- 1Department of Urology II, The First Hospital of Jilin University, Changchun, Jilin, China
- 2Key Laboratory of Pathobiology, Ministry of Education, Jilin University, Changchun, Jilin, China
Senescence is an inevitable biological process. Disturbances in glucose and lipid metabolism are essential features of cellular senescence. Given the important roles of these types of metabolism, we review the evidence for how key metabolic enzymes influence senescence and how senescence-related secretory phenotypes, autophagy, apoptosis, insulin signaling pathways, and environmental factors modulate glucose and lipid homeostasis. We also discuss the metabolic alterations in abnormal senescence diseases and anti-cancer therapies that target senescence through metabolic interventions. Our work offers insights for developing pharmacological strategies to combat senescence and cancer.
1. Introduction
Senescence is a universal and complex biological process that affects all living organisms. While senescence contributes to embryonic development and limits tumorigenesis and tissue damage (1), it involves dynamic biological, environmental, physiological, psychological, behavioral, and social process changes leading to progressive declines in biological functions and physiological processes and increased susceptibility to disease, disability, and death (2, 3). The senescence process increases the risk of chronic diseases such as diabetic neurodegenerative diseases, metabolic syndrome, and cardiovascular disease, which have become more prevalent in the elderly population (4). Therefore, understanding the mechanisms and factors that influence senescence and its consequences is crucial for developing interventions to prevent, diagnose, treat, and delay age-related diseases and improve healthspan (the portion of life spent in good health).
Senescence and cell death are two different but interrelated phenomena. Cell death is the irreversible loss of cell viability and function that can occur through different mechanisms, including apoptosis, autophagy, necrosis, and senescence (5). In 2019, the International Cell Senescence Association reached a consensus on the characteristics and biomarkers of cellular senescence (6). They concluded that cellular senescence is not the same as cell death and that senescent cells remain metabolically active for some time and show distinct changes, with four characteristics typical of cell cycle arrest, senescence-related secretory phenotypes (SRSPs), macromolecular damage, and metabolic disorders. Senescent cells show reduced mitochondrial function, impaired adenosine triphosphate (ATP) production, and increased production of reactive oxygen species (ROS), which cause protein and lipid damage (macromolecular damage). Disorders in glucose and lipid metabolism are among the results and further drive the progression of senescence (7, 8).
Lipids may influence cell activity by altering cytomembrane composition, energy reserves, secondary messenger signaling, and gene expression (9–11). A common component of biological lipid species is fatty acids. One of the signs of senescence is fatty acid metabolic dysregulation, which is related to the transformation from catabolism to synthesis. A decline in fatty acid oxidation (FAO) capacity determines lipid imbalance and age-related obesity (12). Tissue-specific stem cells repair tissue damage and maintain tissue homeostasis. Unbalanced stem cell self-renewal can cause harmful effects such as disease and senescence. Age-related declines in FAO levels harm stem cell maintenance and activity, driving stem cell senescence (13). The imbalance between nuclear receptors (liver-X-receptor α, retinoid acid receptor α, and peroxisome proliferator-activated receptor α [PPARα]) and their target genes (ATP-binding cassette transporter A1, sterol regulatory element binding protein 1c [SREBP1c], and fatty acid synthase [FAS]) likely drives hepatic steatosis in aged patients (14). Moreover, H2O2-induced senescence is significantly decreased by inhibiting lipogenesis using FAS inhibitors or silencing SREBP1 and ATP citrate lyase (15). All these pieces of evidence indicate that fatty acid metabolism seriously affects senescence. Targeting the key enzymes of fatty acid metabolism is the most direct and scientific means to screen for anti-senescence drugs or promote tumor senescence treatment.
Glucose metabolism is also involved in the regulation of intracellular senescence. Glucose transporters (GLUTs), members of the membrane transporter family, transport extracellular glucose into the cell. GLUT1 is highly expressed in senescent cells and increases glucose uptake (16, 17). In contrast, under low-concentration glucose culture conditions, drug-induced senescence in lung cancer cells (17) or replicative senescence of human fibroblasts (18) and stem cells (19) cultured in vitro is delayed (20). Glucose transported into cells by GLUTs is catalyzed to pyruvate via glycolysis enzymes. The levels of multiple glycolytic enzymes, including hexokinases (HKs), lactate dehydrogenase A (LDHA), and pyruvate kinase (PK), are increased in senescent cells (21). Finally, the metabolites generated by pyruvate entering the tricarboxylic acid (TCA) cycle, including α-ketoglutaric acid (αKG) (22–24) and citrate (25, 26), significantly inhibit intracellular senescence.
This review aims to clarify the relationship between lipids, glucose metabolism, and senescence.
2. Lipids and senescence
2.1. Fatty acid catabolism and senescence
Fatty acid catabolism includes mitochondrial β-oxidation of saturated fatty acids, peroxisomal β-oxidation of very-long-chain fatty acids, α-oxidation of branched-chain fatty acids, ω-oxidation, and ketone formation (27–31). Mitochondrial β-oxidation of saturated fatty acids and peroxisomal β-oxidation of very-long-chain fatty acids are generally the primary metabolic pathways, and carnitine palmitoyl transferase 1 (CPT1) and acyl-CoA oxidase 1 (ACOX1) play crucial roles as rate-limiting enzymes.
2.1.1. Mitochondrial β-oxidation
Under sufficient oxygen supply, medium- and long-chain fatty acids can be decomposed into acetyl-CoA, which can be further completely oxidized into CO2, H2O, and significant energy. The whole process of converting acyl CoA to acetyl-CoA, called mitochondrial β-FAO, occurs in the mitochondrial matrix (32). CPT1 assists acyl CoA in the transmembrane entry into the mitochondria and is the primary rate-limiting enzyme (33). All three isoforms of CPT1 (CPT1A, CPT1B, and CPT1C) are associated with senescence. Comparison with proteomic analysis of adult mouse kidneys revealed significantly lower CPT1A expression in aged mice (34). In diabetic nephropathy, a common disease associated with senescence, CPT1A expression is similarly suppressed (35). The same trend in CPT1B expression has been observed in aged rats (36). In addition, deletion of the novel biomarker CPT1C induced mitochondrial dysfunction, leading to senescence-like growth inhibition and cellular senescence in six cancer cell types, including pancreatic cancer cells, and inhibited cancer growth in vivo (37). While these findings suggest that senescence is inextricably linked to the absence of CPT1, the exact mechanism is not yet clear.
In recent years, scholars have made progress in this regard (Figure 1). Telomeres, the protective caps on the ends of chromosomes, guard genetic material during cell division. Telomeres shorten with each cell division until they reach a critical length that triggers cellular senescence or apoptosis (38). Telomere shortening is one of the hallmarks of senescence, as it reflects the cumulative damage and stress that cells experience over time. In human hepatoma (HepG2) cells, the knockdown of patatin-like phospholipase domain-containing 2 (also called adipose triglyceride lipase [ATGL]) and CPT1, two crucial genes for lipolysis, results in reduced telomere length (39).
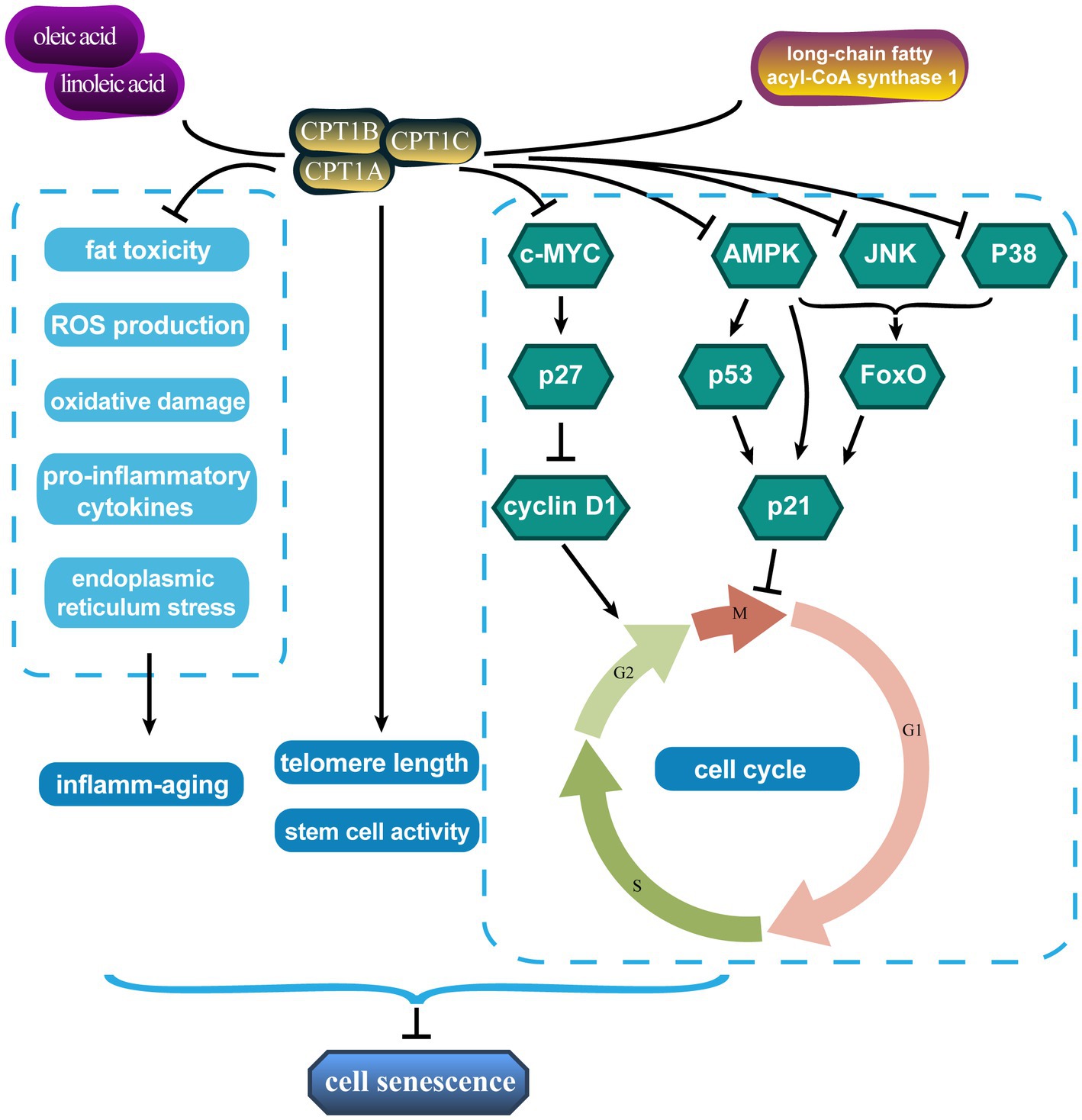
Figure 1. Carnitine palmitoyl transferases affect senescence through multiple pathways. CPT1, carnitine palmitoyl transferase 1.
In addition, the cell cycle regulates cell differentiation, proliferation, and senescence. Moreover, senescent cells are blocked in certain growth phases. Treatment with linoleic and oleic acids significantly increased CPT1 expression and S-phase ratio in primary bovine satellite cells and promoted cell proliferation, while decreasing the G0/G1 phase ratio (40). Long-chain fatty acyl-CoA synthase 1, which catalyzes the bioconversion of exogenous or de novo synthesized fatty acids to fatty acyl-CoA, promoted tumor progression by reducing CPT1 activity and the number of prostate cancer cells remaining in the G1/S phase (41). The triggers of senescence ultimately converge on the p53/p21CIP1 and p16INK4A/pRb pathways, which induce cell cycle arrest (42, 43). The proto-oncogene c-MYC is involved in cell cycle progression, apoptosis, and cellular transformation. CPT depletion, especially CPT1C, leads to cellular senescence by blocking the cell proliferation-promoting and cell cycle progression effects of the c-MYC/p27/cyclin D1 signaling axis (44). CPT1 overexpression increases ATP production from FAO sources and drives cell cycle operation in pulmonary artery smooth muscle cells by inhibiting the adenosine 5′-monophosphate (AMP)-activated protein kinase (AMPK)-p53-p21 signaling pathway (45). CPT1A also represses p21 expression by inhibiting forkhead box O (FoxO), which must be phosphorylated by various protein kinases such as AMPK, c-Jun N-terminal protein kinase (JNK), and p38 to perform their functions (46).
The loss of stem cell number and function is also a visual manifestation of senescence. This age-related change underlays some adverse consequences of senescence. Mihaylova et al. (47) showed that interference with CPT1A reversed fasting-induced enhancement of intestinal stem cell activity and that CPT1A deficiency reduced ISC number and function. Moreover, CPT1A-mediated FAO facilitates the maintenance of neural stem/progenitor cell quiescence (48, 49). Chronic inflammation is a defining characteristic of senescence and has been dubbed “inflamm-senescence” (50). Mitochondrial FAO with CPT1 drives anti-inflammatory protective effects due to its ability to remove pro-inflammatory compounds such as saturated fatty acids by reducing fat toxicity and ROS production and attenuating palmitic acid-induced pro-inflammatory cytokines, oxidative damage, and endoplasmic reticulum stress (51, 52).
In conclusion, these results tentatively explain the anti-senescence effects of mitochondrial β-oxidation and CPT1. Targeted increase or inhibition of CPT1 expression for different diseases may reduce age-related senescence damage or inhibit tumor progression.
2.1.2. Peroxisomal β-oxidation
Mitochondrial β-oxidation metabolizes common saturated fatty acids. However, some fatty acids, such as very long-chain fatty acids (VLCFAs, with >22 carbons), require the peroxisomal β-oxidation-dependent pathway to be converted into chain-shortened acyl-CoA, which is then transported to the mitochondria for complete oxidation (53). ACOX1 catalyzes its rate-limiting step. Numerous senescence-related diseases are directly related to fatty acid peroxisome β-oxidation. Age-related reduction in liver peroxisomal β-oxidation was accompanied by altered brain fatty acid composition and decreased ACOX1 and PPARα expression in old rats (54). In senescence and age-related degenerative diseases, peroxisome β-oxidation and ACOX1 are significantly reduced (55). In the early stages of Alzheimer’s disease (AD), ACOX1 and its transcriptional regulators PPARα and peroxisome proliferator-activated receptor gamma coactivator 1 α (PGC-1α) are induced to be highly expressed due to damaged mitochondria and increased oxidative stress but are significantly reduced in late stages (56). Moreover, decreased ACOX1, CPT1, and PPARα expression in leptin-resistant elderly rats is the basis of age- and lipid-related diseases (57).
ACOX1 deficiency leading to the accumulation of specific metabolites may affect the biogenesis of the peroxisome itself and/or the homeostasis of other cellular organelles such as the mitochondria and endoplasmic reticulum (Figure 2). Inflammation plays an important role in senescence. The main characteristics of pseudo-neonatal adrenoleukodystrophy (a neurodegenerative disease) are VLCFA accumulation and inflammatory demyelination due to activation of the IL-1 inflammatory pathway. The main cause of this lesion is the absence of ACOX1 (58). Fibroblasts from an ACOX1-deficient patient showed downregulation of numerous cytokines and chemokine mRNAs, including CXCL14 and CXCL12, which are relevant to the regulation of cellular homeostasis (58). Mice lacking ACOX1 also exhibited elevated levels of inflammatory cytokines such as interleukin-6 (IL-6) and tumor necrosis factor-α (TNF-α), and induced T cell polarization (59). However, inflammation resolution is a dynamic biosynthetically active process governed by a superfamily of bioactive lipids known as specialized pro-resolving mediators (SPMs), which enhance the remission of inflammatory reactions (60). SPMs can regulate autophagy, change the release of cytokines/chemokines, and affect the migration and function of leukocytes to alleviate inflammation and delay the senescence process. As it is upstream of SPM biosynthesis, ACOX1 has become a potential anti-senescence protein (61, 62).
PPARα, a subtype of PPARs (including α, β/δ, and γ) and a transcriptional activator of ACOX1 and CPT1, regulates the fatty acid β-oxidation pathway (63, 64). PPARα activity and expression decreased in various aged-animal organs, including the liver and heart (65, 66). These reductions are most likely directly connected to lipid metabolic abnormalities and elevated levels of inflammatory mediators in elderly animals (67). Fatty acid-binding protein-1 (FABP1) binds to free fatty acids by upregulating PPARα and protects the liver from fat toxicity (68). Senescence decreases FABP1 levels, which alters PPARα and causes β-oxidation damage, increasing the morbidity of non-alcoholic fatty liver disease (69). PPARα depletion inhibits cell proliferation and induces cell senescence by reducing the expression of the target gene CPT1C (70).
Drugs targeting PPARα may have the potential to fight age-related diseases such as atherosclerosis, vascular diseases, and AD (71). The receptor for advanced glycation end product (RAGE) plays a crucial role in senescence-related vascular disorders, liver damage, and insulin resistance (72–74). Wan et al. (75) observed elevated RAGE levels at the molecular level, which was associated with lower PPARα and β-oxidation levels and was responsible for the deposition of hepatic TG in aged mice. Thus, the RAGE/PPARα regulator axis may be a therapeutic target for fatty liver disease associated with senescence. In Caenorhabditis elegans, the administration of ω-3 polyunsaturated fatty acids (α-linolenic acid) increased longevity by activating the transcription factors NHR-49/PPARα (76). Oleoylethanolamide (OEA) can interact with hepatocyte nuclear factor 4 and PPARα and promote their transcriptional activity. Worms treated with OEA showed PPARα activation and increased lifespans (77).
Fenofibrate, a PPARα agonist, reduces the number of senescent cells by increasing apoptosis and autophagy flux and prevents cartilage degeneration caused by senescent and osteoarthritis (78). Proanthocyanidins correct lipid metabolism disorders and treat senescence-related diseases by regulating key enzymes, including PPARα and CPT1 (79). In a d-galactose-induced senescent mouse model, mussel peptides mitigated lipid metabolic diseases and the senescence phenotype by preserving glucose and lipid homeostasis and increasing PPARα/PPARγ expression levels (80). These findings suggest that PPARs may be essential targets in lipid signaling pathways that promote lifespan. Age-related modifications in PPARα in humans have not been explicitly proven, and determining whether the results of animal experiments are applicable to humans is difficult. However, these results provide molecular support for the anti-senescence application of PPAR agonists (e.g., fibrates).
2.1.3. Fatty acid α-/ω-oxidation
Fatty acid α-oxidation occurs in the peroxisomes of cells. This process involves the degradation of branched-chain fatty acids, such as phytanic acid, produced by the body or obtained through dietary intake. Phytanic acid is a 20-carbon fatty acid with a methyl group at the γ-carbon, which prevents it from undergoing β-oxidation (81). Therefore, phytanic acid is oxidized at the α-carbon, which is adjacent to the carboxyl group and loses one carbon atom as CO2. Phytanoyl-CoA hydroxylase is involved in this process. The resulting product, pristanic acid, can then enter the β-oxidation pathway and generate acetyl-CoA and propionyl-CoA. Fatty acid α-oxidation and senescence are related in several aspects. First, fatty acid α-oxidation is impaired in some senescence-related diseases, such as Refsum disease, a rare genetic disorder that causes the accumulation of phytanic acid in various tissues and plasma, leading to neurological symptoms such as peripheral neuropathy, ataxia, retinitis pigmentosa, and deafness (82). Second, fatty acid α-oxidation generates ROS as by-products (83), which can cause oxidative stress and damage to cellular components, including DNA, proteins, and lipids. Oxidative stress is a major contributor to senescence and age-related diseases such as neurodegeneration, cancer, and diabetes. Third, α-oxidation of fatty acids is also regulated by PPARs (84), the latter in relation to senescence as discussed above regarding peroxisomal β-oxidation.
