- 1School of Molecular and Life Sciences, Curtin University, Bentley, WA, Australia
- 2Ingredients by Design Pty Ltd., Perth, WA, Australia
High pressure processing is a non-thermal method for preservation of various foods while retaining nutritional value and can be utilized for the development of ready-to-eat products. This original research investigated the effects of high pressure processing for development of a ready-to eat chickpea product using Australian kabuli chickpeas. Three pressure levels (200, 400, and 600 MPA) and two treatment times (1 and 5 min) were selected to provide six distinct samples. When compared to the conventionally cooked chickpeas, high pressure processed chickpeas had a more desirable texture due to decrease in firmness, chewiness, and gumminess. The general nutrient composition and individual mineral content were not affected by high pressure processing, however, a significant increase in the slowly digestible starch from 50.53 to 60.92 g/100 g starch and a concomitant decrease in rapidly digestible starch (11.10–8.73 g/100 g starch) as well as resistant starch (50.53–30.35 g/100 g starch) content was observed. Increased starch digestibility due to high pressure processing was recorded, whereas in vitro protein digestibility was unaffected. Significant effects of high pressure processing on the polyphenol content and antioxidant activities (DPPH, ABTS and ORAC) were observed, with the sample treated at the highest pressure for the longest duration (600 MPa, 5 min) showing the lowest values. These findings suggest that high pressure processing could be utilized to produce a functional, ready to eat kabuli chickpea product with increased levels of beneficial slowly digestible starch.
Introduction
High pressure processing (HPP) is a novel method of preserving foods, which is an alternative to standard heat treatment methods. It produces high quality and safe food which have a longer shelf life than conventionally stored food due to reduction of food spoiling microorganisms (1, 2). High pressure processing has been shown to retain quality attributes such as color, flavor and the nutritional value of foods (2, 3). In brief, during high pressure processing, at any given time, the pressure is transmitted uniformly by the pressure transmitting medium and is independent of the shape and size of the product being treated. The effectiveness of high pressure treatment depends on treatment time, pressure and temperature level, rate of pressurization/decompression, and on the composition of the food (4).
The demand for plant based protein is steadily growing around the world and is estimated to be a USD 14.5 billion market by 2025 (5). In the last 5 years increasing number of people shifted animal-based proteins to plant-based proteins sources in their meals (6, 7). Chickpeas (Cicer arietinum L.) are widely grown worldwide, are already a popular food source for humans and are an excellent source of proteins (8). However, the time required to cook chickpeas makes them a less popular choice amongst the younger generation, which prefers healthy and convenient food options requiring less preparation time (9).
As HPP is known to increase the shelf life of foods without affecting the sensory and nutritional properties (2), cooked and high pressure processed chickpeas could be introduced as a ready-to-eat option, with desirable textural, organoleptic and nutritional properties. However, there is a scarcity of knowledge on the effects of HPP on the textural, nutritional and bioactive properties of legumes in general. HPP denatures proteins reversibly and irreversibly. A moderate pressure (<300 MPa) affects the acceleration of enzyme action whereas, a pressure above 300 MPa induces protein denaturation and inactivation of enzymes (3, 10). Previous studies have reported the effect of high pressure on lentil starch (11), mung bean starch (12), and pea starch (13), however, no information is available on both protein and starch digestibility of HPP chickpeas.
A small decrease in the total polyphenols (9) and antioxidant properties of HPP vegetables have been reported by Butz et al. (14) whereas Doblado et al. (15) reported slight changes in the antioxidant capacity and vitamin C content of HPP germinated cowpeas [Vigna unguiculata (L.)], a grain legume common in sub-Saharan Africa, following high pressure treatment. A recent study by Alsalman and Ramaswamy (16) reported changes in the texture, color and antinutrient (tannins and phytic acid) content of raw Canadian kabuli chickpeas, but did not explore the changes in starch or protein digestibility, polyphenol content and antioxidant capacities of cooked chickpeas. Therefore, there is a need to investigate the effect of high pressure processing on the texture profile, general nutrient composition, starch and protein digestibility, polyphenol content and antioxidant capacity of cooked and HPP kabuli chickpeas, compared to cooked only samples to inform ready to eat product development in the near future. To fill this knowledge gap, the objective of this research work was to examine the effects of HPP (200, 400, and 600 MPa for 1 and 5 min) on the textural, nutritional, and bioactive properties of cooked Australian kabuli chickpeas.
Materials and Methods
Representative samples of the cultivar Kimberley Large kabuli chickpeas (17) harvested in 2017 from the Ord River region of Western Australia were collected, sieved, vacuum packaged and stored at 4°C until analysis. The kabuli chickpeas were soaked in excess water overnight (12 h) at room temperature (22°C). After 12 h, the remaining water was drained off and chickpeas were cooked in fresh boiling water on a gas stove for 30 min as the seeds still maintained their shape and firm texture. Cooking for a longer duration resulted in seed coat separation. Following cooking, chickpeas were cooled by washing them under running tap water and excess moisture was removed using paper towel. The cooked and cooled chickpeas were vacuum packaged in a 100 micron vacuum packaging bag using a Multivac double chamber vacuum packaging machine (Model- C450, Multivac, Keilor Park, Victoria, Australia) and stored at 4°C until high pressure processing.
High Pressure Processing
Cooked and vacuum packaged Kimberley Large kabuli chickpeas were commercially high pressure processed at Preshafoods Pty. Ltd. (Derrimut, Victoria, Australia) using hyperbaric high pressure processing equipment (Model- Hyperbaric 300, Burgos, Spain, EU) at the following settings detailed in Table 1.
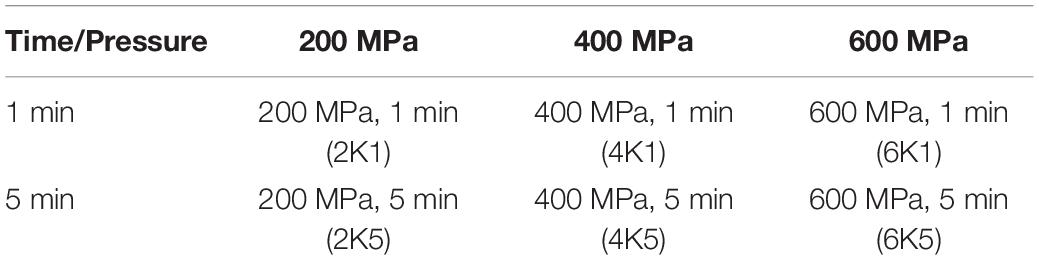
Table 1. Pressure and time combinations for high pressure processing of cultivar Kimberley Large chickpea samples.
The time required to reach 200, 400, and 600 MPa was 60, 130, and 240 s, respectively, and the decompression was instant. Purified water was used as the pressurization media and all the samples were pressurized at 4°C. After processing, the samples were stored at 4°C and transported to Curtin University (Bentley, Western Australia) under refrigerated conditions. Cooked and vacuum packaged Kimberley Large kabuli chickpeas which were not subjected to high pressure processing were used as a control.
Texture Profile Analysis
Texture profile analysis of the control and high pressure processed chickpeas was conducted using a Perten Texture analyzer (TVT6700, Hägersten, Sweden) (18). The samples were subjected to 50% compression with a perspex cylindrical probe (25 mm diameter) at a crosshead speed of 1 mm/s twice in two cycles using a 5 kg load cell. The texture profile was expressed as firmness, cohesiveness, springiness, gumminess and chewiness. Forty replicates were analyzed for control and each HPP sample.
Moisture Content
Moisture content was determined in duplicate by the modified solids-(total) and moisture in flour-oven drying method (Method 925.10, (19).
Protein Content
Protein content was determined in duplicate by Kjeldahl assay: digestion, distillation and titration using a nitrogen conversion factor of 6.25 (Method 920.87) (20).
Total Starch Content
Starch content was determined in duplicate using the standard colorimetric technique (Method 996.11) (21), using the total starch assay kit (AA/AMG) from Megazyme International Ltd (Bray, County Wicklow, Ireland).
Freeze Drying
The chickpea samples were placed in a freezer over night at –20°C. The samples were transferred to a freeze dryer (Christ Alpha 1-4LD Plus, Germany) and freeze-dried at 1 mbar pressure and –50°C for 4 days. The freeze-dried samples were milled (Grindomix, GM200, Retsch, Haan, Germany) to get a fine powder, passing 100% through 500 microns.
Mineral Content
Inductively coupled plasma optical emission spectrometry (ICP-OES) (Varian, Palo Alto, United States) was used to determine the content of individual minerals (mg) in duplicate at the National Measurement Institute, Perth, WA. Freeze-dried control and HPP samples (1 g) were digested at 95°C for 2 h in a DEENA automated digestion block (Thomas Cain, Omaha, Nebraska, United States), after addition of concentrated nitric acid (HNO3) (3 mL) and concentrated HCl (3 mL). After digestion, distilled water was used to make the sample volume up to 40 mL, and the solutions were left to settle. Afterward, all the samples were diluted fivefolds with distilled water before being analyzed using ICP-OES. Appropriate emission wavelengths (higher sensitivity and lower interferences) of 238.204, 317.933, 213.618, 213.857, 279.078, 766.491, and 589.592 nm were chosen to analyze Fe, Ca, P, Zn, Mg, K, and Na, respectively (22). The mineral content was expressed as mg/kg sample.
Polyphenol Extraction and Total Polyphenol Content
To extract total polyphenols, freeze-dried control and HPP samples (1 g) were mixed with 10 mL 50% acetone solution. The mixture was shaken at ambient temperature at 60 rpm for 3 (h) using a suspension mixer (RSM7DC, Ratek, Boronia, Victoria, Australia). The mixture was extracted for an additional 12–16 h in the dark overnight. The extract was centrifuged using an Eppendorf centrifuge (Model—5810r, Hamburg, Germany) at 12,000 g, for 10 min at room temperature, and the extracts were kept in the dark at −20°C until use (23).
Total polyphenol content was determined using Folin-Ciocalteu method (24). In brief, 100 μL of polyphenol extracts were mixed with 2.5 mL of 0.2 N Folin-Ciocalteu reagent, followed by 2 mL of saturated sodium carbonate solution (75 g/L) addition. After a reaction time of 2 h at room temperature in the dark, the absorbance of obtained mixtures was determined using a UV-1800 Spectrophotometer (Shimadzu, Canby, United States) at 765 nm. A standard curve was prepared using Gallic acid (0–360 mg/L) and results were expressed as mg Gallic acid equivalents (GAE)/100 g dry basis (db). All extract were analyzed in duplicate.
Antioxidant Capacity
For the 2, 2-diphenyl-1-picrylhydrazyl (DPPH) assay, firstly, DPPH (24 mg) was added into 100 mL methanol to prepare the stock solution, which was stored at –20°C in the dark until use. To prepare the working solution, a 10 mL stock solution was diluted with 50 mL methanol, giving an absorbance of 1.1 ± 0.02 units at 515 nm. Duplicate kabuli chickpea phenolic extracts (150 μL) were mixed with the DPPH working solution (2,850 μL) and reacted for 2 h in the dark, after which the absorbance was determined at 515 nm and results were expressed in terms of mg Trolox equivalents (TE)/g db. Different concentrations of trolox (20–250 mg/L) were used to prepare a standard curve for DPPH antioxidant activity determination.
