- 1Agricultural Technological Institute of Castilla y León, Valladolid, Spain
- 2Aquaculture Research Center, Agricultural Technological Institute of Castilla y León, Segovia, Spain
The recovery of proteins from microalgae is gaining special attention for animal feed applications especially for fish feed, as the costs of aquaculture feeds represent between 40 and 70% of the costs of the fish produced. Besides, the use of pig manure to produce microalgal biomass could contribute to manure bioremediation and therefore to reduce the environmental impacts of its storage. The objective of this study was to recover protein concentrates from microalgal biomass grown in pig manure, paying especial attention to the quality of the extracted proteins that can be used as feed source for fish, and to the amino acids composition, essential amino acids content and availability. Methane potential of the by-products obtained after protein recovery was also determined in order to fully apply the biorefinery concept to valorize the resulting biomass. Results showed a maximum protein recovery of 54.5 ± 3.2% from initial microalgal biomass. Protein content in the protein concentrate accounted for 84.5 g protein per 100 g biomass in total solids (TS) basis. In the case of the amino acids profile from protein concentrate, essential amino acids accounted for 47.5 g 100 g amino acids−1 in TS basis. After protein recovery, the resulting by-products named spent and liquid fraction were anaerobically digested in batch assays, obtaining a maximum methane production of 181 mL CH4 g VS added-1.
Introduction
The costs of aquaculture feeds represent 40–70% of the costs of the fish produced (FAO, 2014), where the fishmeal is incorporated as main source of protein. The increasing demand of fishmeal for aquaculture feeds has resulted in the overexploitation of fisheries with the subsequent price increase (FAO, 2014). Soybean, rapeseed, gluten meal or wheat meal and other protein-rich terrestrial plants have been introduced into the fish diets as sustainable alternative source of protein, but the presence of anti-nutritional factors (ANFs) in the digestive tract have shown that it affects to the bioavailability of the protein, has evidenced the need to find a suitable and sustainable alternative for aquaculture feed (Collins, 2014).
In order to satisfy this demand, the utilization of microalgae as an alternative to fishmeal is being widely studied in different fish species (Olvera-Novoa et al., 1998; Patterson and Gatlin, 2013; Kiron et al., 2016; Sørensen et al., 2016). Most microalgae are rich in fiber, mineral salts, trace-elements, vitamins, polyunsaturated fatty acids, chlorophylls and essential amino acids (EAA), fulfilling requirements of most commercial fish feed and avoiding supplementation of vitamins and amino acids (Vizcaíno et al., 2014). In addition, microalgae present a low concentration of ANFs like phytic acid, tannins, glucosinolates, saponinsis, isoflavones, among others (Collins, 2014). In this manner, the partial substitution of fishmeal proteins by microalgal proteins may trigger positive effects in fish metabolism, evidencing that they are a suitable fish feed (Dallaire et al., 2007).
However, there are some aspects that must be addressed in order to make microalgae a suitable source for fish feed. Microalgal growth requires high amounts of nutrients, mainly nitrogen (N) and phosphorous (P). The supply of P is limited and N production is energy intensive and contributes to global warming. One alternative to synthetic culture media is to use agro-industrial wastewater, which usually presents high N and P concentration (Riaño et al., 2012; Chisti, 2013; Hernández et al., 2013). Specifically, the use of microalgal for pig manure (PM) bioremediation has been widely studied, reaching high microalgal productivities in the range of 9–55 g volatile suspended solids /m−2 d−1 (Lundquist et al., 2010; Masojídek et al., 2011; Hernández et al., 2016) and removing organic matter, nutrients, pathogen and emerging pollutants (de Godos et al., 2009; Posadas et al., 2017; García et al., 2018).
On the contrary, few studies have been focused on the valorization of macromolecular components (proteins, carbohydrates and lipids) of the microalgae when biomass comes from wastewater treatment. In this context, microalgae biomass grown using PM is usually rich in ash (Kebede-Westhead et al., 2006). This ash content decreases feed digestibility, which affects to the optimal health and development of the fish. In this sense, protein extraction from microalgal biomass could be a suitable tool to increase the protein content and to eliminate ash from the resulting protein concentrate during the extraction process, increasing digestibility of some essential amino acids as histidine (Hys) and lysine (Lys) and decreasing presence of ANFs. However, the high-energy demand required for microalgae cell disruption and the extraction of proteins makes difficult the use of microalgal biomass as fish feed in a cost-effective way. The protein extraction method comprises microalgal cell disruption and the subsequent solubilization, separation, precipitation, and extraction of the proteins. The efficiency of this method is highly affected by the microalgal cell wall composition and the amino acid nature of the proteins. Cell disruption must be carried out by a pretreatment able to release intracellular proteins. Several mechanical, chemical, and biological (i.e., enzymatic) methods have been studied for cell disruption (Möllers et al., 2014; Hernández et al., 2015). Despite of high temperatures may degrade proteins, chemical methods have previously shown to be optimal to break down microalgal cell wall as a previous step for saccharification (Hernández et al., 2015), but they also can lead to the formation of amino acid complexes (Maillard reactions), which limit the availability of amino acids (Boisen et al., 2000). Furthermore, alternative mechanical methods such as tangential and ultrafiltration of proteins obtaining extraction efficiencies up to 76% of the solubilized proteins (Ursu et al., 2014) have also been studied, but they were discarded due to the high costs. Low-cost mechanical methods are still generally preferred for large-scale applications aimed to obtain high added value products (Günerken et al., 2015; Hernández et al., 2015). In this context, more conservative methods like sonication or high pressure cell disruption have been studied, reporting high protein solubilization (from 52.3 to 73.0%) of the total initial proteins in the microalga using pH 11 in Nannochloropsis sp. (Gerde et al., 2013), pH 12 in Chlorella vulgaris (Ursu et al., 2014) and pH 5.7 in Haematococcus pluvialis (Ba et al., 2016). These studies evidence the key role of the pretreatment method and the pH used to solubilize proteins despite of the microalga used. Furthermore, the isoelectric point of the microalgal proteins must be determined to precipitate proteins efficiently for further extraction.
The use of by-products, from microalgal protein extraction, for anaerobic digestion presents many advantages as a consequence of the pretreatment applied such as cell wall rupture, solubilization of macromolecular components (carbohydrates and lipids), increase of biodegradability, improvement of the carbon/nitrogen (C/N) ratio, among others that enhance CH4 production (Ehimen et al., 2011). To the best of our knowledge, little effort has been made to investigate the valorization of residues from microalgal protein extraction.
The objective of this study was to obtain protein concentrates from microalgae biomass, maximizing protein solubilization, separation, precipitation and extraction. The amino acid composition and its suitability, as well as proportion and availability of the extracted proteins as feed source for fish were determined. Finally, and in order to valorize the resulting by-products obtained after protein extraction and to fulfill the biorefinery concept, biological methane potential of different mixtures of the by-products obtained after protein extraction was investigated.