Fatty acid ω-oxidation occurs in the endoplasmic reticulum of some cells, especially in the liver and kidney (29). This process involves the monooxygenase-catalyzed oxidation of the fatty acid ω-carbon (the carbon furthest from the carboxyl group), followed by successive oxidations of the β-carbon until the fatty acid chain is shortened by two carbon atoms. This mechanism exists to break down large, water-insoluble fatty acids that, in greater quantities, would be hazardous to cells (85). The ω-oxidation of fatty acids accelerates the rate of fatty acid degradation as feedback to various stressors such as hypoxia, inflammation, and stimulation by exogenous substances. These substances increase the demand for FAO and detoxification. Correspondingly, the omega-oxidation of fatty acids reduces the likelihood that these stressors will lead to senescence and age-related diseases.
2.2. Fatty acid synthesis and senescence
Triglycerides, phospholipids, sphingolipids, and cholesterol lipids are all produced by a series of enzymatic reactions between fatty acids and different chemical groups. Therefore, the regulation of fatty acid synthesis affects the metabolic homeostasis of lipids to a certain extent.
2.2.1. Acetyl-CoA carboxylase
Acetyl-CoA carboxylase (ACC) catalyzes the rate-limiting step of de novo fatty acid synthesis (86). Among the two subtypes, ACC1 and ACC2, the former locates in the cytoplasm and is used for fatty acid synthesis by converting acetyl coenzyme A into malonyl coenzyme A. ACC2 is located on the cytoplasmic surface of mitochondria, and the product is used to inhibit CPT1 from reducing FAO (87). SiRNA silencing of SREBP1 significantly reduced the expression of its downstream target genes ACC, FAS, and ATP citrate lyase, and weakened H2O2-induced senescence (15). ACC1-dependent lipogenesis is the fundamental metabolic pathway downstream of AMPK, which induces autophagy and maintains cell survival during yeast senescence (88). This evidence suggests that ACC directly modulates the senescence phenotype, and several drugs targeting ACC to slow senescence already exist. Citrate treatment can increase ACC1 expression, promote excessive lipid biosynthesis, and lead to tumor cell senescence and growth inhibition (26). The AD candidate drugs CMS121 and J147 play anti-senescence neuroprotective roles by inhibiting ACC1 and increasing acetyl-CoA levels (89). Resveratrol plays an anti-senescence role by increasing ACC phosphorylation and enhancing mitochondrial lipolysis ability (90). AMPK, the upstream negative regulator of ACC, also shows some anti-senescence activity.
2.2.2. AMP-activated protein kinase
Lipid metabolism depends heavily on AMPK. AMPK enhances lipolysis by upregulating ATGL expression (91). AMPK can also suppress lipid production by inhibiting ACC activation by phosphorylating ACC1 (Ser 79 Ala) and ACC2 (Ser 212 Ala) (92) and downregulating SREBP1c expression (93, 94). AMPK affects organism senescence directly or indirectly in a variety of ways. For example, dietary restrictions delay the senescence of many species, in which AMPK is widely involved. In C. elegans, AMPK and dietary restriction increase FAO through mitochondrial-peroxisome coordination, thereby maintaining homeostasis and plasticity within the mitochondrial network to extend life (95). Greer et al. (96) also reported that the lifetime extension due to food restriction in C. elegans was mediated through the AMPK-FoxO (transcription factors) pathway. AMPKα subunit AAK-2 is activated by either a mutation that reduces insulin-like signaling or an environmental stressor that increases the AMP:ATP ratio, which can extend lifespan in C. elegans (97, 98). A similar situation exists in mice. For instance, the pharmacological stimulation of AMPK can imitate caloric restriction (induced comparable gene expression patterns) and provide extensive protection against age-related diseases (99). Fibroblast growth factor 21 can exhibit anti-senescence effects by elevating the expression of AMPK, which regulates lipid and glucose metabolic balance (100), blocking the p53 signaling pathway in an AMPK-dependent manner (101). As an AMPK agonist, metformin plays a dual role in cancer prevention and anti-senescence (102, 103). Fruit flies’ lifespans also increased by overexpressing AMPK (104).
AMPK also regulates the nuclear factor kappa-light-chain-enhancer of activated B cells (NF-κB) inflammatory pathway (105), mammalian target of rapamycin (mTOR) C1 autophagy-related protein, and PGC-1α, P53/HIF-1α senescence-related signaling to achieve anti-senescence effects (106, 107). In detail, by phosphorylating mTORC1, ULK1, and PIK3C3/VPS34 complexes, AMPK directly stimulates autophagy (108). Inhibiting mTOR signaling plays a crucial role in mammalian senescence by reducing the accumulation of protein toxicity and oxidative stress (99), increasing autophagy to clear damaged proteins and organelles (109), and enhancing the self-renewal capacity of hematopoietic stem cells (110) and intestinal stem cells (111). Metformin can reduce cartilage degeneration in senescence-related osteoarthritis models by modulating AMPK/mTOR (112).
2.3. Ketone bodies and senescence
Ketone bodies are endogenous metabolites produced by the liver from fatty acids and ketogenic amino acids when glucose availability is low, such as during fasting, caloric restriction, or prolonged exercise (113). Ketone bodies can be used as an alternative fuel source by many tissues, especially the brain, heart, and skeletal muscle, when glucose is scarce or absent (114). These bodies can also cross the blood–brain barrier and supply energy to the central nervous system.
Ketone bodies are scary to clinicians because of the high mortality rate of ketoacidosis; however, in reality, the ketone bodies have signaling functions that regulate inflammation, epigenetics, oxidative stress, and other cellular processes by binding to specific receptors and enzymes (115–117). The bodies may also modulate gene expression and protein synthesis. Ketone bodies are linked to multiple mechanisms of senescence and resilience, such as glucose sparing, mitochondrial biogenesis, autophagy, and hormesis (118–121); thus, they have anti-cancer, anti-angiogenic, and anti-atherogenic effects. Ketone bodies also reduce neuroinflammation and β-amyloid and tau accumulation, and improve memory and healthy lifespan in a senescent mouse model (114).
The most well-known ketone body, 3-hydroxybutyrate (3-OHB), binds to specific hydroxycarboxylic acid receptors to inhibit histone deacetylases, free fatty acid receptors, and nucleotide oligomerization domain (NOD)-like receptor protein 3 inflammasomes. This initially inhibits lipolysis, inflammation, oxidative stress, cancer growth, angiogenesis, and atherosclerosis, and may increase lifespan associated with exercise and caloric restriction (122). In addition to helping treat other neurological conditions including dementia, the ketone body/ketogenic diet has been successfully utilized to reduce seizures (123). Additionally, 3-OHB protects muscle proteins from damage caused by systemic inflammation and is a crucial part of the metabolic defense against insulin-induced hypoglycemia (124).
2.4. Phospholipids and senescence
Phospholipids are major components of biological membranes and consist of a glycerol backbone, two fatty acid chains, and a polar head group. Phospholipids are essential for membrane structure, fluidity, and function, as well as for intracellular signaling, trafficking, and metabolism (125). Phospholipids are synthesized in different cellular compartments by various enzymes and transported by specific carriers or vesicles. Phospholipids mainly include phosphatidylcholine, phosphatidylethanolamine, phosphatidylserine, phosphatidylinositol, phosphatidic acid, and cardiolipin. Phospholipids can also be degraded or modified by phospholipases, acyltransferases, and other enzymes that regulate their turnover and diversity (126).
Phospholipids play important roles in senescence and age-related diseases. Senescence is associated with changes in phospholipid composition, metabolism, and transport in different tissues and organs. These changes may affect membrane integrity, fluidity, and function, as well as cellular signaling and homeostasis (127, 128). For example, senescence leads to decreased unsaturated fatty acid levels and increased saturated fatty acid levels in phospholipids, which may impair membrane fluidity and increase oxidative stress (129). Senescence also alters the levels of specific phospholipid species, such as phosphatidylcholine, phosphatidylethanolamine, and cardiolipin, which may affect mitochondrial function and biogenesis (130).
Phospholipid interventions may have beneficial effects on senescence and lifespan. Dietary supplementation or genetic manipulation of phospholipids or their precursors can modulate membrane properties, cellular signaling, and mitochondrial function in various model organisms. For instance, phosphatidylcholine or choline supplementation improved cognitive function and memory in aged rodents (131). Overexpression of cardiolipin synthase or supplementation of cardiolipin precursors can enhance mitochondrial function and extend lifespan in yeast, worms, flies, and mice (132).
2.5. Sphingolipids and senescence
Sphingolipids are a diverse class of lipids that are involved in various cellular processes such as membrane structure, signal transduction, cell cycle regulation, apoptosis, senescence, and inflammation (133). Sphingolipids are synthesized de novo in the endoplasmic reticulum from non-sphingolipid precursors, such as serine and palmitoyl-CoA, and then further modified in the Golgi apparatus and other organelles to generate a variety of complex sphingolipids with different polar head groups (134). The major bioactive sphingolipids include ceramide (Cer), sphingosine, sphingosine-1-phosphate (S1P), and ceramide-1-phosphate, which can act as second messengers or ligands for specific receptors to modulate cellular responses (135).
Sphingolipids have been implicated in the regulation of senescence and age-related diseases, as they can affect several hallmarks of senescence such as genomic instability, telomere attrition, epigenetic alterations, loss of proteostasis, deregulated nutrient sensing, mitochondrial dysfunction, cellular senescence, stem cell exhaustion, and altered intercellular communication (136). In general, Cer and its derivatives induce cellular senescence and promote senescence phenotypes, while S1P and its receptor signaling delay senescence and extend lifespan (137). For example, sphingolipids are involved in sarcopenia, an age-related disorder of loss of skeletal muscle mass and function. Park et al. (133) recently reported that Cer and glucosylceramide accumulate in the skeletal muscle of a senescent mouse model and that deleting serine palmitoyltransferase (SPT), the rate-limiting enzyme for sphingolipid biosynthesis, or using the SPT inhibitor myriocin to inhibit sphingolipid synthesis prevents sarcopenia and improves muscle health. In addition, SPT deletion or myriocin treatment enhances mitochondrial function, autophagy, and proteostasis in aged muscle cells, suggesting that sphingolipids may impair these processes by affecting endoplasmic reticulum stress and calcium homeostasis.
2.6. Sterols and senescence
Sterols are essential components of eukaryotic cell membranes, where they regulate membrane fluidity, permeability, and microdomain formation. Sterols also have important roles in animal physiology, as they are precursors of steroid hormones such as glucocorticoids, mineralocorticoids, androgens, estrogens, and progestins, which modulate various metabolic, reproductive, and immune functions (138). Sterols are synthesized from acetyl-CoA through a complex pathway that involves more than 20 enzymes and intermediates (139). The first steps of sterol biosynthesis occur in the cytosol and endoplasmic reticulum, where acetyl-CoA is converted to mevalonate by three enzymes: acetoacetyl-CoA thiolase, HMG-CoA synthase, and HMG-CoA reductase. Mevalonate is then converted to isopentenyl pyrophosphate (IPP) and dimethylallyl pyrophosphate (DMAPP), which are the building blocks of isoprenoids. IPP and DMAPP are used to synthesize geranyl pyrophosphate, farnesyl pyrophosphate, and squalene, which are the precursors of sterols and other isoprenoids such as ubiquinone, dolichol, and prenylated proteins (140). The final steps of sterol biosynthesis involve the cyclization of squalene to form lanosterol, which is then converted to cholesterol by a series of modifications such as hydroxylation, demethylation, reduction, and isomerization. Take cholesterol, the predominant sterol in animals, for example, which is integral and indispensable to neuronal physiology during both development and adulthood. As a major component of cell membranes and a precursor to steroid hormones, it helps regulate ion permeability, cell shape, intercellular interactions, and transmembrane signaling. Inherited diseases with mutations in cholesterol-related genes lead to impaired brain function. In these cases, brain cholesterol defects may be secondary to pathogenic factors and lead to functional deficits through altered synaptic function. Defects in brain cholesterol metabolism may lead to neurological syndromes such as AD, Huntington’s disease, and Parkinson’s disease, and even cognitive deficits (141–143).
3. Glucose metabolism and senescence
3.1. Glycolysis
The Warburg effect reveals that tumor cells prefer ATP production by glycolysis over oxidative phosphorylation even in the presence of abundant oxygen (144). The Warburg effect plays an important role in the inhibition of cellular senescence and tumor promotion (145). Glycolysis is upregulated in most senescence phenotypes. Glycolytic gene overexpression promotes altered cancer metabolism in a variety of tumor cells. LDHA, involved in the conversion of pyruvate to lactate, was significantly increased in radiotherapy-induced senescent HCT-116 and MDA-MB-231 cells (146, 147). Lactate liberation acidulates the extracellular environment involved in SRSPs (146). Lactic acid-induced acidic intracellular pH activates Snail to promote epithelial-mesenchymal transition. Increased Snail expression facilitated lung cancer cells to escape oncogene-induced senescence by directly suppressing p16INK4a expression (21). In this way, LDHA expression in senescent fibroblasts promotes PC3 cell invasion in the co-culture of senescent fibroblasts and PC3 cells (148). Pharmacological inhibition of LDHA induces tumor cell senescence by suppressing heat shock response (149). Aurora-A, a serine/threonine kinase, can directly bind to the transcription factor SOX8 to promote the expression of glycolytic and senescence genes, including LDHA, HK2, P16, and hTERT in cisplatin-resistant ovarian cancer cells (150). Consequently, radiotherapy/chemotherapy-induced senescent cancer cells exhibit glycolytic vulnerability.
Beyond radiotherapy- and chemotherapy-induced senescent cancer cells, glycolysis is also activated in non-neoplastic senescent cells. The levels of intermediate metabolites of glycolysis, including 3-phosphoglycerate, glucose 6-phosphate, fructose 6-phosphate, and phosphoenolpyruvate, are significantly increased in senescent fibroblasts, which highlights increased glycolytic flux to promote pyruvate and lactate production (151). Senescent spermatogonial stem cells exhibit JNK phosphorylation and enhanced glycolytic capacity compared with young cells (152). Senescence fibroblasts exhibit a significant increase in glucose consumption (18). Increased glycolysis appears to be mediated by the overexpression of multiple glycolytic enzymes in distinct kinds of induced senescence. First, GLUT proteins are encoded by SLC2. GLUTs 1–4 have widely established roles as glucose transporters (153). GLUT1 is overexpressed in aged lungs and regulates fibrogenesis (154). In this way, hyperglycemia-induced GLUT1 overexpression activates the mTOR pathway to increase P16 and P21 levels while promoting macrophage establishment of the SRSP response (16). Second, HKs catalyze the phosphorylation of glucose as the first rate-limiting step in glycolysis (155). HK1 and HK2 (154) isozymes are highly expressed in replicative senescence human fibroblasts (18). Third, PK, the rate-limiting enzyme in the final step of glycolysis, catalyzes pyruvate production. The levels of this enzyme are upregulated in replicative senescence fibroblasts due to increased TCA activity and oxygen consumption (156). In conclusion, glycolysis is increased in replicative senescent cells, but the regulatory mechanism of elevated enzyme expression and activity needs to be further characterized.
3.2. TCA cycle
Tumor cells maintain cell proliferation by activating glycolysis to provide energy and precursors. Pyruvate, a glycolytic terminal product, enters the TCA cycle to produce metabolic intermediates that inhibit cellular senescence (157). Citrate is the product catalyzed by citrate synthase in the initiation of the TCA cycle. Recently, citrate has been reported to extend lifespan through mTOR and AMPK. However, citrate treatment results in cellular senescence and inhibits the proliferation of MCF-7 and HCT116 cancer cells (26). Mechanistically, DNA damage pathways coupled with MAPK and mTOR pathways lead to citrate-induced cellular senescence (26). αKG, a metabolite catalyzed by isocitrate dehydrogenase (IDH), extends the lifespan of C. elegans (23), Drosophila (158), and mice (22); attenuates mouse age-related bone loss (24); and delays age-related fertility decline in mammals (159). Sustaining this biological activity requires a continuous supply of ATP from mitochondria; however, the partial inhibition of the electron transport chain by αKG reportedly extends the lifespan of C. elegans and mammalian cells (23). Mechanistically, αKG inhibits intracellular senescence by regulating the expression of histone epigenetic modifications of BMP signaling proteins (24). In addition, αKG prolongs Drosophila lifespan by activating AMPK and inhibiting mTOR (158). The IDH1 R132H mutant indirectly promotes senescence of malignant glioma cells by reducing αKG production (160).
3.3. Pentose phosphate pathway
Abnormal glucose metabolism in cancer cells activates arrested cellular senescence. Cancer cells not only show active glycolysis for sufficient energy but also precursors from the pentose phosphate pathway (PPP) for intracellular biosynthesis (161). G6PD-mediated oxidative PPP (oxPPP) provides NADPH to counteract oxidative stress, while non-oxPPP generates ribose-5-phosphate (R5P) to provide precursors for nucleotide synthesis (161). G6PD, a unique rate-limiting enzyme in the PPP, is involved in counteracting cellular senescence. Mechanistically, G6PD knockdown increases the levels of P21, a classical marker of senescence, which promotes HCC and HCT116 cell senescence (162). In addition, TAp73 enhances cell cycle inhibitory protein P21 by regulating metabolism (163). TAp73 directly activates G6PD to increase PPP flow to inhibit cancer cell senescence (164). Thus, G6PD may mediate, at least in part, the regulation of the senescence phenotype of cancer cells by TAp73. G6PD also regulates cellular senescence by affecting telomerase activity (165, 166). G6PD-deficient fibroblasts exhibit delayed growth and accelerated senescence. Ectopic expression of the human telomerase reverse transcriptase hTERT activated telomerase activity to prevent cellular senescence in G6PD-deficient fibroblasts (166). Thus, the knockdown of hTERT significantly reduces G6PD expression and telomerase activity to promote cancer cell senescence (165). 6-phosphogluconate dehydrogenase (6PGD) is a key enzyme in oxPPP. Pharmacological inhibition of 6PGD induces cellular senescence and MCF-7 cell cycle arrest (167). Non-oxPPP also regulates cellular senescence. Supplementation of ribose 5-phosphate in drug-induced senescent human dermal fibroblasts significantly inhibited cell enlargement, a morphological alteration of cellular senescence (168). In addition, hTERT knockdown inhibited the expression and activity of transketolase, a key enzyme of non-oxppp; increased cancer cell senescence; and reduced tumor burden in a mouse model of heterotypic xenograft (165).
4. Lipid and glucose metabolism and SRSPs
SRSPs describe the diverse array of proinflammatory and profibrotic factors secreted by senescent cells (169). SRSPs include cytokines, chemokines, growth factors, proteases, and extracellular matrix components that can affect the surrounding tissue microenvironment and modulate various biological processes, including inflammation, immunity, tissue remodeling, and tumorigenesis (170). SRSPs are considered crucial drivers of chronic inflammation and senescence phenotypes, as they accumulate throughout normal senescence and in age-related diseases. SRSPs can have both beneficial and detrimental effects on the organism, depending on the expression context and duration. While SRSPs can promote wound healing, tissue repair, and immune surveillance by stimulating cell proliferation, angiogenesis, and immune cell recruitment (170), they can also induce cellular senescence in neighboring cells, disrupt tissue homeostasis and function, and facilitate the development and progression of age-related diseases such as cancer, neurodegeneration, cardiovascular disease, diabetes, and osteoporosis (171, 172).
Growing evidence has shown that lipid metabolism and SRSPs are interconnected at multiple levels. For instance, some lipids (such as ceramides, S1P, and prostaglandins) can modulate the expression or activity of key regulators of SRSPs, including p53, NF-κB, p38 MAPK, JNK, mTOR, IL-1α/β, and STAT3 (173–175). Conversely, some SRSP factors, such as IL-1β, IL-6, IL-8, and TNF-α, can affect lipid metabolism by altering lipogenic enzymes (e.g., FAS, ACLY, and ACC), lipolytic enzymes (e.g., ATGL, hormone-sensitive lipase [HSL], and MGL), lipid transport proteins (e.g., CD36 and FABP4), fatty acid oxidases (e.g., CPT1A, CPT2, and ACADL), and PPAR expression or activity to influence lipid metabolism (173, 176). SRSP factors can also regulate lipids by affecting key signaling pathways such as the phosphatidylinositol 3-kinase (PI3K)/Akt/mTOR and sphingosine kinase 1/S1P pathways.