For the 3-ethylbenzothiazoline-6-sulfonic acid diammonium salt (ABTS) assay, a fresh stock solution was prepared by mixing 7.4 mM ABTS and 2.6 mM potassium per sulfate in equal amounts, which were kept for the duration of 12 h in the dark. Using the stock solution, a fresh ABTS working solution was prepared by diluting 1 mL of the stock solution with 60 mL of methanol to get a 1.1 ± 0.02 units absorbance at 734 nm. The kabuli chickpea phenolic extract (150 μL) was mixed with the ABTS working solution (2,850 μL) and incubated in the dark for 2 h, followed by absorbance determination at 734 nm. The standard used for the ABTS assay was Trolox (20–250 mg/L) and the results were expressed in terms of mg Trolox equivalents (TE)/g db, with all extracts being analyzed in duplicate (25). The oxygen radical absorbance capacity (ORAC) assay was performed using a modified method (26). All extracts were analyzed in duplicate and the final antioxidant activity results were calculated using Trolox as a standard (0–50 μmol/L), and expressed as μmol TE/100 g sample, db.
Starch Isolation
Starch from the freeze-dried control and high pressure processed chickpea samples was isolated using the method of Sun et al. (27). Freeze-dried high pressure processed chickpea sample (250 g) was steeped in water containing 0.1% sodium sulfite for 12 h at 25°C. The slurry obtained was then diluted with 100 mL distilled water, and the pH was adjusted to pH 10 using 0.5 M sodium hydroxide (NaOH). The slurry was continuously mixed using a magnetic stirrer (500 rpm) for 1 h and then filtered through a 100 mesh sieve (0.149 mm nominal sieve opening) to separate the fiber from the starch. The filtered slurry was centrifuged at 3,000 g for 10 min at 10°C (Eppendorf Centrifuge 5810R made in Germany) and the supernatant was discarded. The centrifugation step was repeated six times until a clear supernatant was obtained whereas, pure starch was the white sediment remaining at the bottom. The isolated starch was dried using a digital hot air oven (Thermotec 200, Contherm, Lower Hutt, New Zealand) at 40°C for 48 h and ground into a fine powder using a pestle and mortar.
Scanning Electron Microscopy
Isolated starch sample morphology was investigated using Tescan Mira3 FESEM with Oxford Instruments X-MaxN 150 silicon drift X-ray detector and Aztec software. Starch samples were fixed on a circular metallic microscope stub with carbon aluminum tape and then coated with a 5 nm platinum coating using a sputter coater (208HR, Cressington). The scanning electron microscopy was performed at an accelerating voltage of 2 kV.
Attenuated Total Reflectance Fourier Transform Infrared Spectra Analysis
Attenuated total reflectance Fourier transform infrared spectra (ATR-FTIR) were collected using a Nicolet IFS50 FTIR spectrometer, and a single bounce diamond ATR accessory. Spectra were recorded by placing the milled freeze-dried sample in contact with the ATR accessory. Spectra were recorded across the spectral range 4,000–400 cm–1 at 4 cm–1 spectral resolution, with 128 co-added scans. The background spectrum was collected from blank diamond ATR crystals. Spectra were post normalized using vector normalization across the spectra range 875–1,190 cm–1, using OPUS software (v7.0). Second-derivatives of the FTIR spectra were then calculated using a 17-smoothing point Savitzky-Golay function.
In vitro Slowly Digested Starch
In vitro slowly digested starch was determined in duplicate on fresh sample by a modified rapid glucometer method (28). A 250 mg weighed sample was placed in a 150 mL glass jar (Ergo Flint Glass 70 mm IM-106PK-1432-FL12, Plasdene Glass-Pak, Canning Vale, Australia) to which 1 mL of porcine α-amylase (250 U/mL in 0.2 M pH 7 carbonate buffer) was added. Twenty seconds later, 5 mL pepsin suspension [9 mg of pepsin (2,500 units/mg) and 5 mL 0.02 molar hydrochloric acid] was added that lowered the pH to that approximating in vivo gastric conditions (pH 2.0). The mixture was incubated at 37°C in a reticulating water bath (85 rpm) for 30 min prior to neutralization with 5 mL 0.02 M NaOH and 50 mL 0.2 M pH 6 sodium acetate buffer. This was followed by 5 mL pancreatin mixture [0.095 g pancreatin (Chem-Supply PL378) added to 3.325 mL Diaxame and 44.175 mL 0.2 M acetate buffer (pH 6.0)]. Incubation continued at 37°C in a reticulating water bath (85 rpm). Duplicate glucose readings were made at 0, 5, 10, 20, 40, 60, 90, and 120 min from the time of addition of the pancreatin mixture using an Accu Check Performa glucometer (Roche Diagnostics Aust. Pty. Ltd, Castle Hill, Australia). Slowly digested starch (SDS) in g per 100 g dry starch was calculated using Eq. (1).
where, G120 = glucometer reading (mM) at 120 min, G20 = glucometer reading (mM) at 20 min, V = volume of digesta (mL), 180 = molecular weight of glucose, W = weight of sample (g), S = starch content of sample (g per 100 g dry sample), M = moisture content of sample (g per 100 g sample), and 0.9 = stoichiometric constant for starch from glucose contents. The glucometer was corrected using a calibration curve with known concentrations of glucose in digesta at 37°C measured in duplicate with the glucometer. The glucometer readings were corrected for the readings at time zero which represented the free glucose present in the enzyme preparations used in the study.
Protein Digestibility
In-vitro protein digestibility of control and HPP sample (6K5) was determined following a pepsin digestion method (29) with a few modifications. Approximately, 100 mg freeze-dried chickpea sample were incubated at 37°C with 0.75 mg pepsin (2,500 units/mg activity; Chem-Supply, Gillman, SA, Australia) in 7.5 ml of 0.1 N hydrochloric acid (HCl) for 5 h. The solution was then neutralized with 3.75 ml of 0.2 N NaOH. The undigested protein in 15 ml of digesta was then precipitated by addition of 25 ml of 10% tricholoroacetic acid and the sample centrifuged for 30 min at 2,000 × g at room temperature (22°C). Nitrogen in the supernatant was determined using the Kjeldahl digestion and distillation method. The in-vitro protein digestibility (IVPD) was calculated according to Eq. (2).
Statistical Analysis
Two-way analysis of variance (ANOVA) was used to compare means of replicate analyzes per treatment followed by Duncan’s multiple range test to separate means when F was significant (<0.05). Dunnett’s post-hoc test was used to compare mean values of the control against those of high pressure treated samples. All tests were performed using Genstat statistical tool (Version- V.20.1.2.24528, VSN International Ltd., United Kingdom).
Results and Discussion
Texture Profile Analysis
A typical texture profile analysis (TPA) curve for cooked kabuli chickpea samples is shown in Figure 1. Texture measurements of control and HPP samples are presented in Figures 2, 3, where high pressure processing resulted in significant (p < 0.05) changes in TPA parameters when compared to the control following ANOVA. In addition, the 5 min application at all pressures lead to a significant (p < 0.05) reduction in TPA values than samples treated for 1 min. The firmness of samples ranged from 15.27 to 25.28 N and was highest for the control and lowest for 6K5. Two-way analysis of variance (ANOVA) showed a significant effect (P < 0.05) of pressure (P) on firmness of samples, however, no effect of time (T) or pressure and time (P x T) interaction was observed. Samples treated at 200 and 400 MPa had similar firmness values, however, with the increase in treatment pressure to 600 MPa, a significant reduction in firmness of samples was observed. When compared to the control, even the lowest processing treatment (2K1) significantly (p < 0.05) reduced the firmness of cooked chickpeas and all high pressure treated samples were significantly (p < 0.05) less firm than the control, with 6K5 being the softest. Alsalman and Ramaswamy (16) also reported a decrease in firmness of chickpeas with increase in pressure (from 100 to 400 MPa). However, an increase in pressure to 600 MPa led to an increase in firmness, possibly due to aggregation of protein in the uncooked samples used in this study (30). Tissue collapse, weakened hydrophobic interactions of protein matrix and internal redistribution of moisture can be attributed to texture degradation (31), resulting in loss of firmness.
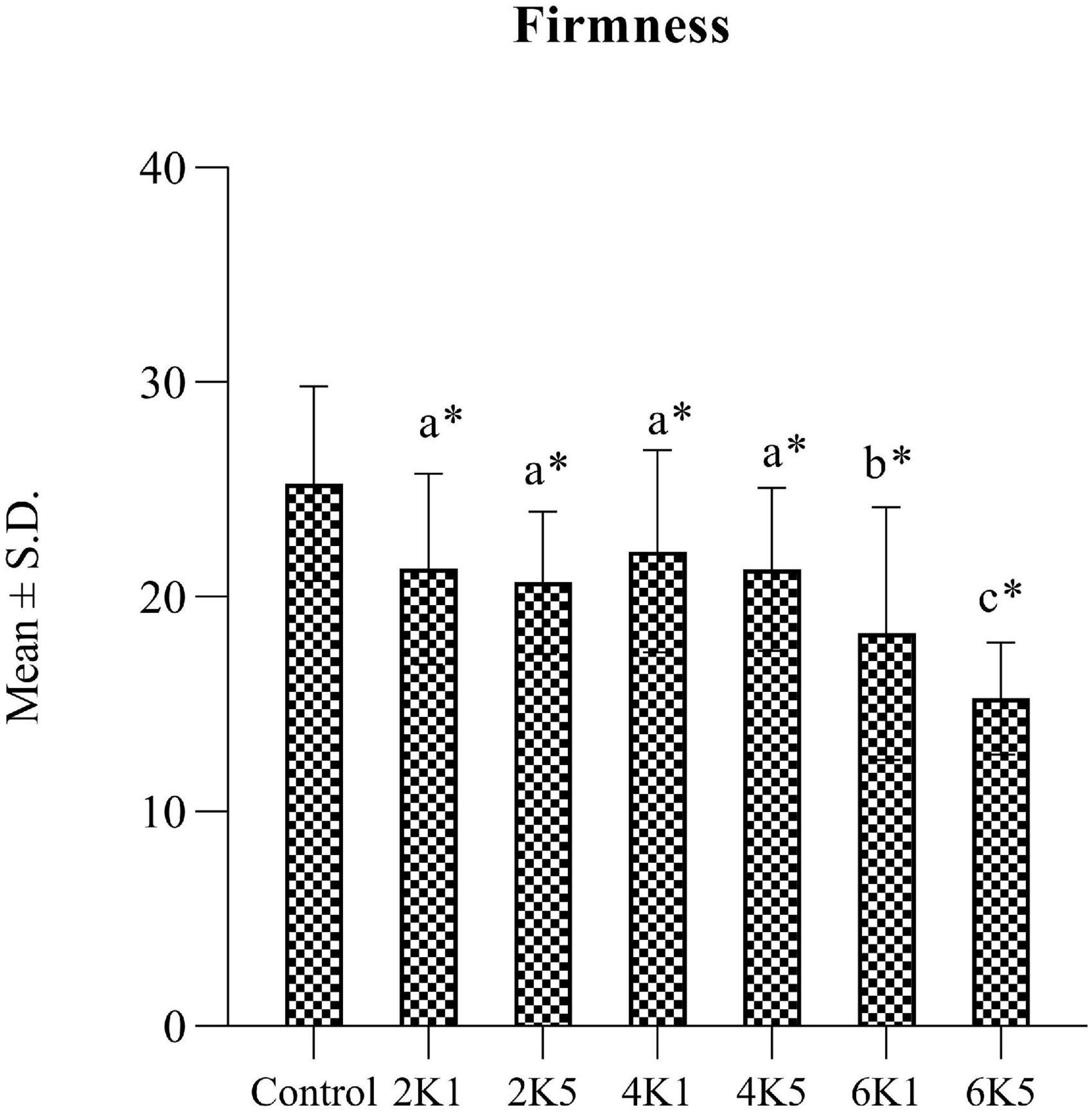
Figure 2. Firmness (N) of control and HPP chickpea samples (2K1 = 200 MPa, 1 min; 2K5 = 200 MPa, 5 min; 4K1 = 400 MPa, 1 min; 4K5 = 400 MPa, 5 min; 6k1 = 600 MPa, 1 min; and 6K5 = 600 MPa, 5 min). Bars bearing different letter are significantly (p < 0.05) different to each other. Bars bearing * are significantly (p < 0.05) different to the control.
