- Department of Laboratory Medicine, National Institutes of Health Clinical Center, Bethesda, MD, United States
The prevalence of obesity has increased dramatically worldwide and has become a critical public health priority. Obesity is associated with many co-morbid conditions, including hypertension, diabetes, and cardiovascular disease. Although the physiology of obesity is complex, a healthy diet and sufficient exercise are two elements known to be critical to combating this condition. Years of research on the Mediterranean diet, which is high in fresh fruits and vegetables, nuts, fish, and olive oil, have demonstrated a reduction in numerous non-communicable chronic diseases associated with this diet. There is strong evidence to support an anti-inflammatory effect of the diet, and inflammation is a key driver of obesity. Changes in diet alter the gut microbiota which are intricately intertwined with human physiology, as gut microbiota-derived metabolites play a key role in biological pathways throughout the body. This review will summarize recent published studies that examine the potential role of gut metabolites, including short-chain fatty acids, bile acids, trimethylamine-N-oxide, and lipopolysaccharide, in modulating inflammation after consumption of a Mediterranean-like diet. These metabolites modulate pathways of inflammation through the NOD-like receptor family pyrin domain-containing 3 (NLRP3) inflammasome, toll-like receptor 4 signaling, and macrophage driven effects in adipocytes, among other mechanisms.
1 Introduction
The World Health Organization (WHO) estimates that over 1 billion individuals worldwide now grapple with overweight/obesity1. Obesity is associated with numerous comorbid conditions, notably non-communicable diseases including hypertension, type 2 diabetes (T2D), and cardiovascular disease (CVD) which contributed to a staggering five million deaths globally in 2019 (1–3). These comorbid conditions present a significant health burden in individuals with overweight/obesity and make combating obesity a public health priority. Complex factors influence the prevalence of obesity, including genetics, physical activity levels, dietary pattern, caloric intake, medical conditions and their treatments, socioeconomic status, sleep habits, stress, and environmental chemicals (4). Inflammation, particularly chronic low-grade inflammation, has been implicated in numerous non-communicable diseases including obesity, metabolic syndrome, T2D, CVDs, and certain cancers (5). Research suggests that inflammation is a key driver of obesity (6) and that excess adipose tissue and dysfunctional adipocytes contribute to increased inflammation (7). Obese individuals have higher circulating inflammatory markers than lean individuals, and those markers are lowered following weight loss (8). Increasing inflammation in rodent models induces weight gain (6), and treatment with the anti-inflammatory cytokine interleukin (IL)-10 alleviated high-fat diet (HFD)-induced obesity (9). The aim of this review is to discuss recent studies that examine the influence of a Mediterranean diet (MedDiet) on inflammation and obesity. Specifically, we are interested in the observed effects of MedDiet adherence on gut-derived metabolites and their role in the physiology of obesity. There are multiple pieces of evidence required to connect specific dietary elements to conditions such as obesity and heart disease: (1) how digested food affects the gut microbiota composition, (2) which/how specific gut microbes in the host environment affect which gut metabolites that are present and in what quantities, (3) which/how gut metabolites influence cellular functions and biological pathways, and (4) which pathways are part of the physiology of healthy or diseased states. There have been a number of excellent reviews on some of the topics within this review, such as reviews on the MedDiet and inflammation (5) the MedDiet and the gut microbiome (10), or the role of some of the gut metabolites in obesity (11–14). We present a review that highlights the most recent literature and discusses all these topics: the MedDiet, obesity, inflammation, and gut metabolites, with a focus on the updates for four of the gut-derived metabolites that have been the focus of multiple recent investigations. Each individual scientific study may focus on only one of the four elements. In this review, we introduce the associations between obesity and inflammation, then we focus in more detail on the evidence for biological roles of specific metabolites. We focus on the most recent research results related to the pathways that include four gut metabolites (short-chain fatty acids, bile acids, trimethylamine N-oxide, and lipopolysaccharide) and discuss gaps in our understanding.
2 Method
For this narrative review, most articles included were chosen from searches in PubMed and Google Scholar. The online searches were conducted from September 2023–May 2024 using the keywords: Mediterranean, diet, food, Western, microbiome, bacteria, microbiota, obesity, obese, short-chain fatty acid (SCFA), lipopolysaccharide (LPS), bile acid (BA), trimethylamine N-oxide (TMAO), inflammation, inflammatory, immune, immunity, gut, metabolites, metabolomics, pathophysiology, pathway, chronic disease, and combinations thereof. Additional relevant publications were found in the citations of the articles found in our literature search. We included original research articles, reviews, meta-analyses, and clinical trials. Publications were restricted to the English language, selected on a discretionary basis by a consensus of the four authors, and we prioritized articles published within the last 4 years, though other older relevant articles were included. We focused on a subset of human studies of recently published original research reports that investigate the association between MedDiet adherence, inflammation, obesity, and gut metabolites, but also included studies in animal models that investigated biological pathways relevant to gut metabolites and inflammation.
3 Dietary contribution to inflammation and obesity
The escalating health burden of obesity has prompted research into its causes and possible preventive measures, particularly in modifiable lifestyle factors such as diet. In addition to energy intake, diet may also mediate other determinates of obesity such as inflammation, and there are multiple studies examining the role of nutrition in low-grade inflammation. Unraveling causality and defining pathways that connect nutrition and inflammation has proven very challenging due to the multifaceted nature of inflammatory pathways (15, 16). Pathways identified as important in inflammation, as related to diet and obesity, include the NLRP3 inflammasome, macrophage-mediated chronic low-grade inflammation in adipose tissue, and the toll-like receptor 4 (TLR4) signaling pathway that is activated by saturated fatty acids. C-reactive protein (CRP), adipocyte-derived metabolites, and inflammatory cytokines [such as tumor necrosis factor alpha (TNF-α), IL-1β, and IL-6] have been shown to play a role in inflammation associated with obesity, and in the development of insulin resistance (7, 15, 17–20). Indices like the Dietary Inflammatory Index (DII) have been developed to assess the inflammatory potential of a diet (21). A large study of more than 27,000 individuals over a period of about a decade found an association of overall obesity and abdominal obesity with a poor quality, pro-inflammatory diet. The authors used three indices, the Alternative Healthy Eating Index (AHEI), DII, and MedDiet Score, and found the AHEI to provide the best assessment of obesogenic potential of a diet, though the three indices have generally similar items in their assessment (22).
Several excellent reviews have been published that outline the connections between obesity, inflammation, and immunity. The review by May and den Hartigh focused on the impact of SCFAs on adipose tissue metabolism (23). A comprehensive review of the association of diet and gastrointestinal immunity itemized specific physiological effects associated with particular dietary macromolecules (24). A review of recent advances in our understanding of intestinal immunometabolism and microbiology provided a description of physiological differences between lean and obese states (25). Grosso et al. effectively summarized the proposed role of specific dietary elements, including macronutrients and phytochemicals, in the regulation of inflammation and immunity as relates to obesity (15). A review of ten meta-analyses summarized the evidence connecting the MedDiet with reduced dyslipidemia and decreased inflammatory mediators through modulation of the gut microbiota (10). Given the extensive data associating obesity and inflammation, combined with the data associating dietary changes with inflammation, dietary changes are justifiably proposed as one critical component of the treatment for obesity.
3.1 MedDiet
The MedDiet, originating from the traditional practices of people in the Mediterranean basin, has captured researchers' attention due to its reported health benefits, and is included as a healthy dietary pattern in the 2020–2025 Dietary Guidelines for Americans (26–28). The MedDiet promotes daily consumption of whole grains, nuts, vegetables, and fruit, with olive oil as the primary fat, moderate intake of fish, poultry, and wine, and rare intake of red meat and sweets (26, 29, 30). Anti-inflammatory effects have been attributed to multiple specific elements of the MedDiet, investigated alone in controlled studies, including olive oil, nuts, fatty fish, legumes, fruit, vegetables, and a reduction of red meat and refined foods (5). Although there is a general consensus regarding the characteristics of the MedDiet, criteria for calculating a “MedDiet score” vary considerably between studies (22, 29, 31). Regardless of the details of the MedDiet score, there is an abundance of data on the benefits of a MedDiet. A systematic review of 84 studies concluded that there is strong evidence to support an association of the MedDiet with fewer chronic diseases, including neurological diseases, CVD, cancer, T2D, liver disease, and renal disease. The MedDiet was also associated with reduced obesity-related metabolic features, inflammation, and lower mortality (31).
The Western diet, in contrast to the MedDiet, is a dietary pattern prevalent across many industrialized nations. Key components of the Western diet include high consumption of refined grains, red meat, and sugar sweetened beverages, which are associated with weight gain and obesity risk (10, 15, 22, 24, 32, 33). The Western diet can also include 50% or more of the calories from foods that are classified as ultra-processed, meaning they contain formulations of ingredients assembled in industrial processes as opposed to whole foods. Studies have associated ultra-processed foods (UPF) with low-grade inflammation and multiple chronic diseases (32, 34). Our review is not primarily focused on UPFs; however, individuals following a MedDiet, or other similar healthy dietary patterns, tend to consume fewer UPFs and would be spared the inflammatory response, and consequence of the inflammation, that may be associated with them.
3.2 Effects of a MedDiet vs. a Western diet on obesity and inflammation
The benefits of the MedDiet have been evaluated in many observational and intervention studies of obesity and its comorbidities, suggesting that the MedDiet can ameliorate obesity across various populations. Although there are numerous investigations of dietary patterns, or specific dietary components, and the health consequences, we will focus on a subset of studies: recently published original research reports that investigate the association between MedDiet adherence, inflammation, and obesity.
3.2.1 Observational studies of the effect of the MedDiet on obesity and inflammation
The results of recent observational studies provide supporting data for the association of the MedDiet with weight loss and reduced inflammation. Dietary intervention studies have shown that the MedDiet, with or without caloric restriction, may induce weight loss in individuals with overweight and obesity (35–37). A study of self-selected diets by individuals with obesity found the MedDiet resulted in an average weight loss of 2.8 kg after 12 months. The weight loss induced by the other diets evaluated, Paleo and intermittent fasting, showed similar results to the MedDiet in this study (38).
Beyond examination of weight changes, studies have reported changes in inflammatory cytokines and a decrease in comorbidities in association with a MedDiet, even without weight loss. A multi-year study of over 39,000 individuals who were included in the Melbourne Collaborative Cohort found an association between the development of T2D and a higher DII, as well as a lower AHEI, but they did not find an association with the MedDiet score in this study (39). A study of 238 individuals who had non-alcoholic fatty liver disease, now called metabolic dysfunction-associated steatotic liver disease (MASLD) (40) showed that adherence to a MedDiet, as assessed by questionnaire, correlated with lower oxidative stress and inflammation (41). Monitoring of 612 subjects during a year-long study found an association between adherence to a MedDiet and lower inflammatory markers, CRP and IL-17. Additionally, changes in the gut microbiome seen with MedDiet adherence correlated with lower frailty, improved cognition, and reduced inflammation (42). In the observational study of 307 male participants as part of the Health Professionals Follow-up study that involved broad examination of sequence data, food logs, and blood biomarkers, long-term adherence to the MedDiet was associated with a change in the gut microbiome and their associated metabolic pathways, including SCFAs, secondary BA production, and fiber metabolism. They did not find an association of the MedDiet with the abundance of Prevotella copri, but they did find an association between the presence of P. copri with reduced risk of CVD, allowing for hypotheses of the pathways of this species that contribute to the observed phenotype (43).
A study of 1,040 individuals, as a subset of the Hellenic National Nutrition and Health Survey, found a significant association between adherence to the MedDiet, lower weight, and reduced hypertension (44). A cross-sectional study of 65 individuals examined the association of diet and inflammation using food diaries, hyperinsulinemic-euglycemic clamps, intravenous glucose tolerance test, dual-energy X-ray absorptiometry, cytokine levels, and adipokine levels. Adherence to a MedDiet was associated with greater insulin sensitivity and decreased inflammatory markers in adults with overweight/obesity (45). One study showed that women with obesity with higher adherence to the MedDiet had lower incidence of MASLD (46). High adherence to the MedDiet was observed to lower the risk of developing an unhealthy metabolic phenotype in individuals with and without obesity (47). Women with obesity and polycystic ovary syndrome who had higher adherence to the MedDiet also had lower cardiometabolic risk factors, including reduced levels of CRP, insulin resistance, and fatty liver index (48). Whole grain consumption, a component of the MedDiet, is also associated with decreased inflammation, in contrast to consumption of refined grains, in part due to its increased amount of dietary fiber (49). The MedDiet also discourages the consumption of red meat which has been consistently associated with inflammation, in favor of poultry or fish, the latter of which are high in omega-3 polyunsaturated fatty acids (50–52).
One strength of observational studies is that they can be quite large with thousands of participants, creating the potential for a statistically very well-powered study. Limitations of observational studies include the uncontrolled variables of each study that are outside of the diet being examined, such as physical activity, sleep habits, stress from injury or other medical conditions, all of which can affect the inflammatory state of the participants. The level of detail of the diets is less than can be obtained in a controlled trial for which food is provided. The range of what is considered a MedDiet might include those scoring anywhere from 10 to 17, out of 17 total points that describe a “fully-compliant” MedDiet on a PREDIMED score for example, so the food consumed by all of the participants in the “MedDiet” group could be quite variable, affecting the results of one study as compared to another. An additional limitation of these studies is that they cannot directly examine specific biological pathways. Despite the limitations of these studies, the strength of the collective evidence supports the role of the MedDiet in reducing obesity-associated inflammation and comorbidities.
3.2.2 Randomized controlled trials of the effect of the MedDiet on obesity and inflammation
Observational studies frequently include large cohorts for statistical power but randomized controlled trials (RCTs) add a layer of rigor and control to the results, moving us closer to determining the cause of the investigated effect. A randomized dietary intervention study of individuals with obesity and features of metabolic syndrome compared 128 genes expressed in abdominal subcutaneous adipose tissue for those on a Nordic diet, which is a Nordic alternative to the MedDiet, and those on a control diet. The authors concluded that the Nordic diet was associated with a decrease in inflammatory gene expression (53). A randomized controlled trial of 82 subjects with overweight/obesity comparing the MedDiet to a control diet demonstrated significant changes in the endocannabinoid system, along with an increase in Akkermansia muciniphila on the MedDiet. The change in the oleoylethanolamide/palmitoylethanolamide (OEA/PEA) endocannabinoid ratio following the MedDiet also diminished the homeostatic model assessment of insulin resistance index and decreased serum high-sensitive CRP, a measure of systemic inflammation. Their results support a role for the MedDiet in ameliorating insulin sensitivity and inflammation (54). A randomized controlled trial involving 28 adults with quiescent ulcerative colitis found that a MedDiet reduced levels of fecal calprotectin, a measure of intestinal inflammation (55). Higher adherence to the MedDiet is associated with lower inflammatory biomarkers, including multiple interleukins, interferon gamma (IFN-γ), TNF-α, and CRP (5, 55).
An evaluation of over 7,000 subjects in the PREDIMED (Prevention with Mediterranean Diet) trial, conducted over a median time of 4.8 years, demonstrated an association between weight gain and increased consumption of refined grains, red meat, potatoes, alcohol, processed meat, white bread, and sweets. Increased waist circumference was associated with increased consumption of snacks, fast-food and pre-prepared dishes, processed meat, alcohol, and sweets (56). Individuals with obesity instructed to follow an energy-restricted MedDiet in the PREDIMED-Plus cohort lost more weight on average than individuals on a standard MedDiet after 1 year (57). A cross-sectional study of 62 individuals with overweight or obesity reported an association of better cardiorespiratory fitness and adherence to a MedDiet with lower blood pressure and lower body fat composition (58). For individuals with genetic risk factors for obesity, those with higher adherence to the MedDiet were less likely to develop obesity in 7–15 years of follow-up (59). A sub-study of the PREDIMED trial examining changes in inflammatory markers after 3 years of MedDiet intervention found reduced plasma levels of several inflammatory cytokines (IL-1β, IL-6, IL-8, TNF-α, IFN-γ, hs-CRP, MCP-1, MIP-1β, RANTES, and ENA78), but these did not reflect at the gene level (60).
RCT MedDiet intervention studies consistently show lower TNF-α, IFN-γ (60–62), and fecal calprotectin (55, 63). Cannabinoids as drugs, particularly those targeting the CB2 receptors, have been associated with relief for a number of inflammatory disorders (64). Bourdeau-Julien et al. (65) and Forteza et al. (66) both detected increased endocannabinoids (OEA and EPEA) following MedDiet intervention in healthy volunteers of normal weight. In contrast, Tagliamonte et al. found that plasma arachidonoylethanolamide (AEA) was decreased following MedDiet intervention in individuals with overweight/obesity, which increased the oleoylethanolamide/arachidonoylethanolamide (OEA/AEA) ratio concomitantly with reduced cholesterol (54).