Disturbances in glucose metabolism can also induce cellular senescence and SRSPs in various tissues, such as pancreatic beta cells, endothelial cells, neurons, astrocytes, myocytes, and adipocytes (177–179). Conversely, SRSPs can modulate its metabolic activity by altering the expression and activity of key metabolic enzymes and transcription factors such as AMPK (180), PGC-1α, sirtuin 1 (181), FoxO (182), NF-κB (183), and nuclear factor erythroid 2-related factor 2 (184). These factors can regulate various aspects of glucose metabolism such as glycolysis, gluconeogenesis, glycogen synthesis and breakdown, pentose phosphate pathway, and the hexosamine biosynthetic pathway. SRSPs can also impair glucose uptake and utilization in these tissues by interfering with insulin signaling and inducing insulin resistance (185).
5. Apoptosis, autophagy, and senescence
Apoptosis and autophagy are two major forms of programmed cell death that play important roles in senescence and age-related diseases. Apoptosis is a regulated process of cell elimination that involves the activation of caspases, cleavage of cellular substrates, and formation of apoptotic bodies that are phagocytosed by macrophages or neighboring cells (186). Autophagy is a catabolic process of self-digestion that involves the formation of double-membrane vesicles called autophagosomes, which engulf cytoplasmic components and deliver them to lysosomes for degradation (187). Both apoptosis and autophagy are essential for maintaining cellular homeostasis, tissue integrity, and organismal health, as they remove damaged or redundant cells and organelles, recycle nutrients, and regulate inflammation and immunity (188). However, both processes also decline with senescence, leading to the accumulation of dysfunctional cells and organelles, oxidative stress, chronic inflammation, and impaired tissue repair and regeneration (189).
The relationship between apoptosis and autophagy in senescence is complex and context-dependent. On the one hand, apoptosis and autophagy can cooperate or compensate for each other to maintain cellular quality control and prevent senescence or tumorigenesis. For example, autophagy can remove damaged mitochondria that produce ROS and trigger apoptosis (190). Autophagy can also degrade pro-apoptotic factors or inhibit apoptotic signaling pathways, thus protecting cells from excessive or inappropriate cell death. On the other hand, apoptosis and autophagy can antagonize or compete to modulate cellular fate and function. For instance, apoptosis can inhibit autophagy by cleaving autophagy-related proteins or blocking autophagosome-lysosome fusion. Apoptosis can also induce autophagy as a survival mechanism or a secondary mode of cell death when caspase activation is impaired or overwhelmed (191).
The balance between apoptosis and autophagy in senescence is influenced by various factors, including genetic background, environmental stimuli, hormonal status, metabolic state, and disease conditions. Lipid and glucose metabolism are the two main pathways that provide energy and substrates for cellular function and survival. Dysregulation of lipid or glucose metabolism can affect the balance between apoptosis and autophagy. Lipid metabolism is regulated by various factors, including hormones, nutrients, and oxygen levels. Lipid metabolism can modulate apoptosis and autophagy by affecting the production of ROS, activation of signaling pathways, and formation of lipid droplets or membrane structures (192). For example, excessive lipid accumulation can induce oxidative stress and ER stress, which can trigger apoptosis or autophagy by activating the JNK, p53, or PKR-like endoplasmic reticulum kinase (PERK) pathways (193–195). Conversely, lipid depletion can also induce apoptosis or autophagy by impairing mitochondrial function, reducing ATP levels, or activating the AMPK pathway (196, 197). Moreover, lipid metabolism can influence autophagic membrane initiation and elongation by providing phospholipids or fatty acids as precursors or modulators (198). Lipid metabolism can be regulated by autophagy through the degradation of lipid droplets or lipogenic enzymes in a process called lipophagy (199).
Similarly, glucose metabolism can regulate apoptosis and autophagy by affecting the production of ROS, activation of signaling pathways, and maintenance of energy homeostasis. For example, high glucose levels can induce oxidative stress and ER stress, which can trigger apoptosis or autophagy by activating the p38 MAPK, NF-κB, or CHOP pathways (200–202). Conversely, low glucose levels can also induce apoptosis or autophagy by impairing mitochondrial function, reducing ATP levels, or activating the AMPK pathway (203). Moreover, glucose metabolism can influence autophagy induction and progression by providing hexosamines or acetyl-CoA as regulators (204, 205). Furthermore, glucose metabolism can be regulated by autophagy through the degradation of glycogen granules or glycolytic enzymes in a process called glycophagy (206).
6. Insulin signaling
The relationship between insulin signaling and glucose and lipid metabolism is essential for maintaining energy homeostasis and preventing metabolic diseases. Insulin stimulates glucose uptake, utilization, and storage by activating the PI3K/Akt pathway and downstream targets such as GLUT4, glycogen synthase, and HK (207). Insulin also stimulates lipid synthesis and storage by activating the same pathway and downstream targets such as SREBPs, ACC, and FAS (208). Insulin also inhibits glucose production by suppressing the expression and activity of enzymes involved in gluconeogenesis, such as phosphoenolpyruvate carboxykinase (PEPCK) and glucose-6-phosphatase (G6Pase), or lipolysis, such as HSL and ATGL (209). However, insulin signaling and glucose and lipid metabolism can be disrupted by various factors such as obesity, inflammation, oxidative stress, endoplasmic reticulum stress, and senescence. These factors can impair insulin action and induce insulin resistance by interfering with insulin receptor function or downstream signaling components. For instance, oxidative stress can reduce insulin receptor tyrosine phosphorylation and Akt activation by increasing the activity of protein tyrosine phosphatase 1B or protein kinase C (PKC) (210). Inflammation can also impair insulin signaling by inducing the production of pro-inflammatory cytokines such as TNF-α and IL-6, which can activate serine/threonine kinases such as JNK or inhibitor of nuclear factor κB kinase subunit β (IKKβ), that can phosphorylate insulin receptor substrate 1 (IRS-1) on serine residues and inhibit its tyrosine phosphorylation (211). Mitochondrial dysfunction can impair lipid oxidation and increase lipid accumulation in non-adipose tissues such as skeletal muscle or liver, which can cause lipotoxicity and impair insulin action by activating PKC, JNK, or IKKβ (212). Endoplasmic reticulum stress can also induce insulin resistance by activating the unfolded protein response (UPR), which can suppress insulin receptor expression or activate JNK or PERK, which can phosphorylate IRS-1 on serine residues and inhibit its tyrosine phosphorylation (213). Conversely, senescence can also affect insulin signaling and glucose and lipid metabolism by reducing the expression or activity of insulin receptors or their substrates, increasing the levels of inflammatory cytokines or oxidative stress markers, impairing mitochondrial function or autophagy, or altering the composition or function of gut microbiota.
Several transcription factors play pivotal roles in modulating insulin action and lipid homeostasis in response to senescence. For example, FoxO transcription factors, which are downstream targets of the PI3K/Akt pathway, have been implicated in regulating glucose and lipid metabolism, oxidative stress resistance, inflammation, autophagy, and lifespan in various organisms (214). FoxO factors can induce the expression of genes involved in gluconeogenesis such as PEPCK and G6Pase, or genes involved in FAO such as CPT1 or ACOX, thereby antagonizing the effects of insulin on glucose and lipid metabolism (215). FoxO factors can also induce the expression of genes involved in antioxidant defense such as superoxide dismutase or catalase, or genes involved in anti-inflammatory responses, such as IL-10 or suppressor of cytokine signaling 3, thereby protecting against oxidative stress and inflammation induced by senescence (216). FoxO factors can also induce the expression of genes involved in autophagy, such as LC3 or Atg12, thereby promoting the clearance of damaged organelles or proteins accumulated during senescence (217). Moreover, FoxO factors can regulate lifespan by modulating the activity of the insulin/insulin-like growth factor signaling (IIS) pathway or other longevity pathways, such as sirtuins or mechanistic/mTOR (218).
7. Environmental factors affecting senescence
7.1. Nutrition
Glucose is one of the main sources of cellular energy. Excessive glucose intake and abnormally elevated circulating blood glucose levels are associated with chronic diseases such as obesity and diabetes. On the one hand, the metabolic changes caused by hyperglycemia and diabetes promote cellular senescence, leading to tissue dysfunction and various complications such as diabetic retinopathy. Pancreatic β-cells sense elevated blood glucose levels and secrete insulin correspondingly to maintain blood glucose levels within a narrow range. Glycolysis is increased in diabetic β-cells, which inhibits β-cell function (219). Increased numbers of SA-β-gal+ cells in the pancreas of healthy older adults compared with younger adults and further increases in the number of SA-β-gal+ cells in the pancreas of patients with type 2 diabetes (T2D) compared with non-diabetic patients suggest that β-cell senescence may contribute to T2D pathogenesis (220). A diabetic environment and SRSPs are considered drivers of diabetic retinopathy senescence in vitro and in vivo. High glucose exposure accelerates cellular senescence (20). High concentrations of 25 mM glucose in culture accelerated the senescence of human retinal microvascular endothelial cells. However, glycolysis was significantly reduced rather than increased in an in vitro model of senescence. The presence of a negative feedback regulatory mechanism in an in vitro model may reduce levels of the glucose transport proteins GLUT1 and GLUT3 and the glycolytic enzyme PFKFB3, affecting the rate of glucose transport (221). On the other hand, cellular senescence may also affect insulin secretion and sensitivity, thereby increasing the risk of developing diabetes. Adipose precursor cells affect neighboring non-senescent cells through the secretion of SRSP factors such as activin A, IL-6, and TNFα, leading to impaired adipogenesis and reduced insulin sensitivity, which may also be involved in T2D progression (222).
Abnormal lipid accumulation during senescence is mainly caused by increased fatty acid uptake, de novo lipogenesis, and decreased FAO processes. Mitochondrial integrity and autophagy induction are also diminished during senescence, leading to decreased lipolysis. These changes further impart lipotoxicity to the cell, depleting energy in the tissues and altering cellular signaling, thereby accelerating senescence and the early onset of age-related diseases (223). Multiple adipogenesis enzymes are upregulated in senescent hepatocytes, including FAS, ACC, and stearoyl-CoA desaturase (SCD) (224). In addition to lipid synthesis, excessive lipid intake increases FAO in the mitochondria and the ROS produced, leading to mitochondrial DNA damage and mitochondrial dysfunction (225). Excessive lipid intake inhibits autophagy activation signaling and reduces autophagy levels. Reduced autophagy levels lead to the accumulation of large amounts of waste products and toxins in the cell, interfering with cellular function and homeostasis and accelerating cellular senescence (226). Excess saturated fatty acids activate the NF-κB and JNK signaling pathways by binding to Toll-like receptor 4 on the cell surface, thereby inducing the expression and release of inflammatory factors (227, 228).
7.2. Stress
Stress can impair glucose and lipid metabolism by altering the levels of insulin, leptin, cortisol, and other hormones that modulate appetite, energy expenditure, and glucose uptake (229). Stress can also induce lipotoxicity, which is the accumulation of excess lipids and their metabolites in non-adipose tissues, such as the liver, muscle, heart, and brain (230). Lipotoxicity can cause cellular damage, inflammation, insulin resistance, and apoptosis, leading to metabolic disorders and age-related diseases (230). Moreover, stress can affect the mitochondrial function and autophagy of cells, which are involved in glucose and lipid metabolism (231). Mitochondria are organelles that produce energy from glucose and fatty acids, while autophagy is the process that degrades damaged or excess cellular components, such as lipids. Stress can induce mitochondrial stress, which is the imbalance between mitochondrial biogenesis and degradation, resulting in mitochondrial dysfunction, oxidative stress, and impaired energy metabolism (231). Stress can also modulate autophagy, which can have both beneficial and detrimental effects on senescence depending on the stress type and duration. Autophagy can enhance stress resistance and protein homeostasis by removing damaged mitochondria and lipids, but it can also promote cell death and inflammation by activating ferroptosis, a form of iron-dependent lipid peroxidation.
7.3. Environment pollution
Environmental pollution, especially air pollution, can expose the body to various harmful substances, such as particulate matter, ozone, nitrogen dioxide, and polycyclic aromatic hydrocarbons. These substances can induce oxidative stress, inflammation, and endothelial dysfunction, which can impair glucose and lipid metabolism (232–234). Oxidative stress can damage DNA, proteins, and lipids, leading to cellular senescence and apoptosis (234). Inflammation can alter the levels of cytokines, such as IL-6 and TNF-α, which can affect the secretion and action of insulin, a hormone that regulates glucose uptake (233).
7.4. Temperature
Glucose and lipid metabolism are regulated by various hormones, enzymes, and signaling pathways that respond to temperature changes, such as thermogenesis, cold exposure, or heat stress (223, 235, 236). Temperature can modulate glucose and lipid metabolism by altering the levels of adipokines, thyroid hormones, catecholamines, and other hormones that modulate energy balance, thermoregulation, and glucose uptake. Temperature can also affect lipid lipolysis and oxidation, which are the processes that break down and utilize lipids for energy. Lipolysis is the hydrolysis of triglycerides into fatty acids and glycerol, while oxidation is the conversion of fatty acids into acetyl-CoA, which is used by the Krebs cycle. Temperature can induce lipolysis and oxidation by activating HSL, ATGL, and CPT, which are enzymes that catalyze lipid breakdown and transport. Temperature can also affect the glycerolipid metabolism and signaling of cells, which are involved in glucose and lipid metabolism (223). Therefore, temperature can influence senescence through multiple aspects of glucose and lipid metabolism that affect cellular health and function (Figure 3).
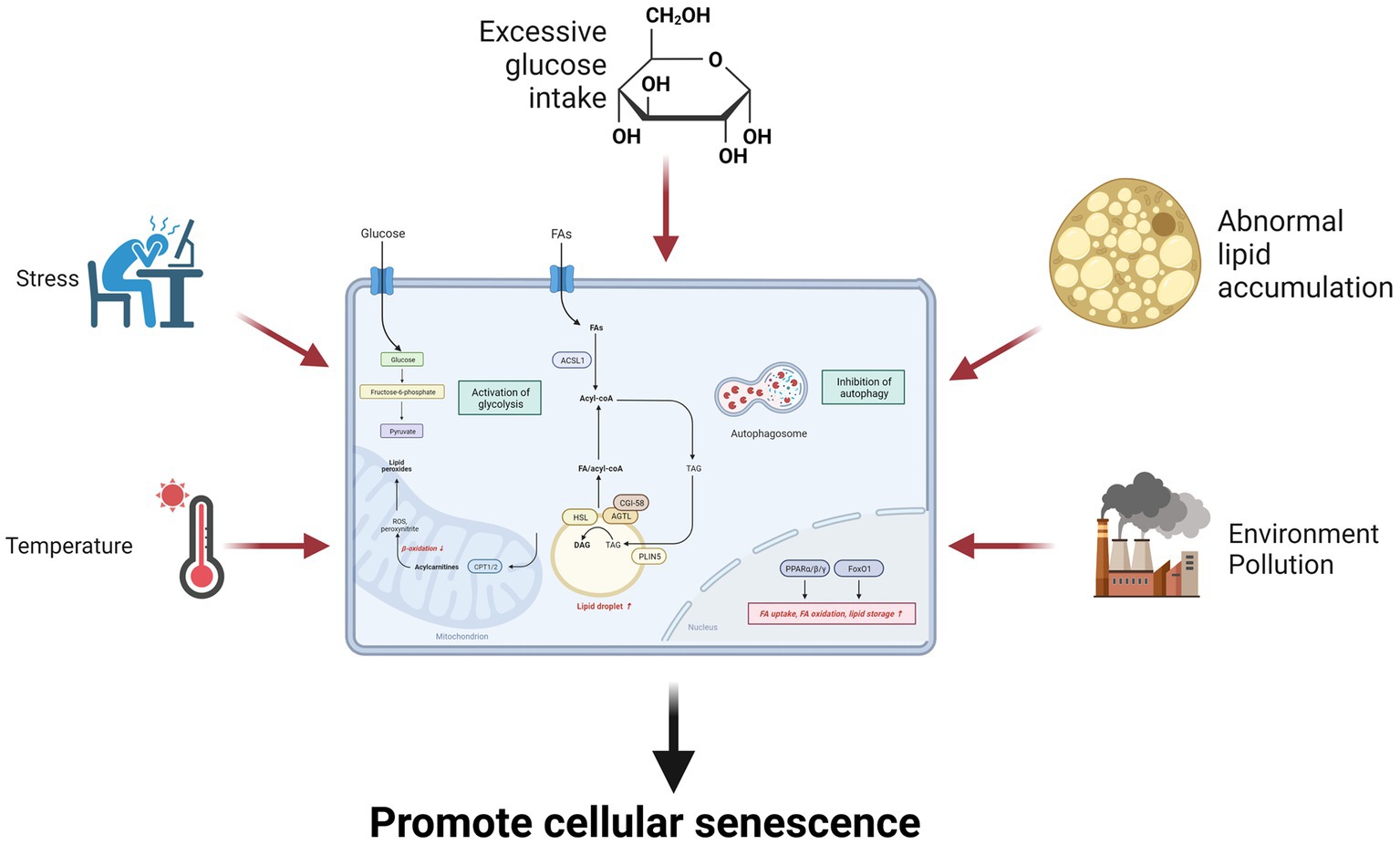
Figure 3. Environmental factors influence senescence through glucose and lipid metabolism. Environmental factors such as excessive glucose intake, lipid overaccumulation, stress, environmental pollution, and temperature can promote cellular senescence by increasing glycolysis and inhibiting fatty acid oxidation.
8. Abnormal senescence
8.1. Premature ovarian insufficiency
Premature ovarian insufficiency (POI) is the condition of ovarian function decline leading to amenorrhea for over a year before 40 years of age (237), which may be associated with genetic, medical, immunological, environmental, and other factors. POI is associated with metabolic disturbances, including glucose and lipid metabolism, which may increase the risk of long-term complications, such as cardiovascular diseases and osteoporosis (238). Glucose metabolism is regulated by insulin. Estrogen, the main ovarian hormone, modulates insulin sensitivity and glucose homeostasis by influencing insulin secretion, signaling, and action in various tissues. Estrogen deficiency in POI may impair glucose metabolism and lead to insulin resistance, hyperglycemia, or diabetes mellitus (239). Estrogen also affects lipid metabolism by regulating the expression and activity of enzymes and receptors involved in lipid synthesis, transport, and degradation (240). Zhou et al. (238) found that patients with POI had higher levels of plasma glucose, insulin, Homeostatic Model Assessment for Insulin Resistance (HOMA-IR, a marker of insulin resistance), plasma triglycerides, total cholesterol, low-density lipoprotein cholesterol, and apolipoprotein B compared with healthy controls. Moreover, they identified several metabolites related to glucose metabolism and lipid metabolism that were altered in patients with POI, including lactate, pyruvate, citrate, carnitine, acylcarnitine, glycerol, and glycerophospholipids. In conclusion, POI affects not only ovarian function but also metabolic health. POI patients may have impaired glucose and lipid metabolism due to estrogen deficiency, which may increase their risks of developing metabolic disorders or cardiovascular diseases. Therefore, it is important to monitor and manage the metabolic status of POI patients and provide appropriate hormonal replacement therapy or lifestyle interventions to prevent or reduce the adverse outcomes associated with POI.