Methods
Microalgae Production
Microalgae biomass was obtained in lyophilized form from the Food Innovation and Sustainability Center (Almería, Spain). Biomass was cultured outdoors using a thin layer photobioreactor with a total working volume of 1,200 L, and a surface of 33 m2 (del Mar Morales-Amaral et al., 2015). The photobioreactor was fed with 10% diluted centrifuged PM at a hydraulic retention time of 0.3 days. The volume of the photobioreactor was checked daily and water lost due to evaporation was fully replenished with treated PM. Biomass productivity was 17.1 ± 4.7 g volatile suspended solids m−2 d−1. The culture was mainly dominated by Scenedesmus almeriensis. Other species such as Oscillatoria, Scenedesmus, Chlorella, and Nitzschia also appeared in less proportion in the culture medium. Finally, the biomass was centrifuged (Watermaster centrifuge, GEA, Westphalia, Germany). Therefore, the microalgal paste was lyophilized at −18°C with a freeze-dryer-cryodo (Telstar, Barcelona, Spain) for further use. Lipid, carbohydrate and protein contents of the lyophilized biomass were determined (Table 1).
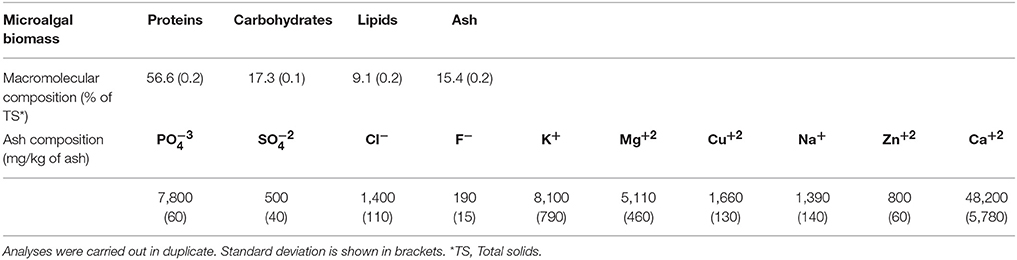
Table 1. Macromolecular and ionic characterization on total solid basis of microalgal biomass grown in diluted pig manure.
Protein Concentrates
Pretreatments for Protein Solubilization
Lyophilized biomass was suspended in distilled water in a ratio 1:20 (weight weight−1) by agitation for 24 h at 4°C. Different pretreatments were tested, obtaining seven different trials (Figure 1). Trial 1 was carried out without cell wall rupture process, while in the rest of trials different physical pretreatments were applied to the microalgae solution. In the case of Trial 2, a thermal pretreatment was applied. Thus, samples were processed in an autoclave (Selecta, Presoclave II, Spain) at 120°C for 15 min. In the case of Trials 3, 4, 5, 6, and 7, biomass was pretreated by sonication (Vibracell W-75043; Sonics and Materials, EEUU). Sonication pretreatment was performed with lyophilized microalgal biomass suspended in 100 mL of distilled water to set a final concentration of 50 g VS L−1. The operational conditions of the probe were optimized and operated as follows: constant frequency of 20 Hz and the ultrasonic energy was applied in continuous (non-pulsed) mode with constant amplitude of 70%. Samples were kept on ice cold to maintain temperature between 4 and 7°C during the sonication. The sonication procedure was repeated three times for 1 min each, followed by a settling step of 10 min. After the physical pretreatment, the microalgae solution was subjected to an alkaline treatment by adjusting the pH with NaOH 2.5 N for all the trials. The alkaline treatment for Trials 1, 2, and 3 was performed in distilled water (i.e., 100 mL) and carried out for 120 min, pH = 12 at 150 rpm and 45°C. For Trials 4, 5, 6, and 7 a double alkaline treatment was carried out after sonication. More specifically, both alkaline treatments were performed at the same conditions (120 min, 150 rpm, and 45°C) followed by a centrifugation step (15 min, 15,006 g, 4–7°C). Double amount of distilled water (i.e., 100 mL + 100 mL) was added for the double alkaline treatments, thus the supernatant from the first alkaline treatment (i.e., 100 mL) was collected and the pellet was resuspended in another 100 ml of distilled water. The pH for each double alkaline treatment was adjusted as follows: Trial 4 (1° pH = 12; 2° pH = 12), Trial 5 (1° pH = 13; 2° pH = 13), Trial 6 (1° pH = 13; 2° pH = 11.5), and Trial 7 (1° pH = 11.5; 2° pH = 13). After the alkaline treatments, a centrifugation step (Avanti J-30I Centrifuge, Beckman Coulter, USA) was performed (15 min, 15,006 g, 4–7°C) obtaining two fractions, namely spent (SP) and primary supernatant (containing the solubilized proteins).
Protein Extraction
Protein precipitation was optimized adjusting pH with HCl in a range of 3.3–4.5, obtaining the higher protein recovery at pH 3.5 (data not shown). Thus, the solutions corresponding to the primary supernatants were adjusted to pH 3.5 in every trial. After that, the acidic supernatants were centrifuged at 15,006 g for 15 min at 4–7°C (Avanti J-30I Centrifuge, Beckman Coulter, USA). Two fractions were obtained for each trial: a protein concentrate (PC) and a supernatant or liquid fraction (LF). The same experimental procedure was followed for all the trials.
Protein recovery was calculated according to Equation (1):
Where the subscript IB correspond to initial biomass, TKN corresponds to total Kjehldhal nitrogen and FW corresponds to fresh weight.
Anaerobic Digestion
Anaerobic biodegradability assays were carried out at 37.1 ± 0.6°C for 46 days in 0.57 L bottles. Quantities were calculated to reach a final volume of 0.30 L, allowing a headspace of 0.27 L for biogas accumulation. The bottles were closed with a septum and the headspace was flushed with N2 for 5 min to ensure anaerobic conditions. The biogas production was determined by measuring the overpressure in the headspace with time frequency (Colleran et al., 1992). Constant agitation was provided by a shaker at 50 rpm. Anaerobic sludge was collected at the municipal wastewater treatment plant of Valladolid (Spain) and used as inoculum. Anaerobic sludge presented a total solids (TS) and volatile solids (VS) concentration of 26.6 ± 0.9 and 14.7 ± 0.1 g L−1, respectively. The batch assays were filled in a substrate/inoculum (S0/X0) ratio of 1.0 (based on VS); this ratio was selected according to Santamaría-Fernández et al. (in press). For these assays, two kinds of by-products obtained from protein extraction were used, namely SP and LF. Two different assays were carried out. Buffered assays using 12 g L−1 of NaHCO3 (3.6 grams in each sample) were performed in M1-M5 trials, while in N1-N5 trials samples were not buffered. More specifically, the following five different mixtures in terms of the percentage of VS of LF used in relation with percentage of VS of SP used (%VSLF ) were studied using the ratios: (100/0) for M1 and N1, (75/25) for M2 and N2, (50/50) for M3 and N3, (25/75) for M4 and N4 and (0/100) for M5 and N5. For the determination of endogenous methane production, blanks containing only anaerobic sludge were also tested. After that, the pH was adjusted to 7.7 using HCl at the beginning of the experiment. All the experiments were carried out in triplicate and the results were expressed as means.