Olive oil, as well as other components of the MedDiet, such as fresh fruits and vegetables, contain polyphenols that have anti-inflammatory properties. In a study of multiple types of olive oil, individuals eating a diet supplemented with olive oil that contained high amounts of polyphenols had significantly improved plasma inflammatory biomarkers (decreased IL-8 and TNF-α) (67), and another study reported a connection between olive oil and reduced body weight, waist circumference, and hepatic steatosis, in subjects with metabolic syndrome. The anti-inflammatory cytokine IL-10 increased, while pro-inflammatory cytokines decreased (IL-6, IL-17, TNF-α, and IL-1β) (68).
Strengths of these randomized controlled trials include that they can control for variables that are not controlled in observational studies. For example, the PREDIMED and PREDIMED-Plus trials each compared two versions of a MedDiet: MedDiet with olive oil vs. MedDiet with nuts, or energy-restricted MedDiet vs. non-energy-restricted MedDiet (57, 60). Researchers are able to collect health information that may not be available in large observational studies, such as information on alcohol consumption, physical activity, and medication/supplement use, which can be used as exclusion criteria or taken to account in statistical analyses (55, 65, 66). Additionally, when studies provide the food for the participants, the content is known in detail and is much better controlled than when participants prepare their own food. Bourdeau-Julien et al. (65) and Forteza et al. (66) provided food to their volunteers, so they could exactly track the nutrient intake and compliance of their volunteers. Limitations of the RCTs include that most often there are a low number of participants: the studies described here had fewer than 100 study subjects, with the exception of the PREDIMED trial studies. Another limitation is that the food consumed is determined from records that are not seven days per week, so extrapolation is required to interpret the information as the individual's whole diet, and data are dependent on the accuracy and adherence of the study subjects. Most of the studies described here have a narrowly defined inclusion criteria, such as those with a specific disorder, so the results may not translate to healthy individuals or individuals with other medical conditions. Most of the studies examine the effect of the intervention over a short period of time, often weeks to several months, raising the question of whether the intervention had time to establish an effect, and whether an effect would be sustainable. However, the PREDIMED trial, which is the exception and covered a long period of time, ended after 4.8 median years of follow-up, and showed strong evidence of the benefit of the MedDiet in many areas, resulting in over 350 publications so far according to their website (69). Overall, the results of the RCTs are consistent with the results of the observational studies and the evidence supports the role of the MedDiet in modulating inflammation and obesity.
3.2.3 Mechanisms of dietary effects on inflammation
Meta-analyses of multiple studies provide support for the conclusion that the MedDiet reduces the risk of obesity. For example, a meta-analysis of 15 RCTs of MedDiet interventions that measured obesity parameters in children and adolescents reported that the interventions had a significant effect on reducing BMI and obesity in this population (70). A systematic review of ten RCTs found that diets such as the MedDiet, and other similar dietary patterns, were associated with a significant reduction of CRP and an increase in adiponectin, both indicators of reduced inflammation (71). A systematic review of 20 RCTs reported the following changes in biomarkers in association with a MedDiet: decreased pro-inflammatory cytokines IL-1α, IL-1β, IL-5, IL-6, IL-7, IL-8, IL-18, IFN-γ, TNF-α, CRP, high-sensitivity CRP and increased anti-inflammatory cytokines IL-4 and IL-10 (5). A meta-analysis of 32 studies concluded that omega-3 polyunsaturated fatty acid dietary supplementation had anti-inflammatory effects, as shown by a decrease in CRP and TNF-α (72). Therefore, the anti-inflammatory effects of the MedDiet as a whole, as well as of the individual dietary components, contribute to its status as a healthy diet that may combat obesity. Data that associate the MedDiet with reduced inflammation are abundant but obtaining an understanding of the detailed pathophysiology is a more challenging goal. Some recent studies delving into the mechanisms of dietary effects on inflammation are reviewed below.
To define biological pathways affected by components of the diet, studies using murine models and in vitro cultures can be quite valuable (23, 73, 74), as specific mechanistic hypotheses can be generated from such studies. A study of 952 individuals using genome-wide genotyping, gut metagenomic sequence data, and fecal SCFA levels, reported that increased butyrate production was associated with impaired insulin response and that abnormal production or absorption of propionate was associated with T2D risk (75). In Section 5.1, we will discuss the evidence that SCFAs are increased in response to the MedDiet and this excellent study by Sanna et al., combined with the other literature, allow us to associate the MedDiet to SCFA changes to an impaired insulin response and obesity.
Cross-sectional studies examining adherence to the MedDiet and CRP concentrations found these to be inversely correlated (76, 77). This was also observed in a large population-based study (78). A recent study attempting to better define specific physiologic connections between obesity and inflammation used a mouse model with a CRP transgene. The investigators provided evidence that CRP is not merely a marker of inflammation, but instead has a causal role in the development of obesity (6).
As part of the CORDIOPREV (CORonary Diet Intervention with Olive oil and cardiovascular PREVention) prospective RCT, researchers suggest that the genetic variant of the NLRP3 inflammasome may modulate the benefits of the MedDiet (79). Murine and human brain cells treated with virgin olive oil reduced activation of the inflammatory TLR4/NLRP3 axis (80). Deficiency of NLRP3 attenuated systemic inflammation, especially with a HFD, caused changes in the plasma metabolome, metabolites in the liver and myocardium, and gut microbiota compared to wild-type mice (81). The saturated fats common in the Western diet are also associated with increases in inflammation by the nuclear factor kappa B (NF-κB) pathway and NLRP3 inflammasome, possibly contributing to obesity, as reviewed by Las Heras et al. (24). Even occasional consumption of Western diet patterns increased inflammation and insulin resistance in a rodent study (82). The effects of dietary patterns on health are complex and understanding their mechanisms will be necessary to use diet for the treatment of obesity and other health conditions.
4 MedDiet and the gut microbiome
Dietary patterns such as the Western diet and UPF consumption likely contribute to obesity partially through their impact on the gut microbiome. The gut microbiome is highly modifiable by diet and multiple studies have shown alterations to the microbiome from dietary patterns like the MedDiet (65, 83–85). Due to the complexity of the microbiome and the variation in how MedDiet is characterized between studies, it is difficult to define one consistent microbiome signature associated with the MedDiet (86). Clear changes in the microbiome have not been found during all MedDiet interventions, especially when the starting microbiome of the individual had high diversity, as the diverse microbiome was somewhat more resistant to changes (65, 87). When trying to assimilate all of the available literature on a topic, it is our view that if a meta-analysis reveals striking differences in results between various studies, this does not negate the results of each individual well-controlled study, but instead the meta-analysis demonstrates that generic conclusions about the MedDiet may not apply to every population and disease state. The discrepancies highlight our lack of understanding regarding which of the key variables in each study are most contributory to the outcome. The MedDiet can also have a considerable impact on microbial metabolites, even without a significant corresponding change to microbiome composition. Regardless of our limited understanding of the complex gut microbial communities, and their individual or overlapping roles, there are data to support beneficial changes to the microbiome from MedDiet intervention.
Several studies have investigated the role of diet in SCFA metabolism. For example, a MedDiet intervention in women with obesity was able to reverse features of dysbiosis by increasing microbiome biodiversity and SCFA-producing taxa (88). MedDiet adherence in both individuals with obesity and normal weight was positively correlated with SCFA-producing taxa such as Bifidobacterium animalis (89). MedDiet intervention has been reported to increase fecal SCFAs (90), and the abundance of butyrate-producing microbes (87).
Some studies have focused on changes in BAs. An 8-week RCT of 82 individuals with overweight and obesity reported that increased adherence to a MedDiet resulted in a reduction of plasma cholesterol and fecal BAs. Gut microbiome analysis revealed an increase in Faecalibacterium prausnitzii and decrease in Ruminococcus gnavus. Furthermore, there were increased urinary urolithins, fecal BAs degradation, and insulin sensitivity in subjects on the MedDiet, which correlated with specific microbial taxa (91).
Fiber is known to be a critical component of the MedDiet. Dietary fiber originates primarily from whole grains and vegetables, foods that can serve as a prebiotic for bacterial growth, but different types of fiber may have different effects. Healthy adults supplemented with resistant potato starch had increased bifidobacteria and butyrate production in their gut, while supplementation with fiber from maize and chicory root did not show a statistically significant difference. Among individuals whose microbiome changed, the highest butyrate concentrations were correlated with Ruminococcus bromii or Clostridium chartatabidum increases (92). The effect of fiber supplementation on the microbiome and SCFA production varies between individuals. The authors report that some individuals are limited in their capacity to produce SCFA from fiber supplementation, and this may be driven by their microbiome (92, 93). Another dietary intervention showed that fiber from a mixture of fruits and vegetables resulted in increased bifidobacteria but no increases in SCFAs over a short 2-week period (94).
There have been numerous studies of the MedDiet component olive oil. Mice supplemented with olive oil had microbial changes associated with reduced inflammation and the prevention of colorectal cancer compared to mice fed other fat types. Interestingly, the olive oil diet in the mice increased the Firmicutes/Bacteroidetes ratio, which correlated with lower colorectal cancer risk but higher risk of obesity in this study (95). Olive oil consumption, particularly oil enriched with phenolic compounds, was also associated with increased bifidobacteria in a RCT in individuals with high cholesterol (96). Olive oil is an important source of flavonoids, and microbial metabolism is required to make flavonoids biologically available (97).
There are a few common patterns to the changes to the microbiome that have been reported repeatedly, either in studies comparing the MedDiet to a Western diet, or in studies comparing individuals with obesity to lean controls. A study of 92 individuals found an association of overweight/obesity with specific gut microbiota patterns when compared to those of normal weight: Bacteroidetes taxa were decreased and several Firmicutes taxa were increased (98). The Western diet is associated with decreased beneficial bacteria such as bifidobacteria and eubacteria in the human gut (99) and, in rodents, decreased Akkermansia spp., species that are associated with a number of human diseases (100). Lean mice receiving fecal transplants from mice with obesity gain weight (101, 102) and individuals with obesity receiving transplants from lean individuals had improved metabolic disease symptoms (103). These studies show the combined value of animal and human studies. The studies make associations between the microbiome, obesity, and metabolic syndrome. The data supporting an association of the MedDiet with a reduction in inflammation and obesity from Section 3 of this review, combined with studies in Section 4 that investigated the microbiome and obesity, serve to connect the MedDiet to inflammation, obesity, and the gut microbiome. Every study does not prove direct causation, but the results allow the development of a larger hypothesis for definitive testing. Human fecal microbiome transplants have successfully altered the microbiomes of individuals with obesity to resemble lean donors, however no change in BMI occurred over the 12-week study. The time required to significantly change the BMI may be longer than the time to alter the microbial community (104). Individuals with obesity have distinct microbial communities, often characterized by having an increased ratio of Firmicutes to Bacteroides compared to lean individuals and decreased microbial diversity (98, 102) although these results are not consistent across all studies (105).
Gut permeability and energy efficiency are two other elements that have been examined closely. The microbiomes of individuals with obesity may result in increased energy absorption from food. Increases in Firmicutes relative to Bacteroides elevate levels of alpha amylases and amylomaltases for more efficient energy extraction from foods, which increases the number of calories absorbed (102). An imbalanced microbiome can also contribute to obesity through its role in inflammation. The dysbiosis of obesity can lead to increased gut permeability and allow proinflammatory molecules to enter systemic circulation. The microbiome of humans and mice with obesity reduced the expression of the zonula occludens-1 tight junction protein, weakening the gut barrier (106). Individuals with obesity also have increased Gram-negative bacterial taxa of the Enterobacteriaceae family in their microbiome, resulting in elevated levels of LPS which can leak from the gut (98). LPS is proinflammatory and promotes low grade inflammation which promotes the storage of excess lipids (107). Further discussion of LPS as it relates to the MedDiet is included in Section 5.4 of this review.
By combining all of the findings from the many investigations discussed above, a positive role of the MedDiet on obesity and inflammation seems quite clear. We have yet to obtain a detailed understanding of the pathophysiology of obesity, but recent work has started to dissect the role of specific gut microbial metabolites in these pathways.
5 Interplay of obesity, the MedDiet, and gut-derived metabolites
Obesity is associated with changes in the composition of the gut microbiota, and in the amounts and types of microbial metabolites that are formed. Two groups of metabolites of demonstrated importance in obesity physiology are SCFAs and BAs. An increase in a third gut-derived metabolite, TMAO, has been associated with obesity and inflammation; however, its effects are proposed to be context-dependent (108, 109). A fourth metabolite associated with inflammation and obesity is LPS. Obesity has been associated with increased intestinal permeability, which allows the movement of bacteria and bacterial products, like LPS, into the bloodstream with an associated increase in inflammation (110). The interactions between the obese gut microbiota, gut-derived metabolites, and the effects on its host are quite complex and multifactorial.
Previous reviews indicate that adults with obesity have been shown to have increased total concentrations of fecal SCFAs (11, 12) and BAs (13, 14), likely due to dysregulated metabolism and absorption. However, analysis of the gut microbiota of over 1,900 individuals in the METS-microbiome study showed an association of obesity with a reduction of fecal SCFA concentrations, gut microbial diversity, and of the bacteria that synthesize SCFAs, while the country of origin for the study subjects was the most important variable. Using predictive modeling, SCFA concentrations could not predict obesity status, suggesting the relationship between SCFAs and obesity is still unclear (111). Many of the studies examining SCFAs in populations with overweight/obesity have been cross-sectional analysis, with or without disease comorbidities and/or medications, and using different biospecimen types (fecal vs. blood), making it difficult to draw definitive conclusions (112–114). Meanwhile, the clinical controlled trials measuring SCFAs in populations with obesity also apply various pre/probiotic, dietary, or weight-loss interventions which make comparing studies difficult (115–117). Several variables such as diet and physical activity can affect SCFA production, and the direction of change for individual SCFAs (i.e., acetate vs. butyrate vs. propionate, etc.) likely differ, as is observed in Table 2, and should be considered when comparing data between studies.
Few studies have examined SCFA levels in children with obesity, however, within the last 5 years, two studies showed increased fecal SCFA concentrations (118, 119), while one study showed fecal SCFAs were reduced (120) in children with obesity. The differences in study results may be due to study design and inclusion criteria, as Wei et al. and Gyarmati et al. excluded volunteers who had received antibiotic, prebiotic, or probiotic treatments within the last 3 months before the studies, while the study by Slizewska et al. did not (118–120).
In Tables 1–3 we have summarized some of the recent human studies that have investigated changes in gut metabolites in association with a MedDiet compared to other diets. Below, we discuss the effects of MedDiet on SCFAs, BAs, TMAO, and LPS, and the mechanisms by which the MedDiet could potentially alter the gut microbiota to combat obesity.
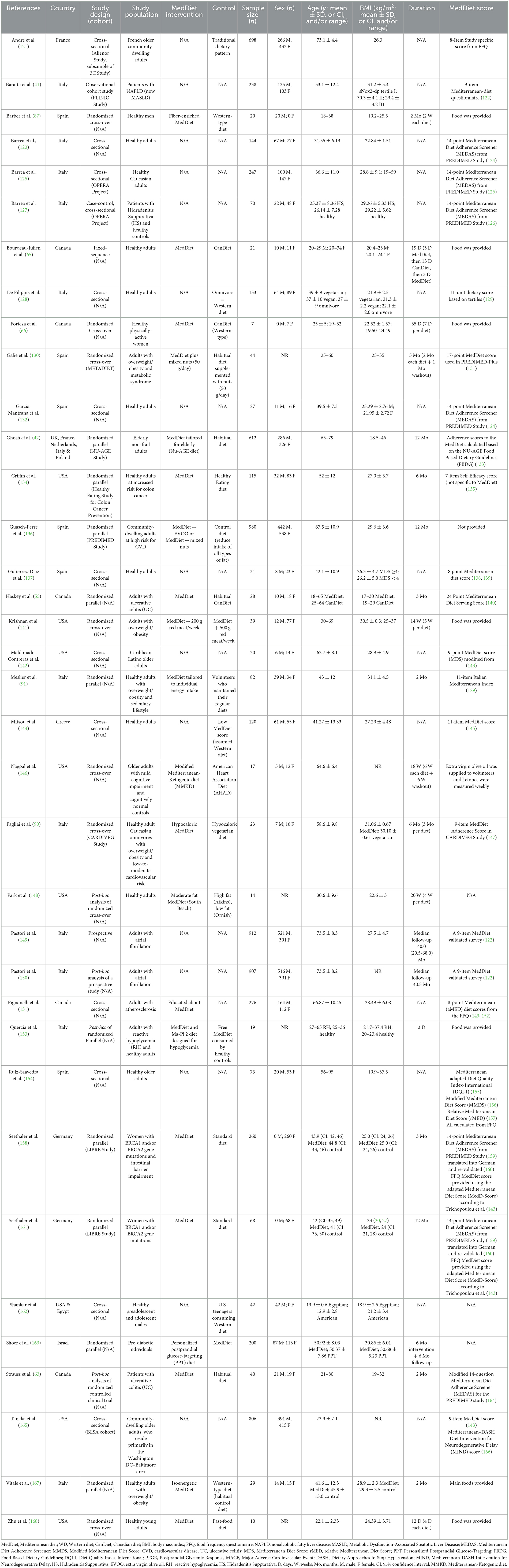
Table 1. Characteristics of recent clinical studies investigating the effects of the MedDiet on gut-derived metabolites.