8.2. Platelet senescence
Platelets are cell fragments without nuclei that participate in hemostasis and thrombosis. The lifespan of platelets is usually 7–10 days, and as age increases, the quantity and function of platelets decrease, resulting in increased bleeding propensity (241). The levels of lipid peroxides in platelets increase during senescence. Lipid peroxidation is important for cell function. It causes extensive damage to cell membranes and subcellular granules, leading to enzyme inactivation and destruction, and inhibition of metabolic pathways and cell division. The accumulation of lipid peroxidation products in aged platelets may cause cumulative damage to the membrane structure of platelets and their subcellular granules (such as alpha granules, mitochondria, and the endoplasmic reticulum). Lipid peroxidation may directly affect metabolic pathways by reducing the activity of lipid-dependent enzymes such as NADH- or succinate-cytochrome c reductase or indirectly by releasing lysosomal enzymes from alpha granules (242). Platelets have a rich glycocalyx on their surface, and platelet glycosylation plays an important role in physiological hemostasis mechanisms, regulating platelet-receptor protein interactions, and dynamically remodeling surface glycosylation through their own glucose metabolism system. Platelet glycosylation also participates in platelet senescence and clearance to regulate platelet count; meanwhile, platelet glycosylation abnormalities are closely related to primary immune thrombocytopenia, coronary heart disease, and related diseases, and are potential targets for anti-platelet therapy (243).
8.3. Down syndrome
Down syndrome (DS) is a genetic disorder caused by an extra copy of chromosome 21, which results in cognitive impairment, physical abnormalities, and increased risks of age-related comorbidities. Individuals with DS typically exhibit premature senescence phenomena, including skin senescence, graying hair, cataracts, osteoporosis, atherosclerosis, immune decline, and memory loss (244). Likewise, the glucose and lipid metabolism of patients with DS also changes, mainly manifesting as reduced serum cholesterol levels, increased triglyceride levels, increased insulin resistance, abnormal glucose tolerance, etc. These changes may be related to genetic abnormalities, oxidative stress, mitochondrial dysfunction, and other factors in these patients. Moreover, many changes occur in the brain lipids of patients with DS, including a significant reduction in esterified polyunsaturated fatty acids, especially fatty acids esterified into phosphatidylinositol and phosphatidylserine.
9. Anti-cancer treatment and senescence
Lipid and glucose metabolism are two essential metabolic pathways that provide energy and building blocks for cancer cells. Cancer cells reprogram their lipid and glucose metabolism to support their rapid growth, survival, invasion, and resistance to therapy.
Cancer cells increase their lipid biosynthesis by upregulating enzymes such as FAS, ACC, and SCD, which are often driven by oncogenic signals such as KRAS, MYC, and PI3K/AKT (245, 246). Cancer cells also enhance their lipid uptake by expressing more lipoprotein receptors, such as low-density lipoprotein receptor (LDLR) and scavenger receptor class B type I (SR-BI), and lipid transporters, such as fatty acid transport proteins and FABPs (245, 247). Moreover, cancer cells can mobilize lipids from adipose tissue or surrounding stromal cells through lipolysis or lipophagy (245, 248). Lipids can be stored in lipid droplets or oxidized through mitochondrial β-oxidation to generate ATP and acetyl-CoA, which can fuel the TCA cycle and oxidative phosphorylation. Lipids can also modulate various signaling pathways, such as RAS, PI3K/AKT, and nuclear receptors, to regulate cell proliferation, survival, migration, and inflammation (245).
In response to this feature, ongoing efforts aim to adapt lipid metabolism as an anti-cancer drug. Some approaches are used in preclinical models in vitro and in vivo, and some drugs have entered clinical trials. Inhibiting lipid biosynthesis enzymes, such as FAS (TVB-2640) (249), ACC (Soraphen A) (250), and SCD (A939572) (251), can induce endoplasmic reticulum stress and activate the unfolded protein response, leading to cell cycle arrest, apoptosis, or senescence. By blocking lipid uptake receptors like LDLR (GW3965) (252) or SR-BI (ML279) (253), less cholesterol and other lipids are available for membrane production and signaling. This blocking can also impair mitochondrial beta-oxidation and increase the accumulation of toxic lipids, such as ceramide and ROS, by inhibiting the lipid oxidase CPT1 (etomoxir) (254). Inhibition of COX-2 or LPA receptors, lipid signaling molecules, modulates various pathways involved in cancer cell proliferation, survival, migration, angiogenesis, and inflammation (255, 256). Finally, cancer progression can also be inhibited by altering the biophysical properties (fluidity, curvature, and permeability) of the membrane bilayer through the localization and activity of membrane-associated proteins and receptors, as typified by statins that reduce cholesterol concentrations in the plasma membrane and affecting lipid raft clustering and displacement of receptors in non-raft domains (257).
The main pathway of glucose metabolism in cancer cells is aerobic glycolysis, also known as the Warburg effect (258). This is a phenomenon in which cancer cells preferentially convert glucose into lactate, even in the presence of oxygen and functional mitochondria. Aerobic glycolysis allows cancer cells to rapidly consume glucose and produce ATP while avoiding the production of ROS that can damage DNA and proteins. Moreover, aerobic glycolysis provides cancer cells with various metabolic intermediates that can be used for the synthesis of nucleotides, amino acids, and lipids (259). To support aerobic glycolysis, cancer cells increase their glucose uptake by overexpressing GLUTs, especially GLUT1 (260). Cancer cells also upregulate key glycolytic enzymes, such as HK2, phosphofructokinase 1, pyruvate kinase M2, and LDHA, which are often regulated by oncogenic signals, such as KRAS, MYC, and HIF1α (261). However, aerobic glycolysis is not the only mode of glucose metabolism in cancer cells. Some cancer cells can also use oxidative phosphorylation (OXPHOS) to generate ATP from glucose-derived pyruvate in the mitochondria. OXPHOS can be activated in cancer cells under conditions, such as hypoxia, nutrient deprivation, or drug resistance (262). OXPHOS is more efficient than glycolysis in terms of ATP production per glucose molecule but also generates more ROS. OXPHOS can also provide cancer cells with acetyl-CoA and NADH, which can modulate various signaling pathways, such as histone acetylation and sirtuin activity. To support OXPHOS, cancer cells can modulate their pyruvate metabolism by regulating the expression or activity of pyruvate dehydrogenase, pyruvate dehydrogenase kinase, or pyruvate carboxylase. Cancer cells can also use alternative substrates for OXPHOS, such as glutamine, fatty acids, or ketone bodies (261).
Cellular senescence can occur in both normal and cancer cells and has complex dependence effects on cancer development and treatment. On the one hand, cellular senescence is a cancer suppressor mechanism that prevents the proliferation of damaged or malignant cells. SRSPs secreted by senescent cells recruit and activate immune cells to remove senescent cells (263). Some immune cells, such as T helper 1 cells, can also trigger cancer cell senescence by secreting inflammatory cytokines. In addition, cellular senescence enhances the expression of cancer-associated antigens and immunogenic molecules on cancer cells, making them more susceptible to recognition and clearance by T cells. Thus, T cells are a key component of cancer immunotherapy (263). On the other hand, cellular senescence can also adversely affect T cell immunity and cancer therapy. First, senescent cells accumulate in cancers and normal tissues, releasing SRSPs with pro-tumorigenic effects such as promoting angiogenesis, invasion, metastasis, and drug resistance. Second, T cell senescence decreases their proliferation, cytokine production, and cytotoxicity and increases the expression of inhibitory receptors and pro-apoptotic molecules (264, 265).
Therefore, cellular senescence is a double-edged sword that can influence T cell immunity and cancer therapy in different ways. Understanding the molecular mechanisms and interactions between how sugar and lipid metabolism regulate T cell senescence could provide new insights and strategies for improving anti-cancer therapy. T cell metabolism is tightly linked to T cell activation, differentiation, and function. T cells undergo metabolic reprogramming upon antigen stimulation, switching from a quiescent state that relies on oxidative phosphorylation to a highly glycolytic state that supports rapid proliferation and effector functions (173). However, chronic or excessive stimulation can lead to T cell exhaustion or senescence, which are associated with metabolic dysregulation, such as impaired glucose uptake, reduced glycolytic capacity, and altered mitochondrial function (266). In addition to glucose metabolism, lipid metabolism plays a crucial role in regulating T cell immunity. Senescent T cells have reduced FAO capacity and increased dependence on FAS. Targeting FAS with pharmacological inhibitors or gene knockdown induces apoptosis in senescent non-functional T cells and improves anti-cancer immunity. Conversely, enhancing FAO with pharmacological activators or gene overexpression can prevent or reverse T cell senescence and exhaustion by restoring mitochondrial function and reducing oxidative stress (267).
10. Conclusion
The relationships between different types of lipid components, glucose metabolites, and senescence have been reported (268, 269). However, an in-depth study of the regulatory relationships of the key enzymes is necessary to screen anti-senescence drugs. New anti-cancer drugs may also be developed by targeting tumor cell senescence.
Various aspects of glucose and lipid metabolism are closely related to senescence. In lipid metabolism, decreased fatty acid catabolism is directly related to senescence onset, including mitochondrial β-oxidation (34, 36, 37, 57, 270), peroxisomal β-oxidation (54, 55), α-oxidation (82, 84), and ω-oxidation (85). The most prominent key enzymes include CPT1 and ACOX1. Drugs such as fenofibrate can exert anti-senescence effects by targeting PPARα, the gene upstream of CPT1 and ACOX1 (Figure 4). Meanwhile, increased fatty acid synthesis aggravates lipotoxicity, and both direct inhibition of ACC and indirect regulation via AMPK/SREBP1 can exert anti-senescence activity (89). In addition to fatty acid catabolism and synthesis, acetyl coenzyme A undergoes a variety of pathways to ketone bodies, phospholipids, sphingolipids, and sterols, which perform a wide range of biological functions and exert pro- or anti-senescence effects (Figure 5). The effects of these lipids on senescence often depend on the amount, subtype, and state of the cellular microenvironment. For example, appropriate levels of ketone bodies are beneficial for the central nervous system’s energy supply, maintenance of mitochondrial biogenesis, and attenuation of oxidative stress; however, excessive ketone bodies can lead to ketoacidosis and even death (118). Cer induces cellular senescence, whereas S1P, another sphingolipid component, delays senescence and extends lifespans (133, 137).
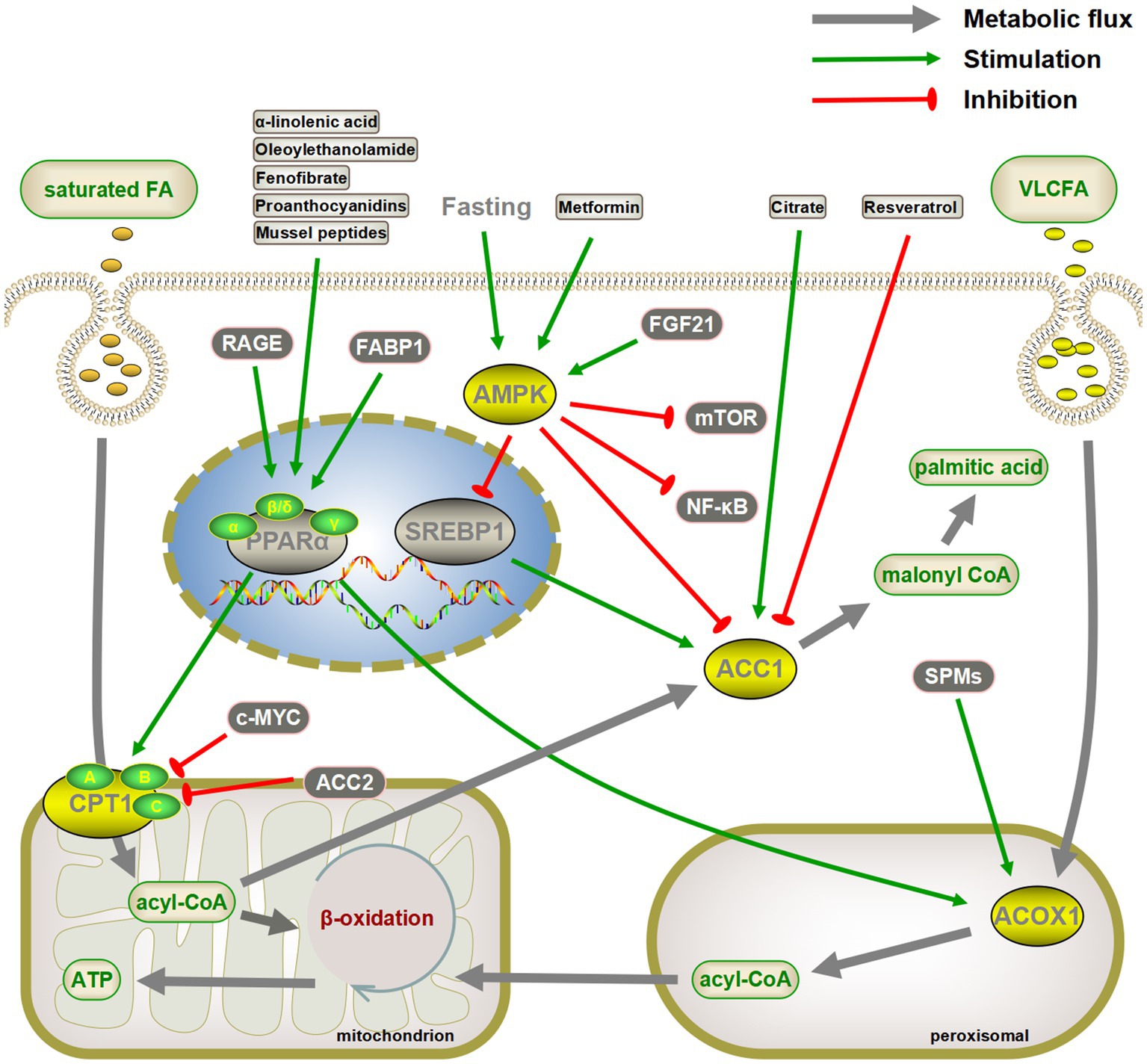
Figure 4. Targeting critical enzymes of fatty acid synthesis and catabolism to delay senescence. Activation of CPT1 and ACOX1 directly or indirectly through the peroxisome proliferator-activated receptor α (PPARα) pathway promotes fatty acid oxidation, both of which may have a senescence-delaying effect. Similarly, inhibiting fatty acid synthesis by the AMPK/SREBP1/ACC pathway is also beneficial in slowing down senescence. AMPK also inhibits senescence by inhibiting the mTOR and NF-κB pathways. The gray arrows represent normal metabolic pathways. The green pointed and blunt red arrows represent activating and inhibiting effects, respectively.
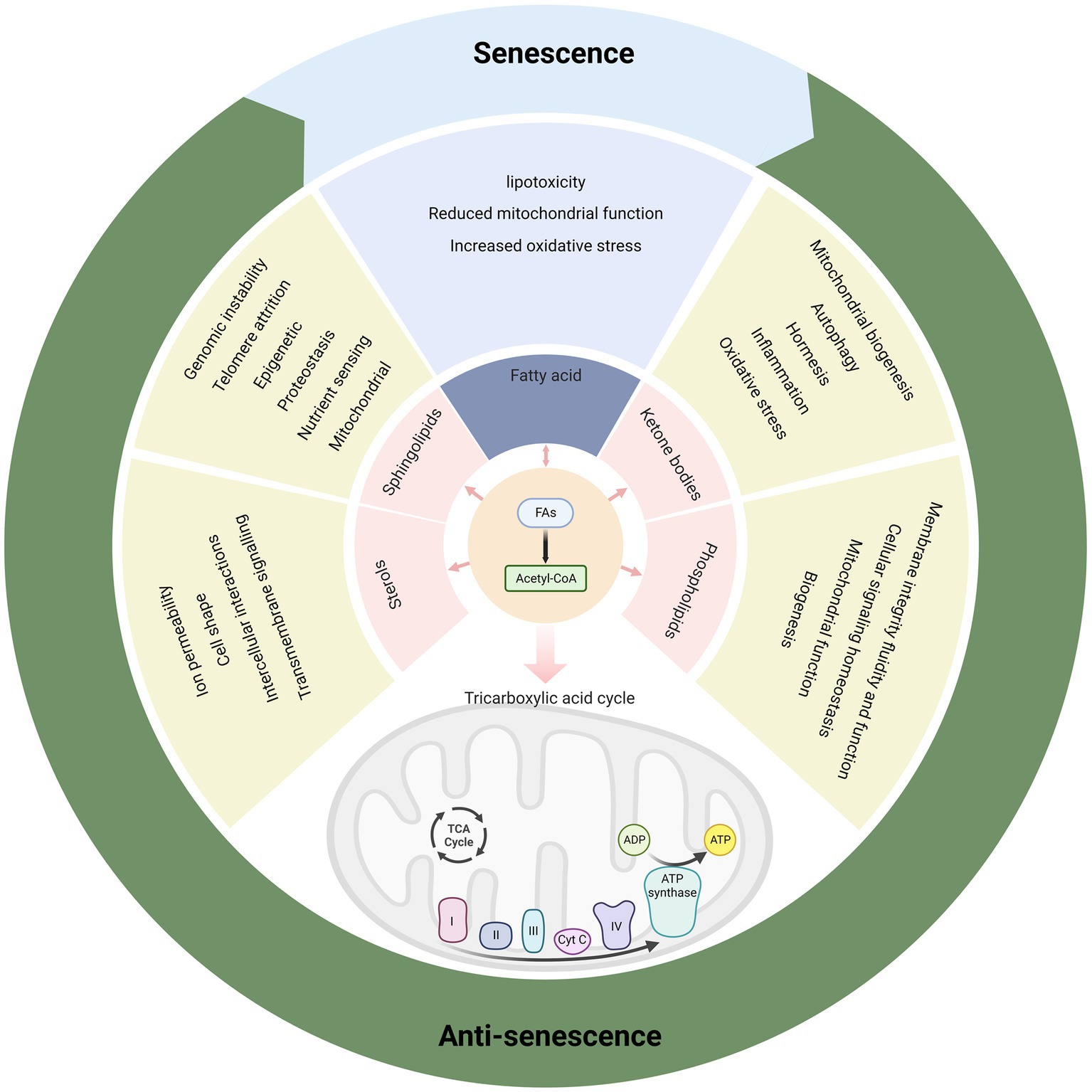
Figure 5. Various metabolic products of fatty acids have rich biological functions and affect senescence.
Multiple glucose metabolism pathways are involved in the regulation of intracellular senescence. Several rate-limiting enzymes in glycolysis, including HK2 and PK, are highly expressed in senescent cells (150, 156). Although the pharmacological activation of Nrf2 increases HK2 expression (18), other mechanisms require further investigation. Several key enzymes of the PPP, a branch of the glycolytic pathway, are also involved in the regulation of intracellular senescence. The key enzymes promote cellular senescence by upregulating P21, P16, and telomerase activity (163). Notably, the dietary TCA-cycling metabolites citrate and αKG delay cellular senescence (23–26). Therefore, supplementation of these metabolites may provide an effective intervention for the treatment of senescence-related dysfunction (Figure 6).