Analytical Procedures
Macromolecular Composition of Microalgal Biomass
Lipids were extracted using chloroform-methanol 2:1 (volume volume−1) as solvent, following the method proposed by Kochert (1978). Once the extraction was completed, the lipid concentration was determined by gravimetric analysis at 45°C until constant weight. The concentration of carbohydrates was determined by using an adaptation of Sluiter et al. (2008) protocol, to determine carbohydrates from lignocellulosic biomass by using phenol-sulfuric acid method (Dubois et al., 1956). For protein determination, the specific N-Protein conversion factor (5.62) was calculated using the amino acid composition of the biomass by Ultra-Performance Liquid Chromatography and TKN. Thus, true protein content in the samples was determined by multiplying TKN content by the calculated conversion factor. The calculation of this conversion factor based on the amino acid profile is a key aspect, since protein and other constituents of microalgae contain nitrogen (i.e., nucleic acids, amines, glucosamides, and cell wall materials). In this manner, this calculation overestimates protein content (crude protein) and must be adapted by using an optimized conversion factor to estimate true protein (Becker, 2007). This author evidenced that the content of non-protein nitrogen amounts reached to 12% in the microalga Scenedesmus, which is the main microalga of the biomass used during this study.
Chemical Characterization and Amino Acid Analysis of By-Products
Lyophilized biomass, PC fractions, SP fractions, and LF fractions were analyzed for TS, VS, TKN, total alkalinity, and partial alkalinity (PA) in duplicates were measured following (APHA, 2005). Intermediate alkalinity (IA) was calculated as the difference between total alkalinity and partial alkalinity. Amino acid composition in lyophilized microalgae, SP fractions, and PC fractions was analyzed. Samples were stored at 4°C, and were homogenized prior to analysis. Firstly, an acid hydrolysis by using HCl 6 N for 24 h was carried out in order to break down proteins into amino acids. Then, the acid volume of the resulting hydrolysis was reduced by vacuum evaporation and extracts were re-suspended in distilled water. The samples were then ready for analysis. After that, Ultra-Performance Liquid Chromatography AccQ Tag Ultra C18, 2.1 × 100 mm column (Waters, Milford MA) and a gradient system with the mobile phase consisting of buffer A (0.5 mM TDFHA) and buffer B (0.5 mM TFHA in acetonitrile; 100%) at a flow rate of 700 μL min−1 (split less) was performed at 37°C. One minute prior to the next sample injection the flow was set to 700 μl min−1. The injected volume was 1 μL. Run-to-run time was 30 min.
In order to characterize free amino acids, the hydrolysis step using HCl was not performed. Thus, only free amino acid contained on the PC fraction were determined True protein content was obtained after summing all the amino acids present in samples.
Ash Characterization
In order to determine ash composition (Table 1), different chromatographic methods were performed. The ionic chromatography Dionex ICS-2000 (Sunnyvale, CA, USA) was used for the separation and suppressed conductivity detection of the following anion: fluorides, chlorides, sulfates, and phosphates. Instrument control and data acquisition were performed using Chromeleon®; 6.60 software. ICS-2000 instrument was used in conjunction with Dionex AS autosampler to enable simultaneous separation of anions. Separation was performed on a column IonPac AS19 (250 mm, 4 mm ID) used with a Dionex AG19 guard column (50 mm, 4 mm ID), coupled to a Dionex ASRS Ultra II 4 mm suppressor. Hydroxide eluent gradients were generated using the Dionex EluGen II EG50 KOH cartridge and ultrapure water (Milipore Corporation). The optimized hydroxide eluent gradient was: 10 mM isocratic for 0–20 min and a gradient of 10–45 mM for 20–25 min.
Different metals were determined by flame atomic absorption spectroscopy (AAS) in VARIAN AA240FS, Atomic Absorption Spectrophotometer, following the method proposed by Xie et al. (2008).
Biogas Composition and Total Volatile Fatty Acids Concentration in Anaerobic Digestion Assays
Biogas composition was analyzed using a gas chromatograph (Agilent 7890A) with a thermal conductivity detector, separated by a HP-Plot column (30 m 0.53 mm 40 μm) followed by a HP-Molesieve column (30 m 0.53 mm 50 μm). Helium (7 mL min−1) was used as the carrier gas. The injection port temperature was set at 250°C and the detector temperature was 200°C. The temperature of the oven was set at 40°C for 4 min and after that increased to 115°C for 1 min and 45 S. Individual volatile fatty acids were determined using a gas chromatograph (Agilent 7890A) equipped with an Teknokroma TRB-FFAP column of 30 m length and 0.25 mm followed by a flame ionization detector. The carrier gas was helium (1 mL min−1). The temperature of the detector and the injector was 280°C. The temperature of the oven was set at 100°C for 4 min, then increased to 155°C for 2 min and thereafter increased to 210°C. Furthermore, the concentration of total volatile fatty acids (VFA) were determined by the sum of individual volatile fatty acids VFA concentration was converted to COD concentration by using the following conversion factors: 1.07 for acetic acid, 1.51 for propionic acid, 1.82 for butyric and isobutyric acid, 2.04 for valeric and isovaleric acid, 2.21 for hexanoic acid, and 2.34 for heptanoic acid according to Yuan et al. (2011).
Results
Initial Biomass Composition
Macromolecular characterization of biomass is presented in Table 1. Microalgae biomass contained 57, 17, and 9% of proteins, carbohydrates and lipids, respectively, on a total solid basis. Ash content accounted for 15% of the total solid basis. The ash composition in the microalgae cells is presented in Table 1, being especially remarkable the high presence of Ca+2 (48,200 mg kg−1), K+ (8,100 mg kg−1), (7,800 mg kg−1), and heavy metals like Cu+2 and Zn+2 (1,660 and 800 mg kg−1, respectively).
Protein Concentrates from Microalgae: Optimization of Solubilization and Precipitation Methods
Figure 2 presents true protein recovery for trials 1 to 7, calculated as the percentage of recovered protein in the concentrates in relation to the protein content in the initial biomass (Equation 1). Protein recovery for Trial 1 was very low (9.1 ± 0.1%), showing the need of a pretreatment to breakdown cell walls as a previous step for protein precipitation. The application of a thermal pretreatment in Trial 2 and ultrasound in Trial 3 increased protein recovery to 21.5 ± 1.3% and to 18.4 ± 0.5%, respectively.
The combination of an ultrasound pretreatment and a double alkaline solubilization and separation (Trials 4, 5, 6, and 7) resulted in up to 3-fold higher protein recovery yields, when compared to simple alkaline extraction pretreated (Trial 3). More specifically, the highest protein recovery yield was achieved in Trial 5 after two consecutive alkaline treatments at pH 13 (i.e., 54.5 ± 3.2%).
Protein Quality of the Protein Concentrates and Suitability as Fish Feed
Mass balances in terms of TS, VS, ash, and protein content were performed for Trial 5 (Table 2). This trial was selected due to the highest protein recovery that was achieved during the protein extraction process. The biomass loss during the extraction process accounted for 1.7% on VS basis (Table 2). After protein extraction, 54.5% of the initial protein was recovered in the PC. This product showed a protein concentration of 84.5 g protein per 100 g TS. Moreover, this PC fraction presented very low ash content, since 87.6% of the ashes from the initial biomass were found in the by-products. More specifically, SP and LF accounted for 38.3 and 49.3% of the total amount of ash from the initial biomass.

