- 1Institute of Systems Biology (INBIOSIS), Universiti Kebangsaan Malaysia, Bangi, Malaysia
- 2Faculty of Pharmacy, Universitas Halu Oleo, Kendari, Indonesia
Xanthones are secondary metabolites rich in structural diversity and possess a broad array of pharmacological properties, such as antitumor, antidiabetic, and anti-microbes. These aromatic compounds are found in higher plants, such as Clusiaceae, Hypericaceae, and Gentianaceae, yet their biosynthetic pathways have not been comprehensively updated especially within the last decade (up to 2021). In this review, plant xanthone biosynthesis is detailed to illuminate their intricacies and differences between species. The pathway initially involves the shikimate pathway, either through L-phenylalanine-dependent or -independent pathway, that later forms an intermediate benzophenone, 2,3′,4,6-tetrahydoxybenzophenone. This is followed by a regioselective intramolecular mediated oxidative coupling to form xanthone ring compounds, 1,3,5-trihydroxyxanthone (1,3,5-THX) or 1,3,7-THX, the core precursors for xanthones in most plants. Recent evidence has shed some lights onto the enzymes and reactions involved in this xanthone pathway. In particular, several biosynthetic enzymes have been characterized at both biochemical and molecular levels from various organisms including Hypericum spp., Centaurium erythraea and Garcinia mangostana. Proposed pathways for a plethora of other downstream xanthone derivatives including swertianolin and gambogic acid (derived from 1,3,5-THX) as well as gentisin, hyperixanthone A, α-mangostin, and mangiferin (derived from 1,3,7-THX) have also been thoroughly covered. This review reports one of the most complete xanthone pathways in plants. In the future, the information collected here will be a valuable resource for a more directed molecular works in xanthone-producing plants as well as in synthetic biology application.
Introduction
Xanthones have been studied for more than five decades and are known to possess diverse structures, functions, and biochemical activities (Carpenter et al., 1969; Sultanbawa, 1980; Bennett and Lee, 1989; Peres et al., 2000; El-Seedi et al., 2010). The word “xanthone” originated from the Greek word “xanthos,” meaning yellow. Xanthones are a class of plant phenolic compound with C6-C1-C6 carbon skeletal structure (Figure 1). The two aromatic rings in the xanthone basic skeleton are numbered and designated based on their biosynthetic origins in higher plants. A-ring is acetate-derived and its carbons are numbered 1–4 whereas B-ring is derived from shikimate pathway and the carbons are numbered 5–8 (Ramawat and Mérillon, 2013; Wezeman et al., 2015; Pinto et al., 2021). Both of these rings can fuse together through an oxygen atom and a carbonyl group to form the simplest class of xanthone known as 9H-xanthen-9-one that is also symmetric with the skeleton of dibenzo-γ-pyron (Figure 1; El-Seedi et al., 2009, 2010; Wezeman et al., 2015).
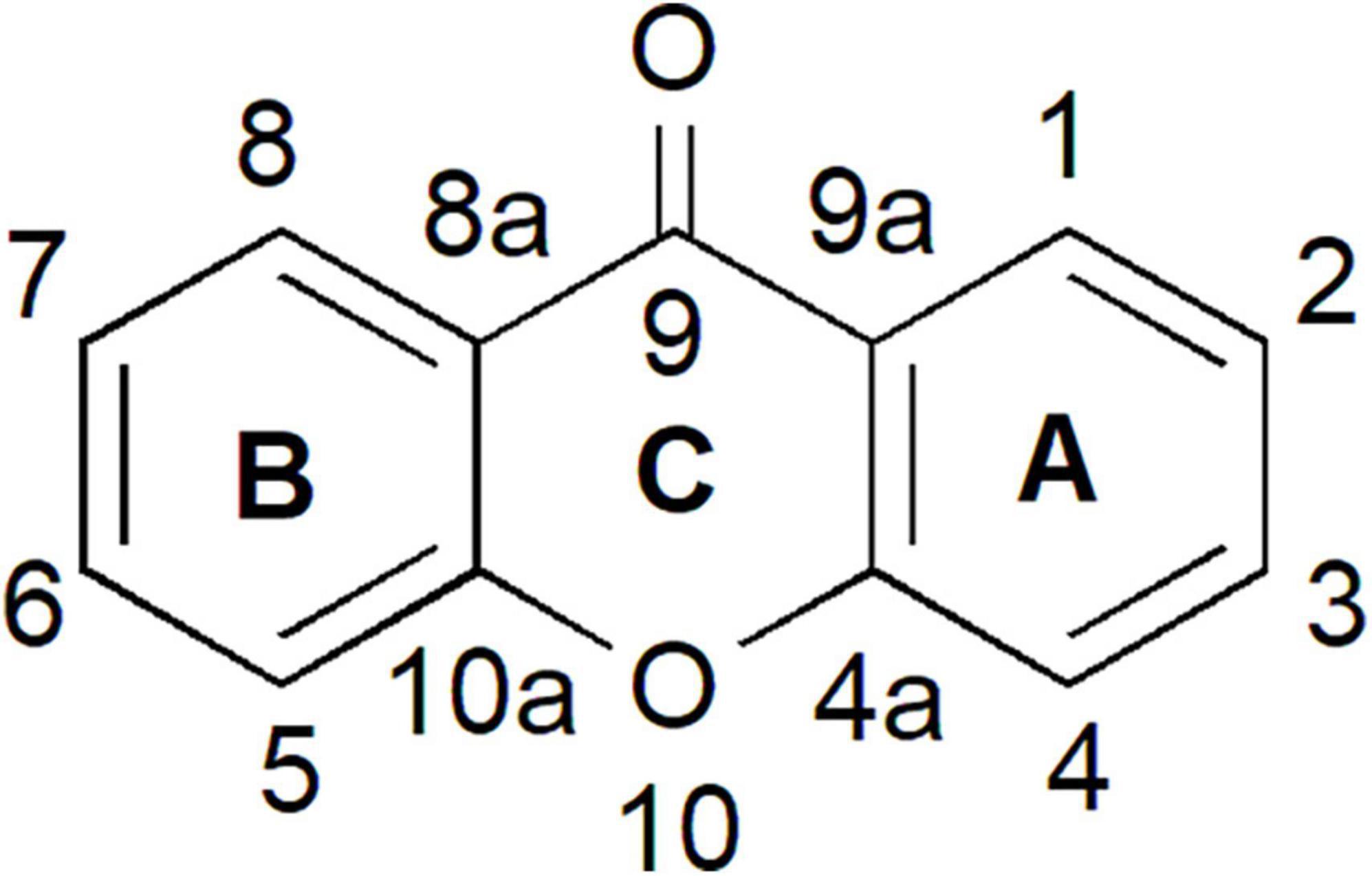
Figure 1. Xanthone is a phenolic compound with a planar dibenzo-γ-pyron scaffold. It contains conjugated aromatic ring system that is composed of two rings; carbons 1–4 (ring A) and carbons 5–8 (ring B) and attached together through an oxygen atom and a carbonyl group (ring C) to form the basic skeleton of xanthone known as 9H-xanthen-9-one.
Xanthones from natural sources contain various substituents on these two benzene rings, thus leading to wide structural diversity with a broad spectrum of activities (Sousa and Pinto, 2005; Tovilovic-Kovacevic et al., 2020). They are mainly classified into six main categories, namely, simple xanthones, glycosylated xanthones, prenylated xanthones, xanthonolignoids, bis-xanthones, and miscellaneous xanthones (Vieira and Kijjoa, 2005; El-Seedi et al., 2009). However, plants mostly produce xanthones in the first three categories (El-Seedi et al., 2009). Xanthones are also organized based on their quantity of oxygenation derivatives, prenylation, and glycosylation patterns. For instance, the simple oxygenated xanthones are further subdivided based on the level of oxygenation, such as non-, mono-, di-, tri-, tetra-, penta-, and hexa-oxygenated substances (Velíšek et al., 2008; El-Seedi et al., 2010; Masters and Bräse, 2012). Such a variety of xanthone structures allow their broad array of valuable pharmacological activities including as anti-microbes, antioxidant, anti-inflammation, antitumor, antidiabetic, anti-arthritis, as well as gastro-, liver- and cardio-protectant (Aizat et al., 2019; Marzaimi and Aizat, 2019).
Although xanthone structures and chromatographic patterns are comparable to that of flavonoids, xanthones are present in only a few restricted species (Jensen and Schripsema, 2002; Vieira and Kijjoa, 2005). For example, xanthones can only be isolated from 20 families of higher plants of which most of them reside within Clusiaceae, Hypericaceae, and Gentianaceae families (Tovilovic-Kovacevic et al., 2020), and to some extent in Calophyllaceae family (Gómez-Verjan et al., 2017; Zailan et al., 2021). Prenylated xanthones can be mainly isolated from different Garcinia species (Clusiaceae). These include α-mangostin, 7-O-methylgarcinone E, gartanin, garcinone E, and tovophyllin A from Garcinia mangostana (mangosteen; Ying et al., 2017; Mamat et al., 2020) and oblongifolixanthone A from Garcinia oblongifolia (also known as Lingnan Garcinia; Shan et al., 2012; Khan et al., 2020). Hypericum spp., such as Hypercium calycinum and Hypercium sampsonii from the Hypericaceae family, also produce prenylated xanthones, such as hyperxanthone E and patulone (Fiesel et al., 2015; Nagia et al., 2019). These prenyl groups can contribute to increasing bioactivities of xanthone due to their increasing lipophilicity to interact with biological membranes (Chen et al., 2017). Pendant sugars are also found as a structural feature of dimers in xanthones, for example, puniceaside C (xanthone glycosides from Swertia punicea; Zafar and Wang, 2018) and tocotrienol quinone dimer isolated from Garcinia nigrolineata (Raksat et al., 2019). In addition to that, C-glycosylated xanthones, such as mangiferin and isomangiferin, can be isolated from Mangifera indica (mango; Haynes and Taylor, 1966) and the aerial parts of Anemarrhena asphodeloides (Chinese herbs; Aritomi and Kawasaki, 1970). Mangiferin is also shown to be present widely among ferns (lower plants), such as Polypodiopsida or Polypodiophyta (Bennett and Lee, 1988). The different xanthone types from plants and their uses are described recently by Tovilovic-Kovacevic et al. (2020), and readers are directed to their review for more details.
Other than plants, xanthones can also originate from fungi, such as Aspergillus, Helminthosporium, Penicillium, and Pyrenochaeta (Bräse et al., 2009; Schätzle et al., 2012; Yoiprommarat et al., 2020), as well as lichens, such as Parmelia (Tuong et al., 2019), and bacteria, such as Streptomyces (Masters and Bräse, 2012). Interestingly, several simple methylated xanthones (1-methylxanthone, 2-methylxanthone, 3-methylxanthone, and 4-methylxanthone) can also be found from fossil fuels (Oldenburg et al., 2002; Masters and Bräse, 2012). The number of xanthone compounds from natural products has risen by 100 times over the last decades (El-Seedi et al., 2009). By July 2020, the number of xanthones recorded in the Dictionary of Natural Product has reached a staggering 2221 compounds1 (Supplementary Table 1), showing the diversity of this compound class. Nevertheless, the source of xanthone from plants remains dominant that counts approximately 80% of total natural xanthones in contrast to non-lichenized fungi (15%) and lichens (5%; Le Pogam and Boustie, 2016), and hence they become interesting subjects for xanthone studies.
Previous xanthone investigation encompassed various studies including structure–activity relationships (Pinto et al., 2005, 2021), xanthone production using biotechnological approaches (Gaid et al., 2019), in vitro and in vivo biological evaluation (Ovalle-Magallanes et al., 2017; Aizat et al., 2019), as well as structural (Wu et al., 2009) and isolation (Wang et al., 2013) studies. Given the increasing global demand for medicinal compounds, it is important to understand the biosynthesis of specialized metabolites, such as xanthones, as complete as possible, so that appropriate plant chemical resources can be established or developed in the future (Rai et al., 2017; Jamil et al., 2020). Thus, in parallel of their intriguing structural, biochemical, and pharmacological properties that they possess, this review aims to focus on gathering and updating the xanthone biosynthetic pathway in plants. This review also highlights the identification of enzymes involved in the pathway and describes their possible arrangement. In the future, this knowledge may improve synthetic biology efforts for a more sustainable production of plant natural products, such as xanthones or their benzophenone precursors, through metabolic engineering.
Several previous plant xanthone reviews have detailed various research aspects, for instance in depth analysis on chemistry/chemical synthesis, phytochemical/biological activities, and/or biotechnological applications through in vitro production of xanthones and their precursors (Mazimba et al., 2013; Li et al., 2017; Gaid et al., 2019; Khattab and Farag, 2020; Tovilovic-Kovacevic et al., 2020; Pinto et al., 2021). Additionally, a review by El-Seedi et al. (2010) covers various biosynthetic pathways in different organisms including plants, but a more updated review is needed considering the current findings in literature within the recent decade. Therefore, this review mainly covers research articles up to 2021, specifically on the topic of xanthone biosynthesis in plants, of which were screened from Web of Science, Scopus, Pubmed and Google Scholar. Furthermore, biosynthetic enzymes related to the xanthone pathway, isolated and/or characterized from xanthone-producing plants are also gathered (Table 1) and discussed to highlight the current advancement, and future direction toward completing the xanthone pathway.