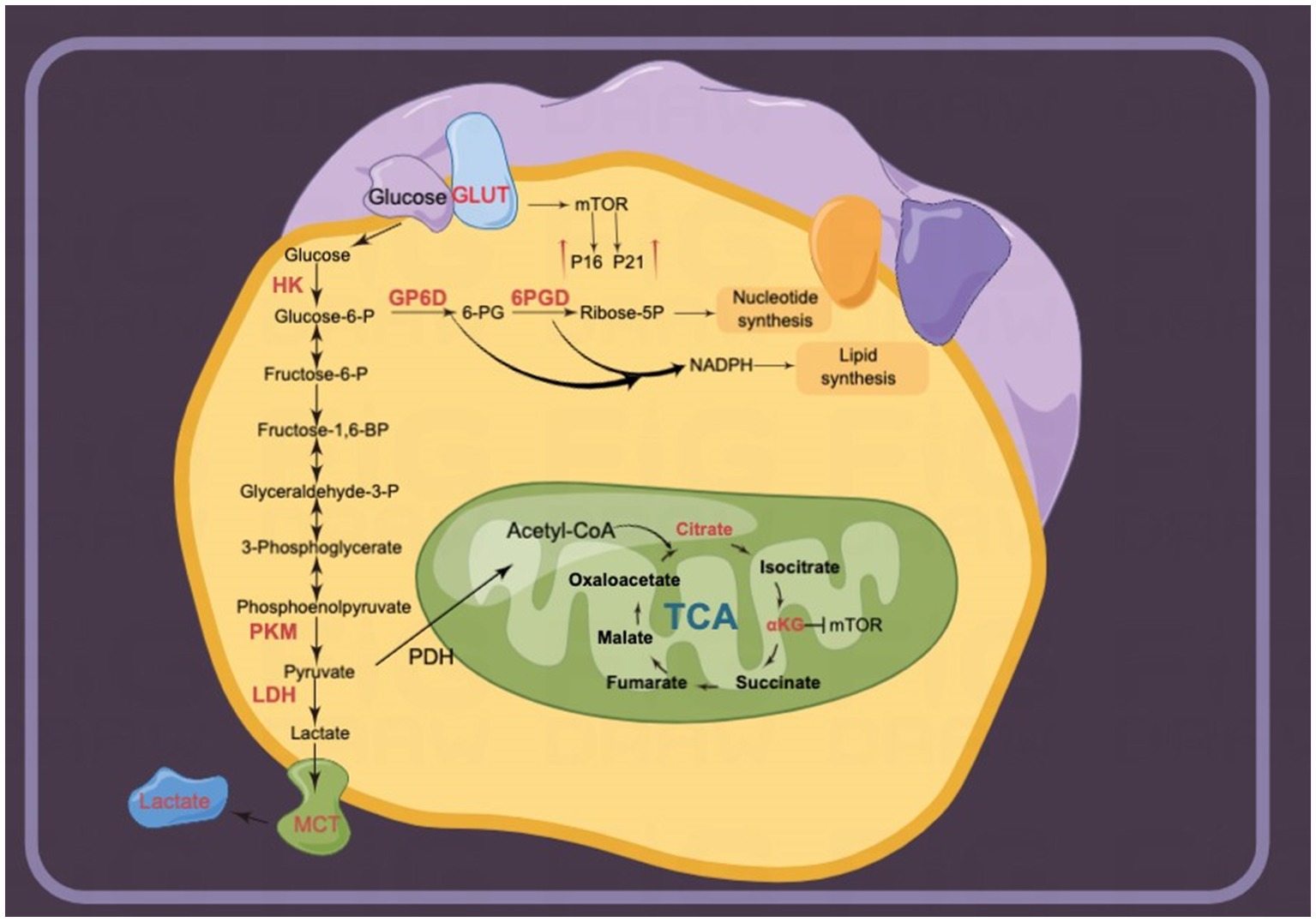
Figure 6. Glucose metabolism is upregulated in senescent cells. Multiple enzymes of glycolysis are upregulated. For instance, lactate release mediated by monocarboxylate transporters (MCTs) is involved in senescence-related secretory phenotypes (SRSPs). Several metabolites in the tricarboxylic acid (TCA) cycle, including α-ketoglutarate (αKG) and citrate, delay senescence.
Environmental factors can affect senescence through glucose and lipid metabolism, which are essential for energy production and cellular function. Alterations in the external environment such as nutrition, stress, environment, pollution, and temperature accelerate cellular senescence by regulating glucose and lipid metabolism through a variety of hormones, enzymes, and signaling pathways. Thus, targeting aberrant metabolism may delay normal cellular senescence.
In summary, fatty acid and glucose metabolism are essential in the maintenance of normal cellular function, both of which are disturbed by senescence. Conversely, disturbances in fatty acid and glucose metabolism can further lead to senescence. Two pathway-related enzymes and metabolites are involved in the regulation of cellular senescence. While many advances have been made in recent years, several important issues must still be addressed, including: (a) What are the initiating factors of senescence and metabolic disorders? (b) While reports suggest a correlation between metabolic disorders and senescence, the true causal relationship remains undetermined. (c) Anti-senescence therapies proven to work in humans are scarce. Understanding the role of enzymes and metabolites in fatty acid and glucose metabolism may provide answers for a more comprehensive understanding of senescence.
Author contributions
BL and QM investigated and wrote the first draft of the manuscript. XG and HS wrote sections of the manuscript. ZX and YW contributed to conception the study and acquire funds. HZ contributed to design of the study and acquire funds. BL, QM, XG, HS, ZX, YW, and HZ were involved in drafting, revising the manuscript, and agree to be accountable for the content of the work. All authors contributed to the article and approved the submitted version.
Funding
This work was supported by the National Natural Science Foundation of China (Nos.: 82270785 and 82020108024) and Science and Technology Department of Jilin Province (No. 20230101138JC).
Acknowledgments
We sincerely thank the reviewers and editors of Frontiers in Nutrition for providing valuable suggestions on this paper.
Conflict of interest
The authors declare that the research was conducted in the absence of any commercial or financial relationships that could be construed as a potential conflict of interest.
Publisher’s note
All claims expressed in this article are solely those of the authors and do not necessarily represent those of their affiliated organizations, or those of the publisher, the editors and the reviewers. Any product that may be evaluated in this article, or claim that may be made by its manufacturer, is not guaranteed or endorsed by the publisher.
References
1. Burton, DG, and Krizhanovsky, V. Physiological and pathological consequences of cellular senescence. Cell Mol Life Sci. (2014) 71:4373–86. doi: 10.1007/s00018-014-1691-3
2. López-Otín, C, Blasco, MA, Partridge, L, Serrano, M, and Kroemer, G. The hallmarks of aging. Cells. (2013) 153:1194–217. doi: 10.1016/j.cell.2013.05.039
3. Ma, R, Kutchy, NA, Chen, L, Meigs, DD, and Hu, G. Primary cilia and ciliary signaling pathways in aging and age-related brain disorders. Neurobiol Dis. (2022) 163:105607. doi: 10.1016/j.nbd.2021.105607
4. Kubben, N, and Misteli, T. Shared molecular and cellular mechanisms of premature ageing and ageing-associated diseases. Nat Rev Mol Cell Biol. (2017) 18:595–609. doi: 10.1038/nrm.2017.68
5. Galluzzi, L, Vitale, I, Aaronson, SA, Abrams, JM, Adam, D, Agostinis, P, et al. Molecular mechanisms of cell death: recommendations of the nomenclature committee on cell death 2018. Cell Death Differ. (2018) 25:486–541. doi: 10.1038/s41418-017-0012-4
6. Gorgoulis, V, Adams, PD, Alimonti, A, Bennett, DC, Bischof, O, Bishop, C, et al. Cellular senescence: defining a path forward. Cells. (2019) 179:813–27. doi: 10.1016/j.cell.2019.10.005
7. Barzilai, N, Huffman, DM, Muzumdar, RH, and Bartke, A. The critical role of metabolic pathways in aging. Diabetes. (2012) 61:1315–22. doi: 10.2337/db11-1300
8. Riera, CE, and Dillin, A. Tipping the metabolic scales towards increased longevity in mammals. Nat Cell Biol. (2015) 17:196–203. doi: 10.1038/ncb3107
9. Dall'armi, C, Devereaux, KA, and Di Paolo, G. The role of lipids in the control of autophagy. Curr Biol. (2013) 23:R33–45. doi: 10.1016/j.cub.2012.10.041
10. Grunt, TW. Interacting cancer machineries: cell signaling, lipid metabolism, and epigenetics. Trends Endocrinol Metab. (2018) 29:86–98. doi: 10.1016/j.tem.2017.11.003
11. Sunshine, H, and Iruela-Arispe, ML. Membrane lipids and cell signaling. Curr Opin Lipidol. (2017) 28:408–13. doi: 10.1097/MOL.0000000000000443
12. Toth, MJ, and Tchernof, A. Lipid metabolism in the elderly. Eur J Clin Nutr. (2000) 54:S121–5. doi: 10.1038/sj.ejcn.1601033
13. Sênos Demarco, R, Clémot, M, and Jones, DL. The impact of ageing on lipid-mediated regulation of adult stem cell behavior and tissue homeostasis. Mech Ageing Dev. (2020) 189:111278. doi: 10.1016/j.mad.2020.111278
14. Kuhla, A, Blei, T, Jaster, R, and Vollmar, B. Aging is associated with a shift of fatty metabolism toward lipogenesis. J Gerontol A Biol Sci Med Sci. (2011) 66:1192–200. doi: 10.1093/gerona/glr124
15. Kim, YM, Shin, HT, Seo, YH, Byun, HO, Yoon, SH, Lee, IK, et al. Sterol regulatory element-binding protein (Srebp)-1-mediated lipogenesis is involved in cell senescence. J Biol Chem. (2010) 285:29069–77. doi: 10.1074/jbc.M110.120386
16. Wang, Q, Nie, L, Zhao, P, Zhou, X, Ding, Y, Chen, Q, et al. Diabetes fuels periodontal lesions via Glut1-driven macrophage inflammaging. Int J Oral Sci. (2021a) 13:11. doi: 10.1038/s41368-021-00116-6
17. Yao, GD, Yang, J, Li, XX, Song, XY, Hayashi, T, Tashiro, SI, et al. Blocking the utilization of glucose induces the switch from senescence to apoptosis in pseudolaric acid B-treated human lung cancer cells in vitro. Acta Pharmacol Sin. (2017) 38:1401–11. doi: 10.1038/aps.2017.39
18. Hariton, F, Xue, M, Rabbani, N, Fowler, M, and Thornalley, PJ. Sulforaphane delays fibroblast senescence by curbing cellular glucose uptake, increased glycolysis, and oxidative damage. Oxidative Med Cell Longev. (2018) 2018:5642148. doi: 10.1155/2018/5642148
19. Stolzing, A, Coleman, N, and Scutt, A. Glucose-induced replicative senescence in mesenchymal stem cells. Rejuvenation Res. (2006) 9:31–5. doi: 10.1089/rej.2006.9.31
20. Wiley, CD, and Campisi, J. The metabolic roots of senescence: mechanisms and opportunities for intervention. Nat Metab. (2021) 3:1290–301. doi: 10.1038/s42255-021-00483-8
21. Li, X, Zhang, Z, Zhang, Y, Cao, Y, Wei, H, and Wu, Z. Upregulation of lactate-inducible snail protein suppresses oncogene-mediated senescence through p16(INK4a) inactivation. J Exp Clin Cancer Res. (2018) 37:39. doi: 10.1186/s13046-018-0701-y
22. Asadi Shahmirzadi, A, Edgar, D, Liao, CY, Hsu, YM, Lucanic, M, Asadi Shahmirzadi, A, et al. Alpha-ketoglutarate, an endogenous metabolite, extends lifespan and compresses morbidity in aging mice. Cell Metab. (2020) 32:447–456 E6. doi: 10.1016/j.cmet.2020.08.004
23. Chin, RM, Fu, X, Pai, MY, Vergnes, L, Hwang, H, Deng, G, et al. The metabolite alpha-ketoglutarate extends lifespan by inhibiting ATP synthase and TOR. Nature. (2014) 510:397–401. doi: 10.1038/nature13264
24. Wang, Y, Deng, P, Liu, Y, Wu, Y, Chen, Y, Guo, Y, et al. Alpha-ketoglutarate ameliorates age-related osteoporosis via regulating histone methylations. Nat Commun. (2020b) 11:5596. doi: 10.1038/s41467-020-19360-1
25. Fan, SZ, Lin, CS, Wei, YW, Yeh, SR, Tsai, YH, Lee, AC, et al. Dietary citrate supplementation enhances longevity, metabolic health, and memory performance through promoting Ketogenesis. Aging Cell. (2021) 20:E13510. doi: 10.1111/acel.13510
26. Zhao, Y, Liu, X, Si, F, Huang, L, Gao, A, Lin, W, et al. Citrate promotes excessive lipid biosynthesis and senescence in tumor cells for tumor therapy. Adv Sci (Weinh). (2022) 9:E2101553. doi: 10.1002/advs.202101553
27. Adeva-Andany, MM, Carneiro-Freire, N, Seco-Filgueira, M, Fernández-Fernández, C, and Mouriño-Bayolo, D. Mitochondrial Β-oxidation of saturated fatty acids in humans. Mitochondrion. (2019) 46:73–90. doi: 10.1016/j.mito.2018.02.009
28. Ferdinandusse, S, Rusch, H, Van Lint, AE, Dacremont, G, Wanders, RJ, and Vreken, P. Stereochemistry of the peroxisomal branched-chain fatty acid alpha- and beta-oxidation systems in patients suffering from different peroxisomal disorders. J Lipid Res. (2002) 43:438–44. doi: 10.1016/S0022-2275(20)30150-4
29. Miura, Y. The biological significance of Ω-oxidation of fatty acids. Proc Jpn Acad Ser B Phys Biol Sci. (2013) 89:370–82. doi: 10.2183/pjab.89.370
30. Nakamura, MT, Yudell, BE, and Loor, JJ. Regulation of energy metabolism by long-chain fatty acids. Prog Lipid Res. (2014) 53:124–44. doi: 10.1016/j.plipres.2013.12.001
31. Puchalska, P, and Crawford, PA. Metabolic and signaling roles of ketone bodies in health and disease. Annu Rev Nutr. (2021) 41:49–77. doi: 10.1146/annurev-nutr-111120-111518
32. Carracedo, A, Cantley, LC, and Pandolfi, PP. Cancer metabolism: fatty acid oxidation in the limelight. Nat Rev Cancer. (2013) 13:227–32. doi: 10.1038/nrc3483
33. Peterson, JM, Aja, S, Wei, Z, and Wong, GW. Ctrp1 protein enhances fatty acid oxidation via amp-activated protein kinase (AMPK) activation and acetyl-Coa carboxylase (ACC) inhibition. J Biol Chem. (2012) 287:1576–87. doi: 10.1074/jbc.M111.278333
34. Braun, F, Rinschen, MM, Bartels, V, Frommolt, P, Habermann, B, Hoeijmakers, JH, et al. Altered lipid metabolism in the aging kidney identified by three layered Omic analysis. Aging (Albany NY). (2016) 8:441–57. doi: 10.18632/aging.100900
35. Murea, M, Freedman, BI, Parks, JS, Antinozzi, PA, Elbein, SC, and Ma, L. Lipotoxicity in diabetic nephropathy: the potential role of fatty acid oxidation. Clin J Am Soc Nephrol. (2010) 5:2373–9. doi: 10.2215/CJN.08160910
36. Houtkooper, RH, Argmann, C, Houten, SM, Cantó, C, Jeninga, EH, Andreux, PA, et al. The metabolic footprint of aging in mice. Sci Rep. (2011) 1:134. doi: 10.1038/srep00134
37. Wang, Y, Chen, Y, Guan, L, Zhang, H, Huang, Y, Johnson, CH, et al. Carnitine Palmitoyltransferase 1C regulates cancer cell senescence through mitochondria-associated metabolic reprograming. Cell Death Differ. (2018) 25:735–48. doi: 10.1038/s41418-017-0013-3
38. Vaiserman, A, and Krasnienkov, D. Telomere length as a marker of biological age: state-of-the-art, open issues, and future perspectives. Front Genet. (2020) 11:630186. doi: 10.3389/fgene.2020.630186
39. Guo, L, Chen, Y, Li, H, Yin, F, Ge, M, Hu, L, et al. Telomere length is maternally inherited and associated with lipid metabolism in Chinese population. Aging (Albany NY). (2022) 14:354–67. doi: 10.18632/aging.203810
40. Belal, SA, Sivakumar, AS, Kang, DR, Cho, S, Choe, HS, and Shim, KS. Modulatory effect of linoleic and oleic acid on cell proliferation and lipid metabolism gene expressions in primary bovine satellite cells. Anim Cells Syst (Seoul). (2018) 22:324–33. doi: 10.1080/19768354.2018.1517824
41. Ma, Y, Zha, J, Yang, X, Li, Q, Zhang, Q, Yin, A, et al. Long-chain fatty acyl-CoA synthetase 1 promotes prostate cancer progression by elevation of lipogenesis and fatty acid beta-oxidation. Oncogene. (2021) 40:1806–20. doi: 10.1038/s41388-021-01667-y
42. Rayess, H, Wang, MB, and Srivatsan, ES. Cellular senescence and tumor suppressor gene P16. Int J Cancer. (2012) 130:1715–25. doi: 10.1002/ijc.27316
43. Rufini, A, Tucci, P, Celardo, I, and Melino, G. Senescence and aging: the critical roles of P53. Oncogene. (2013) 32:5129–43. doi: 10.1038/onc.2012.640
44. Guan, L, Chen, Y, Wang, Y, Zhang, H, Fan, S, Gao, Y, et al. Effects of carnitine palmitoyltransferases on cancer cellular senescence. J Cell Physiol. (2019) 234:1707–19. doi: 10.1002/jcp.27042
45. Zhuang, W, Lian, G, Huang, B, Du, A, Gong, J, Xiao, G, et al. CPT1 regulates the proliferation of pulmonary artery smooth muscle cells through the AMPK-P53-P21 pathway in pulmonary arterial hypertension. Mol Cell Biochem. (2019) 455:169–83. doi: 10.1007/s11010-018-3480-z
46. Shao, H, Mohamed, EM, Xu, GG, Waters, M, Jing, K, Ma, Y, et al. Carnitine palmitoyltransferase 1A functions to repress FOXO transcription factors to allow cell cycle progression in ovarian cancer. Oncotarget. (2016) 7:3832–46. doi: 10.18632/oncotarget.6757
47. Mihaylova, MM, Cheng, CW, Cao, AQ, Tripathi, S, Mana, MD, Bauer-Rowe, KE, et al. Fasting activates fatty acid oxidation to enhance intestinal stem cell function during homeostasis and aging. Cell Stem Cell. (2018) 22:769–778 E4. doi: 10.1016/j.stem.2018.04.001
48. Fadó, R, Rodríguez-Rodríguez, R, and Casals, N. The return of malonyl-CoA to the brain: cognition and other stories. Prog Lipid Res. (2021) 81:101071. doi: 10.1016/j.plipres.2020.101071
49. Knobloch, M, Pilz, GA, Ghesquière, B, Kovacs, WJ, Wegleiter, T, Moore, DL, et al. A fatty acid oxidation-dependent metabolic shift regulates adult neural stem cell activity. Cell Rep. (2017) 20:2144–55. doi: 10.1016/j.celrep.2017.08.029
50. Franceschi, C, Bonafè, M, Valensin, S, Olivieri, F, De Luca, M, Ottaviani, E, et al. Inflamm-aging. An evolutionary perspective on immunosenescence. Ann N Y Acad Sci. (2000) 908:244–54. doi: 10.1111/j.1749-6632.2000.tb06651.x
51. Malandrino, MI, Fucho, R, Weber, M, Calderon-Dominguez, M, Mir, JF, Valcarcel, L, et al. Enhanced fatty acid oxidation in adipocytes and macrophages reduces lipid-induced triglyceride accumulation and inflammation. Am J Physiol Endocrinol Metab. (2015) 308:E756–69. doi: 10.1152/ajpendo.00362.2014
52. Namgaladze, D, Lips, S, Leiker, TJ, Murphy, RC, Ekroos, K, Ferreiros, N, et al. Inhibition of macrophage fatty acid Β-oxidation exacerbates palmitate-induced inflammatory and endoplasmic reticulum stress responses. Diabetologia. (2014) 57:1067–77. doi: 10.1007/s00125-014-3173-4
53. He, A, Chen, X, Tan, M, Chen, Y, Lu, D, Zhang, X, et al. Acetyl-Coa derived from hepatic peroxisomal Β-oxidation inhibits autophagy and promotes steatosis via Mtorc1 activation. Mol Cell. (2020) 79:30–42.E4. doi: 10.1016/j.molcel.2020.05.007
54. Yang, L, Zhang, Y, Wang, S, Zhang, W, and Shi, R. Decreased liver peroxisomal beta-oxidation accompanied by changes in brain fatty acid composition in aged rats. Neurol Sci. (2014) 35:289–93. doi: 10.1007/s10072-013-1509-3
55. Périchon, R, Bourre, JM, Kelly, JF, and Roth, GS. The role of peroxisomes in aging. Cell Mol Life Sci. (1998) 54:641–52. doi: 10.1007/s000180050192
56. Fanelli, F, Sepe, S, D'amelio, M, Bernardi, C, Cristiano, L, Cimini, A, et al. Age-dependent roles of peroxisomes in the hippocampus of a transgenic mouse model of Alzheimer's disease. Mol Neurodegener. (2013) 8:8. doi: 10.1186/1750-1326-8-8
57. Wang, ZW, Pan, WT, Lee, Y, Kakuma, T, Zhou, YT, and Unger, RH. The role of leptin resistance in the lipid abnormalities of aging. FASEB J. (2001) 15:108–14. doi: 10.1096/fj.00-0310com
58. El Hajj, HI, Vluggens, A, Andreoletti, P, Ragot, K, Mandard, S, Kersten, S, et al. The inflammatory response in acyl-CoA oxidase 1 deficiency (pseudoneonatal adrenoleukodystrophy). Endocrinology. (2012) 153:2568–75. doi: 10.1210/en.2012-1137
59. Moreno-Fernandez, ME, Giles, DA, Stankiewicz, TE, Sheridan, R, Karns, R, Cappelletti, M, et al. Peroxisomal Β-oxidation regulates whole body metabolism, inflammatory vigor, and pathogenesis of nonalcoholic fatty liver disease. JCI Insight. (2018) 3:e93626. doi: 10.1172/jci.insight.93626
60. Chiang, N, and Serhan, CN. Specialized pro-resolving mediator network: an update on production and actions. Essays Biochem. (2020) 64:443–62. doi: 10.1042/EBC20200018
61. Kraft, JD, Blomgran, R, Lundgaard, I, Quiding-Järbrink, M, Bromberg, JS, and Börgeson, E. Specialized pro-resolving mediators and the lymphatic system. Int J Mol Sci. (2021) 22:2750. doi: 10.3390/ijms22052750
62. Vamecq, J, Andreoletti, P, El Kebbaj, R, Saih, FE, Latruffe, N, El Kebbaj, MHS, et al. Peroxisomal acyl-CoA oxidase type 1: anti-inflammatory and anti-aging properties with a special emphasis on studies with LPS and argan oil as a model transposable to aging. Oxidative Med Cell Longev. (2018) 2018:6986984. doi: 10.1155/2018/6986984
63. Chakravarthy, MV, Pan, Z, Zhu, Y, Tordjman, K, Schneider, JG, Coleman, T, et al. "New" hepatic fat activates PPARalpha to maintain glucose, lipid, and cholesterol homeostasis. Cell Metab. (2005) 1:309–22. doi: 10.1016/j.cmet.2005.04.002
64. Consortium, T. U. UniProt: the universal protein knowledgebase in 2021. Nucleic Acids Res. (2020) 49:D480–9. doi: 10.1093/nar/gkaa1100
65. Iemitsu, M, Miyauchi, T, Maeda, S, Tanabe, T, Takanashi, M, Irukayama-Tomobe, Y, et al. Aging-induced decrease in the PPAR-Α level in hearts is improved by exercise training. Am J Phys Heart Circ Phys. (2002) 283:H1750–60. doi: 10.1152/ajpheart.01051.2001
66. Sanguino, E, Roglans, N, Alegret, M, Sánchez, RM, Vázquez-Carrera, M, and Laguna, JC. Atorvastatin reverses age-related reduction in rat hepatic PPARα and HNF-4. Br J Pharmacol. (2005) 145:853–61. doi: 10.1038/sj.bjp.0706260
67. Ye, P, Wang, ZJ, Zhang, XJ, and Zhao, YL. Age-related decrease in expression of peroxisome proliferator-activated receptor alpha and its effects on development of dyslipidemia. Chin Med J. (2005) 118:1093–8.