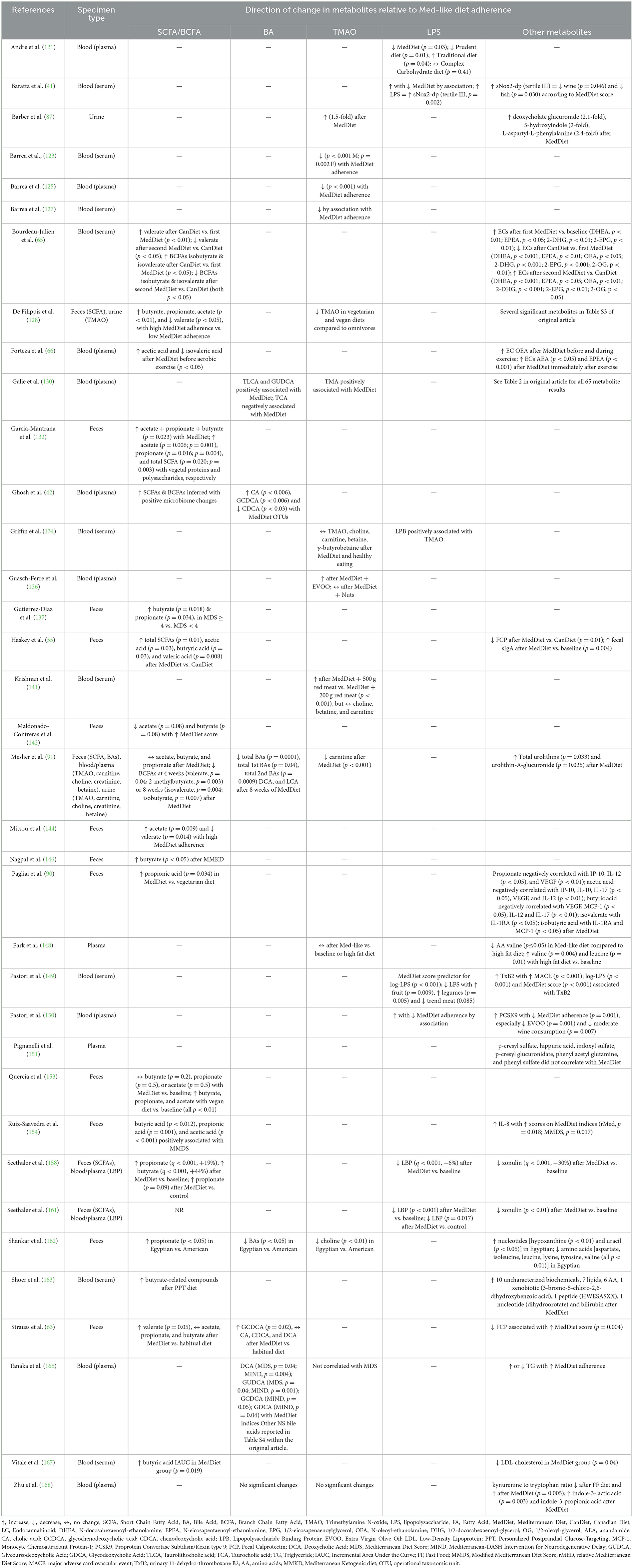
Table 2. Results summary of metabolite changes in recent clinical studies investigating associations between gut-derived metabolites and the MedDiet.
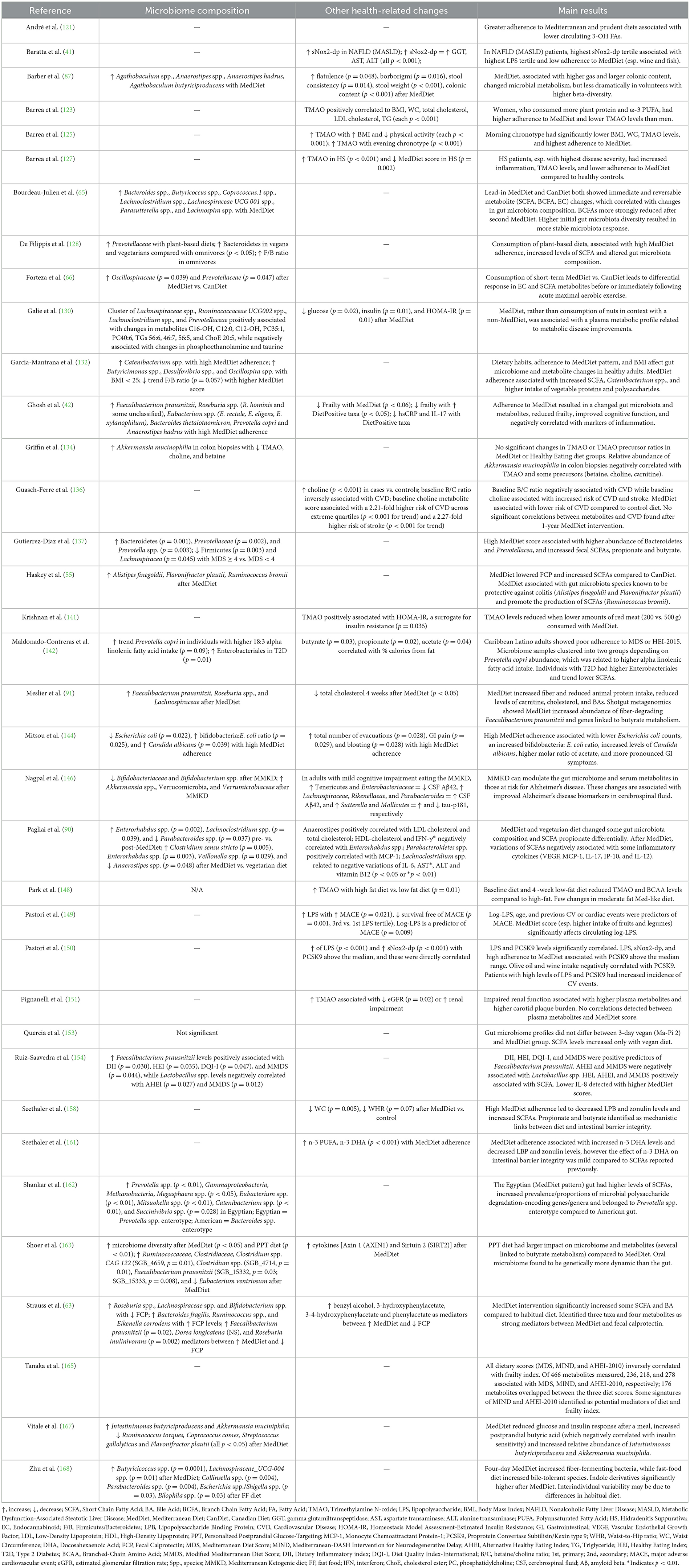
Table 3. Results summary of microbiome and other changes in recent clinical studies investigating associations between gut-derived metabolites and the MedDiet.
5.1 Short-chain fatty acids
SCFAs are derived from the fermentation of non-digestible dietary fiber by gut bacteria, and they play a critical role in intestinal physiology. Acetate, propionate, and butyrate account for 95% of the SCFAs in the intestinal tract. In a healthy individual, < 5% of SCFAs are excreted in feces, as most are absorbed through the gut mucosa and utilized in the gut, while some enters the bloodstream (12). While dietary fiber has been shown to promote weight loss and improve glycemic control, the complete biological role of SCFAs in this process remains unclear (83). Some SCFAs have been shown to beneficially affect host metabolism through secretion of gut hormones, such as glucagon-like peptide-1 (GLP1) and peptide YY, that affect appetite, reduce inflammation, and increase fat oxidation, as reviewed by Hernandez et al. (169). Studies in mice, which allow experimental designs that cannot be performed in humans, have added greatly to our understanding of diet contributions to gut microbiota-derived metabolic changes. Recently, Bachem et al. showed the impact of gut microbiota on the fate of CD8+ T-cells through the production of SCFAs in mice consuming a high-fiber diet (Figure 1) (171). SCFA supplementation also restored the number of enteric neurons that were depleted following antibiotic treatment in mice (177). In mice, reduction of SCFAs by a fiber-deficient diet led to alterations of the gut microbiota, increased intestinal permeability, inflammation, and cognitive impairment. Furthermore, SCFA supplementation improved these deficits (178). These recent studies support a role for SCFAs in modulation of immunity, inflammation, and potentially obesity.
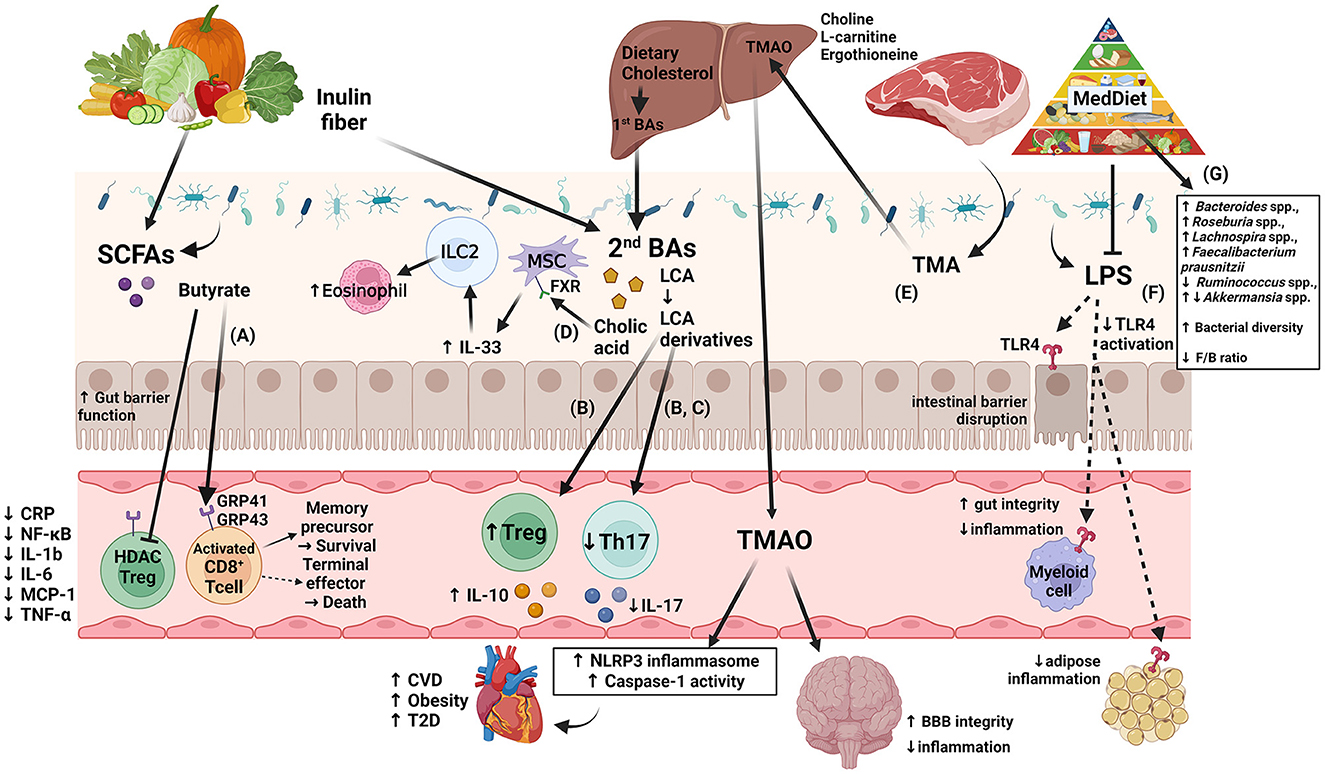
Figure 1. Diagram of several of the proposed immune modulation pathways that incorporate the four gut microbiota-derived metabolites: SCFA, BA, TMAO, and LPS, highlighting new findings from recent human and murine studies. (A) Production of SCFAs improves barrier function, Treg cell activation, and anti-inflammatory effects, as reviewed in (24, 170). A murine study showed that by uncoupling the tricarboxylic acid cycle from glycolysis, butyrate promotes the uptake and oxidation of fatty acids, leading to enhanced cellular metabolism and memory potential of activated CD8+ T-cells (171). (B) Another murine study showed that derivatives of secondary bile acid LCA, 3-oxoLCA and isoalloLCA, were identified as T-cell regulators. While 3-oxoLCA inhibited Th17 cell differentiation through binding to its main transcription factor, RORγt, isoalloLCA upregulates Treg cell differentiation through the production of mitoROS, which increases FoxP3 expression (172). (C) Using murine models and in vitro studies with bacteria derived from humans, several bacterial genera were identified as producers of 3-oxoLCA, including Gordonibacter pamelaeae P7-E3, Eggerthella lenta P7-G7, Raoultibacter massiliensis P7- A2, Collinsella intestinalis P8-C1, Adlercreutzia equolifaciens P11-C8, and Clostridium citroniae P2-B6. Similar to 3-oxoLCA, isoLCA inhibited Th17 cell differentiation through RORγt. Bacterial hydroxysteroid dehydrogenases convert LCA to 3-oxoLCA and isoLCA (173). (D) Mice fed an inulin diet showed changes in the gut microbiota and BA production associated with type 2 inflammation in the intestine and lungs, including production of IL-33 and activation of group 2 innate lymphoid cells and eosinophilia (174). (E) TMAO production induces NLRP3 inflammasome and caspase-1 activity leading to increased inflammation; long-term activation of these pathways contributes to obesity, CVD, and T2D, as reviewed in (15). Interestingly, while dietary TMA disrupted BBB function and tight junction integrity, TMAO enhanced BBB integrity and protected against inflammatory insult through tight junction regulator annexin A1 (175). (F) Generally, LPS binds TLR4 located on myeloid cells and adipocytes activating inflammatory pathways and causing disruption of the intestinal barrier as reviewed in (166, 176). However, MedDiet adherence in human studies resulted in reduced levels of circulating LPS markers, reduced inflammation, and maintained intestinal barrier integrity (158). Dashed arrows represent the outcome following blockage of LPS action. (G) As shown in Table 3, MedDiet alters the gut microbiota in populations with obesity, including the change in prevalence of several taxa: Bacteroides spp. (42, 63, 65), Roseburia spp. (42, 63, 91), Lachnospira spp. (63, 65, 91, 168), Ruminococcus spp. (42, 91, 137), Akkermansia spp. (54, 162, 167), and Faecalibacterium prausnitzii (42, 91, 154, 163), and generally leads to increased bacterial diversity and a reduced Firmicutes/Bacteroides ratio, which were decreased and increased with obesity, respectively, as reviewed in (11, 12). Image created with BioRender.com. 3-oxoLCA, 3-oxolithocholic acid; BAs, bile acids; BBB, blood-brain barrier; CA, cholic acid; CRP, C-reactive protein; CVD, cardiovascular disease; F/B, Firmicutes/Bacteroidetes; FXR, farnesoid X receptor; GRP, G-protein-coupled receptor; IL, interleukin; ILC2, group 2 innate lymphoid cells; isoalloLCA, isoallolithocholic acid; isoLCA, isolithocholic acid; LCA, lithocholic acid; LPS, lipopolysaccharide; MCP, monocyte chemotactic protein; MedDiet, Mediterranean diet; mitoROS, mitochondrial reactive oxygen species; MSC, mesenchymal stromal cell; NF-κB, nuclear factor kappa-light-chain-enhancer of activated B cells; NLRP3, NOD-like receptor family pyrin domain-containing 3; RORγt, retinoid-related orphan receptor γt; SCFAs, short-chain fatty acids; T2D, type-2 diabetes; Th17, T helper cells expressing IL-17; TLR4, toll-like receptor 4; TMA, trimethylamine; TMAO, trimethylamine N-oxide; TNF-α, tumor necrosis factor alpha; Treg, regulatory T cells.