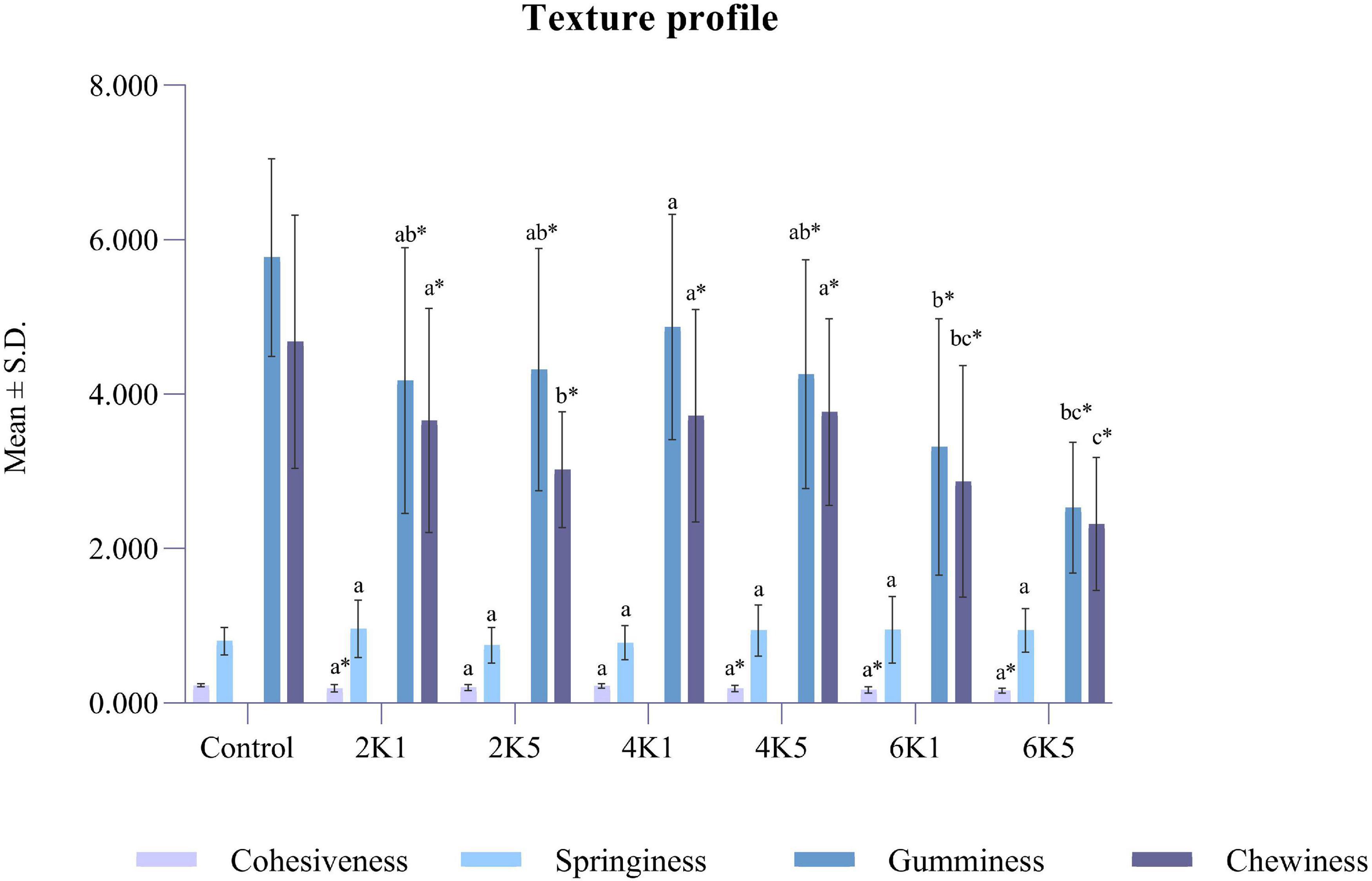
Figure 3. Texture profile of control and HPP chickpea samples (where, 2K1 = 200 MPa, 1 min; 2K5 = 200 MPa, 5 min; 4K1 = 400 MPa, 1 min; 4K5 = 400 MPa, 5 min; 6k1 = 600 MPa, 1 min; and, 6K5 = 600 MPa, 5 min). Bars bearing different letter for the same parameter are significantly (p < 0.05) different. Bars bearing * for the same parameter are significantly (p < 0.05) different to the control.
Cohesiveness in the texture profile analysis indicates how well a product withstands a second deformation following the first. Cohesiveness for cooked and chickpeas ranged between 0.16 and 0.23 and was lowest for 6K5 and highest for the control. Cohesiveness can be influenced by the depressurization process and hence a more pronounced effect can be observed for samples treated at 600 MPa. Springiness, gumminess, and chewiness for the cooked and samples ranged between 0.80 and 0.96 mm, 2.53–5.77 N, and 2.32–4.68 N mm, respectively. Similar to the firmness, cohesiveness, gumminess and chewiness of treated samples were significantly (p < 0.05) affected by the pressure treatment (P), however, there was no effect of time (T) or P x T interaction on these texture properties. Cohesiveness values for 2K1, 4K5, 6K1, and 6K5 were significantly (P < 0.05) lower than the control. Also, all samples were significantly (P < 0.05) less gummy (except 4K1) and less chewy than the control. Springiness is a degree to which cooked grains can return to their original shape after partial compression. Springiness negatively correlates with hardness and chewiness, suggesting that springier samples are less hard and less chewy (32). No effect of high pressure processing on springiness of cooked kabuli chickpeas was observed in this study.
The effect of high pressure on firmness, cohesiveness, springiness, gumminess, and chewiness has been investigated in previous studies on the texture of beetroot (33), meat (30, 34) and chickpeas (16). However, due to the conflicting results from these studies, a conclusion based on the available data to support the clear effects of high pressure processing on these textural properties cannot be reached. Our result for firmness was in agreement with that of Koca et al. (31) for cheese, Yu et al. (35) for brown rice and Alsalman and Ramaswamy (16) for chickpeas, which also reported that increasing pressure during HPP results in reduction in sample firmness.
In this study, softest texture was chosen as desirable based on the results of past sensory studies on legumes such as bengal gram (Cicer arietinum), green gram (Phaseolus aureus Roxb), lentils (Lens esculenta) (36), and beans (Phaseolus vulgaris) (37). Among all the samples, 6K5 showed the lowest firmness, cohesiveness, gumminess and chewiness values, whereas the control had the highest values for these textural properties. Thus, based on instrumental analysis we can concur that 6K5 had the most desirable textural properties out of all the samples in this study.
General Nutrient Composition
The proximate and dietary fiber content of the control and HPP chickpea samples is given in Table 2. The moisture content of the HPP samples ranged from 61.01 to 64.13 g/100 g, and the protein content ranged from 17.8 to 19.02 g/100 g db. None of these measurements were significantly different to the control. On the other hand, the starch content of the HPP samples ranged from 46.13 to 54.97 g/100 g and was significantly (P < 0.05) affected by treatment pressure levels, with an increase in pressure, leading to a decrease in starch content. When compared to the control, 4K1, 4K5, 6K1, and 6K5 had significantly (P < 0.05) less starch content. The reason for reduction in starch content is leaching of starch due to rupture of cell walls by the high pressure applied as observed in common beans as well (38). Based on these results, HPP can be potentially used to reduce starch content of other high starch grains and pulses for designing lower calorie alternatives.
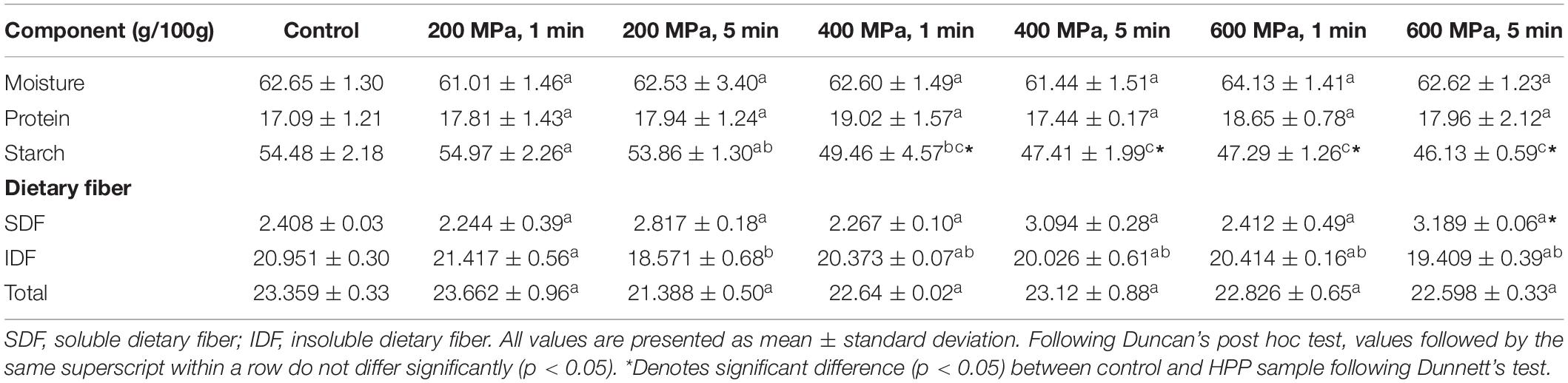
Table 2. Moisture, protein, starch and dietary fiber (soluble, insoluble, and total dietary fiber) content of control and high pressure processed cooked chickpeas.
The soluble dietary fiber (SDF), insoluble dietary fiber, and total dietary fiber content ranged from 2.244 to 3.189 g/100 g db, 18.571 to 21.417 g/100 g db, and 21.388 to 23.662 g/100 g db, respectively. Treatment time (T) had a significant (P < 0.05) effect on the SDF content of the HPP samples, with the increase in treatment time from 1 to 5 min, resulting in a marked increase in SDF levels. Whereas pressure x time (P x T) interaction had a significant (p < 0.05) negative effect on the IDF and total dietary fiber (TDF) levels. With an increase in treatment pressure (from 200 to 600 MPa) and time (1–5 min), the IDF and TDF levels decreased markedly. However, when compared to the control, all the samples had similar SDF, IDF and TDF levels, with only 6K5 having a significantly (P < 0.05) higher SDF content. The significant increase in SDF content of sample 6K5 and the marked reduction in IDF and TDF levels of all samples can be a result of cleavage of glycosidic linkages and/or breakage of weak bonds between polysaccharides (39). Consumption of SDF has shown to reduce postprandial glucose absorption in humans, acting as a glycemic lowering ingredient. Thus, HPP can be used in further research and development of legume based functional foods.