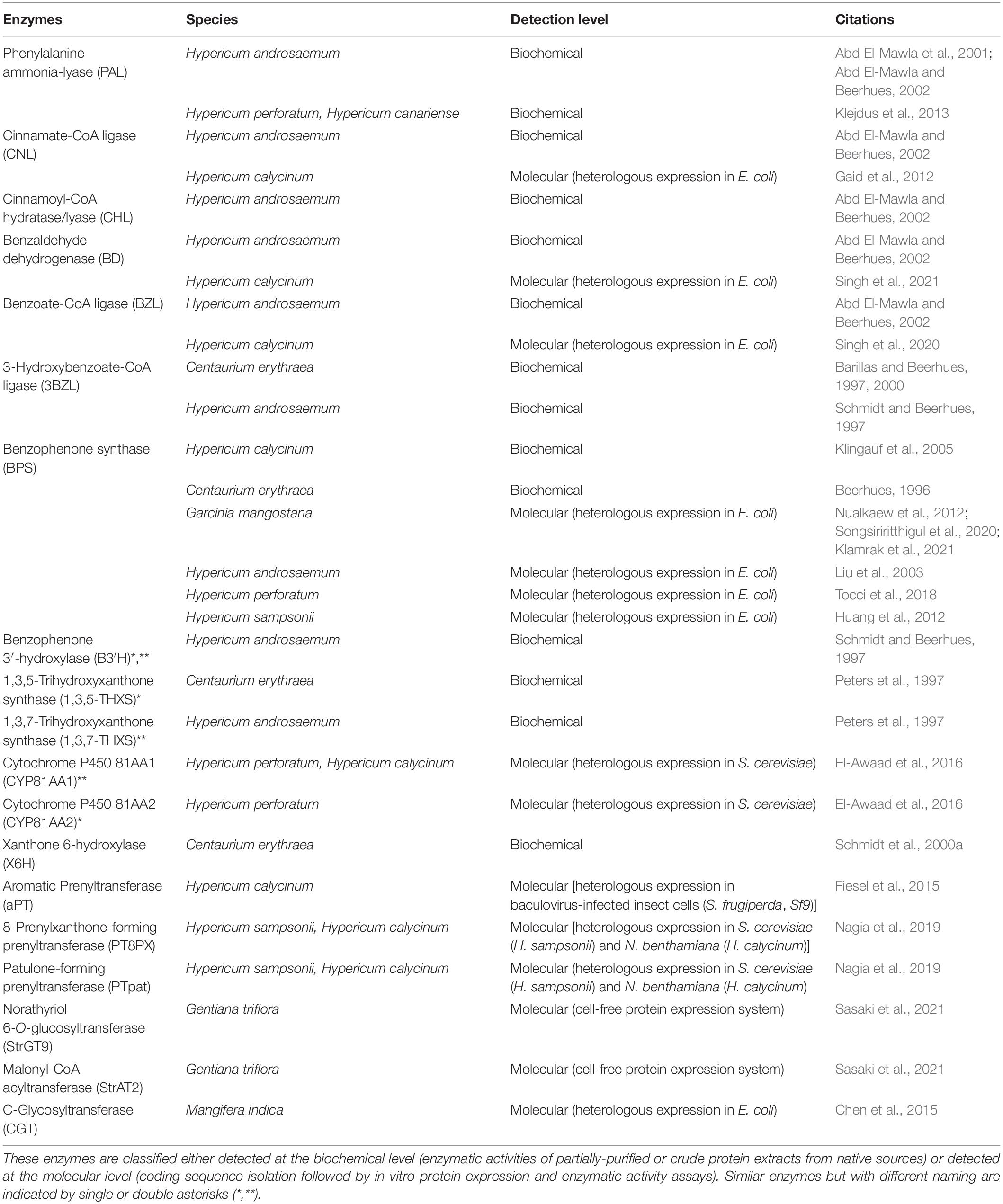
Table 1. Enzymes involved in the xanthone pathway characterized from various xanthone-producing plants.
Biosynthesis of Xanthone Core Structures in Plants
Xanthone biosynthesis in plants generally occurs via the established shikimate pathway, which links carbohydrate metabolism to aromatic compound biosynthesis (Supplementary Figure 1; Kumar et al., 2015; Fu et al., 2017; Li et al., 2017). Precursor compounds from glycolysis (phosphoenolpyruvate) and pentose phosphate pathway (erythrose 4-phosphate) are used in the synthesis of shikimate and subsequently L-phenylalanine through an elaborate pathway involving various enzymes and intermediates (Supplementary Figure 1). These precursors are important to generate benzophenone intermediates especially 2,3′,4,6-tetrahydroxybenzophenone (2,3′,4,6-tetraHBP; Figure 2), a central intermediate for xanthone biosynthesis in plants (El-Seedi et al., 2010). Interestingly, classical studies using radioactively labeled precursor compounds, such as [14C]L-phenylalanine, [14C]benzoic acid, and [14C]hydroxybenzoic acid, among others, showed that the pathway to produce the 2,3′,4,6-tetraHBP can vary between plant species (Atkinson et al., 1968; Gupta and Lewis, 1971; Abd El-Mawla et al., 2001; Abd El-Mawla and Beerhues, 2002). For example, Hypericaceae family (e.g., Hypericum androsaemum, H. calycinum, and H. sampsonii) mainly utilizes benzoic acid from L-phenylalanine to produce the benzophenone, whereas Gentianaceae family (Centaurium erythraea and Swertia chirata) uses 3-hydroxybenzoic acid from shikimate as a precursor compound (Figure 2; Abd El-Mawla et al., 2001; Wang et al., 2003; Singh et al., 2020).
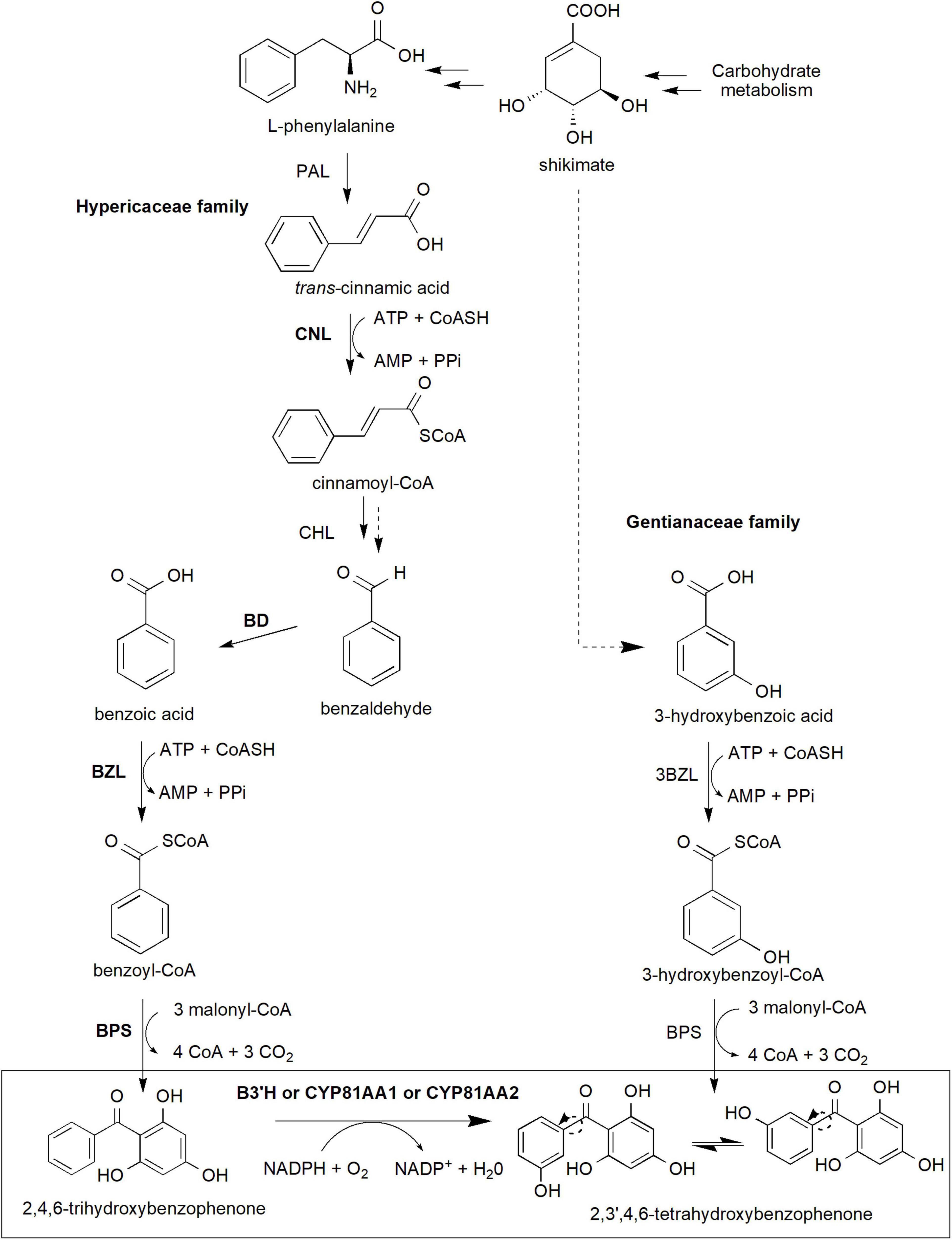
Figure 2. The core xanthone biosynthesis pathway in plants. The shikimate pathway supplies shikimate and L-phenylalanine precursors (detailed pathway is provided in Supplementary Figure 1) to produce benzophenone intermediates, in particular 2,3′,4,6-tetrahydroxybenzophenone isomers used for downstream xanthone biosynthesis. In Gentianaceae family, 3-hydroxybenzoic acid is formed from shikimate and subsequently to 3-hydroxybenzoyl-CoA and later 2,4,5′6-tetrahydroxybenzophenone. Meanwhile, Hypericaceae family utilizes L-phenylalananine-dependent pathway through several more reactions to produce 2,4,6-trihydroxybenzophenone and later the 2,3′,4,6-tetrahydroxybenzophenone. This latter reaction requires B3′H activity of which can be catalyzed by both CYP81AA1 or CYP81AA2 enzymes in Hypericum. Multiple arrows indicate multiple steps between intermediates while dotted arrows indicate hypothesized/proposed pathways. Protein activities that have been detected at molecular level are shown in bold while normal font type indicates protein activities detected at biochemical level (refer to Table 1). The two arrows (one unbroken line and one dotted) for Cinnamoyl-CoA hydratase/lyase (CHL) indicate that the enzymatic reaction has been characterized at the biochemical level from a crude protein extract, but whether this involves one or two enzymatic steps is yet to be validated at the molecular level. 3BZL, 3-hydroxybenzoate-CoA ligase; ATP, adenosine triphosphate; AMP, adenosine monophosphate; BD, benzaldehyde dehydrogenase; BZL, benzoate-CoA ligase; B3′H, benzophenone 3′-hydroxylase; BPS, benzophenone synthase; CHL, cinnamoyl-CoA hydratase/lyase; CNL, cinnamate-CoA ligase; CoASH, coenzyme A; NADPH, reduced nicotinamide adenine dinucleotide phosphate; PAL, phenylalanine ammonia-lyase; PPi, inorganic pyrophosphate.
In the L-phenylalanine-dependent pathway (Hypericaceae), the amino acid is converted to trans-cinnamic acid by the action phenylalanine ammonia-lyase (PAL) enzyme (Abd El-Mawla et al., 2001; Abd El-Mawla and Beerhues, 2002; Figure 2). PAL is the first committed step in the phenylpropanoid pathway, enabling dedicated carbon flux toward specialized metabolism in plants including xanthone biosynthesis (Gaid et al., 2012; Maeda and Dudareva, 2012; Lynch and Dudareva, 2020). However, despite a number of molecular studies (heterologous expression or homologous overexpression) has been conducted in various plant species (Hyun et al., 2011; Castro et al., 2020), PAL detection and characterization have mainly been conducted at the biochemical level in Hypericum spp. (Abd El-Mawla et al., 2001; Abd El-Mawla and Beerhues, 2002; Klejdus et al., 2013). Inhibition of the enzyme using 2-aminoindane-2-phosphonic acid in Hypercium perforatum and Hypercium canariense resulted in the significant reduction of total soluble phenols as well as benzoate and cinnamate derivatives (Klejdus et al., 2013), suggesting the central importance of PAL in the phenylpropanoid metabolism. Subsequently, the synthesis of cinnamoyl-CoA from the trans-cinnamic acid will be catalyzed by cinnamate-CoA ligase (CNL; Gaid et al., 2012). The CNL coding sequence has been previously cloned and characterized from various species including H. calycinum (Gaid et al., 2012), Arabidopsis thaliana (Lee et al., 2012), Petunia hybrida (Klempien et al., 2012) and Malus x domestica “Golden delicious” (Teotia et al., 2019).