Table 2. Mass balances of total solids, volatile solids, ash and protein for Trial 5 using 100 g of biomass (in total solid basis).
As it has been previously described, the highest protein recovery (54.5%) was reached when applying ultrasounds followed by a double alkaline treatment at pH 13 (Trial 5). Protein concentrate from Trial 5 was evaluated as a possible supplement for rainbow trout feed in terms of content, proportion and availability of amino acids. The amino acid profile in terms of grams of amino acid per 100 g of protein is presented in Table 3. Amino acid profile for the initial microalgae biomass was very similar when compared to amino acid profile of the protein concentrate. Only small differences in phenylalanine (Phe) and Lys content were detected. The content of EAA accounted for 48% of the total amino acids concentration in PC and free amino acids reached 6.5%.
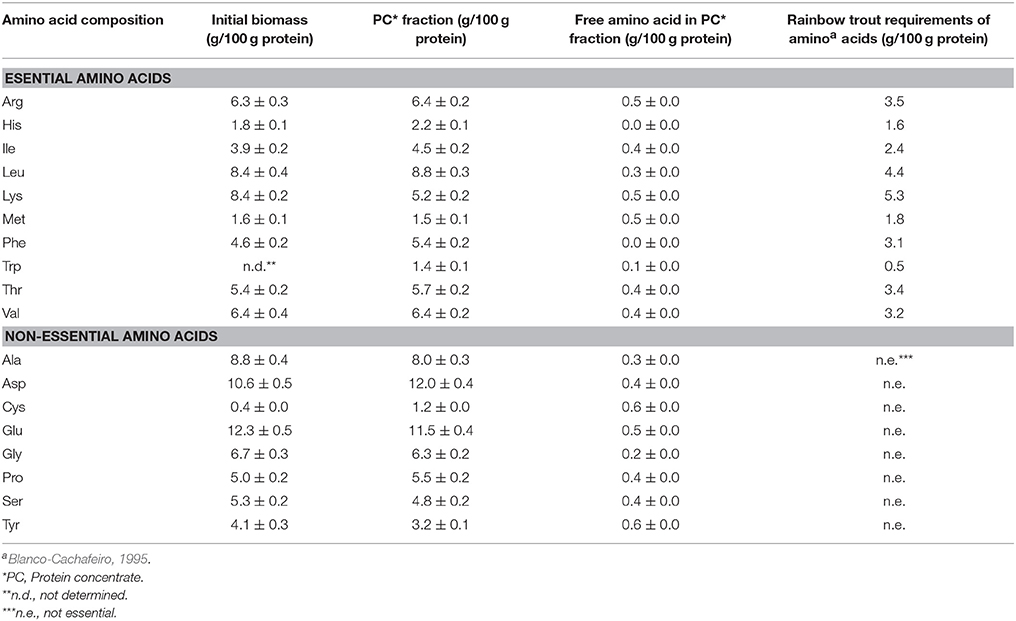
Table 3. Amino acid composition of microalgal biomass and protein concentrate fraction (grams of amino acids correspond to average values ± standard deviation).
Anaerobic Digestion of By-Products Obtained After Protein Extraction
The feasibility of the two by-products obtained after protein extraction, namely SP and LF, as sole and combined substrates for anaerobic digestion was evaluated. Both substrates presented different pH values (3.7 ± 0.1 for LF and 12.9 ± 0.3 for SP) and VS contents (4 g VS L−1 for LF and 143 g VS L−1 for SP). Concerning SP fraction, protein content accounted for 25.4 g protein per 100 g TS, while negligible protein content was found in LF. The concentration of carbohydrates in LF was 3-fold higher than in SP, while lipid content in SP was 1.5-fold higher than in LF. Both substrates were co-digested in five different assays to evaluate the influence of LF/SP mixture ratio in terms of methane yield.
Figure 3 shows the accumulated methane production of the different mixtures of LF and SP in (Figure 3A) buffered (M1–M5) and (Figure 3B) unbuffered (N1–N5) assays. The highest methane yields were achieved by M1 and M2 (181 ± 3.5 and 160 ± 22.3 mL CH4 g ), corresponding to the highest contents of LF in buffered assays. The highest total COD removals were also reached in M1 and M2, accounting for 40.8 and 40.2%, respectively and also the highest VS removals achieving 30.1 and 32.9%, respectively. The methane production in N1–N5 was remarkably lower than in buffered assays with methane productions lower than 97 mL CH4 g in all cases.
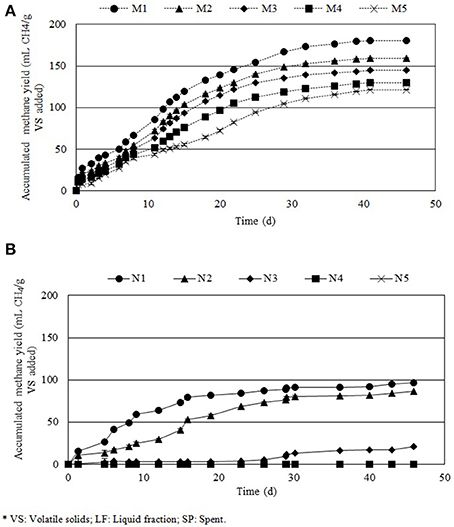
Figure 3. Accumulated methane yield in the evaluated treatments for different mixture of liquid fraction and spent (%VSLF/%VSSP) at a substrate inoculum ratio of 1.0 (•) M1 and N1 (100/0), (▴) M2 and N2 (75/25), (♦) M3 and N3 (50/50), (■) M4 and N4 (25/75) and (X) M5 and N5 (0/100). In (A) M1-M5 samples were buffered with 12 g/L NaHCO3 while in (B) N1–N5 the medium was not buffered.
Discussion
Protein Recovery: Optimization of the Solubilization and Precipitation Methods
Characterization of biomass from Table 1 is similar than that previously reported by Becker (2007) for the same microalga (Scenedesmus). The concentration of ash accounted for 15.4% of the total dry weight, which may be considered as high compared to previous results reported by Sterner (1993) and Becker (2007) who accounted from 3.8 to 17% of ash content in the same microalga. As it is shown in Table 1, the concentration of Cu+2 and Zn+2 in the biomass was considerably high. Also, high amounts of Ca+2, Cl−, K+, Mg+2, Na+ Ca+2 were determined, probably due to the high salinity of the PM to balance the osmotic potential and to maintain the turgor pressure (Bisson and Kirst, 1995), increasing thus the ash content.