Mineral Content
The mineral content of the control and HPP sample (6K5) is reported in Table 3. Potassium was the most abundant mineral (5,371–6,131 mg/kg), followed by phosphorus (3,749–4,173 mg/kg), magnesium (1,267–1,340 mg/kg) and calcium (917–994 mg/kg). Other studies have reported that compared to their raw counterparts, soaked and/or cooked legumes have a reduced mineral content (40–42). The cooked samples from this study demonstrated higher iron, phosphorus, and magnesium levels when compared to uncooked chickpea samples from other region of the world possibly due to solid loss during cooking (43), however, the effect of high pressure processing on the mineral content of cooked kabuli chickpeas has previously not been reported.
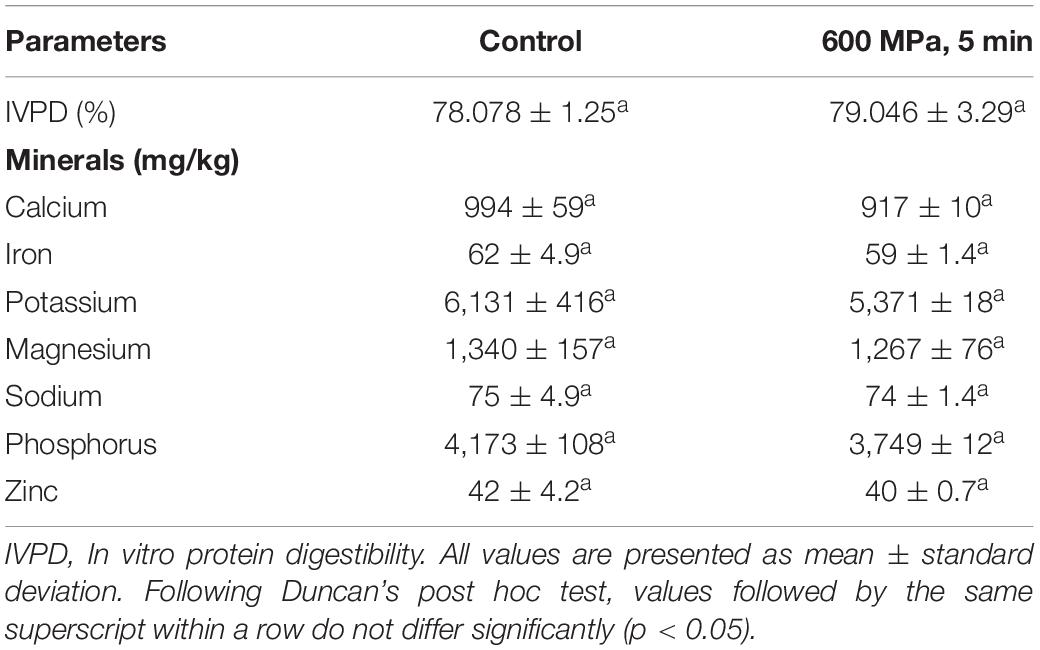
Table 3. In vitro Protein digestibility and mineral content of the control and high pressure processed cooked chickpeas.
High hydrostatic pressure processing is known to not affect smaller molecules such as volatile compounds, pigments, vitamins and compounds related to sensory, nutritional and health promoting effects (3, 44). In our study, no significant (P < 0.05) differences were observed in the mineral content of the control and the highest treatment level sample, meaning when consumers ingest HPP chickpeas, they receive the same total intake of minerals as with traditionally cooked chickpeas. Our results are also in agreement with studies on other foods treated with HPP such as Andrés et al. (45) who reported no effect of HPP on the mineral content of milk and soy smoothies containing fruits (orange, papaya, melon and carrot). As no difference between the control and highest treatment level sample was found, samples with lower HPP treatments were not analyzed for their mineral content.
Polyphenol Content
The polyphenol content of the control and HPP samples is reported in Table 4. It ranged from 31.704 to 52.321 mg gallic acid equivalent/100 g sample (d.b.), with the highest recorded for 2K1 and the lowest for 6K5. Main and significant (P < 0.05) effects of pressure and treatment time were observed, suggesting that with an increase in pressure and treatment time, total polyphenol content (TPC) in samples decreased. However, when compared to the control, only samples treated at 600 MPa were significantly (P < 0.05) lower in their TPC.

Table 4. Polyphenol content and antioxidant capacity of control and high pressure processed cooked chickpeas.
Similar results have been reported by Rodríguez-Roque et al. (46) and Barba et al. (47), who also found a slight decrease in the polyphenol content of orange juice following high pressure treatment above 400 MPa. Linsberger-Martin et al. (9) also reported a reduction in the total phenolic content in split peas and whole white beans after HPP. In contrast, Wang et al. (48), Sánchez-Moreno et al. (49), Patras et al. (50), and Patras et al. (51) reported an increase in the polyphenol content of different plant foods (cereals, tomato puree, tomato, carrot, strawberry, and blueberry, respectively) after high pressure processing.
The type of food, location of phenolic compounds in food, pre-processing treatment, as well as the duration and intensity of the HPP treatment affects the concentration of phenolic compounds in the extract (52). An increase in the total phenolic content of certain foods (in case certain fruits and vegetables) can be due to the disruption of cell wall structures, inactivation of enzymes related to loss of phenolic substances or improved extractability of the antioxidant components following high pressure treatment (47). However, changes in the physicochemical characteristics (46) and enhanced chemical and enzymatic oxidation of polyphenols (53) can occur due to processing, resulting in a lower availability.
Antioxidant Capacity
An effect of high pressure processing on the antioxidant capacity of cooked kabuli chickpeas was observed and the values are reported in Table 4. The DPPH, ABTS and ORAC values for HPP samples ranged from 29.722 to 58.480 mg trolox equivalent/100 g, 85.506–91.022 mg TE/100 g, and 1,253–1,993 μmol TE/100 g, respectively. For DPPH and ORAC antioxidant capacity, significant (P < 0.05) negative effects of pressure and time were observed. Thus, with an increase in pressure (200–600 MPa) and treatment time (1–5 min), the DPPH and ORAC values significantly decreased. However, the reduction in ORAC values was only observed at the highest pressure level (600 MPa). Significant (P < 0.05) main effects of pressure (P), time (T) and PxT interaction were observed for ABTS antioxidant capacity. However, following Duncan’s post hoc test, only sample 6K5 showed significantly (P < 0.05) lower ABTS value when compared to all other samples including control. When compared to the control, 4K1, 4K5 6K1, and 6K5 showed significantly (P < 0.05) lower DPPH antioxidant values, whereas only 6K5 expressed significantly (p < 0.05) lower ABTS and ORAC value than the control. Sample 6K5 had the overall highest decrease in the antioxidant capacities out of all samples analyzed.
The DPPH and ABTS assays are considered more accurate and reliable when compared to other methods such as FRAP (Ferric reducing antioxidant power) because of their rapidity, robustness and reliability in assessing antioxidant capacity of plant materials (54). On the other hand, ORAC assay uses biologically relevant free radicals, is standardized and integrates both degree and time of antioxidant reaction unlike other chemical assays (55). Previous studies have recommended to use at least 2 assays for the antioxidant capacity analysis of plant materials (54, 56), and thus we have chosen these three assays for this study to validate our results.
Negative effects of thermal treatments on the antioxidant capacity of legumes such as chickpeas, soybeans, kidney beans (57), common beans (58), and cowpeas (59) have been reported in previous studies. However, very limited information on the effects of high pressure processing on the antioxidant capacity of legumes or foods in general is available. Doblado et al. (15) observed a significant decrease in the ABTS antioxidant capacity of germinated cowpeas after HPP of up to 500 MPa (room temperature/15 min), and Butz et al. (14) reported an 11% decrease in the ABTS antioxidant capacity of carrots after HPP at 500 MPa for 5 min (4°C). In another study, a decrease in ABTS antioxidant capacity of orange juice was observed when the HPP pressure level was increased from 100 to 800 MPa (at 30–65°C) (60) due to ascorbic acid degradation. In contrast, the DPPH antioxidant capacity of tomato puree was unchanged by HPP at 400 MPa/25°C/15 min (49) and Briones-Labarca et al. (44) reported an increase in the DPPH antioxidant activity of uncooked algarrobo seeds, an underutilized legume after high pressure processing at 500 MPA for 10 min compared to untreated seeds and seeds treated at 500 MPa for 2, 4 and 8 min (20°C). The reduced antioxidant activity recorded in the present study may have occurred because of the combination of cooking and high pressure processing resulting in destruction of the bioactive components or formation of new compounds with pro-oxidant action.
The correlation between total polyphenol content (TPC) and the antioxidant activity revealed by three assays (DPPH, ABTS and ORAC) is also represented in Table 5. A very high and significant (P < 0.001) positive correlation was observed between TPC and DPPH values (r = 0.922), and slightly lower but significant (P < 0.001) positive correlations with ABTS (r = 0.669), and ORAC (r = 0.727) assays were also observed. These results indicate that changes in the antioxidant capacity due to high pressure processing were closely related to the polyphenol content of the samples, supporting previous reports (24, 61).
Scanning Electron Microscopy
Morphological characteristics of starch granules from raw, cooked and CHPP kabuli chickpeas was observed using scanning electron microscope (SEM). Raw chickpea starch (Figure 4A), which was used as an internal control exhibited smooth, oval shaped granules with no evidence of fissures or damage. Similar observations have been reported in previous studies on chickpea starch and starches from other legumes such as adzuki, black, and kidney bean (62, 63). When compared to starch granules from the raw samples, starch granules from the cooked and cooked+ HPP sample were a lot bigger (Figures 4A–H) in response to soaking and subsequent cooking. Scanning electron microscopy confirmed that high pressure processing altered the starch granule structure (Figures 4C–H). SEM pictures showed that, like the control (b), the majority of starch granule retain their granular shape. However, differences in their surface morphology was evident. HPP resulted in formation of fissures and caused surface damage to the starch granules of HPP samples. Similar results have been reported in previous studies on high pressure processing of barley (64) and potato (65) starch.