The cinnamoyl-CoA intermediate is then synthesized to benzoyl-CoA which requires three more enzymatic reaction steps involving cinnamoyl-CoA hydratase/lyase (CHL), benzaldehyde dehydrogenase (BD) and benzoate-CoA ligase (BZL; Abd El-Mawla and Beerhues, 2002; Singh et al., 2020). Although the three proteins have been proven to exist and functioning at the biochemical level from various species (Abd El-Mawla and Beerhues, 2002; Beuerle and Pichersky, 2002; Gaid et al., 2009, 2019; Saini et al., 2017, 2020), only BD and BZL have been verified at the molecular level (coding sequence isolation followed by in vitro heterologous expression and enzymatic assays) in H. calycinum (Singh et al., 2020, 2021) and in snapdragon (Antirrhinum majus; BD only; Long et al., 2009). The latter BZL gene, also known as HcAAE1 (acyl-activating enzyme 1), has been shown to increase its expression prior to xanthone accumulation post-elicitation, suggesting its role upstream of the xanthone pathway (Singh et al., 2020). Additionally, the enzyme prefers benzoic acid substrate and is localized subcellularly at both peroxisomes and cytosol indicating the activation of CoA-dependent non-β-oxidative route for the benzoyl-CoA production (Singh et al., 2020).
Subsequent reaction by a Type III polyketide synthase called benzophenone synthase (BPS) condenses the benzoyl-CoA molecule with three malonyl-CoA resulting in the formation of 2,4,6-trihydroxybenzophenone (2,4,6-triHBP or also known as phlorbenzophenone; Figure 2; Beerhues et al., 2007; Beerhues and Liu, 2009; Nualkaew et al., 2012). The BPS enzyme was previously cloned from H. androsaemum, H. perforatum, H. sampsonii, and G. mangostana before its enzymatic activity and/or subcellular localization characterized (Liu et al., 2003; Klingauf et al., 2005; Huang et al., 2012; Nualkaew et al., 2012; Belkheir et al., 2016; Tocci et al., 2018; Klamrak et al., 2021). The expression of BPS has been shown to precede the increase in xanthone accumulation and that the protein and xanthone products were majorly localized to the exodermis region of the H. perforatum root, suggesting their roles as the first line of defense against soilborne pathogens (Tocci et al., 2018). Recently, the crystal structures of BPS from both H. androsaemum and G. mangostana were reported, further revealing their function and specificity toward benzoyl-CoA substrate to synthesize 2,4,6-triHBP (Stewart et al., 2017; Songsiriritthigul et al., 2020).
The 2,4,6-triHBP intermediate is then converted to 2,3′,4,6-tetraHBP by a cytochrome P450 (CYP) monooxygenase known as CYP81AA that possesses benzophenone 3′-hydroxylase (B3′H) activity (Figure 2; Schmidt and Beerhues, 1997; El-Awaad et al., 2016). Interestingly, two homologous CYP81AA enzymes exist, CYP81AA1 and CYP81AA2 in Hypericum spp. and have a bifunctional role to catalyze another downstream compound, either 1,3,7-trihydroxyxanthone (1,3,7-THX) or 1,3,5-trihydroxyxanthone (1,3,5-THX), respectively (El-Awaad et al., 2016).
Meanwhile, in the Gentianaceae family, the biosynthetic pathway originates from shikimate to produce 3-hydroxybenzoic acid via L-phenylalanine-independent pathway as confirmed by radioactively labeled precursors (Abd El-Mawla et al., 2001; Wang et al., 2003; Figure 2). The latter compound is then thioesterified to 3-hydroxybenzoyl-CoA by 3-hydroxybenzoate-CoA ligase (3BZL) in the presence of ATP and CoA, and subsequently, sequential condensation by BPS leads to the formation of 2,3′,4,6-tetraHBP (Barillas and Beerhues, 1997; Wang et al., 2003; Li et al., 2017). However, both 3BZL and BPS remain yet to be investigated at the molecular level in the Gentianaceae family, and only the former enzyme (3BZL) had been biochemically validated in C. erythraea (Beerhues, 1996; Barillas and Beerhues, 1997). Furthermore, the 3BZL could only efficiently activate 3-hydroxybenzoic acid rather than benzoic acid as substrates (Barillas and Beerhues, 1997), suggesting the activation of the L-phenylalanine-independent pathway in this Centaurium species. On the other hand, Aquilaria spp. from the Thymelaeaceae family is predicted to produce core xanthone structure through 4-hydroxybenzoyl-CoA, instead of the 3-hydroxybenzoyl-CoA (Li et al., 2021; Supplementary Figure 2).
The 2,4,6-triHBP and 2,3′4,6-tetraHBP are precursor compounds to various benzophenones (Supplementary Figure 3). These compounds, such as sampsonione A, hypercalin A, 2,4′,4,6-tetraHBP and 2,3′,4,4′,6-pentaHBP (maclurin), garcinol, and guttiferone A, are known to exhibit various biomedical and pharmaceutical benefits, such as antitumor (Rukachaisirikul et al., 2005; Koeberle et al., 2009; Lay et al., 2014; Behera et al., 2016) and anti-inflammatory (Liao et al., 2005; Pardo-Andreu et al., 2011), among others.
Oxidative phenol coupling reaction involving 2,3′,4,6-tetraHBP ring closure that occurs either at the ortho or para position of the 3′-OH group forms the two main core xanthone structures, 1,3,5-THX and 1,3,7-THX, respectively (Figure 3A). In C. erythraea, 2,3′,4,6-tetraHBP was shown at the biochemical level to regioselectively cyclize to 1,3,5-THX, whereas in H. androsaemum, (Peters et al., 1997; El-Awaad et al., 2016) and G. mangostana to 1,3,7-THX (Atkinson et al., 1968; Gupta and Lewis, 1971; Beerhues and Liu, 2009). The reaction mechanism that underlies the regioselective intramolecular mediated oxidative coupling during cyclization of the benzophenone is proposed to involve two stages of one-electron oxidation (Figure 3A). The loss of the first one-electron and a deprotonation produces a phenoxy radical, which cyclizes benzophenone through electrophilic attack (Figure 3A). Then, the hydroxy-cyclohexadienyl radical intermediate loses an electron and a proton to generate the 1,3,5-THX and 1,3,7-THX compounds (Peters et al., 1997; El-Seedi et al., 2010).
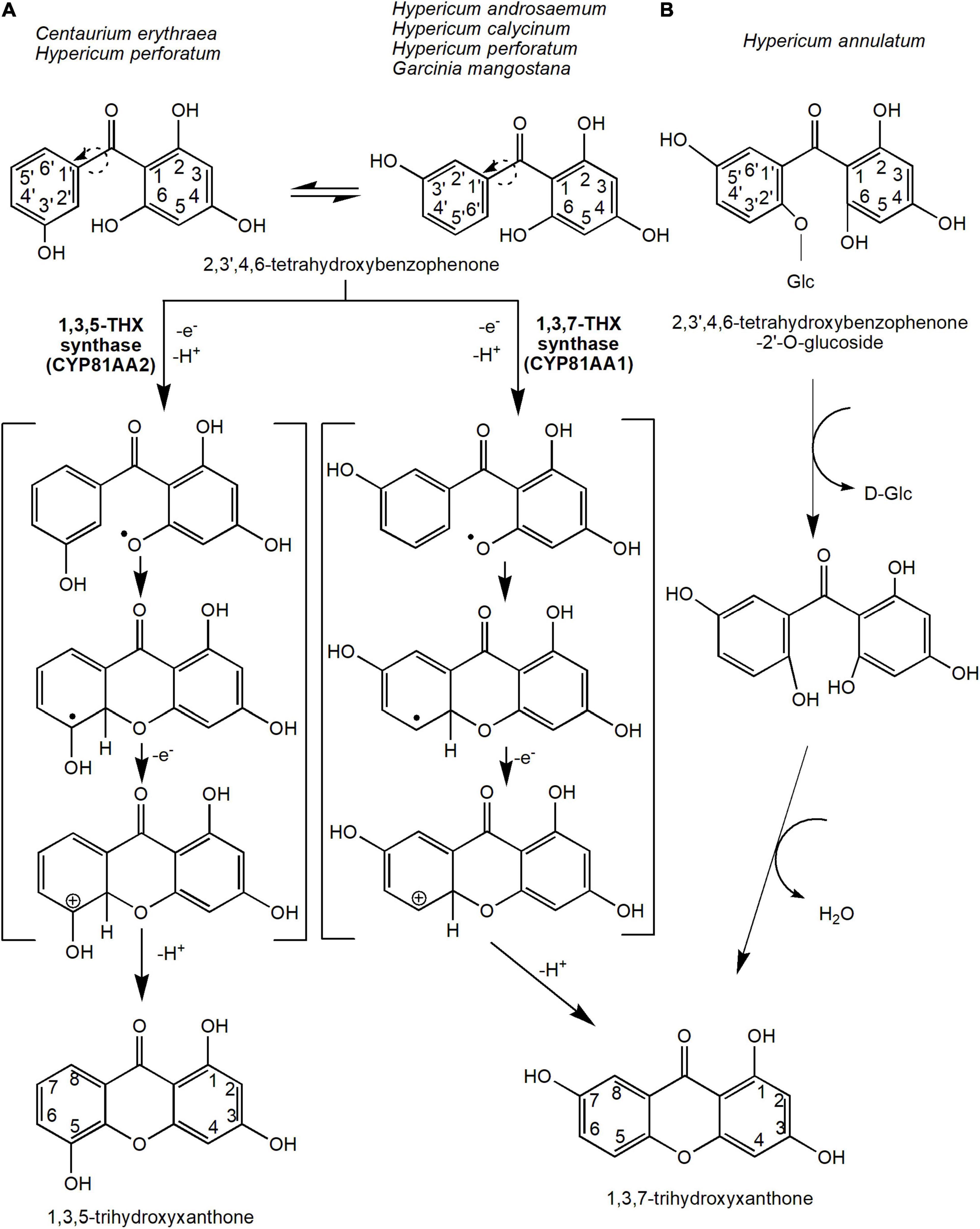
Figure 3. The reaction mechanisms for the two main precursors of xanthones, 1,3,5-trihydroxyxanthone and 1,3,7-trihydroxyxanthone. This oxidative phenol coupling reaction is present in several plant species including C. erythraea, H. androsaemum, H. calycinum, H. perforatum, and G. mangostana. (A) In addition, 1,3,7-trihydroxyxanthone can be formed through the deglycosidation process of 2,3′,4,6-tetrahydroxybenzophenone-2′-O-glucoside in H. annulatum. (B) Dotted arrows indicate hypothetical/proposed pathways while protein activities detected at the molecular level are in bold (refer to Table 1). CYP, cytochrome P450; THX, trihydroxyxanthone.
The enzymes that catalyze these reactions are originally known as xanthone synthases belonging to the CYP oxidases (Peters et al., 1997). However, they were later renamed to 1,3,5-THX synthase (or CYP81AA2) and 1,3,7-THX synthase (or CYP81AA1), respectively (El-Awaad et al., 2016; Khattab and Farag, 2020). These CYP81AA homologs isolated from H. perforatum and H. calycinum were expressed in yeast by El-Awaad et al. (2016), who showed that six substrate recognition sites are responsible for the regiospecificity of the enzymatic reactions, especially for the CYP81AA2. Interestingly, both enzymes were found in Hypericum spp. transcriptome databases (Gaid et al., 2012; El-Awaad et al., 2016). This suggests that both isomeric products could actually be synthesized in any one species, perhaps dependent upon certain physiological responses or signals. Recently, a metabolomics study in mangosteen also found putative 1,3,5-THX and a derivative of 1,3,7-THX at different tissues and stages of ripening (Mamat et al., 2020), further corroborating that certain species may possess both enzymes for the two different cyclization reactions. In the future, the application of sequencing efforts at either the genomics or transcriptomics levels on these xanthone-producing plants will undeniably help the identification and characterization of these vital biosynthetic enzymes.
Alternatively, 1,3,7-THX is proposed to be generated spontaneously from a different precursor compound such as 2,4,5′,6-tetrahydroxybenzophenone-2′-O-glucoside in Hypericum annulatum (Kitanov and Nedialkov, 2001; El-Seedi et al., 2010; Figure 3B). The glucoside group at the 2′ position of the 2,4,5′6-tetrahydroxybenzophenone-2′-O-glucoside molecule is first removed by enzymatic or acidic hydrolysis before cyclization of both rings (Kitanov and Nedialkov, 2001; Figure 3B).
These xanthones (1,3,5-THX and 1,3,7-THX) are the main precursors of most other xanthones; therefore, this intramolecular cyclization contributes an essential branch point from benzophenone intermediate to xanthone biosynthesis (Figure 3A). However, it is to be noted that most of the downstream xanthone pathway from these core xanthone precursors (Figures 3, 4) are only proposed reactions and only a handful of enzymes have been biochemically characterized previously (Table 1).