Dietary fiber that affects production of SCFAs can come from many sources, but mostly fruits, vegetables, and whole grains, which are staples of a MedDiet. Several recent clinical studies have shown that there is increased production of some SCFAs, based on blood and/or feces measurements (see Table 2), following MedDiet intervention in healthy volunteers (66) or individuals with disorders such as ulcerative colitis (63), intestinal barrier impairment (158), overweight, or obesity (90, 167). Observational studies have also shown that better adherence to the MedDiet has been associated with increased levels of SCFAs (128, 132, 144). Another study of individuals who followed a hypocaloric MedDiet (n = 21), a very-low-calorie ketogenic diet (n = 18) and volunteers who underwent sleeve gastrectomy bariatric surgery (n = 22) showed MedDiet was enriched in several pathways related to SCFA fermentation (179). Following MedDiet intervention in volunteers with overweight, SCFAs were negatively correlated with changes in some inflammatory cytokines, including VEGF, MCP-1, IL-17, IP-10, and IL-12 (90). Meanwhile, a few studies have shown no significant changes (91) or decreases (65) in SCFAs following a MedDiet. Tracking the changes in specific SCFAs may be useful to our understanding as future studies continue.
Due to resource constraints, many intervention studies are limited to a very short time interval with the longest dietary intervention investigating changes in SCFAs described above spanning 3 months. This short time frame sets a significant limitation on the conclusions that can be drawn from the studies in terms of generalizing to a stable effect over years. In a small study of 21 healthy-weight individuals who consumed a MedDiet for 3 days, then a Canadian diet [reflecting the average Canadian dietary intake, which would be considered a Western diet (180)] for 13 days, followed by a MedDiet for an additional 3 days, circulating SCFAs and branched-chain fatty acids were not significantly altered by the first MedDiet intervention, but propionate, valerate, isobutyrate, and isovalerate were increased by the Canadian diet, then decreased after the second MedDiet (65). While the data show that circulating SCFA concentrations can be altered following MedDiet, and changes can occur over a short period of time, one must be careful about extrapolation of results from a few days to an effect that might occur after years of following a specific dietary pattern. Additional interventional long-term studies are needed to resolve discrepancies between study results and to assess whether these changes remain stable over time.
A systemic meta-analysis of 34 animal studies showed that diets rich in anthocyanin-rich fruits and vegetables significantly reduced the Firmicutes/Bacteroides ratio and increased SCFA production in rodents. They found that higher production of acetic acid, butanoic acid, and propionic acid was observed with longer periods of dietary intervention (≥4 weeks) and higher doses of anthocyanins. Anthocyanin supplementation had the greatest effect on acetic acid concentration in high fat/cholesterol diet models, while the greatest effect on butanoic acid and propionic acid were in HFD-induced obesity models (181). These studies provide an initial understanding of the role of specific components of the MedDiet in modulating the gut microbiota and gut-derived SCFAs.
Because humans with obesity have been reported to display excessive levels of fecal SCFAs (potentially due to lack of ability to metabolize and absorb these metabolites), as reviewed in (11, 112, 182), a strategy was proposed to combat obesity by altering the gut microbiota with a goal to modulate the number of SCFA-metabolizing or SCFA-producing bacteria through a dietary change. In a small study of 20 elderly women, obesity was associated with an increase in pro-inflammatory Collinsella spp. and Streptococcus spp. There was also a decrease in SCFA-producers, including Lachnospiraceae and Ruminococcaceae. Relative abundance of Collinsella spp. was reduced following both a hypocaloric MedDiet for 15 days and a hypocaloric MedDiet enriched with a probiotic mixture for 15 days (and both included an individual-based exercise regimen) (88). These studies make associations between MedDiet, SCFA, inflammation, and obesity.
In a sub-study of the PREDIMED trial in volunteers with overweight/obesity, an energy-restricted MedDiet resulted in weight loss and changes in the gut microbiota after a 1-year intervention. While SCFAs were not measured directly, there was an increase in some SCFA-producing microbes, including Lachnospira spp. and Lachnospiraceae NK4A136 (57). When selecting subjects with the highest fecal butyrate increase at 4 weeks after MedDiet initiation, higher relative abundances of Faecalibacterium prausnitzii and Lachnospiraceae family were also observed (91). Likewise, the Obekit study found SCFA-producing bacteria, including Bifidobacterium animalis, Oscillibacter valericigenes, Oscillospira (Flavonifractor) plautii, Ruminococcus bromii, Roseburia faecis, and Paraprevotella clara, in a northern-Spanish population with overweight/obesity and high MedDiet adherence (89). While our discussion is focused on the MedDiet, many studies have combined caloric restriction with the MedDiet, so interpretation of results cannot distinguish the contribution of each of these two variables in many cases. However, it is evident that diets rich in fiber, flavonoids, and polyphenols, such as the MedDiet, are shown to impact the gut microbiota composition, and importantly, increase the number of bacteria with the ability to produce and metabolize SCFAs. The studies summarized in Table 2 report circulating and/or fecal SCFA levels. One cautionary note derives from a study by Farhat et al. that demonstrated poor correlation between serum and fecal SCFAs, concluding that one is not a good proxy for the other (183). In summary, many studies associate a MedDiet with an increase in SCFAs, and mechanisms are proposed by which SCFAs modulate immunity/inflammation; however, results are not entirely consistent across studies, so work is ongoing.
5.2 Bile acids
BAs, which are secreted into the intestine in the presence of fats as part of the digestive process, are generated from dietary lipids, cholesterol, and fat-soluble vitamins in hepatocytes via two main synthetic pathways. Primary BAs are stored in the gallbladder and secreted in the gut after conjugation. Secondary BAs are generated via further interaction of primary BAs with the gut microbiome. Similar to SCFAs, BAs are critically important in gut physiology, and secondary BAs alter the gene expression of enterocytes and of gut bacteria (184). Elevated secondary BAs in serum and feces have been associated with increased inflammation. Secondary BAs act as ligands for G-protein-coupled bile acid receptor 1 and farnesoid X receptor (FXR), the activation of which mediates immunity and promotes anti-inflammatory effects. Under normal conditions, there is a balance between primary and secondary BAs; however, this balance can be disrupted by gut microbiota dysbiosis (185).
While some mechanisms of BA metabolism are known and can be reviewed here (186), others have yet to be explored. Hang et al. showed that derivatives of the secondary BA lithocholic acid (LCA), mediate host immune response by mediating T helper cells expressing IL-17A (TH17) and regulatory T (Treg) cell differentiation. The metabolites 3-oxoLCA and isoLCA were shown to inhibit TH17 cell differentiation by binding to transcription factor retinoid-related orphan receptor (ROR) γt (172, 173), while production of mitochondrial reactive oxygen species by isoalloLCA increased expression of FoxP3 and Treg cell differentiation (172) (Figure 1). A diet of inulin fiber altered the composition of mouse microbiota and lead to increased production of BAs, which is presumed to have aided in the production of IL-33 and activation of innate lymphoid cells and eosinophils to promote type 2 inflammation (Figure 1). These affects were BA-dependent because (1) depletion of the BA receptor FXR and (2) genetic deletion of a BA-metabolizing enzyme abrogated these affects (174). HFD-fed mice with obesity had significantly increased taurine-conjugated BAs, but these affects were nearly abrogated in NLRP3-deficiency (81). During the last several years, there have been significant discoveries regarding the role of BAs in immunity and inflammation; it is proposed that manipulation of the gut microbiota, and thus of BA production, may be a useful approach to treatment for obesity.
There is an interdependent relationship between the host biological pathways and bacterial metabolism. The gut microbiome has been shown to impact the chemistry of all organs, including amino acid conjugations of host BAs (186). Conversely, BAs have considerable effects on the structure of the gut microbial community; they can stimulate the growth of microbes that utilize BAs as an energy source and repress the growth of microbes that are intolerant of its effects (184). A recent study suggested that human gut bacteria from many families within the Actinobacteria and Firmicutes phyla produce 3-oxoLCA, including Gordonibacter pamelaeae P7-E3, Eggerthella lenta P7-G7, Raoultibacter massiliensis P7- A2, Collinsella intestinalis P8-C1, Adlercreutzia equolifaciens P11-C8, and Clostridium citroniae P2-B6 and these may work together to affect the immune system (173).
In a fecal microbiota transplant pilot clinical trial in volunteers with obesity, BA profiles were modified to match that of the lean donor after 12 weeks, including sustained reduction in taurocholic acid, without any change in BMI (104). This trial did not document any change in BMI, however the 12-week time period may not have been long enough to capture significant weight change and future studies with a longer trial period are needed. A secondary analysis from fecal samples collected from these volunteers identified Bacteroides ovatus and Phocaeicola dorei, which positively correlated with unconjugated BAs, and Bifidobacterium adolescentis, Collinsella aerofaciens, and Faecalibacterium prausnitzii, which positively correlated with secondary BAs, as the bacterial species candidates that affected gut BA metabolism (187). In addition to dietary components, the caloric level of a diet must be considered, as calorie restriction has been shown to decrease production of BAs (188, 189). Supplementation with non-12α-hydroxylated BAs in mice increased thermogenesis and slowed weight gain (188). It is well-known that BAs impact the gut microbiota and are influenced by dietary changes, however, there are limited studies that have incorporated the measurement of BAs in relation to the MedDiet pattern.
The MedDiet pattern limits the amount and types of dietary fat intake, and therefore has potential to change the amounts and types of BAs produced by the host. In MedDiet intervention studies, lower production of primary and secondary BAs has been observed (42, 91). Fecal secondary BAs were significantly reduced by 4 weeks and primary BAs were reduced by 8 weeks following initiation of a MedDiet. Volunteers with the greatest reduction of total BAs also had higher baseline levels of Bilophila wadsworthia, which significantly decreased after 4 weeks (91). Although circulating BA levels remained unchanged after just 4 days of a MedDiet compared to a fast-food diet, the primary to secondary BA ratio was found to positively correlate with Bifidobacterium spp. and negatively correlate with Roseburia spp. (168).
Dietary diversity has been shown to inversely correlate with several circulating secondary BAs (190), and a MedDiet tends to have more diverse foods than a Western diet (191). A variety of fruits and vegetables eaten on a MedDiet contain flavonoids, which are shown to have anti-inflammatory properties, in part through pathways involving BAs. In murine studies, administration of the hops-derived prenylated flavonoid xanthohumol, and its semi-synthetic derivative tetrahydroxanthohumol, altered the gut microbiota and BA metabolism, and reduced adipose tissue inflammation (192). The MedDiet also promotes intake of whole grains compared to refined grains, as eaten in the typical Western diet. Two secondary BAs were lowest in a diet of unrefined carbohydrates composed from a high proportion of whole grain foods (193). A single fat source alone may not be enough to alter BA production in humans, as consumption of virgin olive oil with or without thyme did not alter BA production when volunteers were asked to limit their polyphenol-rich food intake (96). Compared to Western diet, MedDiet promotes reduced overall fat intake, with primary intake of healthy fats like olive oil, resulting in lowered production of secondary BAs, favoring reduced inflammation and a decreased risk of obesity.
5.3 TMAO and its dietary precursors
TMAO is a product of choline, L-carnitine, betaine, and ergothioneine via metabolism by the gut microbiota. Trimethylamine is generated within the intestinal lumen by enzymatic changes of the aforementioned precursors, absorbed from the intestine, and transferred to the liver where flavin-dependent monooxygenase isoforms 1 and 3 convert it to TMAO. Dietary choline and L-carnitine are primarily found in animal products while betaine is mostly from plants. Dietary ergothioneine is found in both some animal (mostly liver and kidney organs) and plant (including mushrooms and beans) products (83, 194). Krueger et al. describe the negative effects of elevated TMAO on adipose tissue as it relates to the discussion of obesity (108). Increases in TMAO has been found to correlate with an increase in BMI and visceral adipose and TMAO levels over 8.2 μM predict the occurrence metabolic syndrome (77). Obese mice that had a TMAO-producing enzyme (FMO3) conferred protection against obesity (109). TMAO also have an inflammatory effect through activation of the NLRP3 inflammasome (195). However, similar to other metabolites, the physiology of TMAO is complex, as TMAO acts on multiple organs, with evidence of beneficial effects in the brain. Long-term exposure to TMAO in mice protected the brain from inflammatory challenge with LPS and reduced activation of astrocytes and microglia (Figure 1) (175). While some studies might paint TMAO as a negative factor and many proposed healthy diets eliminate or strictly limit red meat, the study by Hoyles et al. suggest that the full picture of TMAO's role in obesity and other metabolic syndromes has yet to be understood.
Elimination of red meats, which are known to be a source of dietary choline, is encouraged on the MedDiet. An observational study comparing healthy adults found circulating levels of TMAO negatively correlated with MedDiet score after adjusting for BMI, physical activity, and total energy intake (123). A similar result was seen in a separate study with a population of volunteers including 30% with obesity, as those with a morning chronotype (a term used to describe a person's circadian preferences) had the highest adherence to a MedDiet and the lowest circulating TMAO concentrations (125, 127). Choline participates in multiple essential functions, including serving as a precursor of essential cellular components, and is oxidized to betaine in the methylation cycle of multiple pathways.
A lower betaine/choline (B/C) ratio is associated with features of metabolic syndrome, so the B/C ratio is a biomarker of metabolic function. A case-cohort study within the PREDIMED trial found that volunteers assigned to the MedDiet intervention with a high B/C ratio had a lower risk of CVD compared to controls with a low B/C ratio (136). In contrast, a separate case-cohort of the PREDIMED study, found that individuals with the highest quartile of baseline TMAO and α-glycerophosphocholine had a lower risk of T2D (196). One-year follow-up data from the Spanish PREDIMED-Plus trial showed the greatest increase in dietary choline or betaine intake was associated with improved serum glucose and HbA1c levels, as well as reduced body weight and total cholesterol in subjects with overweight/obesity (197). A secondary analysis of a randomized clinical trial in adults with overweight/obesity comparing the effects of consuming different concentrations of unprocessed lean red meat, along with a MedDiet, found that lower consumption of red meat resulted in lower serum TMAO concentrations after 5 weeks (141). In individuals with healthy weight, there was a 1.5-fold increase in urinary TMAO after 2 weeks of a MedDiet compared to a Western-type diet (87). However, another study involving adults with overweight/obesity found urinary carnitine was significantly reduced by 4 weeks following MedDiet intervention and remained reduced at 8 weeks (91).
Few studies have examined associations between TMAO, MedDiet, and gut microbiome composition. Prevotella copri was significantly lower in female non-human primates fed a MedDiet compared to a Western Diet. Interestingly, among those fed a Western-diet, those who had highest amounts of P. copri also had elevated levels of urinary carnitine-based metabolites (18). Another study found no difference in plasma TMAO levels, or its precursors, before and after a 6-month MedDiet intervention in healthy adults at risk of colon cancer. However, the relative abundance of Akkermansia mucinophilia in colon biopsies was modestly and inversely associated with TMAO, betaine, choline, and carnitine at baseline, and this association was weaker at 6 months following MedDiet introduction (134).
A study involving children and adolescents with obesity found that non-responders (defined as subjects whose BMI z-score was maintained or increased) to nutritional or exercise regimens had significantly increased choline and a decreased B/C ratio after 6 months. Increased choline was associated with Romboutsia timonensis, Granulicatella adiacens, and Aminipila butyrica, while decreased choline was associated with Enterocloster aldensis. Anaerotignum faecicola and Bacteroides stercoris were associated with a decreased B/C ratio. Volunteers with both increased choline and a decreased B/C ratio had higher abundance of Romboutsia timonensis, Granulicatella adiacens, and Pediococcus stilesii (198). While it is exciting to see specific bacterial species identified as playing a role in TMAO biology, future RCTs that examine the effects of MedDiet on TMAO are required to draw conclusions on this topic.
5.4 Lipopolysaccharide
LPS is also called endotoxin and is derived from Gram-negative bacterial membranes. Previous studies have shown trends toward lower endotoxemia in association with Mediterranean-like diets, while Western-style diets are associated with increased endotoxemia (149, 199, 200). A mechanism by which the MedDiet may contribute to improved metabolic health is through the modulation of the gut microbiota which can lead to a reduction of metabolic endotoxemia (184). We are beginning to discover some of the detailed physiology of LPS action. LPS has also been shown to correlate with the incidence of cardiovascular events, potentially through upregulation of proprotein convertase subtilisin/kexin type 9 involved in a mechanism associated with NADPH oxidase (Nox2)-related oxidative stress (150). Yogurt supplementation, with associated probiotic bacteria, attenuated metabolic endotoxemia and inflammation in mice with obesity likely through reduced activation of the TLR4 signaling pathway (201). In mice, HFD significantly increased levels of LPS binding protein (LBP). However, depletion of the NLRP3 inflammasome using knock-out genotyping abrogated the levels of LBP, implicating the NLRP3 inflammasome as a target to mediate obesity-related inflammation (81).
