Department of Agriculture, Food Safety Inspection Service. (2003b). Use of Chlorine to Treat Poultry Chiller Water. Available online at: http://www.fsis.usda.gov/OPPDE/rdad/FSISNotices/45-03.htm(Accessed October 25, 2007).
United States Environmental Protection Agency (1992). Drinking Water Criteria Document for Chlorine, Hypochlorous Acid and Hypochlorite Ion. Environmental Criteria and Assessment Office, Office of Health and Environmental Assessment, Cincinnati, OH.
United States Environmental Protection Agency (2018). Safer Chemical Ingredients List. Available online at: https://www.epa.gov/saferchoice/safer-ingredients (Accessed September 25, 2018).
United States Environmental Protection Agency (2012). Guidelines for Water Reuse. Available online at: https://www3.epa.gov/region1/npdes/merrimackstation/pdfs/ar/AR-1530.pdf (Accessed 25 May, 2018).
Wagner, M., Brumelis, D., and Gehr, R. (2002). Disinfection of wastewater by hydrogen peroxide or peracetic acid: development of procedures for measurement of residual disinfectant and application to a physicochemically treated municipal effluent. Water Environ. Res. 74, 33–50. doi: 10.2175/106143002X139730
Walsh, R. J., White, B., Hunker, L., Leishman, O., Hilgren, J., and Klein, D. (2018). Peracetic acid and hydrogen peroxide post-dip decay kinetics on red meat and poultry. Food Protect. Trends 38, 96–103.
Warf, C. C. (2001). “The chemistry and mode of action of acidified sodium chlorite,” in 2001 IFT Annual Meeting (New Orleans, LA), 1–91.
Wei, C. I., Cook, D. L., and Kirk, J. R. (1985). Use of chlorine compounds in the food industry. Food Technol. 39, 107–115.
Wesley, R. L. (1977). “Effect of metered chiller input volume on poultry carcass quality,” in Poultry Science. (Savoy, IL: Poultry Science Association Inc), 1769–1769.
White, G. C. (2010). White's Handbook of Chlorination and Alternative Disinfectants. Overland Park, KS: Black & Veatch Corporation.
Wickramanayake, G. B. (1984). Kinetics and Mechanism of Ozone Inactivation of Protozoan Cysts Phil. dissertation. Columbus, OH: Ohio State University.
Winder, C. (2001). The toxicology of chlorine. Environ. Res. 85, 105–114. doi: 10.1006/enrs.2000.4110
Wolfe, R. L., Ward, N. R., and Olson, B. H. (1984). Inorganic chloramines as drinking water disinfectants: a review. J. Am. Water Works Assoc. 76, 74–88.
World Health Organization (2011). Guidelines for Drinking-Water Quality, 4th Edn. Geneva: World Health Organization.
Wynn, C. S., Kirk, B. S., and McNabney, R. (1973). Pilot Plant for Tertiary Treatment of Wastewater with Ozone. Environmental Protection Agency Report EPA-R2-73-146.
Xu, L. (1999). Use of ozone to improve the safety of fresh fruits and vegetables. Food Techn. 53, 58–62.
Yabin, L.i., Slavik, M. F., Walker, J. T., and Xiong, H. (1997). Pre-chill spray of chicken carcasses to reduce Salmonella typhimurium. J. Food Sci. 62, 605–607. doi: 10.1111/j.1365-2621.1997.tb04441.x
Yang, Z., Li, Y., and Slavik, M. (1998). Use of antimicrobial spray applied with an inside–outside birdwasher to reduce bacterial contamination on prechilled chicken carcasses. J. Food Prot. 61, 829–832.
Keywords: poultry processing, water reuse, sanitation, sodium bisulfate, peracetic acid, ozone
Citation: Micciche AC, Feye KM, Rubinelli PM, Wages JA, Knueven CJ and Ricke SC (2018) The Implementation and Food Safety Issues Associated With Poultry Processing Reuse Water for Conventional Poultry Production Systems in the United States. Front. Sustain. Food Syst. 2:70. doi: 10.3389/fsufs.2018.00070
Received: 23 August 2018; Accepted: 04 October 2018;
Published: 24 October 2018.
Edited by:
Joshua B. Gurtler, Agricultural Research Service (USDA), United StatesReviewed by:
Michele Jay-Russell, University of California, Davis, United StatesLisa Gorski, Western Regional Research Center, United States Department of Agriculture, Agricultural Research Service, United States
Walid Alali, United Arab Emirates University, United Arab Emirates
Copyright © 2018 Micciche, Feye, Rubinelli, Wages, Knueven and Ricke. This is an open-access article distributed under the terms of the Creative Commons Attribution License (CC BY). The use, distribution or reproduction in other forums is permitted, provided the original author(s) and the copyright owner(s) are credited and that the original publication in this journal is cited, in accordance with accepted academic practice. No use, distribution or reproduction is permitted which does not comply with these terms.
*Correspondence: Steven C. Ricke, c3JpY2tlQHVhcmsuZWR1