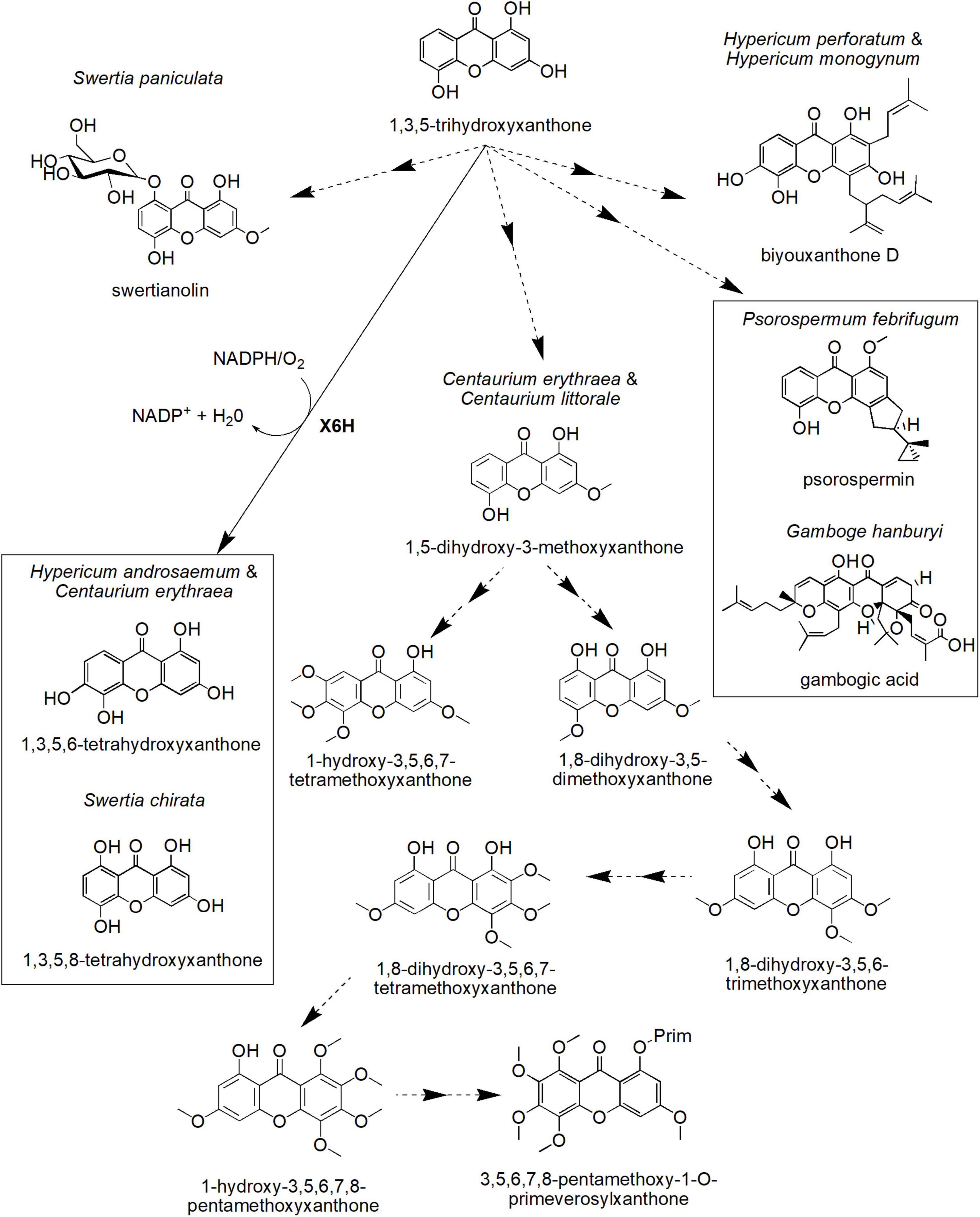
Figure 4. Proposed biosynthetic pathways of several xanthone derivatives derived from 1,3,5-trihydroxyxanthone precursor. X6H, xanthone 6-hydroxylase. Dotted arrows indicate hypothetical/proposed pathways while protein activities detected at the molecular level are in bold (refer to Table 1).
Biosynthetic Pathways of Xanthone Derivatives in Plants Through 1,3,5-Trihydroxyxanthone
One of the xanthone core compounds, 1,3,5-THX, can give rise to many more different xanthone structures (Figure 4). For instance, 1,3,5-THX can be hydroxylated to produce 1,3,5,6-tetrahydroxyxanthone in H. androsaemum and C. erythraea (Schmidt et al., 2000a), a compound known to induce diuresis and saluresis (Mariano et al., 2019, 2021). This process is catalyzed by the plant-specific CYP-dependent monooxygenase known as xanthone 6-hydroxylase (X6H) that has been biochemically determined to require NADPH and O2 (Barillas and Beerhues, 2000; Schmidt et al., 2000b). In S. chirata, 1,3,5-THX is also hydroxylated but at the C-8 position of the ring, contributing to 1,3,5,8-tetrahydroxyxanthone, the key xanthone in this species (Figure 4; Velíšek et al., 2008). The compound is known to be a potent inhibitor for angiopoietin-like protein 3 pathway to regulate ketosis, a metabolic disorder due to ketone body accumulation (Xiao et al., 2012).
On the other hand, the cell cultures of C. erythraea and Centaurium littorale treated with methyl jasmonate as well as yeast extract differentially accumulated other types of xanthones, such as 1,5-dihydroxy-3-methoxyxanthone and 1-hydroxy-3,5,6,7-tetramethoxyxanthone (Figure 4; Beerhues and Berger, 1995). Moreover, the cell cultures of C. erythraea accumulated 3,5,6,7,8-pentamethoxy-1-O-primeverosyl-xanthone (Figure 4), which is in parallel with its cell growth (Beerhues and Berger, 1994). A hypothetical scheme for this compound (3,5,6,7,8-pentamethoxy-1-O-primeverosylxanthone) was proposed by Beerhues and Berger (1995). This includes intermediates, such as 1,8-dihydroxy-3,5-dimethoxyxanthone, 1,8-dihydroxy-3,5,6-trimethoxyxanthone, 1,8-dihydroxy-3,5,6,7-tetramethoxyxanthone, and 1-hydroxy-3,5,6,7,8-pentamethoxyxanthone (Figure 4). However, the full list of enzymes involved in this process is still unknown.
In addition to that, 1,3,5-THX is proposed to form more complex xanthones, such as biyouxanthone D, gambogic acid, psorospermin, and swertianolin with diverse biological activities (Figure 4). For instance, biyouxanthone D, a polyprenylated xanthone isolated from in vitro root cultures of H. perforatum and field-grown roots of Hypercium monogynum has been shown to possess antifungal activity (Tocci et al., 2013) and neuroprotective effects (Xu et al., 2016). Another prenylated xanthone, gambogic acid, the main bioactive compound for Garcinia hanburyi, has been observed to induce apoptosis in many types of cancer cell lines including BGC-823 human gastric cancer line (Liu et al., 2005), human hematoma SMMC-7721 cells (Guo et al., 2004), and prostate tumor (Yi et al., 2008). Recently, gambogic acid has been shown to exert such cytotoxic mechanism against the cancer cell lines by inducing paraptosis, a cell death induced by vacuolization (Seo et al., 2019). Furthermore, psorospermin (Figure 4) isolated from Psorospermum febrifugum also demonstrated a significant antitumor and anti-leukemic activities in mice (Anywar et al., 2021). This woody plant that originated from Africa has been identified to be effective to be used as an anti-pyretic, a leprosy treatment, a poisoning treatment, and a purgative material. Meanwhile, swertianolin (1,5-dihydroxy-3-methoxyxanthone-8-O-ββ-D-glucopyranoside), a glycosylated xanthone, is the active compound isolated from felworts (Swertia paniculata) (Pant et al., 2011). The plant is known for its use as a bitter tonic in Indian traditional medicine as well as for the treatment of certain mental illnesses including melancholia (Pant et al., 2011). Thus, these xanthone compounds could be developed into potential drugs in treating various ailments in the future, but more investigation toward elucidating the identity and activity of respective biosynthetic enzymes may be conducted to allow sustainable in vitro or in vivo production of these compounds.
Biosynthetic Pathways of Xanthone Derivatives in Plants Through 1,3,7-Trihydroxyxanthone
Many more xanthone derivatives are derived from the other core structure, 1,3,7-THX (Figure 5). For instance, 1,3,7-THX is proposed to be a precursor compound for prenylated xanthones, such as rubraxanthone and scortechinone B, as well as simple xanthones, such as 1,7-dihydroxy-3-methoxyxanthone (gentisin) and 1,3-dihydroxy-7-methoxyxanthone (isogentisin; Figure 5). Rubraxanthone is mainly isolated from Garcinia (Jantan et al., 2002; Susanti et al., 2014) and Calophyllum species (Daud et al., 2021; Zailan et al., 2021) and showed significant ability to inhibit platelet aggregation in human whole blood samples (Alkadi et al., 2013). Meanwhile, scortechinone B extracted from the Garcinia scortechinii’s stem, bark, and latex exhibited surprisingly strong antimicrobial activity toward a methicillin-resistant Staphylococcus aureus strain with minimum inhibitory concentration (MIC = 2 μg/mL) compared to vancomycin antibiotic (MIC = 3.13–6.25 μg/mL; Rukachaisirikul et al., 2005; Araújo et al., 2019). Furthermore, an experiment toward Gentiana lutea rhizome has identified the presence of xanthone compounds called gentisin and isogentisin mainly derived from the 1,3,7-THX (Figure 5; Atkinson et al., 1968; Mudrić et al., 2020). Gentisin exhibited potent inhibition against β-glucuronidase enzyme that plays a critical role in drug metabolism and irinotecan-induced diarrhea (Sun et al., 2020), whereas isogentisin has been shown to protect endothelial injury caused by smoking (Schmieder et al., 2007).
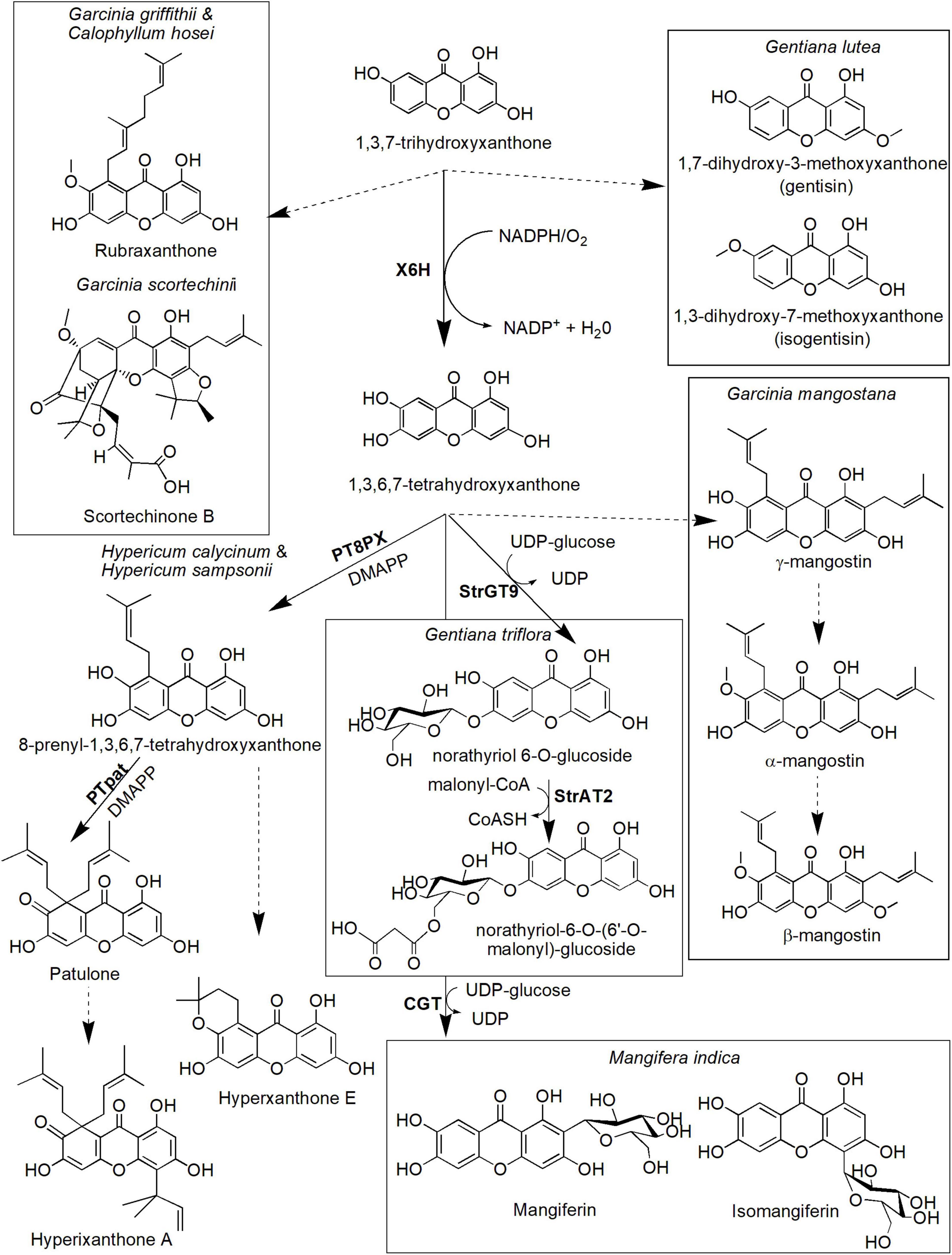
Figure 5. Proposed biosynthetic pathways of several xanthone derivatives derived from 1,3,7-trihydroxyxanthone precursor. Dotted arrows indicate hypothetical/proposed pathways while protein activities detected at the molecular level are in bold (refer to Table 1). CoASH, coenzyme A; DMAPP, dimethylallyl pyrophosphate; PT8PX, 8-prenylxanthone-forming prenyltransferase; PTpat, patulone-forming prenyltransferase; StrAT2, malonyl-CoA acyltransferase; StrGT9, norathyriol 6-O-glucosyltransferase; UDP, uridine diphosphate; X6H, xanthone 6-hydroxylase.