The MedDiet is rich in polyphenols from various foods such as berries, spices, nuts, cocoa, wine, and olive oil, among others. Polyphenol-rich diets have beneficial effects against obesity-related dysbiosis and circulating LPS levels. For example, isoflavones showed reduced production of nitric oxide species and reduced pro-inflammatory cytokine (TNF-α and IL-6) release in response to LPS (202). In a cross-sectional study of older adults (60% with overweight or obesity) greater adherence to Mediterranean-like diets (MedDiet and prudent diet) were associated with lower circulating 3-hydroxy fatty acids levels, a proxy of LPS burden (121). As part of the Progression of Liver Disease and Cardiovascular Disorders in Non-alcoholic Fatty Liver disease (PLINIO) study, soluble Nox2-derived peptide, a marker of systemic oxidative stress, and serum LPS, were higher in patients with overweight/obesity and correlated with low adherence to MedDiet (41). In the LIBRE study, women with intestinal barrier impairment were allocated to follow MedDiet (n=124) or a control diet (n = 136) for 3 months. Adherence to MedDiet was associated with decreased LBP and gut permeability (Figure 1) (158). While circulating LPS is typically enhanced in obesity, the MedDiet may help lessen these levels and reduce inflammation.
Olive oil is a critical component of the MedDiet and it has been shown to be protective against inflammation. Virgin olive oil phenolic extract was protective against LPS treatment in murine and human brain cells by reducing activation of TLR4 and the NLRP3 signaling cascade (80). Olive oil consumption was also associated with a less significant increase in blood glucose, a more marked increase in blood insulin and GLP1, and a significant reduction in LPS and gut permeability, in individuals with impaired fasting glucose (203). These recent studies summarize the ability of the MedDiet to reduce the risk of obesity by maintaining intestinal barrier integrity and reducing the amount of circulating LPS, thus reducing the associated inflammation.
6 Conclusions and future directions
Diet has a major impact on obesity and the composition of the gut microbiota, and in turn, the types of microbial metabolites in the gut. While some studies suggest that diet may be a key component of an effective treatment for obesity and for the restoration of homeostasis (11), a recent systemic review examining the effects of the MedDiet on the gut microbiota and gut metabolites found inconsistent and few significant changes, which may be attributed to differences in methods, cohort characteristics, and study quality (86). For example, scales to evaluate the MedDiet used in the studies we reviewed range from 8- to 24-point scores, thus emphasizing different dietary components (Table 1). The populations evaluated in each study also varied, from healthy adults (65, 77, 128), to older adults (42, 121, 154), to individuals with health conditions such as ulcerative colitis (55, 63) and MASLD (41). While adherence to the MedDiet improves health and has some effect on the gut microbiome, more work needs to be done to determine the extent to which the MedDiet-associated changes in gut microbiota and their metabolites mediate these health-promoting effects. Two other critical elements important in assessing the risk of obesity are an individual's physical activity level and the total calories consumed per day, so examination of diet patterns without controlling for both exercise and caloric intake, and other potential confounders, may contribute to the conflicting results for various studies.
In a recent review, Gundogdu and Nalbantoglu note the mixed results may be due to lack of standardization, study design limitations, and differences in the defined MedDiet (204). Though widely accepted, amplicon sequencing approaches (i.e., 16S ribosomal RNA gene sequencing) lack the depth to capture most strain-specific microbes and their functionality (43, 204). Additionally, an individual's specific dietary preferences and responses, whether from a baseline diet or self-selected food during an intervention, also affect gut microbiota composition, as reviewed in Fassarella et al. (205), and further complicate adequately controlled studies. Given the number of potentially confounding variables, rigorous studies that incorporate measuring or restricting as many of these variables as possible, in addition to sequencing of the gut microbiota and measuring metabolites, are needed.
Obesity is a growing public health concern, and a better understanding of the pathophysiology related to diet and lifestyle is needed. Harnessing the ability to systematically change the gut microbiota, and correspondingly change the microbial metabolites, is a therapeutic target for investigators and clinicians. However, larger and more rigorous clinical trials are needed, in addition to animal studies that can decode the mechanisms, to define the pathophysiology of obesity. This will be challenging given the number of interacting parts between the gut microbiota, metabolites, and host immunity. While unsettling to consider the huge task of dissecting the complex biology of dietary nutrients, inflammation, and health consequences, tremendous progress has been made in the past few decades. The heterogeneity between studies is not surprising given the number of potential confounding variables; nevertheless, there is clear evidence to support the benefits of a Mediterranean-like dietary pattern as a means to alter the gut microbiota, gut metabolites, and essential biological pathways within populations with overweight/obesity.
Author contributions
MF: Writing – original draft. EA: Writing – original draft. KF: Writing – review & editing, Conceptualization. AB: Writing – review & editing, Writing – original draft, Conceptualization.
Funding
The author(s) declare that financial support was received for the research, authorship, and/or publication of this article. This work was supported by the Intramural Research Program of the National Institutes of Health Clinical Center, Bethesda, MD. Project ID: Z99 CL090080.
Acknowledgments
We would like to thank Diamond Gray for administrative assistance.
Conflict of interest
The authors declare that the research was conducted in the absence of any commercial or financial relationships that could be construed as a potential conflict of interest.
Publisher's note
All claims expressed in this article are solely those of the authors and do not necessarily represent those of their affiliated organizations, or those of the publisher, the editors and the reviewers. Any product that may be evaluated in this article, or claim that may be made by its manufacturer, is not guaranteed or endorsed by the publisher.
Author disclaimer
The conclusions of the review are those of the authors and do not represent the official positions of the National Institutes of Health or the Department of Health and Human Services.
Footnotes
1. ^World Health Organization. Available online at: https://www.who.int/news-room/fact-sheets/detail/obesity-and-overweight (accessed on January 11, 2024).
References
1. Chew NWS, Ng CH, Tan DJH, Kong G, Lin C, Chin YH, et al. The global burden of metabolic disease: Data from 2000 to 2019. Cell Metab. (2023) 35:414–28 e3. doi: 10.1016/j.cmet.2023.02.003
2. GBD 2019 Diseases and Injuries Collaborators. Global burden of 369 diseases and injuries in 204 countries and territories, 1990-2019: a systematic analysis for the Global Burden of Disease Study 2019. Lancet. (2020) 396:1204–22. doi: 10.1016/S0140-6736(20)30925-9
3. St-Onge MP, Heymsfield SB. Overweight and obesity status are linked to lower life expectancy. Nutr Rev. (2003) 61:313–6. doi: 10.1301/nr.2003.sept.313-316
4. Masood B, Moorthy M. Causes of obesity: a review. Clin Med. (2023) 23:284–91. doi: 10.7861/clinmed.2023-0168
5. Mukherjee MS, Han CY, Sukumaran S, Delaney CL, Miller MD. Effect of anti-inflammatory diets on inflammation markers in adult human populations: a systematic review of randomized controlled trials. Nutr Rev. (2022) 81:55–74. doi: 10.1093/nutrit/nuac045
6. Li Q, Wang Q, Xu W, Ma Y, Wang Q, Eatman D, et al. C-reactive protein causes adult-onset obesity through chronic inflammatory mechanism. Front Cell Dev Biol. (2020) 8:18. doi: 10.3389/fcell.2020.00018
7. Kawai T, Autieri MV, Scalia R. Adipose tissue inflammation and metabolic dysfunction in obesity. Am J Physiol Cell Physiol. (2021) 320:C375–91. doi: 10.1152/ajpcell.00379.2020
8. Calder PC, Ahluwalia N, Brouns F, Buetler T, Clement K, Cunningham K, et al. Dietary factors and low-grade inflammation in relation to overweight and obesity. Br J Nutr. (2011) 106 Suppl 3:S5–78. doi: 10.1017/S0007114511005460
9. Wang L, Gao T, Li Y, Xie Y, Zeng S, Tai C, et al. A long-term anti-inflammation markedly alleviated high-fat diet-induced obesity by repeated administrations of overexpressing IL10 human umbilical cord-derived mesenchymal stromal cells. Stem Cell Res Ther. (2022) 13:259. doi: 10.1186/s13287-022-02935-8
10. Muscogiuri G, Verde L, Sulu C, Katsiki N, Hassapidou M, Frias-Toral E, et al. Mediterranean diet and obesity-related disorders: what is the evidence? Curr Obes Rep. (2022) 11:287–304. doi: 10.1007/s13679-022-00481-1
11. Lange O, Proczko-Stepaniak M, Mika A. Short-chain fatty acids-A product of the microbiome and its participation in two-way communication on the microbiome-host mammal line. Curr Obes Rep. (2023) 12:108–26. doi: 10.1007/s13679-023-00503-6
12. You H, Tan Y, Yu D, Qiu S, Bai Y, He J, et al. The therapeutic effect of SCFA-mediated regulation of the intestinal environment on obesity. Front Nutr. (2022) 9:886902. doi: 10.3389/fnut.2022.886902
13. Agus A, Clement K, Sokol H. Gut microbiota-derived metabolites as central regulators in metabolic disorders. Gut. (2021) 70:1174–82. doi: 10.1136/gutjnl-2020-323071
14. Sipe LM, Chaib M, Pingili AK, Pierre JF, Makowski L. Microbiome, bile acids, and obesity: How microbially modified metabolites shape anti-tumor immunity. Immunol Rev. (2020) 295:220–39. doi: 10.1111/imr.12856
15. Grosso G, Laudisio D, Frias-Toral E, Barrea L, Muscogiuri G, Savastano S, Colao A. Anti-Inflammatory Nutrients And Obesity-Associated Metabolic-Inflammation: State Of The Art And Future Direction. Nutrients. (2022) 14:1137. doi: 10.3390/nu14061137
16. Lecube A, Lopez-Cano C. Obesity, a diet-induced inflammatory disease. Nutrients. (2019) 11:2284. doi: 10.3390/nu11102284
17. Calabrese FM, Porrelli A, Vacca M, Comte B, Nimptsch K, Pinart M, et al. Metaproteomics approach and pathway modulation in obesity and diabetes: a narrative review. Nutrients. (2021) 14:47. doi: 10.3390/nu14010047
18. Newman TM, Shively CA, Register TC, Appt SE, Yadav H, Colwell RR, et al. Diet, obesity, and the gut microbiome as determinants modulating metabolic outcomes in a non-human primate model. Microbiome. (2021) 9:100. doi: 10.1186/s40168-021-01069-y
19. Rogero MM, Calder PC. Obesity, inflammation, toll-like receptor 4 and fatty acids. Nutrients. (2018) 10:432. doi: 10.3390/nu10040432
20. Zhao Y, Lin J, Fan Y, Lin X. Life cycle of Cryptococcus neoformans. Annu Rev Microbiol. (2019) 73:17–42. doi: 10.1146/annurev-micro-020518-120210
21. Shivappa N, Steck SE, Hurley TG, Hussey JR, Hebert JR. Designing and developing a literature-derived, population-based dietary inflammatory index. Public Health Nutr. (2014) 17:1689–96. doi: 10.1017/S1368980013002115
22. Hodge AM, Karim MN, Hebert JR, Shivappa N, Milne RL, de Courten B. Diet scores and prediction of general and abdominal obesity in the Melbourne collaborative cohort study. Public Health Nutr. (2021) 24:6157–68. doi: 10.1017/S1368980021001713
23. May KS, den Hartigh LJ. Gut microbial-derived short chain fatty acids: impact on adipose tissue physiology. Nutrients. (2023) 15:272. doi: 10.3390/nu15020272
24. Las Heras V, Melgar S, MacSharry J, Gahan CGM. The influence of the Western diet on microbiota and gastrointestinal immunity. Annu Rev Food Sci Technol. (2022) 13:489–512. doi: 10.1146/annurev-food-052720-011032
25. Khan S, Luck H, Winer S, Winer DA. Emerging concepts in intestinal immune control of obesity-related metabolic disease. Nat Commun. (2021) 12:2598. doi: 10.1038/s41467-021-22727-7
26. Guasch-Ferre M, Willett WC. The Mediterranean diet and health: a comprehensive overview. J Intern Med. (2021) 290:549–66. doi: 10.1111/joim.13333
27. Willett WC, Sacks F, Trichopoulou A, Drescher G, Ferro-Luzzi A, Helsing E, et al. Mediterranean diet pyramid: a cultural model for healthy eating. Am J Clin Nutr. (1995) 61:1402S−6S. doi: 10.1093/ajcn/61.6.1402S
28. United States Department of Agriculture. Available online at: https://www.dietaryguidelines.gov/resources/2020-2025-dietary-guidelines-online-materials (accessed August 1, 2024).