The results obtained for the alkaline treatment without any physical pretreatment (Trial 1) evidenced the need of a pretreatment to breakdown microalgal cell wall in order to release intracellular proteins for further protein precipitation (Figure 2). These results are in agreement with Miranda et al. (2012), who reported the need of a pretreatment in Scenedesmus to break down its rigid cell wall. In this context, the use of ultrasounds as a pretreatment previous to protein extraction resulted in a remarkable recovery increase (Trial 3) in comparison with Trial 1. These differences may be attributed to the cell wall breakdown caused by ultrasounds and subsequent solubilization of proteins into the medium. Furthermore, the combination of two consecutive alkaline extractions, especially when using a pH of 13 (Trial 5) resulted in further enhancement of protein recovery. However, despite of the combination of two alkaline extractions enhances protein recovery; the identification of an optimal pH to solubilize proteins resulted to be the most important parameter to maximize protein recovery. In this manner, Trial 4 recovered only 27.4% of the proteins when using pH 12, while Trial 5 recovered 54.5% of the initial protein content. Hence, when pH is not optimized, an important amount of proteins may be joined to cellular structures inside the cell and they may not be recovered.
In contrast to consecutive extraction, during autoclave pretreatment and further alkaline treatment (Trial 2) an important amount of proteins were degraded (close to 20%), probably due to the amino acid hydrolysis during autoclave pretreatment. This hypothesis is in agreement with Papadopoulos et al. (1986), who also evidenced the degradation effects of thermal pretreatments of feather meal on proteins and amino acids.
Mass balances in terms of TS, VS, ash, and protein content were performed under the optimal extraction conditions (Trial 5) for PC and by-products. It is important to note the high protein concentration recovered despite of the thick cell wall of this microalga (Miranda et al., 2012). The maximum protein recovery was 54.5% of initial protein content (Table 2), achieving a final protein concentration in the PC of 845 g protein kg TS−1 of PC. These data demonstrate the effectiveness of the treatment. The results presented herein showed higher protein recoveries than those previously reported by Gerde et al. (2013), who optimized the isolation of proteins from Nannochloropsis biomass obtaining protein recoveries close to 30 g protein per 100 g microalga and with protein concentrations close to 57%.
Protein Quality of the Protein Concentrate and Suitability as Fish Feed
The use of PC obtained from the optimized protein extraction (Trial 5) may be an interesting source of proteins for aquaculture. Table 3 reveals the high nutritional aptitude of this biomass due to its high content in EAA. Additionally, the amino acid profile in PC did not change during the protein extraction process, thus degradation of proteins or amino acids did not take place. The requirements of the rainbow trout fit well with those present in the PC (Blanco-Cachafeiro, 1995); being thus a balanced protein source that could be used for fish feed, partially replacing the use of amino acid supplements. Tryptophan (Trp) was determined in the PC fraction and in the free amino acids present in PC fraction accounting 1.4 g per 100 g proteins.
The key amino acids included in fish feed diet in terms of interest and costs are Lys and methionine (Met), which are EAA and they are usually supplemented since the fish requirements are higher than its content in traditional biomass. However, when protein content comes from microalgal biomass extraction, Lys and Met may be found as free amino acids, increasing digestibility and diminishing its supplementation. According to Fleurence (1999), the use of algae in fish diets improves body weight gain and increases the triglyceride and protein deposition in muscle. Overall, the use of protein concentrates from microalgal biomass for fish feed like rainbow trout may be an interesting alternative to substitute the proteins of traditional ingredients like fish and vegetable meals, due to high protein content, high EAA, important amount of Lys and Met, free amino acids and low ash content. Furthermore, the low pH values reached during protein extraction could inhibit the growth of different enterobacterium (Engberg et al., 2009), proving a protective effect against pathogenic enterobacterium in fish as Yersinia ruckerii and Edwarsiella spp.
Results presented by Vizcaíno et al. (2014), showed that one of the main advantages on the use of microalgal biomass compared to plants is the low content in ANFs, therefore no negative effects on growth or on digestion enzymes activity is expected. In this sense, in a preliminary assay in rainbow trout with increasing concentrations of Scenedesmus spp. (Larrán-García et al., 2017) up to 10% in feed formulation, negative effects in growth rates were not observed. Thus, similar results would be expected using PC, where amino acid digestibility is higher, proteins are more accessible to fish enzymes and ash content is remarkably lower (from 15.4 to 1.9%).
Hence, according to the results previously reported, PC fraction has an ideal nutritional quality due to: (i) high protein concentration, (ii) the amino acid profile fits well with rainbow trout requirements, and (iii) ash content is lower than 5%. Also, the increase in Phe in the PC fraction (from 4.6 to 5.4%) and in free Lys (from 0 to 0.5%) could diminish amino acid supplementation, making therefore, this biomass a suitable candidate source to fulfill fish feed requirements.
Anaerobic Digestion of By-Products Obtained After Protein Extraction
The feasibility of using the resulting by-products (SP and LF) as sole and combined substrates for anaerobic digestion was evaluated. The use of these substrates is interesting due to SP and LF are complementary in terms of pH and volume of resulting by-product. The highest methane yield was obtained for M1 (180.7 mL CH4 ) for buffered samples and N1 (96.5 mL CH4 ) for unbuffered samples. In order to study the biodegradability of the different mixtures, theoretical methane yields were calculated. The theoretical methane yield of the substrates was calculated based on their macromolecular composition in terms of lipids, carbohydrates and proteins. According to Sialve et al. (2009) each gram of lipids, carbohydrates and proteins results in 1.014, 0.415, and 0.521 mL of CH4, respectively. According to the theoretical productivity of CH4 for M1-M5, the highest production would occur on M1 (343 mL CH4 g ), while the lowest would take place on M5 (305 mL CH4 g ). Hence, differences between methane yields in all assays should be small. However, after anaerobic digestion of buffered by-products, experimental methane yields differed between 47 and 60% from the theoretical ones (i.e., in the case of M1, theoretical methane production was 343 mL CH4 g and the experimental yield resulted in 181 mL CH4 g , resulting in a productivity reduction of 47%). Moreover, when the anaerobic digestion media was not buffered (Figure 3B; N1-N5), methane yields ranged between 96.5 and 0 mL CH4 g , differences between theoretical and experimental methane yields differed between 71.3 and 100% from the theoretical ones. The highest experimental methane yield (96.5 mL CH4 g ) corresponds to the assay with the highest content of LF when the medium was buffered (M1), as can be seen in Figure 3. Methane yield decreased concomitantly with the decrease of LF in the mixture. This result evidenced the high biodegradability of LF, which is mainly composed by solubilized monosaccharides, in comparison with SP, which is mainly composed by proteins and lipids inside unbroken cellular structures and hindering thus the access to anaerobic bacteria. Results from Table 4 revealed a direct correlation between methane yield and VS removal and total COD removal for all the assays performed. Hernández et al. (2014) obtained similar methane yields (203 mL CH4 g ) using the same microalga (Scenedesmus) than those observed in the present work, when producing biogas from the resulting by-products after lipid extraction through a mechanical pretreatment (supercritical extraction).