The hydroxylation of 1,3,7-THX also forms 1,3,6,7-tetrahydroxyxanthones in various species including H. androsaemum and G. mangostana (Figure 5; Schmidt et al., 2000a; El-Awaad et al., 2016). This process is catalyzed by the same X6H enzyme that catalyzes hydroxylation of 1,3,5-THX earlier (Barillas and Beerhues, 2000; Schmidt et al., 2000b) and potentially resided in the endoplasmic reticulum (Schmidt et al., 2000a; El-Awaad et al., 2016). In G. mangostana, prenylation of the 1,3,6,7-tetrahydroxyxanthones at the C-2 and C-8 positions is proposed to generate γ-mangostin, and subsequent O-methylation at the hydroxyl group of C-7 produces α-mangostin (Nualkaew et al., 2012; Mazimba et al., 2013), the main xanthone compounds in this species (Mazlan et al., 2019; Mamat et al., 2020). We postulate that another main xanthone in this species called β-mangostin may directly follow the same route of which another O-methylation could occur at the C-3 hydroxyl group position. Whether similar route is present in other β-mangostin-producing species such as Calophyllum spp. (Gómez-Verjan et al., 2017; Zailan et al., 2021) still needs further investigation. These xanthones (α-, β- and γ-mangostin) are known to possess antitumor, antioxidant, antidiabetic, antimicrobial, and anti-inflammatory properties, among others (Aizat et al., 2019; Marzaimi and Aizat, 2019). Unfortunately, specific enzymes that catalyzed these prenylation and methylation reactions in mangosteen have yet to be identified, although ongoing transcriptomics and proteomics work in this species (Abdul-Rahman et al., 2017; Jamil et al., 2021) could shed some lights onto answering this question soon.
The other pathway leading from 1,3,6,7-tetrahydroxyxanthones is the biosynthesis of patulone, hyperxanthone E, and hyperixanthone A that are isolated from Hypericum spp. (Fiesel et al., 2015; Nagia et al., 2019; Figure 5). Recently, aromatic prenyltransferase (aPT) enzymes from H. calycinum and H. sampsonii were transformed into Nicotiana tabacum and Saccharomyces cerevisiae (Nagia et al., 2019). One of the enzymes, 8-prenylxanthone-forming prenyltransferase (PT8PX), was shown to exhibit prenylation activity and mainly localized at the envelope of the chloroplast (Nagia et al., 2019). The reaction product, 8-prenyl-1,3,6,7-tetrahydroxyxanthone, is proposed to be cyclized to become hyperxanthone E or further prenylated by patulone-forming prenyltransferase (PTpat) to patulone (Fiesel et al., 2015; Nagia et al., 2019). Both prenylation reactions by PTpat and earlier PT8PX require dimethylallyl pyrophosphate (DMAPP) as a prenyl donor (Nagia et al., 2019). Interestingly, PTpat has been shown to be able to catalyze patulone directly from 1,3,6,7-tetrahydroxyxanthone via gem-diprenylation, but this reaction is not preferred (Nagia et al., 2019). The patulone compound may then be converted to hyperixanthone A by means of reverse prenylation (Nagia et al., 2019), but further characterization of the responsible enzyme is needed. These xanthone derivatives have also shown pharmaceutical potential, for instance, hyperxanthone E has cytotoxic activity against breast cancer and human lung tumor cell lines (Tanaka et al., 2004) as well as a potent anti-inflammatory agent (Zhang et al., 2014). This compound also can be induced by phytopathogens (Janković et al., 2002; Gaid et al., 2012) and accumulated in plant callus and cell suspension upon hormonal induction (Dias et al., 2000). Meanwhile, patulone was able to inhibit platelet-activating factor responsible for asthma and inflammation (Oku et al., 2005; Singh et al., 2013), whereas hyperixanthone A is a potent anti-bacterial agent against S. aureus (Xiao et al., 2008; Xin et al., 2011).
Additionally, another prenyltransferase from Morus alba called isoliquiritigenin-3′-dimethylallyltransferase (IDT) also has shown regiospecific prenylation of the 1,3,6,7-tetrahydroxyxanthones to generate 2-dimethylallyl-1,3,7-trihydroxyxanthone that can act as a strong neuroprotective agent (Wang et al., 2016). However, M. alba is not known physiologically to produce xanthone which suggests substrate promiscuity property of the IDT enzyme (Wang et al., 2016). Other plant PTs have also been characterized from various species including Cudrania tricuspidata (Wang et al., 2014), Artemisia capillaris (Munakata et al., 2019), Citrus x paradisi (Munakata et al., 2021), Humulus lupulus (Li et al., 2015), and Cannabis sativa (Gülck et al., 2020) but their roles have yet to be elucidated for xanthone prenylation.
Meanwhile, two enzymes responsible for the biosynthesis of two xanthone glucosides, norathyriol 6-O-glucoside(also known as tripteroside or Xt1) and norathyriol-6-O-(6′-O-malonyl)-glucoside (called Xt2) were recently characterized at the molecular level by Sasaki et al. (2021). The first enzyme, norathyriol 6-O-glucosyltransferase (StrGT9) mediates the glucosylation of 1,3,6,7-tetrahydroxyxanthone to Xt1 compound. This reaction requires UDP-glucose as a donor molecule for the glucose moiety. The Xt1 compound will then be malonylated in the presence of malonyl-CoA to Xt2 by the second enzyme called malonyl-CoA acyltransferase (StrAT2). Interestingly, the products of these enzymatic reactions (Xt1 and Xt2) contribute to the red coloration in cultivated Japanese gentians (Gentiana triflora), together with anthocyanin co-pigmentation (Sasaki et al., 2021). Mangiferin, a well-known C-glucoside xanthone, can also be synthesized from 1,3,6,7-tetrahydroxyxanthones (Figure 5). It is originally isolated from mango M. indica L. (Anacardiaceae), and its structure was established as 2-C-β-D-glucopyranosyl-1,3,6,7-tetrahydroxyxanthone after extensive chemical reaction and spectroscopic investigation (Bhatia et al., 1967; Ehianeta et al., 2016). Isomangiferin, its structural isomer isolated from A. asphodeloides, was characterized as 4-C-β-D-glucopyranosyl-1,3,6,7-tetrahydroxyxanthone. Mangiferin was among the first xanthone that was discovered to display a wide range of medicinal properties including activation of the central nervous system (Bhattacharya et al., 1972) and antioxidant, antibiotic, anti-inflammatory, antiproliferative, antidiabetic, chemopreventive, analgesic, and immunomodulatory activities (Khare and Shanker, 2016; Saha et al., 2016). The formation of mangiferin in A. asphodeloides was investigated by means of feeding experiments, and the biosynthetic route for mangiferin and related xanthone C-glycosides has been studied and proposed by Fujita and Inoue (1980). In their route, maclurin 3-C-glucoside is postulated to be an intermediate and has been enzymatically converted to mangiferin and isomangiferin. More recently, an enzyme called C-glycosyltransferase (CGT) that catalyzes this reaction has been isolated and characterized from M. indica (Chen et al., 2015). The enzyme has been shown to exhibit substrate promiscuity to specific benzophenones and xanthones, suggesting its prominent role in catalyzing several biosynthetic reactions within the species (Chen et al., 2015).
Conclusion
This review details the biosynthetic process of xanthone in plants, which has yet to be updated comprehensively in the last decade. The biosynthesis of these xanthones can be either originated from shikimate precursor (L-phenylalanine-independent pathway) as shown in Gentianaceae family or through L-phenylalanine-dependent pathway as evidenced in Hypericaceae family. The pathway also involved benzophenone intermediates, followed by a regioselective oxidative mediated intramolecular coupling to form the xanthone ring structures, 1,3,5-THX and 1,3,7-THX. Several xanthone derivatives can be originated from these xanthone precursors and may differ between plants. In the future, this resource will allow genetic engineering of xanthone biosynthesis in microbial cell factory, hence providing sustainable option for producing this valuable bioactive compound.
Author Contributions
JR analyzed, interpreted, and reviewed the research articles as well as drafted the article. IS analyzed and critically reviewed the manuscript drafts. WA designed the research framework and critically revised the manuscript. All authors contributed to the manuscript editing and revision and approved the final version of the manuscript.
Funding
WA was awarded UKM Research University grants (GUP-2018-122 and GP-2019-K019471) and the NIG-JOINT (1A2021) with Masanori Arita (NIG, Japan).
Conflict of Interest
The authors declare that the research was conducted in the absence of any commercial or financial relationships that could be construed as a potential conflict of interest.
Publisher’s Note
All claims expressed in this article are solely those of the authors and do not necessarily represent those of their affiliated organizations, or those of the publisher, the editors and the reviewers. Any product that may be evaluated in this article, or claim that may be made by its manufacturer, is not guaranteed or endorsed by the publisher.
Acknowledgments
We thank Nurkhalida Kamal for reviewing parts of the writing and biochemical pathways. We also thank Masanori Arita (National Institute of Genetics, Japan) for the critical discussion of the xanthone pathway.
Supplementary Material
The Supplementary Material for this article can be found online at: https://www.frontiersin.org/articles/10.3389/fpls.2022.809497/full#supplementary-material
Footnotes
References
Abd El-Mawla, A. M., and Beerhues, L. (2002). Benzoic acid biosynthesis in cell cultures of Hypericum androsaemum. Planta 214, 727–733. doi: 10.1007/s004250100657
Abd El-Mawla, A. M., Schmidt, W., and Beerhues, L. (2001). Cinnamic acid is a precursor of benzoic acids in cell cultures of Hypericum androsaemum L. but not in cell cultures of Centaurium erythraea RAFN. Planta 212, 288–293. doi: 10.1007/s004250000394
Abdul-Rahman, A., Goh, H.-H., Loke, K.-K., Noor, N. M., and Aizat, W. M. (2017). RNA-seq analysis of mangosteen (Garcinia mangostana L.) fruit ripening. Genomics Data 12, 159–160. doi: 10.1016/j.gdata.2017.05.013
Aizat, W. M., Jamil, I. N., Ahmad-Hashim, F. H., and Noor, N. M. (2019). Recent updates on metabolite composition and medicinal benefits of mangosteen plant. PeerJ 7:e6324. doi: 10.7717/peerj.6324
Alkadi, K. A., Adam, A., Taha, M., Hasan, M. H., and Shah, S. A. A. (2013). Prenylated xanthone and rubraxanthone with antiplatelet aggregation activity in human whole blood isolated from Garcinia griffithii. Orient. J. Chem. 29, 1291–1295. doi: 10.13005/ojc/290404
Anywar, G., Kakudidi, E., Byamukama, R., Mukonzo, J., Schubert, A., Oryem-Origa, H., et al. (2021). A review of the toxicity and phytochemistry of medicinal plant species used by herbalists in treating people living with HIV/AIDS in Uganda. Front. Pharmacol. 12:615147. doi: 10.3389/fphar.2021.615147
Araújo, J., Fernandes, C., Pinto, M., and Tiritan, M. E. (2019). Chiral derivatives of xanthones with antimicrobial activity. Molecules 24:314. doi: 10.3390/molecules24020314
Aritomi, M., and Kawasaki, T. (1970). A new xanthone C-glucoside, position isomer of mangiferin, from Anemarrhena asphodeloides Bunge. Chem. Pharm. Bull. 18, 2327–2333. doi: 10.1016/s0040-4039(01)97704-4
Atkinson, J., Gupta, P., and Lewis, J. (1968). Benzophenone participation in xanthone biosynthesis (Gentianaceae). Chem. Commun. 1968, 1386–1387. doi: 10.1039/c19680001386
Barillas, W., and Beerhues, L. (1997). 3-Hydroxybenzoate: coenzyme A ligase and 4-coumarate: coenzyme A ligase from cultured cells of Centaurium erythraea. Planta 202, 112–116. doi: 10.1007/s004250050109
Barillas, W., and Beerhues, L. (2000). 3-Hydroxybenzoate: coenzyme A ligase from cell cultures of Centaurium erythraea: isolation and characterization. Biol. Chem. 381, 155–160. doi: 10.1515/BC.2000.021
Beerhues, L. (1996). Benzophenone synthase from cultured cells of Centaurium erythraea. FEBS Lett. 383, 264–266. doi: 10.1016/0014-5793(96)00265-7
Beerhues, L., and Berger, U. (1994). Xanthones in cell suspension cultures of two Centaurium species. Phytochemistry 35, 1227–1231. doi: 10.1016/s0031-9422(00)94825-8
Beerhues, L., and Berger, U. (1995). Differential accumulation of xanthones in methyl-jasmonate-and yeast-extract-treated cell cultures of Centaurium erythraea and Centaurium littorale. Planta 197, 608–612.