29. Davis C, Bryan J, Hodgson J, Murphy K. Definition of the Mediterranean diet; a literature review. Nutrients. (2015) 7:9139–53. doi: 10.3390/nu7115459
30. Itsiopoulos C, Mayr HL, Thomas CJ. The anti-inflammatory effects of a Mediterranean diet: a review. Curr Opin Clin Nutr Metab Care. (2022) 25:415–22. doi: 10.1097/MCO.0000000000000872
31. Zupo R, Castellana F, Piscitelli P, Crupi P, Desantis A, Greco E, et al. Scientific evidence supporting the newly developed one-health labeling tool “Med-Index”: an umbrella systematic review on health benefits of mediterranean diet principles and adherence in a planeterranean perspective. J Transl Med. (2023) 21:755. doi: 10.1186/s12967-023-04618-1
32. Mambrini SP, Menichetti F, Ravella S, Pellizzari M, De Amicis R, Foppiani A, et al. Ultra-processed food consumption and incidence of obesity and cardiometabolic risk factors in adults: a systematic review of prospective studies. Nutrients. (2023) 15:25883. doi: 10.3390/nu15112583
33. Schlesinger S, Neuenschwander M, Schwedhelm C, Hoffmann G, Bechthold A, Boeing H, et al. Food groups and risk of overweight, obesity, and weight gain: a systematic review and dose-response meta-analysis of prospective studies. Adv Nutr. (2019) 10:205–18. doi: 10.1093/advances/nmy092
34. Pagliai G, Dinu M, Madarena MP, Bonaccio M, Iacoviello L, Sofi F. Consumption of ultra-processed foods and health status: a systematic review and meta-analysis. Br J Nutr. (2021) 125:308–18. doi: 10.1017/S0007114520002688
35. Deledda A, Palmas V, Heidrich V, Fosci M, Lombardo M, Cambarau G, et al. Dynamics of gut microbiota and clinical variables after ketogenic and Mediterranean diets in drug-naive patients with type 2 diabetes mellitus and obesity. Metabolites. (2022) 12:1092. doi: 10.3390/metabo12111092
36. Di Rosa C, Lattanzi G, Spiezia C, Imperia E, Piccirilli S, Beato I, et al. Mediterranean diet versus very low-calorie ketogenic diet: effects of reaching 5% body weight loss on body composition in subjects with overweight and with obesity-a cohort study. Int J Environ Res Public Health. (2022) 19:13040. doi: 10.3390/ijerph192013040
37. Gioxari A, Grammatikopoulou MG, Katsarou C, Panagiotakos DB, Toutouza M, Kavouras SA, et al. A modified Mediterranean diet improves fasting and postprandial glucoregulation in adults with overweight and obesity: a pilot study. Int J Environ Res Public Health. (2022) 19:215347. doi: 10.3390/ijerph192215347
38. Jospe MR, Roy M, Brown RC, Haszard JJ, Meredith-Jones K, Fangupo LJ, et al. Intermittent fasting, Paleolithic, or Mediterranean diets in the real world: exploratory secondary analyses of a weight-loss trial that included choice of diet and exercise. Am J Clin Nutr. (2020) 111:503–14. doi: 10.1093/ajcn/nqz330
39. Hodge AM, Karim MN, Hébert JR, Shivappa N, de Courten B. Association between diet quality indices and incidence of type 2 diabetes in the Melbourne collaborative cohort study. Nutrients. (2021) 13:114162. doi: 10.3390/nu13114162
40. Hoofnagle JH, Doo E. Letter to the editor: a multi-society Delphi consensus statement on new fatty liver disease nomenclature. Hepatology. (2024) 79:E91–E2. doi: 10.1097/HEP.0000000000000695
41. Baratta F, Pastori D, Bartimoccia S, Cammisotto V, Cocomello N, Colantoni A, et al. Poor adherence to Mediterranean diet and serum lipopolysaccharide are associated with oxidative stress in patients with non-alcoholic fatty liver disease. Nutrients. (2020) 12:1732. doi: 10.3390/nu12061732
42. Ghosh TS, Rampelli S, Jeffery IB, Santoro A, Neto M, Capri M, et al. Mediterranean diet intervention alters the gut microbiome in older people reducing frailty and improving health status: the NU-AGE 1-year dietary intervention across five European countries. Gut. (2020) 69:1218–28. doi: 10.1136/gutjnl-2019-319654
43. Wang DD, Nguyen LH Li Y, Yan Y, Ma W, Rinott E, et al. The gut microbiome modulates the protective association between a Mediterranean diet and cardiometabolic disease risk. Nat Med. (2021) 27:333–43. doi: 10.1038/s41591-020-01223-3
44. Magriplis E, Panagiotakos D, Kyrou I, Tsioufis C, Mitsopoulou AV, Karageorgou D, et al. Presence of hypertension is reduced by Mediterranean diet adherence in all individuals with a more pronounced effect in the obese: The Hellenic National Nutrition and Health Survey (HNNHS). Nutrients. (2020) 12:853. doi: 10.3390/nu12030853
45. Sood S, Feehan J, Itsiopoulos C, Wilson K, Plebanski M, Scott D, et al. Higher adherence to a mediterranean diet is associated with improved insulin sensitivity and selected markers of inflammation in individuals who are overweight and obese without diabetes. Nutrients. (2022) 14:4437. doi: 10.3390/nu14204437
46. Leone A, Bertoli S, Bedogni G, Vignati L, Pellizzari M, Battezzati A. Association between Mediterranean diet and fatty liver in women with overweight and obesity. Nutrients. (2022) 14:3771. doi: 10.3390/nu14183771
47. Golzarand M, Moslehi N, Mirmiran P, Azizi F. Adherence to the DASH, MeDi, and MIND diet scores and the incidence of metabolically unhealthy phenotypes. Obes Res Clin Pract. (2023) 17:226–32. doi: 10.1016/j.orcp.2023.04.001
48. Barrea L, Muscogiuri G, Pugliese G, de Alteriis G, Colao A, Savastano S. Metabolically Healthy Obesity (MHO) vs. Metabolically Unhealthy Obesity (MUO) phenotypes in PCOS: association with endocrine-metabolic profile, adherence to the Mediterranean diet, and body composition. Nutrients. (2021) 13:3925. doi: 10.3390/nu13113925
49. Taskinen RE, Hantunen S, Tuomainen TP, Virtanen JK. The associations between whole grain and refined grain intakes and serum C-reactive protein. Eur J Clin Nutr. (2022) 76:544–50. doi: 10.1038/s41430-021-00996-1
50. Chai W, Morimoto Y, Cooney RV, Franke AA, Shvetsov YB, Le Marchand L, et al. Dietary red and processed meat intake and markers of adiposity and inflammation: the Multiethnic Cohort Study. J Am Coll Nutr. (2017) 36:378–85. doi: 10.1080/07315724.2017.1318317
51. Mazidi M, Kengne AP, George ES, Siervo M. The association of red meat intake with inflammation and circulating intermediate biomarkers of type 2 diabetes is mediated by central adiposity. Br J Nutr. (2021) 125:1043–50. doi: 10.1017/S0007114519002149
52. Montonen J, Boeing H, Fritsche A, Schleicher E, Joost HG, Schulze MB, et al. Consumption of red meat and whole-grain bread in relation to biomarkers of obesity, inflammation, glucose metabolism and oxidative stress. Eur J Nutr. (2013) 52:337–45. doi: 10.1007/s00394-012-0340-6
53. Kolehmainen M, Ulven SM, Paananen J, de Mello V, Schwab U, Carlberg C, et al. Healthy Nordic diet downregulates the expression of genes involved in inflammation in subcutaneous adipose tissue in individuals with features of the metabolic syndrome. Am J Clin Nutr. (2015) 101:228–39. doi: 10.3945/ajcn.114.092783
54. Tagliamonte S, Laiola M, Ferracane R, Vitale M, Gallo MA, Meslier V, et al. Mediterranean diet consumption affects the endocannabinoid system in overweight and obese subjects: possible links with gut microbiome, insulin resistance and inflammation. Eur J Nutr. (2021) 60:3703–16. doi: 10.1007/s00394-021-02538-8
55. Haskey N, Estaki M, Ye J, Shim RK, Singh S, Dieleman LA, et al. A Mediterranean diet pattern improves intestinal inflammation concomitant with reshaping of the bacteriome in ulcerative colitis: a randomized controlled trial. J Crohns Colitis. (2023). doi: 10.1093/ecco-jcc/jjad073
56. Konieczna J, Romaguera D, Pereira V, Fiol M, Razquin C, Estruch R, et al. Longitudinal association of changes in diet with changes in body weight and waist circumference in subjects at high cardiovascular risk: the PREDIMED trial. Int J Behav Nutr Phys Act. (2019) 16:139. doi: 10.1186/s12966-019-0893-3
57. Muralidharan J, Moreno-Indias I, Bullo M, Lopez JV, Corella D, Castaner O, et al. Effect on gut microbiota of a 1-y lifestyle intervention with Mediterranean diet compared with energy-reduced Mediterranean diet and physical activity promotion: PREDIMED-Plus Study. Am J Clin Nutr. (2021) 114:1148–58. doi: 10.1093/ajcn/nqab150
58. Marcos-Pardo PJ, Gonzalez-Galvez N, Espeso-Garcia A, Abelleira-Lamela T, Lopez-Vivancos A, Vaquero-Cristobal R. Association among adherence to the Mediterranean diet, cardiorespiratory fitness, cardiovascular, obesity, and anthropometric variables of overweight and obese middle-aged and older adults. Nutrients. (2020) 12:2750. doi: 10.3390/nu12092750
59. Hosseini-Esfahani F, Koochakpoor G, Daneshpour MS, Sedaghati-khayat B, Mirmiran P, Azizi F. Mediterranean dietary pattern adherence modify the association between FTO genetic variations and obesity phenotypes. Nutrients. (2017) 9:1064. doi: 10.3390/nu9101064
60. Urpi-Sarda M, Casas R, Sacanella E, Corella D, Andres-Lacueva C, Llorach R, et al. The 3-year effect of the Mediterranean diet intervention on inflammatory biomarkers related to cardiovascular disease. Biomedicines. (2021) 9:862. doi: 10.3390/biomedicines9080862
61. Casas R, Sacanella E, Urpi-Sarda M, Corella D, Castaner O, Lamuela-Raventos RM, et al. Long-term immunomodulatory effects of a Mediterranean diet in adults at high risk of cardiovascular disease in the PREvencion con DIeta MEDiterranea (PREDIMED) randomized controlled trial. J Nutr. (2016) 146:1684–93. doi: 10.3945/jn.115.229476
62. Konstantinidou V, Covas MI, Munoz-Aguayo D, Khymenets O, de la Torre R, Saez G, et al. In vivo nutrigenomic effects of virgin olive oil polyphenols within the frame of the Mediterranean diet: a randomized controlled trial. FASEB J. (2010) 24:2546–57. doi: 10.1096/fj.09-148452
63. Strauss JC, Haskey N, Ramay HR, Ghosh TS, Taylor LM, Yousuf M, et al. Weighted gene co-expression network analysis identifies a functional guild and metabolite cluster mediating the relationship between mucosal inflammation and adherence to the Mediterranean diet in ulcerative colitis. Int J Mol Sci. (2023) 24:7323. doi: 10.3390/ijms24087323
64. Camilleri M, Zheng T. Cannabinoids and the gastrointestinal tract. Clin Gastroenterol Hepatol. (2023) 21:3217–29. doi: 10.1016/j.cgh.2023.07.031
65. Bourdeau-Julien I, Castonguay-Paradis S, Rochefort G, Perron J, Lamarche B, Flamand N, et al. The diet rapidly and differentially affects the gut microbiota and host lipid mediators in a healthy population. Microbiome. (2023) 11:26. doi: 10.1186/s40168-023-01469-2
66. Forteza F, Bourdeau-Julien I, Nguyen GQ, Guevara Agudelo FA, Rochefort G, Parent L, et al. Influence of diet on acute endocannabinoidome mediator levels post exercise in active women, a crossover randomized study. Sci Rep. (2022) 12:8568. doi: 10.1038/s41598-022-10757-0
67. Sanchez-Rodriguez E, Biel-Glesson S, Fernandez-Navarro JR, Calleja MA, Espejo-Calvo JA, Gil-Extremera B, et al. Effects of virgin olive oils differing in their bioactive compound contents on biomarkers of oxidative stress and inflammation in healthy adults: a randomized double-blind controlled trial. Nutrients. (2019) 11:561. doi: 10.3390/nu11030561
68. Patti AM, Carruba G, Cicero AFG, Banach M, Nikolic D, Giglio RV, et al. Daily use of extra virgin olive oil with high oleocanthal concentration reduced body weight, waist circumference, alanine transaminase, inflammatory cytokines and hepatic steatosis in subjects with the metabolic syndrome: a 2-month intervention study. Metabolites. (2020) 10:392. doi: 10.3390/metabo10100392
69. Predimed: Prevencion con Dieta Mediterranea. Predimed: 2018 [Supplement]. Available online at: http://www.predimed.es (accessed May 7, 2024).
70. Lopez-Gil JF, Garcia-Hermoso A, Sotos-Prieto M, Cavero-Redondo I, Martinez-Vizcaino V, Kales SN. Mediterranean diet-based interventions to improve anthropometric and obesity indicators in children and adolescents: a systematic review with meta-analysis of randomized controlled trials. Adv Nutr. (2023) 14:858–69. doi: 10.1016/j.advnut.2023.04.011
71. Sanchez-Rosales AI, Guadarrama-Lopez AL, Gaona-Valle LS, Martinez-Carrillo BE, Valdes-Ramos R. The effect of dietary patterns on inflammatory biomarkers in adults with type 2 diabetes mellitus: a systematic review and meta-analysis of randomized controlled trials. Nutrients. (2022) 14:4577. doi: 10.3390/nu14214577
72. Kavyani Z, Musazadeh V, Fathi S, Hossein Faghfouri A, Dehghan P, Sarmadi B. Efficacy of the omega-3 fatty acids supplementation on inflammatory biomarkers: an umbrella meta-analysis. Int Immunopharmacol. (2022) 111:109104. doi: 10.1016/j.intimp.2022.109104
73. Mirabelli M, Chiefari E, Arcidiacono B, Corigliano DM, Brunetti FS, Maggisano V, et al. Mediterranean diet nutrients to turn the tide against insulin resistance and related diseases. Nutrients. (2020) 12:66. doi: 10.3390/nu12041066
74. Moszak M, Szulinska M, Bogdanski P. You are what you eat-the relationship between diet, microbiota, and metabolic disorders-a review. Nutrients. (2020) 12:96. doi: 10.3390/nu12041096
75. Sanna S, van Zuydam NR, Mahajan A, Kurilshikov A, Vich Vila A, Vosa U, et al. Causal relationships among the gut microbiome, short-chain fatty acids and metabolic diseases. Nat Genet. (2019) 51:600–5. doi: 10.1038/s41588-019-0350-x
76. Antoniazzi L, Arroyo-Olivares R, Bittencourt MS, Tada MT, Lima I, Jannes CE, et al. Adherence to a Mediterranean diet, dyslipidemia and inflammation in familial hypercholesterolemia. Nutr Metab Cardiovasc Dis. (2021) 31:2014–22. doi: 10.1016/j.numecd.2021.04.006
77. Barrea L, Arnone A, Annunziata G, Muscogiuri G, Laudisio D, Salzano C, et al. Adherence to the Mediterranean diet, dietary patterns and body composition in women with Polycystic Ovary Syndrome (PCOS). Nutrients. (2019) 11:2278. doi: 10.3390/nu11102278
78. Magno MS, Moschowits E, Morthen MK, Beining MW, Jansonius NM, Hammond CJ, et al. Greater adherence to a mediterranean diet is associated with lower C-reactive protein (CRP) levels, but not to lower odds of having dry eye disease. Ocul Surf. (2023) 30:196–203. doi: 10.1016/j.jtos.2023.09.013
79. Roncero-Ramos I, Rangel-Zuniga OA, Lopez-Moreno J, Alcala-Diaz JF, Perez-Martinez P, Jimenez-Lucena R, et al. Mediterranean diet, glucose homeostasis, and inflammasome genetic variants: The CORDIOPREV Study. Mol Nutr Food Res. (2018) 62:e1700960. doi: 10.1002/mnfr.201700960
80. Taticchi A, Urbani S, Albi E, Servili M, Codini M, Traina G, et al. In vitro anti-inflammatory effects of phenolic compounds from Moraiolo Virgin Olive Oil (MVOO) in brain cells via regulating the TLR4/NLRP3 axis. Molecules. (2019) 24:244523. doi: 10.3390/molecules24244523
81. Sokolova M, Yang K, Hansen SH, Louwe MC, Kummen M, Hov JER, et al. NLRP3 inflammasome deficiency attenuates metabolic disturbances involving alterations in the gut microbial profile in mice exposed to high fat diet. Sci Rep. (2020) 10:21006. doi: 10.1038/s41598-020-76497-1
82. Demaria TM, Crepaldi LD, Costa-Bartuli E, Branco JR, Zancan P, Sola-Penna M. Once a week consumption of Western diet over twelve weeks promotes sustained insulin resistance and non-alcoholic fat liver disease in C57BL/6 J mice. Sci Rep. (2023) 13:3058. doi: 10.1038/s41598-023-30254-2
83. Beam A, Clinger E, Hao L. Effect of diet and dietary components on the composition of the gut microbiota. Nutrients. (2021) 13:2795. doi: 10.3390/nu13082795
84. David LA, Maurice CF, Carmody RN, Gootenberg DB, Button JE, Wolfe BE, et al. Diet rapidly and reproducibly alters the human gut microbiome. Nature. (2014) 505:559–63. doi: 10.1038/nature12820
85. Klimenko NS, Tyakht AV, Popenko AS, Vasiliev AS, Altukhov IA, Ischenko DS, et al. Microbiome responses to an uncontrolled short-term diet intervention in the frame of the citizen science project. Nutrients. (2018) 10:576. doi: 10.3390/nu10050576