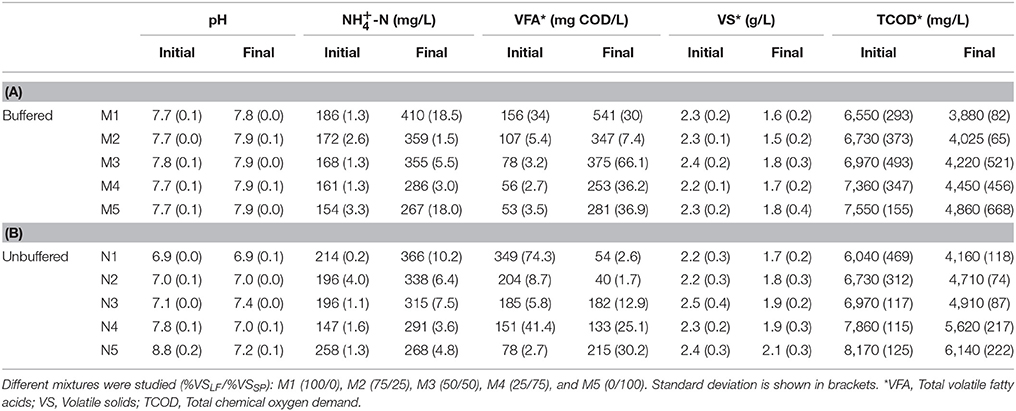
Table 4. Evolution of pH, NH-N, total volatile fatty acids, volatile solids and total chemical oxygen demand previous and after anaerobic digestion process of (A) buffered (M1-M5) and (B) unbuffered samples (N1–N5).
In unbuffered assays (N1–N5), methane production was considerably lower than in buffered assays (M1–M5). These differences may be attributed to a partial inhibition of the anaerobic digestion in the unbuffered assays. Buffered assays were performed to determine the methane potential of each mixture. Table 4 presents initial and final values of pH, NH-N, VFA, VS, and totalCOD concentration before and after anaerobic digestion for buffered and unbuffered assays. All final pH values oscillated from 6.9 to 7.9, being compatible with normal development of microorganisms. Final ammonium concentrations (Table 4) were below inhibitory threshold levels (from 1,700 to 5,000 mg NH-N L−1; Stams et al., 2003) reaching the maximum concentration in M1 (409.5 mg NH-N L−1). Under these ammonia concentrations, the biogas production is not affected and acetate-utilizing methanogenic Archaea, hydrogen-utilizing methanogens and syntrophic bacteria are not influenced (Zeng et al., 2010). Previous anaerobic digestion, the VFA concentration was lower than inhibitory threshold (from 1,000 to 6,000 mg COD L−1 (Siegert and Banks, 2005), ranging from 52.6 to 349.2 mg COD L−1 for all trials performed. After anaerobic digestion, the VFA concentration was also lower than inhibitory values ranging from 253 to 540 mg COD/L for buffered assays and from 40 to 215 mg COD L−1 for unbuffered assays.
The IA and the PA were determined previous and after anaerobic digestion. In PA titration from original sample to pH 5.75 corresponds to bicarbonate alkalinity. In IA alkalinity, titration from pH 5.75 to 4.3 approximates to VFA alkalinity. Successful anaerobic digestion depends on both maintenance of adequate bicarbonate buffering and avoidance of excessive VFA concentrations, hence when the IA:PA ratio ranges from 0.1 to 0.35 it is considered well-operated digesters (Ripley et al., 1986). In the buffered trials, IA:PA ratio ranged from 0.17 to 0.29 while in unbuffered trials IA:PA ratio it ranged from 1.4 to 0.78. Thus, in unbuffered trials a partial inhibition of the process probably took place, especially in N4 and N5, where anaerobic digestion was not even initiated (Figure 3). The percentage of methane content in biogas in N1-N5 varied from 64 to 7%, decreasing when SP content increases, demonstrating that high SP content in the mixture affected negatively to the anaerobic digestion, when assays were not buffered. In this context, the results presented herein, evidenced the need to buffer the culture medium to stabilize the process.
In this context, the optimization of protein extraction from Scenedesmus allows not only to higher protein recovery, but also it could increase the biodegradability of the resultant biomass due to a higher cell wall break down as previously reported by Hernández et al. (2014). The use of NaHCO3 in M1–M5 samples decreased the IA:PA ratio to values lower than 0.3, resulting in higher methane productions. From an economical point of view, the use of NaHCO3 incurs in high cost, decreasing profitability of the anaerobic digestion process that can hardly be performed in a pilot plant. In this context, the production of methane from samples N1 and N2 results more interesting, and therefore a co-substrate should be added in order to buffer the process.
Future Directions
To ensure the successful implementation of the protein extraction process for fish feed and further anaerobic digestion, the following parameters must be optimized: (i) the use of different microalgal species must be carried out to confirm the results obtained herein, (ii) the protein extraction should be performed in a pilot-scale size, (iii) different diets should be tested using intact microalgae and protein concentrates extracted from microalgae as main protein source in trials with different fish species, (iv) a techno-economical evaluation of protein extraction and anaerobic digestion process compared to the use of intact microalgae as protein source should be performed.
Conclusions
It is possible to recover protein concentrates from microalgae biomass obtained after pig manure treatment. The highest protein recovery was 54.5 ± 3.2%, obtained after a sonication pretreatment, followed by solubilization by double alkaline treatment and a precipitation step at pH 3.5. Protein concentration in the protein concentrate reached 845 g protein per 1,000 g biomass (TS), being an optimal biomass in terms of essential amino acids, resulting in a suitable protein source for fish feed. Methane yields up to 181 mL CH4 g were obtained using 100% the liquid fraction obtained after protein extraction as substrate. The higher LF content in the anaerobic digestion, the higher methane yield. Therefore, anaerobic digestion of the obtained by-products is only feasible if the anaerobic media is buffered. Therefore, the use of PC for fish feed may be a suitable source of proteins for fish feed due to: high protein content, high EAA, low ash, absence of ANF and high amino acid digestibility.
Author Contributions
DH performed the experimental set up and wrote the manuscript. BR and MG-G performed the experimental design and contributed to the revision of the discussion. BM-S contributed to discussion and the revision of the manuscript. CT-A and AL-G gave feedback in aquaculture and protein supplementation for fish feeding.
Conflict of Interest Statement
The authors declare that the research was conducted in the absence of any commercial or financial relationships that could be construed as a potential conflict of interest.
Acknowledgments
This study was supported by INIA (INIA project RTA 2013-056-C03). The authors also gratefully acknowledge to Bernar Fradejas (ITACyL) for his technical support and to the Department of Chemical Engineering, University of Almería (Spain).
References
APHA (2005). Standard Methods for the Examination of Water and Wastewater, 21st Edn. Washington, DC: American Public Health Association, American Water Works Association and Water Environment Federation.