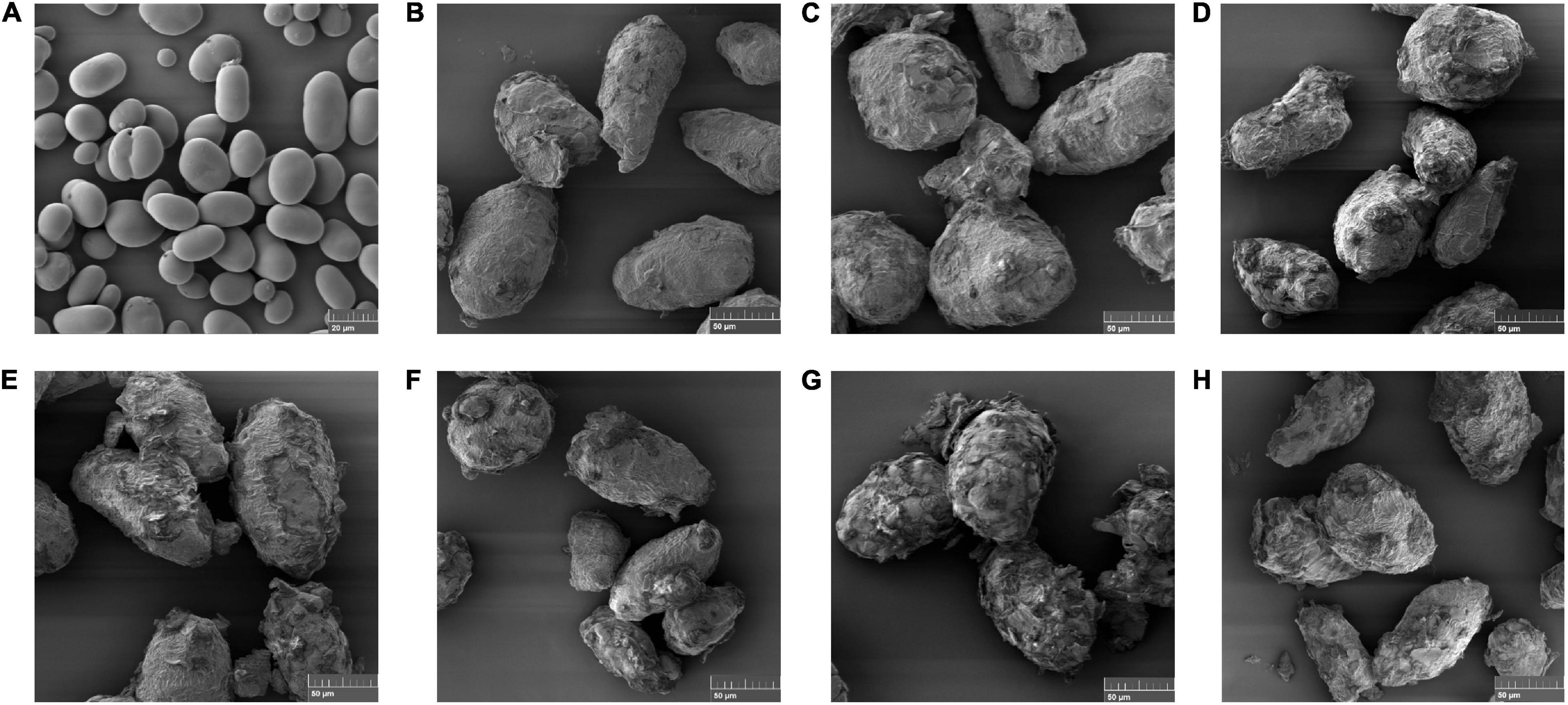
Figure 4. Scanning electron microscopy images (scale 20–50 μm) of isolated native starch (scale = 20 μm) (A), control (B), and high pressure processed cooked chickpeas (where, (C) = 200 MPa, 1 min; (D) = 200 MPa, 5 min; (E) = 400 MPa, 1 min; (F) = 400 MPa, 5 min; (G) = 600 MPa, 1 min; and (H) = s 600 MPa, 5 min).
During high pressure treatment, the available water is forced into the chickpea grains causing rapid hydration driven by the applied external pressure, resulting in rapid swelling even at ambient temperatures (16). Błaszczak et al. (65) reported significant deformations to Freeze-dried potato starch granules caused by high pressure processing (65), which can be observed samples from this study as well. Liu et al. (66) reported that high pressure contributed to a strong interaction between amylose and amylopectin chains leading to formation of fractures and fissures on the surface of starch granules. This may also indicate significant changes in the internal structure of the granule. Starch undergoes a structural collapse that causes an alteration of the granular shape due to the simultaneous diffusion of solvents and gelatinization of starch (67). Taken at the same magnification as the control, the pictures of starch granules (Figure 4G) clearly depicts that very high pressure of 600 MPa is responsible for greater destruction of the granule integrity leading to further damage. As differences in the morphology of the isolated starch granules was observed, an in vitro starch digestibility assay was performed to understand the effect of HPP on starch digestibility of all samples.
Attenuated Total Reflectance Fourier Transform Infrared Spectra Analysis
ATR-FTIR spectroscopy has previously been used to investigate the crystallinity, structure, and intermolecular interactions of starch granules (68). However, in previous studies using purified starch samples specific structural information relating to starch was analyzed (69, 70). In this study, control and HPP chickpeas have been analyzed with ATR-FTIR, and the spectra therefore contain contributions from proteins and lipids, in addition to starch. In this study, it is therefore not possible to draw absolute conclusions concerning starch structure, due to spectra overlap of starch absorbance bands with the absorbance bands of proteins and lipids.
Nonetheless, ATR-FTIR is still a useful method to investigate relative changes in the starch bonding environment and/or structure, as a consequence of high pressure treatments at different times. The major adsorption bands arising from starch can be observed in the region 1,200–1,000 cm–1 and it has been shown that bands at 1,047 and 1,022 cm–1, respectively, describe the crystalline and amorphous indices of starch (71). The ATR-FTIR results (Figure 5) reveal distinct absorbance bands attributed to starch, which are easily viewed as “negative peaks” in the second-derivative spectra (Figures 5A,B). Three specific bands of interest are those at 1,140, 1,040, and 1,020 cm–1 [assigned to ν(C–O) modes] (68). Interestingly, an increased spectra shift to lower wavenumbers was observed at the bands for HPP samples treated at 200, 400 and 600 MPa, indicating treatment-induced alteration to the starch structure and intermolecular bonding environment.
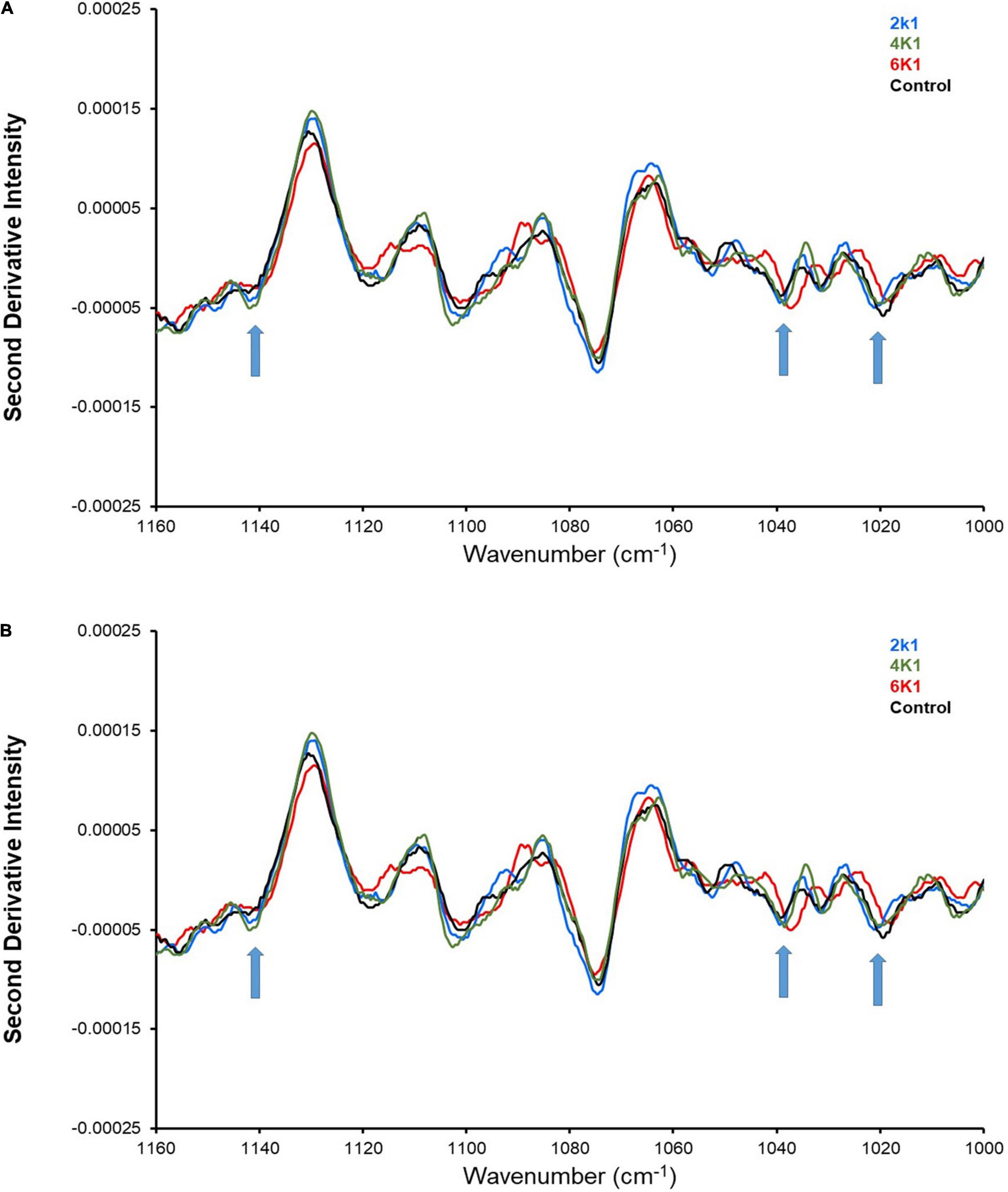
Figure 5. ATR-FTIR analysis of control and HPP kabuli chickpeas with upward arrows indicating shift in spectra at 1,140, 1,040, and 1,020 cm– 1 (where 2K1 = 200 MPa, 1 min; 2K5 = 200 MPa, 5 min; 4K1 = 400 MPa, 1 min; 4K5 = 400 MPa, 5 min; 6k1 = 600 MPa, 1 min; and 6K5 = 600 MPa, 5 min).
Also, the intensity of the bands increased with an increase in treatment pressure and time (Figure 5B), suggesting conversion of starch molecules to its ruptured form and disruption of the chemical bonds (72). High pressure processing has been known to decrease the relative crystallinity of starch granules, which in turn lowers starch digestibility (73, 74). Pressurization during high pressure processing partially gelatinizes starch granules, whereas depressurization promotes retrogradation which results in recrystallization of amylopectin and further reduces starch digestion (75).
In vitro Starch Digestibility
A number of factors such as source of starch, granule size, amylose-amylopectin ratio and the crystalline structure affect the enzymatic susceptibility of starches, with amylose content and the crystallinity being the most important factors (76). Starch gelatinization occurs when starch is heated in the presence of excess water, resulting in a change from crystalline to a more amorphous structure (76), thus becoming more digestible. However, starch entrapped within the cells of edible plant material (e.g., seeds) undergoes limited starch gelatinization (77), retaining its crystalline nature and resulting in a lower digestibility (78). Intracellular components such as proteins also impose restrictions on starch granule swelling, thus affecting starch digestibility (79).
As shown in Figure 6, with an increase in treatment pressure and time, the contents of rapidly digestible starch (RDS), slowly digestible starch (SDS), and resistant starch (RS) in the HPP samples also changed. Significant (P < 0.05) main effects of pressure (P) and time (T) on RDS, SDS and RS levels were observed. It was observed that HPP at higher pressures and longer times (2K5, 4K5, and 6K5) can significantly (P < 0.05) increase the SDS levels in cooked chickpeas when compared to lower pressures and shorter treatment durations (2K1, 4K1, and 6K1), and when the pressure level reached the maximum value (600 MPa, 5 min) of the machine, the SDS fraction was at the highest amount (60.92 g/100 g starch). However, only the SDS content of the 2K5, 6K1, and 6K5 samples and the RS content of the 2K5 and 6K5 samples were significantly (p < 0.05) different from the control following the starch fractions determination.