86. Kimble R, Gouinguenet P, Ashor A, Stewart C, Deighton K, Matu J, et al. Effects of a mediterranean diet on the gut microbiota and microbial metabolites: a systematic review of randomized controlled trials and observational studies. Crit Rev Food Sci Nutr. (2022) 63:1–22. doi: 10.1080/10408398.2022.2057416
87. Barber C, Mego M, Sabater C, Vallejo F, Bendezu RA, Masihy M, et al. Differential effects of Western and Mediterranean-type diets on gut microbiota: a metagenomics and metabolomics approach. Nutrients. (2021) 13:2638. doi: 10.3390/nu13082638
88. Cancello R, Turroni S, Rampelli S, Cattaldo S, Candela M, Cattani L, et al. Effect of short-term dietary intervention and probiotic mix supplementation on the gut microbiota of elderly obese women. Nutrients. (2019) 11:3011. doi: 10.3390/nu11123011
89. Roses C, Cuevas-Sierra A, Quintana S, Riezu-Boj JI, Martinez JA, Milagro FI, Barcelo A. Gut microbiota bacterial species associated with mediterranean diet-related food groups in a Northern Spanish Population. Nutrients. (2021) 13:636. doi: 10.3390/nu13020636
90. Pagliai G, Russo E, Niccolai E, Dinu M, Di Pilato V, Magrini A, et al. Influence of a 3-month low-calorie Mediterranean diet compared to the vegetarian diet on human gut microbiota and SCFA: the CARDIVEG Study. Eur J Nutr. (2020) 59:2011–24. doi: 10.1007/s00394-019-02050-0
91. Meslier V, Laiola M, Roager HM, De Filippis F, Roume H, Quinquis B, et al. Mediterranean diet intervention in overweight and obese subjects lowers plasma cholesterol and causes changes in the gut microbiome and metabolome independently of energy intake. Gut. (2020) 69:1258–68. doi: 10.1136/gutjnl-2019-320438
92. Baxter NT, Schmidt AW, Venkataraman A, Kim KS, Waldron C, Schmidt TM. Dynamics of human gut microbiota and short-chain fatty acids in response to dietary interventions with three fermentable fibers. mBio. (2019) 10:e02566-18. doi: 10.1128/mBio.02566-18
93. Holmes ZC, Villa MM, Durand HK, Jiang S, Dallow EP, Petrone BL, et al. Microbiota responses to different prebiotics are conserved within individuals and associated with habitual fiber intake. Microbiome. (2022) 10:114. doi: 10.1186/s40168-022-01307-x
94. Oliver A, Chase AB, Weihe C, Orchanian SB, Riedel SF, Hendrickson CL, et al. High-fiber, whole-food dietary intervention alters the human gut microbiome but not fecal short-chain fatty acids. mSystems. (2021) 6:e00115-21. doi: 10.1128/mSystems.00115-21
95. Rodriguez-Garcia C, Sanchez-Quesada C, Algarra I, Gaforio JJ. The high-fat diet based on extra-virgin olive oil causes dysbiosis linked to colorectal cancer prevention. Nutrients. (2020) 12:1705. doi: 10.3390/nu12061705
96. Martin-Pelaez S, Mosele JI, Pizarro N, Farras M, de la Torre R, Subirana I, et al. Effect of virgin olive oil and thyme phenolic compounds on blood lipid profile: implications of human gut microbiota. Eur J Nutr. (2017) 56:119–31. doi: 10.1007/s00394-015-1063-2
97. Osborn LJ, Schultz K, Massey W, DeLucia B, Choucair I, Varadharajan V, et al. A gut microbial metabolite of dietary polyphenols reverses obesity-driven hepatic steatosis. Proc Natl Acad Sci USA. (2022) 119:e2202934119. doi: 10.1073/pnas.2202934119
98. Palmas V, Pisanu S, Madau V, Casula E, Deledda A, Cusano R, et al. Gut microbiota markers associated with obesity and overweight in Italian adults. Sci Rep. (2021) 11:5532. doi: 10.1038/s41598-021-84928-w
99. Di Rosa C, Di Francesco L, Spiezia C, Khazrai YM. Effects of animal and vegetable proteins on gut microbiota in subjects with overweight or obesity. Nutrients. (2023) 15:2675. doi: 10.3390/nu15122675
100. Pessoa J, Belew GD, Barroso C, Egas C, Jones JG. The gut microbiome responds progressively to fat and/or sugar-rich diets and is differentially modified by dietary fat and sugar. Nutrients. (2023) 15:2097. doi: 10.3390/nu15092097
101. Ridaura VK, Faith JJ, Rey FE, Cheng J, Duncan AE, Kau AL, et al. Gut microbiota from twins discordant for obesity modulate metabolism in mice. Science. (2013) 341:1241214. doi: 10.1126/science.1241214
102. Turnbaugh PJ, Ley RE, Mahowald MA, Magrini V, Mardis ER, Gordon JI. An obesity-associated gut microbiome with increased capacity for energy harvest. Nature. (2006) 444:1027–31. doi: 10.1038/nature05414
103. Vrieze A, Van Nood E, Holleman F, Salojarvi J, Kootte RS, Bartelsman JF, et al. Transfer of intestinal microbiota from lean donors increases insulin sensitivity in individuals with metabolic syndrome. Gastroenterology. (2012) 143:913–6 e7. doi: 10.1053/j.gastro.2012.06.031
104. Allegretti JR, Kassam Z, Mullish BH, Chiang A, Carrellas M, Hurtado J, et al. Effects of fecal microbiota transplantation with oral capsules in obese patients. Clin Gastroenterol Hepatol. (2020) 18:855–63 e2. doi: 10.1016/j.cgh.2019.07.006
105. Bombin A, Yan S, Bombin S, Mosley JD, Ferguson JF. Obesity influences composition of salivary and fecal microbiota and impacts the interactions between bacterial taxa. Physiol Rep. (2022) 10:e15254. doi: 10.14814/phy2.15254
106. Mishra SP, Wang B, Jain S, Ding J, Rejeski J, Furdui CM, et al. A mechanism by which gut microbiota elevates permeability and inflammation in obese/diabetic mice and human gut. Gut. (2023) 72:1848–65. doi: 10.1136/gutjnl-2022-327365
107. Hersoug LG, Moller P, Loft S. Role of microbiota-derived lipopolysaccharide in adipose tissue inflammation, adipocyte size and pyroptosis during obesity. Nutr Res Rev. (2018) 31:153–63. doi: 10.1017/S0954422417000269
108. Krueger ES, Lloyd TS, Tessem JS. The accumulation and molecular effects of trimethylamine N-oxide on metabolic tissues: it's not all bad. Nutrients. (2021) 13:2873. doi: 10.3390/nu13082873
109. Schugar RC, Shih DM, Warrier M, Helsley RN, Burrows A, Ferguson D, et al. The TMAO-producing enzyme flavin-containing monooxygenase 3 regulates obesity and the Beiging of white adipose tissue. Cell Rep. (2017) 19:2451–61. doi: 10.1016/j.celrep.2017.06.053
110. Genser L, Aguanno D, Soula HA, Dong L, Trystram L, Assmann K, et al. Increased jejunal permeability in human obesity is revealed by a lipid challenge and is linked to inflammation and type 2 diabetes. J Pathol. (2018) 246:217–30. doi: 10.1002/path.5134
111. Ecklu-Mensah G, Choo-Kang C, Maseng MG, Donato S, Bovet P, Viswanathan B, et al. Gut microbiota and fecal short chain fatty acids differ with adiposity and country of origin: the METS-microbiome study. Nat Commun. (2023) 14:5160. doi: 10.1038/s41467-023-40874-x
112. Ilyes T, Silaghi CN, Craciun AM. Diet-related changes of short-chain fatty acids in blood and feces in obesity and metabolic syndrome. Biology. (2022) 11:1556. doi: 10.3390/biology11111556
113. Overby HB, Ferguson JF. Gut microbiota-derived short-chain fatty acids facilitate microbiota:host cross talk and modulate obesity and hypertension. Curr Hypertens Rep. (2021) 23:8. doi: 10.1007/s11906-020-01125-2
114. Yamamura R, Nakamura K, Ukawa S, Okada E, Nakagawa T, Imae A, et al. Fecal short-chain fatty acids and obesity in a community-based Japanese population: The DOSANCO Health Study. Obes Res Clin Pract. (2021) 15:345–50. doi: 10.1016/j.orcp.2021.06.003
115. Chambers ES, Byrne CS, Morrison DJ, Murphy KG, Preston T, Tedford C, et al. Dietary supplementation with inulin-propionate ester or inulin improves insulin sensitivity in adults with overweight and obesity with distinct effects on the gut microbiota, plasma metabolome and systemic inflammatory responses: a randomised cross-over trial. Gut. (2019) 68:1430–8. doi: 10.1136/gutjnl-2019-318424
116. Igudesman D, Crandell JL, Corbin KD, Hooper J, Thomas JM, Bulik CM, et al. Associations of dietary intake with the intestinal microbiota and short-chain fatty acids among young adults with type 1 diabetes and overweight or obesity. J Nutr. (2023) 153:1178–88. doi: 10.1016/j.tjnut.2022.12.017
117. Jamar G, Santamarina AB, Casagrande BP, Estadella D, de Rosso VV, Wagner R, et al. Prebiotic potencial of jucara berry on changes in gut bacteria and acetate of individuals with obesity. Eur J Nutr. (2020) 59:3767–78. doi: 10.1007/s00394-020-02208-1
118. Gyarmati P, Song Y, Dotimas J, Yoshiba G, Christison A. Cross-sectional comparisons of gut microbiome and short-chain fatty acid levels among children with varied weight classifications. Pediatr Obes. (2021) 16:e12750. doi: 10.1111/ijpo.12750
119. Wei Y, Liang J, Su Y, Wang J, Amakye WK, Pan J, et al. The associations of the gut microbiome composition and short-chain fatty acid concentrations with body fat distribution in children. Clin Nutr. (2021) 40:3379–90. doi: 10.1016/j.clnu.2020.11.014
120. Slizewska K, Wlodarczyk M, Sobczak M, Barczynska R, Kapusniak J, Socha P, et al. Comparison of the activity of fecal enzymes and concentration of SCFA in healthy and overweight children. Nutrients. (2023) 15:987. doi: 10.3390/nu15040987
121. Andre P, Pais de Barros JP, Mj Merle B, Samieri C, Helmer C, Delcourt C, et al. Mediterranean diet and prudent diet are both associated with low circulating esterified 3-hydroxy fatty acids, a proxy of LPS burden, among older adults. Am J Clin Nutr. (2021) 114:1080–91. doi: 10.1093/ajcn/nqab126
122. Martinez-Gonzalez MA, Fernandez-Jarne E, Serrano-Martinez M, Wright M, Gomez-Gracia E. Development of a short dietary intake questionnaire for the quantitative estimation of adherence to a cardioprotective Mediterranean diet. Eur J Clin Nutr. (2004) 58:1550–2. doi: 10.1038/sj.ejcn.1602004
123. Barrea L, Annunziata G, Muscogiuri G, Laudisio D, Di Somma C, Maisto M, et al. Trimethylamine N-oxide, Mediterranean diet, and nutrition in healthy, normal-weight adults: also a matter of sex? Nutrition. (2019) 62:7–17. doi: 10.1016/j.nut.2018.11.015
124. Martinez-Gonzalez MA, Corella D, Salas-Salvado J, Ros E, Covas MI, Fiol M, et al. Cohort profile: design and methods of the PREDIMED study. Int J Epidemiol. (2012) 41:377–85. doi: 10.1093/ije/dyq250
125. Barrea L, Muscogiuri G, Pugliese G, Graziadio C, Maisto M, Pivari F, et al. Association of the chronotype score with circulating trimethylamine N-oxide (TMAO) concentrations. Nutrients. (2021) 13:1671. doi: 10.3390/nu13051671
126. Martinez-Gonzalez MA, Garcia-Arellano A, Toledo E, Salas-Salvado J, Buil-Cosiales P, Corella D, et al. A 14-item mediterranean diet assessment tool and obesity indexes among high-risk subjects: the PREDIMED trial. PLoS ONE. (2012) 7:e0043134. doi: 10.1371/journal.pone.0043134
127. Barrea L, Muscogiuri G, Pugliese G, de Alteriis G, Maisto M, Donnarumma M, et al. Association of trimethylamine N-Oxide (TMAO) with the clinical severity of hidradenitis suppurativa (Acne Inversa). Nutrients. (2021) 13:1997. doi: 10.3390/nu13061997
128. De Filippis F, Pellegrini N, Vannini L, Jeffery IB, La Storia A, Laghi L, et al. High-level adherence to a Mediterranean diet beneficially impacts the gut microbiota and associated metabolome. Gut. (2016) 65:1812–21. doi: 10.1136/gutjnl-2015-309957
129. Agnoli C, Krogh V, Grioni S, Sieri S, Palli D, Masala G, et al. A priori-defined dietary patterns are associated with reduced risk of stroke in a large Italian cohort. J Nutr. (2011) 141:1552–8. doi: 10.3945/jn.111.140061
130. Galie S, Garcia-Gavilan J, Papandreou C, Camacho-Barcia L, Arcelin P, Palau-Galindo A, et al. Effects of Mediterranean Diet on plasma metabolites and their relationship with insulin resistance and gut microbiota composition in a crossover randomized clinical trial. Clin Nutr. (2021) 40:3798–806. doi: 10.1016/j.clnu.2021.04.028
131. Martinez-Gonzalez MA, Buil-Cosiales P, Corella D, Bullo M, Fito M, Vioque J, et al. Cohort profile: design and methods of the PREDIMED-Plus randomized trial. Int J Epidemiol. (2019) 48:387–8o. doi: 10.1093/ije/dyy225
132. Garcia-Mantrana I, Selma-Royo M, Alcantara C, Collado MC. Shifts on gut microbiota associated to mediterranean diet adherence and specific dietary intakes on general adult population. Front Microbiol. (2018) 9:890. doi: 10.3389/fmicb.2018.00890
133. Berendsen AAM, van de Rest O, Feskens EJM, Santoro A, Ostan R, Pietruszka B, et al. Changes in dietary intake and adherence to the NU-AGE diet following a one-year dietary intervention among european older adults-results of the NU-AGE randomized trial. Nutrients. (2018) 10:1905. doi: 10.3390/nu10121905
134. Griffin LE, Djuric Z, Angiletta CJ, Mitchell CM, Baugh ME, Davy KP, et al. Mediterranean diet does not alter plasma trimethylamine N-oxide concentrations in healthy adults at risk for colon cancer. Food Funct. (2019) 10:2138–47. doi: 10.1039/C9FO00333A
135. Sidahmed E, Cornellier ML, Ren J, Askew LM Li Y, Talaat N, et al. Development of exchange lists for Mediterranean and Healthy Eating diets: implementation in an intervention trial. J Hum Nutr Diet. (2014) 27:413–25. doi: 10.1111/jhn.12158
136. Guasch-Ferre M, Hu FB, Ruiz-Canela M, Bullo M, Toledo E, Wang DD, et al. Plasma metabolites from choline pathway and risk of cardiovascular disease in the PREDIMED (prevention with mediterranean diet) Study. J Am Heart Assoc. (2017) 6:6524. doi: 10.1161/JAHA.117.006524
137. Gutierrez-Diaz I, Fernandez-Navarro T, Sanchez B, Margolles A, Gonzalez S. Mediterranean diet and faecal microbiota: a transversal study. Food Funct. (2016) 7:2347–56. doi: 10.1039/C6FO00105J
138. Gonzalez S, Fernandez M, Cuervo A, Lasheras C. Dietary intake of polyphenols and major food sources in an institutionalised elderly population. J Hum Nutr Diet. (2014) 27:176–83. doi: 10.1111/jhn.12058
139. Trichopoulou A, Kouris-Blazos A, Wahlqvist ML, Gnardellis C, Lagiou P, Polychronopoulos E, et al. Diet and overall survival in elderly people. BMJ. (1995) 311:1457–60. doi: 10.1136/bmj.311.7018.1457
140. Monteagudo C, Mariscal-Arcas M, Rivas A, Lorenzo-Tovar ML, Tur JA, Olea-Serrano F. Proposal of a Mediterranean Diet Serving Score. PLoS ONE. (2015) 10:e0128594. doi: 10.1371/journal.pone.0128594
141. Krishnan S, O'Connor LE, Wang Y, Gertz ER, Campbell WW, Bennett BJ. Adopting a Mediterranean-style eating pattern with low, but not moderate, unprocessed, lean red meat intake reduces fasting serum trimethylamine N-oxide (TMAO) in adults who are overweight or obese. Br J Nutr. (2021) 128:1–21. doi: 10.1017/S0007114521004694
142. Maldonado-Contreras A, Noel SE, Ward DV, Velez M, Mangano KM. Associations between diet, the gut microbiome, and short-chain fatty acid production among older Caribbean Latino adults. J Acad Nutr Diet. (2020) 120:2047–60 e6. doi: 10.1016/j.jand.2020.04.018
143. Trichopoulou A, Costacou T, Bamia C, Trichopoulos D. Adherence to a Mediterranean diet and survival in a Greek population. N Engl J Med. (2003) 348:2599–608. doi: 10.1056/NEJMoa025039
144. Mitsou EK, Kakali A, Antonopoulou S, Mountzouris KC, Yannakoulia M, Panagiotakos DB, et al. Adherence to the Mediterranean diet is associated with the gut microbiota pattern and gastrointestinal characteristics in an adult population. Br J Nutr. (2017) 117:1645–55. doi: 10.1017/S0007114517001593
145. Panagiotakos DB, Pitsavos C, Stefanadis C. Dietary patterns: a Mediterranean diet score and its relation to clinical and biological markers of cardiovascular disease risk. Nutr Metab Cardiovasc Dis. (2006) 16:559–68. doi: 10.1016/j.numecd.2005.08.006
146. Nagpal R, Neth BJ, Wang S, Craft S, Yadav H. Modified Mediterranean-ketogenic diet modulates gut microbiome and short-chain fatty acids in association with Alzheimer's disease markers in subjects with mild cognitive impairment. EBioMedicine. (2019) 47:529–42. doi: 10.1016/j.ebiom.2019.08.032
147. Sofi F, Macchi C, Abbate R, Gensini GF, Casini A. Mediterranean diet and health status: an updated meta-analysis and a proposal for a literature-based adherence score. Public Health Nutr. (2014) 17:2769–82. doi: 10.1017/S1368980013003169