Ba, F., Ursu, A. V., Laroche, C., and Djelveh, G. (2016). Haematococcus pluvialis soluble proteins: extraction, characterization, concentration/fractionation and emulsifying properties. Bioresour. Technol. 200, 147–152. doi: 10.1016/j.biortech.2015.10.012
Becker, E. W. (2007). Micro-algae as a source of protein. Biotechnol. Adv. 25, 207–210. doi: 10.1016/j.biotechadv.2006.11.002
Bisson, M. A., and Kirst, G. O. (1995). Osmotic acclimation and turgor pressure regulation in algae. Naturwissenschaften 82, 461–471. doi: 10.1007/BF01131597
Boisen, S., Hvelplund, T., and Weisbjerg, M. R. (2000). Ideal amino acid profiles as a basis for feed protein evaluation. Livest. Prod. Sci. 64, 239–251. doi: 10.1016/S0301-6226(99)00146-3
Chisti, Y. (2013). Constraints to commercialization of algal fuels. J. Biotechnol. 167, 201–214. doi: 10.1016/j.jbiotec.2013.07.020
Colleran, E., Concannon, F., Golden, T., Geoghegan, F., Crumlish, B., Killilea, E., et al. (1992). Use of methanogenic activity tests to characterize anaerobic sludges, screen for anaerobic biodegradability and determine toxicity thresholds against individual anaerobic trophic groups and species. Water Sci. Technol. 25, 31–40. doi: 10.2166/wst.1992.0136
Collins, S. (2014). Antinutritional Factors in Modeling Plant-Based Rainbow Trout Diets. Doctoral dissertation, University of Saskatchewan, Saskatoon, 215.
Dallaire, V., Lessard, P., Vandenberg, G., and de la Noüe, J. (2007). Effect of algal incorporation on growth, survival and carcass composition of rainbow trout (Oncorhynchus mykiss) fry. Bioresour. Technol. 98, 1433–1439. doi: 10.1016/j.biortech.2006.05.043
de Godos, L., Blanco, S., García-Encina, P. A., Becares, E., and Mu-oz, R. (2009). Long-term operation of high rate algal ponds for the bioremediation of piggery wastewaters at high loading rates. Bioresour. Technol. 100, 4332–4339. doi: 10.1016/j.biortech.2009.04.016
del Mar Morales-Amaral, M., Gómez-Serrano, C., Acién, F. G., Fernández-Sevilla, J. M., and Molina-Grima, E. (2015). Outdoor production of Scenedesmus sp. in thin-layer and raceway reactors using centrate from anaerobic digestion as the sole nutrient source. Algal Res. 12, 99–108. doi: 10.1016/j.algal.2015.08.020
Dubois, M., Gilles, K. A., Hamilton, J. K., Rebers, P. T., and Smith, F. (1956). Colorimetric method for determination of sugars and related substances. Anal. Chem. 28, 350–356. doi: 10.1021/ac60111a017
Ehimen, E. A., Sun, Z. F., Carrington, C. G., Birch, E. J., and Eaton-Rye, J. J. (2011). Anaerobic digestion of microalgae residues resulting from the biodiesel production process. App. Energy 88, 3454–3463. doi: 10.1016/j.apenergy.2010.10.020
Engberg, R. M., Hammersh j, M., Johansen, N. F., Abousekken, M. S., Steenfeldt, S., and Jensen, B. B. (2009). Fermented feed for laying hens: effects on egg production, egg quality, plumage condition and composition and activity of the intestinal microflora. Br. Poult. Sci. 50, 228–239. doi: 10.1080/00071660902736722
FAO. (2014). “The state of world fisheries and aquaculture, opportunities and challenges,” in World Review of Fisheries and Aquaculture, ed J. Grazianoda Silva (Rome: FAO), 3.
Fleurence, J. (1999). Seaweed proteins: biochemical, nutritional aspects and potential uses. Trends Food Sci. Technol. 10, 25–28. doi: 10.1016/S0924-2244(99)00015-1
García, D., Posadas, E., Blanco, S., Acién, G., García-Encina, P., Bolado, S., et al. (2018). 40) Evaluation of the dynamics of microalgae population structure and process performance during piggery wastewater treatment in algal-bacterial photobioreactors. Bioresour. Technol. 248, 120–126. doi: 10.1016/j.biortech.2017.06.079
Gerde, J. A., Wang, T., Yao, L., Jung, S., Johnson, L. A., and Lamsal, B. (2013). Optimizing protein isolation from defatted and non-defatted Nannochloropsis microalgae biomass. Algal Res. 2, 145–153. doi: 10.1016/j.algal.2013.02.001
Günerken, E., d'Hondt, E., Eppink, M. H. M., Garcia-Gonzalez, L., Elst, K., and Wijffels, R. H. (2015). Cell disruption for microalgae biorefineries. Biotechnol. Adv. 33, 243–260. doi: 10.1016/j.biotechadv.2015.01.008
Hernández, D., Riaño, B., Coca, M., and García-González, M. C. (2013). Treatment of agro-industrial wastewater using microalgae–bacteria consortium combined with anaerobic digestion of the produced biomass. Bioresour. Technol. 135, 598–603. doi: 10.1016/j.biortech.2012.09.029
Hernández, D., Riaño, B., Coca, M., and García-González, M. C. (2015). Saccharification of carbohydrates in microalgal biomass by physical, chemical and enzymatic pre-treatments as a previous step for bioethanol production. Chem. Eng. J. 262, 939–945. doi: 10.1016/j.cej.2014.10.049
Hernández, D., Riaño, B., Coca, M., Solana, M., Bertucco, A., and Garcia-Gonzalez, M. C. (2016). Microalgae cultivation in high rate algal ponds using slaughterhouse wastewater for biofuel applications. Chem. Eng. J. 285, 449–458. doi: 10.1016/j.cej.2015.09.072
Hernández, D., Solana, M., Riaño, B., García-González, M. C., and Bertucco, A. (2014). Biofuels from microalgae: lipid extraction and methane production from the residual biomass in a biorefinery approach. Bioresour. Technol. 170, 370–378. doi: 10.1016/j.biortech.2014.07.109
Kebede-Westhead, E., Pizarro, C., and Mulbry, W. W. (2006). Treatment of swine manure effluent using freshwater algae: production, nutrient recovery, and elemental composition of algal biomass at four effluent loading rates. J. App. Phycol. 18, 41–46. doi: 10.1007/s10811-005-9012-8
Kiron, V., Sørensen, M., Huntley, M., Vasanth, G. K., Gong, Y., Dahle, D., et al. (2016). Defatted biomass of the microalga, Desmodesmus sp., can replace fishmeal in the feeds for Atlantic salmon. Front. Mar. Sci. 3:67. doi: 10.3389/fmars.2016.00067
Kochert, G. (1978). Quantitation of the macromolecular components of microalgae. Handbook of phycological methods. Physiol. Biochem. Meth. 189–195.
Larrán-García, A. M., Tomás Almenar, C., and de Mercado, P. E. (2017). “Estudio del Contenido de Metales Pesados en Músculo de trucha arco Iris (Oncorhynchus mykiss) alimentadas con microalgas procedentes del tratamiento de purines,” in XVI Congreso Nacional de Acuicultura (Zaragoza), P-006.
Lundquist, T. J., Woertz, I., and Benemann, J. R. (2010). “Microalgae for wastewater treatment and biofuels production,” in Abstracts of Papers of the American Chemical Society (Washington, DC) 1155.