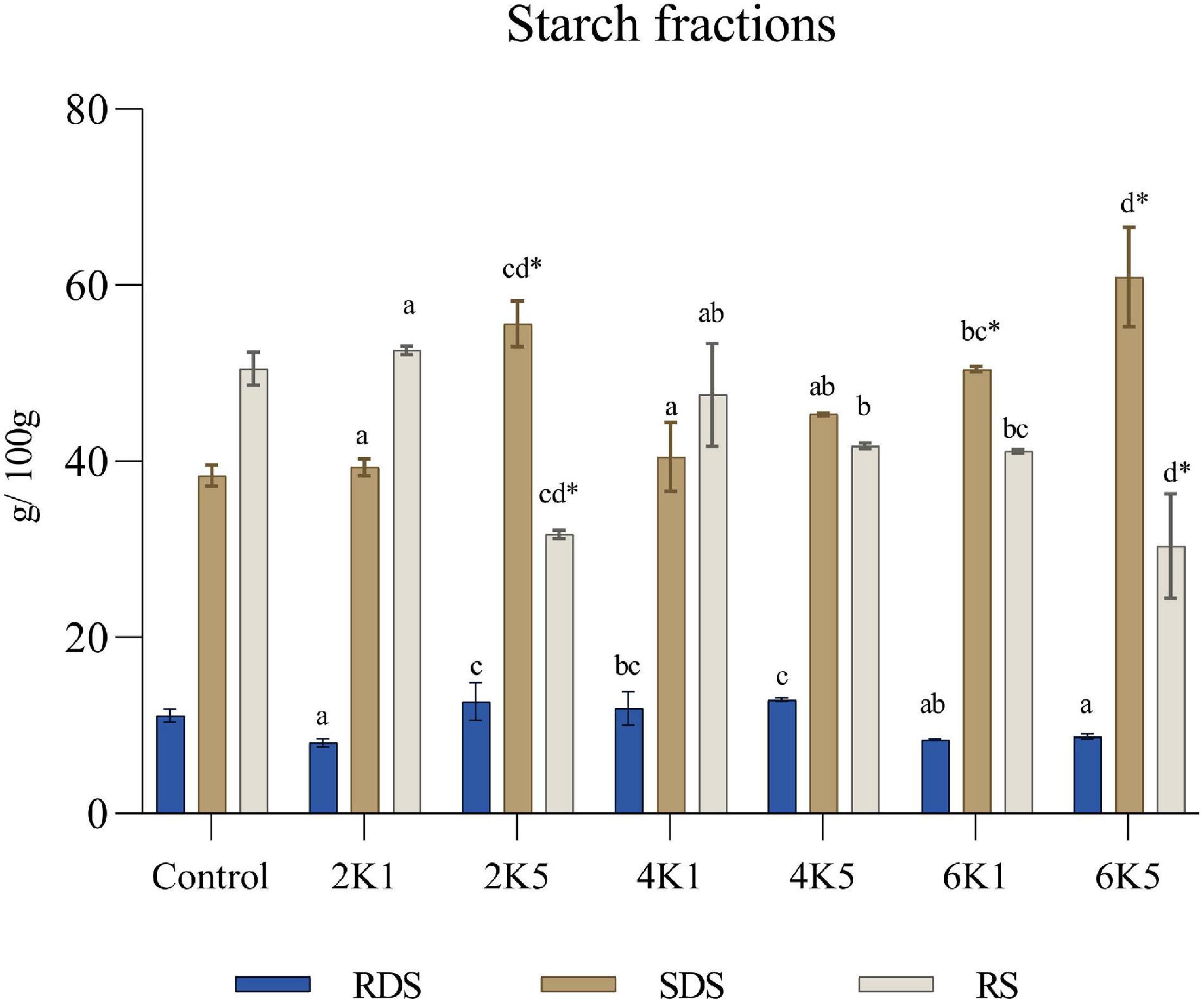
Figure 6. Rapidly digestible starch (RDS), slowly digestible starch (SDS) and resistant starch (RS) in the control and HPP chickpea samples. Bars bearing different letter for the same fraction are significantly (p < 0.05) different. Bars bearing * for the same fraction are significantly (p < 0.05) different than the control (where, 2K1 = 200 MPa, 1 min; 2K5 = 200 MPa, 5 min; 4K1 = 400 MPa, 1 min; 4K5 = 400 MPa, 5 min; 6k1 = 600 MPa, 1 min; and, 6K5 = 600 MPa, 5 min).
Previous research has shown that high pressure processing can completely damage uncooked rice starch granules as well (80), while also promoting a wider distribution of water molecules in the crystalline regions of the starch granules. This in turn disrupts the amylopectin chains (81) and forms imperfect crystallites, which are directly linked to high SDS levels (82). Another reason for the increase in SDS content might be the smaller volume of amylose-lipid complexes formed in starch granules as a result of HPP (83).
Starch digestibility of cooked, waxy wheat starch (84), buckwheat (66), and brown rice (85) has previously been modified by the use of high pressure treatment, when compared to their raw counterparts. It was also observed in these studies that HPP increased the amount of slowly digestible starch (SDS), decreasing the overall starch digestibility of these samples. Slowly digestible starch has been shown to provide nutritional benefits for humans (86), as it leads to a slow and prolonged postprandial release of glucose in the blood stream, thus providing a lower glycemic response (87). It has been suggested that HPP has potential in producing products for glycemic control (85), which can help in the prevention of diseases such as diabetes, cardiovascular diseases and colon cancer (88).
In vitro Protein Digestibility
The percentage in vitro protein digestibility (IVPD) values for the control and the highest HPP treatment (600 MPa, 5 min) are reported in Table 4. There was no significant (P < 0.05) effect of pressure, time or their interaction on the IVPD values for control and HPP sample. Cooking and thermal heat treatments (autoclaving) have been shown to improve the protein digestibility of raw legumes such as black grams, chickpeas, lentils, and red kidney beans due to an increased accessibility of the protein component for enzymatic attack (89), and/or inactivation of proteinacious anti-nutritional factors (90). High pressure cooking of rice has been shown to reduce its protein digestibility (91), whereas Laguna et al. (92) reported a small reduction (8%) in the protein digestibility of apple puree enriched with pea protein following HPP, with no effect of HPP on carrot puree enriched with pea protein. However, the effect of high pressure processing on the protein digestibility of chickpeas is not widely known.
Deol and Bains (93) reported an increase in protein digestibility of cowpeas after steam pressure cooking primarily due to destruction of antinutritional factors. Linsberger-Martin et al. (9) reported a 3.5% increase in the protein digestibility of split peas and a 6% increase in the protein digestibility of whole white beans after HPP at 600 MPa for 60 min. However, a small but significant reduction in protein digestibility was also reported in both split peas and whole white beans after HPP at 100 MPA for 60 min and 350 MPA for 45 min (9). A possible explanation for this reduction in protein digestibility can be due to the formation of intra/intermolecular disulfide bridges or a protein network (94). While the mechanism of pressure induced protein unfolding is not completely understood, the underlying mechanism of pressure induced protein denaturation involves water penetration into cavities within the molecule resulting in varying populations of molecular conformations (95), resulting in increased protein digestibility. However, as in our study all the samples were highly saturated with water (12 h soaking + 30 min boiling) before the HP treatment, no significant changes in the protein digestibility of control and HPP sample was observed. As there was no significant difference between the control and the highest treatment level (6K5), the remaining samples were not subjected to the analysis.
Conclusion
This study demonstrated that the textural and starch digestibility properties of cooked kabuli chickpea is significantly improved by the application of high pressure processing. Significant effects of treatment pressure and time on the starch, polyphenol and antioxidant capacity were also revealed. These combined findings highlight the potential of utilizing non-thermal processing technologies to increase consumer acceptance of plant- based protein sources with desirable textural properties. Our findings suggest that high pressure processing has the potential to enable the design of products with a low glycemic index, compared to traditional products, while retaining dietary fiber percentages, minerals and digestible proteins and thus providing beneficial health effects to consumers. However, the shelf stability of cooked + high pressure processed ready-to eat chickpea product is unknown and thus requires further research.
Data Availability Statement
The original contributions presented in the study are included in the article/supplementary material, further inquiries can be directed to the corresponding author.
Author Contributions
PC, SJ, SB, and RC finalized the experimental design. PC carried out all the experiments and prepared the manuscript. SB, SJ, RC, and RB revised the prepared manuscript, contributed helpful discussion, and scientific advice during preparation of manuscript. All authors contributed to the manuscript and approved the submitted version.
Funding
This research was conducted as part of a Doctorate program through the Curtin International Postgraduate Research Scholarship provided by Curtin University, Bentley, WA.
Conflict of Interest
SJ was employed at Ingredients by Design Pty Ltd.
The remaining authors declare that the research was conducted in the absence of any commercial or financial relationships that could be construed as a potential conflict of interest.
Publisher’s Note
All claims expressed in this article are solely those of the authors and do not necessarily represent those of their affiliated organizations, or those of the publisher, the editors and the reviewers. Any product that may be evaluated in this article, or claim that may be made by its manufacturer, is not guaranteed or endorsed by the publisher.
Acknowledgments
We wish to acknowledge Preshafoods Pty Ltd., Victoria (Australia) for their assistance with high pressure processing, Mark J. Hackett, School of Molecular and Life Sciences, Curtin University for assistance with the FTIR analysis and interpretation, and John de Laeter Centre, Curtin University, Bentley, WA for the microscopy equipment.
References
1. Krebbers B, Matser AM, Koets M, Van den Berg RW. Quality and storage-stability of high-pressure preserved green beans. J Food Eng. (2002) 54:27–33. doi: 10.1016/s0260-8774(01)00182-0
2. Huang H-W, Wu S-J, Lu J-K, Shyu Y-T, Wang C-Y. Current status and future trends of high-pressure processing in food industry. Food Control. (2017) 72:1–8. doi: 10.1016/j.foodcont.2016.07.019
3. Oey I, Van der Plancken I, Van Loey A, Hendrickx M. Does high pressure processing influence nutritional aspects of plant based food systems? Trends Food Sci Technol. (2008) 19:300–8. doi: 10.1016/j.tifs.2007.09.002
4. Knorr D. High Pressure Processing for Preservation, Modification and Transformation of Foods. High Press Res. (2002) 22:595–9. doi: 10.1080/08957950212411
5. Markets and Markets. Plant-based Protein Market by Source (Soy, Wheat, and Pea), Type (Isolates, Concentrates, and Textured), Form (Dry and Liquid), Application (Food and Feed), and Region (North America, Europe, APAC, South America, RoW) - Global Forecast to 2025. (2020). Available online at: https://www.marketsandmarkets.com/Market-Reports/plant-based-protein-market-14715651.html (accessed December 10, 2021).
6. Maphosa Y, Jideani VA. The role of legumes in human nutrition. In: MC Hueda editor. Functional Food: Improve Health through Adequate Food. Crostia: InTech (2017).