Beerhues, L., and Liu, B. (2009). Biosynthesis of biphenyls and benzophenones–evolution of benzoic acid-specific type III polyketide synthases in plants. Phytochemistry 70, 1719–1727. doi: 10.1016/j.phytochem.2009.06.017
Beerhues, L., Liu, B., Raeth, T., Klundt, T., Beuerle, T., and Bocola, M. (2007). “Benzoic acid-specific type III polyketide synthases,” in Polyketides Biosynthesis, Biological Activity, and Genetic Engineering, eds Rimando, A. M. and Baerson, S. R. (Washington, DC: ACS Publications), 97–108. doi: 10.1021/bk-2007-0955.ch007
Behera, A. K., Swamy, M. M., Natesh, N., and Kundu, T. K. (2016). “Garcinol and its role in chronic diseases,” in Anti-inflammatory Nutraceuticals and Chronic Diseases: Advances in Experimental Medicine and Biology Vol. 928, eds S. Gupta, S. Prasad and B. Aggarwal (Cham: Springer), 435–452. doi: 10.1007/978-3-319-41334-1_18
Belkheir, A. K., Gaid, M., Liu, B., Hänsch, R., and Beerhues, L. (2016). Benzophenone synthase and chalcone synthase accumulate in the mesophyll of Hypericum perforatum leaves at different developmental stages. Front. Plant Sci. 7:921. doi: 10.3389/fpls.2016.00921
Bennett, G. J., and Lee, H.-H. (1988). The biosynthesis of mangostin: the origin of the xanthone skeleton. J. Chem. Soc. Chem. Commun. 619–620.
Bennett, G. J., and Lee, H.-H. (1989). Xanthones from Guttiferae. Phytochemistry 28, 967–998. doi: 10.1016/0031-9422(89)80170-0
Beuerle, T., and Pichersky, E. (2002). Purification and characterization of benzoate: coenzyme A ligase from Clarkia breweri. Arch. Biochem. Biophys. 400, 258–264. doi: 10.1016/S0003-9861(02)00026-7
Bhatia, V., Ramanathan, J., and Seshadri, T. (1967). Constitution of mangiferin. Tetrahedron 23, 1363–1368. doi: 10.1016/0040-4020(67)85090-7
Bhattacharya, S., Ghosal, S., Chaudhuri, R., and Sanyal, A. (1972). Canscora decussata (Gentianaceae) xanthones III: pharmacological studies. J. Pharm. Sci. 61, 1838–1840. doi: 10.1002/jps.2600611136
Bräse, S., Encinas, A., Keck, J., and Nising, C. (2009). Chemistry and biology of mycotoxins and related fungal metabolites. Chem. Rev. 109, 3903–3990. doi: 10.1021/cr050001f
Carpenter, I., Locksley, H., and Scheinmann, F. (1969). Xanthones in higher plants: biogenetic proposals and a chemotaxonomic survey. Phytochemistry 8, 2013–2025. doi: 10.1016/s0031-9422(00)88089-9
Castro, A. H. F., Coimbra, M. C., da Fonseca, S. T. D., and de Melo Souza, A. A. (2020). “Phenylalanine ammonia-lyase in higher plants: a key enzyme for plant development,” in Advances in Chemistry Research, ed. J. C. Taylor New York, NY: Nova Science Publishers.
Chen, D., Chen, R., Wang, R., Li, J., Xie, K., Bian, C., et al. (2015). Probing the catalytic promiscuity of a regio-and stereospecific C-glycosyltransferase from Mangifera indica. Angew. Chem. 127, 12869–12873. doi: 10.1002/anie.201506505
Chen, R., Gao, B., Liu, X., Ruan, F., Zhang, Y., Lou, J., et al. (2017). Molecular insights into the enzyme promiscuity of an aromatic prenyltransferase. Nat. Chem. Biol. 13, 226–234. doi: 10.1038/nchembio.2263
Daud, S., Karunakaran, T., Santhanam, R., Nagaratnam, S. R., Jong, V. Y. M., and Ee, G. C. L. (2021). Cytotoxicity and nitric oxide inhibitory activities of xanthones isolated from Calophyllum hosei Ridl. Nat. Prod. Res. 35, 6067–6072. doi: 10.1080/14786419.2020.1819273
Dias, A. C. P., Seabra, R. M., Andrade, P. B., Ferreres, F., and Fernandes-Ferreira, M. (2000). Xanthone biosynthesis and accumulation in calli and suspended cells of Hypericum androsaemum. Plant Sci. 150, 93–101. doi: 10.1016/s0168-9452(99)00178-8
Ehianeta, T. S., Laval, S., and Yu, B. (2016). Bio-and chemical syntheses of mangiferin and congeners. BioFactors 42, 445–458. doi: 10.1002/biof.1279
El-Awaad, I., Bocola, M., Beuerle, T., Liu, B., and Beerhues, L. (2016). Bifunctional CYP81AA proteins catalyse identical hydroxylations but alternative regioselective phenol couplings in plant xanthone biosynthesis. Nat. Commun. 7:11472. doi: 10.1038/ncomms11472
El-Seedi, H. R., El-Barbary, M., El-Ghorab, D., Bohlin, L., Borg-Karlson, A.-K., Goransson, U., et al. (2010). Recent insights into the biosynthesis and biological activities of natural xanthones. Curr. Med. Chem. 17, 854–901. doi: 10.2174/092986710790712147
El-Seedi, H. R., El-Ghorab, D. M., El-Barbary, M. A., Zayed, M. F., Goransson, U., Larsson, S., et al. (2009). Naturally occurring xanthones; latest investigations: isolation, structure elucidation and chemosystematic significance. Curr. Med. Chem. 16, 2581–2626. doi: 10.2174/092986709788682056
Fiesel, T., Gaid, M., Müller, A., Bartels, J., El-Awaad, I., Beuerle, T., et al. (2015). Molecular cloning and characterization of a xanthone prenyltransferase from Hypericum calycinum cell cultures. Molecules 20, 15616–15630. doi: 10.3390/molecules200915616
Fu, X., Zhou, Y., Zeng, L., Dong, F., Mei, X., Liao, Y., et al. (2017). Analytical method for metabolites involved in biosynthesis of plant volatile compounds. RSC Adv. 7, 19363–19372. doi: 10.1039/c7ra00766c
Fujita, M., and Inoue, T. (1980). Biosynthesis of mangiferin in Anemarrhena asphodeloides Bunge. I. The origin of the xanthone nucleus. Chem. Pharm. Bull. 28, 2476–2481. doi: 10.1248/cpb.28.2476
Gaid, M., Singh, P., El-Awaad, I., Nagia, M., and Beerhues, L. (2019). “Biotechnological production of prenylated xanthones for pharmaceutical use,” in Pharmaceutical Biocatalysis, ed. P. Grunwald (Singapore: Jenny Stanford Publishing), 103–142. doi: 10.1201/9780429353116-4
Gaid, M. M., Sircar, D., Beuerle, T., Mitra, A., and Beerhues, L. (2009). Benzaldehyde dehydrogenase from chitosan-treated Sorbus aucuparia cell cultures. J. Plant Physiol. 166, 1343–1349. doi: 10.1016/j.jplph.2009.03.003
Gaid, M. M., Sircar, D., Müller, A., Beuerle, T., Liu, B., Ernst, L., et al. (2012). Cinnamate: CoA ligase initiates the biosynthesis of a benzoate-derived xanthone phytoalexin in Hypericum calycinum cell cultures. Plant Physiol. 160, 1267–1280. doi: 10.1104/pp.112.204180
Gómez-Verjan, J., Rodríguez-Hernández, K., and Reyes-Chilpa, R. (2017). “Bioactive coumarins and xanthones from Calophyllum genus and analysis of their Druglikeness and toxicological properties,” in Studies in Natural Products Chemistry, ed. Atta-ur-Rahman (Amsterdam: Elsevier), 277–307. doi: 10.1016/b978-0-444-63930-1.00008-9
Guo, Q.-L., You, Q.-D., Wu, Z.-Q., Yuan, S.-T., and Zhao, L. (2004). General gambogic acids inhibited growth of human hepatoma SMMC-7721 cells in vitro and in nude mice. Acta Pharmacol. Sin. 25, 769–774.
Gupta, P., and Lewis, J. R. (1971). Biogenesis of xanthones in Gentiana lutea. J. Chem. Soc. C 1971, 629–631. doi: 10.1039/j39710000629
Gülck, T., Booth, J., Carvalho, Â., Khakimov, B., Crocoll, C., Motawia, M., et al. (2020). Synthetic biology of cannabinoids and cannabinoid glucosides in Nicotiana benthamiana and Saccharomyces cerevisiae. J. Nat. Prod. 83, 2877–2893. doi: 10.1021/acs.jnatprod.0c00241
Haynes, L., and Taylor, D. (1966). C-glycosyl compounds. Part V. Mangiferin; the nuclear magnetic resonance spectra of xanthones. J. Chem. Soc. C 1966, 1685–1687. doi: 10.1039/j39660001685
Huang, L., Wang, H., Ye, H., Du, Z., Zhang, Y., Beerhues, L., et al. (2012). Differential expression of benzophenone synthase and chalcone synthase in Hypericum sampsonii. Nat. Prod. Commun. 7, 1615–1618.