68. Yan, T, Luo, Y, Yan, N, Hamada, K, Zhao, N, Xia, Y, et al. Intestinal peroxisome proliferator-activated receptor α-fatty acid-binding protein 1 axis modulates nonalcoholic steatohepatitis. Hepatology. (2022) 77:239–55. doi: 10.1002/hep.32538
69. Woudstra, TD, Drozdowski, LA, Wild, GE, Clandinin, MT, Agellon, LB, and Thomson, AB. The age-related decline in intestinal lipid uptake is associated with a reduced abundance of fatty acid-binding protein. Lipids. (2004) 39:603–10. doi: 10.1007/s11745-004-1272-9
70. Chen, Y, Wang, Y, Huang, Y, Zeng, H, Hu, B, Guan, L, et al. Pparα regulates tumor cell proliferation and senescence via a novel target gene carnitine palmitoyltransferase 1C. Carcinogenesis. (2017) 38:474–83. doi: 10.1093/carcin/bgx023
71. Chung, JH, Seo, AY, Chung, SW, Kim, MK, Leeuwenburgh, C, Yu, BP, et al. Molecular mechanism of PPAR in the regulation of age-related inflammation. Ageing Res Rev. (2008) 7:126–36. doi: 10.1016/j.arr.2008.01.001
72. Chandrashekaran, V, Seth, RK, Dattaroy, D, Alhasson, F, Ziolenka, J, Carson, J, et al. HMGB1-RAGE pathway drives peroxynitrite signaling-induced IBD-like inflammation in murine nonalcoholic fatty liver disease. Redox Biol. (2017) 13:8–19. doi: 10.1016/j.redox.2017.05.005
73. Senatus, LM, and Schmidt, AM. The AGE-RAGE Axis: implications for age-associated arterial diseases. Front Genet. (2017) 8:187. doi: 10.3389/fgene.2017.00187
74. Song, F, Hurtado Del Pozo, C, Rosario, R, Zou, YS, Ananthakrishnan, R, Xu, X, et al. RAGE regulates the metabolic and inflammatory response to high-fat feeding in mice. Diabetes. (2014) 63:1948–65. doi: 10.2337/db13-1636
75. Wan, J, Wu, X, Chen, H, Xia, X, Song, X, Chen, S, et al. Aging-induced aberrant RAGE/PPARalpha Axis promotes hepatic steatosis via dysfunctional mitochondrial Beta oxidation. Aging Cell. (2020) 19:E13238. doi: 10.1111/acel.13238
76. Qi, W, Gutierrez, GE, Gao, X, Dixon, H, Mcdonough, JA, Marini, AM, et al. The Ω-3 fatty acid α-linolenic acid extends Caenorhabditis Elegans lifespan via NHR-49/PPARα and oxidation to oxylipins. Aging Cell. (2017) 16:1125–35. doi: 10.1111/acel.12651
77. Folick, A, Oakley, HD, Yu, Y, Armstrong, EH, Kumari, M, Sanor, L, et al. Aging. Lysosomal signaling molecules regulate longevity in Caenorhabditis Elegans. Science. (2015) 347:83–6. doi: 10.1126/science.1258857
78. Nogueira-Recalde, U, Lorenzo-Gómez, I, Blanco, FJ, Loza, MI, Grassi, D, Shirinsky, V, et al. Fibrates as drugs with senolytic and autophagic activity for osteoarthritis therapy. EBioMedicine. (2019) 45:588–605. doi: 10.1016/j.ebiom.2019.06.049
79. Nie, Y, and Stürzenbaum, SR. Proanthocyanidins of natural origin: molecular mechanisms and implications for lipid disorder and aging-associated diseases. Adv Nutr. (2019) 10:464–78. doi: 10.1093/advances/nmy118
80. Zhou, Y, Xu, Q, Dong, Y, Zhu, S, Song, S, and Sun, S. Supplementation of mussel peptides reduces aging phenotype, lipid deposition and oxidative stress in D-galactose-induce aging mice. J Nutr Health Aging. (2017) 21:1314–20. doi: 10.1007/s12603-016-0862-3
81. Lodhi, IJ, and Semenkovich, CF. Peroxisomes: a nexus for lipid metabolism and cellular signaling. Cell Metab. (2014) 19:380–92. doi: 10.1016/j.cmet.2014.01.002
82. Singh, I, Pahan, K, Singh, AK, and Barbosa, E. Refsum disease: a defect in the alpha-oxidation of phytanic acid in peroxisomes. J Lipid Res. (1993) 34:1755–64. doi: 10.1016/S0022-2275(20)35738-2
83. Lee, J, Ellis, JM, and Wolfgang, MJ. Adipose fatty acid oxidation is required for thermogenesis and potentiates oxidative stress-induced inflammation. Cell Rep. (2015) 10:266–79. doi: 10.1016/j.celrep.2014.12.023
84. Westin, MAK, Hunt, MC, and Alexson, SEH. Peroxisomes contain a specific phytanoyl-CoA/pristanoyl-CoA thioesterase acting as a novel auxiliary enzyme in alpha- and beta-oxidation of methyl-branched fatty acids in mouse. J Biol Chem. (2007) 282:26707–16. doi: 10.1074/jbc.M703718200
85. Talley, JT, and Mohiuddin, SS. Biochemistry, fatty acid oxidation. Statpearls. Treasure Island (Fl): Statpearls Publishing Copyright © 2023, Statpearls Publishing LLC (2023).
86. Ito, H, Nakamae, I, Kato, JY, and Yoneda-Kato, N. Stabilization of fatty acid synthesis enzyme acetyl-CoA carboxylase 1 suppresses acute myeloid leukemia development. J Clin Invest. (2021) 131:e141529. doi: 10.1172/JCI141529
87. Fullerton, MD, Galic, S, Marcinko, K, Sikkema, S, Pulinilkunnil, T, Chen, ZP, et al. Single phosphorylation sites in ACC1 and ACC2 regulate lipid homeostasis and the insulin-sensitizing effects of metformin. Nat Med. (2013) 19:1649–54. doi: 10.1038/nm.3372
88. Gross, AS, Zimmermann, A, Pendl, T, Schroeder, S, Schoenlechner, H, Knittelfelder, O, et al. Acetyl-CoA carboxylase 1-dependent lipogenesis promotes autophagy downstream of AMPK. J Biol Chem. (2019) 294:12020–39. doi: 10.1074/jbc.RA118.007020
89. Currais, A, Huang, L, Goldberg, J, Petrascheck, M, Ates, G, Pinto-Duarte, A, et al. Elevating acetyl-CoA levels reduces aspects of brain aging. elife. (2019) 8:e47866. doi: 10.7554/eLife.47866
90. Shiozaki, M, Hayakawa, N, Shibata, M, Koike, M, Uchiyama, Y, and Gotow, T. Closer association of mitochondria with lipid droplets in hepatocytes and activation of Kupffer cells in resveratrol-treated senescence-accelerated mice. Histochem Cell Biol. (2011) 136:475–89. doi: 10.1007/s00418-011-0847-6
91. Kim, SJ, Tang, T, Abbott, M, Viscarra, JA, Wang, Y, and Sul, HS. AMPK phosphorylates desnutrin/ATGL and hormone-sensitive lipase to regulate lipolysis and fatty acid oxidation within adipose tissue. Mol Cell Biol. (2016) 36:1961–76. doi: 10.1128/MCB.00244-16
92. Lally, JSV, Ghoshal, S, Deperalta, DK, Moaven, O, Wei, L, Masia, R, et al. Inhibition of acetyl-CoA carboxylase by phosphorylation or the inhibitor ND-654 suppresses lipogenesis and hepatocellular carcinoma. Cell Metab. (2019) 29:174–182.E5. doi: 10.1016/j.cmet.2018.08.020
93. Fang, K, Wu, F, Chen, G, Dong, H, Li, J, Zhao, Y, et al. Diosgenin ameliorates palmitic acid-induced lipid accumulation via AMPK/ACC/CPT-1A and SREBP-1c/FAS signaling pathways in LO2 cells. BMC Complement Altern Med. (2019) 19:255. doi: 10.1186/s12906-019-2671-9
94. Gao, L, Xu, Z, Huang, Z, Tang, Y, Yang, D, Huang, J, et al. CPI-613 rewires lipid metabolism to enhance pancreatic cancer apoptosis via the AMPK-ACC signaling. J Exp Clin Cancer Res. (2020a) 39:73. doi: 10.1186/s13046-020-01579-x
95. Weir, HJ, Yao, P, Huynh, FK, Escoubas, CC, Goncalves, RL, Burkewitz, K, et al. Dietary restriction and AMPK increase lifespan via mitochondrial network and peroxisome remodeling. Cell Metab. (2017) 26:884–896.E5. doi: 10.1016/j.cmet.2017.09.024
96. Greer, EL, Dowlatshahi, D, Banko, MR, Villen, J, Hoang, K, Blanchard, D, et al. An AMPK-FOXO pathway mediates longevity induced by a novel method of dietary restriction in C. elegans. Curr Biol. (2007) 17:1646–56. doi: 10.1016/j.cub.2007.08.047
97. Apfeld, J, O'connor, G, Mcdonagh, T, Distefano, PS, and Curtis, R. The AMP-activated protein kinase AAK-2 links energy levels and insulin-like signals to lifespan in C. elegans. Genes Dev. (2004) 18:3004–9. doi: 10.1101/gad.1255404
98. Curtis, R, O'connor, G, and Distefano, PS. Aging networks in Caenorhabditis Elegans: AMP-activated protein kinase (AAK-2) links multiple aging and metabolism pathways. Aging Cell. (2006) 5:119–26. doi: 10.1111/j.1474-9726.2006.00205.x
99. Selman, C, Tullet, JM, Wieser, D, Irvine, E, Lingard, SJ, Choudhury, AI, et al. Ribosomal protein S6 kinase 1 signaling regulates mammalian life span. Science. (2009) 326:140–4. doi: 10.1126/science.1177221
100. Yan, J, Nie, Y, Cao, J, Luo, M, Yan, M, Chen, Z, et al. The roles and pharmacological effects of FGF21 in preventing aging-associated metabolic diseases. Front Cardiovasc Med. (2021) 8:655575. doi: 10.3389/fcvm.2021.655575
101. Wang, XM, Xiao, H, Liu, LL, Cheng, D, Li, XJ, and Si, LY. FGF21 represses cerebrovascular aging via improving mitochondrial biogenesis and inhibiting p53 signaling pathway in An AMPK-dependent manner. Exp Cell Res. (2016) 346:147–56. doi: 10.1016/j.yexcr.2016.06.020
102. Anisimov, VN. Metformin for aging and cancer prevention. Aging (Albany NY). (2010) 2:760–74. doi: 10.18632/aging.100230
103. Anisimov, VN. Metformin: do we finally have an anti-aging drug? Cell Cycle. (2013) 12:3483–9. doi: 10.4161/cc.26928
104. Stenesen, D, Suh, JM, Seo, J, Yu, K, Lee, K-S, Kim, J-S, et al. Adenosine nucleotide biosynthesis and AMPK regulate adult life span and mediate the longevity benefit of caloric restriction in flies. Cell Metab. (2013) 17:101–12. doi: 10.1016/j.cmet.2012.12.006
105. Chen, X, Li, X, Zhang, W, He, J, Xu, B, Lei, B, et al. Activation of AMPK inhibits inflammatory response during hypoxia and reoxygenation through modulating JNK-mediated NF-ΚB pathway. Metabolism. (2018) 83:256–70. doi: 10.1016/j.metabol.2018.03.004
106. Chen, C, Zhou, M, Ge, Y, and Wang, X. Sirt1 and aging related signaling pathways. Mech Ageing Dev. (2020) 187:111215. doi: 10.1016/j.mad.2020.111215
107. Ge, Y, Zhou, M, Chen, C, Wu, X, and Wang, X. Role of AMPK mediated pathways in autophagy and aging. Biochimie. (2022) 195:100–13. doi: 10.1016/j.biochi.2021.11.008
108. Li, Y, and Chen, Y. AMPK And autophagy. Adv Exp Med Biol. (2019) 1206:85–108. doi: 10.1007/978-981-15-0602-4_4
109. Perluigi, M, Di Domenico, F, and Butterfield, DA. mTOR signaling in aging and neurodegeneration: at the crossroad between metabolism dysfunction and impairment of autophagy. Neurobiol Dis. (2015) 84:39–49. doi: 10.1016/j.nbd.2015.03.014
110. Chen, C, Liu, Y, Liu, Y, and Zheng, P. mTOR regulation and therapeutic rejuvenation of aging hematopoietic stem cells. Sci Signal. (2009) 2:ra75. doi: 10.1126/scisignal.2000559
111. Yilmaz, ÖH, Katajisto, P, Lamming, DW, Gültekin, Y, Bauer-Rowe, KE, Sengupta, S, et al. mTORC1 in the Paneth cell niche couples intestinal stem-cell function to calorie intake. Nature. (2012) 486:490–5. doi: 10.1038/nature11163
112. Feng, X, Pan, J, Li, J, Zeng, C, Qi, W, Shao, Y, et al. Metformin attenuates cartilage degeneration in An experimental osteoarthritis model by regulating Ampk/Mtor. Aging (Albany NY). (2020) 12:1087–103. doi: 10.18632/aging.102635
113. Stubbs, BJ, Koutnik, AP, Volek, JS, and Newman, JC. From bedside to battlefield: intersection of ketone body mechanisms in geroscience with military resilience. Geroscience. (2021) 43:1071–81. doi: 10.1007/s11357-020-00277-y
114. Lilamand, M, Mouton-Liger, F, Di Valentin, E, Sànchez Ortiz, M, and Paquet, C. Efficacy and safety of ketone supplementation or ketogenic diets for Alzheimer's disease: a Mini review. Front Nutr. (2021) 8:807970. doi: 10.3389/fnut.2021.807970
115. Dąbek, A, Wojtala, M, Pirola, L, and Balcerczyk, A. Modulation of cellular biochemistry, epigenetics and metabolomics by ketone bodies. Implications of the ketogenic diet in the physiology of the organism and pathological states. Nutrients. (2020) 12:788. doi: 10.3390/nu12030788
116. Ji, L, He, Q, Liu, Y, Deng, Y, Xie, M, Luo, K, et al. Ketone body Β-hydroxybutyrate prevents myocardial oxidative stress in septic cardiomyopathy. Oxidative Med Cell Longev. (2022) 2022:2513837. doi: 10.1155/2022/2513837
117. Youm, YH, Nguyen, KY, Grant, RW, Goldberg, EL, Bodogai, M, Kim, D, et al. The ketone metabolite Β-hydroxybutyrate blocks NLRP3 inflammasome-mediated inflammatory disease. Nat Med. (2015) 21:263–9. doi: 10.1038/nm.3804
118. Kolb, H, Kempf, K, Röhling, M, Lenzen-Schulte, M, Schloot, NC, and Martin, S. Ketone bodies: from enemy to friend and guardian angel. BMC Med. (2021) 19:313. doi: 10.1186/s12916-021-02185-0
119. Mccarthy, CG, Chakraborty, S, Singh, G, Yeoh, BS, Schreckenberger, ZJ, Singh, A, et al. Ketone body Β-hydroxybutyrate is an autophagy-dependent vasodilator. JCI Insight. (2021) 6:e149037. doi: 10.1172/jci.insight.149037
120. Petrick, HL, Pinckaers, PJM, and Brunetta, HS. Ketone body oxidation: glycogen-sparing yet glucose-dependent? J Physiol. (2023) 601:2237–9. doi: 10.1113/JP284561
121. Xu, S, Tao, H, Cao, W, Cao, L, Lin, Y, Zhao, SM, et al. Ketogenic diets inhibit mitochondrial biogenesis and induce cardiac fibrosis. Signal Transduct Target Ther. (2021) 6:54. doi: 10.1038/s41392-020-00411-4
122. Møller, N. Ketone body, 3-hydroxybutyrate: minor metabolite – major medical manifestations. J Clin Endocrinol Metab. (2020) 105:2884–92. doi: 10.1210/clinem/dgaa370
123. Neal, EG, Chaffe, H, Schwartz, RH, Lawson, MS, Edwards, N, Fitzsimmons, G, et al. The ketogenic diet for the treatment of childhood epilepsy: a randomised controlled trial. Lancet Neurol. (2008) 7:500–6. doi: 10.1016/S1474-4422(08)70092-9
124. Amiel, SA, Archibald, HR, Chusney, G, Williams, AJ, and Gale, EA. Ketone infusion lowers hormonal responses to hypoglycaemia: evidence for acute cerebral utilization of a non-glucose fuel. Clin Sci (Lond). (1991) 81:189–94. doi: 10.1042/cs0810189
125. Dai, Y, Tang, H, and Pang, S. The crucial roles of phospholipids in aging and lifespan regulation. Front Physiol. (2021) 12:775648. doi: 10.3389/fphys.2021.775648
126. Vance, JE. Phospholipid synthesis and transport in mammalian cells. Traffic. (2015) 16:1–18. doi: 10.1111/tra.12230
127. Boss, WF, and Im, YJ. Phosphoinositide signaling. Annu Rev Plant Biol. (2012) 63:409–29. doi: 10.1146/annurev-arplant-042110-103840
128. Tsao, FHC, Barnes, JN, Amessoudji, A, Li, Z, and Meyer, KC. Aging-related and gender specific albumin misfolding in Alzheimer's disease. J Alzheimers Dis Rep. (2020) 4:67–77. doi: 10.3233/ADR-200168
129. Pamplona, R. Membrane phospholipids, lipoxidative damage and molecular integrity: a causal role in aging and longevity. Biochim Biophys Acta. (2008) 1777:1249–62. doi: 10.1016/j.bbabio.2008.07.003
130. Dabravolski, SA, Bezsonov, EE, and Orekhov, AN. The role of mitochondria dysfunction and hepatic senescence in NAFLD development and progression. Biomed Pharmacother. (2021) 142:112041. doi: 10.1016/j.biopha.2021.112041
131. Biasi, E. The effects of dietary choline. Neurosci Bull. (2011) 27:330–42. doi: 10.1007/s12264-011-1523-5
132. Ren, M, Phoon, CK, and Schlame, M. Metabolism and function of mitochondrial cardiolipin. Prog Lipid Res. (2014) 55:1–16. doi: 10.1016/j.plipres.2014.04.001
133. Laurila, PP, Wohlwend, M, Imamura De Lima, T, Luan, P, Herzig, S, Zanou, N, et al. Sphingolipids accumulate in aged muscle, and their reduction counteracts sarcopenia. Nat Aging. (2022) 2:1159–75. doi: 10.1038/s43587-022-00309-6
134. Sasset, L, Zhang, Y, Dunn, TM, and Di Lorenzo, A. Sphingolipid de novo biosynthesis: a rheostat of cardiovascular homeostasis. Trends Endocrinol Metab. (2016) 27:807–19. doi: 10.1016/j.tem.2016.07.005