Masojídek, J., Kopecký, J., Giannelli, L., and Torzillo, G. (2011). Productivity correlated to photobiochemical performance of Chlorella mass cultures grown outdoors in thin-layer cascades. J. Industr. Microbiol. Biotechnol. 38, 307–317. doi: 10.1007/s10295-010-0774-x
Miranda, J. R., Passarinho, P. C., and Gouveia, L. (2012). Pre-treatment optimization of Scenedesmus obliquus microalga for bioethanol production. Bioresour. Technol. 104, 342–348. doi: 10.1016/j.biortech.2011.10.059
Möllers, K. B., Cannella, D., Jørgensen, H., and Frigaard, N. U. (2014). Cyanobacterial biomass as carbohydrate and nutrient feedstock for bioethanol production by yeast fermentation. Biotechnol. Biofuels 7:64. doi: 10.1186/1754-6834-7-64
Olvera-Novoa, M. A., Dominguez-Cen, L. J., Olivera-Castillo, L., and Martinez-Palacios, C. A. (1998). Effect of the use of the microalga Spirulina maxima as fish meal replacement in diets for tilapia, Oreochromis mossambicus (Peters), fry. Aquaculture Res. 29, 709–715. doi: 10.1046/j.1365-2109.1998.29100709.x
Papadopoulos, M. C., El Boushy, A. R., Roodbeen, A. E., and Ketelaars, E. H. (1986). Effects of processing time and moisture content on amino acid composition and nitrogen characteristics of feather meal. Animal Feed Sci. Technol. 14, 279–290. doi: 10.1016/0377-8401(86)90100-8
Patterson, D., and Gatlin, D. M. (2013). Evaluation of whole and lipid-extracted algae meals in the diets of juvenile red drum (Sciaenops ocellatus). Aquaculture 416, 92–98. doi: 10.1016/j.aquaculture.2013.08.033
Posadas, E., Muñoz, R., and Guieysse, B. (2017). Integrating nutrient removal and solid management restricts the feasibility of algal biofuel generation via wastewater treatment. Algal Res. 22, 39–46. doi: 10.1016/j.algal.2016.11.019
Riaño, B., Hernández, D., and García-González, M. C. (2012). Microalgal-based systems for wastewater treatment: effect of applied organic and nutrient loading rate on biomass composition. Ecol. Eng. 49, 112–117. doi: 10.1016/j.ecoleng.2012.08.021
Ripley, L. E., Boyle, W. C., and Converse, J. C. (1986). Improved alkalimetric monitoring for anaerobic digestion of high-strength wastes. J. Water Pollution Control 5, 406–411.
Santamaría-Fernández, M., Molinuevo-Salces, B., Lübeck, M., and Uellendahl, H. (in press). Biogas potential of green biomass after protein extraction in an organic biorefinery concept for feed, fuel fertilizer production. Renew Energ. doi: 10.1016/j.renene.2017.03.012
Sialve, B., Bernet, N., and Bernard, O. (2009). Anaerobic digestion of microalgae as a necessary step to make microalgal biodiesel sustainable. Biotechnol. Adv. 27, 409–416. doi: 10.1016/j.biotechadv.2009.03.001
Siegert, I., and Banks, C. (2005). The effect of volatile fatty acid additions on the anaerobic digestion of cellulose and glucose in batch reactors. Process Biochem. 40, 3412–3418. doi: 10.1016/j.procbio.2005.01.025
Sluiter, A., Hames, B., Ruiz, R., Scarlata, C., Sluiter, J., Templeton, D., et al. (2008). Determination of structural carbohydrates and lignin in biomass. Lab. Anal. Procedure 1617, 1–16.
Sørensen, M., Berge, G. M., Reitan, K. I., and Ruyter, B. (2016). Microalga Phaeodactylum tricornutum in feed for Atlantic salmon (Salmo salar) —effect on nutrient digestibility, growth and utilization of feed. Aquaculture 460, 116–123. doi: 10.1016/j.aquaculture.2016.04.010
Stams, A. J. M., Elferink, S. O., and Westermann, P. (2003). “Metabolic interactions between methanogenic consortia and anaerobic respiring bacteria,” in Biomethanation I, ed B. K. Ahring (Berlin; Heidelberg: Springer), 31–56.
Sterner, R. W. (1993). Daphnia growth on varying quality of Scenedesmus: mineral limitation of zooplankton. Ecology 74, 2351–2360. doi: 10.2307/1939587
Ursu, A. V., Marcati, A., Sayd, T., Sante-Lhoutellier, V., Djelveh, G., and Michaud, P. (2014). Extraction, fractionation and functional properties of proteins from the microalgae Chlorella vulgaris. Bioresour. Technol. 157, 134–139. doi: 10.1016/j.biortech.2014.01.071
Vizcaíno, A. J., López, G., Sáez, M. I., Jiménez, J. A., Barros, A., Hidalgo, L., et al. (2014). Effects of the microalga Scenedesmus almeriensis as fishmeal alternative in diets for gilthead sea bream, Sparus aurata, juveniles. Aquaculture 431, 34–43. doi: 10.1016/j.aquaculture.2014.05.010
Xie, F., Lin, X., Wu, X., and Xie, Z. (2008). Solid phase extraction of lead (II), copper (II), cadmium (II) and nickel (II) using gallic acid-modified silica gel prior to determination by flame atomic absorption spectrometry. Talanta 74, 836–843. doi: 10.1016/j.talanta.2007.07.018
Yuan, Q., Sparling, R., and Oleszkiewicz, J. A. (2011). VFA generation from waste activated sludge: effect of temperature and mixing. Chemosphere 82, 603–607. doi: 10.1016/j.chemosphere.2010.10.084
Keywords: microalgae, protein extraction, amino acids, fish feed, nutrient recovery, pig manure, by-products, anaerobic digestion
Citation: Hernández D, Molinuevo-Salces B, Riaño B, Larrán-García AM, Tomás-Almenar C and García-González MC (2018) Recovery of Protein Concentrates From Microalgal Biomass Grown in Manure for Fish Feed and Valorization of the By-Products Through Anaerobic Digestion. Front. Sustain. Food Syst. 2:28. doi: 10.3389/fsufs.2018.00028
Received: 08 February 2018; Accepted: 04 June 2018;
Published: 22 June 2018.
Edited by:
Francisco Javier Salazar, Remehue Regional Research Center, Institute of Agricultural Research, ChileReviewed by:
M. Angeles de la Rubia, Universidad Autonoma de Madrid, SpainAnders Peter S. Adamsen, SEGES Agriculture & Food FmbA, Denmark
Copyright © 2018 Hernández, Molinuevo-Salces, Riaño, Larrán-García, Tomás-Almenar and García-González. This is an open-access article distributed under the terms of the Creative Commons Attribution License (CC BY). The use, distribution or reproduction in other forums is permitted, provided the original author(s) and the copyright owner are credited and that the original publication in this journal is cited, in accordance with accepted academic practice. No use, distribution or reproduction is permitted which does not comply with these terms.
*Correspondence: David Hernández, aGVyZ29uZGFAaXRhY3lsLmVz
Maria C. García-González, Z2FyZ29ubWlAaXRhY3lsLmVz