7. Tziva M, Negro SO, Kalfagianni A, Hekkert MP. Understanding the protein transition: the rise of plant-based meat substitutes. Environ Innov Soc Transit. (2020) 35:217–31. doi: 10.1016/j.eist.2019.09.004
8. Bar-El Dadon S, Abbo S, Reifen R. Leveraging traditional crops for better nutrition and health - The case of chickpea. Trends Food Sci Technol. (2017) 64:39–47. doi: 10.1016/j.tifs.2017.04.002
9. Linsberger-Martin G, Weiglhofer K, Thi Phuong TP, Berghofer E. High hydrostatic pressure influences antinutritional factors and in vitro protein digestibility of split peas and whole white beans. LWT Food Sci Technol. (2013) 51:331–6. doi: 10.1016/j.lwt.2012.11.008
10. Thakur BR, Nelson PE. High−pressure processing and preservation of food. Food Rev Int. (1998) 14:427–47.
11. Ahmed J, Thomas L, Taher A, Joseph A. Impact of high pressure treatment on functional, rheological, pasting, and structural properties of lentil starch dispersions. Carbohydr Polym. (2016) 152:639–47. doi: 10.1016/j.carbpol.2016.07.008
12. Li W, Zhang F, Liu P, Bai Y, Gao L, Shen Q. Effect of high hydrostatic pressure on physicochemical, thermal and morphological properties of mung bean (Vigna radiata L.) starch. J Food Eng. (2011) 103:388–93. doi: 10.1016/j.jfoodeng.2010.11.008
13. Leite TS, de Jesus ALT, Schmiele M, Tribst AAL, Cristianini M. High pressure processing (HPP) of pea starch: effect on the gelatinization properties. LWT Food Sci Technol. (2017) 76:361–9. doi: 10.3390/molecules25112516
14. Butz P, Edenharder R, Fernandez A, Fister H, Merkel C, Tauscher B. Changes in functional properties of vegetables induced by high pressure treatment. Food Res Int. (2002) 35:295–300. doi: 10.1016/s0963-9969(01)00199-5
15. Doblado R, Frías J, Vidal-Valverde C. Changes in vitamin C content and antioxidant capacity of raw and germinated cowpea (Vigna sinensis var. carilla) seeds induced by high pressure treatment. Food Chem. (2007) 101:918–23. doi: 10.1016/j.foodchem.2006.02.043
16. Alsalman FB, Ramaswamy H. Reduction in soaking time and anti-nutritional factors by high pressure processing of chickpeas. J Food Sci Technol. (2020) 57:2572–85. doi: 10.1007/s13197-020-04294-9
17. Siddique KHM, Regan KL. Registration of ‘Kimberley Large’ kabuli chickpea. Crop Sci. (2005) 45:1659–60. doi: 10.2135/cropsci2004.0405
18. Wang N, Daun JK, Malcolmson LJ. Relationship between physicochemical and cooking properties, and effects of cooking on antinutrients, of yellow field peas (Pisum sativum). J Sci Food Agric. (2003) 83:1228–37. doi: 10.1002/jsfa.1531
19. AOAC International. Official Method 925.10. Official Methods of Analysis. Gaithersburg, MD: AOAC International (2008).
20. AOAC International. AOAC Official Method 991.43. Official Methods of Analysis. Gaithersburg, MD: AOAC International (2005).
21. AOAC International. AOAC Official Method 996.11. Official Methods of Analysis. Gaithersburg, MD: AOAC International (2005).
22. Wu G, Johnson SK, Bornman JF, Bennett SJ, Singh V, Simic A, et al. Effects of genotype and growth temperature on the contents of tannin, phytate and in vitro iron availability of sorghum grains. PLoS One. (2016) 11:e0148712. doi: 10.1371/journal.pone.0148712
23. Segev A, Badani H, Kapulnik Y, Shomer I, Oren-Shamir M, Galili S. Determination of polyphenols, flavonoids, and antioxidant capacity in colored chickpea (Cicer arietinum L.). J Food Sci. (2010) 75:S115–9. doi: 10.1111/j.1750-3841.2009.01477.x
24. Wu G, Johnson SK, Bornman JF, Bennett SJ, Fang Z. Changes in whole grain polyphenols and antioxidant activity of six sorghum genotypes under different irrigation treatments. Food Chem. (2017) 214:199–207. doi: 10.1016/j.foodchem.2016.07.089
25. Thaipong K, Boonprakob U, Crosby K, Cisneros-Zevallos L, Hawkins Byrne D. Comparison of ABTS, DPPH, FRAP, and ORAC assays for estimating antioxidant activity from guava fruit extracts. J Food Compos Anal. (2006) 19:669–75. doi: 10.1016/j.jfca.2006.01.003
26. Huang D, Ou B, Hampsch-Woodill M, Flanagan JA, Prior RL. High-throughput assay of oxygen radical absorbance capacity (ORAC) using a multichannel liquid handling system coupled with a microplate fluorescence reader in 96-well format. J Agric Food Chem. (2002) 50:4437–44. doi: 10.1021/jf0201529
27. Sun Y, Wu Z, Hu B, Wang W, Ye H, Sun Y, et al. A new method for determining the relative crystallinity of chickpea starch by Fourier-transform infrared spectroscopy. Carbohydr Polym. (2014) 108:153–8. doi: 10.1016/j.carbpol.2014.02.093
28. Sopade PA, Gidley MJA. Rapid in-vitro digestibility assay based on glucometry for investigating kinetics of starch digestion. Starke. (2009) 61:245–55. doi: 10.1002/star.200800102
29. Villarino CBJ, Jayasena V, Coorey R, Chakrabarti-Bell S, Foley R, Fanning K, et al. The effects of lupin (Lupinus angustifolius) addition to wheat bread on its nutritional, phytochemical and bioactive composition and protein quality. Food Res Int. (2015) 76:58–65. doi: 10.1016/j.foodres.2014.11.046
30. Oliveira FAD, Neto OC, Santos LMRd, Ferreira EHR, Rosenthal A. Effect of high pressure on fish meat quality – A review. Trends Food Sci Technol. (2017) 66:1–19. doi: 10.1016/j.tifs.2017.04.014
31. Koca N, Balasubramaniam VM, Harper WJ. High-pressure effects on the microstructure, texture, and color of white-brined cheese. J Food Sci. (2011) 76:E399–404. doi: 10.1111/j.1750-3841.2011.02201.x
32. Meullenet J-F, Lyon BG, Carpenter JA, Lyon CE. Relationship between sensory and instrumental texture profile attributes. J Sens Stud. (1998) 13:77–93. doi: 10.1111/j.1745-459x.1998.tb00076.x
33. Paciulli M, Medina-Meza IG, Chiavaro E, Barbosa-Cánovas GV. Impact of thermal and high pressure processing on quality parameters of beetroot (Beta vulgaris L.). LWT Food Sci Technol. (2016) 68:98–104. doi: 10.1016/j.lwt.2015.12.029
34. Sun XD, Holley RA. High hydrostatic pressure effects on the texture of meat and meat products. J Food Sci. (2010) 75:R17–23. doi: 10.1111/j.1750-3841.2009.01449.x
35. Yu Y, Pan F, Ramaswamy HS, Zhu S, Yu L, Zhang Q. Effect of soaking and single/two cycle high pressure treatment on water absorption, color, morphology and cooked texture of brown rice. J Food Sci Technol. (2017) 54:1655–64. doi: 10.1007/s13197-017-2598-4
36. Khatoon N, Prakash J. Nutritive value and sensory profile of microwave- and pressure-cooked decorticated legumes (dhals). J Food Process Preserv. (2006) 30:299–313. doi: 10.1111/j.1745-4549.2006.00066.x
37. Ghasemlou M, Gharibzahedi SMT, Emam-Djomeh Z. Relating consumer preferences to textural attributes of cooked beans: development of an industrial protocol and microstructural observations. LWT Food Sci Technol. (2013) 50:88–98. doi: 10.1016/j.lwt.2012.06.018
38. Belmiro RH, Tribst AAL, Cristianini M. Effects of high pressure processing on common beans (Phaseolus Vulgaris L.): cotyledon structure, starch characteristics, and phytates and tannins contents. Stärke. (2020) 72:1900212. doi: 10.1002/star.201900212
39. Wennberg M, Nyman M. On the possibility of using high pressure treatment to modify physico-chemical properties of dietary fibre in white cabbage (Brassica oleracea var. Capitata). Innov Food Sci Emerg Technol. (2004) 5:171–7. doi: 10.1016/j.ifset.2004.02.002
40. Bains K, Uppal V, Kaur H. Optimization of germination time and heat treatments for enhanced availability of minerals from leguminous sprouts. J Food Sci Technol. (2014) 51:1016–20. doi: 10.1007/s13197-011-0582-y
41. Barampama Z, Simard RE. Effects of soaking, cooking and fermentation on composition, in-vitro starch digestibility and nutritive value of common beans. Plant Foods Hum Nutr. (1995) 48:349–65. doi: 10.1007/BF01088494
42. Wang N, Hatcher DW, Tyler RT, Toews R, Gawalko EJ. Effect of cooking on the composition of beans (Phaseolus vulgaris L.) and chickpeas (Cicer arietinum L.). Food Res Int. (2010) 43:589–94. doi: 10.1016/j.foodres.2009.07.012
43. Iqbal A, Khalil IA, Ateeq N, Sayyar Khan M. Nutritional quality of important food legumes. Food Chem. (2006) 97:331–5. doi: 10.1016/j.foodchem.2005.05.011
44. Briones-Labarca V, Venegas-Cubillos G, Ortiz-Portilla S, Chacana-Ojeda M, Maureira H. Effects of high hydrostatic pressure (HHP) on bioaccessibility, as well as antioxidant activity, mineral and starch contents in Granny Smith apple. Food Chem. (2011) 128:520–9. doi: 10.1016/j.foodchem.2011.03.074
45. Andrés V, Villanueva M-J, Tenorio M-D. Influence of high pressure processing on microbial shelf life, sensory profile, soluble sugars, organic acids, and mineral content of milk- and soy-smoothies. LWT Food Sci Technol. (2016) 65:98–105. doi: 10.1016/j.lwt.2015.07.066
46. Rodríguez-Roque MJ, de Ancos B, Sánchez-Moreno C, Cano MP, Elez-Martínez P, Martín-Belloso O. Impact of food matrix and processing on the in vitro bioaccessibility of vitamin C, phenolic compounds, and hydrophilic antioxidant activity from fruit juice-based beverages. J Funct Foods. (2015) 14:33–43. doi: 10.1016/j.jff.2015.01.020
47. Barba FJ, Cortés C, Esteve MJ, Frígola A. Study of antioxidant capacity and quality parameters in an orange juice–milk beverage after high-pressure processing treatment. Food Bioproc Technol. (2012) 5:2222–32. doi: 10.1007/s11947-011-0570-2
48. Wang T, He F, Chen G. Improving bioaccessibility and bioavailability of phenolic compounds in cereal grains through processing technologies: a concise review. J Funct Foods. (2014) 7:101–11. doi: 10.1016/j.jff.2014.01.033
49. Sánchez-Moreno C, Plaza L, De Ancos B, Cano MP. Impact of high-pressure and traditional thermal processing of tomato purée on carotenoids, vitamin C and antioxidant activity. J Sci Food Agric. (2006) 86:171–9. doi: 10.1002/jsfa.2321
50. Patras A, Brunton NP, Da Pieve S, Butler F. Impact of high pressure processing on total antioxidant activity, phenolic, ascorbic acid, anthocyanin content and colour of strawberry and blackberry purées. Innov Food Sci Emerg Technol. (2009) 10:308–13. doi: 10.1016/j.ifset.2008.12.004
51. Patras A, Brunton N, Da Pieve S, Butler F, Downey G. Effect of thermal and high pressure processing on antioxidant activity and instrumental colour of tomato and carrot purées. Innov Food Sci Emerg Technol. (2009) 10:16–22. doi: 10.1016/j.ifset.2008.09.008
52. Chandrasekara A, Naczk M, Shahidi F. Effect of processing on the antioxidant activity of millet grains. Food Chem. (2012) 133:1–9. doi: 10.1016/j.foodchem.2011.09.043
53. Clariana M, Valverde J, Wijngaard H, Mullen AM, Marcos B. High pressure processing of swede (Brassica napus): impact on quality properties. Innov Food Sci Emerg Technol. (2011) 12:85–92. doi: 10.1016/j.ifset.2011.01.011
54. Piluzza G, Bullitta S. Correlations between phenolic content and antioxidant properties in twenty-four plant species of traditional ethnoveterinary use in the Mediterranean area. Pharm Biol. (2011) 49:240–7. doi: 10.3109/13880209.2010.501083
55. Zulueta A, Esteve MJ, Frígola A. ORAC and TEAC assays comparison to measure the antioxidant capacity of food products. Food Chem. (2009) 114:310–6. doi: 10.1016/j.foodchem.2008.09.033
56. Schlesier K, Harwat M, Böhm V, Bitsch R. Assessment of antioxidant activity by using different in vitro methods. Free Radic Res. (2002) 36:177–87. doi: 10.1080/10715760290006411
57. Gujral HS, Sharma P, Gupta N, Wani AA. Antioxidant properties of legumes and their morphological fractions as affected by cooking. Food Sci Biotechnol. (2013) 22:187–94. doi: 10.1007/s10068-013-0026-8
58. Rocha-Guzmán NE, González-Laredo RF, Ibarra-Pérez FJ, Nava-Berúmen CA, Gallegos-Infante J-A. Effect of pressure cooking on the antioxidant activity of extracts from three common bean (Phaseolus vulgaris L.) cultivars. Food Chem. (2007) 100:31–5. doi: 10.1016/j.foodchem.2005.09.005
59. Barros NVDA, Rocha MDM, Glória MBA, Araújo MADM, Moreira-Araújo RSDR. Effect of cooking on the bioactive compounds and antioxidant activity in grains cowpea cultivars. Rev Ciênc Agron. (2017) 48:824–31. doi: 10.5935/1806-6690.20170097
60. Indrawati O, Van Loey A, Hendrickx M. Pressure and temperature stability of water-soluble antioxidants in orange and carrot juice: a kinetic study. Eur Food Res Technol. (2004) 219:161–6.