135. Hannun, YA, and Obeid, LM. Sphingolipids and their metabolism in physiology and disease. Nat Rev Mol Cell Biol. (2018) 19:175–91. doi: 10.1038/nrm.2017.107
136. Czubowicz, K, Jęśko, H, Wencel, P, Lukiw, WJ, and Strosznajder, RP. The role of ceramide and sphingosine-1-phosphate in Alzheimer’s disease and other neurodegenerative disorders. Mol Neurobiol. (2019) 56:5436–55. doi: 10.1007/s12035-018-1448-3
137. Trayssac, M, Hannun, YA, and Obeid, LM. Role of sphingolipids in senescence: implication in aging and age-related diseases. J Clin Invest. (2018) 128:2702–12. doi: 10.1172/JCI97949
138. Michellod, D, Bien, T, Birgel, D, Violette, M, Kleiner, M, Fearn, S, et al. De novo phytosterol synthesis in animals. Science. (2023) 380:520–6. doi: 10.1126/science.add7830
139. Zhang, T, Yuan, D, Xie, J, Lei, Y, Li, J, Fang, G, et al. Evolution of the cholesterol biosynthesis pathway in animals. Mol Biol Evol. (2019) 36:2548–56. doi: 10.1093/molbev/msz167
140. Kenig, F. Infancy of sterol biosynthesis hints at extinct eukaryotic species. Nature. (2023) 618:678–80. doi: 10.1038/d41586-023-01816-1
141. Kanungo, S, Soares, N, He, M, and Steiner, RD. Sterol metabolism disorders and neurodevelopment-an update. Dev Disabil Res Rev. (2013) 17:197–210. doi: 10.1002/ddrr.1114
142. Martín, MG, Pfrieger, F, and Dotti, CG. Cholesterol in brain disease: sometimes determinant and frequently implicated. EMBO Rep. (2014) 15:1036–52. doi: 10.15252/embr.201439225
143. Porter, FD, and Herman, GE. Malformation syndromes caused by disorders of cholesterol synthesis. J Lipid Res. (2011) 52:6–34. doi: 10.1194/jlr.R009548
144. Warburg, O. On the origin of cancer cells. Science (New York, NY). (1956) 123:309–14. doi: 10.1126/science.123.3191.309
145. Wiley, CD, and Campisi, J. From ancient pathways to aging cells-connecting metabolism and cellular senescence. Cell Metab. (2016) 23:1013–21. doi: 10.1016/j.cmet.2016.05.010
146. Liao, EC, Hsu, YT, Chuah, QY, Lee, YJ, Hu, JY, Huang, TC, et al. Radiation induces senescence and a bystander effect through metabolic alterations. Cell Death Dis. (2014) 5:E1255. doi: 10.1038/cddis.2014.220
147. Nagineni, CN, Naz, S, Choudhuri, R, Chandramouli, GVR, Krishna, MC, Brender, JR, et al. Radiation-induced senescence reprograms secretory and metabolic pathways in colon cancer HCT-116 cells. Int J Mol Sci. (2021) 22:4835. doi: 10.3390/ijms22094835
148. Taddei, ML, Cavallini, L, Comito, G, Giannoni, E, Folini, M, Marini, A, et al. Senescent stroma promotes prostate cancer progression: the role of miR-210. Mol Oncol. (2014) 8:1729–46. doi: 10.1016/j.molonc.2014.07.009
149. Manerba, M, Di Ianni, L, Govoni, M, Roberti, M, Recanatini, M, and Di Stefano, G. LDH inhibition impacts on heat shock response and induces senescence of hepatocellular carcinoma cells. Eur J Pharm Sci. (2017) 105:91–8. doi: 10.1016/j.ejps.2017.05.015
150. Sun, H, Wang, H, Wang, X, Aoki, Y, Wang, X, Yang, Y, et al. Aurora-A/SOX8/FOXK1 signaling Axis promotes chemoresistance via suppression of cell senescence and induction of glucose metabolism in ovarian cancer organoids and cells. Theranostics. (2020) 10:6928–45. doi: 10.7150/thno.43811
151. James, EL, Michalek, RD, Pitiyage, GN, De Castro, AM, Vignola, KS, Jones, J, et al. Senescent human fibroblasts show increased glycolysis and redox homeostasis with extracellular metabolomes that overlap with those of irreparable DNA damage, aging, and disease. J Proteome Res. (2015) 14:1854–71. doi: 10.1021/pr501221g
152. Kanatsu-Shinohara, M, Yamamoto, T, Toh, H, Kazuki, Y, Kazuki, K, Imoto, J, et al. Aging of spermatogonial stem cells by JNK-mediated glycolysis activation. Proc Natl Acad Sci U S A. (2019) 116:16404–9. doi: 10.1073/pnas.1904980116
153. Mueckler, M, and Thorens, B. The SLC2 (GLUT) family of membrane transporters. Mol Asp Med. (2013) 34:121–38. doi: 10.1016/j.mam.2012.07.001
154. Cho, SJ, Moon, JS, Lee, CM, Choi, AM, and Stout-Delgado, HW. Glucose transporter 1-dependent glycolysis is increased during aging-related lung fibrosis, and phloretin inhibits lung fibrosis. Am J Respir Cell Mol Biol. (2017) 56:521–31. doi: 10.1165/rcmb.2016-0225OC
155. Roberts, DJ, and Miyamoto, S. Hexokinase II integrates energy metabolism and cellular protection: Akting on mitochondria and TORCing to autophagy. Cell Death Differ. (2015) 22:248–57. doi: 10.1038/cdd.2014.173
156. Sabbatinelli, J, Prattichizzo, F, Olivieri, F, Procopio, AD, Rippo, MR, and Giuliani, A. Where metabolism meets senescence: focus on endothelial cells. Front Physiol. (2019) 10:1523. doi: 10.3389/fphys.2019.01523
157. Bayliak, MM, and Lushchak, VI. Pleiotropic effects of alpha-ketoglutarate as a potential anti-ageing agent. Ageing Res Rev. (2021) 66:101237. doi: 10.1016/j.arr.2020.101237
158. Su, Y, Wang, T, Wu, N, Li, D, Fan, X, Xu, Z, et al. Alpha-ketoglutarate extends Drosophila lifespan by inhibiting mTOR and activating AMPK. Aging (Albany NY). (2019) 11:4183–97. doi: 10.18632/aging.102045
159. Zhang, Z, He, C, Gao, Y, Zhang, L, Song, Y, Zhu, T, et al. Alpha-ketoglutarate delays age-related fertility decline in mammals. Aging Cell. (2021b) 20:E13291. doi: 10.1111/acel.13291
160. Zhan, D, Ma, D, Wei, S, Lal, B, Fu, Y, Eberhart, C, et al. Monoallelic IDH1 R132H mutation mediates glioma cell response to anticancer therapies via induction of senescence. Mol Cancer Res. (2021) 19:1878–88. doi: 10.1158/1541-7786.MCR-21-0284
161. Zhang, Y, Meng, Q, Sun, Q, Xu, ZX, Zhou, H, and Wang, Y. LKB1 deficiency-induced metabolic reprogramming in tumorigenesis and non-neoplastic diseases. Mol Metab. (2021a) 44:101131. doi: 10.1016/j.molmet.2020.101131
162. Hong, X, Song, R, Song, H, Zheng, T, Wang, J, Liang, Y, et al. PTEN antagonises Tcl1/hnRNPK-mediated G6PD pre-mRNA splicing which contributes to hepatocarcinogenesis. Gut. (2014) 63:1635–47. doi: 10.1136/gutjnl-2013-305302
163. Agostini, M, Niklison-Chirou, MV, Catani, MV, Knight, RA, Melino, G, and Rufini, A. TAp73 promotes anti-senescence-anabolism not proliferation. Aging (Albany NY). (2014) 6:921–30. doi: 10.18632/aging.100701
164. Du, W, Jiang, P, Mancuso, A, Stonestrom, A, Brewer, MD, Minn, AJ, et al. TAp73 enhances the pentose phosphate pathway and supports cell proliferation. Nat Cell Biol. (2013) 15:991–1000. doi: 10.1038/ncb2789
165. Ahmad, F, Dixit, D, Sharma, V, Kumar, A, Joshi, SD, Sarkar, C, et al. Nrf2-driven TERT regulates pentose phosphate pathway in glioblastoma. Cell Death Dis. (2016) 7:E2213. doi: 10.1038/cddis.2016.117
166. Wu, YH, Cheng, ML, Ho, HY, Chiu, DT, and Wang, TC. Telomerase prevents accelerated senescence in glucose-6-phosphate dehydrogenase (G6pd)-deficient human fibroblasts. J Biomed Sci. (2009) 16:18. doi: 10.1186/1423-0127-16-18
167. Polat, IH, Tarrado-Castellarnau, M, Bharat, R, Perarnau, J, Benito, A, Cortes, R, et al. Oxidative pentose phosphate pathway enzyme 6-phosphogluconate dehydrogenase plays a key role in breast cancer metabolism. Biology (Basel). (2021) 10:85. doi: 10.3390/biology10020085
168. Zhang, Y, Guo, P, Xiang, W, Liu, Q, Liu, X, Ma, N, et al. Slowly repaired bulky DNA damages modulate cellular redox environment leading to premature senescence. Oxidative Med Cell Longev. (2020) 2020:5367102. doi: 10.1155/2020/5367102
169. Yue, Z, Nie, L, Zhao, P, Ji, N, Liao, G, and Wang, Q. Senescence-associated secretory phenotype and its impact on oral immune homeostasis. Front Immunol. (2022) 13:1019313. doi: 10.3389/fimmu.2022.1019313
170. Schafer, MJ, Zhang, X, Kumar, A, Atkinson, EJ, Zhu, Y, Jachim, S, et al. The senescence-associated secretome as an indicator of age and medical risk. JCI Insight. (2020) 5:e133668. doi: 10.1172/jci.insight.133668
171. Basisty, N, Kale, A, Jeon, OH, Kuehnemann, C, Payne, T, Rao, C, et al. A proteomic atlas of senescence-associated secretomes for aging biomarker development. PLoS Biol. (2020) 18:E3000599. doi: 10.1371/journal.pbio.3000599
172. Takaya, K, Asou, T, and Kishi, K. Downregulation of senescence-associated secretory phenotype by knockdown of secreted frizzled-related protein 4 contributes to the prevention of skin aging. Aging (Albany NY). (2022) 14:8167–78. doi: 10.18632/aging.204273
173. Hamsanathan, S, and Gurkar, AU. Lipids as regulators of cellular senescence. Front Physiol. (2022) 13:796850. doi: 10.3389/fphys.2022.796850
174. Han, X, Lei, Q, Xie, J, Liu, H, Li, J, Zhang, X, et al. Potential regulators of the senescence-associated secretory phenotype during senescence and aging. J Gerontol A Biol Sci Med Sci. (2022) 77:2207–18. doi: 10.1093/gerona/glac097
175. Roger, L, Tomas, F, and Gire, V. Mechanisms and regulation of cellular senescence. Int J Mol Sci. (2021) 22:13173. doi: 10.3390/ijms222313173
176. Ohtani, N. The roles and mechanisms of senescence-associated secretory phenotype (SASP): can it be controlled by senolysis? Inflamm Regen. (2022) 42:11. doi: 10.1186/s41232-022-00197-8
177. Ge, I, Kirschen, GW, and Wang, X. Shifted dynamics of glucose metabolism in the hippocampus during aging. Front Aging Neurosci. (2021) 13:700306. doi: 10.3389/fnagi.2021.700306
178. Marino, F, Scalise, M, Salerno, N, Salerno, L, Molinaro, C, Cappetta, D, et al. Diabetes-induced cellular senescence and senescence-associated secretory phenotype impair cardiac regeneration and function independently of age. Diabetes. (2022) 71:1081–98. doi: 10.2337/db21-0536
179. Palmer, AK, Tchkonia, T, Lebrasseur, NK, Chini, EN, Xu, M, and Kirkland, JL. Cellular senescence in type 2 diabetes: a therapeutic opportunity. Diabetes. (2015) 64:2289–98. doi: 10.2337/db14-1820
180. Van Vliet, T, Varela-Eirin, M, Wang, B, Borghesan, M, Brandenburg, SM, Franzin, R, et al. Physiological hypoxia restrains the senescence-associated secretory phenotype via AMPK-mediated mTOR suppression. Mol Cell. (2021) 81:2041–2052.E6. doi: 10.1016/j.molcel.2021.03.018
181. Marquez-Exposito, L, Tejedor-Santamaria, L, Santos-Sanchez, L, Valentijn, FA, Cantero-Navarro, E, Rayego-Mateos, S, et al. Acute kidney injury is aggravated in aged mice by the exacerbation of proinflammatory processes. Front Pharmacol. (2021) 12:662020. doi: 10.3389/fphar.2021.662020
182. Shinde, A, Deore, G, Navsariwala, KP, Tabassum, H, and Wani, M. We are all aging, and here's why. Aging Med (Milton). (2022) 5:211–31. doi: 10.1002/agm2.12223
183. Wang, Y, Wang, L, Wen, X, Hao, D, Zhang, N, He, G, et al. Nf-Κb signaling in skin aging. Mech Ageing Dev. (2019) 184:111160. doi: 10.1016/j.mad.2019.111160
184. Chen, H, Qin, J, Shi, H, Li, Q, Zhou, S, and Chen, L. Rhoifolin ameliorates osteoarthritis via the Nrf2/NF-ΚB Axis: in vitro and in vivo experiments. Osteoarthr Cartil. (2022) 30:735–45. doi: 10.1016/j.joca.2022.01.009
185. Esser, N, Legrand-Poels, S, Piette, J, Scheen, AJ, and Paquot, N. Inflammation as a link between obesity, metabolic syndrome and type 2 diabetes. Diabetes Res Clin Pract. (2014) 105:141–50. doi: 10.1016/j.diabres.2014.04.006
186. Mejías-Peña, Y, Estébanez, B, Rodriguez-Miguelez, P, Fernandez-Gonzalo, R, Almar, M, De Paz, JA, et al. Impact of resistance training on the autophagy-inflammation-apoptosis crosstalk in elderly subjects. Aging (Albany NY). (2017) 9:408–18. doi: 10.18632/aging.101167
187. Mizushima, N, and Komatsu, M. Autophagy: renovation of cells and tissues. Cells. (2011) 147:728–41. doi: 10.1016/j.cell.2011.10.026
188. Chaabane, W, User, SD, El-Gazzah, M, Jaksik, R, Sajjadi, E, Rzeszowska-Wolny, J, et al. Autophagy, apoptosis, mitoptosis and necrosis: interdependence between those pathways and effects on cancer. Arch Immunol Ther Exp. (2013) 61:43–58. doi: 10.1007/s00005-012-0205-y
189. Zhang, L, Zheng, YL, Wang, R, Wang, XQ, and Zhang, H. Exercise for osteoporosis: a literature review of pathology and mechanism. Front Immunol. (2022) 13:1005665. doi: 10.3389/fimmu.2022.1005665
190. Gu, Y, Han, J, Jiang, C, and Zhang, Y. Biomarkers, oxidative stress and autophagy in skin aging. Ageing Res Rev. (2020) 59:101036. doi: 10.1016/j.arr.2020.101036
191. Giansanti, V, Torriglia, A, and Scovassi, AI. Conversation between apoptosis and autophagy: "is it your turn or mine?". Apoptosis. (2011) 16:321–33. doi: 10.1007/s10495-011-0589-x
192. Wang, B, Wang, Y, Zhang, J, Hu, C, Jiang, J, Li, Y, et al. Ros-induced lipid peroxidation modulates cell death outcome: mechanisms behind apoptosis, autophagy, and ferroptosis. Arch Toxicol. (2023) 97:1439–51. doi: 10.1007/s00204-023-03476-6
193. Haberzettl, P, and Hill, BG. Oxidized lipids activate autophagy in a JNK-dependent manner by stimulating the endoplasmic reticulum stress response. Redox Biol. (2013) 1:56–64. doi: 10.1016/j.redox.2012.10.003
194. Kruiswijk, F, Labuschagne, CF, and Vousden, KH. P53 in survival, death and metabolic health: a lifeguard with a licence to kill. Nat Rev Mol Cell Biol. (2015) 16:393–405. doi: 10.1038/nrm4007
195. Wang, TT, Yang, Y, Wang, F, Yang, WG, Zhang, JJ, and Zou, ZQ. Docosahexaenoic acid monoglyceride induces apoptosis and autophagy in breast cancer cells via lipid peroxidation-mediated endoplasmic reticulum stress. J Food Sci. (2021b) 86:4704–16. doi: 10.1111/1750-3841.15900
196. Cheng, J, Ohsaki, Y, Tauchi-Sato, K, Fujita, A, and Fujimoto, T. Cholesterol depletion induces autophagy. Biochem Biophys Res Commun. (2006) 351:246–52. doi: 10.1016/j.bbrc.2006.10.042
197. Singh, R, Kaushik, S, Wang, Y, Xiang, Y, Novak, I, Komatsu, M, et al. Autophagy regulates lipid metabolism. Nature. (2009) 458:1131–5. doi: 10.1038/nature07976
198. Ogasawara, Y, Cheng, J, Tatematsu, T, Uchida, M, Murase, O, Yoshikawa, S, et al. Long-term autophagy is sustained by activation of CCTβ3 on lipid droplets. Nat Commun. (2020) 11:4480. doi: 10.1038/s41467-020-18153-w
199. Gao, Y, Zhang, W, Zeng, LQ, Bai, H, Li, J, Zhou, J, et al. Exercise and dietary intervention ameliorate high-fat diet-induced NAFLD and liver aging by inducing Lipophagy. Redox Biol. (2020b) 36:101635. doi: 10.1016/j.redox.2020.101635
200. An, Y, Zhang, H, Wang, C, Jiao, F, Xu, H, Wang, X, et al. Activation of ROS/MAPKs/NF-ΚB/NLRP3 and inhibition of efferocytosis in osteoclast-mediated diabetic osteoporosis. FASEB J. (2019) 33:12515–27. doi: 10.1096/fj.201802805RR
201. Liu, Y, Sun, L, Ma, Y, Wei, B, Gao, M, and Shang, L. High glucose and bupivacaine-induced cytotoxicity is mediated by enhanced apoptosis and impaired autophagy via the PERK-ATF4-CHOP and IRE1-TRAF2 signaling pathways. Mol Med Rep. (2019) 20:2832–42. doi: 10.3892/mmr.2019.10524
202. Wang, Y, Zhang, J, Zhang, L, Gao, P, and Wu, X. Adiponectin attenuates high glucose-induced apoptosis through the AMPK/P38 MAPK signaling pathway in NRK-52E cells. PLoS One. (2017) 12:E0178215. doi: 10.1371/journal.pone.0178215