Hyun, M. W., Yun, Y. H., Kim, J. Y., and Kim, S. H. (2011). Fungal and plant phenylalanine ammonia-lyase. Mycobiology 39, 257–265. doi: 10.5941/myco.2011.39.4.257
Jamil, I. N., Remali, J., Azizan, K. A., Nor Muhammad, N. A., Arita, M., Goh, H.-H., et al. (2020). Systematic multi-omics integration (MOI) approach in plant systems biology. Front. Plant Sci. 11:944. doi: 10.3389/fpls.2020.00944
Jamil, I. N., Sanusi, S., Mackeen, M. M., Noor, N. M., and Aizat, W. M. (2021). SWATH-MS proteomics and postharvest analyses of mangosteen ripening revealed intricate regulation of carbohydrate metabolism and secondary metabolite biosynthesis. Postharvest Biol. Technol. 176:111493. doi: 10.1016/j.postharvbio.2021.111493
Janković, T., Krstić, D., Šavikin-Fodulović, K., Menković, N., and Grubišić, D. (2002). Xanthones and secoiridoids from hairy root cultures of Centaurium erythraea and C. pulchellum. Planta Med. 68, 944–946. doi: 10.1055/s-2002-34927
Jantan, I., Pisar, M. M., Idris, M. S., Taher, M., and Ali, R. M. (2002). In vitro inhibitory effect of rubraxanthone isolated from Garcinia parvifolia on platelet-activating factor receptor binding. Planta Med. 68, 1133–1134. doi: 10.1055/s-2002-36343
Jensen, S. R., and Schripsema, J. (2002). “Chemotaxonomy and pharmacology of Gentianaceae,” in Gentianaceae: Systematics and Natural History, eds L. Struwe and V. A. Albert (Cambridge: Cambridge University Press), 573–631. doi: 10.1017/cbo9780511541865.007
Khan, T., Ali, M., Khan, A., Nisar, P., Jan, S. A., Afridi, S., et al. (2020). Anticancer plants: a review of the active phytochemicals, applications in animal models, and regulatory aspects. Biomolecules 10:47. doi: 10.3390/biom10010047
Khare, P., and Shanker, K. (2016). Mangiferin: a review of sources and interventions for biological activities. BioFactors 42, 504–514. doi: 10.1002/biof.1308
Khattab, A. R., and Farag, M. A. (2020). Current status and perspectives of xanthones production using cultured plant biocatalyst models aided by in-silico tools for its optimization. Crit. Rev. Biotechnol. 40, 415–431. doi: 10.1080/07388551.2020.1721426
Kitanov, G. M., and Nedialkov, P. T. (2001). Benzophenone O-glucoside, a biogenic precursor of 1, 3, 7-trioxygenated xanthones in Hypericum annulatum. Phytochemistry 57, 1237–1243. doi: 10.1016/s0031-9422(01)00194-7
Klamrak, A., Nabnueangsap, J., and Nualkaew, N. (2021). Biotransformation of benzoate to 2, 4, 6-trihydroxybenzophenone by engineered Escherichia coli. Molecules 26:2779. doi: 10.3390/molecules26092779
Klejdus, B., Kováčik, J., and Babula, P. (2013). PAL inhibitor evokes different responses in two Hypericum species. Plant Physiol. Biochem. 63, 82–88. doi: 10.1016/j.plaphy.2012.11.019
Klempien, A., Kaminaga, Y., Qualley, A., Nagegowda, D. A., Widhalm, J. R., Orlova, I., et al. (2012). Contribution of CoA ligases to benzenoid biosynthesis in petunia flowers. Plant Cell 24, 2015–2030. doi: 10.1105/tpc.112.097519
Klingauf, P., Beuerle, T., Mellenthin, A., El-Moghazy, S. A., Boubakir, Z., and Beerhues, L. (2005). Biosynthesis of the hyperforin skeleton in Hypericum calycinum cell cultures. Phytochemistry 66, 139–145. doi: 10.1016/j.phytochem.2004.11.003
Koeberle, A., Northoff, H., and Werz, O. (2009). Identification of 5-lipoxygenase and microsomal prostaglandin E2 synthase-1 as functional targets of the anti-inflammatory and anti-carcinogenic garcinol. Biochem. Pharmacol. 77, 1513–1521. doi: 10.1016/j.bcp.2009.02.005
Kumar, V., Sood, H., and Chauhan, R. S. (2015). Detection of intermediates through high-resolution mass spectrometry for constructing biosynthetic pathways for major chemical constituents in a medicinally important herb, Swertia chirayita. Nat. Prod. Res. 29, 1449–1455. doi: 10.1080/14786419.2015.1004175
Lay, M. M., Karsani, S. A., and Abd Malek, S. N. (2014). Induction of apoptosis of 2, 4’, 6-trihydroxybenzophenone in HT-29 colon carcinoma cell line. BioMed Res. Int. 2014:468157. doi: 10.1155/2014/468157
Le Pogam, P., and Boustie, J. (2016). Xanthones of lichen source: a 2016 update. Molecules 21:294. doi: 10.3390/molecules21030294
Lee, S., Kaminaga, Y., Cooper, B., Pichersky, E., Dudareva, N., and Chapple, C. (2012). Benzoylation and sinapoylation of glucosinolate R-groups in Arabidopsis. Plant J. 72, 411–422. doi: 10.1111/j.1365-313X.2012.05096.x
Li, H., Ban, Z., Qin, H., Ma, L., King, A. J., and Wang, G. (2015). A heteromeric membrane-bound prenyltransferase complex from hop catalyzes three sequential aromatic prenylations in the bitter acid pathway. Plant Physiol. 167, 650–659. doi: 10.1104/pp.114.253682
Li, J., Zhao, Y.-L., Huang, H.-Y., and Wang, Y.-Z. (2017). Phytochemistry and pharmacological activities of the genus Swertia (Gentianaceae): a review. Am. J. Chin. Med. 45, 667–736. doi: 10.1142/s0192415x17500380
Li, W., Chen, H.-Q., Wang, H., Mei, W.-L., and Dai, H.-F. (2021). Natural products in agarwood and Aquilaria plants: chemistry, biological activities and biosynthesis. Nat. Prod. Rep. 38, 528–565. doi: 10.1039/d0np00042f
Liao, C.-H., Ho, C.-T., and Lin, J.-K. (2005). Effects of garcinol on free radical generation and NO production in embryonic rat cortical neurons and astrocytes. Biochem. Biophys. Res. Commun. 329, 1306–1314. doi: 10.1016/j.bbrc.2005.02.110
Liu, B., Falkenstein-Paul, H., Schmidt, W., and Beerhues, L. (2003). Benzophenone synthase and chalcone synthase from Hypericum androsaemum cell cultures: cDNA cloning, functional expression, and site-directed mutagenesis of two polyketide synthases. Plant J. 34, 847–855. doi: 10.1046/j.1365-313x.2003.01771.x
Liu, W., Guo, Q.-L., You, Q.-D., Zhao, L., Gu, H.-Y., and Yuan, S.-T. (2005). Anticancer effect and apoptosis induction of gambogic acid in human gastric cancer line BGC-823. World J. Gastroenterol. 11, 3655–3659. doi: 10.3748/wjg.v11.i24.3655
Long, M. C., Nagegowda, D. A., Kaminaga, Y., Ho, K. K., Kish, C. M., Schnepp, J., et al. (2009). Involvement of snapdragon benzaldehyde dehydrogenase in benzoic acid biosynthesis. Plant J. 59, 256–265. doi: 10.1111/j.1365-313X.2009.03864.x
Lynch, J. H., and Dudareva, N. (2020). Aromatic amino acids: a complex network ripe for future exploration. Trends Plant Sci. 25, 670–681. doi: 10.1016/j.tplants.2020.02.005
Maeda, H., and Dudareva, N. (2012). The shikimate pathway and aromatic amino acid biosynthesis in plants. Annu. Rev. Plant Biol. 63, 73–105. doi: 10.1146/annurev-arplant-042811-105439
Mamat, S. F., Azizan, K. A., Baharum, S. N., Noor, N. M., and Aizat, W. M. (2020). GC-MS and LC-MS analyses reveal the distribution of primary and secondary metabolites in mangosteen (Garcinia mangostana Linn.) fruit during ripening. Sci. Hortic. 262:109004. doi: 10.1016/j.scienta.2019.109004
Mariano, L. N. B., Boeing, T., Cechinel Filho, V., Niero, R., Mota da Silva, L., and de Souza, P. (2021). 1, 3, 5, 6-tetrahydroxyxanthone promotes diuresis, renal protection and antiurolithic properties in normotensive and hypertensive rats. J. Pharm. Pharmacol. 73, 700–708. doi: 10.1093/jpp/rgab026
Mariano, L. N. B., Boeing, T., Cechinel-Filho, V., Niero, R., da Silva, L. M., de Souza, P., et al. (2019). 1, 3, 5, 6-tetrahydroxyxanthone, a natural xanthone, induces diuresis and saluresis in normotensive and hypertensive rats. Chem. Biol. Interact. 311:108778. doi: 10.1016/j.cbi.2019.108778
Marzaimi, I. N., and Aizat, W. M. (2019). “Current review on mangosteen usages in antiinflammation and other related disorders,” in Bioactive Food as Dietary Interventions for Arthritis and Related Inflammatory Diseases, eds R. R. Watson and V. R. Preedy (London: Academic Press), 273–289. doi: 10.1016/b978-0-12-813820-5.00017-9
Masters, K.-S., and Bräse, S. (2012). Xanthones from fungi, lichens, and bacteria: the natural products and their synthesis. Chem. Rev. 112, 3717–3776. doi: 10.1021/cr100446h
Mazimba, O., Nana, F., Kuete, V., and Singh, G. S. (2013). “Xanthones and anthranoids from the medicinal plants of Africa,” in Medicinal Plant Research in Africa, ed. V. Kuete (London: Elsevier), 393–434. doi: 10.1016/b978-0-12-405927-6.00011-4
Mazlan, O., Aizat, W. M., Zuddin, N. S. A., Baharum, S. N., and Noor, N. M. (2019). Metabolite profiling of mangosteen seed germination highlights metabolic changes related to carbon utilization and seed protection. Sci. Hortic. 243, 226–234. doi: 10.1016/j.dib.2018.11.072
Mudrić, J., Janković, T., Šavikin, K., Bigović, D., Đukić-Ćosić, D., Ibrić, S., et al. (2020). Optimization and modelling of gentiopicroside, isogentisin and total phenolics extraction from Gentiana lutea L. roots. Ind. Crops Prod. 155:112767. doi: 10.1016/j.indcrop.2020.112767
Munakata, R., Olry, A., Takemura, T., Tatsumi, K., Ichino, T., Villard, C., et al. (2021). Parallel evolution of UbiA superfamily proteins into aromatic O-prenyltransferases in plants. Proc. Natl. Acad. Sci. U.S.A. 118:e2022294118. doi: 10.1073/pnas.2022294118
Munakata, R., Takemura, T., Tatsumi, K., Moriyoshi, E., Yanagihara, K., Sugiyama, A., et al. (2019). Isolation of Artemisia capillaris membrane-bound di-prenyltransferase for phenylpropanoids and redesign of artepillin C in yeast. Commun. Biol. 2:384. doi: 10.1038/s42003-019-0630-0
Nagia, M., Gaid, M., Biedermann, E., Fiesel, T., El-Awaad, I., Hänsch, R., et al. (2019). Sequential regiospecific gem-diprenylation of tetrahydroxyxanthone by prenyltransferases from Hypericum sp. New Phytol. 222, 318–334. doi: 10.1111/nph.15611
Nualkaew, N., Morita, H., Shimokawa, Y., Kinjo, K., Kushiro, T., De-Eknamkul, W., et al. (2012). Benzophenone synthase from Garcinia mangostana L. pericarps. Phytochemistry 77, 60–69. doi: 10.1016/j.phytochem.2012.02.002
Oku, H., Ueda, Y., Iinuma, M., and Ishiguro, K. (2005). Inhibitory effects of xanthones from Guttiferae plants on PAF-induced hypotension in mice. Planta Med. 71, 90–92. doi: 10.1055/s-2005-837760
Oldenburg, T. B., Wilkes, H., Horsfield, B., Van Duin, A. C., Stoddart, D., and Wilhelms, A. (2002). Xanthones—novel aromatic oxygen-containing compounds in crude oils. Organ. Geochem. 33, 595–609. doi: 10.1016/s0146-6380(02)00015-3
Ovalle-Magallanes, B., Eugenio-Perez, D., and Pedraza-Chaverri, J. (2017). Medicinal properties of mangosteen (Garcinia mangostana L.): a comprehensive update. Food Chem. Toxicol. 109, 102–122. doi: 10.1016/j.fct.2017.08.021
Pant, N., Misra, H., and Jain, D. C. (2011). A xanthone glycoside from aerial parts of Swertia paniculata. J. Saudi Chem. Soc. 18, 551–554. doi: 10.1016/j.jscs.2011.11.001
Pardo-Andreu, G. L., Nuñez-Figueredo, Y., Tudella, V. G., Cuesta-Rubio, O., Rodrigues, F. P., Pestana, C. R., et al. (2011). The anti-cancer agent guttiferone-A permeabilizes mitochondrial membrane: ensuing energetic and oxidative stress implications. Toxicol. Appl. Pharmacol. 253, 282–289. doi: 10.1016/j.taap.2011.04.011
Peres, V., Nagem, T. J., and de Oliveira, F. F. (2000). Tetraoxygenated naturally occurring xanthones. Phytochemistry 55, 683–710. doi: 10.1016/s0031-9422(00)00303-4
Peters, S., Schmidt, W., and Beerhues, L. (1997). Regioselective oxidative phenol couplings of 2, 3’, 4, 6-tetrahydroxybenzophenone in cell cultures of Centaurium erythraea RAFN and Hypericum androsaemum L. Planta 204, 64–69. doi: 10.1007/s004250050230
Pinto, M., Sousa, M., and Nascimento, M. (2005). Xanthone derivatives: new insights in biological activities. Curr. Med. Chem. 12, 2517–2538. doi: 10.2174/092986705774370691
Pinto, M. M., Palmeira, A., Fernandes, C., Resende, D. I., Sousa, E., Cidade, H., et al. (2021). From natural products to new synthetic small molecules: a journey through the world of xanthones. Molecules 26:431. doi: 10.3390/molecules26020431
Rai, A., Saito, K., and Yamazaki, M. (2017). Integrated omics analysis of specialized metabolism in medicinal plants. Plant J. 90, 764–787. doi: 10.1111/tpj.13485
Raksat, A., Maneerat, W., Andersen, R. J., Pyne, S. G., and Laphookhieo, S. (2019). A tocotrienol quinone dimer and xanthones from the leaf extract of Garcinia nigrolineata. Fitoterapia 136:104175. doi: 10.1016/j.fitote.2019.104175
Ramawat, K. G., and Mérillon, J.-M. (2013). Natural Products: Phytochemistry, Botany and Metabolism of Alkaloids, Phenolics and Terpenes. Berlin: Springer.