61. Marathe SA, Rajalakshmi V, Jamdar SN, Sharma A. Comparative study on antioxidant activity of different varieties of commonly consumed legumes in India. Food Chem Toxicol. (2011) 49:2005–12. doi: 10.1016/j.fct.2011.04.039
62. Zhang Z, Tian X, Wang P, Jiang H, Li W. Compositional, morphological, and physicochemical properties of starches from red adzuki bean, chickpea, faba bean, and baiyue bean grown in China. Food Sci Nutr. (2019) 7:2485–94. doi: 10.1002/fsn3.865
63. Wani IA, Sogi DS, Gill BS. Physico-chemical properties of acetylated starches from Indian black gram (Phaseolus mungo L.) cultivars. J Food Sci Technol. (2015) 52:4078–89. doi: 10.1007/s13197-014-1480-x
64. Stolt M, Oinonen S, Autio K. Effect of high pressure on the physical properties of barley starch. Innov Food Sci Emerg Technol. (2000) 1:167–75. doi: 10.1016/s1466-8564(00)00017-5
65. Błaszczak W, Valverde S, Fornal J. Effect of high pressure on the structure of potato starch. Carbohydr Polym. (2005) 59:377–83. doi: 10.1016/j.carbpol.2004.10.008
66. Liu H, Wang L, Cao R, Fan H, Wang M. In vitro digestibility and changes in physicochemical and structural properties of common buckwheat starch affected by high hydrostatic pressure. Carbohydr Polym. (2016) 144:1–8. doi: 10.1016/j.carbpol.2016.02.028
67. Pineda-Gómez P, Rosales-Rivera A, Rodríguez-García ME. Modeling calcium and water intake in threshed corn grain during thermo-alkaline treatment. J Food Eng. (2012) 113:434–41. doi: 10.1016/j.jfoodeng.2012.06.026
68. Warren FJ, Gidley MJ, Flanagan BM. Infrared spectroscopy as a tool to characterise starch ordered structure—a joint FTIR–ATR, NMR, XRD and DSC study. Carbohydr Polym. (2016) 139:35–42. doi: 10.1016/j.carbpol.2015.11.066
69. Xiong J, Li Q, Shi Z, Ye J. Interactions between wheat starch and cellulose derivatives in short-term retrogradation: rheology and FTIR study. Food Res Int. (2017) 100:858–63. doi: 10.1016/j.foodres.2017.07.061
70. Chavez-Murillo CE, Orona-Padilla JL, de la Rosa Millan J. Physicochemical, functional properties and ATR-FTIR digestion analysis of thermally treated starches isolated from black and bayo beans. Starke. (2019) 71:1800250. doi: 10.1002/star.201800250
71. Kaptso KG, Njintang YN, Nguemtchouin MMG, Scher J, Hounhouigan J, Mbofung CM. Physicochemical and micro-structural properties of flours, starch and proteins from two varieties of legumes: bambara groundnut (Vigna subterranea). J Food Sci Technol. (2015) 52:4915–24. doi: 10.1007/s13197-014-1580-7
72. Mir SA, Bosco SJD, Shah MA, Mir MM. Effect of puffing on physical and antioxidant properties of brown rice. Food Chem. (2016) 191:139–46. doi: 10.1016/j.foodchem.2014.11.025
73. Katopo H, Song Y, Jane J-l. Effect and mechanism of ultrahigh hydrostatic pressure on the structure and properties of starches. Carbohydr Polym. (2002) 47:233–44. doi: 10.1016/s0144-8617(01)00168-0
74. Colussi R, Kaur L, Zavareze EDR, Dias ARG, Stewart RB, Singh J. High pressure processing and retrogradation of potato starch: influence on functional properties and gastro-small intestinal digestion in vitro. Food Hydrocoll. (2018) 75:131–7. doi: 10.1016/j.foodhyd.2017.09.004
75. Fredriksson H, Björck I, Andersson R, Liljeberg H, Silverio J, Eliasson AC, et al. Studies on α-amylase degradation of retrograded starch gels from waxy maize and high-amylopectin potato. Carbohydr Polym. (2000) 43:81–7. doi: 10.1016/s0144-8617(99)00205-2
76. Zavareze EDR, Dias ARG. Impact of heat-moisture treatment and annealing in starches: a review. Carbohydr Polym. (2011) 83:317–28. doi: 10.1080/10408398.2019.1664978
77. Brummer Y, Mina K, Susan MT. Structural and functional characteristics of dietary fibre in beans, lentils, peas and chickpeas. Food Res Int. (2015) 67:117–25. doi: 10.1016/j.foodres.2014.11.009
78. Baldwin AJ, Egan DL, Warren FJ, Barker PD, Dobson CM, Butterworth PJ, et al. Investigating the mechanisms of amylolysis of starch granules by solution-state NMR. Biomacromolecules. (2015) 16:1614–21. doi: 10.1021/acs.biomac.5b00190
79. Shomer I. Swelling behaviour of cell wall and starch in potato (Solanum tuberosum L.) tuber cells–I. Starch leakage and structure of single cells. Carbohydr Polym. (1995) 26:47–54. doi: 10.1016/0144-8617(95)98834-4
80. Hu X, Xu X, Jin Z, Tian Y, Bai Y, Xie Z. Retrogradation properties of rice starch gelatinized by heat and high hydrostatic pressure (HHP). J Food Eng. (2011) 106:262–6. doi: 10.1016/j.foodchem.2013.11.162
81. Li W, Bai Y, Mousaa SAS, Zhang Q, Shen Q. Effect of high hydrostatic pressure on physicochemical and structural properties of rice starch. Food Bioproc Technol. (2012) 5:2233–41. doi: 10.1016/j.ijbiomac.2018.08.121
82. Tian Y, Li D, Zhao J, Xu X, Jin Z. Effect of high hydrostatic pressure (HHP) on slowly digestible properties of rice starches. Food Chem. (2014) 152:225–9.
83. Lullien-Pellerin V, Balny C. High-pressure as a tool to study some proteins’ properties: conformational modification, activity and oligomeric dissociation. Innov Food Sci Emerg Technol. (2002) 3:209–21. doi: 10.1016/s1466-8564(02)00045-0
84. Hu X-P, Zhang B, Jin Z-Y, Xu X-M, Chen H-Q. Effect of high hydrostatic pressure and retrogradation treatments on structural and physicochemical properties of waxy wheat starch. Food Chem. (2017) 232:560–5. doi: 10.1016/j.foodchem.2017.04.040
85. Xia Q, Wang L, Xu C, Mei J, Li Y. Effects of germination and high hydrostatic pressure processing on mineral elements, amino acids and antioxidants in vitro bioaccessibility, as well as starch digestibility in brown rice (Oryza sativa L.). Food Chem. (2017) 214:533–42. doi: 10.1016/j.foodchem.2016.07.114
86. Sang Y, Seib PA. Resistant starches from amylose mutants of corn by simultaneous heat-moisture treatment and phosphorylation. Carbohydr Polym. (2006) 63:167–75. doi: 10.1016/j.carbpol.2005.07.022
87. Zhang G, Ao Z, Hamaker BR. Slow digestion property of native cereal starches. Biomacromolecules. (2006) 7:3252–8. doi: 10.1021/bm060342i
88. Jenkins DJA, Kendall CWC, Augustin LSA, Franceschi S, Hamidi M, Marchie A, et al. Glycemic index: overview of implications in health and disease. Am J Clin Nutr. (2002) 76:266s–73s. doi: 10.1093/ajcn/76/1.266S
89. Rehman Z-U, Shah WH. Thermal heat processing effects on antinutrients, protein and starch digestibility of food legumes. Food Chem. (2005) 91:327–31. doi: 10.1016/j.foodchem.2004.06.019
90. van der Poel AFB, Gravendeel S, Boer H. Effect of different processing methods on tannin content and in vitro protein digestibility of faba bean (Vicia faba L.). Anim Feed Sci Technol. (1991) 33:49–58. doi: 10.1016/0377-8401(91)90045-t
91. Liu K, Zheng J, Chen F. Effect of domestic cooking on rice protein digestibility. Food Sci Nutr. (2019) 7:608–16. doi: 10.1002/fsn3.884
92. Laguna L, Picouet P, Guàrdia MD, Renard CMGC, Sarkar A. In vitro gastrointestinal digestion of pea protein isolate as a function of pH, food matrices, autoclaving, high-pressure and re-heat treatments. LWT. (2017) 84:511–9. doi: 10.1016/j.lwt.2017.06.021
93. Deol JK, Bains K. Effect of household cooking methods on nutritional and anti nutritional factors in green cowpea (Vigna unguiculata) pods. J Food Sci Technol. (2010) 47:579–81. doi: 10.1007/s13197-010-0112-3
94. Angioloni A, Collar C. Effects of pressure treatment of hydrated oat, finger millet and sorghum flours on the quality and nutritional properties of composite wheat breads. J Cereal Sci. (2012) 56:713–9. doi: 10.1016/j.jcs.2012.08.001
Keywords: chickpeas, high pressure processing, texture, proximate composition, polyphenols, antioxidant activity, digestibility
Citation: Chatur P, Johnson S, Coorey R, Bhattarai RR and Bennett SJ (2022) The Effect of High Pressure Processing on Textural, Bioactive and Digestibility Properties of Cooked Kimberley Large Kabuli Chickpeas. Front. Nutr. 9:847877. doi: 10.3389/fnut.2022.847877
Received: 03 January 2022; Accepted: 14 March 2022;
Published: 07 April 2022.
Edited by:
Qun Shen, China Agricultural University, ChinaReviewed by:
Ashish Rawson, Indian Institute of Food Processing Technology, IndiaKingsley George Masamba, Lilongwe University of Agriculture and Natural Resources, Malawi
Copyright © 2022 Chatur, Johnson, Coorey, Bhattarai and Bennett. This is an open-access article distributed under the terms of the Creative Commons Attribution License (CC BY). The use, distribution or reproduction in other forums is permitted, provided the original author(s) and the copyright owner(s) are credited and that the original publication in this journal is cited, in accordance with accepted academic practice. No use, distribution or reproduction is permitted which does not comply with these terms.
*Correspondence: Sarita Jane Bennett, c2FyaXRhLmJlbm5ldHRAY3VydGluLmVkdS5hdQ==