203. Herzig, S, and Shaw, RJ. AMPK: guardian of metabolism and mitochondrial homeostasis. Nat Rev Mol Cell Biol. (2018) 19:121–35. doi: 10.1038/nrm.2017.95
204. Bradshaw, PC. Acetyl-CoA metabolism and histone acetylation in the regulation of aging and lifespan. Antioxidants (Basel). (2021) 10:572. doi: 10.3390/antiox10040572
205. Nie, H, and Yi, W. O-Glcnacylation, a sweet link to the pathology of diseases. J Zhejiang Univ Sci B. (2019) 20:437–48. doi: 10.1631/jzus.B1900150
206. Dong, J, Guo, C, Yang, Z, Wu, Y, and Zhang, C. Follicle-stimulating hormone alleviates ovarian aging by modulating Mitophagy- and Glycophagy-based energy metabolism in hens. Cells. (2022a) 11:3270. doi: 10.3390/cells11203270
207. Saltiel, AR, and Kahn, CR. Insulin Signalling and the regulation of glucose and lipid metabolism. Nature. (2001) 414:799–806. doi: 10.1038/414799a
208. Brown, MS, and Goldstein, JL. Cholesterol feedback: from Schoenheimer's bottle to Scap's Meladl. J Lipid Res. (2009) 50:S15–27. doi: 10.1194/jlr.R800054-JLR200
209. Samuel, VT, and Shulman, GI. Mechanisms for insulin resistance: common threads and missing links. Cells. (2012) 148:852–71. doi: 10.1016/j.cell.2012.02.017
210. Houstis, N, Rosen, ED, and Lander, ES. Reactive oxygen species have a causal role in multiple forms of insulin resistance. Nature. (2006) 440:944–8. doi: 10.1038/nature04634
211. Hotamisligil, GS. Inflammation and metabolic disorders. Nature. (2006) 444:860–7. doi: 10.1038/nature05485
212. Schrauwen, P, and Hesselink, MK. Oxidative capacity, lipotoxicity, and mitochondrial damage in type 2 diabetes. Diabetes. (2004) 53:1412–7. doi: 10.2337/diabetes.53.6.1412
213. Ozcan, U, Cao, Q, Yilmaz, E, Lee, AH, Iwakoshi, NN, Ozdelen, E, et al. Endoplasmic reticulum stress links obesity, insulin action, and type 2 diabetes. Science. (2004) 306:457–61. doi: 10.1126/science.1103160
214. Van Der Horst, A, and Burgering, BM. Stressing the role of FOXO proteins in lifespan and disease. Nat Rev Mol Cell Biol. (2007) 8:440–50. doi: 10.1038/nrm2190
215. Puigserver, P, Rhee, J, Donovan, J, Walkey, CJ, Yoon, JC, Oriente, F, et al. Insulin-regulated hepatic gluconeogenesis through FOXO1-PGC-1alpha interaction. Nature. (2003) 423:550–5. doi: 10.1038/nature01667
216. Essers, MA, Weijzen, S, De Vries-Smits, AM, Saarloos, I, De Ruiter, ND, Bos, JL, et al. Foxo transcription factor activation by oxidative stress mediated by the small GTPase Ral and JNK. EMBO J. (2004) 23:4802–12. doi: 10.1038/sj.emboj.7600476
217. Zhao, J, Brault, JJ, Schild, A, Cao, P, Sandri, M, Schiaffino, S, et al. Foxo3 coordinately activates protein degradation by the autophagic/lysosomal and proteasomal pathways in atrophying muscle cells. Cell Metab. (2007) 6:472–83. doi: 10.1016/j.cmet.2007.11.004
218. Kenyon, C, Chang, J, Gensch, E, Rudner, A, and Tabtiang, R. A C. elegans mutant that lives twice as long as wild type. Nature. (1993) 366:461–4. doi: 10.1038/366461a0
219. Murao, N, Yokoi, N, Takahashi, H, Hayami, T, Minami, Y, and Seino, S. Increased glycolysis affects Β-cell function and identity in aging and diabetes. Mol Metab. (2022) 55:101414. doi: 10.1016/j.molmet.2021.101414
220. Aguayo-Mazzucato, C, Andle, J, Lee, TB, Midha, A, Talemal, L, Chipashvili, V, et al. Acceleration of Β cell aging determines diabetes and senolysis improves disease outcomes. Cell Metab. (2019) 30:129–142.e4. doi: 10.1016/j.cmet.2019.05.006
221. Bertelli, PM, Pedrini, E, Hughes, D, Mcdonnell, S, Pathak, V, Peixoto, E, et al. Long term high glucose exposure induces premature senescence in retinal endothelial cells. Front Physiol. (2022) 13:929118. doi: 10.3389/fphys.2022.929118
222. Nerstedt, A, and Smith, U. The impact of cellular senescence in human adipose tissue. J Cell Commun Signal. (2023) 17:563–73. doi: 10.1007/s12079-023-00769-4
223. Chung, KW. Advances in understanding of the role of lipid metabolism in aging. Cells. (2021) 10:880. doi: 10.3390/cells10040880
224. Strable, MS, and Ntambi, JM. Genetic control of de novo lipogenesis: role in diet-induced obesity. Crit Rev Biochem Mol Biol. (2010) 45:199–214. doi: 10.3109/10409231003667500
225. Dong, Y, Yu, M, Wu, Y, Xia, T, Wang, L, Song, K, et al. Hydroxytyrosol promotes the mitochondrial function through activating mitophagy. Antioxidants (Basel). (2022b) 11:893. doi: 10.3390/antiox11050893
226. Wu, K, Zhao, T, Hogstrand, C, Xu, Y-C, Ling, S-C, Chen, G-H, et al. FXR-mediated inhibition of autophagy contributes to FA-induced TG accumulation and accordingly reduces FA-induced lipotoxicity. Cell Commun Signal. (2020) 18:47. doi: 10.1186/s12964-020-0525-1
227. Cheon, SY, and Cho, K. Lipid metabolism, inflammation, and foam cell formation in health and metabolic disorders: targeting mTORC1. J Mol Med. (2021) 99:1497–509. doi: 10.1007/s00109-021-02117-8
228. Sears, B, and Perry, M. The role of fatty acids in insulin resistance. Lipids Health Dis. (2015) 14:121. doi: 10.1186/s12944-015-0123-1
229. Chen, L, Chen, X-W, Huang, X, Song, B-L, Wang, Y, and Wang, Y. Regulation of glucose and lipid metabolism in health and disease. Sci China Life Sci. (2019) 62:1420–58. doi: 10.1007/s11427-019-1563-3
230. Xie, Y, Li, J, Kang, R, and Tang, D. Interplay between lipid metabolism and autophagy. Front Cell Dev Biol. (2020) 8:431. doi: 10.3389/fcell.2020.00431
231. Zhang, H, Li, X, Fan, W, Pandovski, S, Tian, Y, and Dillin, A. Inter-tissue communication of mitochondrial stress and metabolic health. Life Metab. (2023) 2:Load001. doi: 10.1093/lifemeta/load001
232. Hwang, M-J, Kim, J-H, Koo, Y-S, Yun, H-Y, and Cheong, H-K. Impacts of ambient air pollution on glucose metabolism in Korean adults: a Korea National Health and Nutrition Examination Survey Study. Environ Health. (2020) 19:70. doi: 10.1186/s12940-020-00623-9
233. Qin, J, Xia, W, Liang, G, Xu, S, Zhao, X, Wang, D, et al. Association of fine particulate matter with glucose and lipid metabolism: a longitudinal study in young adults. Occup Environ Med. (2021) 78:448–53. doi: 10.1136/oemed-2020-107039
234. Yitshak Sade, M, Kloog, I, Liberty, IF, Schwartz, J, and Novack, V. The association between air pollution exposure and glucose and lipids levels. J Clin Endocrinol Metabol. (2016) 101:2460–7. doi: 10.1210/jc.2016-1378
235. Liu, X, Khalil, AEMM, Muthukumarasamy, U, Onogi, Y, Yan, X, Singh, I, et al. Reduced intestinal lipid absorption improves glucose metabolism in aged G2-Terc knockout mice. BMC Biol. (2023) 21:150. doi: 10.1186/s12915-023-01629-8
236. Prentki, M, and Madiraju, SRM. Glycerolipid metabolism and signaling in health and disease. Endocr Rev. (2008) 29:647–76. doi: 10.1210/er.2008-0007
237. Yang, Q, Mumusoglu, S, Qin, Y, Sun, Y, and Hsueh, AJ. A kaleidoscopic view of ovarian genes associated with premature ovarian insufficiency and senescence. FASEB J. (2021) 35:E21753. doi: 10.1096/fj.202100756R
238. Zhou, X-Y, Li, X, Zhang, J, Li, Y, Wu, X-M, Yang, Y-Z, et al. Plasma metabolomic characterization of premature ovarian insufficiency. J Ovarian Res. (2023) 16:2. doi: 10.1186/s13048-022-01085-y
239. Santoro, N, Epperson, CN, and Mathews, SB. Menopausal symptoms and their management. Endocrinol Metab Clin N Am. (2015) 44:497–515. doi: 10.1016/j.ecl.2015.05.001
240. D'eon, TM, Souza, SC, Aronovitz, M, Obin, MS, Fried, SK, and Greenberg, AS. Estrogen regulation of adiposity and fuel partitioning. Evidence of genomic and non-genomic regulation of lipogenic and oxidative pathways. J Biol Chem. (2005) 280:35983–91. doi: 10.1074/jbc.M507339200
242. Paes, AMDA, Gaspar, RS, Fuentes, E, Wehinger, S, Palomo, I, and Trostchansky, A. Lipid metabolism and signaling in platelet function In: A Trostchansky and H Rubbo, editors. Bioactive lipids in health and disease. Cham: Springer International Publishing (2019)
243. Huang, J, Zhang, P, Solari, FA, Sickmann, A, Garcia, A, Jurk, K, et al. Molecular proteomics and signalling of human platelets in health and disease. Int J Mol Sci. (2021) 22:9860. doi: 10.3390/ijms22189860
244. Peng, L, Baradar, AA, Aguado, J, and Wolvetang, E. Cellular senescence and premature aging in down syndrome. Mech Ageing Dev. (2023) 212:111824. doi: 10.1016/j.mad.2023.111824
245. Bian, X, Liu, R, Meng, Y, Xing, D, Xu, D, and Lu, Z. Lipid metabolism and Cancer. J Exp Med. (2020) 218:e20201606. doi: 10.1084/jem.20201606
246. Jeong, D-W, Lee, S, and Chun, Y-S. How cancer cells remodel lipid metabolism: strategies targeting transcription factors. Lipids Health Dis. (2021) 20:163. doi: 10.1186/s12944-021-01593-8
247. Qin, C, Yang, G, Yang, J, Ren, B, Wang, H, Chen, G, et al. Metabolism of pancreatic cancer: paving the way to better anticancer strategies. Mol Cancer. (2020) 19:50. doi: 10.1186/s12943-020-01169-7
248. Wang, W, Bai, L, Li, W, and Cui, J. The lipid metabolic landscape of cancers and new therapeutic perspectives. Front Oncol. (2020a) 10:605154. doi: 10.3389/fonc.2020.605154
249. Dean, EJ, Falchook, GS, Patel, MR, Brenner, AJ, Infante, JR, Arkenau, H-T, et al. Preliminary activity in the first in human study of the first-in-class fatty acid synthase (FASN) inhibitor, TVB-2640. J Clin Oncol. (2016) 34:2512–2. doi: 10.1200/JCO.2016.34.15_suppl.2512
250. Beckers, A, Organe, S, Timmermans, L, Scheys, K, Peeters, A, Brusselmans, K, et al. Chemical inhibition of acetyl-CoA carboxylase induces growth arrest and cytotoxicity selectively in cancer cells. Cancer Res. (2007) 67:8180–7. doi: 10.1158/0008-5472.CAN-07-0389
251. She, K, Fang, S, Du, W, Fan, X, He, J, Pan, H, et al. SCD1 is required for EGFR-targeting cancer therapy of lung cancer via re-activation of EGFR/PI3K/AKT signals. Cancer Cell Int. (2019) 19:103. doi: 10.1186/s12935-019-0809-y
252. Guo, D, Reinitz, F, Youssef, M, Hong, C, Nathanson, D, Akhavan, D, et al. An LXR agonist promotes glioblastoma cell death through inhibition of an EGFR/AKT/SREBP-1/LDLR-dependent pathway. Cancer Discov. (2011) 1:442–56. doi: 10.1158/2159-8290.CD-11-0102
253. Dockendorff, C, Faloon, PW, Germain, A, Yu, M, Youngsaye, W, Nag, PP, et al. Discovery of bisamide-heterocycles as inhibitors of scavenger receptor Bi (SR-BI)-mediated lipid uptake. Bioorg Med Chem Lett. (2015) 25:2594–8. doi: 10.1016/j.bmcl.2015.03.074
254. Iwamoto, H, Abe, M, Yang, Y, Cui, D, Seki, T, Nakamura, M, et al. Cancer lipid metabolism confers antiangiogenic drug resistance. Cell Metab. (2018) 28:E5. doi: 10.1016/j.cmet.2018.05.005
255. Mahboubi Rabbani, SMI, and Zarghi, A. Selective cox-2 inhibitors as anticancer agents: a patent review (2014-2018). Expert Opin Ther Pat. (2019) 29:407–27. doi: 10.1080/13543776.2019.1623880
256. Meduri, B, Pujar, GV, Durai Ananda Kumar, T, Akshatha, HS, Sethu, AK, Singh, M, et al. Lysophosphatidic acid (LPA) receptor modulators: structural features and recent development. Eur J Med Chem. (2021) 222:113574. doi: 10.1016/j.ejmech.2021.113574
257. Preta, G. New insights into targeting membrane lipids for cancer therapy. Front Cell Dev Biol. (2020) 8:571237. doi: 10.3389/fcell.2020.571237
258. Lin, X, Xiao, Z, Chen, T, Liang, SH, and Guo, H. Glucose metabolism on tumor plasticity, diagnosis, and treatment. Front Oncol. (2020) 10:317. doi: 10.3389/fonc.2020.00317
259. Bose, S, and Le, A. Glucose metabolism in cancer. Adv Exp Med Biol. (2018) 1063:3–12. doi: 10.1007/978-3-319-77736-8_1
260. Ancey, PB, Contat, C, Boivin, G, Sabatino, S, Pascual, J, Zangger, N, et al. Glut1 expression in tumor-associated neutrophils promotes lung cancer growth and resistance to radiotherapy. Cancer Res. (2021) 81:2345–57. doi: 10.1158/0008-5472.CAN-20-2870
261. Ganapathy-Kanniappan, S, and Geschwind, J-FH. Tumor glycolysis as a target for cancer therapy: progress and prospects. Mol Cancer. (2013) 12:152. doi: 10.1186/1476-4598-12-152
262. Rodríguez-Enríquez, S, Gallardo-Pérez, JC, Marín-Hernández, A, Aguilar-Ponce, JL, Mandujano-Tinoco, EA, Meneses, A, et al. Oxidative phosphorylation as a target to arrest malignant neoplasias. Curr Med Chem. (2011) 18:3156–67. doi: 10.2174/092986711796391561
263. Zhao, B, Wu, B, Feng, N, Zhang, X, Zhang, X, Wei, Y, et al. Aging microenvironment and antitumor immunity for geriatric oncology: the landscape and future implications. J Hematol Oncol. (2023) 16:28. doi: 10.1186/s13045-023-01426-4
264. Kasakovski, D, Xu, L, and Li, Y. T cell senescence and CAR-T cell exhaustion in hematological malignancies. J Hematol Oncol. (2018) 11:91. doi: 10.1186/s13045-018-0629-x
265. Prasanna, PG, Citrin, DE, Hildesheim, J, Ahmed, MM, Venkatachalam, S, Riscuta, G, et al. Therapy-induced senescence: opportunities to improve anticancer therapy. J Natl Cancer Inst. (2021) 113:1285–98. doi: 10.1093/jnci/djab064
266. Cham, CM, Driessens, G, O'keefe, JP, and Gajewski, TF. Glucose deprivation inhibits multiple key gene expression events and effector functions in CD8+ T cells. Eur J Immunol. (2008) 38:2438–50. doi: 10.1002/eji.200838289
267. Liu, X, Hartman, CL, Li, L, Albert, CJ, Si, F, Gao, A, et al. Reprogramming lipid metabolism prevents effector T cell senescence and enhances tumor immunotherapy. Sci Transl Med. (2021) 13:eaaz6314. doi: 10.1126/scitranslmed.aaz6314
268. Johnson, AA, and Stolzing, A. The role of lipid metabolism in aging, lifespan regulation, and age-related disease. Aging Cell. (2019) 18:E13048. doi: 10.1111/acel.13048
269. Mutlu, AS, Duffy, J, and Wang, MC. Lipid metabolism and lipid signals in aging and longevity. Dev Cell. (2021) 56:1394–407. doi: 10.1016/j.devcel.2021.03.034
Keywords: senescence, lipid metabolism, glycolysis, CPT1, ACOX1, ACC, TCA, PPP
Citation: Liu B, Meng Q, Gao X, Sun H, Xu Z, Wang Y and Zhou H (2023) Lipid and glucose metabolism in senescence. Front. Nutr. 10:1157352. doi: 10.3389/fnut.2023.1157352
Edited by:
Gabriela Salim de Castro, University of São Paulo, BrazilReviewed by:
Rantao Zuo, Dalian Ocean University, ChinaDenggang Fu, Indiana University, United States
Copyright © 2023 Liu, Meng, Gao, Sun, Xu, Wang and Zhou. This is an open-access article distributed under the terms of the Creative Commons Attribution License (CC BY). The use, distribution or reproduction in other forums is permitted, provided the original author(s) and the copyright owner(s) are credited and that the original publication in this journal is cited, in accordance with accepted academic practice. No use, distribution or reproduction is permitted which does not comply with these terms.
*Correspondence: Zhixiang Xu, emhpeGlhbmd4dUBqbHUuZWR1LmNu; Yishu Wang, d2FuZ3lzQGpsdS5lZHUuY24=; Honglan Zhou, aGx6aG91QGpsdS5lZHUuY24=
†These authors have contributed equally to this work and share first authorship