Rukachaisirikul, V., Phainuphong, P., Sukpondma, Y., Phongpaichit, S., and Taylor, W. C. (2005). Antibacterial caged-tetraprenylated xanthones from the stem bark of Garcinia scortechinii. Planta Med. 71, 165–170. doi: 10.1055/s-2005-837785
Saha, S., Sadhukhan, P., and Sil, P. C. (2016). Mangiferin: a xanthonoid with multipotent anti-inflammatory potential. Biofactors 42, 459–474. doi: 10.1002/biof.1292
Saini, S. S., Gaid, M., and Sircar, D. (2020). Benzoate-CoA ligase contributes to the biosynthesis of biphenyl phytoalexins in elicitor-treated pear cell cultures. Plant Cell Rep. 39, 207–215. doi: 10.1007/s00299-019-02484-0
Saini, S. S., Teotia, D., Gaid, M., Thakur, A., Beerhues, L., and Sircar, D. (2017). Benzaldehyde dehydrogenase-driven phytoalexin biosynthesis in elicitor-treated Pyrus pyrifolia cell cultures. J. Plant Physiol. 215, 154–162. doi: 10.1016/j.jplph.2017.06.004
Sasaki, N., Nemoto, K., Nishizaki, Y., Sugimoto, N., Tasaki, K., Watanabe, A., et al. (2021). Identification and characterization of xanthone biosynthetic genes contributing to the vivid red coloration of red-flowered gentian. Plant J. 107, 1711–1723. doi: 10.1111/tpj.15412
Schätzle, M. A., Husain, S. M., Ferlaino, S., and Müller, M. (2012). Tautomers of anthrahydroquinones: enzymatic reduction and implications for chrysophanol, monodictyphenone, and related xanthone biosyntheses. J. Am. Chem. Soc. 134, 14742–14745. doi: 10.1021/ja307151x
Schmidt, W., Abd El-Mawla, A. M., Wolfender, J.-L., Hostettmann, K., and Beerhues, L. (2000a). Xanthones in cell cultures of Hypericum androsaemum. Planta Med. 66, 380–381. doi: 10.1055/s-2000-8542
Schmidt, W., Peters, S., and Beerhues, L. (2000b). Xanthone 6-hydroxylase from cell cultures of Centaurium erythraea RAFN and Hypericum androsaemum L. Phytochemistry 53, 427–431. doi: 10.1016/s0031-9422(99)00566-x
Schmidt, W., and Beerhues, L. (1997). Alternative pathways of xanthone biosynthesis in cell cultures of Hypericum androsaemum L. FEBS Lett. 420, 143–146. doi: 10.1016/s0014-5793(97)01507-x
Schmieder, A., Schwaiger, S., Csordas, A., Backovic, A., Messner, B., Wick, G., et al. (2007). Isogentisin—a novel compound for the prevention of smoking-caused endothelial injury. Atherosclerosis 194, 317–325. doi: 10.1016/j.atherosclerosis.2006.10.019
Seo, M. J., Lee, D. M., Kim, I. Y., Lee, D., Choi, M.-K., Lee, J.-Y., et al. (2019). Gambogic acid triggers vacuolization-associated cell death in cancer cells via disruption of thiol proteostasis. Cell Death Dis. 10:187. doi: 10.1038/s41419-019-1360-4
Shan, W. G., Lin, T. S., Yu, H. N., Chen, Y., and Zhan, Z. J. (2012). Polyprenylated xanthones and benzophenones from the bark of Garcinia oblongifolia. Helv. Chim. Acta 95, 1442–1448. doi: 10.1002/hlca.201200019
Singh, P., Kaufholdt, D., Awadalah, M., Hänsch, R., Beerhues, L., and Gaid, M. (2021). Cytosolic aromatic aldehyde dehydrogenase provides benzoic acid for xanthone biosynthesis in Hypericum. Plant Physiol. Biochem. 160, 82–93. doi: 10.1016/j.plaphy.2021.01.011
Singh, P., Preu, L., Beuerle, T., Kaufholdt, D., Hänsch, R., Beerhues, L., et al. (2020). A promiscuous coenzyme A ligase provides benzoyl-coenzyme A for xanthone biosynthesis in Hypericum. Plant J. 104, 1472–1490. doi: 10.1111/tpj.15012
Singh, P., Singh, I. N., Mondal, S. C., Singh, L., and Garg, V. K. (2013). Platelet-activating factor (PAF)-antagonists of natural origin. Fitoterapia 84, 180–201. doi: 10.1016/j.fitote.2012.11.002
Songsiriritthigul, C., Nualkaew, N., Ketudat-Cairns, J., and Chen, C.-J. (2020). The crystal structure of benzophenone synthase from Garcinia mangostana L. pericarps reveals the basis for substrate specificity and catalysis. Acta Crystallogr. F Struct. Biol. Commun. 76, 597–603. doi: 10.1107/S2053230X20014818
Sousa, M., and Pinto, M. (2005). Synthesis of xanthones: an overview. Curr. Med. Chem. 12, 2447–2479. doi: 10.2174/092986705774370736
Stewart, C., Woods, K., Macias, G., Allan, A. C., Hellens, R. P., and Noel, J. P. (2017). Molecular architectures of benzoic acid-specific type III polyketide synthases. Acta Crystallogr. D Struct. Biol. 73, 1007–1019. doi: 10.1107/S2059798317016618
Sultanbawa, M. (1980). Xanthonoids of tropical plants. Tetrahedron 36, 1465–1506. doi: 10.1016/s0040-4020(01)83114-8
Sun, C.-P., Yan, J.-K., Yi, J., Zhang, X.-Y., Yu, Z.-L., Huo, X.-K., et al. (2020). The study of inhibitory effect of natural flavonoids toward β-glucuronidase and interaction of flavonoids with β-glucuronidase. Int. J. Biol. Macromol. 143, 349–358. doi: 10.1016/j.ijbiomac.2019.12.057
Susanti, M., Lena, D. I., and Dachriyanus, D. (2014). Development and validation of a HPLC method for determination and quantification of rubraxanthone in stem bark extract of mangosteen. Indones. J. Pharm. 25, 237–244. doi: 10.14499/indonesianjpharm25iss4pp237
Tanaka, N., Takaishi, Y., Shikishima, Y., Nakanishi, Y., Bastow, K., Lee, K.-H., et al. (2004). Prenylated benzophenones and xanthones from Hypericum scabrum. J. Nat. Prod. 67, 1870–1875. doi: 10.1021/np040024+
Teotia, D., Gaid, M., Saini, S. S., Verma, A., Yennamalli, R. M., Khare, S. P., et al. (2019). Cinnamate-CoA ligase is involved in biosynthesis of benzoate-derived biphenyl phytoalexin in Malus× domestica ‘Golden Delicious’ cell cultures. Plant J. 100, 1176–1192. doi: 10.1111/tpj.14506
Tocci, N., D’Auria, F. D., Simonetti, G., Panella, S., Palamara, A. T., Debrassi, A., et al. (2013). Bioassay-guided fractionation of extracts from Hypericum perforatum in vitro roots treated with carboxymethylchitosans and determination of antifungal activity against human fungal pathogens. Plant Physiol. Biochem. 70, 342–347. doi: 10.1016/j.plaphy.2013.05.046
Tocci, N., Gaid, M., Kaftan, F., Belkheir, A. K., Belhadj, I., Liu, B., et al. (2018). Exodermis and endodermis are the sites of xanthone biosynthesis in Hypericum perforatum roots. New Phytol. 217, 1099–1112. doi: 10.1111/nph.14929
Tovilovic-Kovacevic, G., Zogovic, N., and Krstic-Milosevic, D. (2020). “Secondary metabolites from endangered Gentiana, Gentianella, Centaurium, and Swertia species (Gentianaceae): promising natural biotherapeutics,” in Biodiversity and Biomedicine, eds M. Ozturk, M. Pešić, and D. Egamberdieva (London: Academic Press), 335–384. doi: 10.1016/b978-0-12-819541-3.00019-0
Tuong, T. L., Aree, T., Do, L. T., Nguyen, P. K., Wonganan, P., and Chavasiri, W. (2019). Dimeric tetrahydroxanthones from the lichen Usnea aciculifera. Fitoterapia 137:104194. doi: 10.1016/j.fitote.2019.104194
Velíšek, J., Davidek, J., and Cejpek, K. (2008). Biosynthesis of food constituents: natural pigments. Part 2–a review. Czech J. Food Sci. 26, 73–98. doi: 10.17221/2463-cjfs
Vieira, L., and Kijjoa, A. (2005). Naturally-occurring xanthones: recent developments. Curr. Med. Chem. 12, 2413–2446. doi: 10.2174/092986705774370682
Wang, C. Z., Maier, U. H., Keil, M., Zenk, M. H., Bacher, A., Rohdich, F., et al. (2003). Phenylalanine-independent biosynthesis of 1, 3, 5, 8-tetrahydroxyxanthone: a retrobiosynthetic NMR study with root cultures of Swertia chirata. Eur. J. Biochem. 270, 2950–2958. doi: 10.1046/j.1432-1033.2003.03669.x
Wang, G., Shi, G., Chen, X., Chen, F., Yao, R., and Wang, Z. (2013). Loading of free radicals on the functional graphene combined with liquid chromatography–tandem mass spectrometry screening method for the detection of radical-scavenging natural antioxidants. Anal. Chim. Acta 802, 103–112. doi: 10.1016/j.aca.2013.09.041
Wang, R., Chen, R., Li, J., Liu, X., Xie, K., Chen, D., et al. (2014). Molecular characterization and phylogenetic analysis of two novel regio-specific flavonoid prenyltransferases from Morus alba and Cudrania tricuspidata. J. Biol. Chem. 289, 35815–35825. doi: 10.1074/jbc.M114.608265
Wang, R., Chen, R., Li, J., Liu, X., Xie, K., Chen, D., et al. (2016). Regiospecific prenylation of hydroxyxanthones by a plant flavonoid prenyltransferase. J. Nat. Prod. 79, 2143–2147. doi: 10.1021/acs.jnatprod.6b00417
Wezeman, T., Bräse, S., and Masters, K.-S. (2015). Xanthone dimers: a compound family which is both common and privileged. Nat. Prod. Rep. 32, 6–28. doi: 10.1039/c4np00050a
Wu, J., Pan, X., Yao, L., Wang, L., and Tang, N. (2009). Synthesis and X-ray crystallography of diverse metal complexes derived from xanthone-crown ether. Supramol. Chem. 21, 707–716. doi: 10.1080/10610270902795384
Xiao, H.-B., Sun, Z.-L., and Zhou, N. (2012). 1, 3, 5, 8-Tetrahydroxyxanthone regulates ANGPTL3–LPL pathway to lessen the ketosis in mice. Eur. J. Pharm. Sci. 46, 26–31. doi: 10.1016/j.ejps.2012.02.001
Xiao, Z. Y., Shiu, W. K. P., Zeng, Y. H., Mu, Q., and Gibbons, S. (2008). A naturally occurring inhibitory agent from Hypericum sampsonii with activity against multidrug-resistant Staphylococcus aureus. Pharm. Biol. 46, 250–253. doi: 10.1080/13880200701739405
Xin, W. B., Mao, Z. J., Jin, G. L., and Qin, L. P. (2011). Two new xanthones from Hypericum sampsonii and biological activity of the isolated compounds. Phytother. Res. 25, 536–539. doi: 10.1002/ptr.3291
Xu, W.-J., Li, R.-J., Quasie, O., Yang, M.-H., Kong, L.-Y., and Luo, J. (2016). Polyprenylated tetraoxygenated xanthones from the roots of Hypericum monogynum and their neuroprotective activities. J. Nat. Prod. 79, 1971–1981. doi: 10.1021/acs.jnatprod.6b00251
Yi, T., Yi, Z., Cho, S.-G., Luo, J., Pandey, M. K., Aggarwal, B. B., et al. (2008). Gambogic acid inhibits angiogenesis and prostate tumor growth by suppressing vascular endothelial growth factor receptor 2 signaling. Cancer Res. 68, 1843–1850. doi: 10.1158/0008-5472.CAN-07-5944
Ying, Y.-M., Yu, K.-M., Lin, T.-S., Ma, L.-F., Fang, L., Yao, J.-B., et al. (2017). Antiproliferative prenylated xanthones from the pericarps of Garcinia mangostana. Chem. Nat. Compd. 53, 555–556. doi: 10.1007/s10600-017-2047-7
Yoiprommarat, S., Kongthong, S., Choowong, W., Boonyuen, N., Isaka, M., and Bunyapaiboonsri, T. (2020). Xanthones from a lignicolous freshwater fungus (BCC 28210). Nat. Prod. Res. 34, 1233–1237. doi: 10.1080/14786419.2018.1556655
Zafar, S., and Wang, W. (2018). Exploring the complex phytochemical and pharmacological value of Swertia punicea Hemsl. Curr. Tradit. Med. 4, 238–248.
Zailan, A. A. D., Karunakaran, T., Abu Bakar, M. H., and Mian, V. J. Y. (2021). The Malaysian genus Calophyllum (Calophyllaceae): a review on its phytochemistry and pharmacological activities. Nat. Prod. Res. 1–11. doi: 10.1080/14786419.2021.1982936
Keywords: synthesis, phytochemical, phenolic, flavonoid, biosynthesis, 9H-xanthen-9-one
Citation: Remali J, Sahidin I and Aizat WM (2022) Xanthone Biosynthetic Pathway in Plants: A Review. Front. Plant Sci. 13:809497. doi: 10.3389/fpls.2022.809497
Received: 05 November 2021; Accepted: 11 March 2022;
Published: 08 April 2022.
Edited by:
Pan Liao, Purdue University, United StatesReviewed by:
Hiroshi Noguchi, Nihon Pharmaceutical University, JapanMariam Gaid, Independent Researcher, Braunschweig, Germany
Copyright © 2022 Remali, Sahidin and Aizat. This is an open-access article distributed under the terms of the Creative Commons Attribution License (CC BY). The use, distribution or reproduction in other forums is permitted, provided the original author(s) and the copyright owner(s) are credited and that the original publication in this journal is cited, in accordance with accepted academic practice. No use, distribution or reproduction is permitted which does not comply with these terms.
*Correspondence: Wan Mohd Aizat, wma@ukm.edu.my