148. Park JE, Miller M, Rhyne J, Wang Z, Hazen SL. Differential effect of short-term popular diets on TMAO and other cardio-metabolic risk markers. Nutr Metab Cardiovasc Dis. (2019) 29:513–7. doi: 10.1016/j.numecd.2019.02.003
149. Pastori D, Carnevale R, Nocella C, Novo M, Santulli M, Cammisotto V, et al. Gut-derived serum lipopolysaccharide is associated with enhanced risk of major adverse cardiovascular events in atrial fibrillation: effect of adherence to Mediterranean diet. J Am Heart Assoc. (2017) 6:5784. doi: 10.1161/JAHA.117.005784
150. Pastori D, Ettorre E, Carnevale R, Nocella C, Bartimoccia S, Del Sordo E, et al. Interaction between serum endotoxemia and proprotein convertase subtilisin/kexin 9 (PCSK9) in patients with atrial fibrillation: a post-hoc analysis from the ATHERO-AF cohort. Atherosclerosis. (2019) 289:195–200. doi: 10.1016/j.atherosclerosis.2019.07.002
151. Pignanelli M, Just C, Bogiatzi C, Dinculescu V, Gloor GB, Allen-Vercoe E, et al. Mediterranean diet score: associations with metabolic products of the intestinal microbiome, carotid plaque burden, and renal function. Nutrients. (2018) 10:779. doi: 10.3390/nu10060779
152. Fung TT, Rexrode KM, Mantzoros CS, Manson JE, Willett WC, Hu FB. Mediterranean diet and incidence of and mortality from coronary heart disease and stroke in women. Circulation. (2009) 119:1093–100. doi: 10.1161/CIRCULATIONAHA.108.816736
153. Quercia S, Turroni S, Fiori J, Soverini M, Rampelli S, Biagi E, et al. Gut microbiome response to short-term dietary interventions in reactive hypoglycemia subjects. Diabetes Metab Res Rev. (2017) 33:2927. doi: 10.1002/dmrr.2927
154. Ruiz-Saavedra S, Salazar N, Suarez A, de Los Reyes-Gavilan CG, Gueimonde M, Gonzalez S. Comparison of different dietary indices as predictors of inflammation, oxidative stress and intestinal microbiota in middle-aged and elderly subjects. Nutrients. (2020) 12:3828. doi: 10.3390/nu12123828
155. Mariscal-Arcas M, Romaguera D, Rivas A, Feriche B, Pons A, Tur JA, et al. Diet quality of young people in southern Spain evaluated by a Mediterranean adaptation of the Diet Quality Index-International (DQI-I). Br J Nutr. (2007) 98:1267–73. doi: 10.1017/S0007114507781424
156. Buckland G, Agudo A, Travier N, Huerta JM, Cirera L, Tormo MJ, et al. Adherence to the Mediterranean diet reduces mortality in the Spanish cohort of the European Prospective Investigation into Cancer and Nutrition (EPIC-Spain). Br J Nutr. (2011) 106:1581–91. doi: 10.1017/S0007114511002078
157. Trichopoulou A, Orfanos P, Norat T, Bueno-de-Mesquita B, Ocke MC, Peeters PH, et al. Modified Mediterranean diet and survival: EPIC-elderly prospective cohort study. BMJ. (2005) 330:991. doi: 10.1136/bmj.38415.644155.8F
158. Seethaler B, Nguyen NK, Basrai M, Kiechle M, Walter J, Delzenne NM, et al. Short-chain fatty acids are key mediators of the favorable effects of the Mediterranean diet on intestinal barrier integrity: data from the randomized controlled LIBRE trial. Am J Clin Nutr. (2022) 116:928–42. doi: 10.1093/ajcn/nqac175
159. Schroder H, Fito M, Estruch R, Martinez-Gonzalez MA, Corella D, Salas-Salvado J, et al. A short screener is valid for assessing mediterranean diet adherence among older Spanish men and women. J. Nutr. (2011) 141:1140–5. doi: 10.3945/jn.110.135566
160. Hebestreit K, Yahiaoui-Doktor M, Engel C, Vetter W, Siniatchkin M, Erickson N, et al. Validation of the German version of the Mediterranean Diet Adherence Screener (MEDAS) questionnaire. BMC Cancer. (2017) 17:341. doi: 10.1186/s12885-017-3337-y
161. Seethaler B, Lehnert K, Yahiaoui-Doktor M, Basrai M, Vetter W, Kiechle M, et al. Omega-3 polyunsaturated fatty acids improve intestinal barrier integrity-albeit to a lesser degree than short-chain fatty acids: an exploratory analysis of the randomized controlled LIBRE trial. Eur J Nutr. (2023) 62:2779–91. doi: 10.1007/s00394-023-03172-2
162. Shankar V, Gouda M, Moncivaiz J, Gordon A, Reo NV, Hussein L, Paliy O. Differences in gut metabolites and microbial composition and functions between Egyptian and U.S. children are consistent with their diets. mSystems. (2017) 62:2779–91. doi: 10.1128/mSystems.00169-16
163. Shoer S, Shilo S, Godneva A, Ben-Yacov O, Rein M, Wolf BC, et al. Impact of dietary interventions on pre-diabetic oral and gut microbiome, metabolites and cytokines. Nat Commun. (2023) 14:5384. doi: 10.1038/s41467-023-41042-x
164. Papadaki A, Johnson L, Toumpakari Z, England C, Rai M, Toms S, et al. Validation of the English Version of the 14-Item Mediterranean diet adherence screener of the PREDIMED study, in people at high cardiovascular risk in the UK. Nutrients. (2018) 10:138. doi: 10.3390/nu10020138
165. Tanaka T, Talegawkar SA, Jin Y, Candia J, Tian Q, Moaddel R, et al. Metabolomic profile of different dietary patterns and their association with frailty index in community-dwelling older men and women. Nutrients. (2022) 14:2237. doi: 10.3390/nu14112237
166. Morris MC, Tangney CC, Wang Y, Sacks FM, Barnes LL, Bennett DA, et al. MIND diet slows cognitive decline with aging. Alzheimers Dement. (2015) 11:1015–22. doi: 10.1016/j.jalz.2015.04.011
167. Vitale M, Giacco R, Laiola M, Della Pepa G, Luongo D, Mangione A, et al. Acute and chronic improvement in postprandial glucose metabolism by a diet resembling the traditional Mediterranean dietary pattern: Can SCFAs play a role? Clin Nutr. (2021) 40:428–37. doi: 10.1016/j.clnu.2020.05.025
168. Zhu CH, Sawrey-Kubicek L, Beals E, Rhodes CH, Houts HE, Sacchi R, et al. Human gut microbiome composition and tryptophan metabolites were changed differently by fast food and Mediterranean diet in 4 days: a pilot study. Nutrition Research. (2020) 77:62–72. doi: 10.1016/j.nutres.2020.03.005
169. Hernandez MAG, Canfora EE, Jocken JWE, Blaak EE. The short-chain fatty acid acetate in body weight control and insulin sensitivity. Nutrients. (2019) 11:1943. doi: 10.3390/nu11081943
170. Arifuzzaman M, Collins N, Guo CJ, Artis D. Nutritional regulation of microbiota-derived metabolites: Implications for immunity and inflammation. Immunity. (2024) 57:14–27. doi: 10.1016/j.immuni.2023.12.009
171. Bachem A, Makhlouf C, Binger KJ, de Souza DP, Tull D, Hochheiser K, et al. Microbiota-derived short-chain fatty acids promote the memory potential of antigen-activated CD8(+) T cells. Immunity. (2019) 51:285–97 e5. doi: 10.1016/j.immuni.2019.06.002
172. Hang S, Paik D, Yao L, Kim E, Trinath J, Lu J, et al. Bile acid metabolites control T(H)17 and T(reg) cell differentiation. Nature. (2019) 576:143–8. doi: 10.1038/s41586-019-1785-z
173. Paik D, Yao L, Zhang Y, Bae S, D'Agostino GD, Zhang M, et al. Human gut bacteria produce Tau(Eta)17-modulating bile acid metabolites. Nature. (2022) 603:907–12. doi: 10.1038/s41586-022-04480-z
174. Arifuzzaman M, Won TH Li TT, Yano H, Digumarthi S, Heras AF, et al. Inulin fibre promotes microbiota-derived bile acids and type 2 inflammation. Nature. (2022) 611:578–84. doi: 10.1038/s41586-022-05380-y
175. Hoyles L, Pontifex MG, Rodriguez-Ramiro I, Anis-Alavi MA, Jelane KS, Snelling T, et al. Regulation of blood brain barrier integrity by microbiome-associated methylamines and cognition by trimethylamine N-oxide. Microbiome. (2021) 9:235. doi: 10.1186/s40168-021-01181-z
176. Islam T, Albracht-Schulte K, Ramalingam L, Schlabritz-Lutsevich N, Park OH, Zabet-Moghaddam M, et al. Anti-inflammatory mechanisms of polyphenols in adipose tissue: role of gut microbiota, intestinal barrier integrity and zinc homeostasis. J Nutr Biochem. (2023) 115:109242. doi: 10.1016/j.jnutbio.2022.109242
177. Vicentini FA, Keenan CM, Wallace LE, Woods C, Cavin JB, Flockton AR, et al. Intestinal microbiota shapes gut physiology and regulates enteric neurons and glia. Microbiome. (2021) 9:210. doi: 10.1186/s40168-021-01165-z
178. Shi H, Ge X, Ma X, Zheng M, Cui X, Pan W, et al. A fiber-deprived diet causes cognitive impairment and hippocampal microglia-mediated synaptic loss through the gut microbiota and metabolites. Microbiome. (2021) 9:223. doi: 10.1186/s40168-021-01172-0
179. Gutierrez-Repiso C, Molina-Vega M, Bernal-Lopez MR, Garrido-Sanchez L, Garcia-Almeida JM, Sajoux I, et al. Different weight loss intervention approaches reveal a lack of a common pattern of gut microbiota changes. J Pers Med. (2021) 11:109. doi: 10.3390/jpm11020109
180. Lane MM, Davis JA, Beattie S, Gomez-Donoso C, Loughman A, O'Neil A, et al. Ultraprocessed food and chronic noncommunicable diseases: A systematic review and meta-analysis of 43 observational studies. Obes Rev. (2021) 22:e13146. doi: 10.1111/obr.13146
181. Kapoor P, Tiwari A, Sharma S, Tiwari V, Sheoran B, Ali U, Garg M. Effect of anthocyanins on gut health markers, Firmicutes-Bacteroidetes ratio and short-chain fatty acids: a systematic review via meta-analysis. Sci. Rep. (2023) 13:1729. doi: 10.1038/s41598-023-28764-0
182. Sowah SA, Riedl L, Damms-Machado A, Johnson TS, Schubel R, Graf M, et al. Effects of weight-loss interventions on short-chain fatty acid concentrations in blood and feces of adults: a systematic review. Adv Nutr. (2019) 10:673–84. doi: 10.1093/advances/nmy125
183. Farhat Z, Sampson JN, Hildesheim A, Safaeian M, Porras C, Cortes B, et al. Reproducibility, temporal variability, and concordance of serum and fecal bile acids and short chain fatty acids in a population-based study. Cancer Epidemiol Biomarkers Prev. (2021) 30:1875–83. doi: 10.1158/1055-9965.EPI-21-0361
184. Bailey MA, Holscher HD. Microbiome-mediated effects of the mediterranean diet on inflammation. Adv Nutr. (2018) 9:193–206. doi: 10.1093/advances/nmy013
185. Hu Y, Chen Z, Xu C, Kan S, Chen D. Disturbances of the gut microbiota and microbiota-derived metabolites in inflammatory Bowel disease. Nutrients. (2022) 14:5140. doi: 10.3390/nu14235140
186. Quinn RA, Melnik AV, Vrbanac A, Fu T, Patras KA, Christy MP, et al. Global chemical effects of the microbiome include new bile-acid conjugations. Nature. (2020) 579:123–9. doi: 10.1038/s41586-020-2047-9
187. Bustamante JM, Dawson T, Loeffler C, Marfori Z, Marchesi JR, Mullish BH, et al. Impact of fecal microbiota transplantation on gut bacterial bile acid metabolism in humans. Nutrients. (2022) 14:5200. doi: 10.3390/nu14245200
188. Li M, Wang S, Li Y, Zhao M, Kuang J, Liang D, et al. Gut microbiota-bile acid crosstalk contributes to the rebound weight gain after calorie restriction in mice. Nat Commun. (2022) 13:2060. doi: 10.1038/s41467-022-29589-7
189. von Schwartzenberg RJ, Bisanz JE, Lyalina S, Spanogiannopoulos P, Ang QY, Cai J, et al. Caloric restriction disrupts the microbiota and colonization resistance. Nature. (2021) 595:272–7. doi: 10.1038/s41586-021-03663-4
190. Xiao C, Wang JT, Su C, Miao Z, Tang J, Ouyang Y, et al. Associations of dietary diversity with the gut microbiome, fecal metabolites, and host metabolism: results from 2 prospective Chinese cohorts. Am J Clin Nutr. (2022) 116:1049–58. doi: 10.1093/ajcn/nqac178
191. Seconda L, Baudry J, Alles B, Hamza O, Boizot-Szantai C, Soler LG, et al. Assessment of the sustainability of the Mediterranean diet combined with organic food consumption: an individual behaviour approach. Nutrients. (2017) 9:61. doi: 10.3390/nu9010061
192. Newman NK, Zhang Y, Padiadpu J, Miranda CL, Magana AA, Wong CP, et al. Reducing gut microbiome-driven adipose tissue inflammation alleviates metabolic syndrome. Microbiome. (2023) 11:208. doi: 10.1186/s40168-023-01637-4
193. Faits T, Walker ME, Rodriguez-Morato J, Meng H, Gervis JE, Galluccio JM, et al. Exploring changes in the human gut microbiota and microbial-derived metabolites in response to diets enriched in simple, refined, or unrefined carbohydrate-containing foods: a post hoc analysis of a randomized clinical trial. Am J Clin Nutr. (2020) 112:1631–41. doi: 10.1093/ajcn/nqaa254
194. Janeiro MH, Ramirez MJ, Milagro FI, Martinez JA, Solas M. Implication of trimethylamine N-oxide (TMAO) in disease: potential biomarker or new therapeutic target. Nutrients. (2018) 10:1398. doi: 10.3390/nu10101398
195. Chen K, Zheng X, Feng M, Li D, Zhang H. Gut microbiota-dependent metabolite trimethylamine N-oxide contributes to cardiac dysfunction in western diet-induced obese mice. Front Physiol. (2017) 8:139. doi: 10.3389/fphys.2017.00139
196. Papandreou C, Bullo M, Zheng Y, Ruiz-Canela M, Yu E, Guasch-Ferre M, et al. Plasma trimethylamine-N-oxide and related metabolites are associated with type 2 diabetes risk in the Prevencion con Dieta Mediterranea (PREDIMED) trial. Am J Clin Nutr. (2018) 108:163–73. doi: 10.1093/ajcn/nqy058
197. Diez-Ricote L, San-Cristobal R, Concejo MJ, Martinez-Gonzalez MA, Corella D, Salas-Salvado J, et al. One-year longitudinal association between changes in dietary choline or betaine intake and cardiometabolic variables in the PREvencion con DIeta MEDiterranea-Plus (PREDIMED-Plus) trial. Am J Clin Nutr. (2022) 116:1565–79. doi: 10.1093/ajcn/nqac255
198. Jang H, Lim H, Park KH, Park S, Lee HJ. Changes in plasma choline and the betaine-to-choline ratio in response to 6-month lifestyle intervention are associated with the changes of lipid profiles and intestinal microbiota: The ICAAN Study. Nutrients. (2021) 13:6. doi: 10.3390/nu13114006
199. Ghanim H, Batra M, Abuaysheh S, Green K, Makdissi A, Kuhadiya ND, et al. Antiinflammatory and ROS suppressive effects of the addition of fiber to a high-fat high-calorie meal. J Clin Endocrinol Metab. (2017) 102:858–69. doi: 10.1210/jc.2016-2669
200. Pendyala S, Walker JM, Holt PR. A high-fat diet is associated with endotoxemia that originates from the gut. Gastroenterology. (2012) 142:1100–1 e2. doi: 10.1053/j.gastro.2012.01.034
201. Hasegawa Y, Pei R, Raghuvanshi R, Liu Z, Bolling BW. Yogurt supplementation attenuates insulin resistance in obese mice by reducing metabolic endotoxemia and inflammation. J Nutr. (2023) 153:703–12. doi: 10.1016/j.tjnut.2023.01.021
202. Johnson SL, Kirk RD, DaSilva NA, Ma H, Seeram NP, Bertin MJ. Polyphenol microbial metabolites exhibit gut and blood(-)brain barrier permeability and protect murine microglia against LPS-induced inflammation. Metabolites. (2019) 9:78. doi: 10.3390/metabo9040078
203. Bartimoccia S, Cammisotto V, Nocella C, Del Ben M, D'Amico A, Castellani V, et al. Extra virgin olive oil reduces gut permeability and metabolic endotoxemia in diabetic patients. Nutrients. (2022) 14:2153. doi: 10.3390/nu14102153
204. Gundogdu A, Nalbantoglu OU. The role of the Mediterranean diet in modulating the gut microbiome: a review of current evidence. Nutrition. (2023) 114:112118. doi: 10.1016/j.nut.2023.112118
Keywords: Mediterranean, obesity, microbiome, metabolites, inflammation, short-chain fatty acids, trimethylamine N-oxide, bile acids
Citation: Florkowski M, Abiona E, Frank KM and Brichacek AL (2024) Obesity-associated inflammation countered by a Mediterranean diet: the role of gut-derived metabolites. Front. Nutr. 11:1392666. doi: 10.3389/fnut.2024.1392666
Received: 27 February 2024; Accepted: 03 June 2024;
Published: 24 June 2024.
Edited by:
Mahta Moussavi, Prairie View A&M University, United StatesReviewed by:
Andrew McLeod, University of Illinois Chicago, United StatesLaura J. Den Hartigh, University of Washington, United States
Copyright © 2024 Florkowski, Abiona, Frank and Brichacek. This is an open-access article distributed under the terms of the Creative Commons Attribution License (CC BY). The use, distribution or reproduction in other forums is permitted, provided the original author(s) and the copyright owner(s) are credited and that the original publication in this journal is cited, in accordance with accepted academic practice. No use, distribution or reproduction is permitted which does not comply with these terms.
*Correspondence: Allison L. Brichacek, YWxsaXNvbi5icmljaGFjZWsmI3gwMDA0MDtuaWguZ292